- 1Institute of Microbiology, Federal University of Rio de Janeiro, Rio de Janeiro, Brazil
- 2Institute of Clinical and Experimental Immunology of Rosario, CONICET, National University of Rosario, Rosario, Argentina
- 3Faculty of Medical Sciences, National University of Rosario, Rosario, Argentina
Chagas disease is a serious illness caused by the protozoan parasite Trypanosoma cruzi. Nearly 30% of chronically infected people develop cardiac, digestive, or mixed alterations, suggesting a broad range of host-parasite interactions that finally impact upon chronic disease outcome. The ability of T. cruzi to persist and cause pathology seems to depend on diverse factors like T. cruzi strains, the infective load and the route of infection, presence of virulence factors, the parasite capacity to avoid protective immune response, the strength and type of host defense mechanisms and the genetic background of the host. The host-parasite interaction is subject to a constant neuro-endocrine regulation that is thought to influence the adaptive immune system, and as the infection proceeds it can lead to a broad range of outcomes, ranging from pathogen elimination to its continued persistence in the host. In this context, T. cruzi evasion strategies and host defense mechanisms can be envisioned as two sides of the same coin, influencing parasite persistence and different outcomes observed in Chagas disease. Understanding how T. cruzi evade host's innate and adaptive immune response will provide important clues to better dissect mechanisms underlying the pathophysiology of Chagas disease.
Introduction
Trypanosoma cruzi is a protozoan parasite that causes Chagas disease (WHO, 2015). Nearly 30% of chronically infected people develop cardiomyopathy, megacolon, and megaesophagus or a mixed of these alterations, suggesting a broad range of host-parasite interactions that finally impact upon chronic disease outcome (Rassi et al., 2010). Different and not mutually exclusive hypotheses have been considered for the pathogenesis of chronic Chagas disease, including autoimmunity by molecular mimicry, microvascular (Ramos and Rossi, 1999), and autonomic dysfunction (Dávila et al., 2004), and tissue damage by parasite persistence (Gironès et al., 2005; Gutierrez et al., 2009; Cunha-Neto et al., 2011). The parasite persistence hypothesis predicts a chronic inflammatory reactivity as result of a failure in parasite burden control, thus promoting the development of disease pathology (Tarleton, 2001). In addition, a subpatent parasite-induced cell lysis as consequence of amastigote differentiation into trypomastigotes (Bonney and Engman, 2008) might fuel inflammation. The presence of parasites (Añez et al., 1999; Buckner et al., 1999) or their products, such as DNA, in blood and myocardium of chronic infected hosts is well documented (Añez et al., 1999; Zhang and Tarleton, 1999; Salomone et al., 2000; Elias et al., 2003). T. cruzi reactivation in HIV co-infected, transplanted or immunocompromised chronic chagasic patients provides convincing evidence of parasite persistence (Tarleton, 2001; Andrade et al., 2014), reinforcing the view that disease pathology and its severity are directly related to T. cruzi presence within the affected tissue (Tarleton, 2001). In this review, we examined the complexity of cellular, molecular and physiologic factors involved in T. cruzi evasion and persistence in the light of current data.
Parasite Evasion Involve Direct Host Immune Regulation and Latency Establishment
T. cruzi has a complex biological cycle involving mammals and insect vectors. The strategies that T. cruzi employs to guarantee its long-term survival within mammalian hosts include evasion from phagolysosome, expression of virulence factors, direct immunomodulation and the establishment of latency sites (Figure 1). Trypomastigotes can invade nucleated cells through different mechanisms depending on whether the target cell is phagocytic or nonphagocytic (Figure 2A; Romano et al., 2012). Macrophages are the most important innate effector cells in the fight against T. cruzi, but when subverted in the infection they can be also exploited by the parasite as its primary niche, thus avoiding cell-mediated immunity. Protective classically activated (M1) macrophages are activated by IFN-γ, increasing the expression of nitric oxide synthase (iNOS) and nitric oxide (NO) production favoring the parasite killing. In contrast, parasite clearance is prevented when macrophages acquire an alternatively activated (M2) phenotype, with reduced NO production thus increasing the parasite persistence (Sizirensen et al., 1994; Desjardins and Descoteaux, 1997; Paulnock and Coller, 2001; Stempin et al., 2002; Martinez and Gordon, 2014). Unlike other parasites that prevent phagolysosome maturation (David Sibley, 2011), T. cruzi evades macrophage microbicidal activity by escaping from phagolysosome to cytoplasm, an event that is mediated by the cytolitic activity of parasite's C9 cross-reactive protein (Tc-Tox; Andrews et al., 1990; Bogdan and Röllinghoff, 1999). Once inside the cytoplasm, T. cruzi parasites promote STAT1 dephosphorylation, thus interfering with the transcription of IL-12 and TNF-α (De Diego et al., 1997) that ultimately abrogate IFN-γ-mediated microbicidal responses (Gazzinelli et al., 1992; Stahl et al., 2014). In addition, parasite-derived proteases shutdown IL-12 expression by interrupting the NF-κB signaling pathway (Doyle et al., 2011). Furthermore, T. cruzi stimulate the secretion of anti-inflammatory cytokines such as IL-10 and TGF-β that impair the development of protective immune responses hence favoring the spread of infection and parasite persistence in the host (Silva et al., 1991; Hunter et al., 1997; Freire-de-Lima et al., 2000). T. cruzi can also disrupt the classical and alternative complement pathways: parasite CRP and T-DAF proteins bind to C3b and C4b fragments, inhibiting the assembly of C3 and C5 convertase on the parasite membrane (Joiner et al., 1986; Norris et al., 1991; Tambourgi et al., 1993; Zambrano-Villa et al., 2002).
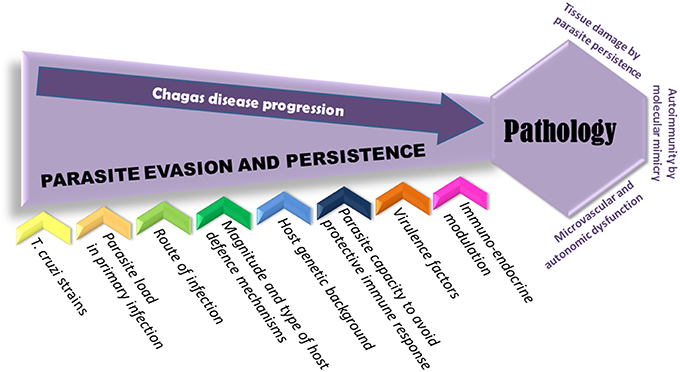
Figure 1. Pathogenesis of Trypanosoma cruzi infection. Several hypotheses have been considered for the pathogenesis of chronic Chagas disease, comprising tissue damage by parasite persistence, autoimmunity, microvascular injury, and autonomic dysfunction. Since diverse factors are involved in parasite evasion and persistence, most of all may influence the infection outcome and the development of pathology in almost 30% of infected individuals. The ability of T. cruzi to evade immune system seems to depend on diverse factors like T. cruzi strains, the infective load and the route of infection and the presence of virulence factors; but also can be determined by the type and strength of host defense mechanisms and the genetic background of the host.
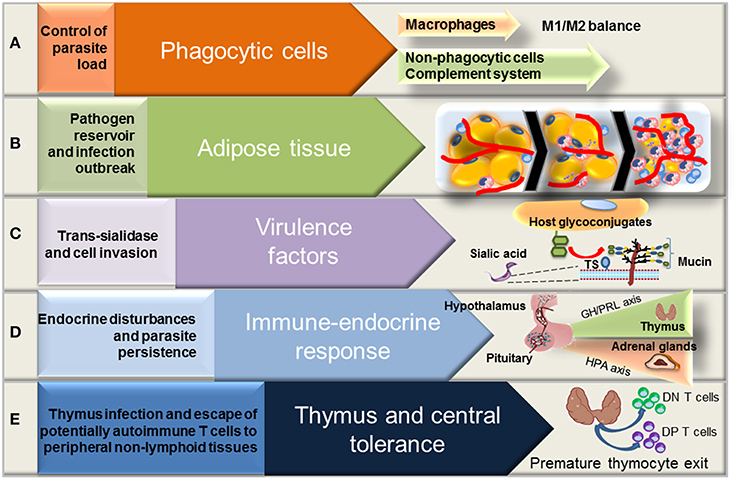
Figure 2. Aspects of Trypanosoma cruzi evasion and persistence in the vertebrate host. T. cruzi parasites develop different strategies to evade the host defenses and establish a persistent infection. T. cruzi parasites evade the host innate immune responses associated with macrophage and complement system (A). The trans-sialidase (TS), a T. cruzi-derived virulence factor, can also overcome the host resistance responses to optimize the invasion and parasite persistence in chronic infection (B). The development of anti-parasite immune response is coupled with the activation of neuroendocrine axes that may affect the course of disease (C). Adipose tissue can be considered as a parasite reservoir and may contribute to the establishment of persistent infections, playing a major role in T. cruzi burst during immunosuppression periods (D). The recognition of T. cruzi-derived antigens in the thymus may restrict the central tolerance to parasite infection, while the release of immature and potentially autoimmune T cells to the peripheral non-lymphoid tissues may be related with disease pathology in the chronic phase (E).
