- 1Environmental Futures Research Institute, School of Environment, Griffith University, Gold Coast, QLD, Australia
- 2Departamento de Ecología Evolutiva, Instituto de Ecología, Universidad Nacional Autónoma de México, Mexico City, Mexico
- 3Department of Biology, James Madison University, Harrisonburg, VA, United States
- 4Amphibian Survival Alliance, London, United Kingdom
- 5Department of Biology, San Francisco State University, San Francisco, CA, United States
- 6School of Science and Engineering, University of the Sunshine Coast, Maroochydore, QLD, Australia
In animals and plants, symbiotic bacteria can play an important role in disease resistance of host and are the focus of much current research. Globally, amphibian population declines and extinctions have occurred due to chytridiomycosis, a skin disease caused by the pathogen Batrachochytrium dendrobatidis (Bd). Currently amphibian skin bacteria are increasingly recognized as important symbiont communities with a relevant role in the defense against pathogens, as some bacteria can inhibit the growth of B. dendrobatidis. This study aims to document the B. dendrobatidis infection status of wild populations of a terrestrial cryptic frog (Philoria loveridgei), and to determine whether infection status is correlated with changes in the skin microbial communities. Skin samples of P. loveridgei were collected along an altitudinal range within the species distribution in subtropical rainforests in southeast Australia. Sampling was conducted in two years during two breeding seasons with the first classified as a “La Niña” year. We used Taqman real-time PCR to determine B. dendrobatidis infection status and 16S amplicon sequencing techniques to describe the skin community structure. We found B. dendrobatidis-positive frogs only in the second sampling year with low infection intensities, and no correlation between B. dendrobatidis infection status and altitude, frog sex or size. Skin bacterial diversity was significantly higher in P. loveridgei frogs sampled in the 1st year than in the 2nd year. In addition, 7.4% of the total OTUs were significantly more abundant in the 1st year compared to the 2nd year. We identified 67 bacterial OTUs with a significant positive correlation between infection intensity and an OTU’s relative abundance. Forty-five percent of these OTUs belonged to the family Enterobacteriaceae. Overall, temporal variation was strongly associated with changes in B. dendrobatidis infection status and bacterial community structure of wild populations of P. loveridgei.
Introduction
Symbiotic relationships between bacteria and macro-organisms are ubiquitous in nature. The study of symbiotic interactions is a well-established field, yet recently has received increased attention due to the development of next generation sequencing technologies. Specifically, the role of beneficial bacterial communities has been the focus of research for wildlife and human hosts, as they can play important roles providing protection against pathogens (Harris et al., 2009; Jiménez and Sommer, 2016; Walke and Belden, 2016). In amphibians, some bacterial species on their skin inhibit the growth of pathogenic fungi (Harris et al., 2006; Becker et al., 2015; Woodhams et al., 2015) and have shown to play a protective role against chytridiomycosis, a deadly skin fungal disease (Harris et al., 2009; Becker and Harris, 2010; Holden et al., 2015).
Worldwide, many amphibian species are facing significant declines and extinctions attributable to chytridiomycosis (Collins and Storfer, 2003; Stuart et al., 2004; Wake and Vredenburg, 2008; Wake, 2012), the infectious diseases caused by Batrachochytrium dendrobatidis (Bd) and B. salamandrivorans (Bsal) (Berger et al., 1998; Longcore et al., 1999; Skerratt et al., 2007; Wake and Vredenburg, 2008; Martel et al., 2013). Interestingly, amphibian species exhibit differential susceptibility to Bd infections, which is reflected in the worldwide variation in mortality due to Bd and in Bd intensity and prevalence among species from different habitats, life histories and taxonomic affinities (reviewed in Skerratt et al., 2007; Kilpatrick et al., 2010). These variations are not completely understood, with research suggesting species-specific physiological and ecological traits, such as presence of symbiotic bacterial communities, differential immune response, production of antimicrobial peptides, behavioral patterns and environmental conditions (Kriger and Hero, 2008; Jiménez and Sommer, 2016; Rebollar et al., 2016a).
Given the beneficial role in disease resistance of symbiotic bacterial communities, recent attention has been given to the interaction occurring between amphibian skin microbiota and Bd. Recent evidence indicates that diversity and structure of skin bacterial communities is influenced by several factors such as species-specific traits (McKenzie et al., 2012; Rebollar et al., 2016b; Assis et al., 2017), developmental changes (Kueneman et al., 2014, 2016; Longo et al., 2015; Longo and Zamudio, 2017b), geographic location (Belden et al., 2015; Longo and Zamudio, 2017b; Muletz Wolz et al., 2017). Moreover, skin bacterial diversity is influenced by the microbial communities present in the environment (Fitzpatrick and Allison, 2014; Loudon et al., 2014, 2016; Walke et al., 2014; Rebollar et al., 2016b) as well as by pathogen infection (Jani and Briggs, 2014; Walke et al., 2015; Longo and Zamudio, 2017a).
It remains unclear how climatic conditions influence the composition of microbial communities on the amphibian skin (Kueneman et al., 2014; Jiménez and Sommer, 2016; Longo and Zamudio, 2017a; Medina et al., 2017; Muletz Wolz et al., 2017; Sabino-Pinto et al., 2017). However, in some amphibian species correlation between the variation in amphibian microbiota and abiotic factors (air temperature, water temperature, pH, conductivity, seasonality) has been reported (Kueneman et al., 2014; Krynak et al., 2015, 2016; Longo et al., 2015; Longo and Zamudio, 2017a; Muletz Wolz et al., 2017). For example, in Lithobates yavapaiensis, an increase in alpha diversity (Shannon index) of skin bacteria from summer to winter was found, as was a difference in community composition between seasons (Bray–Curtis dissimilarities) (Longo et al., 2015). Similarly, the bacterial composition of Eleutherodactylus coqui changed between seasons, potentially allowing frogs to limit Bd infection in the warm wet season (Longo and Zamudio, 2017a).
In addition to the potential role that climatic conditions and abiotic factors have on skin microbiota, Bd prevalence and infection has also been found to be influenced by these factors. Several environmental factors such as altitude, temperature and moisture have been associated with chytridiomycosis outbreaks (Berger et al., 2004; Drew et al., 2006; Lips et al., 2006; Kriger and Hero, 2007, 2008; Kriger et al., 2007; Longo et al., 2010). For example, several studies have shown a decrease in severity of infection at warmer temperatures and or dryer seasons (Retallick et al., 2004; Woodhams and Alford, 2005; Kriger and Hero, 2007; Kriger et al., 2007; Rowley and Alford, 2013). Numerous studies suggest that chytridiomycosis presence, prevalence or intensity can be higher under cooler conditions (Berger et al., 2004; McDonald et al., 2005; Ouellet et al., 2005; Drew et al., 2006; Kriger and Hero, 2007; Kriger et al., 2007; Longo et al., 2010) and/or high elevation (Bosch et al., 2001; Burrowes et al., 2004; McDonald et al., 2005; Woodhams and Alford, 2005; Lips et al., 2006; Frías-Alvarez et al., 2008; Walker et al., 2010). Collectively, these studies support the hypothesis that amphibian populations declines associated with chytridiomycosis occur predominantly at high elevations and during cooler conditions. This pattern, along with the preference of Bd for relatively cool and humid conditions for growth in culture of approximately 23°C, suggests that environmental temperature and humidity are likely to be an important factor in disease dynamics (Muths et al., 2008).
Moreover, studies on Australian amphibian species have revealed that Bd infection is strongly associated with aquatic habitats (Kriger and Hero, 2007) and in frog species that hibernate in aquatic microhabitats rather than terrestrial (Skerratt et al., 2010). This pattern of aquatic amphibian species presenting higher infection and prevalence rates has also been reported among other amphibian species worldwide and has led researchers to hypothesize that terrestrial species may avoid Bd infection through microhabitat protection (Berger et al., 1998; Lips et al., 2006; Kriger and Hero, 2007; Brem and Lips, 2008; Catenazzi et al., 2011). Whilst Bd is more associated with aquatic habitats, terrestrial amphibians can also be infected with Bd, with some species experiencing population declines (Berger et al., 1998; Bell et al., 2004; Burrowes et al., 2004; Lips et al., 2006; Kriger and Hero, 2007; Brem and Lips, 2008; Longo and Burrowes, 2010; Catenazzi et al., 2011). To the best of our knowledge, only a few Australian terrestrial species have been reported with Bd infections. Negative Bd infection was reported on terrestrial Australian microhylids of the Wet Tropics (Hauselberger and Alford, 2012). However, Bd infection was reported for Cophixalus ornatus and Assa darlingtoni (Kriger and Hero, 2006, 2007). These limited and inconclusive results warrant further investigation of Bd suceptibility in Australian terrestrial amphibians, and the role of skin microbiota on host-pathogen interactions.
Philoria loveridgei is a microendemic, diurnal terrestrial frog with terrestrial and nidicolous development to metamorphosis (embryos develop in the broken-down jelly capsules in a nest basin in mud, but they do not go to water) (Knowles et al., 2004; Anstis, 2013). This species is among the rarest vertebrates in eastern Australia, listed as vulnerable due to their habitat specialization and restricted mountaintop distribution (Hines et al., 1999; Knowles et al., 2004). Frogs from this fossorial species are small and highly cryptic making them difficult to find. Males construct shallow burrows under moist forest leaf litter or rocks, where they emit a soft mating call during breeding season to attract females. Inside this nest inguinal amplexus, egg laying and larval metamorphosis occur (Hines et al., 1999; Knowles et al., 2004; Anstis, 2013).
A few studies have addressed the role of climatic changes and temporal variation on host–pathogen interactions of amphibians including the role of skin microbiota and Bd (Jani and Briggs, 2014; Longo et al., 2015; Longo and Zamudio, 2017a; Medina et al., 2017; Muletz Wolz et al., 2017; Sabino-Pinto et al., 2017). However, temporal variation in these studies has been related to changes in seasons within the same year (Longo et al., 2015; Longo and Zamudio, 2017a; Muletz Wolz et al., 2017; Sabino-Pinto et al., 2017). Therefore, to better understand the influence skin microbiota has on host–pathogen interactions, we describe the relationship of the amphibian skin microbiota of wild populations and the climatic factors across two years. In this study, we hypothesized that climatic conditions would influence the extent of Bd infection as well as the structure of skin bacterial communities. To examine this hypothesis we documented Bd infection status and described skin microbial structure of wild populations of a terrestrial cryptic frog (P. loveridgei) during two years across their breeding season that showed clear differences in precipitation levels. We also determined whether infection status correlated with changes in the diversity and structure of skin microbial communities.
Materials and Methods
Ethics Statement
This study was carried out in accordance with the recommendations of a Scientific Purpose permit issued by the Department of Environment and Resource Management (WITK10308811) Queensland, Australia. Griffith University Animal Ethics Committee (ENV/21/12AEC) approved the protocol.
