- 1Division of Pediatric Gastroenterology and Hepatology, Department of Pediatrics, Shuang Ho Hospital, Taipei Medical University, Taipei, Taiwan
- 2Department of Pediatrics, School of Medicine, College of Medicine, Taipei Medical University, Taipei, Taiwan
- 3Master Program for Clinical Pharmacogenomics and Pharmacoproteomics, College of Pharmacy, Taipei Medical University, Taipei, Taiwan
- 4Graduate Institute of Biochemical and Biomedical Engineering, National Taipei University of Technology, Taipei, Taiwan
- 5Graduate Institution of Engineering Technology-Doctoral, National Taipei University of Technology, Taipei, Taiwan
- 6Institute of Biomedical Engineering and Nanomedicine, National Health Research Institutes, Zhunan, Taiwan
- 7Department of Surgery, Shuang Ho Hospital, Taipei Medical University, Taipei, Taiwan
- 8Department of Surgery, School of Medicine, College of Medicine, Taipei Medical University, Taipei, Taiwan
The speG gene has been reported to regulate polyamine metabolism in Escherichia coli and Shigella, but its role in Salmonella remains unknown. Our preliminary studies have revealed that speG widely affects the transcriptomes of infected in vitro M and Caco-2 cells and that it is required for the intracellular replication of Salmonella enterica serovar Typhimurium (S. Typhimurium) in HeLa cells. In this study, we demonstrated that speG plays a time-dependent and cell type-independent role in the intracellular replication of S. Typhimurium. Moreover, high-performance liquid chromatography (HPLC) of four major polyamines demonstrated putrescine, spermine, and cadaverine as the leading polyamines in S. Typhimurium. The deletion of speG significantly increased the levels of the three polyamines in intracellular S. Typhimurium, suggesting the inhibitory effect of speG on the biosynthesis of these polyamines. The deletion of speG was associated with elevated levels of these polyamines in the attenuated intracellular replication of S. Typhimurium in host cells. This result was subsequently validated by the dose-dependent suppression of intracellular proliferation after the addition of the polyamines. Furthermore, our RNA transcriptome analysis of S. Typhimurium SL1344 and its speG mutant outside and inside Caco-2 cells revealed that speG regulates the genes associated with flagellar biosynthesis, fimbrial expression, and functions of types III and I secretion systems. speG also affects the expression of genes that have been rarely reported to correlate with polyamine metabolism in Salmonella, including those associated with the periplasmic nitrate reductase system, glucarate metabolism, the phosphotransferase system, cytochromes, and the succinate reductase complex in S. Typhimurium in the mid-log growth phase, as well as those in the ilv–leu and histidine biosynthesis operons of intracellular S. Typhimurium after invasion in Caco-2 cells. In the present study, we characterized the phenotypes and transcriptome effects of speG in S. Typhimurium and reviewed the relevant literature to facilitate a more comprehensive understanding of the potential role of speG in the polyamine metabolism and virulence regulation of Salmonella.
Introduction
Non-typhoidal Salmonella are important pathogens that cause a wide spectrum of diseases and considerable morbidity and mortality in humans and animals worldwide (Hohmann, 2001). Salmonella are invading and intracellularly replicating bacteria (Dougan et al., 2011). Host cell invasion (Pace et al., 1993) and intracellular replication (Leung and Finlay, 1991) are essential for the pathogenesis of Salmonella enterica serovar Typhimurium (S. Typhimurium). More than 100 virulence-associated genes have been discovered among the approximately 4,500 genes present in the genome of S. Typhimurium (McClelland et al., 2001). Most virulence genes are clustered in at least 23 Salmonella pathogenicity islands (SPIs) distributed on the Salmonella chromosome (Espinoza et al., 2017), including 11 common SPIs in S. Typhimurium and S. Typhi (Sabbagh et al., 2010). The most commonly studied SPIs, SPI-1 and SPI-2, encode type III secretion systems (T3SSs), which can translocate effector proteins into host cells or secrete them into the extracellular environment to manipulate host cell physiology and biochemistry (Coburn et al., 2007). SPI-1 genes facilitate bacterial invasion in non-phagocytic cells and uptake into phagocytic cells in the early phase of infection (Bueno et al., 2010). By contrast, SPI-2 genes account for intracellular survival and the evasion of the oxidase defense system of host cells, particularly in the systemic phase of salmonellosis (Coburn et al., 2007). The SPI-2 T3SS is essential for bacterial intracellular replication in Salmonella-containing vacuoles in host cells through the translocation of approximately 30 SPI-2 T3SS effector proteins into the host endomembrane system and cytosol (Figueira and Holden, 2012). However, the physiological relevance of SPI-2 T3SS effectors and the effects of their coordination on SPI-2 T3SS-mediated intracellular replication remain unclear (Helaine et al., 2010). Until now, SPI-2 T3SS genes associated with the intracellular replication of Salmonella have been mostly reported in phagocytic cells (Helaine et al., 2010; Figueira and Holden, 2012; Figueira et al., 2013). A few studies have demonstrated that SPI-1 T3SS genes are required for intracellular replication in human cervical epithelial cells (Steele-Mortimer et al., 2002) and 3-dimensional colonic epithelial cells (Radtke et al., 2010). Furthermore, additional studies have reported that auxotrophic mutations in aromatic amino acid metabolism and purine biosynthesis attenuated the intracellular replication of S. Typhimurium in various cell lines, including Madin–Darby canine kidney epithelial cells, human cervical HeLa cells, and intestinal epithelial Caco-2 and T-84 cells (Leung and Finlay, 1991; Holzer and Hensel, 2012). However, virulence genes involved in the intracellular replication of Salmonella in human non-phagocytic epithelial cells have not been thoroughly investigated.
Following the isolation of 10 auxotrophic replication-defective mutants from the 45,000 transposon mutants of S. Typhimurium in 1991 (Leung and Finlay, 1991), large-scale screening studies using high-throughput technologies, including libraries of transposon mutants and transcriptomic analysis, have identified previously unreported genes that are required for intracellular replication in non-phagocytic cells. The intracellular proliferation of S. Typhimurium occurs in cultured epithelial and macrophage cells but not in normal fibroblast cells (Martinez-Moya et al., 1998; Cano et al., 2001; Nunez-Hernandez et al., 2013). Thus, 50,000 independent transposon MudJ-generated mutants derived from wild-type S. Typhimurium were selected in rat kidney fibroblasts after 72-h intracellular incubation. Genome analysis of the non-proliferating intracellular mutants revealed that a novel gene, igaA, suppresses their growth within fibroblasts (Cano et al., 2001). Meanwhile, mutations in phoQ, rpoS, slyA, and spvR, which have been demonstrated to be essential for the in vivo intracellular proliferation of Salmonella, resulted in the attenuation of intracellular bacterial growth in fibroblasts. This suggested that the PhoP–PhoQ two-component system is a negative regulator of bacterial growth in fibroblasts and so are the different phenotypes of these genes in diverse cell types (Cano et al., 2001). A recent genome-wide study conducted using the expression profiling of non-growing wild-type S. Typhimurium collected at 24 h postinfection in the same rat fibroblasts revealed that approximately 2% of the S. Typhimurium genome was differentially expressed in non-proliferating intracellular bacteria. This included the 98 genes involved in metabolic reprogramming for microaerophilic conditions, the induction of virulence plasmid genes, the upregulation of SPI-1 and SPI-2, and the shutdown of chemotaxis and flagellation (Nunez-Hernandez et al., 2013). Similarly, the transcriptome showed activated functions of PhoP–PhoQ-regulating PagN, PagP, and VirK in dormant intracellular bacteria after sensing vacuolar acidic pH for preventing intracellular overgrowth (Nunez-Hernandez et al., 2013). Another non-phagocytic cell line, HeLa cell line, has been extensively used to study intracellular replication of not only Salmonella spp. but also of enteroinvasive Escherichia coli (E. coli) and Yersinia spp. (Small et al., 1987; Leung and Finlay, 1991; Hautefort et al., 2008). The microarray analysis of time-dependent changes in Salmonella gene expression in HeLa cells and J774A.1 murine macrophages demonstrated the upregulation of iro, mgtBC, and pstACS; genes for iron, magnesium, and phosphate uptake; and SPI-2 (Hautefort et al., 2008). The invasion-associated SPI-1 and flagellar genes are upregulated in epithelial cells at 6 h postinfection when bacteria are intracellularly replicating but are constantly downregulated in J774A.1 murine macrophages (Hautefort et al., 2008). A recent study conducted using a mutational approach reported that the replication of S. Typhimurium in murine colonic epithelial cells requires glycosis and ubiquinone, but not an intact tricarboxylic acid cycle (TCA cycle), adenosine triphosphate (ATP) synthase, and fermentation (Garcia-Gutierrez et al., 2016). It remains unclear whether malate could be replenished by succinate or its precursors from non-phagocytic cells similar to phagocytes, although conversion from succinate to fumarate, from fumarate to malate, and from malate to both oxaloacetate and pyruvate in the TCA cycle are required for full virulence of S. Typhimurium in mice (Tchawa Yimga et al., 2006; Mercado-Lubo et al., 2008, 2009). So far, the virulence genes involved in the intracellular replication of Salmonella in human intestinal epithelial cells have not been thoroughly investigated.
Our preliminary study conducted using a library of 1,440 transposon mutants of S. Typhimurium to invade HeLa cell monolayers for 10 h and a high-throughput genome-wide analysis through transposon-directed insertion-site sequencing (Chaudhuri et al., 2013) identified speG as a gene essential for the intracellular replication of Salmonella in human epithelial cells (Fang, 2011). However, it remains unknown whether this result is applicable to other cells. The speG mutant of S. Typhimurium is a non-replicating strain in human cells and is thus a candidate vaccine vector for interacting with intestinal epithelial cells (Wang et al., 2016b). We used RNA microarrays to determine whether S. Typhimurium and speG affect the transcriptomes of two human intestinal epithelial cells and identified speG-regulated genes, including KYL4, SCTR, IL6, TNF, and CELF4 in Caco-2 cells and JUN, KLF6, and KCTD11 in in vitro M cells, which are specialized intestinal epithelial cells conferring host immunity (Wang et al., 2016b). However, it is unclear whether speG regulates the expression of other genes in Salmonella before and after bacterial invasion in human intestinal epithelium.
Until now, knowledge regarding speG has been obtained from studies mainly conducted in E. coli and Shigella, but rarely in Salmonella. speG is involved in polyamine metabolism and stress responses in bacterial pathogenesis. It encodes spermidine acetyltransferase (SAT), which catalyzes spermidine to acetylspermidine in E. coli. However, the speG-dependent acetylation of spermidine and the speE-dependent catabolization of cadaverine into aminopropyl cadaverine are not conserved in Shigella spp. (Barbagallo et al., 2011). The accumulation of spermidine is toxic for E. coli and reduces the viability of the speG-deficient mutant of E. coli at the late stationary growth phase. However, excessive spermidine can be inactivated by its speG-catalyzed acetylation to acetylspermidine, which is either stored or secreted by the cells (Fukuchi et al., 1995). The other spe genes, including speB, speC, speE, and speF, have been reported to contribute to the intracellular survival and replication of S. Typhimurium in human epithelial cells for 18 h (Jelsbak et al., 2012) and in macrophages for 21 h (Espinel et al., 2016). However, the role of speG in regulating polyamine metabolism and influencing the intracellular replication of Salmonella in human intestinal epithelial cells has been rarely investigated.
Polyamine composition and the predominant polyamine in Salmonella are unclear. Until now, our understanding of polyamines in bacteria has mainly been established through studies of E. coli. Putrescine, spermidine, spermine, and cadaverine are the major cellular polyamines essential for the normal cellular proliferation and growth of both prokaryotic and eukaryotic cells (Cohen, 1997; Shah and Swiatlo, 2008). The intracellular concentration of spermidine is much higher than that of putrescine in almost all bacteria, but 10 times lower than that of putrescine in E. coli (Cohen, 1997; Shah and Swiatlo, 2008). Spermine is only found in the presence of exogenous spermine in most bacteria, whereas cadaverine, typically absent in E. coli, is the least widespread of naturally occurring bacterial polyamines (Cohen, 1997). Putrescine constitutes the outer membrane of S. Typhimurium and E. coli (Koski and Vaara, 1991). However, whether putrescine is the predominant intracellular polyamine in Salmonella, similar to E. coli, requires further validation.
In this study, we examined whether the deletion of speG affects the intracellular proliferation of S. Typhimurium in various human cells. Subsequently, we studied the polyamine metabolism of S. Typhimurium and the effect of speG by quantifying the four major polyamines in extracellular and intracellular wild-type and speG-deleted strains. We verified whether the accumulation of polyamines suppresses the intracellular proliferation of S. Typhimurium. Moreover, we investigated how speG regulates the transcriptome of S. Typhimurium before and after invasion in human intestinal epithelium. Finally, we determined whether the deletion of speG affects the motility and flagellation of S. Typhimurium.
