- MOST-USDA Joint Research Center for Food Safety, School of Agriculture and Biology, and State Key Laboratory of Microbial Metabolism, Shanghai Jiao Tong University, Shanghai, China
Salmonella is one of the primary causes of foodborne disease, especially Salmonella enterica subsp. enterica (I) which has caused ~99% of clinical salmonellosis cases for humans and domestic mammals. The flagella genes, fliC and fljB, which encode the Salmonella phase 1 and phase 2 antigens respectively, are considered as the Salmonella serotype determinant genes, and contribute to the virulence of Salmonella. However, the evolution of the two flagellin genes is still not well-understood. In this study, the fliC and fljB gene clusters were analyzed among 205 S. enterica subspecies I genomes. The dataset covered 87 different serovars of S. enterica subsp. enterica and included 9 genomes (six serovars) of four other Salmonella subspecies. Based on a pan-genome definition and flanked gene linkages, the fliC and fljB gene clusters were identified in 207 (91 serovars) and 138 (61 serovars) genomes, respectively. A phylogenetic tree constructed based on SNPs (Single Nucleotide Polymorphisms) of core genes were used to reflect the essential evolutionary relationships among various serovars. Congruence analysis was performed among the core genome and each gene of fliC and fljB gene clusters, with only fliA and fliS showing congruence to Salmonella core genome. Congruence was also observed among fliB, fliC/fljB, and fliD genes, and their phylogeny revealed a division into two major groups, which strongly corresponded to monophasic and biphasic serovars. Besides, homologous recombination events referring fliB, fliC, and fliD were found to have mainly occurred within each group. These results suggested two distinct evolutionary patterns of Salmonella flagellin gene clusters. Further insight on the evolutionary implication of the two patterns and a framework for phase variation mechanism are needed to be further processed.
Introduction
Salmonella is one of the primary causes of foodborne illness around the world, leading to a huge burden of illness calculated at nearly 93.8 million global cases annually (Majowicz et al., 2010). For understanding the epidemiology of these microorganisms and the investigation of outbreaks caused by Salmonella, serotyping has been greatly facilitated by the characterization of isolates. The Salmonella genus is composed of two species, Salmonella bongori and Salmonella enterica, the latter being further classified into six subspecies, enterica (I), salamae (II), arizonae (IIIa), diarizonae (IIIb), houtenae (IV), and indica (VI) (Grimont and Weill, 2007). Salmonella is an extremely diverse species, of which more than 2,500 serovars has been reported. S. enterica subsp. enterica (I) strains, the vast majority of Salmonella strains, could be isolated from humans and warm-blooded animals, while all the other subspecies and S. bongori are more frequently isolated from cold-blooded animals (Nataro et al., 2011). Importantly, S. enterica subsp. enterica (I) has caused ~99% of diagnosed salmonellosis cases for humans and domestic mammals (Centers for Disease Control and Prevention (CDC), 2011). Strains with different serotypes show different levels of variation, a single host (e.g., S. Typhi) being adapted by monomorphic serotypes comprise a single strain in a single lineage, whilst polymorphic serotypes comprise multiple lineages of strains which may or may not be host adapted (e.g., S. Choleraesuis) (Wain and O'Grady, 2017).
Based on White-Kauffmann scheme, the Salmonella strains have been identified by their surface antigens, on the basis of somatic (O), flagellar (H), and capsular (Vi) antigens (Brenner et al., 2000) and their reactivity to antisera (Schrader et al., 2008). Flagella (fliC or fljB) protein is the primary globular protein forming the filament of flagellum in the majority of Salmonella strains, which functions as a main structural subunit (Haiko and Westerlund-Wikstrom, 2013). These two flagellin genes in most Salmonella strains, fliC and fljB, encode the phase 1 and phase 2 flagellins, respectively. The fliC gene is existent in all Salmonella cells and has homologs in other enteric bacteria, located in one of the flagellar biosynthesis operons (Macnab, 1992). The fljB gene is unique to S. enterica and is present in four of the S. enterica subspecies (subspecies I, II, IIIb, and VI), separately located on the chromosome from the fliC gene (McQuiston et al., 2004). However, these two flagellin genes cannot be expressed simultaneously. The second flagellin locus is considered as a genetic “spare tyre” availed in certain environmental circumstances (McQuiston et al., 2008). Salmonella isolates are indicated biphasic, monophasic, or non-motile according to the “phase variation,” expressing both, one, or no flagellar phases, which is regulated by a switch mechanism, an invertible element hin (Bonifield and Hughes, 2003). The fljB is considered as a duplication of fliC but is flanked with the genes, fljA and hin (Szekely and Simon, 1983), involving in the switch mechanism, which appears to be specific to Salmonella (Zieg et al., 1978). Recombination of these genes is the basic source of flagella antigenic variation (Smith et al., 1990) and may occur between fliC of co-infecting organisms or fliC and fljB of the same isolate (Okazaki et al., 1993). The function of flagellar genes have been studied well; the fliA existing upstream of the coding region is specific to promoters for flagellar operons (Ohnishi et al., 1990), fliB is responsible for flagellar methylation (Parkhill et al., 2001), fliC encodes the flagellar filament protein (Ogushi et al., 2001), and fliD encodes the filament cap protein of the flagellar apparatus (Yokoseki et al., 1995). The expression of fliS facilitates the export of flagellin through the flagellum-specific export pathway (Yokoseki et al., 1995), whereas the fljB gene, which expresses phase II flagellin protein, constitutes an operon with the fljA gene, which encodes a negative regulator for fliC expression (Yamamoto and Kutsukake, 2006).
The flagellin genes are useful as a model system for studying genetic differentiation (Okazaki et al., 1993). Previous study has revealed that the 5′ and 3′ ends of the fliC and fljB gene sequences from different antigenic types are highly conserved, but the central region sequences can be highly variable (Okazaki et al., 1993). A molecular serotyping for the H antigens by targeting the genes encoding the immunologically recognized antigen could be linked to traditional serotyping methods (McQuiston et al., 2011). Based on phylogenetic relationships adapted from previous studies, the acquisition of phase variation, the biphasic Salmonella flourished (McQuiston et al., 2008). However, this conclusion was drawn from analysis of limited numbers of samples and the basic evolutionary process of this apparently high level of surface-antigen diversity in the Salmonella is not well-understood (McQuiston et al., 2008). There is also a lack of data on the detailed phylogenetic description of the flagellar gene relationship among the Salmonella, as a framework for evolutionary studies. Therefore, it is worthwhile to seek full evolutionary insights and the evolutionary relationships of the Salmonella flagellin alleles to estimate the recombination within these loci. So, in this study, comparative genomics was performed to obtain additional evolutionary information regarding flagellin genes, which showed that there are two distinct evolutionary patterns of fliC and fljB clusters during the speciation and serovar diversification of Salmonella.
Materials and Methods
Data Collection
A total of 214 Salmonella complete genomes and genome assemblies of highest quality (Table S1) were retrieved from the NCBI (National Center for Biotechnology Information, https://www.ncbi.nlm.nih.gov/). All the Salmonella genomes with annotations of different serotypes were downloaded as of May, 2016, including most of complete genomes as well as draft genomes of type strains or strains of some serovars for which a complete genome was not available. Five subspecies of S. enterica (I), salamae (II), arizonae (IIIa), diarizonae (IIIb), and houtenae (IV), and a total of 87 serovars of enterica (I), were under consideration. MLST (Multilocus Sequence Typing) (Achtman et al., 2012), together with checking the original studies was implemented to identify their serovars. Housekeeping genes, thrA, purE, sucA, hisD, aroC, hemD, and dnaN were targeted for the MLST scheme (Achtman et al., 2012). The nucleotide sequences were then aligned against seven housekeeping genes. Outcome of sequences were subsequently submitted to the MLST database (http://mlst.warwick.ac.uk/mlst/dbs/Senterica) and assigned existing or novel allele type numbers. The composite sequence STs (sequence types) were defined by the database based on the set of allelic profiles derived from each of the seven loci.