Field Sites
Field surveys were done in four national parks that make up part of the Tweed Caldera Rim within Gondwana Rainforests of Australia World Heritage Area, in mid-eastern Australia (Figure 1). This region is characterized by subtropical rainforest vegetation and is dominated by a moist subtropical climate. At altitudes above 800 m above sea level (asl) a cool high-altitude subtropical rainforest occurs as rainfall is significantly augmented by fog-drip. In these mountain ranges the monthly summer average temperatures range between a maximum of 24.1°C and a minimum of 19.7°C, while winter temperatures range from 17.8 to 12.3°C, correspondingly. Rainfall in this area is seasonal with 65–70% of the annual total (average annual rainfall >3000 mm) occurring in the summer months (McDonald, 2010).
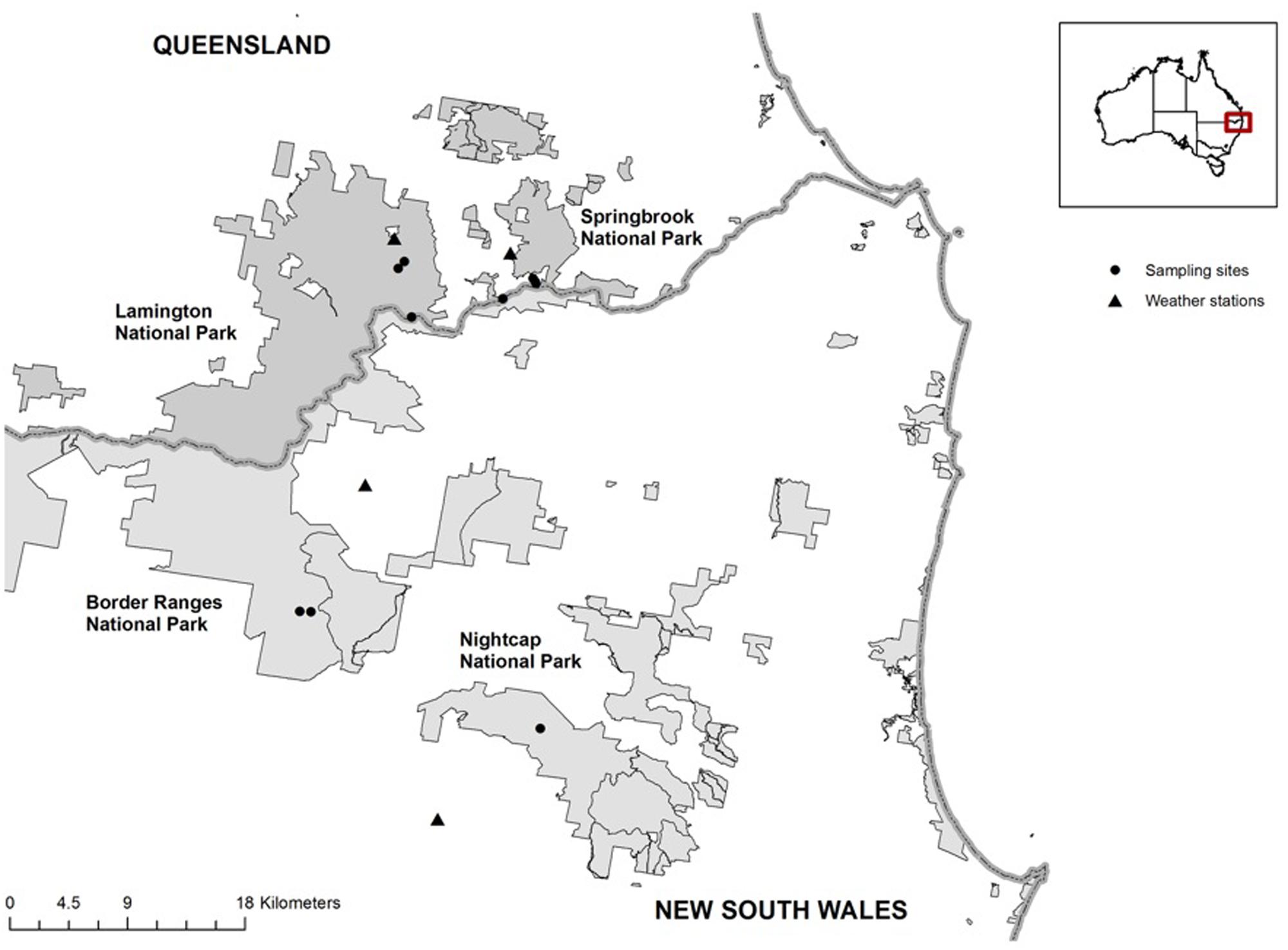
FIGURE 1. Study area in south–east Queensland and north–east New South Wales National Parks, indicating P. loveridgei survey sites (dots) throughout the range of the species and the nearest Australian Bureau of Meteorology stations (triangles).
Sample Collection
Surveys were conducted in two separate years during the frog species breeding season of each year (Australian spring and summer months) from November 2011 to January 2012 (here after year 1), and from November 2013 to January 2014 (here after year 2). Sample collection took place at nine sites covering the altitudinal and distribution ranges of P. loveridgei, including six sites in southeast Queensland (Springbrook and Lamington National Parks), and three sites in northeast New South Wales (Nightcap and Border Ranges National Parks). These sites were selected as they are highly occupied by the target species (MFL personal observation). The altitudes of the sites where frogs were encountered ranged from 680 to 1080 m asl (Table 1).
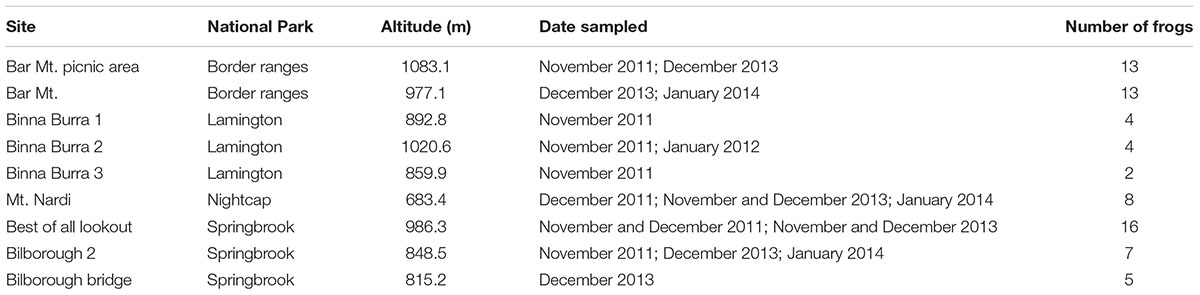
TABLE 1. Field surveys sites and dates of P. loveridgei across the species distribution range, across two years during the breeding seasons.
Sample collection consisted of microhabitat surveys and intensive burrow searches performed by carefully excavating under leaf litter or rocks at sites where calling males were heard, with a minimum target of 10 frogs per site. P. loveridgei frogs, particularly males, were found sitting inside burrows. Call mimicry was sometimes used to incite males to call, thereby aiding the discovery of their location. Frogs were captured with freezer plastic bags and kept individually, and two skin swabs samples were collected following standard published protocols for Bd first and skin bacteria second (Brem et al., 2007; Hyatt et al., 2007; Kriger and Hero, 2007; Vredenburg et al., 2010). Before swabbing, frogs were rinsed with sterile distill water to eliminate transient bacteria (Rebollar et al., 2014). Swabbing consisted of moving a swab across the frog’s skin in a standardized way, using sterile rayon-tipped swabs (MW113; Lakewood Biochemical CO., United States), five strokes on each side of the abdominal midline, five strokes on the inner thighs of each hind leg, and five strokes on the foot webbing of each hind leg for a total of 30 strokes per frog (Kriger et al., 2006b; Vredenburg et al., 2010; Rebollar et al., 2014). Swabs were immediately placed in individually labeled microtubes and stored in a dry, cool place until transported back to the laboratory and stored at 4°C. To ensure consistency in swabbing technique all frogs were swabbed by MFL (Kriger et al., 2006b; Simpkins et al., 2014).
The morphological characteristics of all individuals, including body mass and size (snout-vent length, SVL), were recorded after skin samples were taken. All frogs were captured and handled at all times using individual, clean, unused freezer plastic bags. Additionally, all equipment used was washed with a solution of commercial bleach (1:9, bleach: water) and thoroughly cleaned between collection sites to prevent potential spread of the fungus pathogen (Phillott et al., 2010). Frog sampling was biased toward males as there sedentary calling behavior made them relatively more conspicuous than females. All burrows were individually marked to prevent resampling the same individual and to allow the release of individual frogs to their exact site of capture.
Molecular Methods and Data Analyses for Bd Analyses
Real-time quantitative PCR (qPCR) analyses of the frog swabs were used to test for Bd infection, prevalence and intensity. Swabs were analyzed at San Francisco State University (San Francisco, California, United States), using the protocol described by Boyle et al. (2004) with the following changes: swab extracts were analyzed singly instead of in triplicate since use of singletons and triplicates has been found to give similar results in previous studies (Kriger et al., 2006a; Vredenburg et al., 2010). In addition, BSA was added to the qPCR master mix (1 μL BSA per reaction) (Garland et al., 2010).
Prevalence of Bd was calculated as the number of frogs that were Bd positive (when zoospore equivalents were ≥0.1) relative to the total number of frogs sampled. Infection intensity was defined as the number of zoospore equivalents per swab. Zoospore equivalents were estimated by multiplying the qPCR genomic value by 80, as DNA extracts from swabs were diluted 80-fold during extraction and qPCR (Briggs et al., 2010; Vredenburg et al., 2010).
Spearman correlations were used to assess the relationship between altitude, body size, weight, and the intensity of Bd infection. Mann–Whitney U test was used to assess the relationship between sex of the frog and the intensity of Bd infection. Analyses were conducted using R statistical software (R Core Team, 2015) specifically using the function cor.test (“spearman”) and wilcox.test().
Molecular Methods and Sequencing for Bacterial Analyses
Whole genomic DNA was extracted from 72 swab frog samples using the DNeasy Blood and Tissue kit (Qiagen, Valencia, CA, United States) following the manufacturer’s instructions including a pretreatment with lysozyme included in the protocol titled: Pretreatment for Gram-Positive Bacteria (page 45). This pretreatment is recommended when trying to extract DNA from Gram-positive bacteria in addition to Gram-negative bacteria. DNA extracted from swabs was used to amplify the V4 region of the 16S rRNA gene using barcoded primers (F515/R806) and PCR conditions adapted from Caporaso et al. (2011). Amplicons were quantified using QuantifluorTM (Promega, Madison, WI, United States). Composite samples for sequencing were created by combining equimolar ratios of amplicons from the individual samples, followed by cleaning with the QIAquick PCR clean up kit (Qiagen, Valencia, CA, United States). Barcoded composite PCR products were sent to the Dana Farber Cancer Institute’s Molecular Biology Core Facilities (Boston, MA, United States) for MiSeq Illumina sequencing using a 250 bp single read strategy.
