- 1Laboratory of Microbiology, Department of Veterinary Public Health and Food Safety, Faculty of Veterinary Medicine, Ghent University, Ghent, Belgium
- 2Section for Microbiology, Department of Biology, University of Copenhagen, Copenhagen, Denmark
- 3Laboratory of Protistology and Aquatic Ecology, Department of Biology, Faculty of Sciences, Ghent University, Ghent, Belgium
Biofilm formation has been shown to confer protection against grazing, but little information is available on the effect of grazing on biofilm formation and protection in multispecies consortia. With most biofilms in nature being composed of multiple bacterial species, the interactions and dynamics of a multispecies bacterial biofilm subject to grazing by a pelagic protozoan predator were investigated. To this end, a mono and multispecies biofilms of four bacterial soil isolates, namely Xanthomonas retroflexus, Stenotrophomonas rhizophila, Microbacterium oxydans and Paenibacillus amylolyticus, were constructed and subjected to grazing by the ciliate Tetrahymena pyriformis. In monocultures, grazing strongly reduced planktonic cell numbers in P. amylolyticus and S. rhizophila and also X. retroflexus. At the same time, cell numbers in the underlying biofilms increased in S. rhizophila and X. retroflexus, but not in P. amylolyticus. This may be due to the fact that while grazing enhanced biofilm formation in the former two species, no biofilm was formed by P. amylolyticus in monoculture, either with or without grazing. In four-species biofilms, biofilm formation was higher than in the best monoculture, a strong biodiversity effect that was even more pronounced in the presence of grazing. While cell numbers of X. retroflexus, S. rhizophila, and P. amylolyticus in the planktonic fraction were greatly reduced in the presence of grazers, cell numbers of all three species strongly increased in the biofilm. Our results show that synergistic interactions between the four-species were important to induce biofilm formation, and suggest that bacterial members that produce more biofilm when exposed to the grazer not only protect themselves but also supported other members which are sensitive to grazing, thereby providing a “shared grazing protection” within the four-species biofilm model. Hence, complex interactions shape the dynamics of the biofilm and enhance overall community fitness under stressful conditions such as grazing. These emerging inter- and intra-species interactions could play a vital role in biofilm dynamics in natural environments like soil or aquatic systems.
Introduction
In recent years, protozoa–bacteria interactions have received increasing attention in studies ranging from ecology to consumer health and diseases. Free-living protozoa are commonly found in natural environments like soils and aquatic habitats (Foissner, 1999; Ekelund et al., 2001; Pfister et al., 2002; Pernthaler, 2005) and in anthropogenic environments like swimming pools (Rivera et al., 1993), drinking water systems (Thomas and Ashbolt, 2011), kitchens (Chavatte et al., 2014) and health care facilities (Singh and Coogan, 2005; Cateau et al., 2014). Various studies have also reported the presence of bacterial biofilms in such environments (Bryers, 2008; Burmølle et al., 2011; Besemer et al., 2012). Though most studies emphasize that the main role played by the protozoa lies in control of the bacterial populations by predation (Jürgens and Güde, 1994; Brown and Barker, 1999; Arndt et al., 2003; Logares et al., 2012), another potential impact involves the induction of biofilm formation by bacterial communities (Joubert et al., 2006) to avoid grazing.
Biofilm formation represents a surface attached mode of life (Donlan, 2002) that can contain multiple species of archaea, bacteria, fungi, and algae (Flemming et al., 2016). Biofilms offer physical protection through the secreted polymeric matrix (Joubert et al., 2006) that creates a protective microhabitat against predation (Darby et al., 2002; Matz et al., 2005; DePas et al., 2014). Close interactions between bacteria and protozoa in biofilms are also thought to give rise to a series of adaptations in bacterial communities by promoting horizontal gene transfer events, quorum sensing abilities and induce bacterial protein secretion systems (Darby et al., 2002; Matz et al., 2004) enhancing their survival, dynamics and coexistence (Matz and Kjelleberg, 2005).
Grazing by protozoa has been reported to stimulate micro-colony formation, alter mass transfer of nutrients and induce biofilm development by stimulating bacterial layer thickness (Matz et al., 2004; Weitere et al., 2005; Kaminskaya et al., 2007; Wey et al., 2008; Böhme et al., 2009). Other studies, however, argue that protozoa do not induce biofilm formation (Huws et al., 2005) but instead show a marked preference for grazing on attached or aggregated bacterial cells or only change biofilm community structure (Caron, 1987; Sibbald and Albright, 1988; Huws et al., 2005; Wey et al., 2008). Furthermore, studies have also shown that the grazed or consumed bacterial cells can become adapted to resist uptake or digestion and are even capable of intracellular replication within the protozoan host cells (Rowe and Grant, 2006; Taylor et al., 2009; Lambrecht et al., 2015). Although feeding interactions between protozoa and planktonic bacteria are well understood (Jürgens and Matz, 2002; Matz and Jürgens, 2005; Roberts et al., 2011) only few studies have attempted to assess the impact of grazing on biofilms at the multi-species level. In multispecies biofilm settings, interactions between different bacteria play an important role in determining the structure, function and dynamics of the biofilms and it has been suggested that they contribute to defense mechanisms of bacterial biofilms against predators (Wey et al., 2008; Matz, 2011). Moreover, it has been shown that interspecific interactions within the mixed bacterial communities in the presence of a grazing protist promoted co-aggregation of bacterial members and enhanced complex biopolymer degradation pathways leading to an overall increase in carbon transfer efficiency (Corno et al., 2013, 2015). Mixed biofilms have in other cases been shown to offer the harbored species protection against antibacterial compounds and enhanced capabilities of invasion and virulence within host organisms (Burmølle et al., 2014).
