- 1Department of Biology, Connecticut College, New London, CT, USA
- 2The Ecosystems Center, Marine Biological Laboratory, Woods Hole, MA, USA
Since the discovery of ammonia-oxidizing archaea (AOA), new questions have arisen about population and community dynamics and potential interactions between AOA and ammonia-oxidizing bacteria (AOB). We investigated the effects of long-term fertilization on AOA and AOB in the Great Sippewissett Marsh, Falmouth, MA, USA to address some of these questions. Sediment samples were collected from low and high marsh habitats in July 2009 from replicate plots that received low (LF), high (HF), and extra high (XF) levels of a mixed NPK fertilizer biweekly during the growing season since 1974. Additional untreated plots were included as controls (C). Terminal restriction fragment length polymorphism analysis of the amoA genes revealed distinct shifts in AOB communities related to fertilization treatment, but the response patterns of AOA were less consistent. Four AOB operational taxonomic units (OTUs) predictably and significantly responded to fertilization, but only one AOA OTU showed a significant pattern. Betaproteobacterial amoA gene sequences within the Nitrosospira-like cluster dominated at C and LF sites, while sequences related to Nitrosomonas spp. dominated at HF and XF sites. We identified some clusters of AOA sequences recovered primarily from high fertilization regimes, but other clusters consisted of sequences recovered from all fertilization treatments, suggesting greater physiological diversity. Surprisingly, fertilization appeared to have little impact on abundance of AOA or AOB. In summary, our data reveal striking patterns for AOA and AOB in response to long-term fertilization, and also suggest a missing link between community composition and abundance and nitrogen processing in the marsh.
Introduction
Oxidation of ammonia to nitrite is a critical process in nitrogen cycling and is carried out by a suite of distinct microorganisms within the bacterial and archaeal domains. The fate of nitrogen is of particular importance in nitrogen-sensitive coastal systems, such as estuaries and salt marshes, where primary productivity is typically nitrogen-limited (Valiela and Teal, 1974; Howarth, 1988). Nitrogen-cycling processes in salt marshes, therefore, play a significant role in the health and preservation of coastal habitats, yet our understanding of the ecology of the organisms responsible and their responses to perturbations is still evolving.
Over the last decade, researchers have made great progress in describing the diversity and distribution of ammonia-oxidizing bacteria (AOB) in estuaries (see review by Bernhard and Bollmann, 2010) and to a lesser extent in salt marshes (Dollhopf et al., 2005; Moin et al., 2009). Surveys of 16S rRNA genes and ammonia monooxygenase genes (coding for the enzyme responsible for the first step in ammonia oxidation) in estuaries and salt marshes indicate highly similar AOB communities in geographically distinct habitats that are typically dominated by sequences most closely related to Nitrosospira spp., although representative AOB have yet to be obtained in pure culture. Most studies have also reported salinity to be a major factor regulating the distribution and diversity of AOB in these coastal systems (Francis et al., 2003; Bernhard et al., 2005; Ward et al., 2007). However, with the recent discovery of ammonia-oxidizing archaea (AOA; Könneke et al., 2005; Treusch et al., 2005) and their apparent wide distribution in many habitats, the relative importance of AOB to nitrification and their potential interactions with AOA have been brought into question.
The diversity and distribution of AOA have begun to be explored in estuaries and salt marshes, but, unlike the AOB, no consistent patterns or common regulatory factors have been identified. Although archaeal amoA gene sequences from both the water column/sediment and soil/sediment clusters as described in Francis et al. (2005) have been recovered from estuaries and salt marshes, to date, most archaeal amoA genes fall into the water column/sediment cluster, but the diversity of sequence types is quite high (Bernhard and Bollmann, 2010). Furthermore, factors that have been identified as potentially regulating their distribution are many, including salinity, temperature, pH, dissolved oxygen, nitrogen, and net primary productivity (Beman and Francis, 2006; Sahan and Muyzer, 2008; Santoro et al., 2008; Moin et al., 2009). Thus, our understanding of AOA communities and what regulates their diversity and distribution, and ultimately, their activity in these important coastal ecosystems is still rudimentary.
Manipulating the environment and following changes in communities can be a powerful approach to help tease apart important regulatory factors. Using this approach, Lage et al. (2010) identified changes in AOB communities in relation to short-term N and P additions in a salt marsh. Others have taken a similar approach in soil systems to study changes in AOB communities (Chu et al., 2007, 2008; Shen et al., 2011), and have generally reported significant shifts in activity, community composition, and/or abundance. However, studies of AOA in response to fertilization have yielded conflicting results (Kelly et al., 2011; Verhamme et al., 2011; Wu et al., 2011).
In this study, we explored the diversity and distribution of AOA and AOB in a series of salt marsh plots that have received different levels of commercial fertilizer for nearly 40 years. The plots were originally fertilized to study the capacity of salt marshes to remove and retain nutrients (Valiela et al., 1973). Significant effects of fertilization on other components of the marsh, including vegetation (Valiela et al., 1975, 1976), invertebrates (Meany et al., 1976; Sarda et al., 1996), diatoms (Van Raalte et al., 1976), and nitrogen-cycling processes (Van Raalte et al., 1974; Hamersley and Howes, 2005) have been documented. More recently, Bowen et al. (2011) reported little evidence of fertilization impacts on bacterial and denitrifying communities in the marsh. To further the work of Bowen et al. (2011) on describing effects of fertilization on microbial communities, we report the first investigation of fertilization impacts on nitrifying microorganisms in the marsh. Two of the questions addressed by our study are: (1) Are there major shifts in AOA and AOB communities that correspond with fertilization treatments? and (2) Do specific populations of AOA or AOB respond predictably to fertilization?
Known effects of experimental enrichment on the nitrogen cycle in salt marsh sediments lead to a prediction that there must be significant responses by ammonia oxidizers to the supply of nitrogen entering salt marsh ecosystems. We know that denitrification rates increase as N supply increases (Hamersley and Howes, 2005), and concentrations of ammonium are consistently higher in fertilized plots relative to those in control plots (Hamersley and Howes, 2005). Since denitrification in the fertilized salt marsh sediments is supported largely by in situ oxidation of ammonium (Hamersley and Howes, 2005), we anticipated an increase in abundance of ammonia oxidizers accompanied by shifts in community composition.
Materials and Methods
Site Description
This study was conducted in the Great Sippewissett Marsh, Falmouth, MA, USA (Figure 1). Circular plots (10 m diameter) of salt marsh vegetation have been fertilized every 2 weeks during the growing season since the early 1970s (Valiela et al., 1973, 1975). Three different levels of mixed NPK fertilizer were applied to each set of two replicate plots: low (LF; 8.4 g/m2/week), high (HF; 25 g/m2/week), and extra high (XF; 75 g/m2/week). The fertilizer is a commercially available sewage sludge fertilizer (10% N, 6% P, 4% K by weight). Forty percent of the N is inorganic, but only 28% is immediately soluble (Valiela et al., 1973). An additional two replicate plots were left unfertilized as controls (C). The rates of fertilization correspond to nitrogen loading rates of 12 (C), 182 (LF), 532 (HF), and 1572 (XF) kg N/ha/year (Fox et al., 2012), and include atmospheric deposition on the marsh surface. The plots are located within an experimental area of 10 ha. Areas within the plots receiving frequent (daily) tidal inundation and dominated by Spartina alterniflora are designated as low marsh habitat. Areas above mean high tide and dominated by S. patens (in control plots) or Distichlis spicata (in XF plots) and are designated as high marsh habitat. More detailed information regarding recent vegetation cover within the plots is reported elsewhere (Fox et al., 2012).