The acute phase of infection is marked by a transient state of immunosuppression (Liew et al., 1987; Kierszenbaum et al., 1999, 2002; Van Overtvelt et al., 2002; Alcaide and Fresno, 2004; Gutierrez et al., 2009; Padilla et al., 2009; DosReis, 2011; Oladiran and Belosevic, 2012; Pinazo et al., 2013) involving, among other things, a strong polyclonal B cell stimulation which restricts the development of antigen-specific lymphocytes, promoting apoptosis and cell cycle arrest (Ortiz-Ortiz et al., 1980; Maleckar and Kierszenbaum, 1983; Zuñiga et al., 2000). In fact T. cruzi provides a good example of such immunosuppression strategy: T cells from infected mice respond poorly to mitogens (Kierszenbaum et al., 1999, 2002; Alcaide and Fresno, 2004) and they also undergo enhanced apoptosis upon stimulation of T cell receptor (TCR), increasing the unresponsiveness of host immunity (Abrahamsohn and Coffman, 1995; Martins et al., 1998; Nunes et al., 1998). Studies have supported that IL-2 deficiency is one of the hallmarks of the disease-induced T cell immunosuppression (Abrahamsohn and Coffman, 1995). The T cell unresponsiveness in Chagas disease is also the result of a direct downregulation of IL-2 receptor by the parasite glycoprotein AGC10 (Kierszenbaum et al., 1999). Recently, a novel immunosuppressive mechanism was described, which implies the IFN-γ-dependent NO secretion by immature myeloid cells (Goñi et al., 2002).
Adipose Tissue Acts as a Reservoir of Trypanosoma cruzi
Another adaptive strategy displayed by T. cruzi parasites to optimize its persistence in the host consists in targeting tissues with particular characteristics. Some studies have shown that adipose tissue (AT) might serve as a reservoir where parasite could persist in a latent state to avoid the host-defense mechanisms, acting as a possible site of reactivation, similarly to that observed for other intracellular pathogens (Figure 2B; Neyrolles et al., 2006; Bechah et al., 2010, 2014). Adipocytes could harbor a significant number of parasites even in the chronic phase of infection (Combs et al., 2005). Recently, more robust evidence that AT can act as a reservoir of T. cruzi have arisen from experiments in which infected mice were subsequently treated with an anti-parasitic drug and afterwards immunosuppressed. Intriguingly, in a significant number of animals, the AT was the major site of parasite recrudescence (Fortes Francisco et al., 2015). Moreover, studies carried out in patients with chronic chagasic cardiomyopathy have revealed the presence of parasite-derived DNA in AT (Ferreira et al., 2011). In this sense, AT may be a reservoir from which reactivation of infection may occur, especially during periods of immunosuppression, as observed in chagasic patients co-infected with HIV, transplanted or undergoing immunosuppressive therapies (Almeida et al., 1996; Sartori et al., 1998; Campos et al., 2008; Cordova et al., 2008; Pinazo et al., 2013). Moreover, in HIV co-infected chagasic patients, periods of lipoatrophy may result in the release of parasites into the circulation (Ferreira et al., 2011). It remains to be investigated why T. cruzi persists in the AT. Possible reasons could be the special metabolic conditions that T. cruzi finds inside the adipocyte and the slow turnover of these cells. After infection, there is an influx of inflammatory cells to AT, accompanied by an in situ upregulation of both TNF-α and IL-6, concomitantly to a diminution of adipocytokine levels (Desjardins and Descoteaux, 1997; Chandrasekar et al., 2000; Manarin et al., 2013). Moreover, some studies suggested that metabolic alterations induced by T. cruzi persistence in AT may increase the risk of diabetes, metabolic syndrome and cardiovascular disease (Chandrasekar et al., 2000; Nagajyothi et al., 2009; Manarin et al., 2013).
Trypanosoma cruzi Virulence Factors Overcome the Host Resistance Response to Establish Persistent Infections
The ability of T. cruzi parasites to persist and cause pathology partially depends on factors such as the parasite strain, the genetic background of the host (Andrade et al., 2002; Savino et al., 2007) and the route of infection (Barreto-de-Albuquerque et al., 2015). T. cruzi species display a broad range of biological, biochemical, molecular and genetic characteristics, being grouped in six discrete typing units (DTUs; Macedo et al., 2004; Zingales et al., 2009). The parasite immune modulatory effects seems to be strain-dependent, a feature that might influence parasite-host interactions (Lauria-Pires, 1996; Briones et al., 1999). Different parasite strains coexist dynamically in natural reservoirs and combinations of them have been found in triatomine bugs from domestic and peridomestic areas (Noireau et al., 2009), as well as in infected patients (Cura et al., 2015; Monje-Rumi et al., 2015).
The damping of host immune response during the acute phase of Chagas disease is partially caused by T. cruzi-derived virulence factors (Figure 2C; DosReis et al., 2005; Gutierrez et al., 2009; Nagajyothi et al., 2012). One of the hallmarks of parasite's cell membrane composition is the presence of mucin-like molecules presenting sialic acid residues attached to their terminal β-galactosyl residues. These residues are transferred from host glycoconjugates by the action of parasite trans-sialidase (Schenkman et al., 1991, 1994; Previato et al., 1995; Eugenia Giorgi and De Lederkremer, 2011). Parasite mucins are encoded by more than 800 genes comprising ~1% of the parasite genome, represented as O-glycosylated Thr/Ser/Pro-rich glycoproteins (Di Noia et al., 1995; Buscaglia et al., 2006; Mendonça-Previato et al., 2013). The T. cruzi-derived mucin molecules are determinant in the host-parasite interplay, since they mediate processes related to invasion of the vertebrate cells as well as subvert the host immune system. The sialylated forms of mucin-like molecules protect the parasite antigenic determinants from host humoral responses, avoiding the humoral attack mediated by anti-galactosyl antibodies and complement factor B (Kipnis et al., 1981; Joiner et al., 1986; Gazzinelli et al., 1991; Pereira-Chioccola et al., 2000). Moreover, it has been shown that once sialylated, mucin-like molecules are able to impair the host dendritic cell function through inhibition of the IL-12 expression (Erdmann et al., 2009), possibly at transcriptional level as described for IL-2 gene (Kierszenbaum et al., 1999, 2002). Furthermore, the parasite sialoglycoproteins are able to inhibit tyrosine phosphorylation of the adapter protein SLP-76 and tyrosine kinase ZAP-70, both involved in the early events of T cell activation (Alcaide and Fresno, 2004).
Recently, it has been shown that in vivo exposure to T. cruzi mucins enhances the host susceptibility, as seen by the increased parasitemia and heart tissue damage. These effects were associated with a reduction in Th1 and Th2 cytokine production, together with decreased levels in the frequency of IFN-γ producing CD4+ T cells in the spleen of mice treated with parasite mucins in comparison with untreated controls (Nunes et al., 2013). Interestingly, it has been shown that the binding of acid-binding Ig-like lectin Siglec-E (CD33) by T. cruzi mucins inhibits the mitogenic responses of CD4+ T cells. Studies conducted to address the molecular mechanisms underlying these effects have shown that the impairment of TCR/CD3-mediated activation of CD4+ T cells by T. cruzi-derived mucins was correlated with induction of G1-phase cell cycle arrest. Importantly, it has been demonstrated that interactions of the terminal sialyl residues of T. cruzi mucins with CD4+ T cells led to the induction of the cell cycle regulator p27/Kip1 responsible to block the transition from G1 to S phase of mytosis, thus preventing the proliferative responses (Nunes et al., 2013).