Different protozoan members have different impact on the microbial communities (Brown and Barker, 1999; Paisie et al., 2014). In soils, protozoa present themselves as a diverse group of flagellates, ciliates, and naked amoebae (Ekelund and Rønn, 1994; Bonnet et al., 2005). Like flagellates, ciliates display a substantial diversity in motility, morphology and feeding strategies (Dopheide et al., 2011) and are considered to be important predators of bacteria. Hence, there is a need to unravel different prey-predator interactions and their impact on mixed species bacterial biofilm, as mixed biofilms are the predominant lifestyle in most ecosystems (Battin et al., 2003; Costerton, 2007; Mielich-Süss and Lopez, 2015). Grazing on diverse biofilms is likely to shape the existing complex interactions within the biofilm communities (Wen et al., 2010; Hansen et al., 2017) or alter the feeding traits of protozoa. Examples include Gram-negative bacteria being more vulnerable to grazing than Gram-positive bacteria (Rønn et al., 2002) or altered feeding responses of protozoa to one bacterial group over another (Dopheide et al., 2011).
The aim of the present study is to assess whether individual biofilm bacterial species gain enhanced protection by other members in multispecies consortia under grazing pressure. Therefore, we examined the effect of grazing by the ciliate Tetrahymena pyriformis on biofilm formation and population dynamics in a consortium composed of four bacterial soil species Xanthomonas retroflexus, Stenotrophomonas rhizophila, Microbacterium oxydans, and Paenibacillus amylolyticus. These four strains when combined have been shown to act synergistically resulting in increased biofilm development (Ren et al., 2015). Ciliates were shown to be effective bacterial grazers with often extremely high ingestion rates (Iriberri et al., 1995), making them a specialized subgroup within the protist (Parry, 2004). Under such extreme grazing pressure, we hypothesize that multispecies biofilms will generate a protective effect compared to single species biofilms. We used a qPCR protocol developed previously for these model consortia (Ren et al., 2014) to quantify the species-specific impact of protozoan grazing.
Materials and Methods
Soil Isolates and Protozoa Culture Conditions
The bacterial species X. retroflexus (JQ890537), S. rhizophila (JQ890538), M. oxydans (JQ890539), and P. amylolyticus (JQ890540) stored in the culture collection of the Section of Microbiology, University of Copenhagen, were subcultured from frozen glycerol stocks onto TSA plates (Tryptic Soy Agar, Sigma–Aldrich, Germany). The plates were incubated at 24°C for 48 h. Single colonies were inoculated into 5 ml TSB (Tryptic Soy Broth, Sigma–Aldrich, Germany) media and incubated with shaking at 180 rpm for 24 h at 24°C when required. These strains were used as bacterial prey for the protozoan predator.
A T. pyriformis (Tp) culture (Culture Collection of Algae and Protozoa, CCAP nr 1630/w1) was provided by Department of Veterinary Public Health and Food Safety, Ghent University, Belgium. Axenic cultures of this protozoan were maintained in 25 cm2 culture flasks with 20 ml PPY medium [proteose peptone yeast extract; 20 g Proteose peptone (Merck KgaAm Germany), 2.5 g yeast extract (Merck KgaAm Germany) in 1 L H2O; autoclaved]. Weekly maintenance of the ciliate cultures at 24°C was done by aseptically transferring 5 ml of the culture into 15 ml fresh PPY medium incubated. For biofilm grazing experiments, T. pyriformis cells in exponential phase (after 48 h at 24°C) were washed twice in PAS (Page’s amoeba saline) solution followed by centrifuging at 850 g after which the cells were re-suspended into 10 ml TSB media.
Biofilm Cultivation and Grazing Experiments
Biofilm cultivation experiments were performed in 96-well cell culture plates (cat. no. 655180, Greiner Bio One, Germany). The four selected strains were screened for biofilm formation as single species (monospecies) and in three/four-species combination (multispecies) as described (Ren et al., 2015) both in the presence and absence of protozoa. Briefly, bacterial cell cultures in exponential growth phase (OD600 between 0.3 – 0.6) were selected and adjusted to a start OD600 of 0.15 in TSB media for all cultures. For monospecies biofilms, aliquots of 150 μl of cell culture and for three- and four-species biofilms, respectively, 50 or 37.5 μl of each bacterial strain were added into the wells so that the final inocula were 150 μl in all the settings. To the wells that were to be grazed, an volume of 1.5 μl containing ∼approximately 1000 cells T. pyriformis cells in TSB media were added. The plates were incubated at 24°C for 12, 24, and 96 h. Wells containing only 150 μl TSB media and TSB media with T. pyriformis cells served as blank/control. Three wells each time served as one technical replicate and this was repeated at five different times.
Quantification of Biofilm and Planktonic Fractions
Biofilm formation was assayed and quantified using the traditional crystal violet (CV) method as previously described (Ren et al., 2014). The biofilm attached to the wells was then washed twice gently with 160 μl 1X PBS (phosphate buffer saline) solution and stained with 180 μl 1% (w/v) CV solution. After 20 min of staining, the CV solution was removed by pipette, and the stained biofilm was gently washed five times with 200 μl PBS solution. The remaining CV dye retained by the biofilm was de-stained into 200 μl 96% ethanol for 30 min. Biofilm formation was then quantified by measuring the absorbance of de-stained CV at 590 nm using EL340 BioKinetics reader (BioTek Instruments, United States) and expressed as biofilm forming index (BFI) according to the equation BFI = (AB-CW)/G (Niu and Gilbert, 2004) where, AB: OD590 of attached microorganisms, CW: OD590 control wells and G: OD600 of cells in planktonic fraction. Biodiversity (BD) effect was calculated as the difference between the observed biofilm yield (biofilm of mixed cultures) and the expected yield (average of the monoculture yields) (Loreau and Hector, 2001; Vanelslander et al., 2009). Biofilm fold (Fd), i.e., the observed increase in biofilm formation due to grazing is the ratio between OD590 of grazed three-species biofilm and OD590 of non-grazed three-species biofilm.