16S Amplicon Data Processing
The 250 bp single reads were filtered and processed with the Quantitative Insights Into Microbial Ecology (QIIME) pipeline (Caporaso et al., 2010b). Sequences were de-multiplexed and filtered to retain high quality reads using the following filtering parameters: no N characters were allowed in retained sequences, no errors in barcode sequence were allowed, a minimum of five high quality consecutive base pairs were needed to include a read, and a maximum of five consecutive low quality base pairs were allowed before truncating a read. After filtering, 5,991,855 sequences were retained for the 72 samples.
The de-multiplexed data set was deposited in the NCBI sequence read archive (SRA) with the accession number SRR5957156 which is linked to biosample SAMN07501977 and bioproject PRJNA398139.
De-multiplexed and filtered sequences were clustered into operational taxonomic units (OTUs) at a sequence similarity threshold of 97% with the UCLUST method (Edgar, 2010). Sequences were matched against the Greengenes database released in May 2013 (McDonald et al., 2012), and those that did not match were clustered as de novo OTUs at 97% sequence similarity. Taxonomy was assigned using the RDP classifier (Wang et al., 2007) and the Greengenes database. Representative sequences were aligned to the Greengenes database with PyNAST (Caporaso et al., 2010a), and a ML phylogenetic tree was constructed with FastTree 2 (Price et al., 2010). The OTU table was filtered using a minimum cluster size of 0.001% of the total reads as suggested by Bokulich et al. (2013). Samples were rarefied according to the sample with lower number of reads (i.e., 25,605 reads). The final rarefied OTU table had 7,673 OTUs including a total of 1,843,560 reads.
Bacterial Skin Data Analysis and Statistics
To describe the diversity of host skin bacteria in P. loveridgei across two years in the breeding season Shannon diversity index was calculated for all 72 samples. ANOVA and post hoc Tukey’s tests were carried out to determine differences between samples taken every month during the two years inbreeding seasons. Stacked bar charts of bacterial taxa at the class level were obtained to show the mean relative abundance of the 10 most abundant taxa on each month during the two years in the breeding seasons.
Weighted Unifrac and distance matrices were used to calculate the beta diversity and were visualized with a principal coordinates analysis. Differences in beta diversity between the two years samples and between Bd positive and negative samples were tested with non-parametric analysis of variance based on 999 permutations (PERMANOVA) using the software PRIMER-E (Clarke and Gorley, 2006). Alpha and beta diversity metrics and relative abundance comparisons at the class level were obtained using QIIME (Caporaso et al., 2010b). Analysis of multivariate homogeneity of group dispersions was calculated to determine whether skin communities from different breeding seasons had different dispersion values using the function betadisper in vegan package in R (Oksanen et al., 2015).
To determine the bacterial taxa that most likely explain differences between the two years, we used the linear discriminant analysis (LDA) effect size (LEfSe) method (Segata et al., 2011). Classes were defined as years 1 and 2, and subclasses corresponded to the months per breeding season. OTUs with LDA scores >2.0 were considered informative based on previous studies (Segata et al., 2011; Clemente et al., 2015; Rebollar et al., 2016a). Lefse was calculated using Galaxy (Afgan et al., 2016) implemented at the Huttenhower Lab1.
To determine if Bd infection intensities were correlated with bacterial relative abundance across frog samples we calculated Spearman correlations with false discovery rate (Benjamini–Hochberg) to account for multiple comparisons using R (R Core Team, 2015) using the functions cor.test(“spearman”) and p.adjust(“BH”). To perform this analysis we only included the data from year 2 since on year 1 Bd was not detected and the bacterial community was different than on year 2. Correlation values with p < 0.05 were considered significant. Wilcoxon Rank Sum Test in R (R Core Team, 2015), using the function wilcox.test(), was calculated to compare the relative abundance of bacterial taxa that had significant Spearman correlations between Bd-positive and Bd-negative samples.
Results
Bd Infection Status of Philoria loveridgei
We detected Bd infection in seven individuals of 72 P. loveridgei tested (8 females and 64 males), with an overall prevalence of 9.7% (n = 7, 6 males and 1 female), across the two years during the breeding season (year 1: 2011/2012 and year 2: 2013/2014). The seven frogs infected were all sampled in year 2 (n = 55) while year 1 yielded none (n = 17). The pathogen was found only at two of the nine sites surveyed, both located in the southern limits of P. loveridgei’s distribution range in New South Wales (Figure 1). The majority of infected frogs (n = 6) were from one site located in Border Ranges National Park (Bar Mt area), with a site infection prevalence of 35.3%. The only other Bd infected frog was from Nightcap National Park, with a site prevalence of 12.5% (Table 2). These sites have a difference in altitude of 300 m asl; the Nightcap site was the lowest area surveyed in this study. Altitude, however, was not significantly correlated with Bd infection (ρ = -0.126, P = 0.290, n = 72).
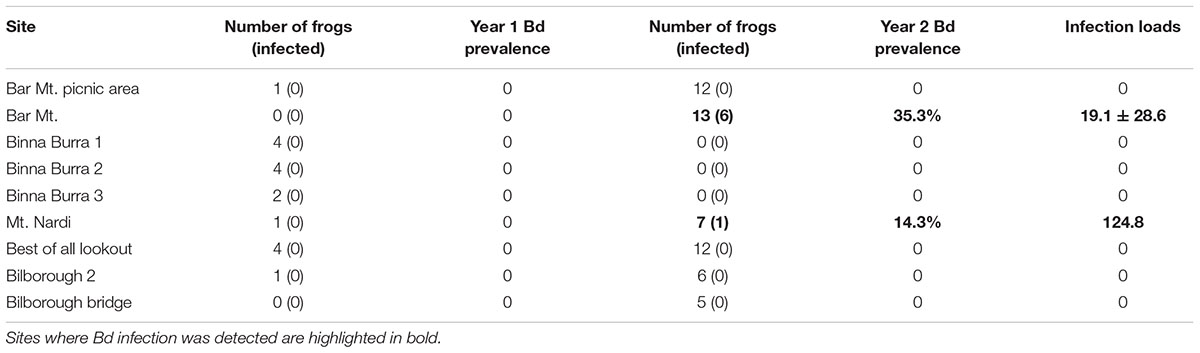
TABLE 2. Disease prevalence and infection loads (Bd zoospore equivalents) of individual P. loveridgei per site and per year sampled (year 1 = 2011/2012 and year 2 = 2013/2014).
We found that overall, infection intensity was relatively low with an average of 34.2 ± 47.7 zoospore equivalents (mean ± standard deviation), ranging from as low as 0.79 to 124.8 zoospore equivalents per individual. The frog presenting the highest infection intensity (124.8 zoospore equivalents) was from the Nightcap site (Table 2).
Male frogs weighed an average 2.2 ± 0.7 g and had a mean SVL of 25.6 ± 0.3 mm (mean ± standard deviation). As expected, female frogs were bigger than male frogs weighing an average 2.8 ± 0.6 g and had a mean SVL of 28.8 ± 0.2 mm. No association was found between sex of the frog and infection status (Mann–Whitney U = 250, P = 0.848, n = 72). Additionally, size and weight of frogs were not significantly correlated with Bd intensity (size; ρ = 0.130, P = 0.278, n = 72; weight; ρ = -0.082, P = 0.496, n = 72). No clinical signs of chytridiomycosis were observed in any of the frogs surveyed (Berger et al., 1998, 2005).
Temporal Variation of the Skin Bacterial Community Structure of ylum Proteobacteria was the most a
A total of 7,673 OTUs were identified from 72 frog samples collected during three months across two years in the breeding season. Overall, phylum Proteobacteria was the most abundant (49.9%) across all samples, followed by Actinobacteria (13.37%), Acidobacteria (7.71%), Verrucomicrobia (4.74%), and Planctomycetes (3.16%).
According to Shannon index estimates, skin bacterial alpha diversity was significantly higher in P. loveridgei skin samples from year 1 in comparison to year 2 [Figure 2A; ANOVA F(5,66) = 8.215, p = 4.46 × 10-6]. These diversity values indicate significant differences in bacterial community richness and relative abundance between months of different years but not between months within the same year.
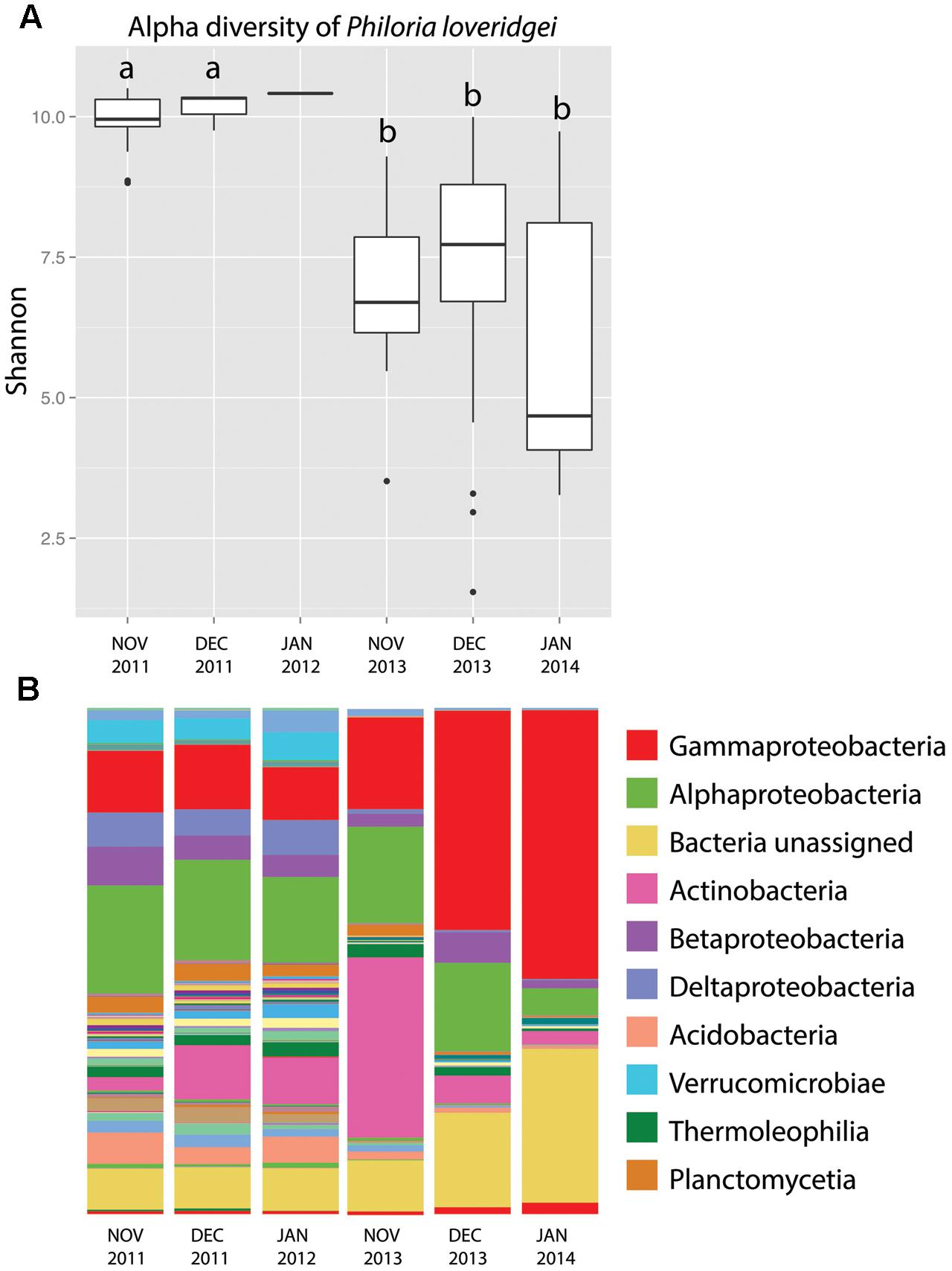
FIGURE 2. Skin bacterial community structure of P. loveridgei across time in two breeding seasons. (A) Alpha diversity (Shannon) of P. loveridgei [ANOVA F(5,66) = 8.215, P = 4.46 × 10–6]. Different letters (a and b) signify statistically significant differences across months, as indicated by a Tukey’s post hoc test. (B) Stacked bar chart of mean relative abundances of bacterial taxa (class level) of P. loveridgei. Colored bars (legend) indicate the 10 most abundant taxa.