Determination of Homologous Groups
OrthoMCL (version 2.0.9) (Li et al., 2003) was used to determine homologous clusters of the pan-genome for subsequent analyses. All extracted protein sequences were adjusted to a prescribed format and were grouped into homologous clusters based on sequence similarity. The BLAST reciprocal best hit algorithm (Moreno-Hagelsieb and Latimer, 2008) was employed with 70% match cutoff, and Markov Cluster Algorithms (MCL) (Enright et al., 2002) were applied with an inflation index of 1.5.
Phylogenetic Analysis
To determine the phylogenetic relationships among Salmonella subspecies and different serovars, a phylogenetic tree was inferred from single nucleotide polymorphisms (SNPs) of core genes. According to the identification of homologous clusters, a total of 127,544 genes presented in single-copy orthologous genes shared by all 214 Salmonella strains were selected for phylogenetic analysis. For each orthologous cluster, nucleotide sequences were aligned and then concatenated. Custom Perl scripts were used to parse the concatenated sequence and extract SNPs. The organismal phylogeny of SNPs and other genes were inferred using the maximum likelihood (ML) algorithm in RAxML version 8.2.9 (Stamatakis, 2014). The tree reconstruction is repeated 1,000 times, and the percentage of times each interior branch which is noted as bootstrap-value is given. Each tree of genes in the two flagellin gene clusters was constructed by MEGA (Kumar et al., 2016), using Neighbor-Joining method with 1,000 bootstraps.
Identification of fliC and fljB
To characterize the evolution pattern of flagellin genes, reference sequences of fliC, fljB, and the flanked genes, fliA, fliB, fliD, fliS, fljA, and hin, were extracted from the S. Typhimurium LT2 genome (GenBank accession number AE006468) (McClelland et al., 2001). The relevant homologous clusters in the pan-genome were determined by BLAST with 70% match cutoff and 50% coverage cutoff. In cases of shared homologous genes related to flagellin genes, phylogenetic trees were inferred by ML algorithms in RAxML version 8.2.9 (Stamatakis, 2014). Together with the phylogenetic trees, the flanked gene contents were taken in account to confirm the gene loci of fliA, fliB, fliC, fliD, fliS, fljA, fljB, and hin.
Congruence Analysis
PAUP version 4.0a152 (Swofford, 1998) was used to conduct maximum-likelihood (ML) analysis of the congruence of gene trees, as described by Feil et al. (2001). The HKY85 model of DNA substitutions was used to estimate α parameter, and the transition/transversion (Ti/Tv) ratio, assuming gamma distribution. The difference between the log likelihood scores of the trees of two genes Δ-lnL was calculated, generating from the comparison of one gene tree against another based on the 99th percentile of the distribution of log likelihood scores for 200 trees from random topology. If the Δ-lnL values for the comparisons is less than that reference gene comparisons within the 99th percentile of this distribution, then these two gene trees should be considered significantly congruent (Octavia and Lan, 2006).
Regression Analysis for Evolutionary Synchronization
A key element of our study was to determine whether the evolution of flagella gene was associated with the evolutionary rate estimate. To test for this pattern we conducted a least squares linear regression for the genome-wide rate as a function of sampling distance. The MEGA version 7 (Kumar et al., 2016) was used for pairwise distance calculating. A potential shortcoming of this analysis is that the data do not necessarily represent independent samples because some correspond to closely related lineages. This can be addressed by using phylogenetic independent contrasts, or phylogenetic generalized least squares. For the regression of the rate as a function of sampling evolutionary consensus the degree of association was estimated when fitting a regression for a ratio as a function of its denominator.
Recombination Analysis
Potential recombination within fliB, fliC, and fliD genes was screened using seven methods (RDP, GENECONV, MaxChi, Bootscan, Chimera, SiScan, and 3Seq) implemented in the Recombination Detection Program version 4.88 (RDP4) (Martin et al., 2015). The breakpoints were also defined by RDP4. Similarity between the recombinants and their possible major and minor parents was estimated using BootScan, embedded in RDP4 (Stamatakis, 2014). The recombination event evaluated by RDP4 was considered significant if it satisfied at least two criteria when the P-value (p) < 0.05 and the RDP recombination consensus score (RDPRCS) was >0.4. When p < 0.05 and the RDPRCS was between 0.4 and 0.6, the recombination event was possible. An RDPRCS < 0.4 with p < 0.05 indicated the rejection of the recombination event (Martin et al., 2015).
Results
Core Genome Tree Revealed Detailed Phylogenetic Relationships among Different Serovars
A total of 214 Salmonella genomes were analyzed in this study, consisting of 205 S. enterica subsp. enterica genomes and 9 genomes of other four S. enterica subspecies, which covered 87 serovars. More than one genome (maximum twelve) was included for some famous serovars (e.g. S. Enteritidis and S. Typhi), while only one genome was considered for most other serovars because no more genomes were available. More detailed information on these genomes was summarized in Table S1. After homologous gene clustering, the total 947,793 proteins were grouped into 14,583 homologous clusters, including common genes represented by 596 single-copy groups of 712 core homologous clusters. A total of 57,946 SNPs in the “core” regions were identified that were present and aligned with high confidence in all taxa. These SNPs were used to construct the phylogeny (Figure 1).
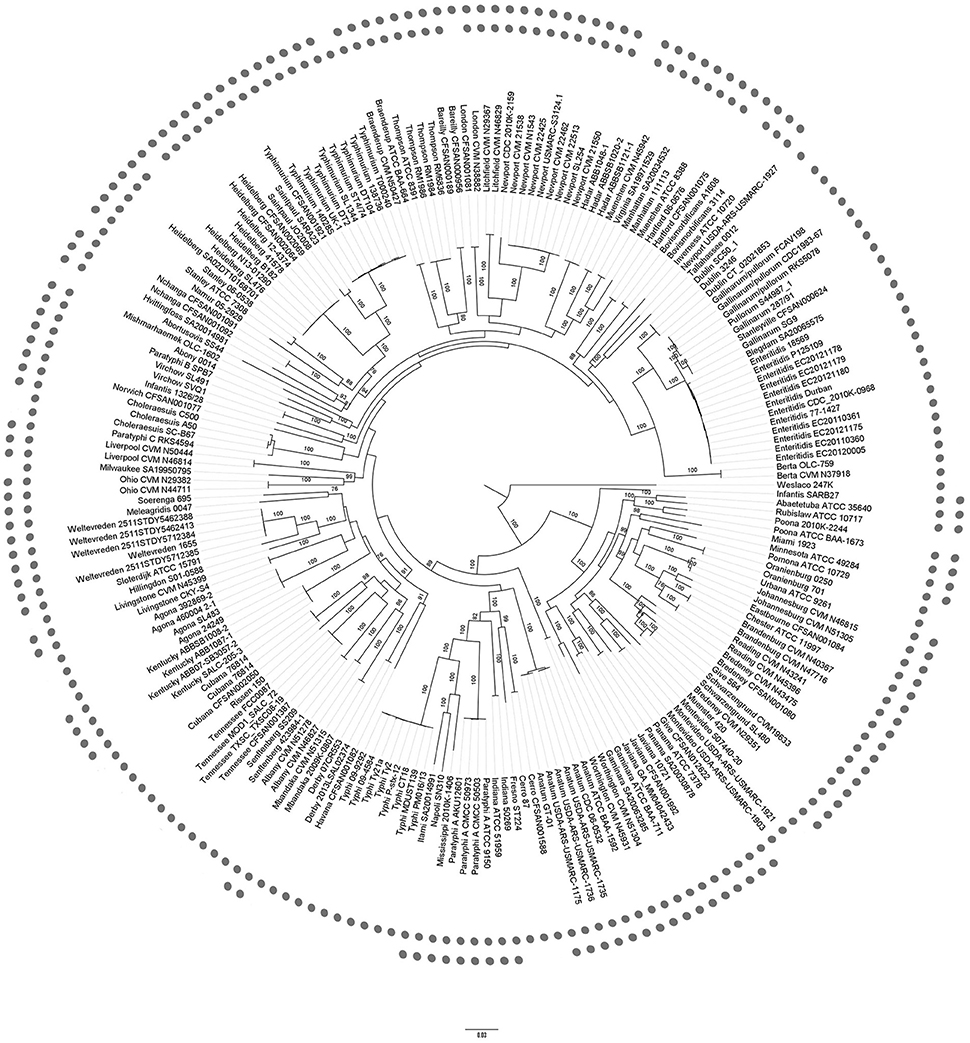
Figure 1. Phylogenetic tree of Salmonella enterica. Maximum Likelihood phylogenetic tree based on 57,946 SNPs of 596 single copy core genes of 214 genomes. Bootstrap values (>70%) are presented on the branches. The inner dots indicated the presence of fliC gene clusters, while the outer dots indicated fljB gene clusters. The bootstrap values near the tips of the trees were omitted. The other four subspecies of S. salamae (II), arizonae (IIIa), diarizonae (IIIb), and houtenae (IV) were far distant from subspecies enterica (I) and omitted.