Quantification of the biofilm and planktonic fractions was performed by plating. 100 μl of the planktonic fraction from the wells after 24 and 96 h incubation was suspended in 900 μl 1X PBS solution. Once the planktonic fractions were removed, the wells with attached biofilm were gently washed twice with 160 μl 1X PBS solution. The wells were then filled with 200 μl 1X PBS and mixed thoroughly by pipetting. Serial dilutions in 900 μl 1X PBS were performed and 100 μl of the dilutions were plated onto TSA plates by spread plating after which the plates were allowed to dry completely at room temperature. Drying restricts the movement of T. pyriformis on plates. The plates were then incubated for 48 h at 24°C. Single colonies formed after incubation were counted and the results were calculated in CFU (colony forming units). Grazing fold, i.e., the percentage reduction in planktonic fraction due to grazing was expressed by 100 × [CFU(culture) - CFU(culture+Tp)/CFU(culture)]. Changes in cell counts from biofilm fraction were expressed by log [CFU(culture+Tp)/CFU(culture)].
Ciliate Growth on Bacterial Cultures
We determined the ciliate numbers of T. pyriformis grown on the four bacteria separately (monospecies) and as a mixture (four-species) for up to 96 h at regular intervals in microtiter plates. The protozoa cells were counted using a Sedgewick-Rafter chamber and an inverted microscope (40× magnification) as described previously (Gittleson and Ganapathy, 2011) with minor modifications. The wells containing the suspension of bacteria and protozoa were homogenized by pipetting and 150 μl of the cell suspension was fixed in 1% (w/v) Lugol’s iodine solution to a final volume of 1.2 ml in dH2O. The contents were then immediately transferred to the counting chamber and the cells were allowed to settle for few minutes. The change in protozoa cell numbers over time was expressed using ΔN = log10 (Nt -N0)/t. To visualize the changes in protozoa numbers over time in co-culture with bacteria, 50 μl spots of the fixed suspension were made on glass slides and micrographs were taken at different time points using Zeiss Axioplan II, Carl Zeiss with a 10× objective.
16S rRNA Based Fluorescent in Situ Hybridization (FISH) and Confocal Imaging to Investigate Grazing
To visualize the effects of grazing by the protozoan and the internalization of bacteria within the food vacuoles of T. pyriformis, FISH was performed with 16S rRNA gene probes targeting the specific bacteria (Liu et al., 2017). 50 μl spots of co-culture suspensions (bacteria and protozoa) after 24 h were collected after thorough pipetting to homogenize the suspension. The collected cells were then left to air dry on a glass slide. The above step was repeated five times (5 μl × 50 μl) with the aim to collect more cells. The attached cells were coated with 0.5% (w/v) agarose by immersing the slides into a tube containing 45 ml molten agarose and fixed using 4% PFA (paraformaldehyde) at 4°C. Samples were dehydrated and the hybridization protocol was performed according to (Amann, 1995; Daims, 2009) with 30% formamide concentration. After hybridization, the slides were washed in cold water and dried at room temperature. The slides were stored in the dark and visualized under confocal microscopy (Point-scanning confocal and multiphoton microscope SP5-X MP, Leica Microsystems). Images were processed using Leica Application Suite X.
qPCR Quantification of Bacterial Cell Numbers in Multispecies Setting
The biofilm formation assay was conducted both in the presence and absence of T. pyriformis in 96-well microtiter plates as described above. After 24 h, the planktonic fractions were collected in Eppendorf tubes and the biofilm fraction was rinsed twice with weak phosphate buffer to remove loosely attached cells. Three replicate wells were prepared for each treatment. The cell numbers of the four strains in multispecies planktonic and biofilm fractions with and without protozoa were quantified by SYBR Green qPCR using standard curves generated by serial 10-fold dilutions of plasmid DNA using the species specific primers and thermal profile setup previously reported (Ren et al., 2014). All samples were run in triplicate and a no template control was included in each run. Bacterial DNA was extracted using FastDNATM SPIN Kit for soil (MP Biomedicals, Germany) according to manufacturer’s instruction.
Results
T. pyriformis Grazing Promotes Biofilm Formation and Reduces the Number of Bacteria in the Planktonic Fractions
Monocultures and four-species mixed cultures of X. retroflexus, S. rhizophila, M. oxydans, and P. amylolyticus were tested for biofilm formation in the absence and presence of protozoa (Figure 1). T. pyriformis grazing on monospecies cultures of X. retroflexus and S. rhizophila resulted in significantly enhanced biofilm formation (paired t-test, P < 0.05) whereas M. oxydans and P. amylolyticus monocultures did not form biofilms neither in the presence nor in the absence of T. pyriformis. Biofilm formation was enhanced in the four-species mixtures, and was even more strongly induced in these mixtures in the presence of grazing for up to 96 h (n = 5, paired t-test, P < 0.05), suggesting a strong biodiversity effect (Supplementary Figure S1). Moreover, biofilm formation in the mixtures was higher than in the best performing monoculture both in the absence and presence of grazing.
