- 1Department of Nanoscience and Nanoengineering, Faculty of Engineering and Natural Sciences, Istanbul Medeniyet University, Istanbul, Turkei
- 2Department of Engineering Physics, Faculty of Engineering and Natural Sciences, Istanbul Medeniyet University, Istanbul, Turkei
Structural lubricity, characterized by nearly frictionless behavior at solid incommensurate interfaces with weak interactions, holds significant technological importance. However, various factors can lead to the breakdown of structural lubricity, such as spontaneous reorientation to a commensurate state, applied load, edge effects, deformations, and wear. To overcome these challenges, clusters can be employed at interfaces. With their high Young’s modulus and stiffness, clusters can withstand high loads and tolerate elastic deformations. Therefore, Pt cluster, which inherently possess incommensurate contact with graphite surface, are expected to exhibit structural superlubric behavior, even under high loads, as long as they can sustain incommensurate contact. Our molecular dynamics (MD) simulations, however, have revealed that a Pt cluster on graphite can undergo metastable transitions from the incommensurate state to a commensurate state, resulting in subsequent stick-slip behavior. In the absence of any external load, the Pt cluster has demonstrated the ability to maintain incommensurate contact with almost zero friction force, primarily attributed to its weak interaction with graphite. However, the presence of an applied load force leads to the loss of the initial incommensurate contact between the Pt cluster and graphite, resulting in the emergence of high friction forces and the breakdown of structural lubricity with a similar stick-slip behavior to that observed in the comparative simulations conducted for the commensurate state. It becomes evident that the maintenance of incommensurate contact is crucial for achieving superlubric behavior in Pt cluster-graphite systems, while the presence of an applied load force can disrupt this behavior and lead to higher friction forces.
1 Introduction
Structural incommensurability, arising from surface lattice mismatch or natural misalignment, as shown in Figure 1, can prevent interlocking and collective stick-slip motion of interface asperities, with a consequent vanishingly small static frictional force (Mandelli et al., 2017; He et al., 2022). Hirano et al. introduced the foundational framework of structural superlubricity. Since then, numerous experiments at both the nano and meso-scales have consistently confirmed its validity (Hirano and Shinjo, 1990). In principle, both crystalline and amorphous particles could be suitable candidates for structural superlubricity. Yet, even though structural superlubricity has been observed in different material contacts (Lee and Spencer, 2008; Sweeney et al., 2012; Bylinskii et al., 2016; Rees et al., 2016; Berman et al., 2018; Gigli et al., 2018; Mandelli et al., 2019), its implementation in practical solid-solid lubrication schemes remains a challenging task. Several factors, such as the spontaneous pinning of sliding layers to a commensurate state during sliding, the range of applied load, sliding velocity, elastic deformations, edge effects, and contact size, act as key limiting factors for structural superlubricity (Dietzel et al., 2017; Vanossi et al., 2020). Moreover, contact size plays a crucial role in achieving superlubricity, as the threshold for the transition from an incommensurate to a commensurate state is closely related to the interaction energies between the contact surfaces and the lateral stiffness compliance.
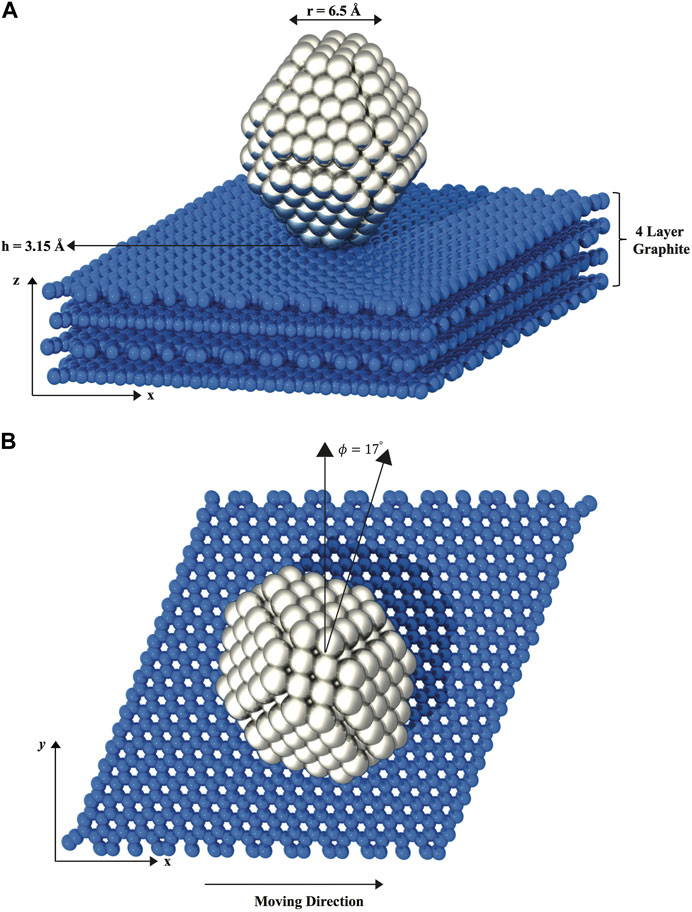
FIGURE 1. Simulated model of Pt cluster and 4 layer graphite system (A) side view of the system. Initial equilibrium distance is h = 3.15 Å and Pt cluster has radius of 6.5 Å (B) top view of the simulated system at incommensurate contact. The moving direction with pseudo AFM tip is also shown.