Interestingly, the limited T cell responses observed in T. cruzi infection contrast with the large polyclonal expansion of B lymphocytes seen in the acute phase (Ortiz-Ortiz et al., 1980), as demonstrated by the increased frequency of IgG2a and IgG2b secreting B cells in peripheral lymphoid organs of infected mice. This phenomenon results in high frequency of nonspecific antibodies with low affinity for T. cruzi antigens (Ouaissi et al., 2001), some of them cross-reacting with heart and neural autoantigens (Acosta and Santos-Buch, 1985; Kierszenbaum, 1999; Engman and Leon, 2002). The auto-reactive B cell responses are thought to play secondary roles in the pathogenesis of Chagas disease. The extensive polyclonal expansion of the B cells could partly affect lymphoid compartments by increasing the competition for activation and survival signals needed to promote the generation of antigen-specific lymphocyte responses against T. cruzi (Freitas and Rocha, 2000; Montaudouin et al., 2013).
In addition, parasite-derived glycol-inositol-phospholipids (GIPLs), which are components of the dense glycolipid layer covering the parasite cell surface, also promote alterations in the B cell compartment. These molecules work as TLR4 agonists, mediating pro-inflammatory effects (Oliveira et al., 2004). Another virulence factor encoded by T. cruzi that target the B cell compartment is the proline racemase, which participates in arginine and proline metabolism, acting as a potent mitogen for B cells. Shortly, T. cruzi -derived virulence factors are active players in the subversion of the host immune system and are determinant for the establishment of chronic persistent infection (Reina-San-Martín et al., 2000; Chamond et al., 2003).
The Immune-Endocrine Imbalance is a Key Determinant of Parasite Persistence
Immune and neuro-endocrine systems are integrated through a complex network of mediators, involving cytokines, adipocytokines, hormones, and neuropeptides that collectively act to maintain homeostasis (Besedovsky and del Rey, 1996; Fantuzzi, 2005). However, when vertebrate hosts are challenged by infectious pathogens, acute and short-term stress signals are delivered by this network to initiate and build global host mechanisms of defense (Besedovsky and del Rey, 1996). In parallel, pathogens could interfere with this neuro-endocrine response at several levels. Thus, a race between pathogen-mediated evasion mechanisms and host immune response will determine whether the microorganisms will be rapidly eliminated or persist in the host (Figure 2D). In mice, the anti-T. cruzi immune response is associated with the activation of neuro-endocrine circuitries, mainly the hypothalamic-pituitary-adrenocortical (HPA) axis (Roggero et al., 2006; Corrêa-De-Santana et al., 2006b). In this scenario, pro-inflammatory cytokines released during infection activate the HPA axis, leading to production of glucocorticoids (GC), crucial for host survival. Evidently, the neuro-endocrine circutries initiates an anti-inflammatory response attempting to minimize the infection-induced collateral tissue damage. However its immunoregulatory effect ultimately favor the parasitism and establishment of persistent infection. Comparative studies between susceptible and resistant experimental mice models have indicated that the course of T. cruzi infection strongly depends on the appropriate timing and magnitude of the immune-endocrine response (Roggero et al., 2006). Susceptible animals succumb as consequence of increased inflammatory response poorly counteracted by the HPA axis, while resistant animals develop a more balanced immune-endocrine response that lead to the establishment of a chronic infection and mild pathology. Moreover, when GC signaling was abrogated by adrenalectomy or treatment with GC receptor antagonist RU486, the severity of infection increased dramatically as a result of an augmented inflammation-based immunopathology (Roggero et al., 2006; Pérez et al., 2007). These findings indicate that a delicate balance between the immune and endocrine systems play a role in the establishment of chronic infections. Additionally, the activation of HPA axis leads to secretion of other adrenal steroids, such as dehydroepiandrosterone (DHEA). In this regard, the increased vulnerability of T. cruzi infected young animals was associated with a high corticosterone/DHEA-sulfate ratio as compared to the adult counterparts (Pérez et al., 2011). Similarly, patients with severe chronic chagasic myocarditis also revealed a disruption in the activation of HPA axis as characterized by decreased concentrations of DHEA-sulfate and unbalanced cortisol/DHEA-sulfate ratio in comparison to asymptomatic or healthy individuals (Pérez et al., 2011). Overall, these findings reinforce the view that during T. cruzi human infection there are endocrine disturbances that might favor parasite persistence, thus influencing the disease pathology.
Moreover, pro-inflammatory cytokines associated to T. cruzi infection such as TNF-α, IL-6, or IL-1β could affect the release of hypothalamic, pituitary or adrenal hormones by their direct action on the endocrine glands (Kanczkowski et al., 2013, 2015; Hueston and Deak, 2014). During experimental T. cruzi infection, TNF-α has been implicated in the HPA activation at central level (Roggero et al., 2006; Pérez et al., 2007), although inhibitory actions at adrenal level has been also observed (Villar et al., 2013). Acutely infected TNF-R1 knock-out mice showed an enhanced transcription of adrenal steroidogenic proteins StAR, CYP11A1, CYP11B1 and 11β-HSD1 as compared to wild type mice, suggesting that GC secretion can be down regulated by TNF-α in situ, independently of the signaling pathway induced by adrenocorticotropic hormone (ACTH; Corrêa-De-Santana et al., 2006a; Villar et al., 2013). Since both parasites and their antigens had been detected within adrenal glands (Corrêa-De-Santana et al., 2006a; Villar et al., 2013), their presence might induce in situ the release of TNF-α, with the consequent modulation of GC secretion. In addition, IL-6 has also been associated with enhanced activity of the HPA axis during experimental T. cruzi infection. In this regard, supernatants of adenopituitary cell cultures challenged with the parasite contained more IL-6, while infected mice also showed augmented circulating levels of this cytokine systemically (Corrêa-De-Santana et al., 2006a). The activation of hypothalamus-pituitary unit also results in the release of both growth hormone (GH) and prolactin (PRL), which are capable of improving the immune response, counteracting the GC-driven immunosuppression. T. cruzi infection appears to directly modulate the secretion of both hormones, since in vitro infection of mammosomatotrophic cell line diminished GH and PRL secretion, similarly to observed in the pituitary glands of infected mice (Corrêa-De-Santana et al., 2009). The modulation of GH and PRL secretion by diminishing the Pit-1 gene expression, a major transcription factor for both hormone genes (Corrêa-De-Santana et al., 2009). Moreover, the downregulation of these hormones during the infection might be also related to the presence of parasites or their antigens in the glands, favoring T cell and macrophage infiltration, vascular stasis along with increased depots of extracellular matrix proteins (Corrêa-De-Santana et al., 2006b, 2009). The downmodulation of GH and PRL hormones is also observed in African trypanosomiasis and may illustrates a common modulatory mechanism (Radomski et al., 1994, 1996). Moreover, there is a bulk of evidence indicating that sex steroid hormones might influence the development and course of diverse parasitic infections (Romano et al., 2015). Particularly, it has been shown that T. cruzi parasites have the capacity to metabolize steroid hormones (Vacchina et al., 2008), suggesting a possible role of this mechanism in the host-parasite interplay.
Trypanosoma cruzi Infection may Influence Central Tolerance
Several alterations in the thymic environment occur in infectious diseases (Watson et al., 1983, 1984; Savino et al., 1986; Leite de Moraes et al., 1991; Godfraind et al., 1995; Brito et al., 2003; Chen et al., 2005; Seixas and Ostler, 2005; Suzuki et al., 2005). The most evident alteration is the atrophy of the thymus due to the apoptotic death of differentiating thymocytes (Savino, 2006). In T. cruzi infected mice present a marked imbalance between intrathymic and systemic stress-related endocrine circuits, where the rise of intrathymic levels of GC affect the viability of double positive CD4+CD8+ (DP) cells, double-negative CD4−CD8− (DN) and simple positive (SP) thymocytes (Roggero et al., 2006; Pérez et al., 2007). The induction of GC-driven apoptosis of DP cells is clearly associated with the activation of caspases 8 and 9 (Farias-de-Oliveira et al., 2013). In mice, the thymic atrophy is also influenced by the premature export of immature DP and DN thymocytes to the periphery, exhibiting a pro-inflammatory profile (Figure 2E; Leite-de-Moraes et al., 1992; De Meis et al., 2009; Morrot et al., 2011). Interestingly, increased numbers of circulating undifferentiated DP T lymphocytes was observed in patients with cardiac forms of chronic Chagas disease (Lepletier et al., 2014). Studies have identified a potential role for sphingosine-1-phosphate receptor-1 in this abnormal exit of undifferentiated thymocytes to the periphery in Chagas disease (Lepletier et al., 2014).