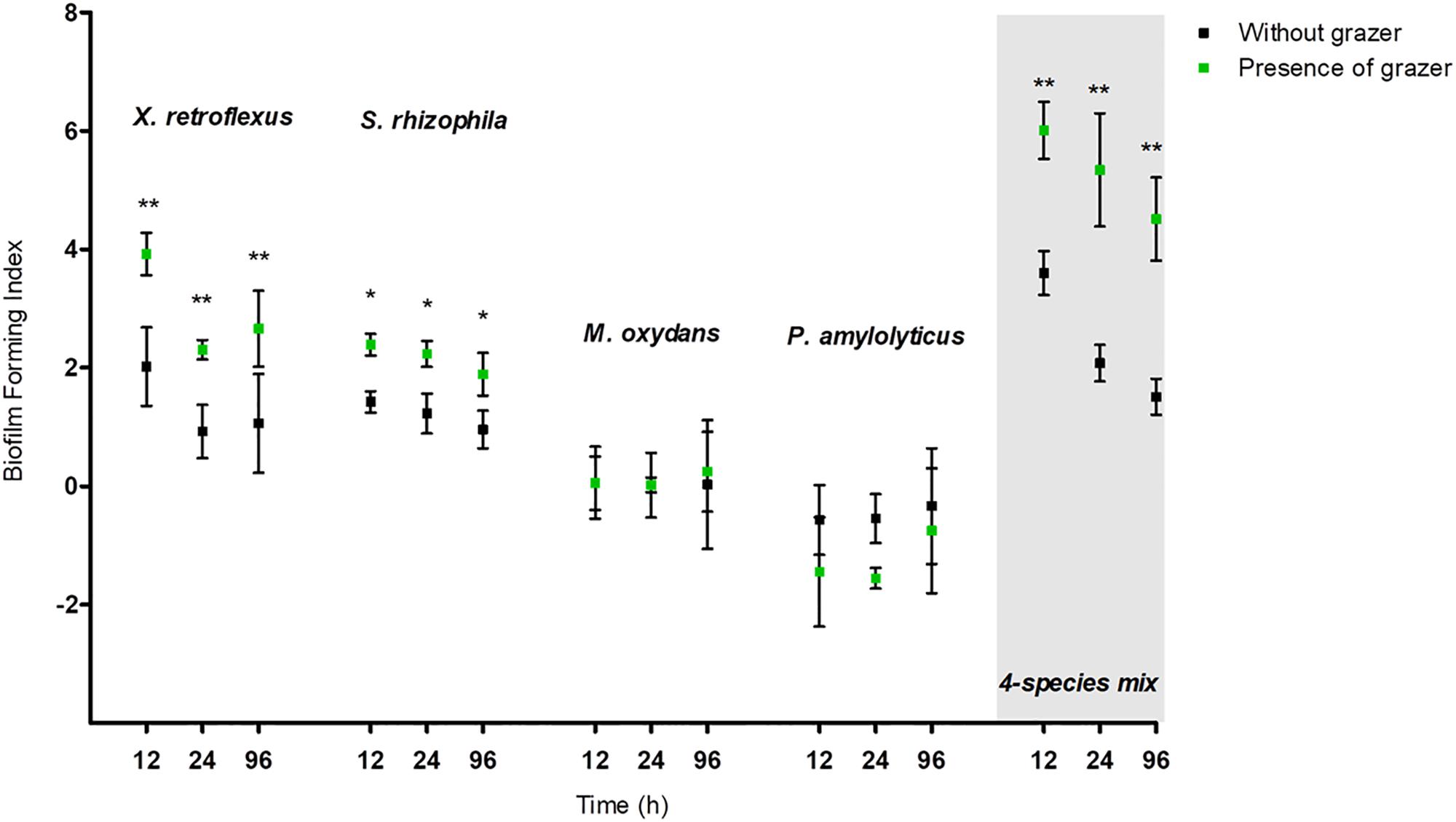
FIGURE 1. Biofilm forming index (BFI) of mono and mixed species cultures subject to Tetrahymena pyriformis (Tp) grazing and non-grazed cultures at 12, 24, and 96 h. The data points indicate the biofilm mean ± standard error of the mean (SEM) obtained from five biological replicates.∗P < 0.05, ∗∗P < 0.01.
Planktonic fractions of three of the four bacterial species were effectively grazed upon in monoculture, but less so in the four-species co-culture. P. amylolyticus was the most intensively grazed species at 24 h, whereas after 96 h S. rhizophila monocultures were the most highly grazed followed by P. amylolyticus and X. retroflexus monocultures. Among all the strains, M. oxydans was the least preferred prey, and S. rhizophila and P. amylolyticus were the most favored prey (Figure 2). These grazing experiments verified the ability of T. pyriformis to feed on planktonic bacteria. In the four-species mixed cultures, overall grazing by T. pyriformis on the planktonic community was reduced compared to the monospecies cultures observed by the low grazing fold values at 24 and 96 h (Figure 2).