The role of the phylogenetic tree based on SNPs in genome variation and evolution has been documented previously (Filliol et al., 2006; Holt et al., 2008). The core genome tree can support the major genetic group phylogeny and reveal much more phylogenetic detail, including the divergence of major phylogenetic groups. Moreover, the core genome tree also revealed a number of strong concordances with serotyping. From Figure 1, core genome tree was able to categorize S. Typhimurium isolates but unable to ultimately separate the S. Stanleyville from the S. Gallinarum with high resolution. In most cases, the genomes within a cluster belonged to the same serovar, as for S. Typhi or Enteritidis. Although some isolates were mixed up with each other, for example, it was hard to differentiate S. Give, S. Schwarzengrund, and S. Bredney, the core genome tree generally separated the serovars from each other.
The Monophasic and Biphasic Strains Were Less Grouped into the Same Cluster on Core Genome Tree
The prevalence of phase I and phase II related flagelllin gene locus of the 214 genomes was also indicated in Figure 1. In total, fliC gene cluster was found in 207 genomes (96.7%) of 91 serovars (97.8%), including subspecies, while fljB gene cluster was found in 138 genomes (64.5%) of 61 serovars (65.6%). The detailed list (Table S2) was dominated by fliC and fljB genes and included their flanked genes and hin. Some well-studied serovars (Enteritidis, Dublin, Typhi etc.) are monophasic the same as the previous study (Selander et al., 1992; Baker et al., 2007). Nevertheless, the fliC gene was not found present in the genomes of strain S. Poona 2010K-2244, S. Heidelberg B182, S. Abortusovis SS44, S. enterica subsp. salamae 3588/07, S. Bovismorbificans A1608, S. Hadar ABBSB1020-2, and S. London CFSAN001081, of which only a draft genome was obtained for these strains. Duplicated annotations and misprediction of some genes that span contig boundaries may occur in draft genomes. The homologs (Table S2) were able to mitigate but not eliminate these limitations. We further checked the contigs and related annotation of seven genomes of fliC gene cluster absent, and found some residue of the gene cluster at some contigs' ends in each genome. On the other hand, there was no report on the absence of fliC in these serovars, for example, fliC should be present in all S. Heidelberg (Macnab, 1992). Therefore, the absence of fliC gene in these genomes should be a result of misassembly or the related fragment not fully sequenced.
Interestingly, the monophase and biphase being linked to the evolution of S. enterica subsp. enterica showed discrete phylogenetic clusters on the core genome tree (Figure 1). A few serovars with identical phase, clustered together in the core genome tree. In one larger monophasic clade, with 25 genomes, containing S. Dublin, Gallinarum, Pullorum, Stanleyville, Blegdam, Enteritidis, and Berta, all the genomes of each serovar were located together in one distinct cluster in the core genome tree. For the other monophasic serovars, the 45 genomes were identified at different locations, of which another larger clade of 12 genomes, containing S. enterica subsp. enterica serovars Cubana, Rissen, Tennessee, Senftenberg, and Albany were located adjacent to Derby, Havana, and Agona, but were disrupted by Mbandaka and Kentucky of biphase.
Evolutionary Congruence and Phylogenetic Analysis Implied Two Distinct Evolution Patterns of the Flagellin Gene Clusters
Evolutionary analysis of flagellin gene fliC and fljB in Salmonella has been performed previously (Mortimer et al., 2007). However, the co-evolution of these two genes and their flanking genes has never been studied. To evaluate evolutionary congruence between and within the fliC and fljB clusters, ML analysis was carried out, and the results were summarized in Table 1. As is shown, none of the genes in fliC gene cluster were congruent to all the genes in fljB gene cluster, and none of the genes in fljB gene cluster were congruent to all the genes in fliC gene cluster. The gene trees with the largest number of congruencies were those of fliC and hin, which were congruent to four other gene trees. More concretely, fliC gene tree was similar to those of fliB, fliD, fljA, and fljB gene, and hin gene tree was similar to those of fliB, fliD, fljA, and fljB gene. As for fliB (equaling to fljA and fljB gene trees) and fljB (equaling to fliB and fljA trees), they were congruent to two gene trees. And fliA and fliD trees were congruent to fljA and fliB respectively. Overall, only 29.2% (14/48) of the flagellar gene tree comparisons were congruent among these S. enterica strains. As for the fliC and its neighbored genes, fliB and fliD, they were closely related, while for fljA and fljB genes, their relationship was more distant. Moreover, there was generally higher level of congruence between fliC and fljB/fliD genes than between fliA and fliB genes and between fliD and fliS genes. However, correlation calculations were not available between fliC and fliS genes, because approximation limit dynamically readjusted too low.
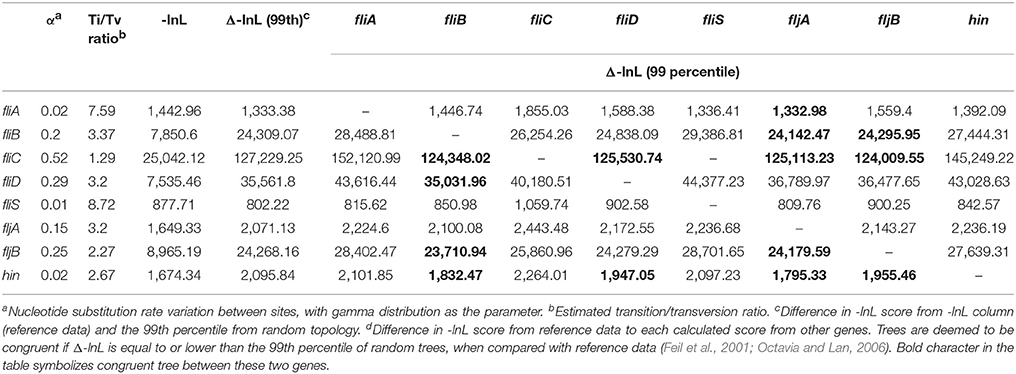
Table 1. ML analysis for congruence between each gene tree of the flagella genes analyzed in this study.