The T. cruzi infected thymus undergoing atrophy is still able to carry out negative selection, remaining important considerations in the context of host-pathogen interactions (Mendes-da-Cruz et al., 2003; Morrot et al., 2011). T. cruzi parasites also colonize the thymus (Savino et al., 1989), so their antigens may be presented to recirculating parasite-specific memory T cells migrating from the periphery to the thymic microenvironment. Alternatively, the parasite colonization of thymus could lead to the generation of T. cruzi-specific Tregs with high affinity TCR (Pacholczyk and Kern, 2008), thus promoting the host tolerance to persistent infection. Interestingly, it has been observed that chagasic patients in the indeterminate phase shown high frequencies of circulating Tregs as compared to chronic cardiac ones (De Araujo et al., 2011), suggesting a beneficial role of Tregs in suppressing the pathology associated to disease progression. In contrast, in experimental lethal models of Chagas disease with highly Th1-polarized inflammatory responses, the expansion of Tregs is clearly restricted (González et al., 2015).
Concluding Remarks
Recent studies suggest that the immuno-endocrine host response may favor T. cruzi chronic persistence. Future studies attempting to understand how T. cruzi evade host immune response or the extent by which parasite persistence might be favored by immune-neuro-endocrine regulation will provide important clues to better dissect mechanisms underlying the pathophysiology of Chagas disease.
Author Contributions
AM, FBG, SV and AP wrote the paper. All authors read and approved the final version of the manuscript.
Conflict of Interest Statement
The authors declare that the research was conducted in the absence of any commercial or financial relationships that could be construed as a potential conflict of interest.
Acknowledgments
We thank Dr. Oscar Bottasso for their advices critical reading of text. This work was supported by grants from CNPq and FAPERJ (Brazil); ANPCyT (PICT 2013-1892), CONICET (PIP 0641), and ASACTeI (Argentine). AM is recipient of fellowship from CNPq and FG is recipient of fellowship from CONICET.
References
Abrahamsohn, I. A., and Coffman, R. L. (1995). Cytokine and nitric oxide regulation of the immunosuppression in Trypanosoma cruzi infection. J. Immunol. 155, 3955–3963.
Acosta, A. M., and Santos-Buch, C. A. (1985). Autoimmune myocarditis induced by Trypanosoma cruzi. Circulation 71, 1255–1261. doi: 10.1161/01.CIR.71.6.1255
Alcaide, P., and Fresno, M. (2004). The Trypanosoma cruzi membrane mucin AgC10 inhibits T cell activation and IL-2 transcription through L-selectin. Int. Immunol. 16, 1365–1375. doi: 10.1093/intimm/dxh138
Almeida, D. R., Carvalho, A. C., Branco, J. N., Pereira, A. P., Correa, L., Vianna, P. V., et al. (1996). Chagas' disease reactivation after heart transplantation: efficacy of allopurinol treatment. J. Heart Lung Transplant. 15, 988–992.
Andrade, D. V., Gollob, K. J., and Dutra, W. O. (2014). Acute chagas disease: new global challenges for an old neglected disease. PLoS Negl. Trop. Dis. 8:e3010. doi: 10.1371/journal.pntd.0003010
Andrade, L. O., Machado, C. R. S., Chiari, E., Pena, S. D. J., and Macedo, A. M. (2002). Trypanosoma cruzi: role of host genetic background in the differential tissue distribution of parasite clonal populations. Exp. Parasitol. 100, 269–275. doi: 10.1016/S0014-4894(02)00024-3
Andrews, N. W., Abrams, C. K., Slatin, S. L., and Griffiths, G. (1990). A T. cruzi-secreted protein immunologically related to the complement component C9: evidence for membrane pore-forming activity at low pH. Cell 61, 1277–1287. doi: 10.1016/0092-8674(90)90692-8
Añez, N., Carrasco, H., Parada, H., Crisante, G., Rojas, A., Fuenmayor, C., et al. (1999). Myocardial parasite persistence in chronic chagasic patients. Am. J. Trop. Med. Hyg. 60, 726–732.
Barreto-de-Albuquerque, J., Silva-dos-Santos, D., Pérez, A. R., Berbert, L. R., Santana-van-Vliet, E., De Farias-de-Oliveira, D. A., et al. (2015). Trypanosoma cruzi Infection through the oral route promotes a severe infection in mice: new disease form from an old infection? PLoS Negl. Trop. Dis. 9:e0003849. doi: 10.1371/journal.pntd.0003849
Bechah, Y., Paddock, C. D., Capo, C., Mege, J. L., and Raoult, D. (2010). Adipose tissue serves as a reservoir for recrudescent Rickettsia prowazekii infection in a mouse model. PLoS ONE 5:e8547. doi: 10.1371/journal.pone.0008547
Bechah, Y., Verneau, J., Amara, A., Ben, B.arry, A. O., Lépolard, C., et al. (2014). Persistence of Coxiella burnetii, the agent of Q fever, in murine adipose tissue. PLoS ONE 9:e97503. doi: 10.1371/journal.pone.0097503
Besedovsky, H. O., and del Rey, A. (1996). Immune-neuro-endocrine interations: facts and hypotheses. Endocr. Rev. 17, 64–102. doi: 10.1210/edrv-17-1-64
Bogdan, C., and Röllinghoff, M. (1999). How do protozoan parasites survive inside macrophages? Parasitol. Today 15, 22–28. doi: 10.1016/S0169-4758(98)01362-3
Bonney, K. M., and Engman, D. M. (2008). Chagas heart disease pathogenesis: one mechanism or many? Curr. Mol. Med. 8, 510–518. doi: 10.2174/156652408785748004
Briones, M. R. S., Souto, R. P., Stolf, B. S., and Zingales, B. (1999). The evolution of two Trypanosoma cruzi subgroups inferred from rRNA genes can be correlated with the interchange of American mammalian faunas in the Cenozoic and has implications to pathogenicity and host specificity. Mol. Biochem. Parasitol. 104, 219–232. doi: 10.1016/S0166-6851(99)00155-3
Brito, V. N., Souto, P. C. S., Cruz-Höfling, M. A., Ricci, L. C., and Verinaud, L. (2003). Thymus invasion and atrophy induced by Paracoccidioides brasiliensis in BALB/c mice. Med. Mycol. 41, 83–87. doi: 10.1080/714043902
Buckner, F. S., Wilson, A. J., and Van Voorhis, W. C. (1999). Detection of live Trypanosoma cruzi in tissues of Infected mice by using histochemical stain for β-galactosidase. Infect Immun. 67, 403–409.