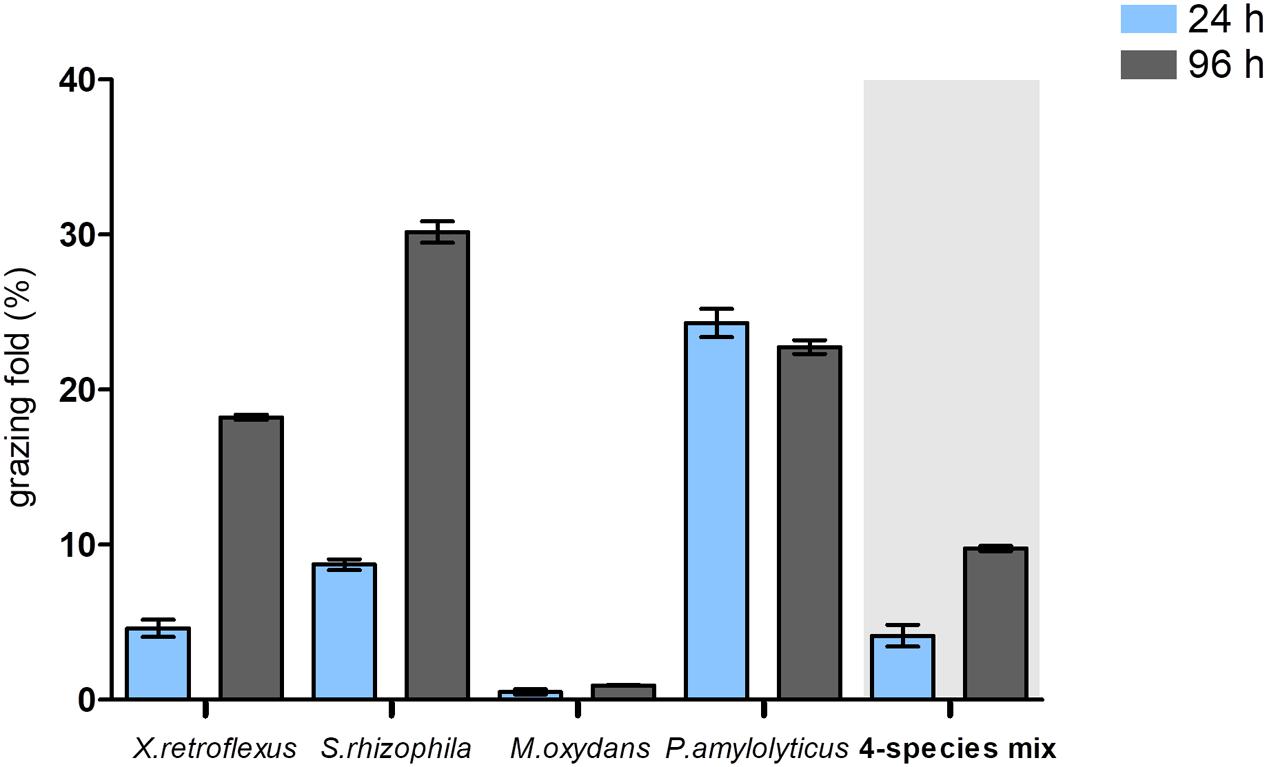
FIGURE 2. Percentage of mono and multispecies planktonic cultures grazed by T. pyriformis after 24 and 96 h compared to the non-grazed cultures. The data points indicate the percentage reduction in cell numbers (%) ± SEM obtained from five biological replicates.
In the biofilm fraction, cell numbers of X. retroflexus and S. rhizophila increased at 24 and 96 h in the grazed relative to the non-grazed monocultures whereas the cell numbers of M. oxydans and P. amylolyticus decreased with grazing compared to the non-grazed monocultures (Figure 3). This underscores the inability of M. oxydans and P. amylolyticus to form a biofilm in monoculture. In the four-species culture, total cell numbers increased both at 24 and 96 h compared to the non-grazed biofilm (Figure 3 and Supplementary Figure S2).
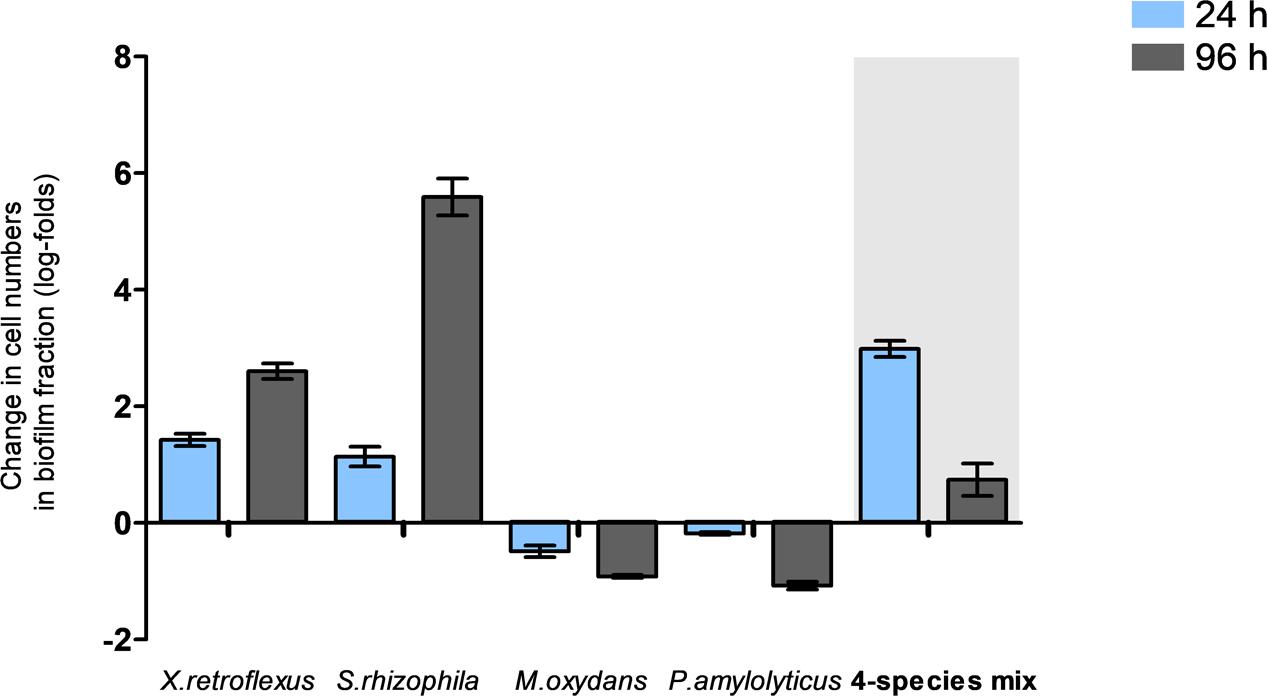
FIGURE 3. Change in viable cell numbers from the biofilm fractions of mono and multispecies cultures grazed with T. pyriformis after 24 and 96 h obtained from plating. The data points indicate the change in cell numbers of grazed biofilm fraction relative to the non-grazed biofilm fraction ± SEM obtained from two biological replicates.