Specifically, at the class level, Alphaproteobacteria were more abundant in year 1 while Gammaproteobacteria were more abundant in year 2 (Figure 2B). Beta diversity showed significant differences between these two years as seen with Bray–Curtis distances [Adonis test: F(1,71) = 5.8841, p = 0.001]. In addition, beta disperse analysis indicated a greater variance among samples from the 2nd year [Figures 3A,B; ANOVA F(1,70) = 5.982, p = 0.016].
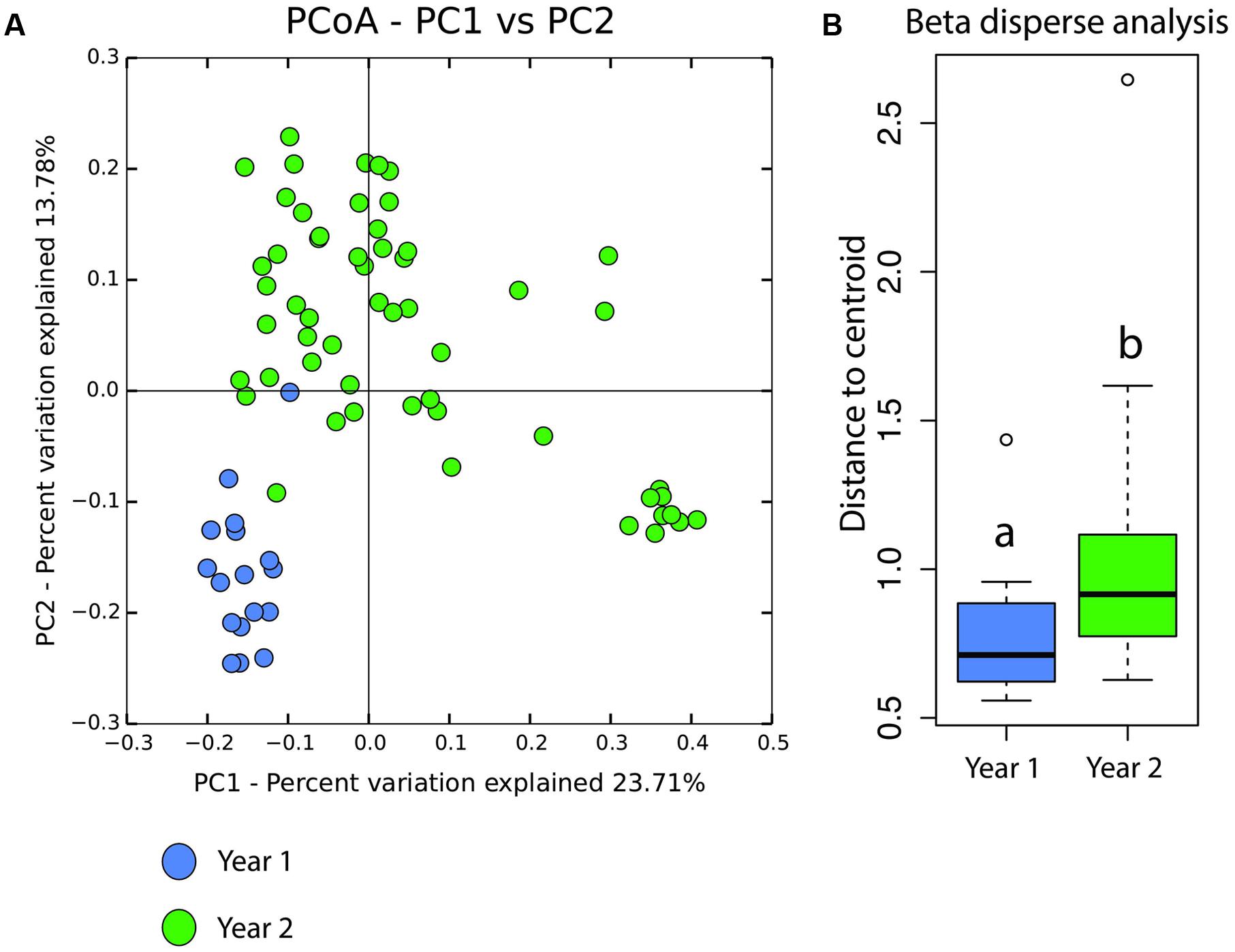
FIGURE 3. (A) Beta diversity of P. loveridgei between year 1 (blue) and year 2 (green). Principal coordinate analysis is based on weighted Unifrac distances [ADONIS F(1,71) = 5.8841, P = 0.001]. (B) Analysis of multivariate homogeneity of group dispersions (variance) of P. loveridgei skin microbial communities. Box plot of distances to each centroid’s group. Different letters (a and b) signify statistically significant differences between year 1 and year 2 [ANOVA F(1,70) = 5.9827, P = 0.01696].
With the aim to determine which OTUs significantly differed between years, a LEfse analysis was performed. This analysis determined that 569 out of 7673 OTUs (7.4% of the total OTUs) were significantly different and more abundant in year 1. In contrast, in year 2, only seven OTUs from the classes Alphaproteobacteria, Gammaproteobacteria, and Bacilli had a higher relative abundance (Supplementary Table 1).
Climatic Differences between Years
Differences between years 1 and 2 may be caused by several factors, but we found that rainfall patterns varied significantly between the two breeding seasons evaluated in this study (Figure 4). Specifically December and January, the rainy month of the year, showed significantly higher mean monthly rainfall in year 1 when compared to year 2 (Dec: ρ = 0.024; Jan: ρ = 0.024) (Figure 4). The 1st year being a “La Niña” year, which was characterized by heavy summer rainfalls, compared to a neutral phase year for the 2nd year. Furthermore, the “La Niña” year of the 1st year of the study (2011) followed a stronger “La Niña” event of 2010, bringing the highest two years of Australian rainfall on record, including heavy rainfall at the study area. Additionally, 2010 and 2011 were the coolest years recorded since 2001 due to the two consecutive “La Niña” events (CSIRO, 2012). Year 2 was a neutral year and Australia’s warmest year on record, with widespread warmth throughout the year, and below average rainfall. Particularly, summer rainfall at the study area was in the lowest 10% of records, with some small areas recording their lowest summer rainfall on record. Additionally, year 2 (2013) was preceded by 2012 climatic patterns which reflected a shift from a “La Niña” year to a neutral year, but warmer than average (CSIRO, 2014).
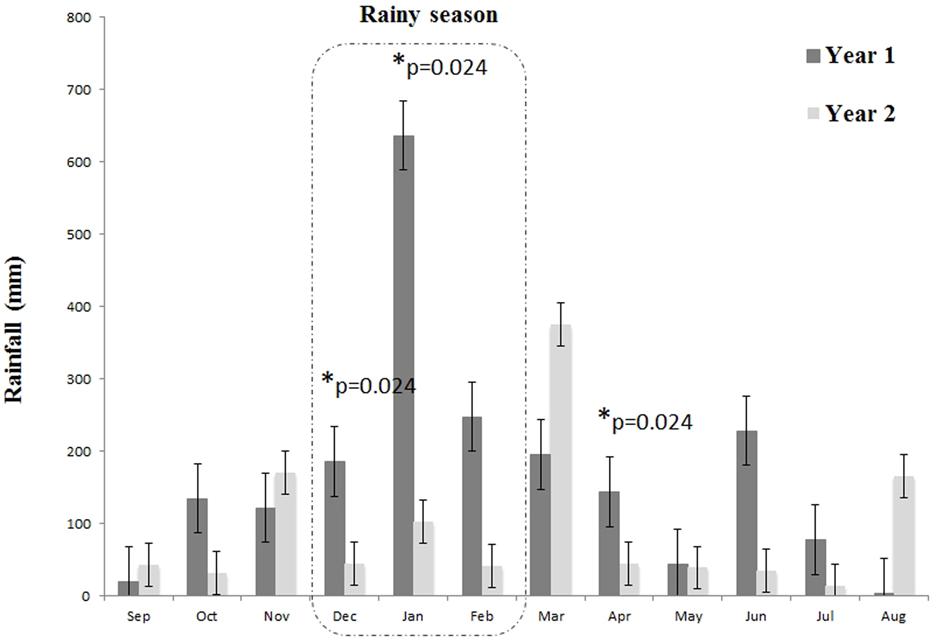
FIGURE 4. Rainfall levels during the two study years including the breeding seasons were P. loveridgei frogs were surveyed. Asterisks indicate months were year 1 was significantly higher than year 2 according to t-test.