Clusters are promising candidates for addressing the key challenges in structural superlubricity and for gaining insights into friction mechanisms at the atomistic level. This is due to their higher Young’s modulus compared to bulk structures and their inherent high stiffness. Thus they can exhibit less elastic deformation during sliding. For instance, gold clusters have been shown to have a sliding regime called “ballistic sliding” on graphite with simulations (Guerra et al., 2010). Intriguingly, relatively large metal clusters make friction-free motions. Antimony clusters consisting of atoms within the range of 2,300–250, as well as gold clusters comprising 250 atoms, exhibit rapid diffusion on the graphite surface (Bardotti et al., 1996). Also, clusters that have an inert character to oxidize under ambient conditions and thus maintain an atomically flat interface with the substrate, preventing penetration by dirt molecules, have been shown to exhibit structurally lubric behavior (Cihan et al., 2016). Moreover, even for the same material, monoatomic islands and thick clusters will not exhibit the same friction as shown for metal clusters depending on their size and thickness (Guerra et al., 2010; Brndiar et al., 2011; Reguzzoni and Righi, 2012; Guerra et al., 2016). For instance, the friction force results obtained for Kr islands show that the commensurate configurations, below a critical size, are stable at this configuration and present static friction, as revealed by the stick-slip behavior commonly observed for tips in friction force measurement experiments (Reguzzoni and Righi, 2012). On the other hand, as stated by Aubry theory, an incommensurate 1D chain/substrate interface experience a superlubric sliding state or a pinned frictional state depending on the critical value of the substrate potential corrugation. That is, chemical interactions between sliding surfaces are likely more potent to govern nano friction than a critical size of nanoparticles as confirmed in (Dietzel et al., 2017). Since this theory is also applicable for nano contacts, it is clear that atom-by-atom control of a low friction state is possible only when mutual interactions at the contact satisfy the required conditions governed completely by quantum mechanical effects at the interface (Zanca et al., 2018).
Atomic force microscopy (AFM) tips serve as a valuable tool for investigating the dynamical friction of contacting bodies and measuring static friction. The challenge in using AFM to establish friction laws at the nanoscale lies in the large sensitivity of contact behavior to specific experimental conditions, such as surface chemistry or tip geometry. Overcoming these challenges has been made possible through the use of molecular dynamics simulations, which have proven invaluable in identifying and understanding the atomistic phenomena that underlie friction (Gao et al., 2007; Chandross et al., 2008; Klemenz et al., 2014; Gigli et al., 2018; Mandelli et al., 2019; Jiang et al., 2020). Despite limitations, all levels of MD modeling of friction at nanoscale with specific potentials are highly informative and predictive. Because we still do not have a comprehensive and concise theory of friction applicable to all cases due to its intricate behavior at the atomic scale.
Therefore, we selected Pt clusters to investigate the frictional characteristics of Pt cluster on a graphite surface. Pt clusters are known to resist oxidation under ambient conditions. Because of the high Young modulus of clusters compared to their bulk counterparts, we would expect that the Pt cluster can tolerate high loads owing to its intrinsic stiffness. Moreover, it has been observed that large Pt clusters exhibit superlubric sliding on graphite, although they tend to generate relatively higher frictional forces compared to similarly sized Au clusters (Ozoğul et al., 2017). We performed molecular dynamics simulations to examine the frictional properties of a small Pt cluster comprising 200 atoms arranged in 11 atomic layers from the (111) plane of bulk Pt. Our investigation focused on assessing the effects of commensurate and incommensurate orientations, considering both heat dissipation and load effects. Within our simulation constraints, we analyzed the friction force, friction coefficient, and potential energy changes at a temperature of 4 K. Additionally, we implemented a thermal thermostat to maintain the temperature at 4 K, allowing us to analyze the friction behavior of the Pt cluster while accounting for thermal dissipation.
2 Materials and methods
Figure 1 illustrates our simulation setup, depicting the friction between a 200-atom Pt cluster stacked within an 11-layer (cut from the (111) plane of bulk Pt) and a 4-layer graphite surface. The Pt cluster, with a radius of 6.5 Å, is simulated to slide on top of the graphite substrate. We anticipate that utilizing 11 atomic layers for the Pt cluster would be adequate to maintain an incommensurate state at the interface. This is because thick clusters have a smaller critical contact size required to sustain an incommensurate state during sliding, compared to thin clusters and islands. In general, the Pt (111) slab surface and graphite exhibit mutual incommensurability due to a geometric lattice mismatch between them. To facilitate a comparative analysis of the frictional properties, we intentionally created a Pt cluster orientation that is incommensurate with the graphite surface, as well as a commensurate one. This deliberate selection allows us to examine and contrast the frictional behavior between these different orientations more effectively. The interactions between Pt-Pt, C-C, and Pt-C atoms are modeled using Reaxx potential due to its capability of describing these intralayer and interlayer interactions (Sanz-Navarro et al., 2008).
The Reaxx potential incorporates a self-energy term to account for different charge states of atoms, a Coulomb energy term for electrostatic interactions, and a long-range van der Waals energy term to capture non-bonded interactions. Consequently, a properly parameterized Reaxx potential can effectively describe the atomic-level chemical and mechanical interactions, as well as the relaxation mechanisms, at the Pt-C interface. It offers computational efficiency advantages compared to first-principles methods (Liang et al., 2013).