Growth of T. pyriformis on Bacterial Cultures
The growth of T. pyriformis cells on all bacterial isolates cultured as both mono and mixed planktonic cultures was followed over time (Figure 4). The change in cell numbers over time demonstrated that S. rhizophila and P. amylolyticus were suitable prey for the protozoa (Figures 4B,D) and that TSB media can support the axenic growth of protozoa (Figure 4F). Growth on M. oxydans was not pronounced (Figure 4C); while X. retroflexus monocultures had a negative impact on the growth of the protozoa at 96 h (Figure 4A). Our results thus indicate that T. pyriformis may prefer to graze on S. rhizophila and P. amylolyticus. The numbers of protozoa grazing on the four-species mixed cultures represent a smoother curve over time indicating that the protozoa can adapt to an available prey in multispecies bacterial environments (Figure 4E).
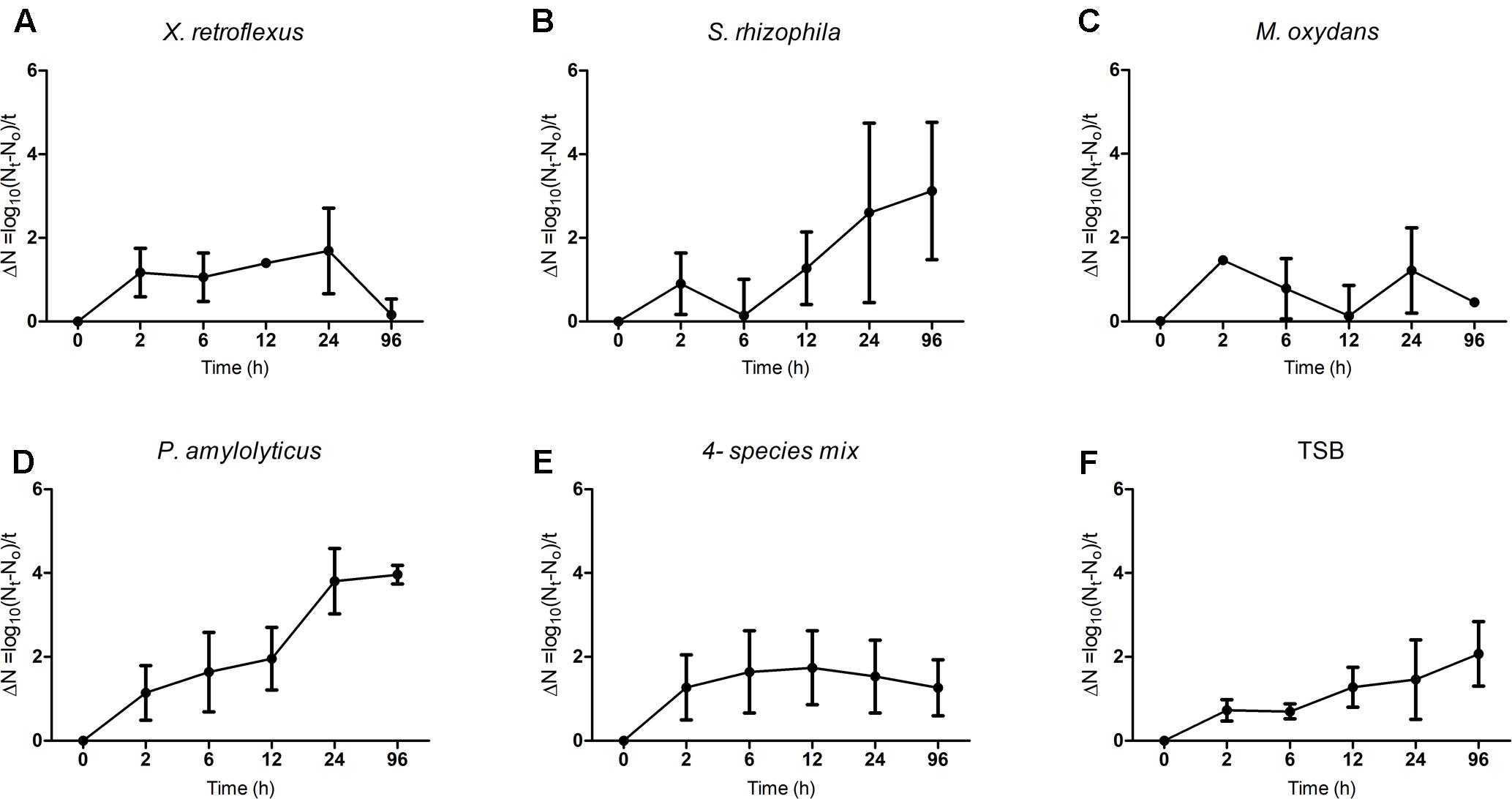
FIGURE 4. Tetrahymena pyriformis growth curves. The data points indicate the change in protozoan cell numbers with respect to time (t = 0 h) that were grown in co-culture with the bacterial isolates (A–D) as monocultures and (E) as four-species mixed culture. (F) Change in protozoan cell numbers under axenic conditions over time in TSB media. The data shown are mean ± SEM from three biological replicates.
To visualize the change in protozoan numbers over time, micrographs showing T. pyriformis cells raised on both mono and multispecies bacterial cultures are shown (Supplementary Figure S3). The protozoan population raised on the four-species mixtures remained viable for up to 96 h. However, in monospecies cultures; it can be seen that the protozoan cell numbers increased from 24 h and reached a maximum at 96 h when co-cultured with S. rhizophila and P. amylolyticus whereas the protozoa population declined from 24 to 96 h in co-culture with X. retroflexus and M. oxydans.