Besides the relationship among the genes in the fliC and fljB gene clusters, we further studied their congruence to the core genome using linear regression analysis instead of ML analysis, because datasets of the core genome were too large to calculate -lnL score. The mutual information analysis indicated a weak correlation between each flagellin gene and core genome. The low values of both trees for each linear regression indicate a high level of incongruence between individual tree of each gene and the core genome tree, as shown in Figures 2, 3. The topology of fljB, fliC, and fliD gene trees was much distinct from that of the core genome tree, while those of the fliA and fliS genes were more similar to the core genome tree than those of fljB, fliC, and fliD genes. However, the fljA tree for serovars displayed a similar topology to that of the core genome tree, while fljB did not.
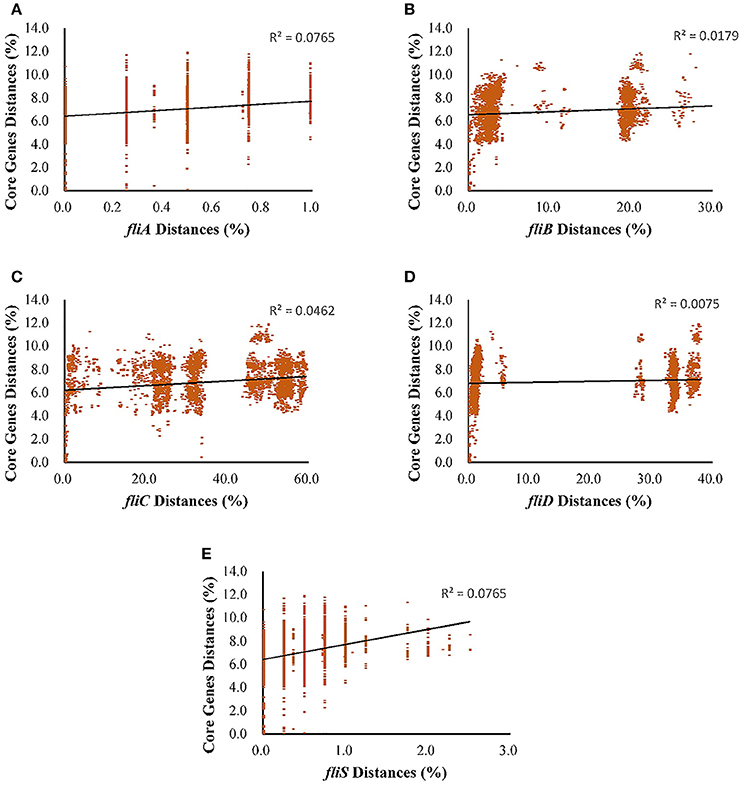
Figure 2. Regressions between each gene of the fliC gene clusters and core genetic distance (expected nucleotide substitutions per site) as a function of sampling the evolutionary distance for S. enterica (I). (A), fliA; (B), fliB; (C), fliC; (D), fliD; and (E), fliS. Each dot corresponds to a pair of sampled genomes, and the linear regression is shown as a solid line using least squares; the R2 coefficients are also shown.
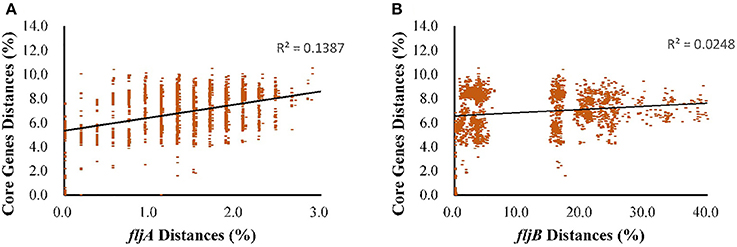
Figure 3. Regressions between each gene of the fljB gene clusters and core genetic distance (expected nucleotide substitutions per site) as a function of sampling the evolutionary distance for S. enterica (I). (A), fljA; and (B), fljB. Each dot corresponds to a pair of sampled genomes, and the linear regression is shown as a solid line using least squares; the R2 coefficients are also shown.
To infer evolutionary relationships among different serovars, based upon similarities and differences in their genetic characteristics, we constructed phylogenetic trees of fliA, fliB, fliC, fliD, fliS, and fljA, fljB, hin, and fliC/fljB (Figure 4). Diagrammatic representation of fliB, fliC, and fliD indicated bifurcation to form two distinct lineages, where other Salmonella enteric subspecies joined together with subspecies enteric serovars. The other four subspecies could be easily recognized from fliA and fliS gene tree because of their distant branches, which was more consistent with core genome tree. Subspecies salamae was close to three neighbors, serovar Weltervreden, Sloterdijk and Kentucky, in fljB tree, while these serovars were scattered to different branches in fljA tree. The results of phylogenetic trees were consistent to the congruence analysis (Table 1, Figure 2, 3). Dramatically, the individual gene trees of fliB, fliC (fliC of S. Typhi was an exception) and fliD were consistent with a clustering dividing monophasic and biphasic serovars. This seemed to imply two distinct evolutionary paths of fliC/fljB corresponding to monophasic and biphasic serovars.
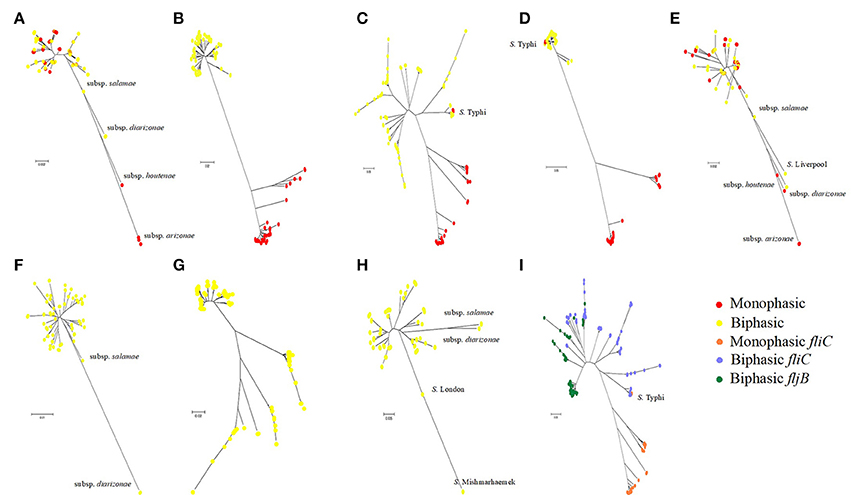
Figure 4. Phylogenetic trees of Salmonella flagella related genes. Neighbor-joining phylogenetic trees of flagella related genes are based on the nucleotide sequences: fliA (A), fliB (B), fliC (C), fliD (D), fliS (E), fljA (F), fljB (G), hin (H) and fliC/fljB (I). Different color dot implies the monophasic or biphasic serovars.
Phylogeny of fliC and fljB Showed Complex Relationships
Because different genes of the flagellin diverge under different evolutionary pressures, we manually curated the phylogenetic pattern of genes in flagellin gene clusters. For all flagellin and their flanked genes, the individual gene trees (Figure 4) can be used to demonstrate the evolutionary story on individual gene basis. To study the genetic evolution of the 207 fliC and 138 fljB allelic variants, phylogenetic tree based on nucleotide sequences was constructed (Figure 4I). There is a clear separation of monophasic Salmonella groups from the biphasic groups based on the fliC gene, while all the fljB alleles clustered with the biphasic fliC alleles. This may either reflect that monophasic were acting as incipient serotypes with a barrier to recombination with biphasic serotypes, or indicate that there were insufficient incidents in which recombination could have taken place between monophasic and biphasic serotypes. However, S. Typhi was not clustered into other monophasic genomes as one branch together. Similarly, previous study had also identified that monophasic S. Typhimurium existed, of which the serotype was always biphasic (Laorden et al., 2010).