To simulate a real atomic force microscopy (AFM) experiment and gain insights into the frictional mechanism between Pt and the graphite surface depending on the contact orientation, we employed a pulling tip connected to each Pt cluster atom through harmonic springs. These springs had a force constant (K) value of 16 meV/Å2, which was chosen to mimic the stiffness of an actual AFM tip. The force constant value ensures that the simulation closely approximates the experimental conditions encountered in AFM studies. This simulation setup is an extended version of the Frenkel-Kontorova-Tholimson model (Weiss and Elmer, 1996) in which the simulated nanosystem is driven by a constant velocity with attached springs. In our MD approach, interaction potentials U(r) between atoms are calculated using Reaxx potential. The forces on atoms at coordinates Ri are written as:
Since in a real AFM experiment, cantilever bends depend on the elastic interactions with a body, these elastic interactions can be treated as springs with a certain stiffness (Vanossi et al., 2013). In our simulations, normal loads are applied to the Pt cluster perpendicular to its surface to replicate the effect of normal loads applied in an atomic force microscopy (AFM) experiment. These loads are maintained as a constant force applied through the center of mass of the tip, and the values are positive. To investigate the friction behavior under different loads, the Pt cluster experiences normal loads ranging from 0.05 to 0.38 nN/atom. These loads can be further correlated to pressures ranging from 0.25 to 1.9 GPa. By varying the applied loads within this range, the study aims to examine how friction changes in response to different pressure conditions. To investigate the frictional behavior of the system, we simulate the dragging of the Pt cluster on the graphite surface with a constant lateral velocity of 0.001 Å/fs. It is important to note that the applied normal load remains constant during this dragging process. This approach allows us to examine the interatomic forces and the influence of the applied normal load on the frictional properties of the system, providing valuable insights into the mechanisms underlying the observed friction behavior. In this study, all simulations were carried out using LAMMPS code (Plimpton, 1995). Our quasistatic simulation approach is also previously followed by Fasolino (van Wijk et al., 2013) and Bonelli (Bonelli et al., 2009).
Instantaneous pulling force Fx is defined as the total force experienced by the tip in the direction of sliding x with a pulling velocity of
Here, K represents the spring constant and is assigned a value of 16 meV/Å2, while x represents the sliding direction of the tip.
Since all Pt atoms are attached and dragged with a pseudo AFM tip with these springs, no torque can form, and the Pt cluster cannot rotate during simulations. Therefore, the Pt cluster can keep its initial commensurate or incommensurate position with respect to the graphite surface. Since sliding velocity
Also, Fx can be written in terms of Normal load (N) and friction coefficient μ. The time average of Fx is equal to the friction force, which arises from the constant velocity of the dragged Pt cluster with the spring. This friction force is also equivalent to the total energy change of the system (ΔW) along the path given as
The total force Ftotal experienced by the Pt cluster atoms results from the interaction between Pt and graphite. This interaction has contributions from intermolecular and intramolecular interactions as given by:
Here, FReaxx denotes the force between Pt-C, C-C, and Pt-Pt atoms, while Fx represents the force acting in the sliding direction. Additionally, FLoad accounts for the load force experienced by the Pt atoms in the z direction (perpendicular to the Pt surface).
The load force experienced per Pt atom, denoted as FLoad, can be calculated by dividing the total load force, denoted as Load, by the total number of Pt cluster atoms, denoted as N is given by:
It is also a constant for certain values of different loads during each separate simulation. The friction coefficient μ is calculated by dividing the time average of frictional force to load for each load value and is given by:
All simulations were performed along a 5 Å path on graphite.
3 Results
Superlubricity implies that the frictional forces are significantly reduced or effectively eliminated, resulting in smooth and effortless sliding motion of the AFM tip over the surface. Therefore, if the friction is almost zero, it would generally be referred to as superlubricity, regardless of the applied load, in the context of AFM experiments. In our simulations, we dragged the Pt particle with constant velocity on the graphite surface. If a particle is sliding with a constant velocity along a lateral direction, the lateral forces acting on the particle should ideally be zero in the absence of any external influences, resulting in a frictionless motion. However, it is important to note that achieving such perfect superlubricity in practice may be challenging due to various factors such as surface roughness, interfacial interactions, and the elastic response of the system. For instance, the role of intermolecular forces, particularly van der Waals interactions, is significant in understanding the relationship between the friction coefficient and changes in the applied load. Van der Waals forces, which include London dispersion forces and dipole-dipole interactions, are responsible for the attraction and repulsion between atoms and molecules.
To elucidate the friction behavior of the Pt cluster, we initially started with commensurate and incommensurate contacts with the graphite surface. As shown in Figure 1, if the misfit angle ϕ is 0° between graphite surface normal and Pt cluster, contact atoms are mostly registered very well, resulting in commensurate contact corresponding to the zigzag direction of graphite. Whereas there are possibly many incommensurate contacts between the Pt cluster and graphite depending on the misfit angle ϕ. Since incommensurate contact leads to less registering of atoms at the interface, giving rise to less contact of atoms with each other compared to commensurate contact, we have deliberately selected a misfit angle of ϕ = 17° as one of the potential incommensurate contacts to obtain the simulation results. Under zero load conditions (L = 0 nN), the initial incommensurate contact between the Pt cluster and the graphite surface exhibits smooth motion as shown in Figure 2, characterized by a lack of significant resistance or friction. This behavior can be attributed to the weak interactions between the two surfaces, which allow for easy sliding and minimal energy dissipation. When no additional load is applied, the Pt cluster exhibits superlubric behavior during sliding.