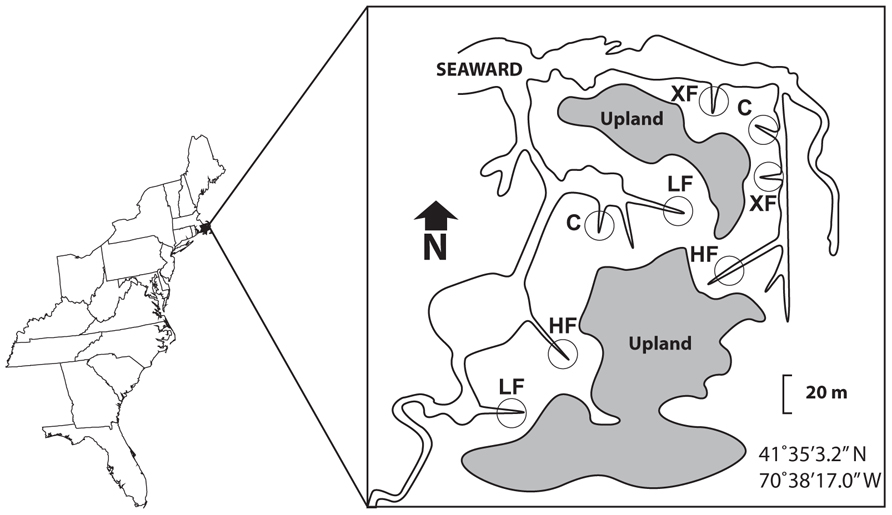
FIGURE 1. Map of study plots in the Great Sippewissett Marsh, Falmouth, MA, USA. C, control plots with no fertilizer added; LF, low fertilization; HF, high fertilization; XF, extra high fertilization (levels of fertilization can be found in Section “Materials and Methods”).
Sample Collection
Duplicate sediment cores (6.5 cm in diameter) were collected from low marsh habitat in each of eight experimental plots described above in July 2009 during low tide. Within the C and XF plots, two additional cores were collected from the high marsh habitat. Cores were kept on ice in the dark for several hours during transport to the lab. Salinity was measured by a handheld refractometer from pore water extracted from each core by centrifugation.
DNA Extraction
Subsamples (ca. 0.5 g) were taken from sediment cores at a depth of 0–2 cm, and stored at -80°C. DNA was extracted using the PowerSoil DNA Isolation Kit (MO BIO, Carlsbad, CA, USA) according to the manufacturer’s protocol. DNA concentrations were measured spectrophotometrically using a SmartSpec spectrophotometer (Bio-Rad, Hercules, CA, USA) and fluorometrically using a Quant-It fluorometer (Invitrogen, Grand Island, NY, USA). Extracted DNA was also assessed by gel electrophoresis (1% agarose) with 0.1 μl/ml of ethidium bromide. All DNA extractions were performed on duplicate 0.5 g aliquots of sediment and the better of each extraction (assessed by quality and quantity) was selected for downstream analysis.
TRFLP Analysis
Archaeal amoA genes were amplified with Arch-AmoAF and Arch-AmoAR (Francis et al., 2005). Betaproteobacterial amoA genes were amplified with amoA-1F and amoA-2R-TC (Rotthauwe et al., 1997; Nicolaisen and Ramsing, 2002). Each 20 μl reaction contained 1× DreamTaq Buffer with KCl and (NH4)2SO4 (Fermentas, Inc., Glen Burnie, MD, USA), 320 ng/μl of bovine serum albumin, 3.75 mM of MgCl2, 0.2 mM of dNTPs, 0.5 U of DreamTaq DNA Polymerase (Fermentas), 0.4 μM of each primer, and approximately 2–10 ng DNA. Each forward primer was labeled at the 5′ end with 6-FAM (Eurofins MWG Operon, Huntsville, AL, USA) and all reactions were carried out in an iCycler (Bio-Rad). Archaeal amoA genes were amplified with the following cycle conditions: 30 s of initial denaturation at 95°C, followed by 35 cycles of 95°C for 15 s, 54°C for 20 s, and 72°C for 45 s, and ending with a final elongation at 72°C for 5 min. Betaproteobacterial amoA genes were amplified with the same parameters except the annealing temperature was 57°C.
Polymerase chain reaction (PCR) products were digested with 10 U of AciI (NEB), 2 μl of 10× NEBuffer 3 in a 20-μl reaction volume for 6 h at 37°C. Following ethanol precipitation, 10 μl of formamide, 5 μl of filter-sterilized MilliQ water, and 0.2 μl of GeneScan 500 Size Standard (Applied Biosystems, Foster City, CA, USA) were added to the samples. Samples were analyzed at the Cornell University Life Sciences Core Laboratories Center using an Applied Biosystems 3730xl DNA Analyzer. Community fingerprints were analyzed using GeneMarker 1.4 (SoftGenetics, State College, PA, USA). Only terminal restriction fragments (TRFs) that correspond to a sequence either in our sequences reported here or from GenBank were included in the final community analyses. Although including only TRFs represented by a known sequence likely underestimates the community diversity in the terminal restriction fragment length polymorphism (TRFLP) analysis, particularly for the less well-described archaeal amoA diversity, we believe it minimizes artifacts that may significantly skew the analysis.
amoA Gene Cloning and Sequencing
Archaeal and betaproteobacterial amoA genes were amplified and cloned as previously described (Moin et al., 2009). A total of 14 clone libraries were constructed. Betaproteobacterial and archaeal amoA libraries were constructed from plots 3 (C), 5 (LF), 9 (HF), 6 (XF) in the low marsh and plots 7 (C) and 6 (XF) in the high marsh. An additional archaeal amoA clone library was constructed from low marsh in plot 8 (XF) for comparison between plots and an additional betaproteobacterial amoA clone library was constructed from a duplicate core collected from low marsh in plot 3 (C). Each library consisted of 96 randomly selected clones. Approximately 48–96 clones from each library were sequenced using the T3 primer and aligned in ARB (Ludwig et al., 2004). In silico TRF sizes were determined for all sequences.
Real-Time PCR Analyses
Betaproteobacterial amoA genes were quantified as described in Bernhard et al. (2007). Archaeal amoA genes were quantified as described in Moin et al. (2009). Bacterial 16S rRNA genes were quantified as described in Bernhard et al. (2012). Average amplification efficiencies from at least three separate runs were 101.3 ± 13.3% (betaproteobacterial amoA genes), 107.4 ± 5.2% (archaeal amoA genes), and 91.7 ± 4.5% (bacterial 16S rRNA genes). We calculated copy numbers of amoA genes per gram of sediment by correcting the number of copies detected in each PCR for the DNA extraction volume obtained from a known amount of sediment for each sample. We also normalized amoA gene copies to μg DNA (measured fluorometrically) and to copies of bacterial 16S rRNA genes. We detected no significant differences in amount of DNA among fertilization treatments (ANOVA, P = 0.2)
Statistical Analysis
ANOVA, t-test, Pearson correlation, and multiple regression analyses were performed in InStat v3.0b (GraphPad, La Jolla, CA, USA). Data from duplicate cores in each plot were averaged prior to statistical analyses. Gene abundance data were log transformed prior to analyses to alleviate heteroscedasticity.
Non-metric multidimensional scaling (NMS) was used to ordinate arcsine transformed TRFLP data with Sørensen’s distance measure (Bray–Curtis), using a stability criterion of 0.00001. All ordinations used the Autopilot mode set to slow and thorough, and a suggested iterative optimization procedure (McCune and Mefford, 1999). Final stress, final instability, number of iterations, difference from Monte Carlo test, recommended dimensionality, and final ordination scores were used to evaluate the results. Ordinations were rotated to maximize the correlation of fertilization rates on axis 1.