Furthermore, all biphasic fliC and fljB alleles were analyzed based on the amino acid sequences (Figure S1). The genetic distance between fliC gene and fljB gene sequences showed no relationship within/among serovars, which was reflected in the phylogenetic tree. For example, serovar Schwarzengrund, Liverpool, and Livingstone were closely related based on fliC gene while they were distant based on fljB. S. Heidelberg and S. Newport were clustered together according to the fljB gene, while they were clustered separately according to the fliC gene. It suggested that the evolutionary histories were independent between fliC and fljB genes and frequent homologous recombination referring fliC and fljB occurred between biphasic serovars. The phylogenetic tree of 5′ and 3′ conserved segment of fliC and fljB genes was also constructed (data not shown), showing the similar division.
Less Recombination Events Were Found between Monophasic and Biphasic Serovars
Recombination is a significant source of genetic diversity in Salmonella (Silverman et al., 1979). This phenomenon, which occurs during co-infection with different strains, is usually observed at the fliC and its flanked genes. It is apparent that there were different levels of recombination within flagellar genes of S. enterica. Comparison of recombination of monophasic genes with biphasic genes, which represents the unique ability to change flagellar composition, by both ML (Table 1) and recombination detective analysis (Figure 5), showed that recombination occured far more frequently within monophasic or biphasic gene clusters than between them. For example, the serovar Heidelberg, whose major parent (a sequence closely related to that from which the greater part of the recombinant's sequence may have been derived, Martin et al., 2015) was Virchow, and minor parent (a sequence closely related to that from which sequences in the proposed recombinant region may have been derived, Martin et al., 2015) was Newport, did not recombine to any monophasic genes. It was identical to Hartford, whose major parent was Pomona, and minor parent was Anatum. Even though it was found that Senftenberg (monophase) was minor parent for Newport (biphase), and other similar recombinant events of referring small segments were also found, recombination was believed mainly to have occurred within the same phase. Moreover, there were lots of breakpoints and the breakpoints tended to cluster far more around the edges of genes than they did within the central parts of genes (Figure 5).
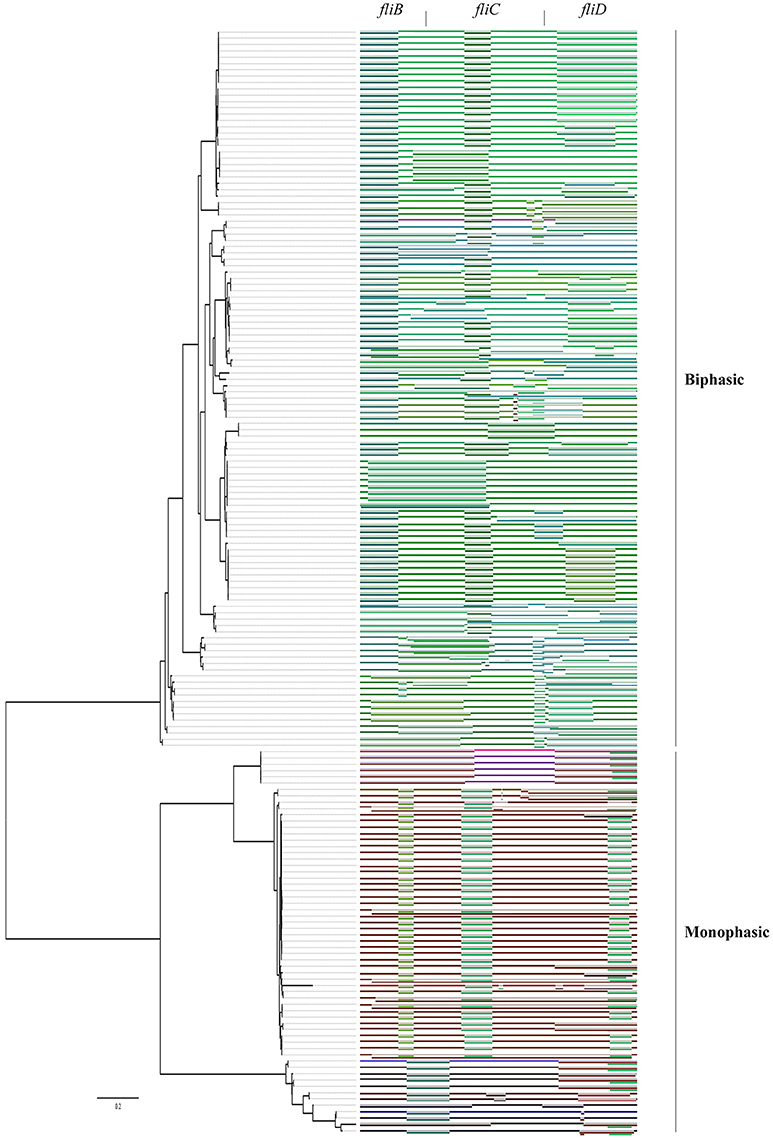
Figure 5. Recombination events in Salmonella fliBCD gene cluster. The recombination properties of flagella genes are indicated as different colors on the right, indicating positions of potential recombination events. Piece of sequence from major parent refer to the top of rectangles. Piece of sequence from minor parent is a graphical representation of a sequence fragment that has potentially been derived through recombination from a sequence resembling the next two bars of the rectangle. On the left, a phylogenetic tree of the phase I alleles based on concatenated fliBCD nucleotide sequences was constructed by using the maximum-likelihood method with a bootstrap value of 1,000.
Discussion
With the cost of whole genome sequencing (WGS) decreasing dramatically (Didelot et al., 2012) and the technology becoming available for routine use, WGS is very useful and practical in various bacterial infectious studies including diagnostic and public health microbiology (Koser et al., 2012). Many Salmonella serovars are human-specific, which may cause gastroenteritis, a self-limiting illness. A few Salmonella serovars, especially S. Typhi, may elicit typhoid-like diseases and serious systemic infections (Parkhill et al., 2001; Crump and Mintz, 2010). Recent studies support the phylogenetic tree based on SNPs as an outbreak surveillance tool (Allard et al., 2012; Hoffmann et al., 2013; Zhou et al., 2013). The performance of these 214 genomes based on SNPs was validated on the phylogenetic analysis. The fractions of the core genome tree (Figure 1) supported the partition of the taxa in previous study and reflected robust evolutionary relationship of Salmonella serovars (Chan et al., 2003; Jacobsen et al., 2011; Zou et al., 2014). Moreover, the rich information of Salmonella subspecies enterica, covering 87 serovars, provided a framework for determination of the evolutionary relationships of the flagellin alleles to assess the co-evolution and recombination events.
Flagellin genes are considered as a dominant antigen of the adaptive immune response (Haiko and Westerlund-Wikstrom, 2013). The fliC gene is present in most of Salmonella isolates, and has a homologous locus in Escherichia coli (Macnab, 1992). Selection within specific niches might have an impact in antigenic variation (McQuiston et al., 2008). For adapting to environmental changes, Salmonella adjust their expression, introducing a second set of antigens, to survive in the new niches. The complex branching division of diphasic serotypes, compared to monophasic serotypes, is suggestive of an expanded mutation rate or recombination in the flagellin when Salmonella acquired the second flagellin gene. Perhaps monophasic serotypes obtain enough antigenic diversity from SNPs, letting these serotypes to be successful as monophasic strains (Mortimer et al., 2007). However, the reason why S. Typhi is a monophasic serotype and cluster with biphasic serotypes in the phylogenetic trees of fliBCD is still unclear. We hypothesize that S. Typhi had a trend to be diphasic, and the substitute of fljB was present in the history but got lost during speciation.