Materials and Methods
Bacterial Strains and Culture Conditions
The S. Typhimurium wild-type strain SL1344, its isogenic speG-deleted mutant ΔspeG, the speG-complemented strain of ΔspeG (ΔspeG′), the fliC-deleted flagellin-deficient mutant ΔfliC, and spaS-deleted invasion-deficient SPI-1 mutant ΔspaS were used in this study. SL1344 (Mo et al., 2006) and ΔspaS (Buckley et al., 2010) were kindly provided by Prof. Duncan Maskell. The S. Typhimurium SL1344 genome has been completely sequenced, and its complete sequence and annotation are available in Genbank (accession numbers FQ312003 and HE654724-6). The mutants ΔfliC and ΔspaS were used as controls. ΔspeG and ΔfliC were constructed using the lambda red recombinase-mediated integration of linear polymerase chain reaction (PCR) amplicons to replace the target gene with a kanamycin resistance gene cassette, as previously reported (Gust et al., 2003; Wang et al., 2016a,b). For generating the speG-complemented S. Typhimurium strain (ΔspeG′), the speG-coding sequence was amplified using speG-specific primers (forward, 5′-ATCTTACTGCGCGGTGGGTT-3′, and reverse, 5′-GATGCAGGATAACTAAAAGGAAGTGTAAGGATACAGTATGA-3′) and cloned into the pBluescript II KS(−) vector in the EcoRV site. Subsequently, the cloned vector was digested by EcoRV and ligated with the apramycin resistance gene, aac(3)IV, which was then amplified using the aac(3)IV specific primers (forward, 5′-TACCACCGCTGGTAGCGGT-3′, and reverse, 5′-AAACCGGGCGCGGTGCGACTCTCCGTGACTACCGCGCCGCGACGCTGATCGTGCGGGAG-3′) in the same gene orientation. With 41 nucleotides at both ends homologous to the replacing kanamycin resistant gene, the fused speG-aac(3)IV DNA fragment was amplified using the primers (forward, 5′-GATGCAGGATAACTAAAAGGAAGTGTAAGGATACAGTATGA-3′, and reverse, 5′-AAACCGGGCGCGGTGCGACTCTCCGTGACTACCGCGCCGCGACGCTGATCGTGCGGGAG-3′), then transferred into the ΔspeG mutant. The speG expression was restored following the same red recombination strategy as previously prescribed (Wang et al., 2016a). The ΔspeG′ was maintained in LB broth supplemented with apramycin (50 μg/mL) at 37°C. This study was conducted in the Biosafety Level 2 Laboratory that had been approved by the Biosafety Committee of Taipei Medical University Shuang Ho Hospital (No. BSL-2-0001).
In Vitro Cell Cultures
Four human cell lines used in this study were purchased from Bioresource Collection and Research Center, Taiwan. HeLa cells (BCRC No. 60005, originally from ATCC CCL-2), which are an epithelial cell line of human cervical carcinoma, and LS174T cells (BCRC No. 60053, originally derived from ATCC CL-188), which are a human intestinal epithelial cell line of Caucasian Duke's type B colorectal adenocarcinoma, were cultured in 90% Dulbecco's modified Eagle medium (DMEM, 4,500 mg/L glucose; Gibco) complemented with 2 mM L-glutamine (Gibco) adjusted to contain 1.5 g/L sodium bicarbonate (Sigma), 0.1 mM non-essential amino acids (Gibco), 1 mM sodium pyruvate (Gibco), and 10% fetal bovine serum (FBS, Sigma). Furthermore, Caco-2 cells (BCRC No. 67001, originally from ATCC HTB-37), a human intestinal epithelial cell line of a Caucasian colon adenocarcinoma, were cultured in the same medium as that for HeLa cells, except for the substitution of 10% FBS with 20% FBS. THP-1 cells (BCRC No. 60430, originally from ATCC TIB-202), a cell line of human acute monocytic leukemia, were cultured in a suspension of 90% RPMI 1640 medium (Gibco) complemented with 2 mM L-glutamine (Gibco) adjusted to contain 1.5 g/L sodium bicarbonate, 2.5 g/L glucose, 10 mM 4-(2-hydroxyethyl)piperazine-1-ethanesulfonic acid (HEPES), 1 mM sodium pyruvate, 0.05 mM 2-mercaptoethanol (Gibco), and 10% FBS. For maintenance, these cells were grown in 75-cm2 flasks in humidified 5% CO2 at 37°C and were split in a 1:4 ratio of 0.25% trypsin–ethylenediaminetetra acetic acid (Gibco) before complete confluence.
For in vitro infection assays, these cells were seeded at a density of 5 × 105 cells/well into 12-well plates and were maintained in humidified 5% CO2 at 37°C. The cell culture medium was replaced every other day when the cells were incubated for 3, 4, and 5 days until a complete confluence of the cell monolayers was achieved at a density of approximately 1 × 106 cells/well for HeLa cells, 8 × 105 cells/well for Caco-2 cells, and 2 × 106 cells/well for LS 174T cells, respectively. Meanwhile, THP-1 cells in suspension were seeded at a density of 2 × 106 cells/well into 12-well plates and were incubated with 10 ng/well phorbol myristate acetate (Sigma) for 24 h to induce differentiation into adherent macrophages for further assays. The medium in each well of the cell monolayer was replaced with their corresponding complete medium without FBS 1 h before assays.
Bacterial Intracellular Replication Assay
HeLa cell monolayers were infected with overnight cultures of S. Typhimurium SL1344, ΔspeG, and ΔspeG′ [multiplicity of infection (MOI) = 5] in duplicate wells for each bacterial strain and were incubated in 5% CO2 at 37°C for 2 h. After washing three times with phosphate-buffered saline (PBS), the infected cells were incubated in FBS-free DMEM supplemented with gentamicin (100 μg/mL) for 1 h; thereafter, the cells were washed with PBS three times to kill extracellular bacteria. At this point, one set of the cells infected with the three S. Typhimurium strains was lysed with 1% Triton X-100 to generate output pool A, which represents invading bacteria. The other two sets of the cells infected with the S. Typhimurium strains were incubated for an additional 7 and 10 h in FBS-free DMEM supplemented with low-dose gentamicin (10 μg/mL) to allow intracellular infections to continue. The cells were subsequently washed with PBS three times and lysed with 1% Triton X-100 (Sigma) to generate output pools B1 and B2, respectively, which represent intracellularly replicating bacteria after incubation at different durations.
The prepared confluent HeLa, Caco-2, LS 174T, and THP-1 cells in 12-well plates were infected with overnight cultures of the three S. Typhimurium strains (MOI = 5) for 2 h and treated with gentamicin (100 μg/mL) for 1 h using the same protocol as that used for obtaining output pool A. After washing with PBS three times, the cells were incubated for an additional 15 h. Finally, the cells were lysed with 1% Triton X-100 to generate output pool B, which contained bacteria proliferated in the cells for a total of 18 h.
The infected cell monolayers were stained with trypan blue to confirm a viability of >95% in all the wells before cell lysis to obtain output pools B1, B2, and B. The intracellular bacterial counts respective to the initial inoculums in output pools A, B1, B2, and B were compared between the two recombinant strains and wild-type strain of S. Typhimurium by using the Student's t-test. The intracellular bacterial counts in all output pools are expressed as mean ± standard error colony-forming units (CFU) per inoculum of 107 CFU. p < 0.05 was considered statistically significant.
High-Performance Liquid Chromatography Quantification of Four Major Polyamines in S. Typhimurium SL1344 and ΔspeG before and after Invasion in Caco-2 Cells
Before the experiment, overnight cultures of S. Typhimurium SL1344 and ΔspeG were 1:100 diluted in Luria–Bertani (LB) broth and incubated with shaking at 225 rpm in 5% CO2 at 37°C for 3 h to generate mid-log cultures, which were considered as extracellular bacteria. The prepared confluent Caco-2 cells in the 75-cm2 flasks were infected with mid-logarithmic cultures of S. Typhimurium SL1344 and ΔspeG (MOI = 5) for a total of 18 h by using the same protocol as that for obtaining the output pool B in three independent experiments. The Caco-2 cells infected by these two strains of S. Typhimurium were lysed with 1% Triton X-100 to obtain the intracellular bacteria.
Next, polyamines in extracellular bacteria from the mid-log cultures of S. Typhimurium SL1344 and ΔspeG, and the Caco-2 cell lysates containing intracellular bacteria of these two strains were extracted using trichloroacetic acid (TCA; Sigma). The mid-log cultures were centrifuged (4,000 × g) at 4°C for 10 min; the supernatants were removed, and the bacterial pellets were washed with PBS. The centrifugation and PBS washing protocols were performed twice, and the bacterial pellets were resuspended in lysis buffer [20 mM 3-(N-morpholino) propanesulfonic acid, pH 8.0, 10 mM NaCl, and 4 mM MgCl2]. The bacterial cells were lysed through ultrasonic vibration. Finally, 100 μL of 40% TCA was added to the bacterial lysates on ice for 5 min and centrifuged (13,000 × g) at 4°C for 3 min. The supernatants of both extracellular S. Typhimurium strains were decanted and stored at −20°C for high-performance liquid chromatography (HPLC) analysis.
The intracellular bacteria in the Caco-2 cell lysates were filtered using a 7-μm filter to remove the cell debris, and the pellets were resuspended in LB broth under shaking at 225 rpm in 5% CO2 at 37°C for 2 h to amplify the bacterial concentration of the two host cell-primed S. Typhimurium strains. The bacterial pellets were subsequently processed as TCA precipitation and polyamine extraction for the mid-log cultures of both S. Typhimurium strains. Finally, the supernatants of both intracellular strains were collected and stored at −20°C for HPLC.
The standard solutions of putrescine (purity: 99.9%; TCI), spermidine (purity: 99.7%; Fluka), spermine (purity: 99.8%; Fluka), and cadaverine (purity: 98.7%; 1,5-diaminopentane, Fluka), as well as the TCA-treated supernatants of extracellular and intracellular S. Typhimurium SL1344 and ΔspeG were processed before HPLC analysis. Furthermore, 1 mL of 2 N NaOH (Sigma) and 10 μL of benzoyl chloride (Sigma) were added into each of the eight samples, vortexed for 30 s, and incubated with shaking at room temperature for 20 min to generate solutions of benzoyl-polyamines. Subsequently, 2 mL of saturated NaCl (Sigma) was added to the mixtures, which were then vortexed for 30 s. Finally, diethyl ether (Tedia) was added, and the mixtures were vigorously shaken. The solutions were stored at −20°C for 1 h. The diethyl ether phase of the solutions was then collected and incubated at 37°C, and the ether was removed through evaporation. Finally, individual samples were dissolved in 50% methanol (Echo Chemical) and stored at −20°C for 1 h.
The concentrations of TCA-treated extracellular and intracellular bacterial samples were determined through HPLC, as reported previously (Slocum et al., 1989; Lee et al., 2009). The published methods were modified (flow rate: 0.3 mL/min, absorbance detection: 254 nm, and temperature: 25°C) and applied during the mobile phase by using distilled water as buffer A and methanol as buffer B in the solvent gradient conditions as follows: initial, 50% B; 0–40 min, 50–70% B; 40–50 min, 70–50% B; and 50–70 min, 50% B. First, the standard solutions of 80 mM putrescine (purity 99.9%, TCI 110-60-1, 2 mM cadaverine (1,5-diaminopentane purity 98.7%, Fluka FL-33211), 8 mM spermidine (purity 99.7%, Fluka FL85561), and 40 mM spermine (purity 99.8%, Fluka FL85590) were 1:4 diluted and mixed for HPLC analysis to obtain the retention times of putrescine at 35 min, cadaverine at 40 min, spermidine at 54 min, and spermine at 65 min (Supplemetary Figure 1). Next, the five dilutions of the four polyamine standard solutions and the four TCA-treated bacterial samples were filtered using Hypersil ODS C18 Columns (Thermo Scientific) and injected into the HPLC apparatus (Waters 600 controller). Subsequently, the peak area values of the four polyamines in the bacterial samples and the serial dilutions of the standard solutions in the HPLC chromatogram were obtained and analyzed using the Autochro-3000 Chromatography Data System (Young Lin, Taiwan). Finally, the concentrations of the four polyamines in the TCA-treated bacterial samples were calculated by applying their peak area values to the regression equations derived from the five dilutions of the analyzed standard solutions (Supplementary Figure 2). These samples were bracketed with standards in five dilutions, including putrescine (1, 2, 5, 10, and 20 mM; peak areas between 40 and 1,200 mm2), cadaverine (0.1, 0.2, 0.5, 1, and 2 mM; peak areas between 100 and 3,500 mm2), spermidine (0.1, 0.2, 0.5, 1, and 2 mM; peak areas between 50 and 2,500 mm2), and spermine (1, 2, 5, 10, and 20 mM; peak areas between 40 and 1,500 mm2). The concentrations of the individual polyamines were compared between S. Typhimurium SL1344 and ΔspeG before and after their invasion in Caco-2 cells by using the Student's t-test. Simiarly, the polyamine concentrations of extracellular and intracellular bacteria were also compared in S. Typhimurium SL1344 and ΔspeG, respectively. The polyamine concentrations are expressed as mean ± standard error (mM per 109 bacteria). p < 0.05 was considered statistically significant.
Polyamine Suppression Assay
By using the same protocol as that used for obtaining output pool B, confluent Caco-2 cells in 12-well plates were infected with overnight cultures of S. Typhimurium SL1344, and the infected cells were treated with putrescine (625 and 312.5 μM), spermine (375 and 187.5 μM), and cadaverine (125 and 62.5 μM) or left untreated for 15 h. After 18-h incubation, the intracellular bacterial numbers from the treated Caco-2 cells were calculated as prescribed in the bacterial intracellular replication assays and compared between the treated and untreated groups by using the Student's t-test. The data are expressed as mean ± standard error CFU per inoculum of 107 CFU. p < 0.05 was considered statistically significant.
RNA Microarrays of S. Typhimurium SL1344 and ΔspeG before and after Invasion in Caco-2 Cells
Confluent Caco-2 cells in the 75-cm2 flasks were infected with overnight cultures of S. Typhimurium SL1344 or ΔspeG (MOI = 5) in two independent experiments by using the same protocol as that used for obtaining the output pool B. After 18-h incubation, the infected cells were lysed with 1% Triton X-100, and the cell lysates were passed through a 3-μm filter to remove the cell debris. After centrifugation at 800 × g for 10 min and the removal of supernatants, the bacterial pellets were washed with PBS to obtain the intracellular bacteria. The extracellular bacteria from the overnight cultures and the intracellular bacteria from the aforementioned processing of S. Typhimurium SL1344 and ΔspeG were dissolved in TRIzol (Gibco) for isolating the total RNA according to the manufacturer's instruction. The purity of the RNA samples was validated using the ratio of absorbance at 260 and 280 nm, as well as the RNA integrity number determined using Bioanalyzer 2100 (Agilent Technology) with an RNA 6000 Nano LabChip kit (Agilent).