Sequence Analysis
Sequences were compared to published sequences in GenBank using the Basic Local Alignment Search Tool (blastn) to identify related sequences and aligned using the sequence editor and Fast Align in ARB. All alignments were checked manually and regions of ambiguous alignments were excluded from the analysis. Phylogenetic relationships were analyzed by the neighbor-joining algorithm with the Kimura two-parameter correction in ARB. Confidence in tree topology was assessed by 100 bootstrap replicates using PHYLIP v.3.69. Sequences were checked for chimeras by comparing phylogenetic placement in trees constructed with the 5′ and the 3′ ends of the sequence. Pairwise sequence comparisons were calculated in ARB and operational taxonomic units (OTUs) were defined as sequences sharing ≥95% nucleotide sequence identity using MOTHUR (Schloss et al., 2009). Coverage of each clone library was calculated with the equation: C = 1 - (n/N) where n = number of singleton sequences and N = total number of sequences analyzed. A total of 526 betaproteobacterial amoA sequences and 310 archaeal amoA sequences were included in the final analysis. Sequence data have been submitted to the GenBank database under accession numbers JX283750–JX284059 (archaeal amoA) and JX306111–JX306636 (betaproteobacterial amoA).
Results
Salinity ranged from 23.6 ± 4.4 in the LF plots to 26.8 ± 1.8 psu in the C plots in the low marsh habitat and was not significantly different among fertilization treatments. In the high marsh plots, however, salinity was significantly lower (P = 0.0004) at the XF plots (11.7 ± 2.7 psu) compared to salinity in the control plots (31.0 ± 0.3 psu). Other variables, such as ammonium and sulfide, vary considerably among plots and a summary of these differences can be found elsewhere (Hamersley and Howes, 2005; Bowen et al., 2011).
Shifts in AOB Community Composition
Terminal restriction fragment length polymorphism analysis of betaproteobacterial amoA genes revealed a total of 12 TRFs and an average of 8.3 TRFs per sample. TRF 130 and 192 were the most abundant overall. Only one TRF (472) was not identified in our sequence dataset, but this TRF represents Nitrosomonas eutropha, so we included it in our analysis.
Analysis of betaproteobacterial amoA TRFLP patterns using NMS ordinations revealed distinct communities related to fertilization in both the low and high marsh habitats (Figure 2A). In the low marsh, there was generally a linear shift from AOB communities in control plots to AOB communities in XF plots, with AOB communities from LF and HF plots falling in between those from C and XF plots.
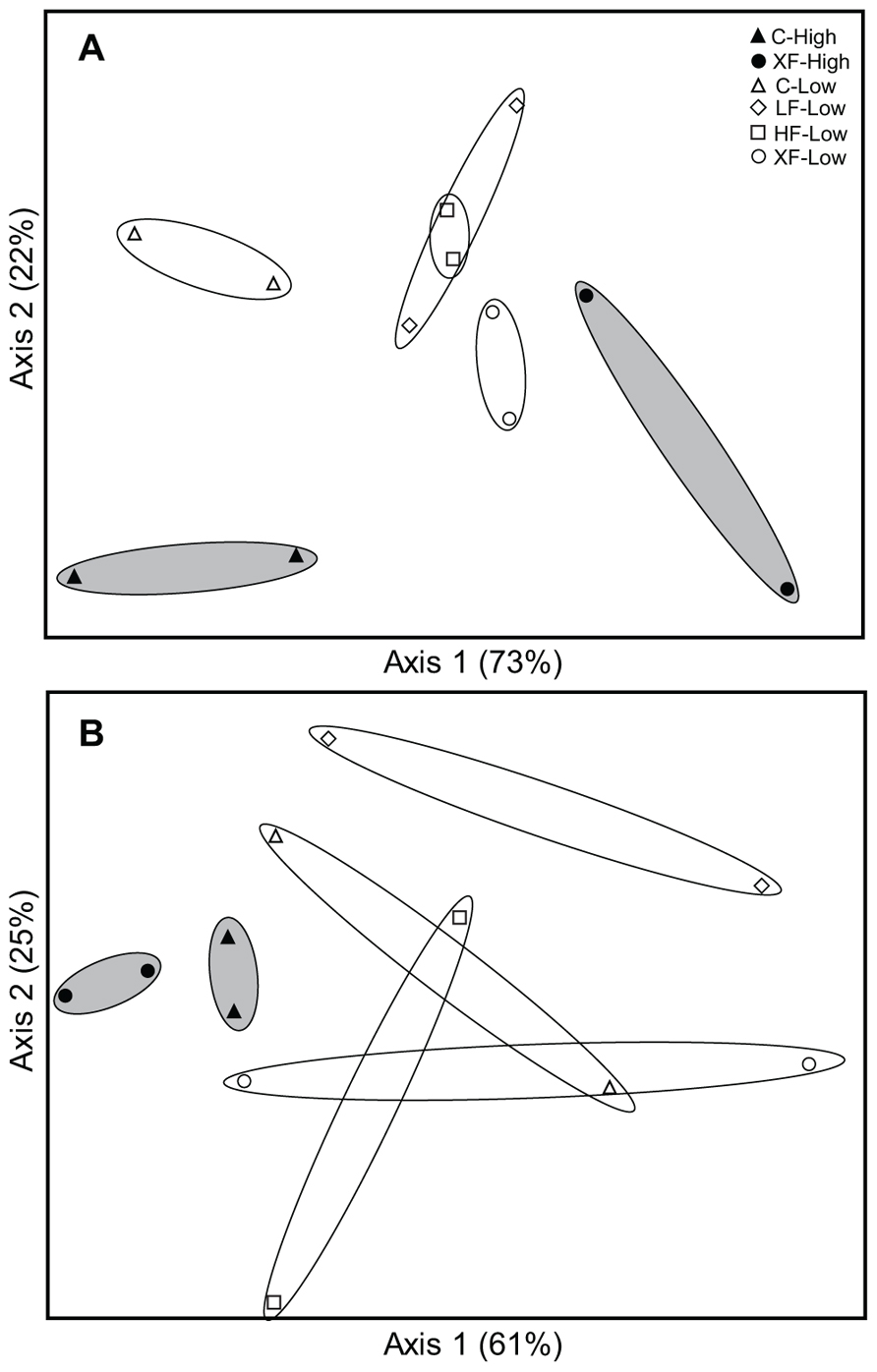
FIGURE 2. Non-metric multidimensional scaling ordinations based on TRFLP profiles for betaproteobacterial amoA genes (A) and archaeal amoA genes (B). Low marsh samples are represented by open symbols; high marsh samples are represented by filled symbols. Polygons (white-filled for low marsh, gray-filled for high marsh) are drawn around replicate samples in each plot. The percent variability explained by each axis is indicated parenthetically.
Correlation of ordination axis scores with environmental variables indicated that axis 1 was most strongly correlated with fertilization rate (r = 0.76, P < 0.004) and N loading rate (r = 0.76, P = 0.004), followed by salinity (r = -0.61, P = 0.03). Axis 2 was not significantly correlated with any of the variables.
Coverage of the betaproteobacterial amoA gene clone libraries ranged from 89.8 to 100% and we detected a total of 12 OTUs that closely matched the TRFs (Figure 3). Sixty-one percent of the sequences were affiliated with the uncultured Nitrosospira-like cluster, with the remainder affiliated with the Nitrosomonas cluster.