As an important virulence factor in pathogenic bacteria, especially in Salmonella genus, flagellin might regulate their virulence and pathogenesis (Stecher et al., 2004). For example, it is beneficial to immunologic escape by turning on/off the expression of genes related to these structures, niche adaptation by expressing the second flagellin and avoiding predation by different motility or antigenic expression. All of these selective advantages are importantly involved in evolutionary step of having the second flagellin. The gain or loss of their unique ability to encode the major flagellin protein by switching expression can be discussed by an evolutionary model. Based on the phylogenetic tree of the fliC and fljB genes in the broad serovars involved, it is supposed that the phylogeny of flagellin genes illustrated a strong serovar-specific lineage, with strains of the same serovar clustering either together or in a few distinct branches (Figure 1), as described before (Yue et al., 2015). Clustering of the fliC and fljB genes showed that fljB arose from fliC gene duplication (Mortimer et al., 2007), which have highly conserved 5′ and 3′ ends and highly variable center regions (Masten and Joys, 1993; McQuiston et al., 2004). Most studies of flagellin genes focus either on its evolutionary relationships or as a dominant antigen of the sequence diversity (Mortimer et al., 2007) but do not undertake subsequent congruence analysis of their evolutionary process. The systematic approach, ML analysis and regression analysis (Table 1, Figures 2, 3), determined that fliB, fliC, and fliD gene trees were evolutionarily congruent to each other but not to the core genome. In the regression analysis of fliA and fliS against the core genome, low levels of correlation were found (low R2 values). The fliA and fliS genes were highly conserved within subspecies enterica with limited variation (Figure 2), which will disturb the regression analysis, while their phylogenetic trees indicated a clear classification as that of core genome, especially other subspecies (Figure 4). These results suggested that fliA and fliS evolved congruently with the core genome. Notably, homologous recombination of fliC and flanked genes occurred frequently within monophasic and diphasic serovars (Figure 5). These results suggested that the level of clonality of flagellar genes within subspecies I was low.
Dramatically, the phylogenetic trees of fliB, fliC, and fliD revealed a similar division of the serovars into two distinct groups, corresponding to monophasic and biphasic serovars, respectively. This implied two distinct evolutionary patterns of Salmonella fliC/fljB gene cluster. This division was not pointed out previously, but similar phylogenetic structures of fliC/fljB were reported (Smith et al., 1990; Mortimer et al., 2007). It was divided into Non-G antigens and G antigens based on fliCg, m, s encoding three factors. Through the phylogenetic tree in Mortimer (Mortimer et al., 2007) study, Non-G group and G group were clustered into different branches, which equaled to biphasic and monophasic groups respectively. According to these results, a flow diagram was constructed to demonstrate the evolutionary path of the monophasic and biphasic gene clusters (Figure 6). The Salmonella ancestor should just harbor a fliC gene cluster as most of E. coli isolates (McQuiston et al., 2008). Subsequently, some lineages suffered fliC duplication to assemble an fljB gene cluster under certain natural selection conditions (biphasic), while the others not (monophasic). Both monophasic and biphasic lineages evolved and adapted to different environments and occupied diverse ecological niches, resulting in H antigen (serovar) diversification. Ancestors of some biphasic serovars might have lost fljB gene cluster under natural selection because it was useless, becoming monophasic again (e g., S. Typhi and some S. Typhimurium isolates). Frequent homologous recombination occurred within monophasic and biphasic serovars. Finally, the current diversity of Salmonella was formed. This theory assumed that fljB gene cluster should perform a role together with fliC cluster in most biphasic serovars, but present knowledge suggested that just one of them was expressed in a cell (Imre et al., 2005). Therefore, the role of fljB needs to be further determined to clarify the implication of the two distinct evolutionary patterns.
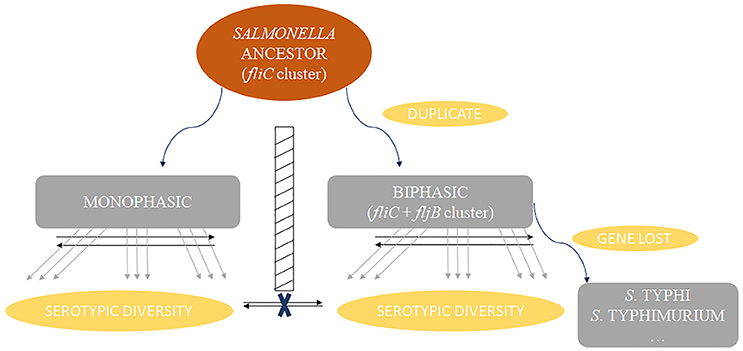
Figure 6. Schema diagram showing the two distinct evolution patterns of Salmonella fagellin gene clusters. Homologous recombination (represented by black arrows) rarely occurred between monophasic and biphasic serovars, but frequently occurred within the same phase. Some serovars and some isolates of certain serovars should have been biphasic historically but become monophasic because of gene loss of fljB gene cluster, such as serovar Typhi and Typhimurium.
Collectively, we provide the viewpoint that flagellin genes of S. enterica subsp. enterica represents two evolutionary patterns with a barrier between monophasic and biphasic serovars. The evolutionary barrier may inhibit an overlapping recombination. However, it is still unclear what the implication of the barriers on the immunological escape, niche adaptation, and avoiding predation is. That is an interesting area for further study. Besides, different serovar genome repertoires were included, suggesting that the core genome evolution of S. enterica was accompanied by fliA/fliS but distinct from those of fliBCD and fljB gene cluster. This study will provide a robust genetic framework for future studies on the evolution of the phase I and phase II flagellar characteristics in S. enterica.
Author Contributions
YL and DFZ designed and initiated the study, interpreted the results, and wrote the manuscript; YL downloaded and analyzed the data; LZ, XZ, LX, and XS contributed to discussion of the results and improvement of the manuscript and designed the outline of this study and manuscript and provided laboratory equipment and space.
Conflict of Interest Statement
The authors declare that the research was conducted in the absence of any commercial or financial relationships that could be construed as a potential conflict of interest.
Acknowledgments
This work was supported by the National Natural Science Foundation of China [No. 31230058] and the National Key R&D program of China [No. 2016YFE0106100]. The authors would like to thank Dr. Harold Corke, Dr. Hongyu Ou, Dr. Xiangyu Deng, and Dr. Arun Bhunia for their useful suggestions on this manuscript. In addition, we want to thank Mr. Ben Jia and Mr. Lincheng Shi who kindly provided help in custom script coding for this work.
Supplementary Material
The Supplementary Material for this article can be found online at: https://www.frontiersin.org/articles/10.3389/fmicb.2017.02604/full#supplementary-material
Table S1. Complete list of all Salmonella genome sequences. The 214 genomes included 205 Salmonella enterica subsp. enterica and nine genomes of four other subspecies, arizonae, diarizonae, houtenae, and salamae.
Table S2. Presence/absence of flagellin genes of all analyzed Salmonella. The fliA, fliB, fliC, fliD, and fliS for fliC clusters, and fljA, fljB, and hin for fljB clusters were analyzed. a –, absence of the gene; gene name, the locus tag in the genome.
Figure S1. The genetic relationships of biphasic fliC and fljB genes. Maximum Likelihood phylogenetic tree shows the genetic relationships of biphasic fliC and fljB genes. It shows the details of the biphasic lineage in Figure 4I. The fliC and fljB genes were shown in green and blue characters, respectively. Each square represented a group of alleles with identical amino acid (83 sequence types in total). The fliC and fljB genes from the same genomes were linked by solid lines.