Buscaglia, C. A., Campo, V. A., Frasch, A. C. C., and Di Noia, J. M. (2006). Trypanosoma cruzi surface mucins: host-dependent coat diversity. Nat. Rev. Microbiol. 4, 229–236. doi: 10.1038/nrmicro1351
Campos, S. V., Strabelli, T. M. V., Amato Neto, V., Silva, C. P., Bacal, F., Bocchi, E. A., et al. (2008). Risk factors for chagas' disease reactivation after heart transplantation. J. Hear Lung Transplant. 27, 597–602. doi: 10.1016/j.healun.2008.02.017
Chamond, N., Grégoire, C., Coatnoan, N., Rougeot, C., Freitas-Junior, L. H., Da Silveira, J. F., et al. (2003). Biochemical characterization of proline racemases from the human protozoan parasite Trypanosoma cruzi and definition of putative protein signatures. J. Biol. Chem. 278, 15484–15494. doi: 10.1074/jbc.M210830200
Chandrasekar, B., Melby, P. C., Troyer, D. A., and Freeman, G. L. (2000). Differential regulation of nitric oxide synthase isoforms in experimental acute Chagasic cardiomyopathy. Clin. Exp. Immunol. 121, 112–119. doi: 10.1046/j.1365-2249.2000.01258.x
Chen, W., Kuolee, R., Austin, J. W., Shen, H., Che, Y., and Conlan, J. W. (2005). Low dose aerosol infection of mice with virulent type A Francisella tularensis induces severe thymus atrophy and CD4+CD8+ thymocyte depletion. Microb. Pathog. 39, 189–196. doi: 10.1016/j.micpath.2005.08.005
Combs, T. P., Nagajyothi, M. S., De Almeida, C. J. G., Jelicks, L. A., Schubert, W., Lin, Y., et al. (2005). The adipocyte as an important target cell for Trypanosoma cruzi infection. J. Biol. Chem. 280, 24085–24094. doi: 10.1074/jbc.M412802200
Cordova, E., Boschi, A., Ambrosioni, J., Cudos, C., and Corti, M. (2008). Reactivation of Chagas disease with central nervous system involvement in HIV-infected patients in Argentina, 1992-2007. Int. J. Infect. Dis. 12, 587–592. doi: 10.1016/j.ijid.2007.12.007
Corrêa-De-Santana, E., Paez-Pereda, M., Theodoropoulou, M., Kenji Nihei, O., Gruebler, Y., Bozza, M., et al. (2006a). Hypothalamus-pituitary-adrenal axis during Trypanosoma cruzi acute infection in mice. J. Neuroimmunol. 173, 12–22. doi: 10.1016/j.jneuroim.2005.08.015
Corrêa-De-Santana, E., Paez-Pereda, M., Theodoropoulou, M., Renner, U., Stalla, J., Stalla, G. K., et al. (2009). Modulation of growth hormone and prolactin secretion in Trypanosoma cruzi -infected mammosomatotrophic cells. Neuroimmunomodulation 16, 208–212. doi: 10.1159/000205513
Corrêa-De-Santana, E., Pinto-Mariz, F., and Savino, W. (2006b). Immunoneuroendocrine interactions in Chagas disease. Ann. N.Y. Acad. Sci. 1088, 274–283. doi: 10.1196/annals.1366.005
Cunha-Neto, E., Teixeira, P. C., Nogueira, L. G., and Kalil, J. (2011). Autoimmunity. Adv. Parasitol. 76, 129–152. doi: 10.1016/B978-0-12-385895-5.00006-2
Cura, C. I., Duffy, T., Lucero, R. H., Bisio, M., Péneau, J., Jimenez-Coello, M., et al. (2015). Multiplex real-time PCR assay using TaqMan probes for the identification of Trypanosoma cruzi DTUs in biological and clinical samples. PLoS Negl. Trop. Dis. 9:e0003765. doi: 10.1371/journal.pntd.0003765
David Sibley, L. (2011). Invasion and intracellular survival by protozoan parasites. Immunol. Rev. 240, 72–91. doi: 10.1111/j.1600-065X.2010.00990.x
Dávila, D. F., Donis, J. H., Torres, A., and Ferrer, J. A. (2004). A modified and unifying neurogenic hypothesis can explain the natural history of chronic Chagas heart disease. Int. J. Cardiol. 96, 191–195. doi: 10.1016/j.ijcard.2003.06.015
De Araujo, F. F., Vitelli-Avelar, D. M., Teixeira-Carvalho, A., Renato Zuquim Antas, P., Assis Silva Gomes, J., Sathler-Avelar, R., et al. (2011). Regulatory T cells phenotype in different clinical forms of chagas' disease. PLoS Negl. Trop. Dis. 5:e992. doi: 10.1371/journal.pntd.0000992
De Diego, J., Punzón, C., Duarte, M., and Fresno, M. (1997). Alteration of macrophage function by a Trypanosoma cruzi membrane mucin. J. Immunol. 159, 4983–4989.
De Meis, J., Morrot, A., Farias-de-Oliveira, D. A., Villa-Verde, D. M. S., and Savino, W. (2009). Differential regional immune response in Chagas disease. PLoS Negl. Trop. Dis. 3:e417. doi: 10.1371/journal.pntd.0000417
Desjardins, M., and Descoteaux, A. (1997). Inhibition of phagolysosomal biogenesis by the Leishmania lipophosphoglycan. J. Exp. Med. 185, 2061–2068.
Di Noia, J. M., Sanchez, D. O., and Frasch, A. C. C. (1995). The protozoan Trypanosoma cruzi has a family of genes resembling the mucin genes of mammalian cells. J. Biol. Chem. 270, 24146–24149. doi: 10.1074/jbc.270.41.24146
DosReis, G. A. (2011). Evasion of immune responses by Trypanosoma cruzi, the etiological agent of Chagas disease. Braz. J. Med. Biol. Res. 44, 84–90. doi: 10.1590/S0100-879X2011007500005
DosReis, G. A., Freire-De-Lima, C. G., Nunes, M. P., and Lopes, M. F. (2005). The importance of aberrant T-cell responses in Chagas disease. Trends Parasitol. 21, 237–243. doi: 10.1016/j.pt.2005.03.008
Doyle, P. S., Zhou, Y. M., Hsieh, I., Greenbaum, D. C., McKerrow, J. H., and Engel, J. C. (2011). The Trypanosoma cruzi protease cruzain mediates immune evasion. PLoS Pathog. 7:e1002139. doi: 10.1371/journal.ppat.1002139
Elias, F. E., Vigliano, C. A., Laguens, R. P., Levin, M. J., and Berek, C. (2003). Analysis of the presence of Trypanosoma cruzi in the heart tissue of three patients with chronic Chagas' heart disease. Am. J. Trop. Med. Hyg. 68, 242–247.
Engman, D. M., and Leon, J. S. (2002). Pathogenesis of Chagas heart disease: role of autoimmunity. Acta Trop. 81, 123–132. doi: 10.1016/S0001-706X(01)00202-9
Erdmann, H., Steeg, C., Koch-Nolte, F., Fleischer, B., and Jacobs, T. (2009). Sialylated ligands on pathogenic Trypanosoma cruzi interact with Siglec-E (sialic acid-binding Ig-like lectin-E). Cell. Microbiol. 11, 1600–1611. doi: 10.1111/j.1462-5822.2009.01350.x
Eugenia Giorgi, M., and De Lederkremer, R. M. (2011). Trans-sialidase and mucins of Trypanosoma cruzi: an important interplay for the parasite. Carbohydr. Res. 346, 1389–1393. doi: 10.1016/j.carres.2011.04.006
Fantuzzi, G. (2005). Adipose tissue, adipokines, and inflammation. J. Allergy Clin. Immunol. 115, 911–920. doi: 10.1016/j.jaci.2005.02.023
Farias-de-Oliveira, D. A., Villa-Verde, D. M. S., Nunes Panzenhagen, P. H., Silva dos Santos, D., Berbert, L. R., Savino, W., et al. (2013). Caspase-8 and caspase-9 mediate thymocyte apoptosis in Trypanosoma cruzi acutely infected mice. J. Leukoc. Biol. 93, 227–234. doi: 10.1189/jlb.1211589
Ferreira, A. V. M., Segatto, M., Menezes, Z., Macedo, A. M., Gelape, C., de Oliveira Andrade, L., et al. (2011). Evidence for Trypanosoma cruzi in adipose tissue in human chronic Chagas disease. Microbes Infect. 13, 1002–1005. doi: 10.1016/j.micinf.2011.06.002
Fortes Francisco, A., Lewis, M. D., Jayawardhana, S., Taylor, M. C., Chatelain, E., and Kelly, J. M. (2015). The limited ability of posaconazole to cure both acute and chronic Trypanosoma cruzi infections revealed by highly sensitive in vivo imaging. Antimicrob. Agents Chemother. 59, 4653–4661. doi: 10.1128/AAC.00520-15
Freire-de-Lima, C. G., Nascimento, D. O., Soares, M. B., Bozza, P. T., Castro-Faria-Neto, H. C., de Mello, F. G., et al. (2000). Uptake of apoptotic cells drives the growth of a pathogenic trypanosome in macrophages. Nature 403, 199–203. doi: 10.1038/35003208
Freitas, A. A., and Rocha, B. (2000). Population biology of lymphocytes: the flight for survival. Annu. Rev. Immunol. 18, 83–111. doi: 10.1146/annurev.immunol.18.1.83
Gazzinelli, R. T., Oswald, I. P., Hieny, S., James, S. L., and Sher, A. (1992). The microbicidal activity of interferon-gamma-treated macrophages against Trypanosoma cruzi involves an L-arginine-dependent, nitrogen oxide-mediated mechanism inhibitable by interleukin-10 and transforming growth factor-beta. Eur. J. Immunol. 22, 2501–2506. doi: 10.1002/eji.1830221006
Gazzinelli, R. T., Pereira, M. E., Romanha, A., Gazzinelli, G., and Brener, Z. (1991). Direct lysis of Trypanosoma cruzi: a novel effector mechanism of protection mediated by human anti-gal antibodies. Parasite Immunol. 13, 345–356. doi: 10.1111/j.1365-3024.1991.tb00288.x
Gironès, N., Cuervo, H., and Fresno, M. (2005). Trypanosoma cruzi -induced molecular mimicry and Chagas' disease. Curr. Top. Microbiol. Immunol. 296, 89–123. doi: 10.1007/3-540-30791-5_6
Godfraind, C., Holmes, K. V., and Coutelier, J. P. (1995). Thymus involution induced by mouse hepatitis virus A59 in BALB/c mice. J. Virol. 69, 6541–6547.