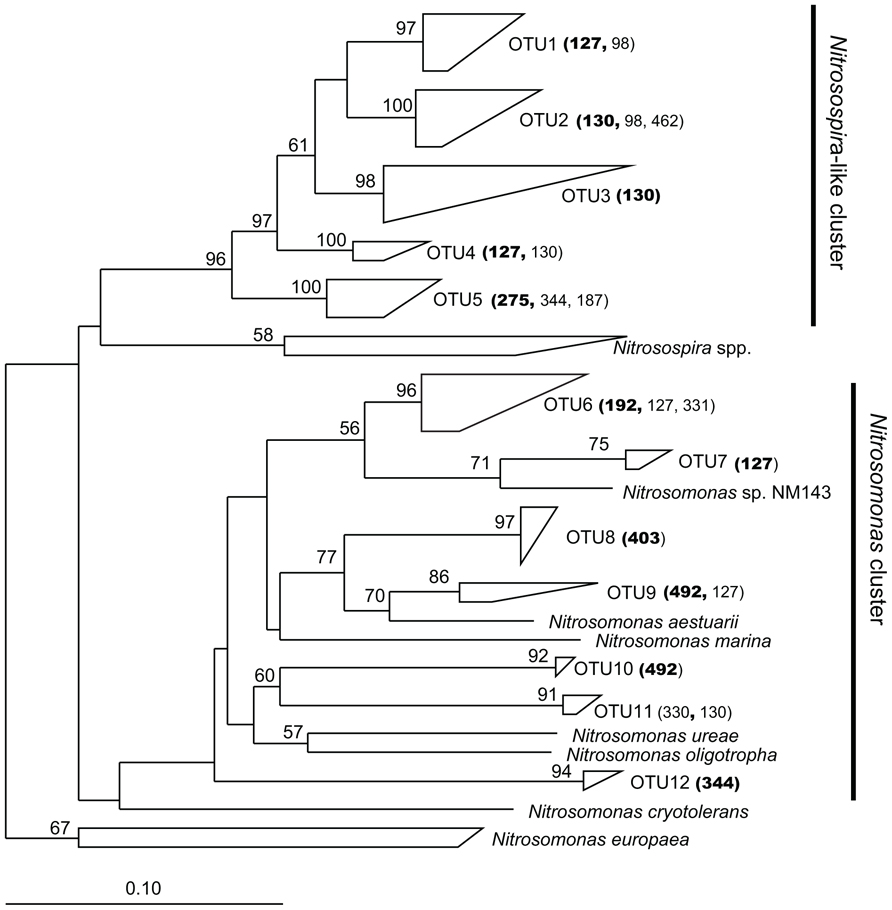
FIGURE 3. Phylogenetic relationships among betaproteobacterial amoA nucleotide sequences recovered from plots in the Great Sippewissett Marsh. The tree was inferred from 466 nucleotides using the neighbor-joining algorithm with the Kimura two-parameter correction. Bootstrap values >50% are shown at the internal nodes. Each polygon represents sequences from a single OTU with the TRF sizes of the sequences (number of base pairs determined in silico) indicated parenthetically. TRF sizes representing ≥85% of the sequences in the cluster are shown in bold.
Responses of AOB Populations to Fertilization
We evaluated relative abundance of each TRF in relation to fertilization rates to characterize responses of specific AOB populations. Four TRFs were significantly correlated with fertilization rates (Table 1), with TRF492 showing the strongest positive response to fertilization. Distribution of sequences among the fertilized treatments corroborated the TRF patterns. For example, all 31 sequences representing TRF 492 in OTUs 9 and 10 were recovered exclusively from HF and XF plots.
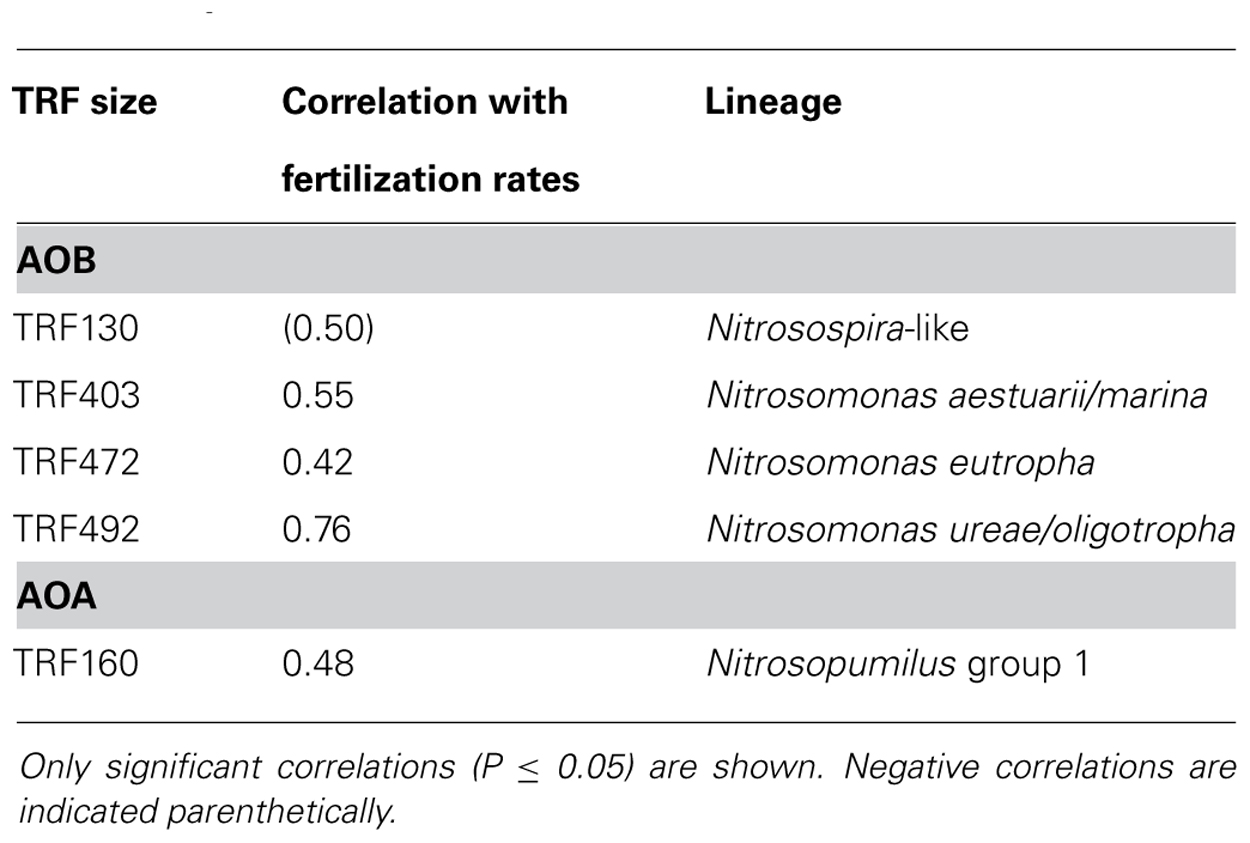
TABLE 1. Pearson’s correlation coefficients (r) describing the relationships between abundance of TRFs and fertilization rates.
We also observed a striking shift in the distribution of Nitrosospira-like and Nitrosomonas-related sequences related to fertilization rates (Figure 4A). Ninety-one percent of the Nitrosospira-like sequences were recovered from C and LF sites, while Nitrosomonas sequences were found mostly in HF and XF sites.
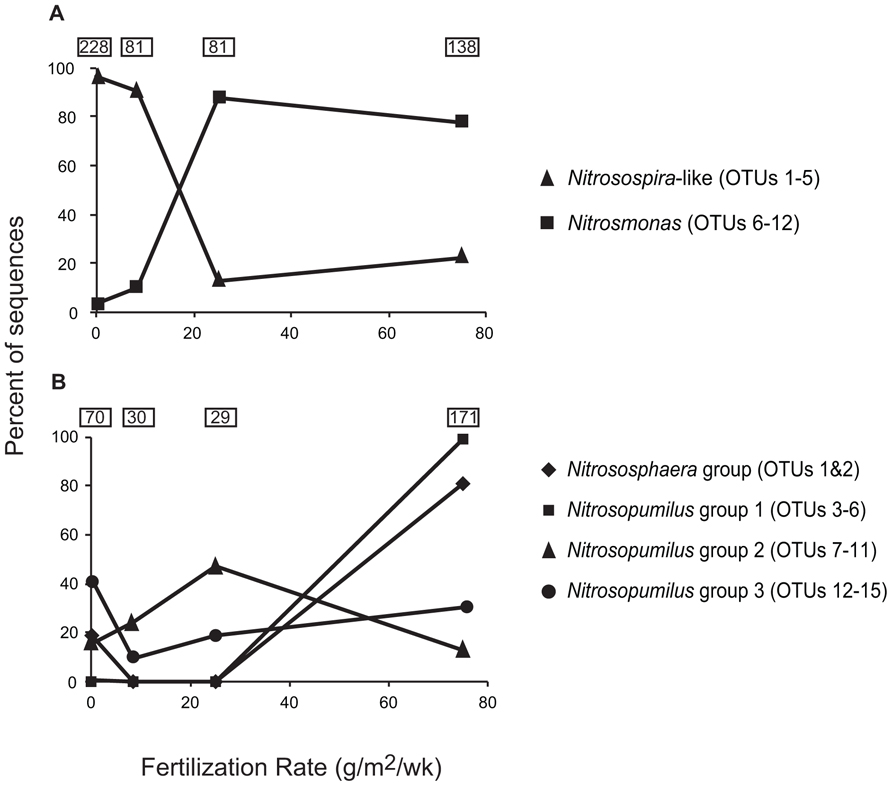
FIGURE 4. Distribution of betaproteobacterial amoA clones (A) and archaeal amoA clones (B) related to fertilization rates. Groups are based on phylogenetic clusters designated in Figures 3 and 5. The number of sequences used to generate data points for each fertilization rate are shown in boxes.