References
Achtman, M., Wain, J., Weill, F. X., Nair, S., Zhou, Z., Sangal, V., et al. (2012). Multilocus sequence typing as a replacement for serotyping in Salmonella enterica. PLoS Pathog. 8:e1002776. doi: 10.1371/journal.ppat.1002776
Allard, M. W., Luo, Y., Strain, E., Li, C., Keys, C. E., Son, I., et al. (2012). High resolution clustering of Salmonella enterica serovar Montevideo strains using a next-generation sequencing approach. BMC Genomics 13:32. doi: 10.1186/1471-2164-13-32
Baker, S., Hardy, J., Sanderson, K. E., Quail, M., Goodhead, I., Kingsley, R. A., et al. (2007). A novel linear plasmid mediates flagellar variation in Salmonella Typhi. PLoS Pathog. 3:e59. doi: 10.1371/journal.ppat.0030059
Bonifield, H. R., and Hughes, K. T. (2003). Flagellar phase variation in Salmonella enterica is mediated by a posttranscriptional control mechanism. J. Bacteriol. 185, 3567–3574. doi: 10.1128/JB.185.12.3567-3574.2003
Brenner, F. W., Villar, R. G., Angulo, F. J., Tauxe, R., and Swaminathan, B. (2000). Salmonella nomenclature. J. Clin. Microbiol. 38, 2465–2467.
Centers for Disease Control and Prevention (CDC) (2011). National Salmonella Surveillance Annual Summary 2009. Atlanta, GA: US Department of Health and Human Services, CDC.
Chan, K., Baker, S., Kim, C. C., Detweiler, C. S., Dougan, G., and Falkow, S. (2003). Genomic comparison of Salmonella enterica serovars and Salmonella bongori by use of an S. enterica serovar typhimurium DNA microarray. J. Bacteriol. 185, 553–563. doi: 10.1128/JB.185.2.553-563.2003
Crump, J. A., and Mintz, E. D. (2010). Global trends in typhoid and paratyphoid Fever. Clin. Infect. Dis. 50, 241–246. doi: 10.1086/649541
Didelot, X., Bowden, R., Wilson, D. J., Peto, T. E., and Crook, D. W. (2012). Transforming clinical microbiology with bacterial genome sequencing. Nat. Rev. Genet. 13, 601–612. doi: 10.1038/nrg3226
Enright, A. J., Van Dongen, S., and Ouzounis, C. A. (2002). An efficient algorithm for large-scale detection of protein families. Nucleic Acids Res. 30, 1575–1584. doi: 10.1093/nar/30.7.1575
Feil, E. J., Holmes, E. C., Bessen, D. E., Chan, M. S., Day, N. P. J., Enright, M. C., et al. (2001). Recombination within natural populations of pathogenic bacteria: short-term empirical estimates and long-term phylogenetic consequences. Proc. Natl. Acad. Sci. U.S.A. 98, 182–187. doi: 10.1073/pnas.98.1.182
Filliol, I., Motiwala, A. S., Cavatore, M., Qi, W., Hazbón, M. H., Bobadilla del Valle, M., et al. (2006). Global phylogeny of Mycobacterium tuberculosis based on single nucleotide polymorphism (SNP) analysis: insights into tuberculosis evolution, phylogenetic accuracy of other DNA fingerprinting systems, and recommendations for a minimal standard SNP set. J. Bacteriol. 188, 759–772. doi: 10.1128/JB.188.2.759-772.2006
Grimont, P. A. D., and Weill, F. X. (2007). Antigenic Formulae of the Salmonella serovars, 9th Edn. Paris: WHO Collaborating Centre for Reference and Research on Salmonella.
Haiko, J., and Westerlund-Wikström, B. (2013). The role of the bacterial flagellum in adhesion and virulence. Biology 2, 1242–1267. doi: 10.3390/biology2041242
Hoffmann, M., Luo, Y., Lafon, P. C., Timme, R., Allard, M. W., McDermott, P. F., et al. (2013). Genome sequences of Salmonella enterica serovar Heidelberg isolates isolated in the United States from a multistate outbreak of human Salmonella infections. Genome Announc. 1:e00004–12. doi: 10.1128/genomeA.00004-12
Holt, K. E., Parkhill, J., Mazzoni, C. J., Roumagnac, P., Weill, F. X., Goodhead, I., et al. (2008). High-throughput sequencing provides insights into genome variation and evolution in Salmonella Typhi. Nat. Genet. 40, 987–993. doi: 10.1038/ng.195
Imre, A., Olasz, F., and Nagy, B. (2005). Development of a PCR system for the characterisation of Salmonella flagellin genes. Acta Vet. Hung. 53, 163–172. doi: 10.1556/AVet.53.2005.2.2
Jacobsen, A., Hendriksen, R. S., Aaresturp, F. M., Ussery, D. W., and Friis, C. (2011). The Salmonella enterica pan-genome. Microb. Ecol. 62, 487–504. doi: 10.1007/s00248-011-9880-1
Köser, C. U., Ellington, M. J., Cartwright, E. J., Gillespie, S. H., Brown, N. M., Farrington, M., et al. (2012). Routine use of microbial whole genome sequencing in diagnostic and public health microbiology. PLoS Pathog. 8:e1002824. doi: 10.1371/journal.ppat.1002824
Kumar, S., Stecher, G., and Tamura, K. (2016). MEGA7: molecular evolutionary genetics analysis version 7.0 for bigger datasets. Mol. Biol. Evol. 33, 1870–1874. doi: 10.1093/molbev/msw054
Laorden, L., Herrera-Leon, S., Martinez, I., Sanchez, A., Kromidas, L., Bikandi, J., et al. (2010). Genetic evolution of the Spanish multidrug-resistant Salmonella enterica 4,5,12:i:- monophasic variant. J. Clin. Microbiol. 48, 4563–4566. doi: 10.1128/JCM.00337-10
Li, L., Stoeckert, C. J., and Roos, D. S. (2003). OrthoMCL: identification of ortholog groups for Eukaryotic genomes. Genome Res. 13:2178. doi: 10.1101/gr.1224503
Macnab, R. M. (1992). Genetics and biogenesis of bacterial flagella. Annu. Rev. Genet. 26, 131–158. doi: 10.1146/annurev.ge.26.120192.001023
Majowicz, S. E., Musto, J., Scallan, E., Angulo, F. J., Kirk, M., O'Brien, S. J., et al. (2010). The global burden of nontyphoidal Salmonella gastroenteritis. Clin. Infect. Dis. 50, 882–889. doi: 10.1086/650733
Martin, D. P., Murrell, B., Golden, M., Khoosal, A., and Muhire, B. (2015). RDP4: Detection and analysis of recombination patterns in virus genomes. Virus Evol. 1:vev003. doi: 10.1093/ve/vev003
Masten, B. J., and Joys, T. M. (1993). Molecular analyses of the Salmonella g… flagellar antigen complex. J. Bacteriol. 175, 5359–5365. doi: 10.1128/jb.175.17.5359-5365.1993
McClelland, M., Sanderson, K. E., Spieth, J., Clifton, S. W., Latreille, P., Courtney, L., et al. (2001). Complete genome sequence of Salmonella enterica serovar Typhimurium LT2. Nature 413, 852–856. doi: 10.1038/35101614
McQuiston, J. R., Fields, P. I., Tauxe, R. V., and Logsdon, J. M. Jr. (2008). Do Salmonella carry spare tyres? Trends Microbiol. 16, 142–148. doi: 10.1016/j.tim.2008.01.009
McQuiston, J. R., Parrenas, R., Ortiz-Rivera, M., Gheesling, L., Brenner, F., and Fields, P. I. (2004). Sequencing and comparative analysis of flagellin genes fliC, fljB, and flpA from Salmonella. J. Clin. Microbiol. 42, 1923–1932. doi: 10.1128/JCM.42.5.1923-1932.2004
McQuiston, J. R., Waters, R. J., Dinsmore, B. A., Mikoleit, M. L., and Fields, P. I. (2011). Molecular determination of H antigens of Salmonella by use of a microsphere-based liquid array. J. Clin. Microbiol. 49, 565–573. doi: 10.1128/JCM.01323-10
Moreno-Hagelsieb, G., and Latimer, K. (2008). Choosing BLAST options for better detection of orthologs as reciprocal best hits. Bioinformatics 24, 319–324. doi: 10.1093/bioinformatics/btm585
Mortimer, C. K., Gharbia, S. E., Logan, J. M., Peters, T. M., and Arnold, C. (2007). Flagellin gene sequence evolution in Salmonella. Infect. Genet. Evol. 7, 411–415. doi: 10.1016/j.meegid.2006.12.001
Nataro, J. P., Bopp, C. A., Fields, P. I., Kaper, J. B., Strockbine, N. A., Murray, P. R., et al. (2011). “Escherichia, Shigella, and Salmonella,” in Manual of Clinical Microbiology, 10th Edn, eds P. R. Murray, E. J. Baro, J. H. Jorgensen, M. A. Pfaller, and R. H. Yolken (Washington, DC: ASM Press), 603–626.