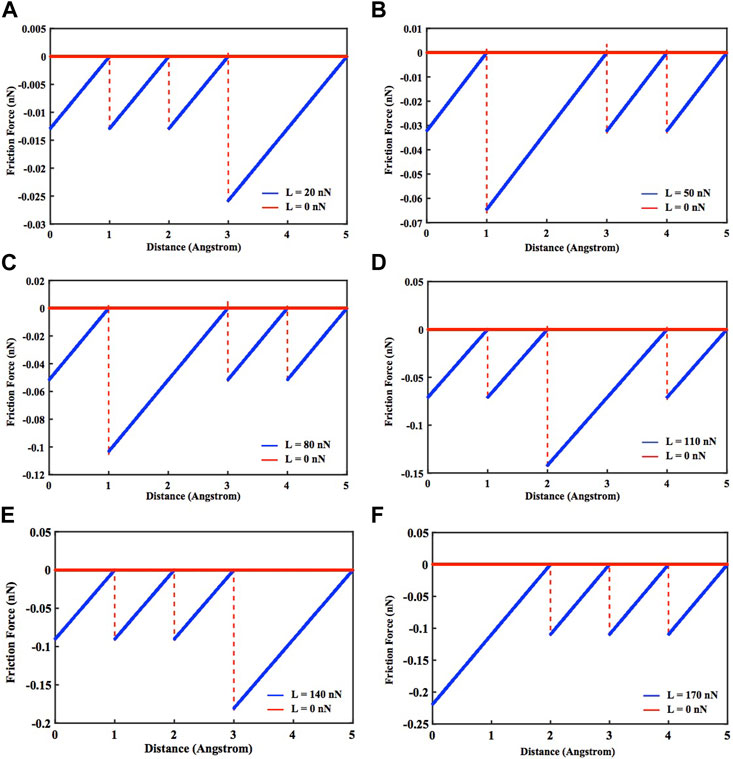
FIGURE 2. Panels (A–F) illustrate the change in Friction force (nN) of Pt-graphite incommensurate contact under various loads as a function of sliding distance (Å). The red line depicts the friction force with zero loads. L stands for the total load force on the system during the simulation.
Initially, as the Pt cluster moves under load, weak intermolecular forces such as van der Waals interactions between the Pt atoms and the graphite surface allow the cluster to adhere or “stick” to the surface. This adhesive force resists the motion of the cluster, resulting in a buildup of stress and deformation in the interfacial region.
However, as the applied load exceeds a certain threshold of 20 nN and above or when the stress accumulation becomes sufficiently high, the interfacial bonds weaken or rupture, leading to a sudden release of stored elastic energy and a rapid “slip” of the Pt cluster across the surface. This slip is accompanied by a decrease in the frictional forces, resulting in a temporary reduction in the resistance to motion and transitioning from an incommensurate state to a local commensurate state. The steep jumps observed in the stick-slip behavior represent the abrupt transition from static or near-static sticking to dynamic slipping. The release of stored elastic energy during this transition results in a rapid displacement of the Pt cluster, causing a sudden drop in frictional forces. This intermittent sticking and rapid slipping lead to a jerky motion, with the stick phase characterized by temporary pinning of the cluster to the surface due to stronger interatomic forces and a higher energy barrier for sliding. With increasing applied load, the interatomic forces between the Pt cluster and the graphite surface become stronger, resulting in a decrease in their initial equilibrium distance from 3.15 Å to 3.007 Å. These strengthened interatomic forces contribute to the observed stick-slip behavior under higher loads. Because it can influence the balance between attractive and repulsive van der Waals forces at the interface between the Pt particle and the graphite surface. The competition between attractive and repulsive forces at the interface determines the magnitude of frictional forces. As the load increases, the balance between these forces shifts, affecting the overall frictional response of the system. In fact, stick-slip motion is not possible for incommensurate contacts. When the initial incommensurate contact is transformed into a pinned commensurate state, we anticipate that the resulting metastable state, which locks the Pt cluster in that commensurate configuration, will correspond to stick-slip motion during sliding. We have checked the Pt cluster position during sliding on the graphite surface. Despite the Pt cluster being continuously dragged at a constant velocity during each molecular dynamics (MD) step, which causes a certain displacement in position, these metastable transitions are not explicitly evident in the position changes due to limitations in the time scale. However, the stick-slip motion of the Pt cluster shows that the Pt cluster rearranges in locally commensurate configuration during sliding as previously stated in (Kim and Falk, 2009). Moreover, before locking in a major position shift of the Pt cluster, the whole system can make abrupt transitions among the available metastable states depending on the energy barrier between these states in the potential energy surface. The occurrence of these abrupt transitions, leading to the eventual locking into a local commensurate state, is manifested as sudden changes in the friction force at different sliding positions, as confirmed in Figure 2. Although the initial configuration of the system is incommensurate with a certain misfit angle, the existence of these metastable states can drive the system into commensurate contact with a potential energy landscape. Since our simulation methodology is quasistatic, it can not capture the required time scale to analyze transitions among possible metastable configurations in the potential energy surface beneath the Pt cluster. Therefore, the time scale for these transitions is shorter compared to the time scale for position change of the Pt cluster. The system follows local potential energy minima surface during these transitions. As a result, the transition to a commensurate contact configuration leads to the occurrence of stick-slip motion upon applied load.
In addition, despite the weak van der Waals interactions at the interface, locally commensurate or incommensurate states can still form during sliding as a result of planar strains. These states can even induce localized structural deformations (He et al., 2022).
To examine the variation of μ for incommensurate and commensurate contact under different loads, we have computed μ by taking the time average of the friction force during the simulation. Our findings demonstrate that the friction coefficient μ is lower for incommensurate Pt cluster-graphite contact compared to commensurate contact, as depicted in Figure 3. This observation is consistent with previous studies on bulk counterparts (Ozoğul et al., 2017). The friction coefficient is 0.2 and 0.08 at maximum load values of 0.5 nN/atom for commensurate and incommensurate contacts, respectively. Incommensurate contact can exhibit friction coefficients as low as 0.02, higher than recent carbon quantum dots on graphite (Ma et al., 2017). The observed linear relationship between the friction coefficient and the applied load signifies that changes in the applied load result in proportional changes in the frictional forces. This consistent response suggests a linear dependency between these two variables. The linear relationship between the friction coefficient and the applied load can be physically interpreted in terms of the contact interactions between the Pt particle and the graphite surface. When the applied load on the system increases, it affects the nature and extent of contact between the two surfaces. As the applied load increases, the Pt particle experiences higher compressive forces, resulting in a larger contact area and increased interatomic interactions with the graphite surface. The increased contact area between the Pt cluster and graphite surface results in a higher number of interfacial bonds and stronger adhesion forces. This can be attributed to the reduced distance between the Pt cluster and graphite, facilitating closer atomic interactions. Consequently, the frictional forces acting on the Pt particle also increase. Similar behavior has been observed in CNx thin film (Kar et al., 2020). Furthermore, in commensurate contacts, the friction coefficient μ is higher compared to incommensurate contacts, indicating stronger registration of Pt atoms with the bottom graphite atoms in commensurate contact.