Shifts in AOA Community Composition
A total of 12 AOA TRFs were identified among all plots. TRF 383 was the most abundant overall in both the low and high marsh habitats (23.9 and 39.9% relative abundance, respectively) and 29.8% of the sequences recovered from all the sites had this predicted TRF. This is also one of two TRFs (along with TRF 257) representing sequences most closely related to Nitrosopumilus maritimus (Figure 5). TRF52 was identified from sequence data (Figure 5), but was not included in the TRFLP profile because we could not discriminate actual signal from background for TRFs smaller than 70 bp.
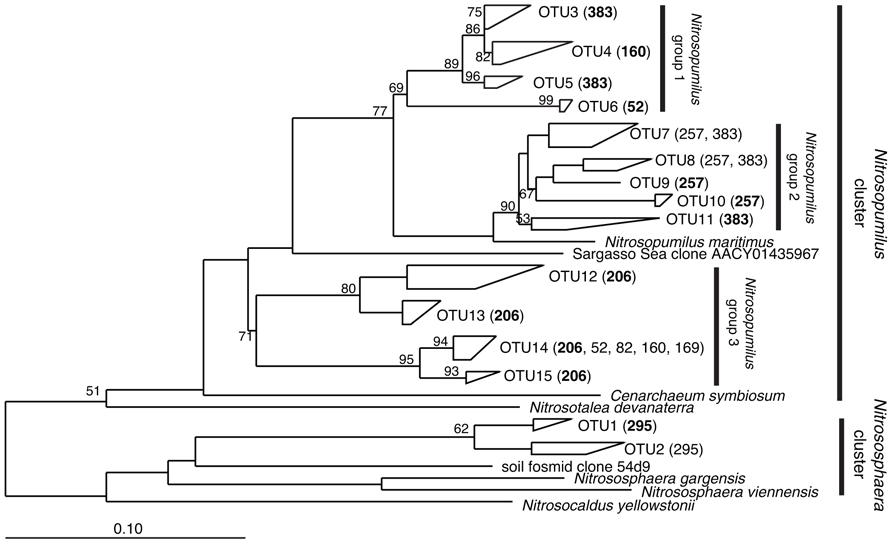
FIGURE 5. Phylogenetic relationships among archaeal amoA nucleotide sequences recovered from plots in the Great Sippewissett Marsh. The tree was inferred from 569 nucleotides using the neighbor-joining algorithm with the Kimura two-parameter correction. Bootstrap values >50% are shown at the internal nodes. Each polygon represents sequences from a single OTU with the TRF sizes of the sequences (number of base pairs determined in silico) indicated parenthetically. TRF sizes representing ≥85% of the sequences in the cluster are shown in bold.
Non-metric multidimensional scaling ordination of archaeal communities based on TRFLP data suggest some effect of fertilization treatment, but the patterns were not robust (Figure 2B). There were distinct AOA communities in the control and XF plots in the high marsh sites, but the patterns among the low marsh samples were less convincing. Furthermore, unlike the results for AOB communities, we found no significant correlations of ordination scores for axis 1 (or 2) with fertilization rates, N loading rates, or salinity.
Analysis of archaeal amoA gene sequences revealed 15 OTUs (Figure 5). Although a few of the phylogenetic clusters were characterized by a unique TRF, there was generally less agreement between AOA TRF and OTU distribution compared to AOB. The majority of sequences were affiliated with the water column/sediment cluster as designated by Francis et al. (2005), more recently defined as the Nitrosopumilus cluster described by Pester et al. (2012); Figure 6). Twenty-one sequences fell within the Nitrososphaera cluster as identified by Pester et al. (2012), a subset of the soil/sediment cluster. Coverage of archaeal amoA gene diversity in the clone libraries ranged from 92.9 to 100%.
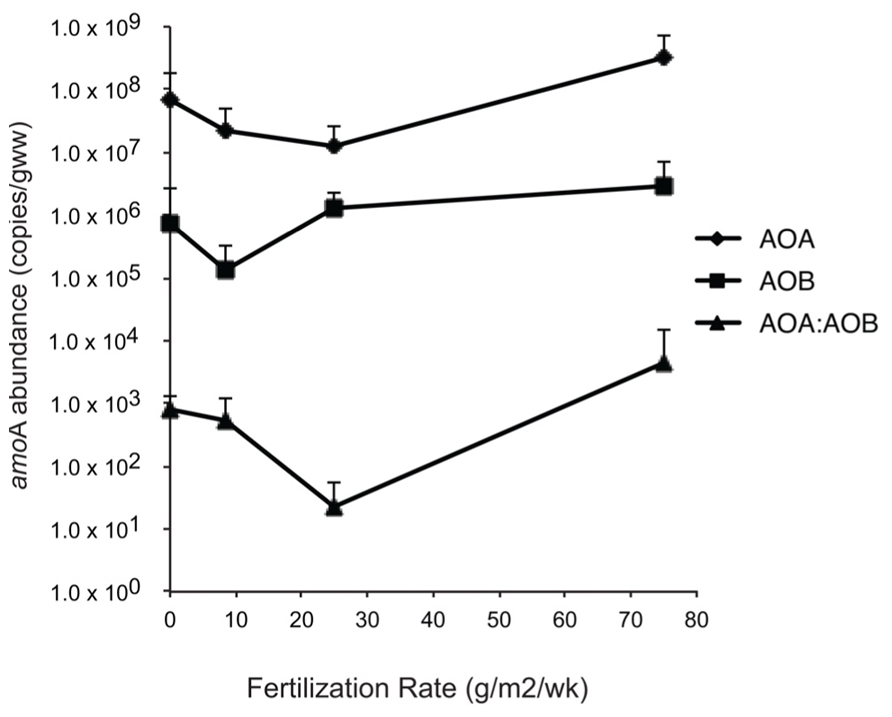
FIGURE 6. Abundance of betaproteobacterial and archaeal amoA genes and ratios of amoA genes relative to levels of fertilization.
Response of AOA Populations to Fertilization
Contrary to patterns observed for AOB, only one TRF showed a significant correlation with fertilization rates (Table 1). However, there were more distinct patterns within the sequence data (Figure 4B). We identified a subcluster of 109 sequences within the Nitrosopumilus cluster that was composed exclusively (with the exception of a single sequence) of sequences recovered from XF plots. We also observed a similar pattern for sequences affiliated with the Nitrososphaera cluster.
AOA and AOB Abundance
Abundance of betaproteobacterial amoA genes ranged from 4.5 × 103 to 1.3 × 107 gene copies/g sediment, and was one to three orders of magnitude lower than archaeal amoA abundance (Figure 6). Although abundance was highest in the XF plots, the differences among mean values were not significant and linear regression analysis also confirmed no significant fertilization effect.
Abundance of archaeal amoA genes ranged from 2.0 × 105 to 1.2 × 109 gene copies/g sediment (wet weight) and were not significantly different among fertilization treatments. Linear regression analysis also confirmed no significant fertilization effect. Ratios of AOA:AOB abundance ranged from 0.5 to 3.1 × 104 and were highest at the XF plots (Figure 6), although the differences were not significant.