Okazaki, N., Matsuo, S., Saito, A., Tominaga, M., and Enomoto, M. (1993). Conversion of the Salmonella phase 1 flagellin gene fliC to the phase 2 gene fljB on the Escherichia coli K-12 chromosome. J. Bacteriol. 175, 758–766. doi: 10.1128/jb.175.3.758-766.1993
Octavia, S., and Lan, R. T. (2006). Frequent recombination and low level of clonality within Salmonella enterica subspecies I. Microbiology Sgm 152, 1099–1108. doi: 10.1099/mic.0.28486-0
Ogushi, K., Wada, A., Niidome, T., Mori, N., Oishi, K., Nagatake, T., et al. (2001). Salmonella enteritidis FliC (flagella filament protein) induces human beta-defensin-2 mRNA production by Caco-2 cells. J. Biol. Chem. 276, 30521–30526. doi: 10.1074/jbc.M011618200
Ohnishi, K., Kutsukake, K., Suzuki, H., and Iino, T. (1990). Gene fliA encodes an alternative sigma factor specific for flagellar operons in Salmonella typhimurium. Mol. Gen. Genet. 221, 139–147. doi: 10.1007/BF00261713
Parkhill, J., Dougan, G., James, K. D., Thomson, N. R., Pickard, D., Wain, J., et al. (2001). Complete genome sequence of a multiple drug resistant Salmonella enterica serovar Typhi CT18. Nature 413, 848–852. doi: 10.1038/35101607
Schrader, K. N., Fernandez-Castro, A., Cheung, W. K., Crandall, C. M., and Abbott, S. L. (2008). Evaluation of commercial antisera for Salmonella serotyping. J. Clin. Microbiol. 46, 685–688. doi: 10.1128/JCM.01808-07
Selander, R. K., Smith, N. H., Li, J., Beltran, P., Ferris, K. E., Kopecko, D. J., et al. (1992). Molecular evolutionary genetics of the cattle-adapted serovar Salmonella dublin. J. Bacteriol. 174, 3587–3592. doi: 10.1128/jb.174.11.3587-3592.1992
Silverman, M., Zieg, J., Hilmen, M., and Simon, M. (1979). Phase variation in Salmonella: genetic analysis of a recombinational switch. Proc. Natl. Acad. Sci. U.S.A. 76, 391–395. doi: 10.1073/pnas.76.1.391
Smith, N. H., Beltran, P., and Selander, R. K. (1990). Recombination of Salmonella phase 1 flagellin genes generates new serovars. J. Bacteriol. 172, 2209–2216. doi: 10.1128/jb.172.5.2209-2216.1990
Stamatakis, A. (2014). RAxML version 8: a tool for phylogenetic analysis and post-analysis of large phylogenies. Bioinformatics 30, 1312–1313. doi: 10.1093/bioinformatics/btu033
Stecher, B., Hapfelmeier, S., Müller, C., Kremer, M., Stallmach, T., and Hardt, W. D. (2004). Flagella and chemotaxis are required for efficient induction of Salmonella enterica serovar Typhimurium colitis in streptomycin-pretreated mice. Infect. Immun. 72, 4138–4150. doi: 10.1128/IAI.72.7.4138-4150.2004
Swofford, D. L. (1998). PAUP*. Phylogenetic Analysis Using Parsimony (*and Other Methods). Sunderland, MA: Sinauer Associates.
Szekely, E., and Simon, M. (1983). DNA sequence adjacent to flagellar genes and evolution of flagellar-phase variation. J. Bacteriol. 155, 74–81.
Wain, J., and O'Grady, J. (2017). “Genomic diversity in Salmonella enterica,” in Applied Genomics of Foodborne Pathogens, eds X. Deng, H. C. den Bakker, and R. S. Hendriksen (Cham: Springer), 91–107.
Yamamoto, S., and Kutsukake, K. (2006). FljA-mediated posttranscriptional control of phase 1 flagellin expression in flagellar phase variation of Salmonella enterica serovar Typhimurium. J. Bacteriol. 188, 958–967. doi: 10.1128/JB.188.3.958-967.2006
Yokoseki, T., Kutsukake, K., Ohnishi, K., and Iino, T. (1995). Functional analysis of the flagellar genes in the fliD operon of Salmonella typhimurium. Microbiology 141 (Pt 7), 1715–1722. doi: 10.1099/13500872-141-7-1715
Yue, M., Han, X., De Masi, L., Zhu, C., Ma, X., Zhang, J., et al. (2015). Allelic variation contributes to bacterial host specificity. Nat. Commun. 6:8754. doi: 10.1038/ncomms9754
Zhou, Z., McCann, A., Litrup, E., Murphy, R., Cormican, M., Fanning, S., et al. (2013). Neutral genomic microevolution of a recently emerged pathogen, Salmonella enterica serovar Agona. PLoS Genet. 9:e1003471. doi: 10.1371/journal.pgen.1003471
Zieg, J., Hilmen, M., and Simon, M. (1978). Regulation of gene expression by site-specific inversion. Cell 15, 237–244. doi: 10.1016/0092-8674(78)90098-3
Keywords: Salmonella enterica, flagellin genes, fliC, fljB, recombination
Citation: Liu Y, Zhang D-F, Zhou X, Xu L, Zhang L and Shi X (2017) Comprehensive Analysis Reveals Two Distinct Evolution Patterns of Salmonella Flagellin Gene Clusters. Front. Microbiol. 8:2604. doi: 10.3389/fmicb.2017.02604
Received: 01 September 2017; Accepted: 14 December 2017;
Published: 22 December 2017.
Edited by:
John R. Battista, Louisiana State University, United StatesReviewed by:
Xiaohui Zhou, University of Connecticut, United StatesSunil D. Saroj, Symbiosis International University, India
Copyright © 2017 Liu, Zhang, Zhou, Xu, Zhang and Shi. This is an open-access article distributed under the terms of the Creative Commons Attribution License (CC BY). The use, distribution or reproduction in other forums is permitted, provided the original author(s) or licensor are credited and that the original publication in this journal is cited, in accordance with accepted academic practice. No use, distribution or reproduction is permitted which does not comply with these terms.
*Correspondence: Xianming Shi, eG1zaGlAc2p0dS5lZHUuY24=
†These authors have contributed equally to this work.