In vitro transcription was performed as previously described (Lee et al., 2009). Briefly, the total RNA samples were reverse transcribed to cDNAs and subsequent cRNAs, which were amplified and labeled with Cy3 (CyDye, Agilent). The Cy3-labeled cRNAs were subsequently fragmented to an average size of 50–100 nucleotides, pooled, and hybridized to Agilent Technologies custom Salmonella GE 8 × 15K microarray that had been tiled with 4,631 gene probes of S. Typhimurium SL1344. After washing the array chips and drying them through nitrogen gun blowing, the microarrays were scanned with an Agilent microarray scanner at 535 nm for Cy3-CTP. The scanned images were quantified and analyzed using Feature Extraction 10.5.1.1 software (Agilent). The background values were corrected using the spatial detrend surface value and were normalized by quantile. Finally, the gene expression in each array group was analyzed using the DAVID database (https://david.ncifcrf.gov/). A heap map with genes in each group that showed more than two-fold upregulation or downregulation was constructed based on their normalized values by using GeneSpring multiomic analysis software (Agilent). The microarray data has been deposited in GEO (http://www.ncbi.nlm.nih.gov/geo/) and is accessible via the GEO Accession Number GSE102885.
The transcriptomes derived from S. Typhimurium SL1344 and ΔspeG were compared before and after their invasion to Caco-2 cells by using the Student's t-test. The data were expressed as mean log2 fold change relative to S. Typhimurium SL1344. p < 0.05, with a fold change of >1 log2 or <−1 log2 was considered statistically significant.
Quantitative Real-Time Polymerase Chain Reaction for Confirmation of RNA Analysis
Pairs of oligonucleotide primers specific to the selected genes identified from the microarrays and the housekeeping gene 16s (Table 1) were designed using Primer3 and BLAST (http://www.ncbi.nlm.nih.gov/tools/primer-blast/). The total RNAs of extracellular and intracellular S. Typhimurium SL1344 and ΔspeG were isolated from their mid-log cultures and lysates of the infected Caco-2 cells by using the Total RNA Miniprep Purification Kit (Genemark, Taichung, Taiwan) according to the manufacturer's instruction. The total RNA samples were purified, and the residual DNA was eliminated using RNase-free DNase I (NEB, Beverly, MA, USA). Next, 0.1 μg of RNA was reverse transcribed to cDNA by using the Transcriptor High Fidelity cDNA Synthesis Kit (Roche Applied Science, Mannheim, Germany), according to the manufacturer's instruction. By using the Bio-Rad C100 Real-Time PCR System, quantitative real-time PCR (qRT-PCR) was performed in triplicate in a reaction volume of 25-μL solution containing 0.2 μM of primer pairs, 12.5 μL of iQ SyBr green supermix (BioRad), 9.5 μL of distilled H2O, and 1 μL of cDNA. The reaction solutions were heated at 95°C for 3 min and amplified for 40 cycles of 95°C for 15 s, 50°C for 30 s, and 72°C for 30 s. The mRNA transcription levels were determined using the ΔΔCt method, as previously described (Wang et al., 2016b), and the expression of 16s ribosomal RNA was considered for normalization. The mRNA expression of the selected genes in extracellular and intracellular S. Typhimurium ΔspeG mutant was compared with that of the same genes in the corresponding extracellular and intracellular S. Typhimurium SL1344 by using the Student's t-test. The data were expressed as mean ± standard error log2 fold change relative to S. Typhimurium SL1344. p < 0.05 was considered statistically significant.
Bacterial Motility Assays
Semisolid LB agar plates containing 0.3% agar were used for performing the bacterial motility assay. Ten microliters of overnight cultures of S. Typhimurium SL1344, ΔspeG, ΔspeG′, ΔspaS, and ΔfliC were inoculated on the centers of the semisolid LB agar plates, followed by incubation at 37°C for 6 h. In this assay, the flagellated non-invasive SPI-1 mutant S. Typhimurium ΔspaS was considered the positive control, whereas the flagella-deficient mutants S. Typhimurium ΔfliC was used as the negative control. Furthermore, 10 μL of overnight cultures of S. Typhimurium SL1344 were inoculated on the centers of the semisolid LB agar plates supplemented with putrescine (625 and 312.5 μM), spermine (375 and 187.5 μM), cadaverine (125 and 62.5 μM), spermidine (6 and 3 μM), or left without any polyamine, and all the plates were incubated at 37°C for 6 h. The intensity of bacterial motility was determined by the diameters of bacterial growth zones. All the above assays were performed in three independent experiments.
Transmission Electron Microscopy
S. Typhimurium SL1344 and ΔspeG were visualized through transmission electron microscopy after negative staining. The overnight bacterial cultures were centrifuged at 5,000 rpm for 20 min, washed with distilled water twice, and fixed with 4% paraformaldehyde for 10 min. The bacteria on the grids were washed with distilled water twice, negatively stained with 2% uranyl acetate for 30 s, and subsequently rinsed with distilled water thrice. Finally, the samples were observed under a transmission electron microscope (FEI Tecnai G2 F20 S-Twin FEG).
Results
speG Affects the Intracellular Replication of S. Typhimurium in Human Cell Lines
To confirm whether speG affects the intracellular proliferation of S. Typhimurium in infected human epithelial cells with time, HeLa cells were infected with S. Typhimurium SL1344 and ΔspeG for 10, 13, and 18 h after killing extracellular bacteria during the third hour postinfection by performing gentamicin protection assays. Although S. Typhimurium ΔspeG showed non-significant attenuation in bacterial proliferation in HeLa cells after 10 and 13 h postinfection (Figure 1A), intracellular S. Typhimurium ΔspeG was significantly attenuated in output pool B in HeLa cells at 18 h postinfection, but not in output pool A at 3 h postinfection (1.1 × 107 vs. 1.9 × 107 CFU/well, p < 0.05; Figure 1B). Moreover, to investigate whether such a phenomenon existed in different cell types, the same in vitro intracellular replication assays were performed in Caco-2, LS174T, and THP-1 cells. We observed that the intracellular concentrations of S. Typhimurium ΔspeG significantly decreased in LS174T cells (1.9 × 107 vs. 2.1 × 107 CFU/well, p < 0.05; Figure 1D) and THP-1 cells (1.0 × 107 vs. 1.3 × 107 CFU/well, p < 0.05; Figure 1E) compared with those of S. Typhimurium SL1344. The results revealed that S. Typhimurium Sl1344 and ΔspeG invaded HeLa, LS174T, and THP-1 cells in similar concentrations, but the deletion of speG significantly attenuated the intracellular concentration of S. Typhimurium in these three cell lines after 18 h. Intracellular S. Typhimurium ΔspeG significantly attenuated in both output pools A and B in Caco-2 cells (3.5 × 105 vs. 2.4 × 105 CFU/well and 1.3 × 106 vs. 8.8 × 106 CFU/well, respectively, p < 0.05; Figure 1C), with significantly higher attenuation of S. Typhimurium ΔspeG than of S. Typhimurium SL1344 in output pool B than in output pool A (9.6 × 105 vs. 6.4 × 105 CFU/well, p < 0.05; Figure 1C). This suggested that the knockout of speG decelerated the intracellular amplification of S. Typhimurium in Caco-2 cells.
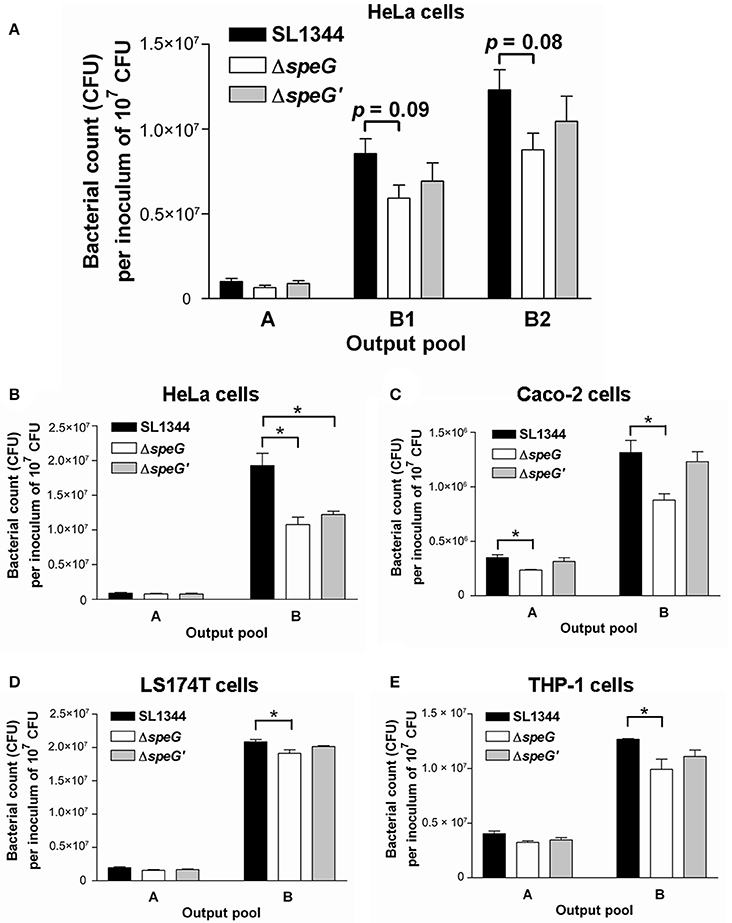
Figure 1. Intracellular bacterial replication assays yielded the intracellular bacterial concentrations of S. Typhimurium SL1344 and its ΔspeG mutant at various time points after invasion in different human cell lines. Human cell lines were infected with S. Typhimurium wild-type SL1344, its ΔspeG mutant, and the speG-complemented strain ΔspeG′ (multiplicity of infection = 5) for 1 h. The extracellular bacteria were killed using gentamicin after another 2 h, and the intracellular bacteria in the infected cells were allowed to proliferate for additional 7 h (output pool B1), 10 h (output pool B2), or 15 h (output pool B). (A) S. Typhimurium ΔspeG was non-significantly attenuated in output pools B1 and B2, in which intracellular bacteria in HeLa cells had been maintained for 10 and 13 h, respectively. (B–E) S. Typhimurium ΔspeG was significantly attenuated in output pool B, in which intracellular bacteria had been maintained in HeLa, Caco-2, LS174T, and THP-1 cells for 18 h, as indicated by asterisks (*p < 0.05; n = 3).
Putrescine, Spermine, and Cadaverine Are the Major Polyamines in S. Typhimurium, Regardless of speG and Internalization in Caco-2 Cells
To quantify the concentrations of putrescine, cadaverine, spermidine, and spermine in S. Typhimurium SL1344 before and after invasion in Caco-2 cells and to investigate whether speG affects their concentrations in S. Typhimurium SL1344 outside and inside Caco-2 cells, as well as extracellular and intracellular S. Typhimurium SL1344, we performed HPLC to quantify these polyamines from the mid-log cultures of both strains and from their 18-h postinfection intracellular concentrations in Caco-2 cells. Our HPLC analysis of the mid-log cultures of S. Typhimurium SL1344 revealed that the concentration of putrescine (47.5 mM), the predominant polyamine, was approximately six times higher than that of spermine (8.0 mM) and approximately 10 times higher than that of cadaverine (4.8 mM). The concentration of spermidine (0.2 mM) was the lowest (Figure 2A). The order of the cellular contents of all these cytoplasmic polyamines was the same in S. Typhimurium after the deletion of speG (Figure 2B), as well as in intracellular S. Typhimurium and ΔspeG (Figures 2C,D). In summary, putrescine, spermine, and cadaverine were the polyamines with the highest concentrations in extracellular and intracellular S. Typhimurium SL1344 and ΔspeG (Figures 2A–D).