To account for possible differences in DNA extraction efficiencies, we normalized AOB and AOA amoA gene abundances to numbers of bacterial 16S rRNA gene copies and to μg DNA (Table 2). Patterns of abundance for normalized data were virtually identical to patterns based on copy numbers per gram of sediment (data not shown).
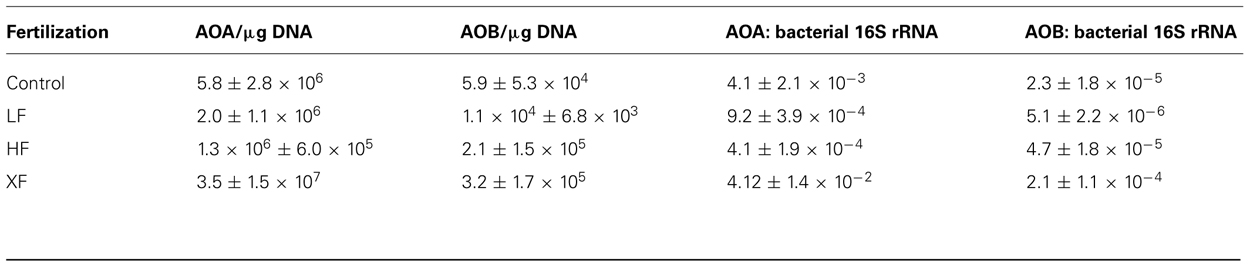
TABLE 2. Mean (±SE) betaproteobacterial (AOB) and archaeal amoA (AOA) gene abundances presented as gene copies per μg DNA or normalized to copies of bacterial 16S rRNA genes in plots receiving different levels of fertilization.
Discussion
The effects of long-term fertilization in the Great Sippewissett Marsh have been documented for many of the major biological components of the marsh over the past four decades, elucidating fundamental mechanisms driving salt marsh ecology and environmental change. However, studies investigating the effects of fertilization on microbial communities have been lacking. Understanding how microorganisms respond to fertilization is important not only for predicting how microbially mediated ecosystem services may be altered by anthropogenically driven changes to the environment, but also for increasing our understanding of fundamental ecological principles that drive community dynamics. Just recently, Bowen et al. (2011) published the first study on microbial responses in the same plots that were sampled in our study and, rather surprisingly, found no fertilization effect on the composition of total bacterial or denitrifying communities. However, it is possible that there are differential responses among some microbial groups that were not detected in the analysis by Bowen et al. (2011), warranting additional studies within the marsh microbial community, particularly given the significant differences reported for some nitrogen-cycling processes in the marsh (Van Raalte et al., 1974; Hamersley and Howes, 2005). Our investigation of ammonia-oxidizing microorganisms in the marsh suggest, in fact, a complex pattern of responses that provide further insights into mechanisms controlling nitrifying populations and communities in coastal ecosystems.
AOB Community Composition and Response to Fertilization
We found significant shifts in AOB communities that corresponded to fertilization, which is in agreement with several previous studies of AOB in fertilized soils and sediments (Chu et al., 2007; Shen et al., 2011; Wu et al., 2011). Our results, however, are somewhat contrary to the recent study by Lage et al. (2010) who found shifts in salt marsh AOB community composition only when a single nutrient (N or P) was applied, but not when both were applied together. Since the fertilizer applied in our study is a complex mix, it is difficult to attribute changes in community composition to a particular nutrient. However, our fertilization rates were 4–12 times higher than those used by Lage et al. (2010), and may account for the differences in community response.
The distribution of sequences we recovered for the betaproteobacterial amoA gene was generally similar to distributions found from other estuarine and salt marsh studies, with the majority of sequences falling into the Nitrosospira-like cluster (see Bernhard and Bollmann, 2010 for review). Sequences within this cluster were recovered from all fertilization treatments as well as both marsh habitats, suggesting that AOB within this cluster are either physiologically diverse or have high physiological plasticity.
Conversely, all of the OTUs within the Nitrosomonas group were restricted by marsh habitat or fertilization treatment, with the exception of OTU 7, suggesting that Nitrosomonas-related AOB in the marsh are more specialized compared to their Nitrosospira counterparts. Particularly striking was the lack of sequences from control or LF sites among OTUs affiliated with the Nitrosomonas aestuarii/marina and the Nitrosomonas ureae/oligotropha clusters. Other studies of AOB in fertilized or polluted sediments have also found AOB communities to be dominated by Nitrosomonas-related sequences (Beman and Francis, 2006; Cao et al., 2011a,b).
Identification of specific AOB populations that showed consistent response to fertilization may help to better characterize the ecophysiology of uncultured AOB, and may serve as important clues to assist in the ultimate cultivation of these culturally recalcitrant AOB. The differential responses of specific AOB populations also suggest mechanisms for niche-differentiation within this relatively constrained phylogenetic group. Such distinct and reproducible patterns of AOB community composition in relation to fertilization also support the use of AOB as bioindicators, as has been previously suggested (Dang et al., 2010).
AOA Community Composition and Response to Fertilization
The lack of a consistent response of the AOA community composition to fertilization was somewhat unexpected given the fertilization history and significant differences in nitrogen-processing rates previously reported. However, results from other studies of AOA community composition in response to fertilization report a similar lack of response. Wu et al. (2011) found that long-term fertilization (22 years) with urea did not alter AOA communities in a paddy soil, and Verhamme et al. (2011) found that AOA communities in soils were not different from controls after short-term (28 days) ammonium additions. We detected distinct AOA communities related to fertilization in the high marsh, but because the salinity in the XF high marsh plots was significantly lower than in the control plots, the shift in AOA communities in the high marsh may be due to differences in salinity rather than fertilization. Salinity has been shown previously to correlate with changes in AOA abundance (Moin et al., 2009) and community composition (Sahan and Muyzer, 2008). It is also possible that the AOA community may respond to fertilization at other times of the year as their requirements change with seasonal environmental changes. Seasonal shifts in AOA communities have been reported in some estuaries (Sahan and Muyzer, 2008).
Although we did not detect robust patterns among the AOA communities related to fertilization rates based on TRFLP data, we identified some patterns among the sequence data suggesting a possible fertilization effect. OTUs 3, 4, 5, and 6 (Nitrosopumilus group 1 in Figure 5) were composed exclusively (with one exception) of sequences recovered from XF sites, suggesting that these AOA may be well-adapted to high nutrient conditions. Unfortunately, some of the TRFs representing OTUs within Nitrosopumilus group 1 were also found in other OTUs, thus obscuring any patterns related to fertilization in the TRFLP analysis. Sequences representing the Nitrososphaera group were also dominant among XF plots, but the number of sequences in this group was relatively small compared to other groups. Additionally, the sequences we recovered from both Nitrosopumilus group 1 and the Nitrososphaera group were closely related to AOA recovered from environments that were not nutrient-enriched, indicating that the sequences in these clusters are not necessarily indicative of high nutrient conditions.
Environmental factors identified as potentially important in regulating AOA community composition are many and include salinity, temperature, pH, nitrite, dissolved oxygen, net primary productivity, and some heavy metals (reviewed in Erguder et al., 2009). However, common factors regulating AOA communities in coastal systems have not clearly emerged, suggesting that AOA may be a more physiologically diverse group relative to the AOB and may respond differentially to changing environmental conditions. Other studies in marine environments suggest that AOB and AOA respond to different environmental cues (Hollibaugh et al., 2011; Bouskill et al., 2012), but exactly what the cues for AOA are in salt marshes remain unclear.