Goñi, O., Alcaide, P., and Fresno, M. (2002). Immunosuppression during acute Trypanosoma cruzi infection: involvement of Ly6G (Gr1(+))CD11b(+)immature myeloid suppressor cells. Int. Immunol. 14, 1125–1134. doi: 10.1093/intimm/dxf076
González, F. B., Villar, S. R., Fernández, R., Martin, G. H., Pérol, L., Manarin, R., et al. (2015). Immunoendocrine dysbalance during uncontrolled T. cruzi infection is associated with the acquisition of a Th-1-like phenotype. Brain Behav. Immun. 45, 219–232. doi: 10.1016/j.bbi.2014.11.016
Gutierrez, F. R. S., Guedes, P. M. M., Gazzinelli, R. T., and Silva, J. S. (2009). The role of parasite persistence in pathogenesis of Chagas heart disease. Parasite Immunol. 31, 673–685. doi: 10.1111/j.1365-3024.2009.01108.x
Hueston, C. M., and Deak, T. (2014). The inflamed axis: The interaction between stress, hormones, and the expression of inflammatory-related genes within key structures comprising the hypothalamic-pituitary-adrenal axis. Physiol. Behav. 124, 77–91. doi: 10.1016/j.physbeh.2013.10.035
Hunter, C. A., Ellis-Neyes, L. A., Slifer, T., Kanaly, S., Grünig, G., Fort, M., et al. (1997). IL-10 is required to prevent immune hyperactivity during infection with Trypanosoma cruzi. J. Immunol. 158, 3311–3316.
Joiner, K., Sher, A., Gaither, T., and Hammer, C. (1986). Evasion of alternative complement pathway by Trypanosoma cruzi results from inefficient binding of factor B. Proc. Natl. Acad. Sci. U.S.A. 83, 6593–6597. doi: 10.1073/pnas.83.17.6593
Kanczkowski, W., Alexaki, V.-I., Tran, N., Großklaus, S., Zacharowski, K., Martinez, A., et al. (2013). Hypothalamo-pituitary and immune-dependent adrenal regulation during systemic inflammation. Proc. Natl. Acad. Sci. U.S.A. 110, 14801–14806. doi: 10.1073/pnas.1313945110
Kanczkowski, W., Sue, M., Zacharowski, K., Reincke, M., and Bornstein, S. R. (2015). The role of adrenal gland microenvironment in the HPA axis function and dysfunction during sepsis. Mol. Cell. Endocrinol. 408, 241–248. doi: 10.1016/j.mce.2014.12.019
Kierszenbaum, F. (1999). Chagas' disease and the autoimmunity hypothesis. Clin. Microbiol. Rev. 12, 210–223.
Kierszenbaum, F., De Diego, J. L., Fresno, M., and Sztein, M. B. (1999). Inhibitory effects of the Trypanosoma cruzi membrane glycoprotein AGC10 on the expression of IL-2 receptor chains and secretion of cytokines by subpopulations of activated human T lymphocytes. Eur. J. Immunol. 29, 1684–1691.
Kierszenbaum, F., Fresno, M., and Sztein, M. B. (2002). The Trypanosoma cruzi membrane glycoprotein AGC10 inhibits human lymphocyte activation by a mechanism preceding translation of both, interleukin-2 and its high-affinity receptor subunits. Mol. Biochem. Parasitol. 125, 91–101. doi: 10.1016/S0166-6851(02)00217-7
Kipnis, T. L., David, J. R., Alper, C. A., Sher, A., and da Silva, W. D. (1981). Enzymatic treatment transforms trypomastigotes of Trypanosoma cruzi into activators of alternative complement pathway and potentiates their uptake by macrophages. Proc. Natl. Acad. Sci. U.S.A. 78, 602–605. doi: 10.1073/pnas.78.1.602
Lauria-Pires, L. T. A. (1996). Virulence and pathogenicity associated with diversity of Trypanosoma cruzi stocks and clones derived from Chagas' disease patients. Am. J. Trop. Med. Hyg. 55, 304–310.
Leite de Moraes, M. C., Hontebeyrie-Joskowicz, M., Leboulenger, F., Savino, W., Dardenne, M., and Lepault, F. (1991). Studies on the thymus in Chagas' disease. II. Thymocyte subset fluctuations in Trypanosoma cruzi-infected mice: relationship to stress. Scand. J. Immunol. 33, 267–275. doi: 10.1111/j.1365-3083.1991.tb01772.x
Leite-de-Moraes, M. C., Hontebeyrie-Joskowicz, M., Dardenne, M., and Savino, W. (1992). Modulation of thymocyte subsets during acute and chronic phases of experimental Trypanosoma cruzi infection. Immunology 77, 95–98.
Lepletier, A., de Almeida, L., Santos, L., da Silva Sampaio, L., Paredes, B., González, F. B., et al. (2014). Early double-negative thymocyte export in Trypanosoma cruzi infection is restricted by sphingosine receptors and associated with human chagas disease. PLoS Negl. Trop. Dis. 8:e3203. doi: 10.1371/journal.pntd.0003203
Liew, F. Y., Scott, M. T., Liu, D. S., and Croft, S. L. (1987). Suppressive substance produced by T cells from mice chronically infected with Trypanosoma cruzi. I. Preferential inhibition of the induction of delayed-type hypersensitivity. J. Immunol. 139, 2452–2457.
Macedo, A. M., Machado, C. R., Oliveira, R. P., and Pena, S. D. J. (2004). Trypanosoma cruzi: genetic structure of populations and relevance of genetic variability to the pathogenesis of chagas disease. Mem. Inst. Oswaldo Cruz 99, 1–12. doi: 10.1590/S0074-02762004000100001
Maleckar, J. R., and Kierszenbaum, F. (1983). Inhibition of mitogen-induced proliferation of mouse T and B lymphocytes by bloodstream forms of Trypanosoma cruzi. J. Immunol. 130, 908–911.
Manarin, R., Villar, S. R., Bussy, R. F., González, F. B., Deschutter, E. V., Bonantini, A. P., et al. (2013). Reciprocal influences between leptin and glucocorticoids during acute Trypanosoma cruzi infection. Med. Microbiol. Immunol. 202, 339–352. doi: 10.1007/s00430-013-0294-1
Martinez, F. O., and Gordon, S. (2014). The M1 and M2 paradigm of macrophage activation: time for reassessment. F1000Prime Rep. 6:13. doi: 10.12703/P6-13
Martins, G. A., Cardoso, M. A., Aliberti, J. C., and Silva, J. S. (1998). Nitric oxide-induced apoptotic cell death in the acute phase of Trypanosoma cruzi infection in mice. Immunol. Lett. 63, 113–120. doi: 10.1016/S0165-2478(98)00066-2
Mendes-da-Cruz, D. A., de Meis, J., Cotta-de-Almeida, V., and Savino, W. (2003). Experimental Trypanosoma cruzi infection alters the shaping of the central and peripheral T-cell repertoire. Microbes Infect. 5, 825–832. doi: 10.1016/S1286-4579(03)00156-4
Mendonça-Previato, L., Penha, L., Garcez, T. C., Jones, C., and Previato, J. O. (2013). Addition of α-O-GlcNAc to threonine residues define the post-translational modification of mucin-like molecules in Trypanosoma cruzi. Glycoconj. J. 30, 659–666. doi: 10.1007/s10719-013-9469-7
Monje-Rumi, M. M., Brandán, C. P., Ragone, P. G., Tomasini, N., Lauthier, J. J., Alberti D'Amato, A. M., et al. (2015). Trypanosoma cruzi diversity in the Gran Chaco: mixed infections and differential host distribution of TcV and TcVI. Infect. Genet. Evol. 29, 53–59. doi: 10.1016/j.meegid.2014.11.001
Montaudouin, C., Anson, M., Hao, Y., Duncker, S. V., Fernandez, T., Gaudin, E., et al. (2013). Quorum sensing contributes to activated IgM-secreting B cell homeostasis. J. Immunol. 190, 106–114. doi: 10.4049/jimmunol.1200907
Morrot, A., Terra-Granado, E., Pérez, A. R., Silva-Barbosa, S. D., Milićević, N. M., Farias-de-Oliveira, D. A., et al. (2011). Chagasic thymic atrophy does not affect negative selection but results in the export of activated CD4+CD8+ T cells in severe forms of human disease. PLoS Negl. Trop. Dis. 5:e1268. doi: 10.1371/journal.pntd.0001268
Nagajyothi, F., Desruisseaux, M. S., Weiss, L. M., Chua, S., Albanese, C., Machado, F. S., et al. (2009). Chagas disease, adipose tissue and the metabolic syndrome. Mem. Inst. Oswaldo Cruz 104(Suppl.), 219–225. doi: 10.1590/S0074-02762009000900028
Nagajyothi, F., Machado, F. S., Burleigh, B. A., Jelicks, L. A., Scherer, P. E., Mukherjee, S., et al. (2012). Mechanisms of Trypanosoma cruzi persistence in Chagas disease. Cell. Microbiol. 14, 634–643. doi: 10.1111/j.1462-5822.2012.01764.x
Neyrolles, O., Hernández-Pando, R., Pietri-Rouxel, F., Fornès, P., Tailleux, L., Payán, J. A. B., et al. (2006). Is adipose tissue a place for Mycobacterium tuberculosis persistence? PLoS ONE 1:e43. doi: 10.1371/journal.pone.0000043
Noireau, F., Diosque, P., and Jansen, A. M. (2009). Trypanosoma cruzi: adaptation to its vectors and its hosts. Vet. Res. 40:26. doi: 10.1051/vetres/2009009
Norris, K. A., Bradt, B., Cooper, N. R., and So, M. (1991). Characterization of a Trypanosoma cruzi C3 binding protein with functional and genetic similarities to the human complement regulatory protein, decay-accelerating factor. J. Immunol. 147, 2240–2247.