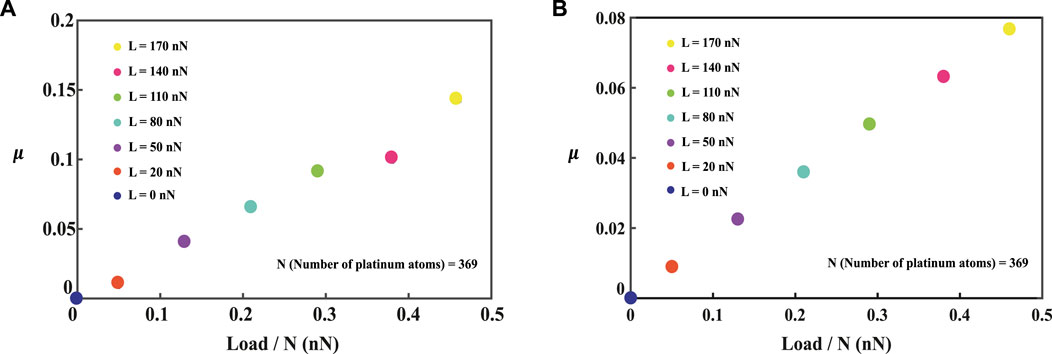
FIGURE 3. Friction coefficient for varying loads of (A) commensurate contact and (B) incommensurate contact of Pt-graphite system, respectively.
Structural lubricity is typically less pronounced in the presence of smaller atomic asperities, primarily influenced by the contact area. Because only then has critical contact size been reached for the cluster, incommensurate contact may occur otherwise, commensurate contact can easily develop as confirmed by some AFM experiments with atomic asperities (Guerra et al., 2016; Trillitzsch et al., 2018; He et al., 2022). It is well-documented that larger Pt clusters, when compared to the simulated cluster in this study, demonstrate higher friction forces on graphite compared to Au particles (Ozoğul et al., 2017).
To compare the behavior of incommensurate and commensurate contacts, we also performed simulations where the Pt cluster is dragged in commensurate contact with the graphite surface. In commensurate contact, friction force follows stick-slip behavior for different values of normal load as seen in Figure 4. As the Pt cluster remains pinned within the potential energy minima formed between the Pt cluster and the graphite surface, the spring force gradually becomes increasingly stronger as the Pt cluster continues to slide further. This squeezing of the spring force becomes so stiff that the Pt cluster no longer slides smoothly but instead jumps to the next minima on the potential energy surface, resulting in a discontinuous sliding motion. Therefore these steep jumps occur in friction force with stick-slip profiles in Figure 4.
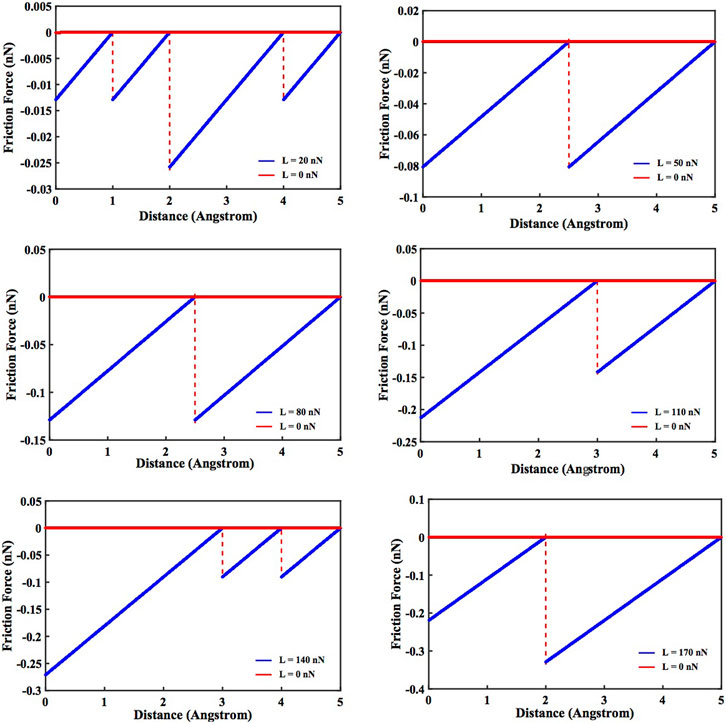
FIGURE 4. Friction force (nN) of Pt-graphite commensurate contact under various loads as a function of sliding distance (Å). The red line depicts the friction force with zero loads. L stands for the total load force on the system during the simulation. Notice the change from smooth to stick-slip behavior.
As the load increases from 20 nN to 170 nN, friction force also increases smoothly. This result confirms that the Pt cluster does not show single-asperity contact behavior, which is also argued in (Luan and Robbins, 2005) as a result of local contact roughness. The variations in the heights of the friction force peaks indicate that the Pt cluster becomes pinned at different depths of the potential energy surface along the sliding path. This pinning occurs due to the roughness or corrugation of the potential energy landscape at the local contact.