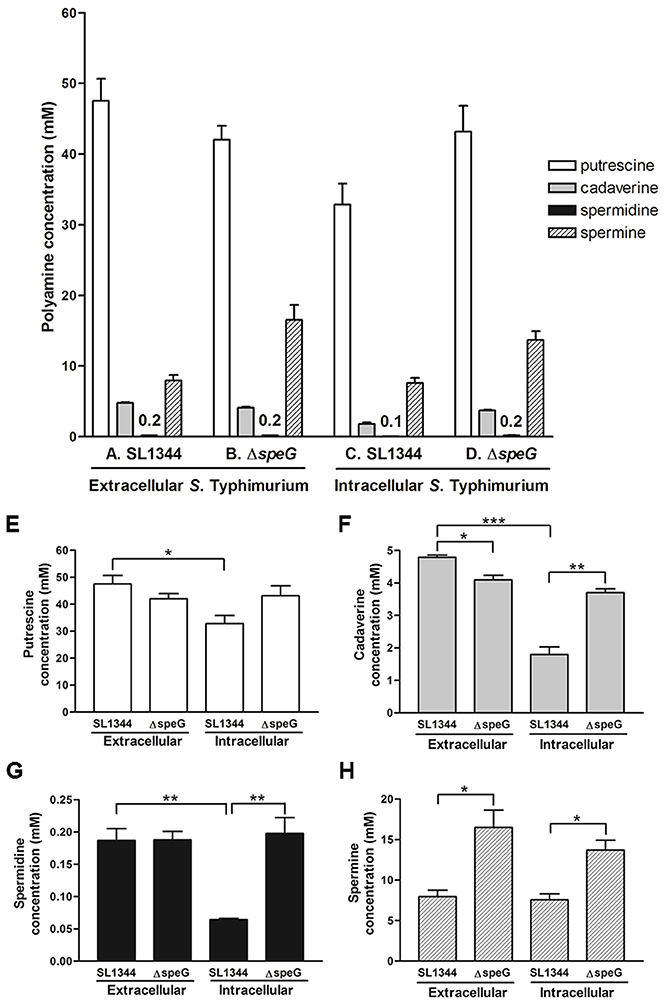
Figure 2. HPLC quantification of cellular polyamines in S. Typhimurium SL1344 and ΔspeG before and after invasion in Caco-2 cells for 18 h. By using the same protocol as that for obtaining output pool B in the intracellular bacterial replication assay, the cellular polyamines of S. Typhimurium SL1344 and ΔspeG before (A,B) and after (C,D) 18-h intracellular internalization in Caco-2 cells were extracted through TCA precipitation and quantified through HPLC for measuring the four major polyamines. The concentration of each polyamine in S. Typhimurium was compared between the SL1344 and ΔspeG strains extracellularly and intracellularly (E–H). For the same strain of SL1344 or ΔspeG, the concentration of each polyamine was also compared between extracellular bacteria from mid-log cultures and intracellular bacteria after invasion in Caco-2 cells. Statistical significances in the comparisons are indicated by asterisks (*p < 0.05, **p < 0.01, and ***p < 0.001; n = 3).
speG Suppresses the Concentration of Spermine but Increases That of Cadaverine in Extracellular S. Typhimurium
To investigate the effects of speG on the contents of polyamines in S. Typhimurium, the HPLC-quantified concentrations of the polyamines in S. Typhimurium SL1344 and ΔspeG were compared. S. Typhimurium ΔspeG contained significantly lower concentrations of cadaverine and higher concentrations of spermine than did S. Typhimurium SL1344 (4.1 vs. 4.8 mM and 16.5 vs. 8.0 mM, respectively; Figures 2F,H), indicating that speG expression might enhance cadaverine production and suppress spermine synthesis. The depletion of speG did not significantly change the content of spermidine in S. Typhimurium.
Biosynthesis of Putrescine, Cadaverine, and Spermidine Is Reduced in Intracellular S. Typhimurium in Caco-2 Cells and speG is Involved in Inhibition of Polyamine Production in Intracellular S. Typhimurium
After bacterial invasion in Caco-2 cells for 18 h, the concentrations of putrescine, cadaverine, and spermidine significantly decreased in S. Typhimurium SL1344 (32.8 vs. 47.5 mM, 1.8 vs. 4.8 mM, and 0.2 vs. 0.1 mM, respectively; Figures 2E–G); however, the levels of spermine were similar to those in extracellular S. Typhimurium SL1344 (7.6 vs. 8 mM; Figure 2H). Briefly, the biosynthesis of purescine, cadaverine, and spermidine was significantly suppressed in S. Typhimurium SL1344 in Caco-2 cells 18 h after invasion. To verify the hypothesis that deficiency in the intracellular replication of S. Typhimurium ΔspeG is due to its quantitative alteration in polyamines, the concentrations of four polyamines were compared between intracellular S. Typhimurium SL1344 and ΔspeG. We observed that the concentrations of putrescine, spermine, and cadaverine were higher in intracellular S. Typhimurium ΔspeG than in intracellular S. Typhimurium SL1344 (Figures 2C,D). Briefly, the deletion of speG significantly increased the concentrations of cadaverine, spermidine, and spermine in intracellular S. Typhimurium in Caco-2 cells for 18 h (3.7 vs. 1.8 mM, 0.2 vs. 0.1 mM, and 13.7 vs. 7.6 mM, respectively; Figures 2F–H) and non-significantly increased the concentration of putrescine (43.2 vs. 32.8 mM, p = 0.093; Figures 2E). These results suggested that speG can inhibit the biosynthesis of polyamines in intracellular S. Typhimurium, with a different tendency for modulating putrescine, cadaverine, and spermidine compared with extracellular S. Typhimurium.
Intracellular Proliferation of S. Typhimurium Is Dose-Dependently Suppressed by Putrescine, Cadaverine, and Spermine
Because non-replicating intracellular S. Typhimurium ΔspeG contains higher concentrations of putrescine, spermine, and cadaverine, we hypothesized that the accumulation of these polyamines inhibits the intracellular replication of S. Typhimurium SL1344. A polyamine suppression assay similar to the 18-h intracellular replication assay was performed by infecting Caco-2 cells with S. Typhimurium SL1344 for 2 h, followed by 1-h treatment with gentamicin to kill extracellular bacteria and 15-h coincubation of Caco-2 cells with intracellular S. Typhimurium SL1344 and the three polyamines in two estimated concentrations. The HPLC analysis of polyamines in intracellular S. Typhimurium ΔspeG was conducted after 2-h amplification through the shaking incubation of bacteria released from lysed Caco-2 cells, and the doubling time of S. Typhimurium was 20–30 min. Therefore, the estimated concentrations of putrescine (625 and 312 μM), cadaverine (125 and 62.5 μM), and spermine (375 and 187.5 μM) were 25 to 26 times diluted compared with their corresponding concentrations (43.2, 3.7, and 13.7 mM) in the HPLC quantification (intracellular S. Typhimurium ΔspeG; Figure 2D). Intracellular S. Typhimurium SL1344 (1.1 × 108 CFU per inoculum of 107 CFU) was significantly suppressed by 625 and 312.5 μM of putrescine (7.2 × 107 CFU per inoculum of 107 CFU, p = 0.006, and 8.5 × 107 CFU per inoculum of 107 CFU, p = 0.04, respectively), 125 μM of cadaverine (7.7 × 107 CFU per inoculum of 107 CFU, p = 0.017), and 375 and 187.5 μM of spermine (7.1 × 107 CFU per inoculum of 107 CFU, p = 0.015 and 9 × 107 CFU per inoculum of 107 CFU, p = 0.04, respectively). The concentration of intracellular S. Typhimurium SL1344 was non-significantly lower in Caco-2 cells treated with 62.5 μM of cadaverine (9.2 × 107 CFU per inoculum of 107 CFU, p = 0.138) than that of Caco-2 cells not treated with polyamines (Figure 3). Altogether, S. Typhimurium SL1344 was dose-dependently suppressed by putrescine, cadaverine, and spermine (Figure 3).
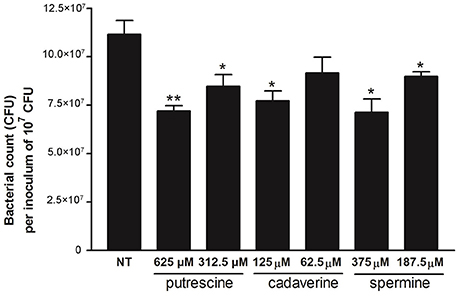
Figure 3. Polyamine suppression assays of putrescine, cadaverine, and spermine in intracellular replication of S. Typhimurium SL1344 in Caco-2 cells. By using the same protocol as that for obtaining output pool B of the intracellular bacterial replication assay, confluent Caco-2 cells in 12-well plates were infected with overnight cultures of S. Typhimurium SL1344, and the infected cells were treated with putrescine, spermine, and cadaverine during the last 15 h in concentrations estimated from the HPLC quantification analysis. Statistical significances in the intracellular bacterial concentrations of S. Typhimurium SL1344 between the treated and untreated groups are indicated by asterisks (*p < 0.05 and **p < 0.01; n = 3).
speG Is Involved in Suppressing Upregulation of Genes Associated with Periplasmic Nitrate Reductase System, Glucarate Metabolism, Phosphotransferase System, Cytochromes, and Succinate Reductase Complex
To investigate the effects of speG on the expression of other genes in S. Typhimurium, the entire RNA transcriptome of S. Typhimurium ΔspeG was compared with that of S. Typhimurium SL1344 (Data Sheet 1). In the mid-log cultures, 29 genes were significantly upregulated in S. Typhimurium ΔspeG compared with S. Typhimurium SL1344, including yojF and napF, which are involved in the periplasmic nitrate reductase system; ygcX, ygcZ, garL, garR, and SL1344_2942, which are associated with glucarate metabolism; SL1344_3736 and SL1344_4467, which are related to the phosphotransferase system; cyoA, cyoB, and cyoC, which encode cytochrome-related proteins; and sdhA, sdhB, sdhC, and sdhD, which are involved in the succinate reductase complex (Table 2A). Moreover, narK, malK, ybfM, SL1344_2997, SL1344_3662, dctA, hutU, and hutH as well as five genes encoding hypothetical proteins were significantly upregulated (Table 2A). Thus, speG could be involved in suppression of the expression of these significantly upregulated genes in S. Typhimurium.
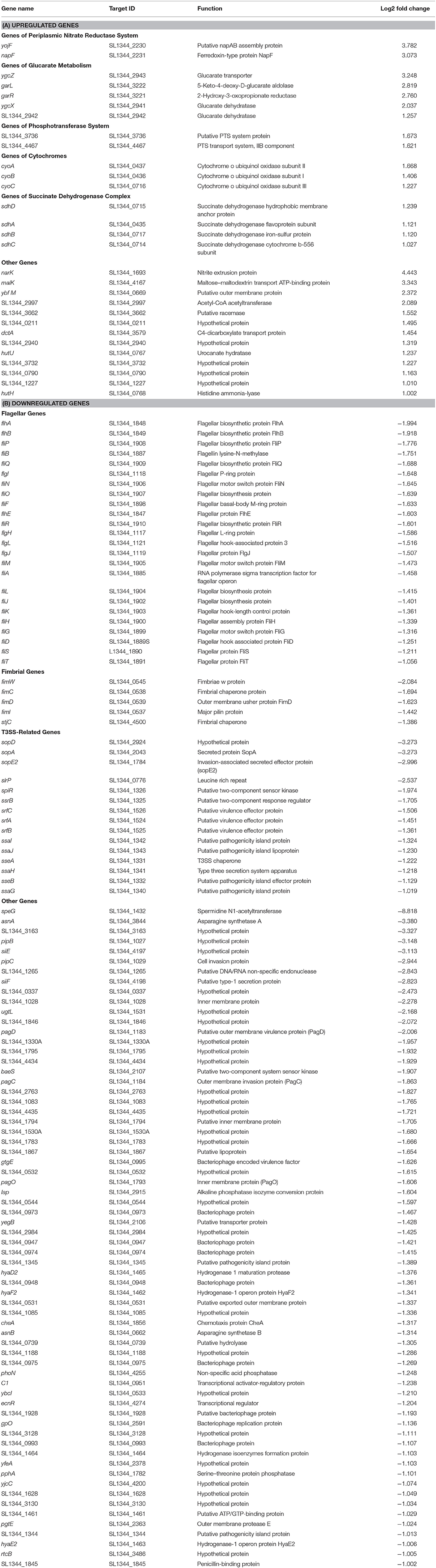
Table 2. The 29 significantly upregulated genes (A) and the 110 significantly downregulated genes (B) of the S. Typhimurium ΔspeG mutant compared with its parental wild-type strain SL1344.
speG Is Involved in Flagellar Biosynthesis, Fimbrial Expression, and T3SS and T1SS Functions
In the mid-log cultures, 110 genes were significantly downregulated in S. Typhimurium ΔspeG compared with S. Typhimurium SL1344 in the RNA microarrays (Table 2B). First, speG was the most significantly downregulated gene (−8.818 log2 fold change) in S. Typhimurium ΔspeG, validating the successful deletion of speG in its parental wild-type S. Typhimurium SL1344 (Table 2B). However, speG deletion did not significantly affect the expression of genes involved in polyamine metabolism, namely speA (−0.52 log2 fold change, p = 0.44), speB (−0.07 log2 fold change, p = 0.91), speC (0.99 log2 fold change, p = 0.22), speD (−0.28 log2 fold change, p = 0.70), speE (−0.21 log2 fold change, p = 0.88), cadA (−0.99 log2 fold change, p = 0.46), and metK (−0.51 log2 fold change, p = 0.76).
The transcriptome of the S. Typhimurium ΔspeG mutant relative to its parental wild-type strain SL1344 revealed that the protein functions of the significantly downregulated genes include flagellar biosynthesis, fimbrial chaperone, pathogenicity island 4 (e.g., siiE), and type I secretion system (e.g., siiF; Table 2B), suggesting the involvement of speG in the flagellar motility regulation of S. Typhimurium. Moreover, many T3SS-related genes were significantly downregulated after speG deletion in S. Typhimurium SL1344 (Table 2B). These RNA microarray results were confirmed through qRT-PCR, which demonstrated significantly downregulated mRNA expression of six flagellar genes (flhA, flhB, fliP, fliQ, flgI, and fliH) and four fimbrial genes (fimC, fimD, fimI, and fimW; Figure 4A).