Grazed Bacterial Prey within the Food Vacuoles of T. pyriformis
To visualize grazing on monocultures and mixed cultures, a 16S rRNA gene based FISH was performed, similar to a previous study (Jezbera et al., 2005), after 24 h of grazing and samples were visualized by laser scanning confocal microscopy. It was confirmed that T. pyriformis can consume the bacteria in all tested monospecies settings, however, at seemingly different rates as indicated by the number of food vacuoles formed within the ciliates (Figure 5). In co-cultures of T. pyriformis with X. retroflexus or S. rhizophila monocultures, the bacteria were abundantly present within the food vacuoles of T. pyriformis cells (Figures 5A,B) showing that these bacterial strains are readily consumed. P. amylolyticus cells were also found to be localized within the food vacuoles of grazers but not as abundantly as compared to X. retroflexus and S. rhizophila (Figure 5D). Most protozoan cells appeared to form cysts when co-cultured with M. oxydans (Figure 5C), but some bacterial cells were found to be internalized within T. pyriformis indicating that the protozoa were able to consume M. oxydans cells. In the case of grazing on four-species mixed cultures (Figures 6A–H), most food vacuoles were dominated by X. retroflexus indicating that at 24 h most protozoan cells prefer to graze on X. retroflexus. This was in accordance with the fact that this bacterium previously was shown to dominate the 24 h mixed biofilm population (Ren et al., 2014) and thus could be readily available for the grazers.
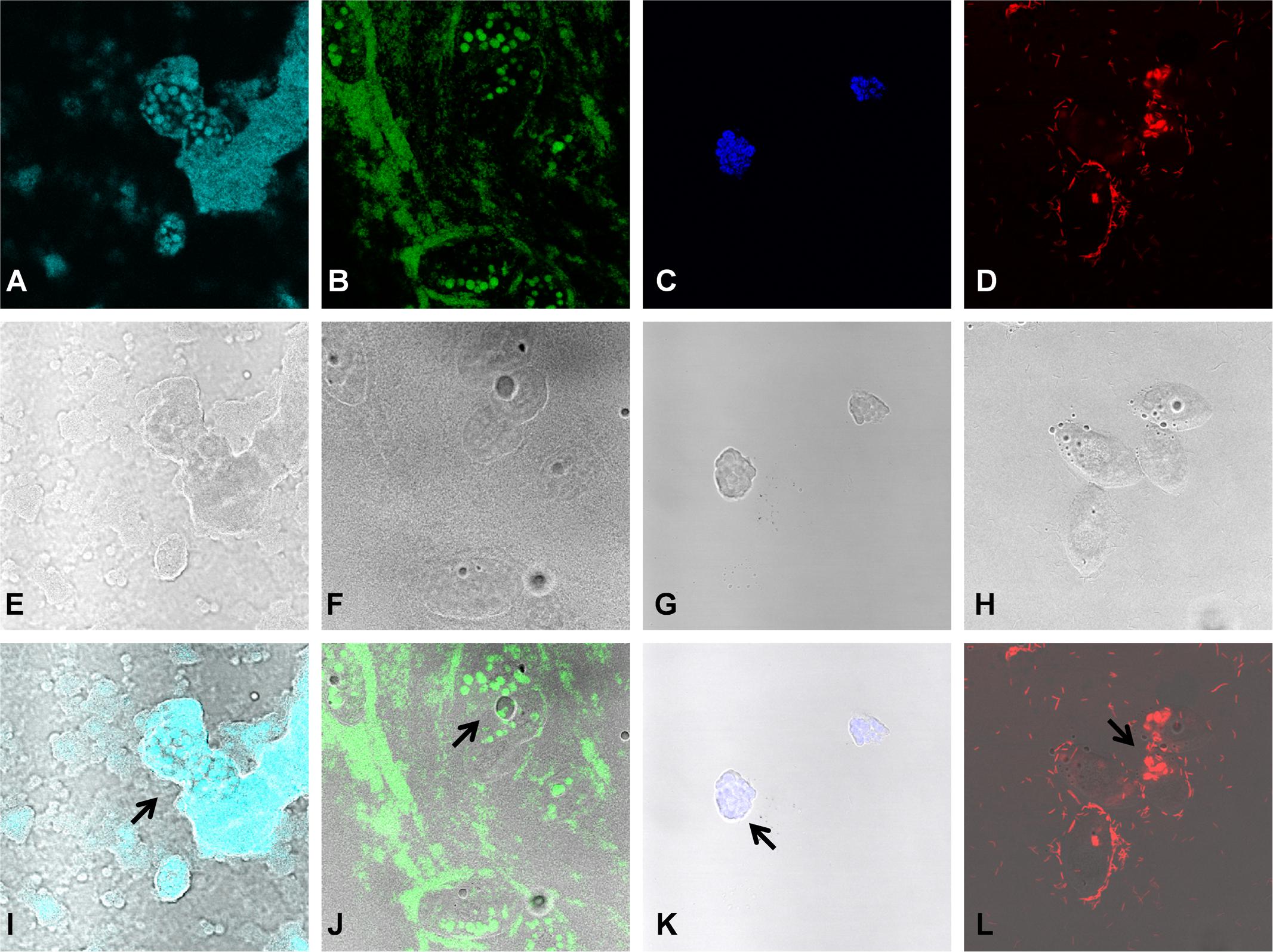
FIGURE 5. Grazing by protozoa on monospecies bacterial cultures. FISH based staining and confocal imaging shows Xanthomonas retroflexus (FL: A, BF: E, and OL: I), Stenotrophomonas rhizophila (FL: B, BF: F, and OL: J), Microbacterium oxydans (FL: C, BF: G, and OL: K) and Paenibacillus amylolyticus (FL: D, BF: H, and OL: L) cells, cultured as monospecies, localized within the food vacuoles (indicated by the arrows) of T. pyriformis cells after 24 h of grazing. ‘FL’ denotes fluorescence, ‘BF’ denotes bright-field and ‘OL’ denotes overlay images, respectively.