Correlation between Bd Infection Intensity and Skin Bacterial Diversity in Two Breeding Seasons
To determine the correlation between Bd infection status and the skin bacterial community structure we only analyzed the data from year 2, as Bd was not detected in year 1 and there were significant differences on the bacterial communities between years 1 and 2. We found no significant differences between Bd status (infected/not infected) based on bacterial alpha [Supplementary Figure 1A; ANOVA F(1,70) = 2.579, p = 0.113] and beta diversity estimates [Supplementary Figure 1B; Adonis test: F(1,71) = 1.707, p = 0.07]. However, when analyzing Bd infection loads we found positive significant Spearman correlations between Bd infection intensity and the relative abundance of 67 bacterial OTUs (Supplementary Table 2). These OTUs had a higher relative abundance in infected individuals than in non-infected individuals (Figure 5A) and Wilcoxon rank sum test indicated significant differences between these two groups (W = 4102, p < 2.2 × 10-16). OTUs with a higher relative abundance in Bd-infected individuals were classified in eight different bacterial phyla and one archaeal phylum (Figure 5B). Moreover, of the 67 OTUs, 41 (63%) were from the phylum Proteobacteria and 29 of these OTUs (45%) were from the family Enterobacteriaceae. Interestingly, one specific OTU from the family Enterobacteriaceae accounted for 19% of the relative abundance in Bd-infected frogs (Figure 5B).
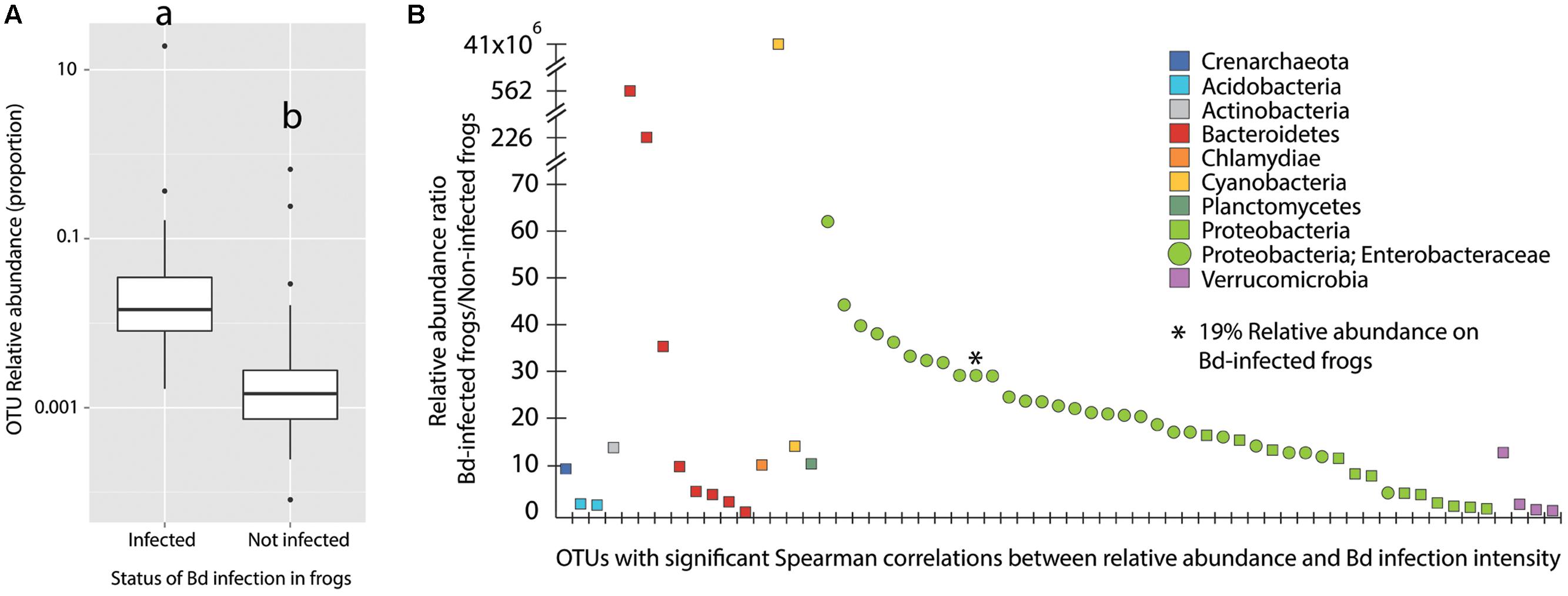
FIGURE 5. (A) Relative abundance of 67 bacterial OTUs with a significant Spearman correlation (Bd loads vs. OTUs relative abundance) on Bd-infected individuals in comparison to non-infected individuals. Different letters (a and b) signify statistically significant differences, as indicated by a Wilcoxon rank sum test (W = 4102, p-value < 2.2e–16). (B) Relative abundance ratio (Bd-infected frogs/Non-infected frogs) of OTUs with a significant Spearman correlation. Y-axis was adapted to fit the highest values.
Discussion
In this study, we found differences on Bd status and skin microbial communities on P. loveridgei populations across the two years in the breeding season. Overall, even though sampling sites differed on altitude, temporal variation across the two breeding seasons was the factor that better explained the changes in Bd infection status and bacterial community structure of wild populations of P. loveridgei. Importantly, these two breeding seasons differed dramatically on their climatic conditions, with the 1st year being part of “la Niña year” characterized by high precipitation levels.
We found Bd in two wild populations of P. loveridgei at low (683 m) and mid-high (977 m) altitude subtropical rainforest sites in mid-eastern Australia in only one year of the two that were surveyed. Our results indicate low infection intensity and low disease prevalence in this frog species, however, we still lack knowledge on the impact that this disease may have on P. loveridgei wild populations. Our findings of low infection intensity are in agreement with those observed in two other Australian terrestrial species C. ornatus and A. darlingtoni, where Bd infection was also relatively low (31 and 189 zoospore equivalents, respectively) (Kriger and Hero, 2006, 2007). B. dendrobatidis presence and infection intensity on P. loveridgei had not been reported before, yet our study suggests that P. loveridgei populations are likely to be exposed to the pathogen throughout their altitudinal range. Moreover, some of the subtropical rainforest areas and lowlands that bordered our sites were previously known to have undergone disease outbreaks, with various stream breeding frog species found to be infected with Bd (Berger et al., 2004; Kriger and Hero, 2008; Narayan et al., 2014). Furthermore, disease dynamics in P. loveridgei may depend on additional variables associated to changes in climatic conditions, such as temperature and precipitation as reported for other species (Longo et al., 2010). For example, prevalence was related to annual environmental changes in Litoria wilcoxii (a stream breeding frog), with higher prevalence levels in winter and early spring months (Kriger and Hero, 2008). Similarly, a multi-species study of wild frogs from Queensland and New South Wales reported a higher incidence of chytridiomycosis during winter months compared to other seasons of the year, when mean temperatures are low and in the optimal growth range of Bd compared with summer temperatures which are higher and can restrict Bd (Berger et al., 2004). Interestingly, a study on wild populations of two direct-developing frogs, E. coqui and E. portoricensis, found an association between Bd prevalence and infection intensity and seasons (Longo et al., 2010). The authors proposed a lower infection intensity in the wet and warm season and a higher in the dry and cool, as a result of the climatic differences but also of differential behavioral patterns (Longo et al., 2010).
The Bd infection intensity and prevalence patterns we observed in P. loveridgei suggest an enzootic state between the host and the pathogen. Despite finding populations of P. loveridgei infected with Bd, we found no clinical signs of chytridiomycosis or death related to this illness, nor have they been reported elsewhere. Additionally, no population declines related to Bd have been documented for any Australian direct-developing amphibian species (Hines et al., 1999; Hero et al., 2006; Hauselberger and Alford, 2012) in contrast with reports from other parts of the world (Bell et al., 2004; Burrowes et al., 2004; Lips et al., 2006; Longo and Burrowes, 2010; Longo et al., 2013). These results imply that Australian direct-developing amphibian species are surviving in an enzootic state with Bd perhaps through an innate immune response that could be providing them with protection against Bd, or by harboring beneficial skin microbiota. Brem and Lips (2008) suggest that terrestrial amphibians may share similar skin microbial fauna that provides them with protection to Bd and in turn make them less susceptible as a group. A study of four Australian frogs showed higher Bd resistance in species with powerful skin peptide defenses when experimentally infected with Bd (Woodhams et al., 2007b). Other studies have shown the inhibitory role of skin bacteria in the growth of Bd (Harris et al., 2006, 2009; Woodhams et al., 2007a; Walke et al., 2011). In wild P. loveridgei populations, higher Bd infection intensity may be found in seasons of the year we could not sample (e.g., cool and dry winter). Individuals of this fossorial cryptic frog species remain in their underground burrows during non-breeding months, making sampling extremely difficult. Because high Bd infection intensity implies susceptibility to chytridiomycosis (Vredenburg et al., 2010), the low level we discovered in this frog species could suggest resistance or tolerance to the infection, due to a more effective immune system, antimicrobial skin peptides or the presence of skin microbiota (Harris et al., 2009; Rollins-Smith, 2009).
We found differences in skin bacterial community structure across the two years in the breeding seasons. A high diversity of bacteria was found during the rainy and cool year (year 1: 2011), which was characterized by a higher abundance of Alphaproteobacteria. This was followed by an overall decrease on diversity during year 2 (2013) and the increase in abundance of Gammaproteobacteria and Actinobacteria. Interestingly, the 2nd year was the only time where Bd was detected on these frogs. The decrease of bacterial diversity has been correlated with the presence of Bd in previous field studies (Jani and Briggs, 2014; Rebollar et al., 2016b; Longo and Zamudio, 2017b). In Rana sierrae wild populations, Bd infection was strongly correlated with bacterial community composition with several taxa decreasing in abundance (Betaproteobacteria, Gammaproteobacteria, Actinobacteria and also a few members from the Acidobacteria and Alphaproteobacteria) (Jani and Briggs, 2014). Similarly, on a field survey of the terrestrial Panamanian frog Craugastor fitzingeri, Bd presence seemed to influence skin bacterial community structure with the Bd-naïve site dominated by OTUs from the genus Acinetobacter (Gammaproteobacteria) compared to the Bd-endemic sites dominated by Pseudomonas (Gammaproteobacteria), Cellulomonas and Sanguibacter (both Actinobacteria) (Rebollar et al., 2016b). Proteobacteria were also the most abundant phyla in skin samples of R. sphenocephala (Holden et al., 2015). Interestingly, this study found isolates of Proteobacteria and Actinobacteria that are able to inhibit the growth of Bd.