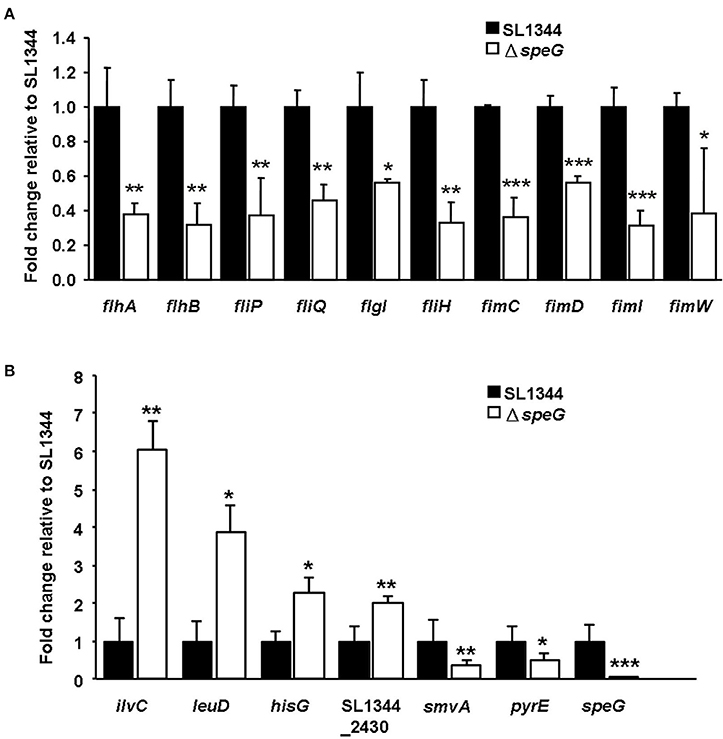
Figure 4. Quantitative real-time polymerase chain reaction analysis of 17 selected genes for validation of the RNA microarray data. (A) The mRNA expression levels of the six flagellar genes and four fimbrial genes were significantly downregulated in S. Typhimurium ΔspeG relative to its parental wild-type strain SL1344. (B) The mRNA expressions of ilvC, leuD, hisG, and SL1344_2430 were significantly upregulated and those of smvA, pyrE, and speG were significantly downregulated in intracellular S. Typhimurium ΔspeG compared with S. Typhimurium SL1344 after invasion in Caco-2 cells for 18 h. Statistical significances in mRNA expression of the selected genes between S. Typhimurium SL1344 and ΔspeG are indicated by asterisks (*p < 0.05, **p < 0.01, ***p < 0.001; n = 3).
speG Is Involved in Suppressing Upregulation of Genes in the ilv–leu Operon and Histidine Operon of Intracellular S. Typhimurium after Invasion in Caco-2 Cells
To understand whether speG affects the gene expression of intracellular S. Typhimurium, the transcriptomes of intracellular S. Typhimurium SL1344 and its ΔspeG mutant were compared after internalization in Caco-2 cells for 18 h. Compared with the expression pattern in intracellular S. Typhimurium SL1344, 1,964 genes were upregulated and 2,664 genes were downregulated in the intracellular S. Typhimurium ΔspeG mutant (Data Sheet 2). The depletion of speG led to the significant upregulation of 11 genes in intracellular S. Typhimurium, namely ilvC, ilvM, ilvG, ilvA, leuD, leuC, and leuB in the ilv–leu operon and hisG and hisC in the histidine operon (Table 3A). Only four genes, namely speG, smvA, pyre, and citD2, were significantly downregulated in the intracellular S. Typhimurium ΔspeG mutant (Table 3B). The RNA microarray results were validated through qRT-PCR, which revealed the significantly upregulated mRNA expression of four genes (ilvC, leuD, hisG, and SL1344_2430) and significantly downregulated mRNA expression of three genes (smvA, pyrE, and speG; Figure 4B).
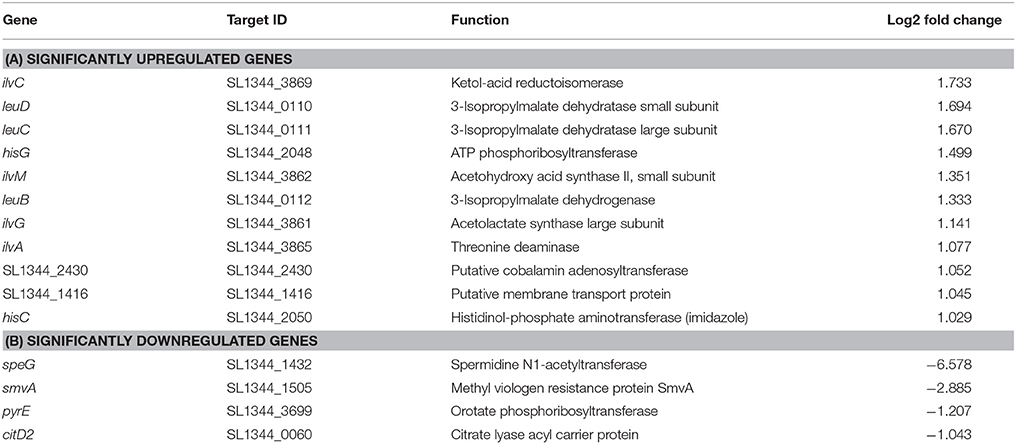
Table 3. The 11 significantly upregulated genes (A) and the four significantly downregulated genes (B) of the intracellular S. Typhimurium ΔspeG mutant compared with its parental wild-type strain SL1344 within Caco-2 cells.
speG Affects Flagellation and Motility of S. Typhimurium and Exogenous Polyamins Attenuate Motility of S. Typhimurium
To validate the RNA microarray data on the involvement of speG in flagellar biosynthesis, the bacterial morphology and motility of S. Typhimurium SL1344 and ΔspeG were examined. Transmission electron micrographs after negative staining revealed sparse defective flagella in S. Typhimurium ΔspeG compared with the wild-type SL1344 strain (Figure 5). Moreover, the motility of S. Typhimurium ΔspeG was poorer than that of the wild-type SL1344 strain but better than that of S. Typhimurium ΔfliC (Figure 6). The bacterial motility was restored after speG complementation in the ΔspeG mutant (Figure 6C), suggesting the influence of speG on bacterial motility. In addition, the motility of S. Typhimurium SL1344 was dose-dependently attenuated by exogenous putrescine, cadaverine, and spermidine (Figure 7).
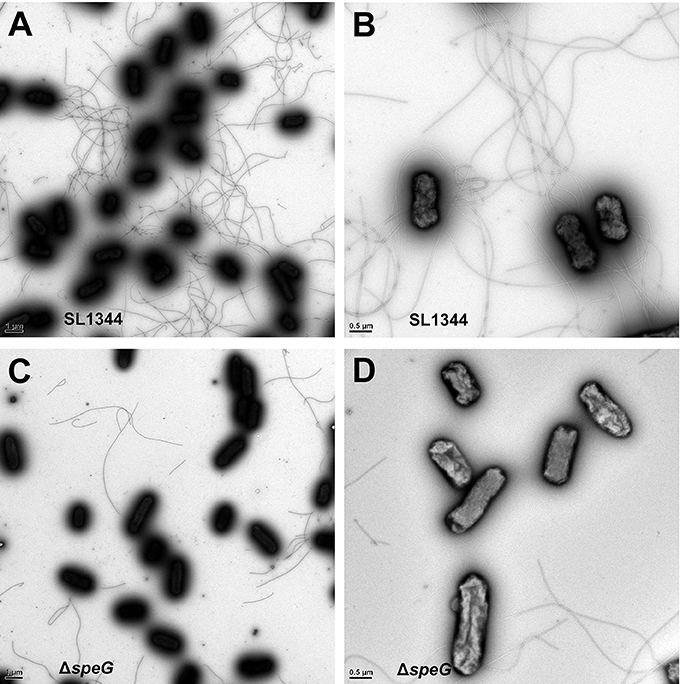
Figure 5. Transmission electron micrographs of S. Typhimurium SL1344 and ΔspeG. Transmission electron micrographs after negative staining revealed the morphology of (A,B) S. Typhimurium SL1344 and (C,D) ΔspeG. Numerous long flagella were observed in S. Typhimurium SL1344 [magnification: (A) 10,000× and (B) 22,500×]. Only a small number of fragmented flagella were observed in S. Typhimurium ΔspeG [magnification: (C) 10,000× and (D) 22,500×].
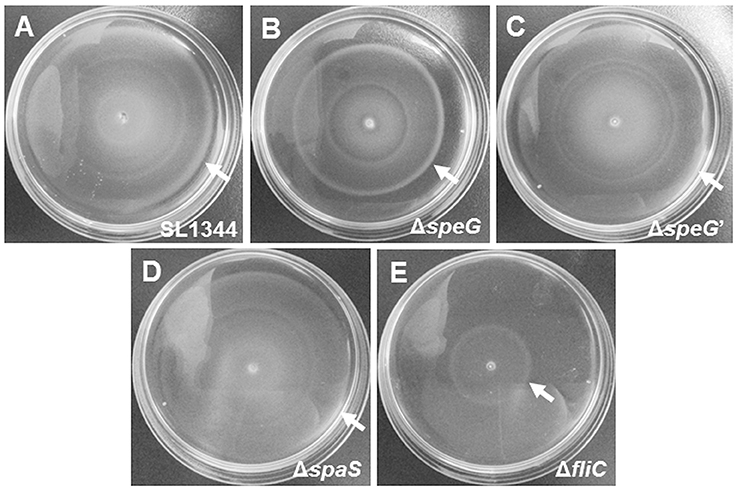
Figure 6. Bacterial motility assays of S. Typhimurium SL1344 and ΔspeG. The motilities of S. Typhimurium SL1344 (A), ΔspeG (B), ΔspeG′ (C), ΔspaS (D), and ΔfliC (E) were examined by bacterial inoculation on semisolid agar plates with 6-h incubation at 37°C. S. Typhimurium SL1344, ΔspeG′, and ΔspaS similarly exhibited the maximal diameters of their motility zones. The motility zone of ΔspeG was smaller than that of the aforementioned strains, but still larger than that of ΔfliC.
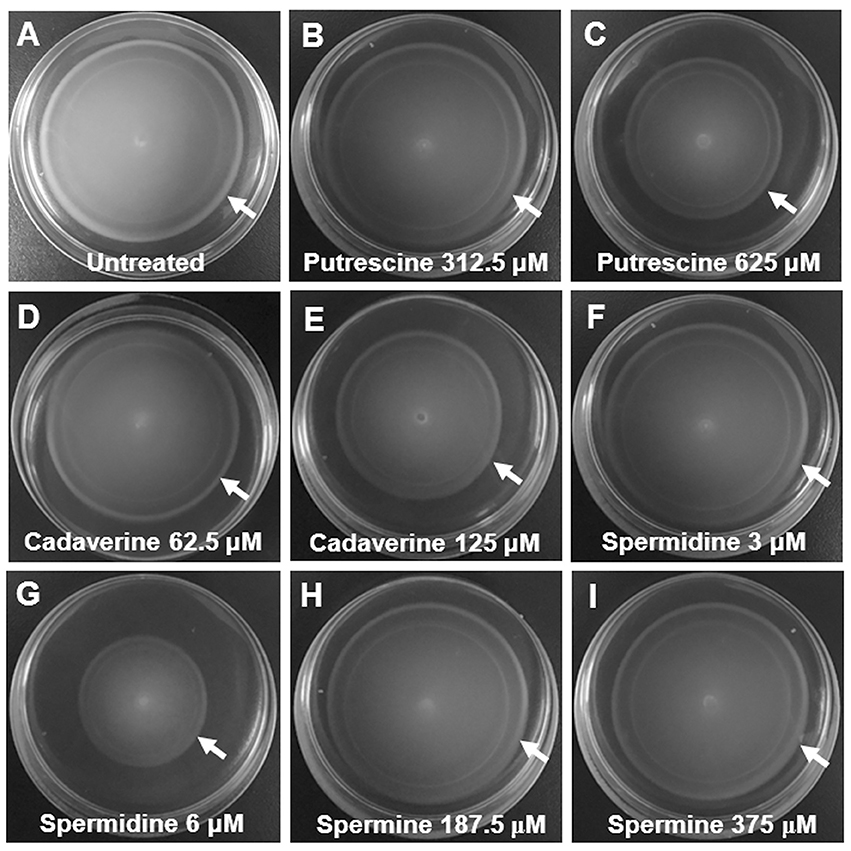
Figure 7. Polyamine-suppressing bacterial motility assays of S. Typhimurium SL1344. The motilities of S. Typhimurium SL1344 were examined by bacterial inoculation on semisolid agar plates supplemented with four polyamines after 6-h incubation at 37°C. The motility zones of S. Typhimurium SL1344 in the semisolid LB agar plates supplemented with putrescine (625 μM; C), cadaverine (125 μM; E), and spermidine (6 μM; G) were smaller than that of S. Typhimurium SL1344 in the plates containing no polyamine (A). The motility zones of S. Typhimurium SL1344 were slightly inhibited by cadaverine (62.5 μM; D), but not suppressed by putrescine (312.5 μM; B), spermidine (3 μM; F), and two concentrations of spermine (187.5 μM; H and 375 μM; I) in the semisolid LB agar plates.