AOA and AOB Abundance
Abundances of AOA and AOB were within the ranges of abundances reported in other estuarine and salt marsh studies (Bernhard and Bollmann, 2010). However, we were somewhat surprised by the lack of robust and consistent fertilization effects on either AOA or AOB abundance, particularly given what we know about increases in nitrogen-processing rates in the fertilized plots. Although we did detect a significant fertilization effect for AOA, the pattern of abundance was not compelling, suggesting something other than fertilization is regulating abundance. Others have reported significant changes in AOA and AOB abundance in response to fertilization in agricultural soil systems (Chu et al., 2008; Kelly et al., 2011; Shen et al., 2011; Wu et al., 2011), but Lage et al. (2010) reported no effect of fertilization on AOB abundance in a salt marsh. The lack of a compelling change in abundance in our fertilized plots is that AOA and AOB may be limited by some other factor, such as pH, oxygen, or sulfide. Sulfide levels can be quite high in salt marsh sediments due to tidal flooding, and effects of sulfide on nitrification are well-documented (Joye and Hollibaugh, 1995; Caffrey et al., 2010). Periods of anoxia may also be quite frequent and may further limit nitrification and thus, abundance of nitrifiers.
The lack of a consistent and robust response of AOA and AOB abundance to differences in fertilization may also be due to the complex nature of the fertilizer applied. Differential responses of AOA and AOB populations to different components of the fertilizer may diminish any effects on overall abundance. Studies that isolate individual environmental factors may be necessary to determine the effects of specific components on the AOA and AOB.
Conclusion
Our results revealed differential responses of AOA and AOB communities and abundance to long-term fertilization in a New England salt marsh and provide some insight into population dynamics, particularly among AOB, and potential regulatory factors of nitrifiers in the marsh. AOB communities showed robust and consistent responses to fertilization, while responses of AOA communities were less clear, possibly reflecting differences in physiological tolerances. However, abundance patterns of AOA and AOB showed minimal responses to fertilization treatments, suggesting that factors other than nutrients, such as redox conditions, may limit abundance of these organisms in the marsh.
We find it notable that in spite of what we know about nitrogen cycling in the marsh, there was no compelling response of AOA or AOB abundance or AOA community composition to long-term fertilization. This leads us to suspect that either the methods are not inclusive of all nitrifiers in the marsh or that some targeted populations are not obligate nitrifiers. Additionally, since the metabolic pathway for ammonia oxidation in archaea has not been fully elucidated, it is possible that the archaeal amoA gene is not an appropriate marker for characterizing AOA populations. However, given the equally surprising results reported by Bowen et al. (2011) showing no response of denitrifying communities using different methods to analyze samples from the same plots used in our study, we are forced to consider that we are missing a critical link between community composition and abundance and nitrogen processes in the marsh.
Conflict of Interest Statement
The authors declare that the research was conducted in the absence of any commercial or financial relationships that could be construed as a potential conflict of interest.
Acknowledgments
This work was supported in part by the National Science Foundation award DEB-0814586 (to Anne E. Bernhard). Additional support was provided by the George and Carol Milne Endowment at Connecticut College. We thank the students in Bernhard’s Molecular Ecology class for generating clone libraries of archaeal amoA genes and providing preliminary sequence analysis. The Great Sippewissett Marsh fertilization experiment was originally initiated by Valiela and John Teal. We thank Brian Howes and Dale Goehringer for maintaining the experimental plots in recent years.
References
Beman, J. M., and Francis, C. A. (2006). Diversity of ammonia-oxidizing archaea and bacteria in the sediments of a hypernutrified subtropical estuary: Bahi’a del To’bari, Mexico. Appl. Environ. Microbiol. 72, 7767–7777.
Bernhard, A. E., and Bollmann, A. (2010). Estuarine nitrifiers: new players, patterns, and processes. Estuar. Coast. Shelf Sci. 88, 1–11.
Bernhard, A. E., Donn, T., Giblin, A. E., and Stahl, D. A. (2005). Loss of diversity of ammonia-oxidizing bacteria correlates with increasing salinity in an estuary system. Environ. Microbiol. 7, 1289–1297.
Bernhard, A. E., Marshall, D., and Yiannos, L. (2012). Increased variability of microbial communities in restored salt marshes nearly 30 years after tidal flow restoration. Estuaries Coast. 35, 1049–1059.
Bernhard, A. E., Tucker, J., Giblin, A. E., and Stahl, D. A. (2007). Functionally distinct communities of ammonia-oxidizing bacteria along an estuarine salinity gradient. Environ. Microbiol. 9, 1439–1447.
Bouskill, N. J., Eveillard, D., Chien, D., Jayakumar, A., and Ward, B. B. (2012). Environmental factors determining ammonia-oxidizing organism distribution and diversity in marine environments. Environ. Microbiol. 14, 714–729.
Bowen, J. L., Ward, B. B., Morrison, H. G., Hobbie, J. E., Valiela, I., Deegan, L. A., et al. (2011). Microbial community composition in sediments resists perturbation by nutrient enrichment. ISME J. 5, 1540–1548.
Caffrey, J. M., Hollibaugh, J. T., Bano, N., and Haskins, J. (2010). Effects of upwelling on short-term variability in microbial and biogeochemical processes in estuarine sediments from Elkhorn Slough, California, USA. Aquat. Microb. Ecol. 58, 261–271.
Cao, H., Hong, Y., Li, M., and Gu, J. D. (2011a). Community shift of ammonia-oxidizing bacteria along an anthropogenic pollution gradient from the Pearl River Delta to the South China Sea. Appl. Microbiol. Biotechnol. 94, 1–13.
Cao, H., Li, M., Dang, H., and Gu, J. D. (2011b). Responses of aerobic and anaerobic ammonia/ammonium-oxidizing microorganisms to anthropogenic pollution in coastal marine environments. Methods Enzymol. 496, 35–62.
Chu, H., Fujii, T., Morimoto, S., Lin, X., and Yagi, K. (2008). Population size and specific nitrification potential of soil ammonia-oxidizing bacteria under long-term fertilizer management. Soil Biol. Biochem. 40, 1960–1963.
Chu, H., Fujii, T., Morimoto, S., Lin, X., Yagi, K., Hu, J., et al. (2007). Community structure of ammonia-oxidizing bacteria under long-term application of mineral fertilizer and organic manure in a sandy loam soil. Appl. Environ. Microbiol. 73, 485–491.
Dang, H., Li, J., Chen, R., Wang, L., Guo, L., Zhang, Z., et al. (2010). Diversity, abundance, and spatial distribution of sediment ammonia-oxidizing betaproteobacteria in response to environmental gradients and coastal eutrophication in Jiaozhou Bay, China. Appl. Environ. Microbiol. 76, 4691–4702.
Dollhopf, S. L., Hyun, J.-H., Smith, A. C., Adams, H. J., O’Brien, S., and Kostka, J. E. (2005). Quantification of ammonia-oxidizing bacteria and factors controlling nitrification in salt marsh sediments. Appl. Environ. Microbiol. 71, 240–246.
Erguder, T. H., Boon, N., Wittebolle, L., Marzorati, M., and Verstraete, W. (2009). Environmental factors shaping the ecological niches of ammonia-oxidizing archaea. FEMS Microbiol. Rev. 33, 855–869.
Fox, L., Valiela, I., and Kinney, E. L. (2012). Vegetation cover and elevation in long-term experimental nutrient-enrichment plots in Great Sippewissett Salt Marsh, Cape Cod, Massachusetts: implications for eutrophication and sea level rise. Estuaries Coast. 35, 445–458.
Francis, C. A., O’Mullan, G. D., and Ward, B. B. (2003). Diversity of ammonia monooxygenase (amoA) genes across environmental gradients in Chesapeake Bay sediments. Geobiology 1, 129–140.
Francis, C. A., Roberts, K. J., Beman, J. M., Santoro, A. E., and Oakley, B. B. (2005). Ubiquity and diversity of ammonia-oxidizing archaea in water columns and sediments of the ocean. Proc. Natl. Acad. Sci. U.S.A. 102, 14683–14688.
Hamersley, M. R., and Howes, B. L. (2005). Coupled nitrification-denitrification measured in situ in a Spartina alterniflora marsh with a 15NH4+ tracer. Mar. Ecol. Prog. Ser. 299, 123–135.