The changes in microbial diversity observed between breeding seasons may be due to several factors including climatic fluctuations (precipitation and temperature) as seen in previous studies (Longo and Zamudio, 2017a,b; Muletz Wolz et al., 2017; Sabino-Pinto et al., 2017). Climatic conditions may in turn influence host behavior, host susceptibility to pathogens, as well as Bd infection capacity (Longo et al., 2010; Longo and Zamudio, 2017a,b). For example, aggregation behavior was observed in two direct-developing frogs, E. coqui and E. portoricensis, during the dry season, while dispersion of the host occurred during the wet season (Longo et al., 2010). Heavily infected terrestrial frogs may remain inactive and hidden in fossorial retreats under dry and cool conditions, hindering their detection. In contrast, during warm and wet seasons frogs have lower infection levels and are more active in the forest, increasing the probability of sampling an infected frog and yielding higher prevalence estimates (Longo et al., 2010). Furthermore, climatic conditions can also lead to changes in microbial communities and in turn Bd infection and prevalence (Longo and Zamudio, 2017a). In another study of the two direct-developing frogs, Bd infection may have been limited during the warm and wet season by recruitment of putatively beneficial bacteria followed by a return to pre-infected levels of bacteria richness and diversity was observed (Longo and Zamudio, 2017a). Additionally, during the cool and dry season Bd infection increased through time and bacterial diversity did not change (Longo and Zamudio, 2017a). Overall, host-pathogen interactions may be strongly influenced by climatic fluctuations as seen in previous studies. Thus, we hypothesize that changes in climatic conditions between the two years in the breeding seasons that we surveyed could explain the presence of Bd and the decrease of skin microbial diversity. Thus, climatic changes may directly influence the skin bacteria community as well as the success of Bd to infect host, but also there might be an interaction occurring between the skin symbiotic community structure and the presence of the pathogen on the skin. However, it is important to consider that, besides climate, our results could be explained by other factors that we did not consider in this study.
Our study identified a group of 67 bacterial taxa that were enriched on the frogs from year 2, which were characterized by harboring higher Bd loads (significant positive Spearman correlations). A great proportion of these OTUs belonged specifically to the family Enterobacteriaceae. Interestingly, a data base for culturable Bd inhibitory bacteria published by Woodhams et al. (2015) showed that 94% of the culturable bacteria from this family inhibit Bd growth. Our field survey suggests that the increase in the abundance of Enterobacteriaceae OTUs might be related to Bd infection, e.g., these bacteria may be recruited to inhibit the pathogen or may be a consequence of Bd infection on the skin. To further test this hypothesis additional experimental studies will need to be performed.
Overall, our study contributes on the understanding of the role of temporal variation on Bd infection and skin microbial community structure. However, in order to fully determine an effect of the climatic conditions on the skin microbial structure and pathogen infection, a long term study is essential. This study supports previous findings showing that Bd estimates and microbial community description are not static and therefore require multiple temporal and spatial samplings to fully understand the ecological interactions occurring between the host, the pathogen and bacterial symbiotic communities.
Author Contributions
MFL contributed to the conception of the idea and design of the research, and carried out the fieldwork. MFL, ER, and VV contributed with laboratory analysis. MFL and ER analyzed the data with advise from RH and J-MH. All authors contributed with the interpretation of the data. MFL and ER wrote the manuscript and all authors provided critical feedback.
Funding
This research project was funded by the NSF Dimensions in Biodiversity Program: DEB-1136602 to RH and by NFS 1258133 to VV. Funding from Tricia Waters (Office of Environment and Heritage) and the Advisory Committees of the Gondwana Rainforests of Australia World Heritage Area to MFL and J-MH are acknowledged. MFL was also partially funded by School of Environment, Griffith University.
Conflict of Interest Statement
The authors declare that the research was conducted in the absence of any commercial or financial relationships that could be construed as a potential conflict of interest.
Acknowledgments
We are grateful for the frogs that made this research possible. A special thank you goes to all field volunteers from Griffith University.
Supplementary Material
The Supplementary Material for this article can be found online at: https://www.frontiersin.org/articles/10.3389/fmicb.2017.02535/full#supplementary-material
Footnotes
References
Afgan, E., Baker, D., Van den Beek, M., Blankenberg, D., Bouvier, D., Èech, M., et al. (2016). The galaxy platform for accessible, reproducible and collaborative biomedical analyses: 2016 update. Nucleic Acids Res 44, W3–W10. doi: 10.1093/nar/gkw343
Assis, A. B., Barreto, C. C., and Navas, C. A. (2017). Skin microbiota in frogs from the Brazilian Atlantic forest: species, forest type, and potential against pathogens. PLOS ONE 12:e0179628. doi: 10.1371/journal.pone.0179628
Becker, M. H., and Harris, R. N. (2010). Cutaneous bacteria of the redback salamander prevent morbidity associated with a lethal disease. PLOS ONE 5:e10957. doi: 10.1371/journal.pone.0010957
Becker, M. H., Walke, J. B., Cikanek, S., Savage, A. E., Mattheus, N., Santiago, C. N., et al. (2015). Composition of symbiotic bacteria predicts survival in Panamanian golden frogs infected with a lethal fungus. Proc. R. Soc. Lond. B Biol. Sci. 282:20142881. doi: 10.1098/rspb.2014.2881
Belden, L. K., Hughey, M. C., Rebollar, E. A., Umile, T. P., Loftus, S. C., Burzynski, E. A., et al. (2015). Panamanian frog species host unique skin bacterial communities. Front. Microbiol. 6:1171. doi: 10.3389/fmicb.2015.01171
Bell, B. D., Carver, S., Mitchell, N. J., and Pledger, S. (2004). The recent decline of a New Zealand endemic: how and why did populations of Archey’s frog Leiopelma archeyi crash over 1996–2001? Biol. Conserv. 120, 189–199. doi: 10.1016/j.biocon.2004.02.011
Berger, L., Speare, R., Daszak, P., Green, D. E., Cunningham, A. A., Goggin, C. L., et al. (1998). Chytridiomycosis causes amphibian mortality associated with population declines in the rain forests of Australia and Central America. Proc. Natl. Acad. Sci. U.S.A. 95, 9031–9036. doi: 10.1073/pnas.95.15.9031
Berger, L., Speare, R., Hines, H., Marantelli, G., Hyatt, A., McDonald, K. R., et al. (2004). Effect of season and temperature on mortality in amphibians due to chytridiomycosis. Aust. Vet. J. 82, 434–439. doi: 10.1111/j.1751-0813.2004.tb11137.x
Berger, L., Spear, R., and Skerratt, L. F. (2005). Distribution of Batrachochytrium dendrobatidis and pathology in the skin of green tree frogs Litoria caerulea with severe chytridiomycosis. Dis. Aquat. Organ. 68, 65–70. doi: 10.3354/dao068065
Bokulich, N. A., Subramanian, S., Faith, J. J., Gevers, D., Gordon, J. I., Knight, R., et al. (2013). Quality-filtering vastly improves diversity estimates from Illumina amplicon sequencing. Nat. Methods 10, 57–59. doi: 10.1038/nmeth.2276
Bosch, J., Martìnez-Solano, I., and Garcìa-Parìs, M. (2001). Evidence of a chytrid fungus infection involved in the decline of the common midwife toad (Alytes obstetricans) in protected areas of central Spain. Biol. Conserv. 97, 331–337. doi: 10.1016/s0006-3207(00)00132-4
Boyle, D., Boyle, D., Olsen, V., Morgan, J., and Hyatt, A. (2004). Rapid quantitative detection of chytridiomycosis (Batrachochytrium dendrobatidis) in amphibian samples using real-time Taqman PCR assay. Dis. Aquat. Organ. 60, 141–148. doi: 10.3354/dao060141
Brem, F., Mendelson, J. R. III., and Lips, K. R. (2007). Field-Sampling Protocol for Batrachochytrium dendrobatidis from Living Amphibians, Using Alcohol Preserved Swabs. Version 1.0. Arlington, VA: Conservation International.
Brem, F. M. R., and Lips, K. R. (2008). Batrachochytrium dendrobatidis infection patterns among Panamanian amphibian species, habitats and elevations during epizootic and enzootic stages. Dis. Aquat. Organ. 81, 189–202. doi: 10.3354/dao01960
Briggs, C. J., Knapp, R. A., and Vredenburg, V. T. (2010). Enzootic and epizootic dynamics of the chytrid fungal pathogen of amphibians. Proc. Natl. Acad. Sci. U.S.A. 107, 9695–9700. doi: 10.1073/pnas.0912886107
Burrowes, P. A., Joglar, R. L., and Green, D. E. (2004). Potential causes for amphibian declines in puerto rico. Herpetologica 60, 141–154. doi: 10.1655/03-50
Caporaso, J. G., Bittinger, K., Bushman, F. D., DeSantis, T. Z., Andersen, G. L., and Knight, R. (2010a). PyNAST: a flexible tool for aligning sequences to a template alignment. Bioinformatics 26, 266–267. doi: 10.1093/bioinformatics/btp636
Caporaso, J. G., Kuczynski, J., Stombaugh, J., Bittinger, K., Bushman, F. D., Costello, E. K., et al. (2010b). QIIME allows analysis of high-throughput community sequencing data. Nat. Methods 7, 335–336. doi: 10.1038/nmeth.f.303
Caporaso, J. G., Lauber, C. L., Walters, W. A., Berg-Lyons, D., Lozupone, C. A., Turnbaugh, P. J., et al. (2011). Global patterns of 16S rRNA diversity at a depth of millions of sequences per sample. Proc. Natl. Acad. Sci. U.S.A. 108(Suppl. 1), 4516–4522. doi: 10.1073/pnas.1000080107
Catenazzi, A., Lehr, E., Rodriguez, L., and Vredenburg, V. (2011). Batrachochytrium dendrobatidis and the collapse of anuran species richness and abundance in the upper Manu National Park, southeastern Peru. Conserv. Biol. 25, 382–391. doi: 10.1111/j.1523-1739.2010.01604.x
Clemente, J. C., Pehrsson, E. C., Blaser, M. J., Sandhu, K., Gao, Z., Wang, B., et al. (2015). The microbiome of uncontacted Amerindians. Sci. Adv. 1:e1500183. doi: 10.1126/sciadv.1500183
Collins, J. P., and Storfer, A. (2003). Global amphibian declines: sorting the hypotheses. Div. Distrib. 9, 89–98. doi: 10.1046/j.1472-4642.2003.00012.x
CSIRO (2012). State of the Climate 2012. Melbourne, VIC: Australian Government Bureau of Meteorology.
CSIRO (2014). State of the Climate 2014. Melbourne, VIC: Australian Government Bureau of Meteorology.