To confirm the differences in potential well depths between commensurate and incommensurate contacts, we have calculated the per-atom potential energy change (ΔE/atom) during the sliding motion. The initial state serves as the reference for ΔE/atom, which corresponds to the potential energy corrugation and provides insight into the adhesion of the surfaces at zero loads and the equilibrium distance. Under a 20 nN load, the incommensurate contact exhibits a higher potential energy change compared to the commensurate contact (Figure 5A). This difference in potential energy change explains the pinning of the Pt cluster in a metastable state, resulting in stick-slip motion during sliding despite the initial geometric mismatch. The varying distance between the Pt cluster and graphite during sliding, along with the elastic deformation of the edge atoms, likely contributes to the observed stick-slip behavior.
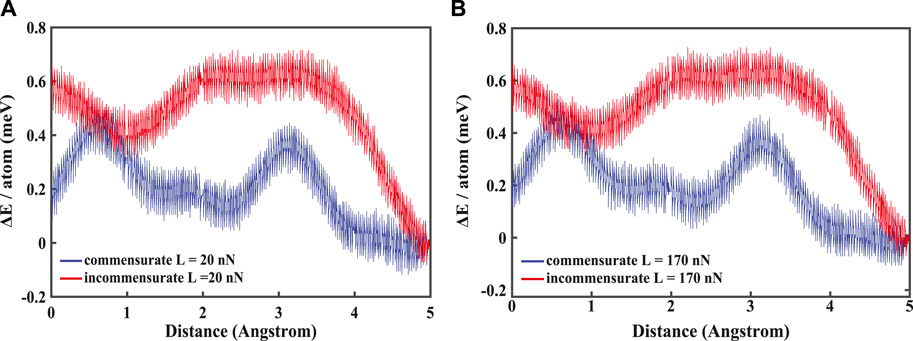
FIGURE 5. A. Potential energy change (ΔE/atom) (meV) for commensurate and incommensurate contacts under different load forces. (A) Load force: L = 20 nN. (B) Load force: L = 170 nN.
The potential well is also much deeper as much as 0.2 meV, and wider in incommensurate contact compared to commensurate contact as shown in Figures 5A, B. These barriers also indicate the positions where the Pt cluster jumps over these energy barriers without sliding. Since increasing the load does not significantly affect the potential well heights, the elimination of superlubricity between the Pt cluster and graphite mostly originates from local potential energy corrugations between the Pt cluster and graphite. The loss of superlubricity due to local metastable states can be hindered by clusters that have a larger radius, as shown in (Ma et al., 2015). This will turn from pinned to a superlubric condition as soon as cluster size gets large enough to accommodate the natural orientational mismatch, resulting in interface incommensurability. In general, the critical size will be thickness dependent, small for a thick cluster, and larger for a monolayer (Guerra et al., 2016). Larger clusters can experience fewer edge effects that may also eliminate the superlubricity (van Wijk et al., 2013). Elastic deformations of the substrate can further cancel superlubricity and hence affect friction. Since we have considered bulk graphite as substrate, elastic deformation of the substrate can not contribute to the total friction. Therefore depending on the local potential energy corrugation, the Pt cluster remains in commensurate contact, with similar behavior also observed in Si and Kr clusters on Cu (111) (Reguzzoni and Righi, 2012).
To examine the effect of cluster size on the sliding behavior, we performed simulations with a larger Pt cluster measuring 20 Å radius on a graphite surface, as shown in Supplementary Figures S1, S2. Interestingly, even with a larger cluster, we observed a loss of superlubric sliding when a load of 170 nN was applied as shown in Supplementary Figure S3. This finding is consistent with experimental observations where only Pt slabs or significantly larger Pt clusters (average 100 nm) were able to slide smoothly, as supported by both experimental and atomic simulation studies (Ozoğul et al., 2017). From these results, we infer that the geometric incommensurability of the Pt cluster with the substrate is primarily determined by the size of the cluster, assuming the cluster-substrate interaction remains consistent with the Pt (111) surface-graphite system.
4 Discussion
Our simulation setup examined the friction force between Pt clusters and graphite under different load conditions for both commensurate and incommensurate contacts. The concept of superlubricity, where two surfaces slide against each other with minimal friction, is of particular interest. When the applied load is zero (L = 0 nN), our system exhibited nearly superlubric behavior, with no significant frictional force observed. This is due to the minimal van der Waals forces present at the equilibrium distance between the Pt clusters and the graphite surface. However, we observed that the Pt cluster and graphite cannot sustain the superlubric state, even in initially incommensurate contacts. This is primarily caused by the local pinning of the Pt cluster on the graphite surface, influenced by its size and applied load. As a result, stick-slip behavior was observed, indicating the loss of structural lubricity. Similar observations have been reported in other systems, such as Kr islands and Si clusters, where the size of the asperities also leads to the breakdown of superlubricity (Reguzzoni and Righi, 2012).
These findings emphasize the challenges associated with maintaining structural lubricity in Pt cluster-graphite systems and highlight the importance of sustaining incommensurate contact. The frictional behavior is significantly influenced by applied load forces, leading to a transition from an incommensurate to a commensurate state. This observation highlights the detrimental effect of load forces on the frictional properties. This suggests that the incommensurate state may not be truly incommensurate but rather transitioning to a commensurate state. Understanding these mechanisms is crucial for future research aiming to preserve incommensurate contact and achieve superlubric behavior in nanoscale systems. These insights contribute to fundamental understanding of frictional behavior at solid interfaces and have implications for the design of advanced lubrication systems and nanoscale devices.
In summary, this study provides valuable insights into the breakdown of structural lubricity in Pt cluster-graphite systems and advances our understanding of frictional behavior at the nanoscale.