Discussion
According to our review of relevant literature, the present study is the first to demonstrate that speG is required for the intracellular replication of Salmonella in human non-phagocytic cells. Our results revealed that the effect of speG on the intracellular replication of S. Typhimurium in HeLa cells is time dependent (Figure 1A). Moreover, we observed that speG influenced the intracellular replication of S. Typhimurium in four cell lines as hosts (Figures 1B–E). Although the speG mutant was attenuated in bacterial invasion in Caco-2 cells, it was more significantly attenuated in bacterial replication after 18-h internalization in Caco-2 cells (Figure 1C), suggesting the effect of speG on the suppression of intracellular bacterial replication. The depletion of speG did not affect the internalization of S. Typhimurium in THP-1 cells (Figure 1E), which indicated that the speG phenotype is mainly exhibited within host cells rather than outside the host cells. In E. coli, speG encodes SAT, which catalyzes spermidine to N1- or N8-acetylspermidine (Igarashi and Kashiwagi, 2010; Barbagallo et al., 2011; Jelsbak et al., 2012), which can be secreted by the bacteria to inactivate the toxicity of excessive spermidine (Fukuchi et al., 1995) and can reduce intracellular polyamine levels to prevent spermidine toxicity under stressful growth conditions (Limsuwun and Jones, 2000). The amino acid sequence of SAT in E. coli is similar to that in Enterococcus faecalis, Pseudomonas aeruginosa plasmid pSCH884, Klebsiella pneumoniae, Serratia marcessencens, and Agrobacterium tumefaciences (Fukuchi et al., 1994). However, speG is not present in all bacteria and is lost in Shigella, resulting in higher concentrations of endogenous spermidine (Barbagallo et al., 2011). Although the absence of speG enhances the survival of Shigella to oxidative stress and the adverse environments inside macrophages (Barbagallo et al., 2011), our study demonstrated the requirement of speG for the intracellular replication of S. Typhimurium in human macrophages and epithelial cells. speG deletion did not affect the ability of Shigella flexneri to infect HeLa cells (Barbagallo et al., 2011), but it caused spermidine accumulation to reduce the intracellular viability of E. coli (Fukuchi et al., 1995). These observations are consistent with our results that the loss of speG in S. Typhimurium did not impair bacterial invasion in HeLa cells but attenuated its intracellular replication (Figure 1). Such a phenotype of speG associated with the intracellular replication of S. Typhimurium is significantly expressed after bacterial invasion in cells for a sufficiently long duration (Figures 1B–E) rather than for short durations (Figure 1A), possibly because of the time-dependent accumulation of intracellular metabolites caused by impaired SAT synthesis in the speG mutant. Therefore, whether the accumulation of spermidine in Salmonella is detrimental to bacterial intracellular proliferation expedited our subsequent quantification of the four major polyamines in S. Typhimurium and its speG-deleted mutant outside and inside Caco-2 cells.
This study was the first to report that putrescine, spermine, and cadaverine are the polyamines with the highest concentrations and that the concentration of spermidine is the lowest among the four major polyamines in S. Typhimurium, regardless of whether speG is in the bacterial genome. Our HPLC analysis indicated that putrescine is the predominant polyamine in S. Typhimurium SL1344, followed by spermine and cadaverine. The same order was observed in extracellular S. Typhimurium ΔspeG, as well as in intracellular S. Typhimurium SL1344 and ΔspeG in Caco-2 cells (Figure 2). Consistent with our result, a recent study used gas chromatography mass spectrometry and reported that putrescine has the highest positive loading in intracellular profiles of S. Typhimurium, just second to succinic acid (Wong et al., 2015). Similar to E. coli, putrescine is the most abundant polyamine in S. Typhimurium. It is the main constituent of the outer membrane of both bacteria (Koski and Vaara, 1991; Cohen, 1997; Shah and Swiatlo, 2008). Moreover, we observed that S. Typhimurium contains a high concentration of spermine and cadaverine, both of which are scarce or absent in E. coli (Cohen, 1997; Shah and Swiatlo, 2008). This study was the first to address the relevance of spermine and cadaverine in invasive bacteria such as Salmonella. Shigella and all enteroinvasive E. coli strains lack lysine decarboxylase for the decarboxylation of lysine to synthesize cadaverine, a small polyamine that inhibits the inflammation induced by dysentery bacteria (Casalino et al., 2003). Therefore, the high cellular levels of cadaverine suppressing host inflammation could explain the resistance of Salmonella to the intracellular immunity of host cells. Piperidine, a cadaverine metabolite, dose-dependently inhibits the virulence of S. Typhimurium during pathogen-host interactions in vitro and in vivo (Kohler et al., 2002). Further studies must determine how the orchestration of endogenous and exogenous cadaverine modulates the pathogenesis of Salmonella. In contrast to the loss of speG in Shigella inducing the accumulation of intracellular spermidine, which favors bacterial survival under oxidative stress conditions (Campilongo et al., 2014), the intracellular proliferation and survival of S. Typhimurium is independent of the accumulation of intracellular spermidine because it has considerably lower concentration of spermidine than of the polyamines in bacterial cells (Figure 2). Furthermore, numerous cellular transport systems can regulate the levels of polyamine in bacteria. E. coli exerts polyamine import by the spermidine-preferential PotABCD, putrescine-specific PotFGHI and PuuP transporters (Kashiwagi et al., 1993; Igarashi et al., 2001; Kurihara et al., 2009), putrescine–ornithine exchange by PotE (Kashiwagi et al., 1997), cadaverine–lysine exchange by CadB (Soksawatmaekhin et al., 2004), spermidine excretion by MdtJI (Higashi et al., 2008), and pustrescine export by SapBCDF (Sugiyama et al., 2016). Nevertheless, speG did not significantly affect the expression of these genes in S. Typhimurium in our study.
speG-regulated polyamine synthesis in S. Typhimurium varies before and after invasion in human intestinal epithelial cells. Our HPLC analysis indicated that the depletion of speG significantly and constantly increased the concentrations of spermine before and after invasion in Caco-2 cells (Figures 2B,D), suggesting that speG affects the inhibition of spermine synthesis without being influenced by the intracellular environments of host cells. Contrastingly, the effect of speG on intracellular S. Typhimurium after 18-h internalization in Caco-2 cells exhibited a different phenotype by modulating the cytoplasmic contents of putrescine, cadaverine, and spermidine, namely the intracellular inhibition of the accumulation of these polyamines. The knockout effect of speG on the suppression of the contents of putrescine and cadaverine in extracellular S. Typhimurium was the reverse of the enhancement of these polyamines after the invasion of S. Typhimurium in Caco-2 cells. speG did not influence the concentration of spermidine in extracellular S. Typhimurium but significantly increased it in intracellular S. Typhimurium. Until now, most studies have investigated polyamines in E. coli. In E. coli, putrescine is synthesized as in mammalian cells via two pathways, either by the catalyzation of L-ornithine by ornithine decarboxylase (SpeC/SpeF), as in mammalian cells, or by the catalyzation of L-arginine by arginine decarboxylase (SpeA) into agmatine, which is further catalyzed by agmatine ureohydrolase (SpeB). However, spermidine is synthesized from putrescine and decarboxylated S-adenosylmethonine catalyzed by spermidine synthase (SpeE), with the simultaneous metabolization of decarboxylated S-adenosylmethonine to methylthioadenosine. S-adenosylmethonine decarboxylase (SpeD) catalyzes the synthesis of decarboxylated S-adenosylmethonine from S-adenosylmethonine that is originally metabolized from its upstream precursor L-methionine catalyzed by MetK with ATP consumption. Theoretically, the impaired function of SpeG leads directly to the accumulation of spermidine or indirectly to its precursor metabolites, namely putrescine, agmatine, arginine, or ornithine. Unlike the loss of speG in Shigella that results in higher concentrations of endogenous spermidine (Barbagallo et al., 2011), our study indicated that the genuine speG effect is mainly dependent on spermine, rather than on spermidine, in both extracellular and intracellular S. Typhimurium, with its versatile modulation on putrescine and cadaverine affected by the intracellular environment of host cells. Considering our finding regarding the effect of speG on the intracellular replication of S. Typhimurium, we hypothesized that the loss of speG could result in the marked accumulation of putrescine, spermine, and cadaverine in intracellular S. Typhimurium or affect the expression of other Salmonella virulence genes via an unknown pathway to hinder intracellular bacterial replication.
The accumulation of cellular polyamines, including putrescine, spermine, and cadaverine, impedes the intracellular replication of Salmonella in human intestinal epithelial cells. Polyamines are indispensable for normal cell growth and affect the stimulation of cell division and proliferation; gene expression for the survival of cells, the regulation of apoptosis, oxidative stress, cell–cell communication, and the synthesis and functions of DNA and protein synthesis, particularly RNA because most of cellular polyamines exist in a polyamine–RNA complex in cells (Igarashi and Kashiwagi, 2010; Lenis et al., 2017). However, studies have rarely determined whether insufficient or excessive polyamines are detrimental to bacterial proliferation. Decreased concentrations of spermidine and putrescine reduce the growth rate of E. coli (Cunningham-Rundles and Maas, 1975; Xie et al., 1993). A study reported that exogenous spermine dose-dependently inhibits the in vitro growth of S. Typhimurium, E. coli, and Staphylococcus aureus, but not P. aeruginosa (Kwon and Lu, 2007). To validate our observation of the increased polyamine contents of non-replicating intracellular S. Typhimurium ΔspeG, we demonstrated that the intracellular proliferation of S. Typhimurium was dose-dependently suppressed by putrescine, cadaverine, and spermine in the concentrations estimated from our earlier HPLC analysis (Figure 3). Recent studies have reported several cellular mechanisms controlling the levels of intracellular polyamines fine-tuned by their biosynthesis, catabolism, and transport at the transcription, translation, and protein degradation levels involving feedback loops controlled by polyamine concentrations. These mechanisms include the transcriptional and translational control of ornithine decarboxylase, regulation of polyamine synthesis by antizyme levels, catalyzation of S-adenosylmethionine into decarboxylated S-adenosylmethonine for synthesizing spermidine and spermine from putrescine, controlling polyamine levels through catabolism by oxidation or acetylation to maintain their cellular activity or concentration, and controlling polyamine levels through transport (Filippou et al., 2007; Igarashi and Kashiwagi, 2010; Miller-Fleming et al., 2015; Lenis et al., 2017). Furthermore, the function of polyamines in S. Typhimurium had remained largely unknown until the transcriptome of intracellular S. Typhimurium revealed the upregulation of genes for putrescine and spermidine biosyntheses during the infection of epithelial cells and macrophages, suggesting an important role of polyamines in bacterial invasion and intracellular survival (Eriksson et al., 2003; Hautefort et al., 2008; Di Martino et al., 2013). A polyamine mutant of S. Typhimurium with speB, speC, speE, and speF deletions exhibited a defective invasion of epithelial cells and attenuation in intracellular replication compared with its wild-type counterpart and the typhoid mouse model (Jelsbak et al., 2012). This defective intracellular replication was enhanced by the complementation of speB in the polyamine mutant and exogenous putrescine and spermidine in the culture media before the infection of the cell cultures (Jelsbak et al., 2012). These results indicated a critical role of putrescine and spermidine in controlling virulence in S. Typhimurium, most likely through the upregulation of essential virulence loci in SPI-1 and SPI-2 (Jelsbak et al., 2012). However, the genotypes of speG in bacterial virulence and their role in regulating the toxicity of excessive polyamines in Salmonella remain unclear.
The relationship of polyamines with bacterial flagellation and motility in Salmonella has rarely been reported. Polyamines may be involved in the intracellular virulence of S. Typhimurium. An early study reported that a housekeeping gene, sifA, located within the potABCD operon and involved in the periplasmic transport of polyamines, is required for synthesizing Salmonella-induced filaments in epithelial cells and for in vivo virulence in mice (Stein et al., 1996). Our study demonstrated that speG contributes not only to intracellular replication in host cells but also the flagellar biosynthesis and swimming motility of S. Typhimurium, suggesting that the speG-associated metabolism of polyamines accounts for bacterial morphology and motility and is associated with intracellular growth. In addition, we demonstrated remarkable dose-dependent suppression of Salmonella motility by exogenous putrescine, cadaverine, and spermidine. However, speG did not significantly affect the expression of other polyamine genes, suggesting that speG affects the flagellation of Salmonella independently of speA, speB, speC, speD, speE, cadA, and metK. The production of flagellar protein requires the concomitant synthesis of RNA in S. Typhimurium (Aamodt and Eisenstadt, 1968). Because polyamines in a polyamine–RNA complex in cells are essential for RNA synthesis and functions (Igarashi and Kashiwagi, 2010; Lenis et al., 2017), it is reasonably assumed that the unbalanced metabolism of polyamines could interfere with the flagellation of S. Typhimurium. A recent proteomic study confirmed the suppression of bacterial flagellation, chemotaxis, SPI-1, TCA cycle, and anaerobic respiration pathways in the metabolic reshuffling of intracellular-replicating Salmonella in infected HeLa cells (Liu et al., 2017). Furthermore, the defective intracellular replication of the polyamine mutants with speB, speC, speE, and speF deletions was in concordance with the downregulation of SPI-1 and SPI-2 genes in S. Typhimurium (Jelsbak et al., 2012). Consistently, our microarray study demonstrated the significant downregulation of SPI-1 genes (e.g., sopD, sopA, and sopE2) and SPI-2 genes (e.g., sseA, ssaH, sseB, and ssaG) through speG deletion in S. Typhimurium (Table 2B). Altogether, polyamines are required for the induction of SPI-1 and SPI-2 for Salmonella flagellation and motility, and they function as an environmental stimulus to prime S. Typhimurium for intracellular proliferation in human epithelial cells.
speG can significantly regulate the expression of different genes before and after the internalization of S. Typhimurium in human intestinal epithelial cells. For S. Typhimurium outside host cells, speG is required for the expression of the well-documented genes encoding flagella, fimbria, and T3SS, as well as for the expression of 66 other genes, including those involved in the biosynthesis of inner and outer membranes, bacteriophages, and hydrogenase-1 operon proteins and a few genes encoding hypothetical proteins (Table 2B). Moreover, speG can suppress the upregulation of several groups of genes involved in the periplasmic nitrate reductase system (yojF and napF), glucarate metabolism (e.g., garL and garR), the phosphotransferase system (SL1344_3736 and SL1344_4467), cytochromes (cyoABC), and the succinate reductase complex (sdhABCD; Table 2A). These genes have rarely been reported to correlate with the polyamine metabolism and intracellular replication of Salmonella. The nap genes encoding periplasmic nitrate reductase can be upregulated under low nitrate conditions from aerobic to anaerobic metabolism to contribute to the luminal growth and virulence of Salmonella in vivo (Paiva et al., 2009; Rowley et al., 2012; Lopez et al., 2015). Meanwhile, speG could be involved in carbon metabolism for energy because garL and garR correlate with hydrogen-stimulated carbon acquisition for hydrogen-dependent growth (Lamichhane-Khadka et al., 2011). speG modulates SPI-1 virulence because phosphotransferase controls the global transcription regulator Mlc for the complete expression of hilA, hilD, and invF (Poncet et al., 2009). Similar to the upregulation of cyoA, cyoB, and cyoC after speG deletion in S. Typhimurium in our study (Result 3.6), cytochrome bo oxidase genes (cyoA, cyoB, and cyoC) were significantly upregulated on exposure to nitrogen oxide stress, which reduces their vulnerability to oxidative injury (Calderon et al., 2014). Therefore, without speG expression, S. Typhimurium tends to lose protection against oxidative injury by activating cytochrome bo oxidase. In our study, speG significantly regulated the expression of four genes encoding the four subunits of the succinate dehydrogenase complex, which is the only enzyme participating in both the electron transport chain and TCA cycle (Oyedotun and Lemire, 2004). Altogether, speG can influence a broad spectrum of bacterial metabolisms involving reduction, oxidation, and energy consumption in addition to influencing known virulence factors. In Caco-2 cells, the ilv–leu operon (ilvA, ilvC, ilvG, ilvM, leuB, leuC, and leuD) and the histidine operon (hisC and hisG) were significantly upregulated after speG deletion in intracellular S. Typhimurium (Table 3A), suggesting the inhibitory function of both operons in the intracellular replication of Salmonella. Mutations in hisG trigger the intracellular filamentous growth of S. Typhimurium and bacterial cell division in eukaryotic host cells (Henry et al., 2005), which is in concordance with our observation of hisG upregulation in the poorly proliferating speG mutant of intracellular S. Typhimurium in Caco-2 cells.