Drew, A., Allen, E. J., and Allen, L. J. S. (2006). Analysis of climatic and geographic factors affecting the presence of chytridiomycosis in Australia. Dis. Aquat. Organ. 68, 245–250. doi: 10.3354/dao068245
Edgar, R. C. (2010). Search and clustering orders of magnitude faster than BLAST. Bioinformatics 26, 2460–2461. doi: 10.1093/bioinformatics/btq461
Fitzpatrick, B. M., and Allison, A. L. (2014). Similarity and differentiation between bacteria associated with skin of salamanders (Plethodon jordani) and free-living assemblages. FEMS Microbiol. Ecol. 88, 482–494. doi: 10.1111/1574-6941.12314
Frías-Alvarez, P., Vredenburg, V. T., Familiar-López, M., Longcore, J. E., González-Bernal, E., Santos-Barrera, G., et al. (2008). Chytridiomycosis survey in wild and captive Mexican amphibians. EcoHealth 5, 18–26. doi: 10.1007/s10393-008-0155-3
Garland, S., Baker, A., Phillott, A. D., and Skerratt, L. F. (2010). BSA reduces inhibition in a TaqMan assay for the detection of Batrachochytrium dendrobatidis. Dis. Aquat. Organ. 92, 113–116. doi: 10.3354/dao02053
Harris, R., James, T., Lauer, A., Simon, M., and Patel, A. (2006). Amphibian pathogen Batrachochytrium dendrobatidis is inhibited by the cutaneous bacteria of amphibian species. EcoHealth 3, 53–56. doi: 10.1007/s10393-005-0009-1
Harris, R. N., Lauer, A., Simon, M. A., Banning, J. L., and Alford, R. A. (2009). Addition of antifungal skin bacteria to salamanders ameliorates the effects of chytridiomycosis. Dis. Aquat. Organ. 83, 11–16. doi: 10.3354/dao02004
Hauselberger, K. F., and Alford, R. A. (2012). Prevalence of Batrachochytrium dendrobatidis infection is extremely low in direct-developing Australian microhylids. Dis. Aquat. Organ. 100, 191–200. doi: 10.3354/dao02494
Hero, J.-M., Morrison, C., Gillespie, G., Roberts, J. D., Newell, D., Meyer, E., et al. (2006). Overview of the conservation status of Australian frogs. Pac. Conserv. Biol. 12:313. doi: 10.1071/PC060313
Hines, H., Mahony, M., and McDonald, K. (1999). “An assessment of frog declines in wet subtropical Australia,” in Declines and Disappearances of Australian Frogs, ed. A. Campbell (Canberra, ACT: Environment Australia), 44–63.
Holden, W. M., Hanlon, S. M., Woodhams, D. C., Chappell, T. M., Wells, H. L., Glisson, S. M., et al. (2015). Skin bacteria provide early protection for newly metamorphosed southern leopard frogs (Rana sphenocephala) against the frog-killing fungus, Batrachochytrium dendrobatidis. Biol. Conserv. 187, 91–102. doi: 10.1016/j.biocon.2015.04.007
Hyatt, A. D., Boyle, D. G., Olsen, V., Boyle, D. B., Berger, L., Obendorf, D., et al. (2007). Diagnostic assays and sampling protocols for the detection of Batrachochytrium dendrobatidis. Dis. Aquat. Organ. 73, 175–192. doi: 10.3354/dao073175
Jani, A. J., and Briggs, C. J. (2014). The pathogen Batrachochytrium dendrobatidis disturbs the frog skin microbiome during a natural epidemic and experimental infection. Proc. Natl. Acad. Sci. U.S.A. 111, E5049–E5058. doi: 10.1073/pnas.1412752111
Jiménez, R. R., and Sommer, S. (2016). The amphibian microbiome: natural range of variation, pathogenic dysbiosis, and role in conservation. Biodiver. Conserv. 26, 763–786. doi: 10.1007/s10531-016-1272-x
Kilpatrick, A. M., Briggs, C. J., and Daszak, P. (2010). The ecology and impact of chytridiomycosis: an emerging disease of amphibians. Trends Ecol. Evolut. 25, 109–118. doi: 10.1016/j.tree.2009.07.011
Knowles, R., Mahony, M., Armstrong, J., and Donnellan, S. (2004). Systematics of sphagnum frogs of the Genus Philoria (Anura: Myobatrachidae) in Eastern Australia, with the description of two new species. Rec. Aust. Mus. 56, 57–74. doi: 10.3853/j.0067-1975.56.2004.1391
Kriger, K. M., and Hero, J.-M. (2006). Cophixalus ornatus (Ornate Nursery Frog) chytridiomycosis. Herpetol. Rev. 37:443.
Kriger, K. M., and Hero, J.-M. (2007). Large-scale seasonal variation in the prevalence and severity of chytridiomycosis. J. Zool. 271, 352–359. doi: 10.1111/j.1469-7998.2006.00220.x
Kriger, K. M., and Hero, J.-M. (2008). Altitudinal distribution of chytrid (Batrachochytrium dendrobatidis) infection in subtropical Australian frogs. Austral. Ecol. 33, 1022–1032. doi: 10.1111/j.1442-9993.2008.01872.x
Kriger, K. M., Hero, J.-M., and Ashton, K. J. (2006a). Cost efficiency in the detection of chytridiomycosis using PCR assay. Dis. Aquat. Organ. 71, 149–154.
Kriger, K. M., Hines, H., Hyatt, A. D., Boyle, D. G., and Hero, J.-M. (2006b). Techniques for detecting chytridiomycosis in wild frogs: comparing histology with real-time Taqman PCR. Dis. Aquat. Organ. 71, 141–148.
Kriger, K. M., Pereoglou, F., and Hero, J.-M. (2007). Latitudinal variation in the prevalence and intensity of chytrid (Batrachochytrium dendrobatidis) infection in eastern Australia. Conserv. Biol. 21, 1280–1290. doi: 10.1111/j.1523-1739.2007.00777.x
Krynak, K. L., Burke, D. J., and Benard, M. F. (2015). Larval environment alters amphibian immune defenses differentially across life stages and populations. PLOS ONE 10:e0130383. doi: 10.1371/journal.pone.0130383
Krynak, K. L., Burke, D. J., and Benard, M. F. (2016). Landscape and water characteristics correlate with immune defense traits across Blanchard’s cricket frog (Acris blanchardi) populations. Biol. Conserv. 193, 153–167. doi: 10.1016/j.biocon.2015.11.019
Kueneman, J. G., Parfrey, L. W., Woodhams, D. C., Archer, H. M., Knight, R., and McKenzie, V. J. (2014). The amphibian skin-associated microbiome across species, space and life history stages. Mol. Ecol. 23, 1238–1250. doi: 10.1111/mec.12510
Kueneman, J. G., Woodhams, D. C., Van Treuren, W., Archer, H. M., Knight, R., and McKenzie, V. J. (2016). Inhibitory bacteria reduce fungi on early life stages of endangered Colorado boreal toads (Anaxyrus boreas). Int. Soc. Microb. Ecol. J. 10, 934–944. doi: 10.1038/ismej.2015.168
Lips, K. R., Brem, F., Brenes, R., Reeve, J. D., Alford, R. A., Voyles, J., et al. (2006). Emerging infectious disease and the loss of biodiversity in a Neotropical amphibian community. Proc. Natl. Acad. Sci. U.S.A. 103, 3165–3170. doi: 10.1073/pnas.0506889103
Longcore, J. E., Pessier, A. P., and Nichols, D. K. (1999). Batrachochytrium dendrobatidis gen. et sp. nov., a chytrid pathogenic to amphibians. Mycologia 91, 219–227. doi: 10.2307/3761366
Longo, A. V., and Burrowes, P. A. (2010). Persistence with chytridiomycosis does not assure survival of direct-developing frogs. Ecohealth 7, 185–195. doi: 10.1007/s10393-010-0327-9
Longo, A. V., Burrowes, P. A., and Joglar, R. L. (2010). Seasonality of Batrachochytrium dendrobatidis infection in direct-developing frogs suggests a mechanism for persistence. Dis. Aquat. Organ. 92, 253–260. doi: 10.3354/dao02054
Longo, A. V., Ossiboff, R. J., Zamudio, K. R., and Burrowes, P. A. (2013). Lability in host defenses: terrestrial frogs die from chytridiomycosis under enzootic conditions. J. Wildl. Dis. 49, 197–199. doi: 10.7589/2012-05-129
Longo, A. V., Savage, A. E., Hewson, I., and Zamudio, K. R. (2015). Seasonal and ontogenetic variation of skin microbial communities and relationships to natural disease dynamics in declining amphibians. R. Soc. Open Sci. 2:140377. doi: 10.1098/rsos.140377
Longo, A. V., and Zamudio, K. R. (2017a). Environmental fluctuations and host skin bacteria shift survival advantage between frogs and their fungal pathogen. Int. Soc. Microb. Ecol. J. 11, 349–361. doi: 10.1038/ismej.2016.138
Longo, A. V., and Zamudio, K. R. (2017b). Temperature variation, bacterial diversity, and fungal infection dynamics in the amphibian skin. Mol. Ecol. 26, 4787–4797. doi: 10.1111/mec.14220
Loudon, A. H., Venkataraman, A., Van Treuren, W., Woodhams, D. C., Parfrey, L. W., McKenzie, V. J., et al. (2016). Vertebrate hosts as islands: dynamics of selection, immigration, loss, persistence, and potential function of bacteria on salamander skin. Front. Microbiol. 7:333. doi: 10.3389/fmicb.2016.00333
Loudon, A. H., Woodhams, D. C., Parfrey, L. W., Archer, H., Knight, R., McKenzie, V., et al. (2014). Microbial community dynamics and effect of environmental microbial reservoirs on red-backed salamanders (Plethodon cinereus). Int. Soc. Microb. Ecol. J. 8, 830–840. doi: 10.1038/ismej.2013.200
Martel, A., Spitzen-van der Sluijs, A., Blooi, M., Bert, W., Ducatelle, R., Fisher, M. C., et al. (2013). Batrachochytrium salamandrivorans sp. nov. causes lethal chytridiomycosis in amphibians. Proc. Natl. Acad. Sci. 110, 15325–15329. doi: 10.1073/pnas.1307356110
McDonald, B. (2010). “The tweed caldera group,” in Remnants of Gondwana: a Natural and Social History of the Gondwana Rainforests of Australia, eds R. Kitching, R. Braithwaite, and J. Cavanaugh (Chipping Norton, NSW: Surrey Beatty & Sons), 39–50.