Data availability statement
The original contributions presented in the study are included in the article/Supplementary Material, further inquiries can be directed to the corresponding author.
Author contributions
SC has done simulation calculations. SI has prepared all simulation inputs and analyzed the data. SI wrote the paper. Figures were plotted by SC. All authors contributed to the article and approved the submitted version.
Funding
This work was supported by Istanbul Medeniyet University Scientific Research Projects Office under Project No. FB169. S.
Acknowledgments
Sİ acknowledges the dedicated work of all medical doctors and nurses during the COVID-19 pandemic.
Conflict of interest
The authors declare that the research was conducted in the absence of any commercial or financial relationships that could be construed as a potential conflict of interest.
Publisher’s note
All claims expressed in this article are solely those of the authors and do not necessarily represent those of their affiliated organizations, or those of the publisher, the editors and thereviewers. Any product that may be evaluated in this article, or claim that may be made by its manufacturer, is not guaranteed or endorsed by the publisher.
Supplementary material
The Supplementary Material for this article can be found online at: https://www.frontiersin.org/articles/10.3389/fmech.2023.1211072/full#supplementary-material
References
Bardotti, L., Jensen, P., Hoareau, A., Treilleux, M., Cabaud, B., Perez, A., et al. (1996). Diffusion and aggregation of large antimony and gold clusters deposited on graphite. Surf. Sci. 367, 276–292. doi:10.1016/S0039-6028(96)00875-8
Berman, D., Narayanan, B., Cherukara, M. J., Sankaranarayanan, S. K. R. S., Erdemir, A., Zinovev, A., et al. (2018). Operando tribochemical formation of onion-like-carbon leads to macroscale superlubricity. Nat. Commun. 9, 1164. doi:10.1038/s41467-018-03549-6
Bonelli, F., Manini, N., Cadelano, E., and Colombo, L. (2009). Atomistic simulations of the sliding friction of graphene flakes. Eur. Phys. J. B 70, 449–459. doi:10.1140/epjb/e2009-00239-7
Brndiar, J., Turanský, R., and Štich, I. (2011). Simulation of frictional behavior of sb nanoparticles on Hopg: Frictional duality and biduality. Phys. Rev. B 84, 085449. doi:10.1103/PhysRevB.84.085449
Bylinskii, A., Gangloff, D., Counts, I., and Vuletić, V. (2016). Observation of aubry-type transition in finite atom chains via friction. Nat. Mater. 15, 717–721. doi:10.1038/nmat4601
Chandross, M., Lorenz, C. D., Stevens, M. J., and Grest, G. S. (2008). Simulations of nanotribology with realistic probe tip models. Langmuir 24, 1240–1246. doi:10.1021/la702323y
Cihan, E., İpek, S., Durgun, E., and Baykara, M. Z. (2016). Structural lubricity under ambient conditions. Nat. Commun. 7, 12055. doi:10.1038/ncomms12055
Dietzel, D., Brndiar, J., Štich, I., and Schirmeisen, A. (2017). Limitations of structural superlubricity: Chemical bonds versus contact size. ACS Nano 11, 7642–7647. doi:10.1021/acsnano.7b02240
Gao, G., Cannara, R. J., Carpick, R. W., and Harrison, J. A. (2007). Atomic-scale friction on diamond: A comparison of different sliding directions on (001) and (111) surfaces using md and afm. Langmuir 23, 5394–5405. doi:10.1021/la062254p
Gigli, L., Manini, N., Tosatti, E., Guerra, R., and Vanossi, A. (2018). Lifted graphene nanoribbons on gold: From smooth sliding to multiple stick-slip regimes. Nanoscale 10, 2073–2080. doi:10.1039/C7NR07857A
Guerra, R., Tartaglino, U., Vanossi, A., and Tosatti, E. (2010). Ballistic nanofriction. Nat. Mater. 9, 634–637. doi:10.1038/nmat2798
Guerra, R., Tosatti, E., and Vanossi, A. (2016). Slider thickness promotes lubricity: From 2d islands to 3d clusters. Nanoscale 8, 11108–11113. doi:10.1039/c6nr00520a
He, Y., She, D., Liu, Z., Wang, X., Zhong, L., Wang, C., et al. (2022). Atomistic observation on diffusion-mediated friction between single-asperity contacts. Nat. Mater. 21, 173–180. doi:10.1038/s41563-021-01091-3
Hirano, M., and Shinjo, K. (1990). Atomistic locking and friction. Phys. Rev. B 41, 11837–11851. doi:10.1103/PhysRevB.41.11837
Jiang, B., Zhao, Z., Gong, Z., Wang, D., Yu, G., and Zhang, J. (2020). Superlubricity of metal-metal interface enabled by graphene and mows4 nanosheets. Appl. Surf. Sci. 520, 146303. doi:10.1016/j.apsusc.2020.146303
Kar, S., Sahu, B. B., Kousaka, H., Han, J. G., and Hori, M. (2020). Study of the effect of normal load on friction coefficient and wear properties of cnx thin films. AIP Adv. 10, 065214. doi:10.1063/5.0009783
Kim, W. K., and Falk, M. L. (2009). Atomic-scale simulations on the sliding of incommensurate surfaces: The breakdown of superlubricity. Phys. Rev. B 80, 235428. doi:10.1103/PhysRevB.80.235428