Hollibaugh, J. T., Gifford, S., Sharma, S., Bano, N., and Moran, M. A. (2011). Metatranscriptomic analysis of ammonia-oxidizing organisms in an estuarine bacterioplankton assemblage. ISME J. 5, 866–878.
Howarth, R. W. (1988). Nutrient limitation of net primary production in marine ecosystems. Annu. Rev. Ecol. Syst. 19, 89–110.
Joye, S. B., and Hollibaugh, J. T. (1995). Influence of sulfide inhibition of nitrification on nitrogen regeneration in sediments. Science 270, 623–625.
Kelly, J. J., Policht, K., Grancharova, T., and Hundal, L. S. (2011). Distinct responses in ammonia-oxidizing archaea and bacteria after addition of biosolids to an agricultural soil. Appl. Environ. Microbiol. 77, 6551–6558.
Könneke, M., Bernhard, A. E., de la Torre, J. R., Walker, C. B., Waterbury, J. B., and Stahl, D. A. (2005). Isolation of an autotrophic ammonia-oxidizing marine archaeon. Nature 437, 543–546.
Lage, M. D., Reed, H. E., Weihe, C., Crain, C. M., and Martiny, J. B. H. (2010). Nitrogen and phosphorus enrichment alter the composition of ammonia-oxidizing bacteria in salt marsh sediments. ISME J. 4, 933–944.
Ludwig, W., Strunk, O., Westram, R., Richter, L., Meier, H., Yadhukumar, et al. (2004). ARB: a software environment for sequence data. Nucleic Acids Res. 32, 1363–1371.
McCune, B., and Mefford, M. J. (1999). PC-ORD: Multivariate Analysis of Ecological Data. Gleneden Beach, OR: MjM Software.
Meany, R. A., Valiela, I., and Teal, J. M. (1976). Growth, abundance, and distribution of larval tabanids in experimentally fertilized plots on a Massachusetts salt marsh. J. Appl. Ecol. 13, 323–332.
Moin, N. S., Nelson, K. A., Bush, A., and Bernhard, A. E. (2009). Distribution and diversity of archaeal and bacterial ammonia-oxidizers in salt marsh sediment. Appl. Environ. Microbiol. 75, 7461–7468.
Mosier, A. C., and Francis, C. A. (2008). Relative abundance and diversity of ammonia-oxidizing archaea and bacteria in the San Francisco Bay estuary. Environ. Microbiol. 10, 3002–3016.
Nicolaisen, M. H., and Ramsing, N. B. (2002). Denaturing gradient gel electrophoresis (DGGE) approached to study the diversity of ammonia-oxidizing bacteria. J. Microbiol. Methods 50, 189–203.
Pester, M., Rattei, T., Flechl, S., Gröngröft, A., Richter, A., Overmann, J., et al. (2012). AmoA-based consensus phylogeny of ammonia-oxidizing archaea and deep sequencing of amoA genes from soils of four different geographic regions. Environ. Microbiol. 14, 525–539.
Rotthauwe, J., Witzel, K., and Liesack, W. (1997). The ammonia monooxygenase structural gene amoA as a functional marker: molecular fine-scale analysis of natural ammonia-oxidizing populations. Appl. Environ. Microbiol. 63, 4704–4712.
Sahan, E., and Muyzer, G. (2008). Diversity and spatio-temporal distribution of ammonia-oxidizing archaea and bacteria in sediments of the Westerschelde estuary. FEMS Microbiol. Ecol. 64, 175–186.
Santoro, A. E., Francis, C. A., De Sieyes, N. R., and Boehm, A. B. (2008). Shifts in the relative abundance of ammonia-oxidizing bacteria and archaea across physicochemical gradients in a subterranean estuary. Environ. Microbiol. 10, 1068–1079.
Sarda, R., Valiela, I., and Foreman, K. (1996). Decadal shifts in a salt marsh macroinfaunal community in response to sustained long-term experimental nutrient enrichment. J. Exp. Mar. Biol. Ecol. 205, 63–81.
Schloss, P. D., Westcott, S. L., Ryabin, T., Hall, J. R., Hartmann, M., Hollister, E. B., et al. (2009). Introducing mothur: open-source, platform-independent, community-supported software for describing and comparing microbial communities. Appl. Environ. Microbiol. 75, 7537–7541.
Shen, X. Y., Zhang, L. M., Shen, J. P., Li, L. H., Yuan, C. L., and He, J. Z. (2011). Nitrogen loading levels affect abundance and composition of soil ammonia oxidizing prokaryotes in semiarid temperate grassland. J. Soils Sediments 11, 1243–1252.
Treusch, A. H., Leininger, S., Kietzin, A., Schuster, S. C., Klenk, H. P., and Schleper, C. (2005). Novel genes for nitrite reductase and Amo-related proteins indicate a role of uncultivated mesophilic crenarchaeota in nitrogen cycling. Environ. Microbiol. 7, 1985–1995.
Valiela, I., and Teal, J. M. (1974). “Nutrient limitation in salt marsh vegetation,” in Ecology of Halophytes, eds R. J. Reimold and W. H. Queen (New York: Academic Press), 547–563.
Valiela, I., Teal, J. M., and Persson, N. Y. (1976). Production and dynamics of experimentally enriched salt marsh vegetation: belowground biomass. Limnol. Oceanogr. 21, 245–252.
Valiela, I., Teal, J. M., and Sass, W. (1973). Nutrient retention in salt marsh plots experimentally fertilized with sewage sludge. Estuar. Coast. Mar. Sci. 1, 261–269.
Valiela, I., Teal, J. M., and Sass, W. J. (1975). Production and dynamics of salt marsh vegetation and the effects of experimental treatment with sewage sludge. J. Appl. Ecol. 12, 973–981.
Van Raalte, C. D., Valiela, I., Carpenter, E. J., and Teal, J. M. (1974). Inhibition of nitrogen fixation in salt marshes measured by acetylene reduction. Estuar. Coast. Mar. Sci. 2, 301–305.
Van Raalte, C. D., Valiela, I., and Teal, J. M. (1976). The effect of fertilization on the species composition of salt marsh diatoms. Water Res. 10, 1–4.
Verhamme, D. T., Prosser, J. I., and Nicol, G. W. (2011). Ammonia concentration determines differential growth of ammonia-oxidising archaea and bacteria in soil microcosms. ISME J. 5, 1067–1071.
Ward, B. B., Eveillard, D., Kirshtein, J. D., Nelson, J. D., Voytek, M. A., and Jackson, G. A. (2007). Ammonia-oxidizing bacterial community composition in estuarine and oceanic environments assessed using a functional gene microarray. Environ. Microbiol. 9, 2522–2538.
Keywords: amoA, TRFLP, Great Sippewissett Marsh, fertilization, salt marsh
Citation: Peng X, Yando E, Hildebrand E, Dwyer C, Kearney A, Waciega A, Valiela I and Bernhard AE (2013) Differential responses of ammonia-oxidizing archaea and bacteria to long-term fertilization in a New England salt marsh. Front. Microbio. 3:445. doi: 10.3389/fmicb.2012.00445
Received: 10 August 2012; Accepted: 31 December 2012;
Published online: 22 January 2013.
Edited by:
Chris Francis, Stanford University, USAReviewed by:
James T. Hollibaugh, University of Georgia, USAGraeme W. Nicol, University of Aberdeen, UK
Copyright: © 2013 Peng, Yando, Hildebrand, Dwyer, Kearney, Waciega, Valiela and Bernhard. This is an open-access article distributed under the terms of the Creative Commons Attribution License, which permits use, distribution and reproduction in other forums, provided the original authors and source are credited and subject to any copyright notices concerning any third-party graphics etc.
*Correspondence: Anne E. Bernhard, Department of Biology, Connecticut College, 270 Mohegan Avenue, Box 5327, New London, CT 06320, USA. e-mail:YWViZXJAY29ubmNvbGwuZWR1