In conclusion, speG is required for the intracellular replication of S. Typhimurium and is dependent on the duration after bacterial invasion and independent on cell types. Putrescine, spermine, and cadaverine are the predominant polyamines in S. Typhimurium. The effects of speG on the biosynthesis of these polyamines vary outside and inside host cells and affect the expression of previously reported and unreported Salmonella virulence genes involved in a broad spectrum of cellular functions rather than other polyamine-associated genes. Thus, speG plays an independent key role in the polyamine metabolism and virulence regulation of Salmonella.
Ethics Statement
This study was approved by Taipei Medical University-Joint Institutional Review Board (No. 201205007). Neither human participants nor animals were involved in this study.
Author Contributions
S-BF designed the research and wrote the article; C-JH, C-HH, K-CW, N-WC, and H-YP performed the experiments and analyzed the data; S-BF, H-WF, M-TH, and C-KC conceived the experiments and contributed reagents, materials, and analysis tools.
Conflict of Interest Statement
The authors declare that the research was conducted in the absence of any commercial or financial relationships that could be construed as a potential conflict of interest.
Acknowledgments
The study was funded by the Career Development Grant, National Health Research Institutes, Taiwan (NHRI-EX103-10234SC); the Ministry of Science and Technology, Taiwan (NSC 102-2320-B-038-009); and the National Taipei University of Technology-Taipei Medical University Joint Research Program (NTUT-TMU-101-18, NTUT-TMU-102-09). We thank Shih-Min Ding and Ya-Chun Lee for their assistance in the laboratory.
Supplementary Material
The Supplementary Material for this article can be found online at: https://www.frontiersin.org/articles/10.3389/fmicb.2017.02245/full#supplementary-material
Supplemetary Figure 1. The HPLC chromatogram of the mixed four diluted polyamine standards.
Supplemetary Figure 2. The regression equations derived from the five dilutions of four polyamine standards.
Data Sheet 1. The 29 significantly upregulated genes (A) and the 110 significantly downregulated genes (B) of the S. Typhimurium ΔspeG mutant compared with its parental wild-type strain SL1344 (in the order of log2 fold change).
Data Sheet 2. The 1,964 upregulated genes (A) and 2,664 downregulated genes (B) of the intracellular S. Typhimurium ΔspeG mutant compared with the intracellular S. Typhimurium SL1344 after their invasion in Caco-2 cells for 18 h (in the order of log2 fold change).
Abbreviations
ATP, adenosine triphosphate; cDNA, complementary deoxyribonucleic acid; CFU, colony-forming units; DMEM, Dulbecco's modified Eagle medium; DNA, deoxyribonucleic acid; E. coli, Escherichia coli; FBS, fetal bovine serum; HEPES, 4-(2-hydroxyethyl)piperazine-1-ethanesulfonic acid; HPLC, high-performance liquid chromatography; LB, Luria–Bertani; MOI, multiplicity of infection; mRNA, messenger ribonucleic acid; PBS, phosphate-buffered saline; PCR, polymerase chain reaction; qRT-PCR, quantitative real-time polymerase chain reaction; RNA, ribonucleic acid; S. Typhimurium, Salmonella enterica serovar Typhimurium; SAT, spermidine acetyltransferase; SPI, Salmonella pathogenicity island; T1SS, type I secretion system; T3SS, type III secretion system; TCA, trichloroacetic acid.
References
Aamodt, L. W., and Eisenstadt, J. M. (1968). Flagellar synthesis in Salmonella typhimurium: requirement for ribonucleic acid synthesis. J. Bacteriol. 96, 1079–1088.
Barbagallo, M., Martino, M. L., Marcocci, L., Pietrangeli, P., Carolis, E. D., Casalino, M., et al. (2011). A new piece of the Shigella Pathogenicity puzzle: spermidine accumulation by silencing of the speG gene [corrected]. PLoS ONE 6:e27226. doi: 10.1371/journal.pone.0027226
Buckley, A. M., Wang, J., Hudson, D. L., Grant, A. J., Jones, M. A., Maskell, D. J., et al. (2010). Evaluation of live-attenuated Salmonella vaccines expressing Campylobacter antigens for control of C. jejuni in poultry. Vaccine 28, 1094–1105. doi: 10.1016/j.vaccine.2009.10.018
Bueno, S. M., Wozniak, A., Leiva, E. D., Riquelme, S. A., Carreno, L. J., Hardt, W. D., et al. (2010). Salmonella pathogenicity island 1 differentially modulates bacterial entry to dendritic and non-phagocytic cells. Immunology 130, 273–287. doi: 10.1111/j.1365-2567.2009.03233.x
Calderon, P. F., Morales, E. H., Acuna, L. G., Fuentes, D. N., Gil, F., Porwollik, S., et al. (2014). The small RNA RyhB homologs from Salmonella typhimurium participate in the response to S-nitrosoglutathione-induced stress. Biochem. Biophys. Res. Commun. 450, 641–645. doi: 10.1016/j.bbrc.2014.06.031
Campilongo, R., Di Martino, M. L., Marcocci, L., Pietrangeli, P., Leuzzi, A., Grossi, M., et al. (2014). Molecular and functional profiling of the polyamine content in enteroinvasive E. coli: looking into the gap between commensal E. coli and harmful Shigella. PLoS ONE 9:e106589. doi: 10.1371/journal.pone.0106589
Cano, D. A., Martinez-Moya, M., Pucciarelli, M. G., Groisman, E. A., Casadesus, J., and Garcia-Del, P. F. (2001). Salmonella enterica serovar Typhimurium response involved in attenuation of pathogen intracellular proliferation. Infect. Immun. 69, 6463–6474. doi: 10.1128/IAI.69.10.6463-6474.2001
Casalino, M., Latella, M. C., Prosseda, G., and Colonna, B. (2003). CadC is the preferential target of a convergent evolution driving enteroinvasive Escherichia coli toward a lysine decarboxylase-defective phenotype. Infect. Immun. 71, 5472–5479. doi: 10.1128/IAI.71.10.5472-5479.2003
Chaudhuri, R. R., Morgan, E., Peters, S. E., Pleasance, S. J., Hudson, D. L., Davies, H. M., et al. (2013). Comprehensive assignment of roles for Salmonella Typhimurium genes in intestinal colonization of food-producing animals. PLoS Genet. 9:e1003456. doi: 10.1371/journal.pgen.1003456
Coburn, B., Grassl, G. A., and Finlay, B. B. (2007). Salmonella, the host and disease: a brief review. Immunol. Cell Biol. 85, 112–118. doi: 10.1038/sj.icb.7100007
Cunningham-Rundles, S., and Maas, W. K. (1975). Isolation, characterization, and mapping of Escherichia coli mutants blocked in the synthesis of ornithine decarboxylase. J. Bacteriol. 124, 791–799.
Di Martino, M. L., Campilongo, R., Casalino, M., Micheli, G., Colonna, B., and Prosseda, G. (2013). Polyamines: emerging players in bacteria-host interactions. Int. J. Med. Microbiol. 303, 484–491. doi: 10.1016/j.ijmm.2013.06.008
Dougan, G., John, V., Palmer, S., and Mastroeni, P. (2011). Immunity to salmonellosis. Immunol. Rev. 240, 196–210. doi: 10.1111/j.1600-065X.2010.00999.x
Eriksson, S., Lucchini, S., Thompson, A., Rhen, M., and Hinton, J. C. (2003). Unravelling the biology of macrophage infection by gene expression profiling of intracellular Salmonella enterica. Mol. Microbiol. 47, 103–118. doi: 10.1046/j.1365-2958.2003.03313.x
Espinel, I. C., Guerra, P. R., and Jelsbak, L. (2016). Multiple roles of putrescine and spermidine in stress resistance and virulence of Salmonella enterica serovar Typhimurium. Microb. Pathog. 95, 117–123. doi: 10.1016/j.micpath.2016.03.008
Espinoza, R. A., Silva-Valenzuela, C. A., Amaya, F. A., Urrutia, I. M., Contreras, I., and Santiviago, C. A. (2017). Differential roles for pathogenicity islands SPI-13 and SPI-8 in the interaction of Salmonella Enteritidis and Salmonella Typhi with murine and human macrophages. Biol. Res. 50:5. doi: 10.1186/s40659-017-0109-8
Fang, S. B. (2011). Early Interactions of Non-Tyhpoidal Salmonella with Human Epithelium. Ph.D. Thesis, University College London.
Figueira, R., and Holden, D. W. (2012). Functions of the Salmonella pathogenicity island 2 (SPI-2) type III secretion system effectors. Microbiology 158, 1147–1161. doi: 10.1099/mic.0.058115-0
Figueira, R., Watson, K. G., Holden, D. W., and Helaine, S. (2013). Identification of Salmonella pathogenicity island-2 type III secretion system effectors involved in intramacrophage replication of S. enterica serovar Typhimurium: implications for rational vaccine design. MBio 4:e00065. doi: 10.1128/mBio.00065-13
Filippou, P. S., Lioliou, E. E., Panagiotidis, C. A., Athanassopoulos, C. M., Garnelis, T., Papaioannou, D., et al. (2007). Effect of polyamines and synthetic polyamine-analogues on the expression of antizyme (AtoC) and its regulatory genes. BMC Biochem. 8:1. doi: 10.1186/1471-2091-8-1
Fukuchi, J., Kashiwagi, K., Takio, K., and Igarashi, K. (1994). Properties and structure of spermidine acetyltransferase in Escherichia coli. J. Biol. Chem. 269, 22581–22585.
Fukuchi, J., Kashiwagi, K., Yamagishi, M., Ishihama, A., and Igarashi, K. (1995). Decrease in cell viability due to the accumulation of spermidine in spermidine acetyltransferase-deficient mutant of Escherichia coli. J. Biol. Chem. 270, 18831–18835. doi: 10.1074/jbc.270.32.18831
Garcia-Gutierrez, E., Chidlaw, A. C., Le Gall, G., Bowden, S. D., Tedin, K., Kelly, D. J., et al. (2016). A comparison of the ATP generating pathways used by S. Typhimurium to fuel replication within human and murine macrophage and epithelial cell lines. PLoS ONE 11:e0150687. doi: 10.1371/journal.pone.0150687
Gust, B., Challis, G. L., Fowler, K., Kieser, T., and Chater, K. F. (2003). PCR-targeted Streptomyces gene replacement identifies a protein domain needed for biosynthesis of the sesquiterpene soil odor geosmin. Proc. Natl. Acad. Sci. U.S.A. 100, 1541–1546. doi: 10.1073/pnas.0337542100
Hautefort, I., Thompson, A., Eriksson-Ygberg, S., Parker, M. L., Lucchini, S., Danino, V., et al. (2008). During infection of epithelial cells Salmonella enterica serovar Typhimurium undergoes a time-dependent transcriptional adaptation that results in simultaneous expression of three type 3 secretion systems. Cell. Microbiol. 10, 958–984. doi: 10.1111/j.1462-5822.2007.01099.x
Helaine, S., Thompson, J. A., Watson, K. G., Liu, M., Boyle, C., and Holden, D. W. (2010). Dynamics of intracellular bacterial replication at the single cell level. Proc. Natl. Acad. Sci. U.S.A. 107, 3746–3751. doi: 10.1073/pnas.1000041107
Henry, T., Garcia-Del Portillo, F., and Gorvel, J. P. (2005). Identification of Salmonella functions critical for bacterial cell division within eukaryotic cells. Mol. Microbiol. 56, 252–267.