McDonald, D., Price, M. N., Goodrich, J., Nawrocki, E. P., DeSantis, T. Z., Probst, A., et al. (2012). An improved greengenes taxonomy with explicit ranks for ecological and evolutionary analyses of bacteria and archaea. Int. Soc. Microb. Ecol. J. 6, 610–618. doi: 10.1038/ismej.2011.139
McDonald, K., Mendez, D., Müller, R., Freeman, A., and Speare, R. (2005). Decline in the prevalence of chytridiomycosis in frog populations in North Queensland, Australia. Pac. Conserv. Biol. 11, 114–120. doi: 10.1071/PC050114
McKenzie, V. J., Bowers, R. M., Fierer, N., Knight, R., and Lauber, C. L. (2012). Co-habiting amphibian species harbor unique skin bacterial communities in wild populations. Int. Soc. Microb. Ecol. J. 6, 588–596. doi: 10.1038/ismej.2011.129
Medina, D., Hughey, M. C., Becker, M. H., Walke, J. B., Umile, T. P., Burzynski, E. A., et al. (2017). Variation in metabolite profiles of amphibian skin bacterial communities across elevations in the Neotropics. Microb. Ecol. 74, 227–238. doi: 10.1007/s00248-017-0933-y
Muletz Wolz, C. R., Yarwood, S. A., Grant, E. H. C., Fleischer, R. C., and Lips, K. R. (2017). Effects of host species and environment on the skin microbiome of Plethodontid salamanders. J. Anim. Ecol. doi: 10.1111/1365-2656.12726 [Epub ahead of print].
Muths, E., Pilliod, D. S., and Livo, L. J. (2008). Distribution and environmental limitations of an amphibian pathogen in the Rocky Mountains, USA. Biol. Conserv. 141, 1484–1492. doi: 10.1016/j.biocon.2008.03.011
Narayan, E. J., Graham, C., McCallum, H., and Hero, J.-M. (2014). Over-wintering tadpoles of Mixophyes fasciolatus act as reservoir host for Batrachochytrium dendrobatidis. PLOS ONE 9:e92499. doi: 10.1371/journal.pone.0092499
Oksanen, J., Kindt, R., Legendre, P., O’Hara, B., Stevens, M. H. H., Oksanen, M. J., et al. (2015). vegan: Community Ecology Package, R Package Version 2.2–1. Available at: https://github.com/vegandevs/vegan
Ouellet, M., Mikaelian, I., Pauli, B. D., Rodrigue, J., and Green, D. M. (2005). Historical evidence of widespread chytrid infection in North American amphibian populations. Conserv. Biol. 19, 1431–1440. doi: 10.1111/j.1523-1739.2005.00108.x
Phillott, A. D., Speare, R., Hines, H. B., Skerratt, L. F., Meyer, E., McDonald, K. R., et al. (2010). Minimising exposure of amphibians to pathogens during field studies. Dis. Aquat. Organ. 92, 175–185. doi: 10.3354/dao02162
Price, M. N., Dehal, P. S., and Arkin, A. P. (2010). FastTree 2–approximately maximum-likelihood trees for large alignments. PLOS ONE 5:e9490. doi: 10.1371/journal.pone.0009490
R Core Team (2015). R: A Language and Environment for Statistical Computing. Vienna: R Foundation for Statistical Computing. Availabel at: http://www.R-project.org/
Rebollar, E. A., Antwis, R. E., Becker, M. H., Belden, L. K., Bletz, M. C., Brucker, R. M., et al. (2016a). Using “Omics” and integrated multi-omics approaches to guide probiotic selection to mitigate chytridiomycosis and other emerging infectious diseases. Front. Microbiol. 7:68. doi: 10.3389/fmicb.2016.00068
Rebollar, E. A., Hughey, M. C., Harris, R. N., Domangue, R. J., Medina, D., Ibáñez, R., et al. (2014). The lethal fungus Batrachochytrium dendrobatidis is present in lowland tropical forests of far eastern Panamá. PLOS ONE 9:e95484. doi: 10.1371/journal.pone.0095484
Rebollar, E. A., Hughey, M. C., Medina, D., Harris, R. N., Ibáñez, R., and Belden, L. K. (2016b). Skin bacterial diversity of Panamanian frogs is associated with host susceptibility and presence of Batrachochytrium dendrobatidis. ISME J. 10, 1682–1695. doi: 10.1038/ismej.2015.234
Retallick, R. W. R., McCallum, H., and Speare, R. (2004). Endemic infection of the amphibian chytrid fungus in a frog community post-decline. PLOS Biol. 2:e351 doi: 10.1371/journal.pbio.0020351
Rollins-Smith, L. A. (2009). The role of amphibian antimicrobial peptides in protection of amphibians from pathogens linked to global amphibian declines. Biochim. Biophys. Acta 1788, 1593–1599. doi: 10.1016/j.bbamem.2009.03.008
Rowley, J. J. L., and Alford, R. A. (2013). Hot bodies protect amphibians against chytrid infection in nature. Sci. Rep. 3:1515. doi: 10.1038/srep01515
Sabino-Pinto, J., Galán, P., Rodríguez, S., Bletz, M. C., Bhuju, S., Geffers, R., et al. (2017). Temporal changes in cutaneous bacterial communities of terrestrial- and aquatic-phase newts (Amphibia). Environ. Microbiol. 19, 3025–3038. doi: 10.1111/1462-2920.13762
Segata, N., Izard, J., Waldron, L., Gevers, D., Miropolsky, L., Garrett, W. S., et al. (2011). Metagenomic biomarker discovery and explanation. Genome Biol. 12:R60. doi: 10.1186/gb-2011-12-6-r60
Simpkins, C., Van Sluys, M., and Hero, J.-M. (2014). Swabber effect: swabbing technique affects the detectability of Batrachochytrium dendrobatidis. Herpetol. Rev. 45, 443–445.
Skerratt, L., Berger, L., Speare, R., Cashins, S., McDonald, K., Phillott, A., et al. (2007). Spread of chytridiomycosis has caused the rapid global decline and extinction of frogs. Ecohealth 4, 125–134. doi: 10.1007/s10393-007-0093-5
Skerratt, L., McDonald, K., Hines, H., Berger, L., Mendez, D., Phillott, A., et al. (2010). Application of the survey protocol for chytridiomycosis to Queensland, Australia. Dis. Aquat. Organ. 92, 117–129. doi: 10.3354/dao02272
Stuart, S. N., Chanson, J. S., Cox, N. A., Young, B. E., Rodrigues, A. S., Fischman, D. L., et al. (2004). Status and trends of amphibian declines and extinctions worldwide. Science 306, 1783–1786. doi: 10.1126/science.1103538
Vredenburg, V. T., Knapp, R. A., Tunstall, T. S., and Briggs, C. J. (2010). Dynamics of an emerging disease drive large-scale amphibian population extinctions. Proc. Natl. Acad. Sci. U.S.A. 107, 9689–9694. doi: 10.1073/pnas.0914111107
Wake, D. B. (2012). Facing extinction in real time. Science 335, 1052–1053. doi: 10.1126/science.1218364
Wake, D. B., and Vredenburg, V. T. (2008). Are we in the midst of the sixth mass extinction? A view from the world of amphibians. Proc. Natl. Acad. Sci. U.S.A. 105(Suppl. 1), 11466–11473. doi: 10.1073/pnas.0801921105
Walke, J. B., Becker, M. H., Loftus, S. C., House, L. L., Cormier, G., Jensen, R. V., et al. (2014). Amphibian skin may select for rare environmental microbes. Int. Soc. Microb. Ecol. J. 8, 2207–2217. doi: 10.1038/ismej.2014.77
Walke, J. B., Becker, M. H., Loftus, S. C., House, L. L., Teotonio, T. L., Minbiole, K. P. C., et al. (2015). Community structure and function of amphibian skin microbes: an experiment with bullfrogs exposed to a chytrid fungus. PLOS ONE 10:e0139848. doi: 10.1371/journal.pone.0139848
Walke, J. B., and Belden, L. K. (2016). Harnessing the microbiome to prevent fungal infections: lessons from amphibians. PLOS Pathog. 12:e1005796. doi: 10.1371/journal.ppat.1005796
Walke, J. B., Harris, R. N., Reinert, L. K., Rollins-Smith, L. A., and Woodhams, D. C. (2011). Social immunity in amphibians: evidence for vertical transmission of innate defenses. Biotropica 43, 396–400. doi: 10.1111/j.1744-7429.2011.00787.x
Walker, S. F., Bosch, J., Gomez, V., Garner, T. W., Cunningham, A. A., Schmeller, D. S., et al. (2010). Factors driving pathogenicity vs. prevalence of amphibian panzootic chytridiomycosis in Iberia. Ecol. Lett. 13, 372–382. doi: 10.1111/j.1461-0248.2009.01434.x
Wang, Q., Garrity, G. M., Tiedje, J. M., and Cole, J. R. (2007). Naive Bayesian classifier for rapid assignment of rRNA sequences into the new bacterial taxonomy. Appl. Environ. Microbiol. 73, 5261–5267. doi: 10.1128/AEM.00062-07
Woodhams, D. C., and Alford, R. A. (2005). Ecology of chytridiomycosis in rainforest stream frog assemblages of tropical Queensland. Conserv. Biol. 19, 1449–1459. doi: 10.1111/j.1523-1739.2005.004403.x
Woodhams, D. C., Alford, R. A., Antwis, R. E., Archer, H., Becker, M. H., Belden, L. K., et al. (2015). Antifungal isolates database of amphibian skin-associated bacteria and function against emerging fungal pathogens. Ecology 96, 595–595. doi: 10.1890/14-1837.1
Woodhams, D. C., Ardipradja, K., Alford, R. A., Marantelli, G., Reinert, L. K., and Rollins-Smith, L. A. (2007a). Resistance to chytridiomycosis varies among amphibian species and is correlated with skin peptide defenses. Anim. Conserv. 10, 409–417. doi: 10.1111/j.1469-1795.2007.00130.x
Keywords: chytridiomycosis, skin bacteria, amphibians, Philoria loveridge, bacteria diversity
Citation: Familiar López M, Rebollar EA, Harris RN, Vredenburg VT and Hero J-M (2017) Temporal Variation of the Skin Bacterial Community and Batrachochytrium dendrobatidis Infection in the Terrestrial Cryptic Frog Philoria loveridgei. Front. Microbiol. 8:2535. doi: 10.3389/fmicb.2017.02535
Received: 07 September 2017; Accepted: 06 December 2017;
Published: 22 December 2017.
Edited by:
David Berry, University of Vienna, AustriaReviewed by:
David Vieites, Consejo Superior de Investigaciones Científicas (CSIC), SpainRoberta Fulthorpe, University of Toronto Scarborough, Canada
Copyright © 2017 Familiar López, Rebollar, Harris, Vredenburg and Hero. This is an open-access article distributed under the terms of the Creative Commons Attribution License (CC BY). The use, distribution or reproduction in other forums is permitted, provided the original author(s) or licensor are credited and that the original publication in this journal is cited, in accordance with accepted academic practice. No use, distribution or reproduction is permitted which does not comply with these terms.
*Correspondence: Mariel Familiar López, bWFyaWVsLmZhbWlsaWFybG9wZXpAZ3JpZmZpdGh1bmkuZWR1LmF1