Nunes, M. P., Andrade, R. M., Lopes, M. F., and DosReis, G. A. (1998). Activation-induced T cell death exacerbates Trypanosoma cruzi replication in macrophages cocultured with CD4+ T lymphocytes from infected hosts. J. Immunol. 160, 1313–1319.
Nunes, M. P., Fortes, B., Silva-Filho, J. L., Terra-Granado, E., Santos, L., Conde, L., et al. (2013). Inhibitory Effects of Trypanosoma cruzi Sialoglycoproteins on CD4+ T cells are associated with increased susceptibility to infection. PLoS ONE 8:e77568. doi: 10.1371/journal.pone.0077568
Oladiran, A., and Belosevic, M. (2012). Immune evasion strategies of trypanosomes: a review. J. Parasitol. 98, 284–292. doi: 10.1645/GE-2925.1
Oliveira, A.-C., Peixoto, J. R., de Arruda, L. B., Campos, M. A., Gazzinelli, R. T., Golenbock, D. T., et al. (2004). Expression of functional TLR4 confers proinflammatory responsiveness to Trypanosoma cruzi glycoinositolphospholipids and higher resistance to infection with T. cruzi. J. Immunol. 173, 5688–5696. doi: 10.4049/jimmunol.173.9.5688
Ortiz-Ortiz, L., Parks, D. E., Rodriguez, M., and Weigle, W. O. (1980). Polyclonal B lymphocyte activation during Trypanosoma cruzi infection. J. Immunol. 124, 121–126.
Ouaissi, A., Da Silva, A. C., Guevara, A. G., Borges, M., and Guilvard, E. (2001). Trypanosoma cruzi -induced host immune system dysfunction: a rationale for parasite immunosuppressive factor(s) encoding gene targeting. J. Biomed. Biotechnol. 2001, 11–17. doi: 10.1155/S1110724301000055
Pacholczyk, R., and Kern, J. (2008). The T-cell receptor repertoire of regulatory T cells. Immunology 125, 450–458. doi: 10.1111/j.1365-2567.2008.02992.x
Padilla, A. M., Simpson, L. J., and Tarleton, R. L. (2009). Insufficient TLR activation contributes to the slow development of CD8+ T cell responses in Trypanosoma cruzi infection. J. Immunol. 183, 1245–1252. doi: 10.4049/jimmunol.0901178
Paulnock, D. M., and Coller, S. P. (2001). Analysis of macrophage activation in African trypanosomiasis. J. Leukoc. Biol. 69, 685–690.
Pereira-Chioccola, V. L., Acosta-Serrano, A., Correia de Almeida, I., Ferguson, M. A., Souto-Padron, T., Rodrigues, M. M., et al. (2000). Mucin-like molecules form a negatively charged coat that protects Trypanosoma cruzi trypomastigotes from killing by human anti-alpha-galactosyl antibodies. J. Cell Sci. 113(Pt 7), 1299–1307.
Pérez, A. R., Bertoya, A. A., Revelli, S., and García, F. (2011). A high corticosterone/DHEA-s ratio in young rats infected with Trypanosoma cruzi is associated with increased susceptibility. Mem. Inst. Oswaldo Cruz 106, 416–423. doi: 10.1590/S0074-02762011000400006
Pérez, A. R., Roggero, E., Nicora, A., Palazzi, J., Besedovsky, H. O., del Rey, A., et al. (2007). Thymus atrophy during Trypanosoma cruzi infection is caused by an immuno-endocrine imbalance. Brain Behav. Immun. 21, 890–900. doi: 10.1016/j.bbi.2007.02.004
Pérez, R., Silva-Barbosa, S. D., Berbert, L. R., Revelli, S., Beloscar, J., Savino, W., et al. (2011). Immunoneuroendocrine alterations in patients with progressive forms of chronic Chagas disease. J. Neuroimmunol. 235, 84–90. doi: 10.1016/j.jneuroim.2011.03.010
Pinazo, M. J., Espinosa, G., Cortes-Lletget, C., Posada Ede, J., Aldasoro, E., Oliveira, I., et al. (2013). Immunosuppression and chagas disease: a management challenge. PLoS Negl. Trop. Dis. 7:e1965. doi: 10.1371/journal.pntd.0001965
Previato, J. O., Jones, C., Xavier, M. T., Wait, R., Travassos, L. R., Parodi, A. J., et al. (1995). Structural characterization of the major glycosylphosphatidylinositol membrane-anchored glycoprotein from epimastigote forms of Trypanosoma cruzi Y-strain. J. Biol. Chem. 270, 7241–7250. doi: 10.1074/jbc.270.13.7241
Radomski, M. W., Buguet, A., Bogui, P., Doua, F., Lonsdorfer, A., Tapie, P., et al. (1994). Disruptions in the secretion of cortisol, prolactin, and certain cytokines in human African trypanosomiasis patients. Bull. Soc. Pathol. Exot. 87, 376–379.
Radomski, M. W., Buguet, A., Doua, F., Bogui, P., and Tapie, P. (1996). Relationship of plasma growth hormone to slow-wave sleep in African sleeping sickness. J. Neuroendocrinol. 63, 393–396. doi: 10.1159/000126980
Ramos, S. G., and Rossi, M. A. (1999). Invited review microcirculation and Chagas' disease: hypothesis and recent results. Rev. Inst. Med. Trop. Sao Paulo 41, 123–129. doi: 10.1590/S0036-46651999000200011
Rassi, A. Jr., Rassi, A., and Marin-Neto, J. A. (2010). Chagas disease. Lancet 375, 1388–1402. doi: 10.1016/S0140-6736(10)60061-X
Reina-San-Martín, B., Degrave, W., Rougeot, C., Cosson, A., Chamond, N., Cordeiro-Da-Silva, A., et al. (2000). A B-cell mitogen from a pathogenic trypanosome is a eukaryotic proline racemase. Nat. Med. 6, 890–897. doi: 10.1038/78651
Roggero, E., Pérez, A. R., Tamae-Kakazu, M., Piazzon, I., Nepomnaschy, I., Basedovsky, H. O., et al. (2006). Edogenous glucocorticoids cause thymus atrophy but are protective during acute Trypanosoma cruzi infection. J. Endocrinol. 190, 495–503. doi: 10.1677/joe.1.06642
Romano, M. C., Jiménez, P., Miranda-Brito, C., and Valdez, R. A. (2015). Parasites and steroid hormones: corticosteroid and sex steroid synthesis, their role in the parasite physiology and development. Front. Neurosci. 9:224. doi: 10.3389/fnins.2015.00224
Romano, P. S., Cueto, J. A., Casassa, A. F., Vanrell, M. C., Gottlieb, R. A., and Colombo, M. I. (2012). Molecular and cellular mechanisms involved in the Trypanosoma cruzi /host cell interplay. IUBMB Life 64, 387–396. doi: 10.1002/iub.1019
Salomone, O. A., Juri, D., Omelianiuk, M. O., Sembaj, A., Aguerri, A. M., Carriazo, C., et al. (2000). Prevalence of circulating Trypanosoma cruzi detected by polymerase chain reaction in patients with Chagas' cardiomyopathy. Am. J. Cardiol. 85, 1274–1276. doi: 10.1016/S0002-9149(00)00747-5
Sartori, A. M. C., Lopes, M. H., Benvenuti, L. A., Caramelli, B., Di Pietro, A. O., Nunes, E. V., et al. (1998). Reactivation of Chagas' disease in a human immunodeficiency virus- infected patient leading to severe heart disease with a late positive direct microscopic examination of the blood. Am. J. Trop. Med. Hyg. 59, 784–786.