Higashi, K., Ishigure, H., Demizu, R., Uemura, T., Nishino, K., Yamaguchi, A., et al. (2008). Identification of a spermidine excretion protein complex (MdtJI) in Escherichia coli. J. Bacteriol. 190, 872–878. doi: 10.1128/JB.01505-07
Hohmann, E. L. (2001). Nontyphoidal salmonellosis. Clin. Infect. Dis. 32, 263–269. doi: 10.1086/318457
Holzer, S. U., and Hensel, M. (2012). Divergent roles of Salmonella pathogenicity island 2 and metabolic traits during interaction of S. enterica serovar Typhimurium with host cells. PLoS ONE 7:e33220. doi: 10.1371/journal.pone.0033220
Igarashi, K., Ito, K., and Kashiwagi, K. (2001). Polyamine uptake systems in Escherichia coli. Res. Microbiol. 152, 271–278. doi: 10.1016/S0923-2508(01)01198-6
Igarashi, K., and Kashiwagi, K. (2010). Modulation of cellular function by polyamines. Int. J. Biochem. Cell Biol. 42, 39–51. doi: 10.1016/j.biocel.2009.07.009
Jelsbak, L., Thomsen, L. E., Wallrodt, I., Jensen, P. R., and Olsen, J. E. (2012). Polyamines are required for virulence in Salmonella enterica serovar Typhimurium. PLoS ONE 7:e36149. doi: 10.1371/journal.pone.0036149
Kashiwagi, K., Miyamoto, S., Nukui, E., Kobayashi, H., and Igarashi, K. (1993). Functions of potA and potD proteins in spermidine-preferential uptake system in Escherichia coli. J. Biol. Chem. 268, 19358–19363.
Kashiwagi, K., Shibuya, S., Tomitori, H., Kuraishi, A., and Igarashi, K. (1997). Excretion and uptake of putrescine by the PotE protein in Escherichia coli. J. Biol. Chem. 272, 6318–6323. doi: 10.1074/jbc.272.10.6318
Kohler, H., Rodrigues, S. P., Maurelli, A. T., and McCormick, B. A. (2002). Inhibition of Salmonella typhimurium enteropathogenicity by piperidine, a metabolite of the polyamine cadaverine. J. Infect. Dis. 186, 1122–1130. doi: 10.1086/344236
Koski, P., and Vaara, M. (1991). Polyamines as constituents of the outer membranes of Escherichia coli and Salmonella typhimurium. J. Bacteriol. 173, 3695–3699. doi: 10.1128/jb.173.12.3695-3699.1991
Kurihara, S., Tsuboi, Y., Oda, S., Kim, H. G., Kumagai, H., and Suzuki, H. (2009). The putrescine importer PuuP of Escherichia coli K-12. J. Bacteriol. 191, 2776–2782. doi: 10.1128/JB.01314-08
Kwon, D. H., and Lu, C. D. (2007). Polyamine effects on antibiotic susceptibility in bacteria. Antimicrob. Agents Chemother. 51, 2070–2077. doi: 10.1128/AAC.01472-06
Lamichhane-Khadka, R., Frye, J. G., Porwollik, S., McClelland, M., and Maier, R. J. (2011). Hydrogen-stimulated carbon acquisition and conservation in Salmonella enterica serovar Typhimurium. J. Bacteriol. 193, 5824–5832. doi: 10.1128/JB.05456-11
Lee, J., Sperandio, V., Frantz, D. E., Longgood, J., Camilli, A., Phillips, M. A., et al. (2009). An alternative polyamine biosynthetic pathway is widespread in bacteria and essential for biofilm formation in Vibrio cholerae. J. Biol. Chem. 284, 9899–9907. doi: 10.1074/jbc.M900110200
Lenis, Y. Y., Elmetwally, M. A., Maldonado-Estrada, J. G., and Bazer, F. W. (2017). Physiological importance of polyamines. Zygote 25, 244–255. doi: 10.1017/S0967199417000120
Leung, K. Y., and Finlay, B. B. (1991). Intracellular replication is essential for the virulence of Salmonella typhimurium. Proc. Natl. Acad. Sci. U.S.A. 88, 11470–11474. doi: 10.1073/pnas.88.24.11470
Limsuwun, K., and Jones, P. G. (2000). Spermidine acetyltransferase is required to prevent spermidine toxicity at low temperatures in Escherichia coli. J. Bacteriol. 182, 5373–5380. doi: 10.1128/JB.182.19.5373-5380.2000
Liu, Y., Yu, K., Zhou, F., Ding, T., Yang, Y., Hu, M., et al. (2017). Quantitative proteomics charts the landscape of salmonella carbon metabolism within host epithelial cells. J. Proteome Res. 16, 788–797. doi: 10.1021/acs.jproteome.6b00793
Lopez, C. A., Rivera-Chavez, F., Byndloss, M. X., and Baumler, A. J. (2015). The periplasmic nitrate reductase NapABC supports luminal growth of Salmonella enterica Serovar Typhimurium during colitis. Infect. Immun. 83, 3470–3478. doi: 10.1128/IAI.00351-15
Martinez-Moya, M., De Pedro, M. A., Schwarz, H., and Garcia-Del, P. F. (1998). Inhibition of Salmonella intracellular proliferation by non-phagocytic eucaryotic cells. Res. Microbiol. 149, 309–318. doi: 10.1016/S0923-2508(98)80436-1
McClelland, M., Sanderson, K. E., Spieth, J., Clifton, S. W., Latreille, P., Courtney, L., et al. (2001). Complete genome sequence of Salmonella enterica serovar Typhimurium LT2. Nature 413, 852–856. doi: 10.1038/35101614
Mercado-Lubo, R., Gauger, E. J., Leatham, M. P., Conway, T., and Cohen, P. S. (2008). A Salmonella enterica serovar typhimurium succinate dehydrogenase/fumarate reductase double mutant is avirulent and immunogenic in BALB/c mice. Infect. Immun. 76, 1128–1134. doi: 10.1128/IAI.01226-07
Mercado-Lubo, R., Leatham, M. P., Conway, T., and Cohen, P. S. (2009). Salmonella enterica serovar Typhimurium mutants unable to convert malate to pyruvate and oxaloacetate are avirulent and immunogenic in BALB/c mice. Infect. Immun. 77, 1397–1405. doi: 10.1128/IAI.01335-08
Miller-Fleming, L., Olin-Sandoval, V., Campbell, K., and Ralser, M. (2015). Remaining mysteries of molecular biology: the role of polyamines in the cell. J. Mol. Biol. 427, 3389–3406. doi: 10.1016/j.jmb.2015.06.020
Mo, E., Peters, S. E., Willers, C., Maskell, D. J., and Charles, I. G. (2006). Single, double and triple mutants of Salmonella enterica serovar Typhimurium degP (htrA), degQ (hhoA) and degS (hhoB) have diverse phenotypes on exposure to elevated temperature and their growth in vivo is attenuated to different extents. Microb. Pathog. 41, 174–182. doi: 10.1016/j.micpath.2006.07.004
Nunez-Hernandez, C., Tierrez, A., Ortega, A. D., Pucciarelli, M. G., Godoy, M., Eisman, B., et al. (2013). Genome expression analysis of nonproliferating intracellular Salmonella enterica serovar Typhimurium unravels an acid pH-dependent PhoP-PhoQ response essential for dormancy. Infect. Immun. 81, 154–165. doi: 10.1128/IAI.01080-12
Oyedotun, K. S., and Lemire, B. D. (2004). The quaternary structure of the Saccharomyces cerevisiae succinate dehydrogenase. Homology modeling, cofactor docking, and molecular dynamics simulation studies. J. Biol. Chem. 279, 9424–9431. doi: 10.1074/jbc.M311876200
Pace, J., Hayman, M. J., and Galan, J. E. (1993). Signal transduction and invasion of epithelial cells by S. typhimurium. Cell 72, 505–514. doi: 10.1016/0092-8674(93)90070-7
Paiva, J. B., Penha Filho, R. A., Pereira, E. A., Lemos, M. V., Barrow, P. A., Lovell, M. A., et al. (2009). The contribution of genes required for anaerobic respiration to the virulence of Salmonella enterica serovar Gallinarum for chickens. Braz. J. Microbiol. 40, 994–1001. doi: 10.1590/S1517-83822009000400035
Poncet, S., Milohanic, E., Maze, A., Nait Abdallah, J., Ake, F., Larribe, M., et al. (2009). Correlations between carbon metabolism and virulence in bacteria. Contrib. Microbiol. 16, 88–102. doi: 10.1159/000219374
Radtke, A. L., Wilson, J. W., Sarker, S., and Nickerson, C. A. (2010). Analysis of interactions of Salmonella type three secretion mutants with 3-D intestinal epithelial cells. PLoS ONE 5:e15750. doi: 10.1371/journal.pone.0015750
Rowley, G., Hensen, D., Felgate, H., Arkenberg, A., Appia-Ayme, C., Prior, K., et al. (2012). Resolving the contributions of the membrane-bound and periplasmic nitrate reductase systems to nitric oxide and nitrous oxide production in Salmonella enterica serovar Typhimurium. Biochem. J. 441, 755–762. doi: 10.1042/BJ20110971
Sabbagh, S. C., Forest, C. G., Lepage, C., Leclerc, J. M., and Daigle, F. (2010). So similar, yet so different: uncovering distinctive features in the genomes of Salmonella enterica serovars Typhimurium and Typhi. FEMS Microbiol. Lett. 305, 1–13. doi: 10.1111/j.1574-6968.2010.01904.x
Shah, P., and Swiatlo, E. (2008). A multifaceted role for polyamines in bacterial pathogens. Mol. Microbiol. 68, 4–16. doi: 10.1111/j.1365-2958.2008.06126.x
Slocum, R. D., Flores, H. E., Galston, A. W., and Weinstein, L. H. (1989). Improved method for HPLC analysis of polyamines, agmatine and aromatic monoamines in plant tissue. Plant Physiol. 89, 512–517. doi: 10.1104/pp.89.2.512
Small, P. L., Isberg, R. R., and Falkow, S. (1987). Comparison of the ability of enteroinvasive Escherichia coli, Salmonella typhimurium, Yersinia pseudotuberculosis, and Yersinia enterocolitica to enter and replicate within HEp-2 cells. Infect. Immun. 55, 1674–1679.
Soksawatmaekhin, W., Kuraishi, A., Sakata, K., Kashiwagi, K., and Igarashi, K. (2004). Excretion and uptake of cadaverine by CadB and its physiological functions in Escherichia coli. Mol. Microbiol. 51, 1401–1412. doi: 10.1046/j.1365-2958.2003.03913.x
Steele-Mortimer, O., Brumell, J. H., Knodler, L. A., Meresse, S., Lopez, A., and Finlay, B. B. (2002). The invasion-associated type III secretion system of Salmonella enterica serovar Typhimurium is necessary for intracellular proliferation and vacuole biogenesis in epithelial cells. Cell. Microbiol. 4, 43–54. doi: 10.1046/j.1462-5822.2002.00170.x
Stein, M. A., Leung, K. Y., Zwick, M., Garcia-Del Portillo, F., and Finlay, B. B. (1996). Identification of a Salmonella virulence gene required for formation of filamentous structures containing lysosomal membrane glycoproteins within epithelial cells. Mol. Microbiol. 20, 151–164. doi: 10.1111/j.1365-2958.1996.tb02497.x
Sugiyama, Y., Nakamura, A., Matsumoto, M., Kanbe, A., Sakanaka, M., Higashi, K., et al. (2016). A novel putrescine exporter SapBCDF of Escherichia coli. J. Biol. Chem. 291, 26343–26351. doi: 10.1074/jbc.M116.762450
Tchawa Yimga, M., Leatham, M. P., Allen, J. H., Laux, D. C., Conway, T., and Cohen, P. S. (2006). Role of gluconeogenesis and the tricarboxylic acid cycle in the virulence of Salmonella enterica serovar Typhimurium in BALB/c mice. Infect. Immun. 74, 1130–1140. doi: 10.1128/IAI.74.2.1130-1140.2006
Wang, K. C., Huang, C. H., Ding, S. M., Chen, C. K., Fang, H. W., Huang, M. T., et al. (2016a). Role of yqiC in the pathogenicity of Salmonella and innate immune responses of human intestinal epithelium. Front. Microbiol. 7:1614. doi: 10.3389/fmicb.2016.01614
Wang, K. C., Huang, C. H., Huang, C. J., and Fang, S. B. (2016b). Impacts of Salmonella enterica Serovar Typhimurium and its speG gene on the transcriptomes of in vitro M cells and Caco-2 cells. PLoS ONE 11:e0153444. doi: 10.1371/journal.pone.0153444
Wong, H. S., Maker, G. L., Trengove, R. D., and O'handley, R. M. (2015). Gas chromatography-mass spectrometry-based metabolite profiling of Salmonella enterica serovar Typhimurium differentiates between biofilm and planktonic phenotypes. Appl. Environ. Microbiol. 81, 2660–2666. doi: 10.1128/AEM.03658-14
Keywords: speG, polyamine, transcriptome, Salmonella Typhimurium, RNA microarray, flagella, motility, intracellular replication
Citation: Fang S-B, Huang C-J, Huang C-H, Wang K-C, Chang N-W, Pan H-Y, Fang H-W, Huang M-T and Chen C-K (2017) speG Is Required for Intracellular Replication of Salmonella in Various Human Cells and Affects Its Polyamine Metabolism and Global Transcriptomes. Front. Microbiol. 8:2245. doi: 10.3389/fmicb.2017.02245
Received: 11 August 2017; Accepted: 31 October 2017;
Published: 15 November 2017.
Edited by:
Lorenza Putignani, Bambino Gesù Ospedale Pediatrico (IRCCS), ItalyReviewed by:
Lydia Bogomolnaya, Texas A&M University Health Science Center, United StatesBryan Troxell, Alcami Corporation, United States
Copyright © 2017 Fang, Huang, Huang, Wang, Chang, Pan, Fang, Huang and Chen. This is an open-access article distributed under the terms of the Creative Commons Attribution License (CC BY). The use, distribution or reproduction in other forums is permitted, provided the original author(s) or licensor are credited and that the original publication in this journal is cited, in accordance with accepted academic practice. No use, distribution or reproduction is permitted which does not comply with these terms.
*Correspondence: Shiuh-Bin Fang, c2JmYW5nQHRtdS5lZHUudHc=