Klemenz, A., Pastewka, L., Balakrishna, S. G., Caron, A., Bennewitz, R., and Moseler, M. (2014). Atomic scale mechanisms of friction reduction and wear protection by graphene. Nano Lett. 14, 7145–7152. doi:10.1021/nl5037403
Lee, S., and Spencer, N. D. (2008). Sweet, hairy, soft, and slippery. Science 319, 575–576. doi:10.1126/science.1153273
Liang, T., Shin, Y. K., Cheng, Y.-T., Yilmaz, D. E., Vishnu, K. G., Verners, O., et al. (2013). Reactive potentials for advanced atomistic simulations. Annu. Rev. Mater. Res. 43, 109–129. doi:10.1146/annurev-matsci-071312-121610
Luan, B., and Robbins, M. O. (2005). The breakdown of continuum models for mechanical contacts. Nature 435, 929–932. doi:10.1038/nature03700
Ma, M., Benassi, A., Vanossi, A., and Urbakh, M. (2015). Critical length limiting superlow friction. Phys. Rev. Lett. 114, 055501. doi:10.1103/PhysRevLett.114.055501
Ma, W., Gong, Z., Gao, K., Qiang, L., Zhang, J., and Yu, S. (2017). Superlubricity achieved by carbon quantum dots in ionic liquid. Mater. Lett. 195, 220–223. doi:10.1016/j.matlet.2017.02.135
Mandelli, D., Leven, I., Hod, O., and Urbakh, M. (2017). Sliding friction of graphene/hexagonal -boron nitride heterojunctions: A route to robust superlubricity. Sci. Rep. 7, 10851. doi:10.1038/s41598-017-10522-8
Mandelli, D., Ouyang, W., Hod, O., and Urbakh, M. (2019). Negative friction coefficients in superlubric graphite–hexagonal boron nitride heterojunctions. Phys. Rev. Lett. 122, 076102. doi:10.1103/PhysRevLett.122.076102
Ozoğul, A., İpek, S., Durgun, E., and Baykara, M. Z. (2017). Structural superlubricity of platinum on graphite under ambient conditions: The effects of chemistry and geometry. Appl. Phys. Lett. 111, 211602. doi:10.1063/1.5008529
Plimpton, S. (1995). Fast parallel algorithms for short-range molecular dynamics. J. Comput. Phys. 117, 1–19. doi:10.1006/jcph.1995.1039
Rees, D. G., Beysengulov, N. R., Lin, J.-J., and Kono, K. (2016). Stick-slip motion of the wigner solid on liquid helium. Phys. Rev. Lett. 116, 206801. doi:10.1103/PhysRevLett.116.206801
Reguzzoni, M., and Righi, M. C. (2012). Size dependence of static friction between solid clusters and substrates. Phys. Rev. B 85, 201412. doi:10.1103/PhysRevB.85.201412
Sanz-Navarro, C. F., Östrand, P.-O., Chen, D., Rønning, M., van Duin, A. C. T., Jacob, T., et al. (2008). Molecular dynamics simulations of the interactions between platinum clusters and carbon platelets. J. Phys. Chem. A 112, 1392–1402. doi:10.1021/jp074806y
Sweeney, J., Hausen, F., Hayes, R., Webber, G. B., Endres, F., Rutland, M. W., et al. (2012). Control of nanoscale friction on gold in an ionic liquid by a potential-dependent ionic lubricant layer. Phys. Rev. Lett. 109, 155502. doi:10.1103/PhysRevLett.109.155502
Trillitzsch, F., Guerra, R., Janas, A., Manini, N., Krok, F., and Gnecco, E. (2018). Directional and angular locking in the driven motion of au islands on mos2. Phys. Rev. B 98, 165417. doi:10.1103/PhysRevB.98.165417
van Wijk, M. M., Dienwiebel, M., Frenken, J. W. M., and Fasolino, A. (2013). Superlubric to stick-slip sliding of incommensurate graphene flakes on graphite. Phys. Rev. B 88, 235423. doi:10.1103/PhysRevB.88.235423
Vanossi, A., Manini, N., Urbakh, M., Zapperi, S., and Tosatti, E. (2013). Colloquium: Modeling friction: From nanoscale to mesoscale. Rev. Mod. Phys. 85, 529–552. doi:10.1103/RevModPhys.85.529
Vanossi, A., Bechinger, C., and Urbakh, M. (2020). Structural lubricity in soft and hard matter systems. Nat. Commun. 11, 4657. doi:10.1038/s41467-020-18429-1
Weiss, M., and Elmer, F.-J. (1996). Dry friction in the frenkel-kontorova-tomlinson model: Static properties. Phys. Rev. B 53, 7539–7549. doi:10.1103/PhysRevB.53.7539
Keywords: Pt cluster, graphite, superlubricity, molecular dynamics simulation, nanotribologly, tomlinson model, nanofriction, molecular dynamics simulations
Citation: Cörüt S and İpek S (2023) Molecular dynamics simulation of frictional properties of Pt cluster on graphite under load. Front. Mech. Eng 9:1211072. doi: 10.3389/fmech.2023.1211072
Received: 24 April 2023; Accepted: 06 June 2023;
Published: 20 June 2023.
Edited by:
Kadir Bilisik, Erciyes University, TürkiyeReviewed by:
Iakov A. Lyashenko, Technical University of Berlin, GermanyGuoxin Xie, Tsinghua University, China
Kemal Özdogan, Yıldız Technical University, Türkiye
Ozhan Unverdi, Yaşar University, Türkiye
Copyright © 2023 Cörüt and İpek. This is an open-access article distributed under the terms of the Creative Commons Attribution License (CC BY). The use, distribution or reproduction in other forums is permitted, provided the original author(s) and the copyright owner(s) are credited and that the original publication in this journal is cited, in accordance with accepted academic practice. No use, distribution or reproduction is permitted which does not comply with these terms.
*Correspondence: S. İpek, c2VtcmFuLmlwZWtAbWVkZW5peWV0LmVkdS50cg==