Savino, W. (2006). The thymus is a common target organ in infectious diseases. PLoS Pathog. 2:e62. doi: 10.1371/journal.ppat.0020062
Savino, W., Dardenne, M., Marche, C., Trophilme, D., Dupuy, J. M., Pekovic, D., et al. (1986). Thymic epithelium in AIDS. An immunohistologic study. Am. J. Pathol. 122, 302–307.
Savino, W., Leite-de-Moraes, M. C., Hontebeyrie-Joskowicz, M., and Dardenne, M. (1989). Studies on the thymus in Chagas' disease. I. Changes in the thymic microenvironment in mice acutely infected with Trypanosoma cruzi. Eur. J. Immunol. 19, 1727–1733. doi: 10.1002/eji.1830190930
Savino, W., Villa-Verde, D. M. S., Mendes-da-Cruz, D. A., Silva-Monteiro, E., Perez, A. R., Aoki M del, P., et al. (2007). Cytokines and cell adhesion receptors in the regulation of immunity to Trypanosoma cruzi. Cytokine Growth Factor Rev. 18, 107–124. doi: 10.1016/j.cytogfr.2007.01.010
Schenkman, S., Eichinger, D., Pereira, M. E., and Nussenzweig, V. (1994). Structural and functional properties of Trypanosoma trans-sialidase. Annu. Rev. Microbiol. 48, 499–523. doi: 10.1146/annurev.mi.48.100194.002435
Schenkman, S., Jiang, M. S., Hart, G. W., and Nussenzweig, V. (1991). A novel cell surface trans-sialidase of Trypanosoma cruzi generates a stage-specific epitope required for invasion of mammalian cells. Cell 65, 1117–1125. doi: 10.1016/0092-8674(91)90008-M
Seixas, E., and Ostler, D. (2005). Plasmodium chabaudi chabaudi (AS): differential cellular responses to infection in resistant and susceptible mice. Exp. Parasitol. 110, 394–405. doi: 10.1016/j.exppara.2005.03.024
Silva, J. S., Twardzik, D. R., and Reed, S. G. (1991). Regulation of Trypanosoma cruzi infections in vitro and in vivo by transforming growth factor beta (TGF-β). J. Exp. Med. 174, 539–545. doi: 10.1084/jem.174.3.539
Sizirensen, A. L., Hey, A. S., and Kharazmi, A. (1994). Leishmania major surface protease Gp63 interferes with the function of human monocytes and neutrophils in vitro. APMIS 102, 65–271. doi: 10.1111/j.1699-0463.1994.tb04874.x
Stahl, P., Ruppert, V., Schwarz, R. T., and Meyer, T. (2014). Trypanosoma cruzi evades the protective role of interferon-gamma-signaling in parasite-infected cells. PLoS ONE 9:e110512. doi: 10.1371/journal.pone.0110512
Stempin, C., Giordanengo, L., Gea, S., and Cerbán, F. (2002). Alternative activation and increase of Trypanosoma cruzi survival in murine macrophages stimulated by cruzipain, a parasite antigen. J. Leukoc. Biol. 72, 727–734.
Suzuki, H., Motohara, M., Miyake, A., Ibuki, K., Fukazawa, Y., Inaba, K., et al. (2005). Intrathymic effect of acute pathogenic SHIV infection on T-lineage cells in newborn macaques. Microbiol. Immunol. 49, 667–679. doi: 10.1111/j.1348-0421.2005.tb03646.x
Tambourgi, D. V., Kipnis, T. L., Da Silva, W. D., Joiner, K. A., Sher, A., Heath, S., et al. (1993). A partial cDNA clone of trypomastigote decay-accelerating factor (T-DAF), a developmentally regulated complement inhibitor of Trypanosoma cruzi, has genetic and functional similarities to the human complement inhibitor DAF. Infect. Immun. 61, 3656–3663.
Tarleton, R. L. (2001). Parasite persistence in the aetiology of Chagas disease. Int. J. Parasitol. 31, 550–554. doi: 10.1016/S0020-7519(01)00158-8
Vacchina, P., Valdéz, R. A., Gómez, Y., Revelli, S., and Romano, M. C. (2008). Steroidogenic capacity of Trypanosoma cruzi trypomastigotes. J. Steroid Biochem. Mol. Biol. 111, 282–286. doi: 10.1016/j.jsbmb.2008.06.016
Van Overtvelt, L., Andrieu, M., Verhasselt, V., Connan, F., Choppin, J., Vercruysse, V., et al. (2002). Trypanosoma cruzi down-regulates lipopolysaccharide-induced MHC class I on human dendritic cells and impairs antigen presentation to specific CD8(+) T lymphocytes. Int. Immunol. 14, 1135–1144. doi: 10.1093/intimm/dxf077
Villar, S. R., Ronco, M. T., Fernández Bussy, R., Roggero, E., Lepletier, A., Manarin, R., et al. (2013). Tumor necrosis factor-α regulates glucocorticoid synthesis in the adrenal glands of Trypanosoma cruzi acutely-infected mice. The Role of TNF-R1. PLoS ONE 8:e63814. doi: 10.1371/journal.pone.0063814
Watson, S. R., Miller, T. B., Redington, T. J., and Bullock, W. E. (1983). Immunoregulation in experimental disseminated histoplasmosis: flow microfluorometry (FMF) studies of the Thy and Lyt phenotypes of T lymphocytes from infected mice. J. Immunol. 131, 984–990.
Watson, S. R., Redington, T. J., Miller, T. B., and Bullock, W. E. (1984). Flow microfluorometry analysis of alterations in T-lymphocyte subsets during murine listeriosis. Infect. Immun. 45, 372–377.
WHO (2015). Third WHO Report on Neglected Tropical Diseases. Invest to Overcome Global Impact of Neglected Tropical Disease. Geneva.
Zambrano-Villa, S., Rosales-Borjas, D., Carrero, J. C., and Ortiz-Ortiz, L. (2002). How protozoan parasites evade the immune response. Trends Parasitol. 18, 272–278. doi: 10.1016/S1471-4922(02)02289-4
Zhang, L., and Tarleton, R. L. (1999). Parasite persistence correlates with disease severity and localization in chronic Chagas' disease. J. Infect. Dis. 180, 480–486. doi: 10.1086/314889
Zingales, B., Andrade, S. G., Briones, M. R. S., Campbell, D. A., Chiari, E., Fernandes, O., et al. (2009). A new consensus for Trypanosoma cruzi intraspecific nomenclature: second revision meeting recommends TcI to TcVI. Mem. Inst. Oswaldo Cruz 104, 1051–1054. doi: 10.1590/S0074-02762009000700021
Zuñiga, E., Motran, C., Montes, C. L., Diaz, F. L., Bocco, J. L., and Gruppi, A. (2000). Trypanosoma cruzi -induced immunosuppression: B cells undergo spontaneous apoptosis and lipopolysaccharide (LPS) arrests their proliferation during acute infection. Clin. Exp. Immunol. 119, 507–515. doi: 10.1046/j.1365-2249.2000.01150.x
Keywords: evasion strategies, persistence, Chagas disease, immunoendocrine, thymus, virulence factors
Citation: Morrot A, Villar SR, González FB and Pérez AR (2016) Evasion and Immuno-Endocrine Regulation in Parasite Infection: Two Sides of the Same Coin in Chagas Disease?. Front. Microbiol. 7:704. doi: 10.3389/fmicb.2016.00704
Received: 27 November 2015; Accepted: 28 April 2016;
Published: 23 May 2016.
Edited by:
Abhay Satoskar, The Ohio State University, USAReviewed by:
Hajime Hisaeda, Gunma University, JapanJulio Aliberti, Cincinnati Children's Hospital Medical Center, USA
Copyright © 2016 Morrot, Villar, González and Pérez. This is an open-access article distributed under the terms of the Creative Commons Attribution License (CC BY). The use, distribution or reproduction in other forums is permitted, provided the original author(s) or licensor are credited and that the original publication in this journal is cited, in accordance with accepted academic practice. No use, distribution or reproduction is permitted which does not comply with these terms.
*Correspondence: Ana R. Pérez, cGVyZXpAaWRpY2VyLWNvbmljZXQuZ29iLmFy; cGVyZXpfYW5hcm9zYUB5YWhvby5jb20uYXI=