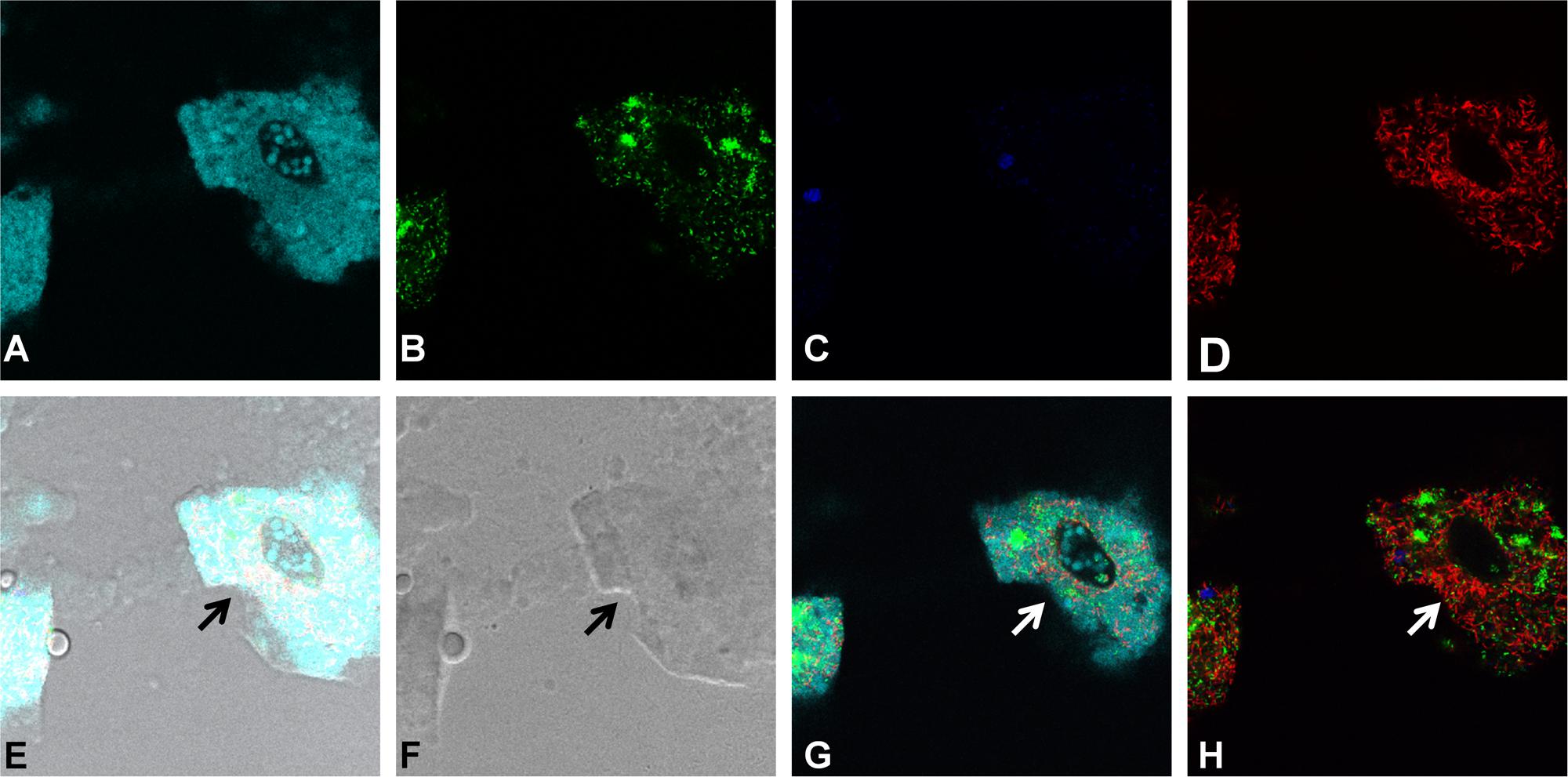
FIGURE 6. Grazing for 24 h by protozoa on the four-species mixed cultures. FISH based staining and confocal imaging shows the distribution of the different bacterial species in and around the protozoan cells. Applying the fluorescence filter channels, it is observed that X. retroflexus is abundantly present within the food vacuoles (indicated by the arrows) of T. pyriformis (A). S. rhizophila (B) is detected to a lesser extent whereas M. oxydans (C) and P. amylolyticus (D) cells are not visibly present in the food vacuoles. (E,F) Depict the overlay and bright-field images, respectively. Panels (G,H) were included to phase out the dominating fluorescence signals from X. retroflexus and visualize the other bacterial members in the biofilm consortia around the ciliate.
Biofilm Formation by X. retroflexus Is Vital to the Overall Biofilm Development
From the above results, biofilm formation in the presence of T. pyriformis was further assessed to better understand the dynamics. To this end, either the least preferred prey M. oxydans or the best biofilm producer X. retroflexus were excluded three-species consortia (Figure 7). Biofilm formation (biofilm-fold Fd) was enhanced when X. retroflexus remained in the consortium together with S. rhizophila and P. amylolyticus, indicating that the interaction between these three members is vital for biofilm stability. However, in the absence of X. retroflexus and in the presence of M. oxydans, the consortium was effectively grazed, although there seemed for this consortium to be a gradual adaptation to predation (as evidenced by increased biofilm formation) over time.
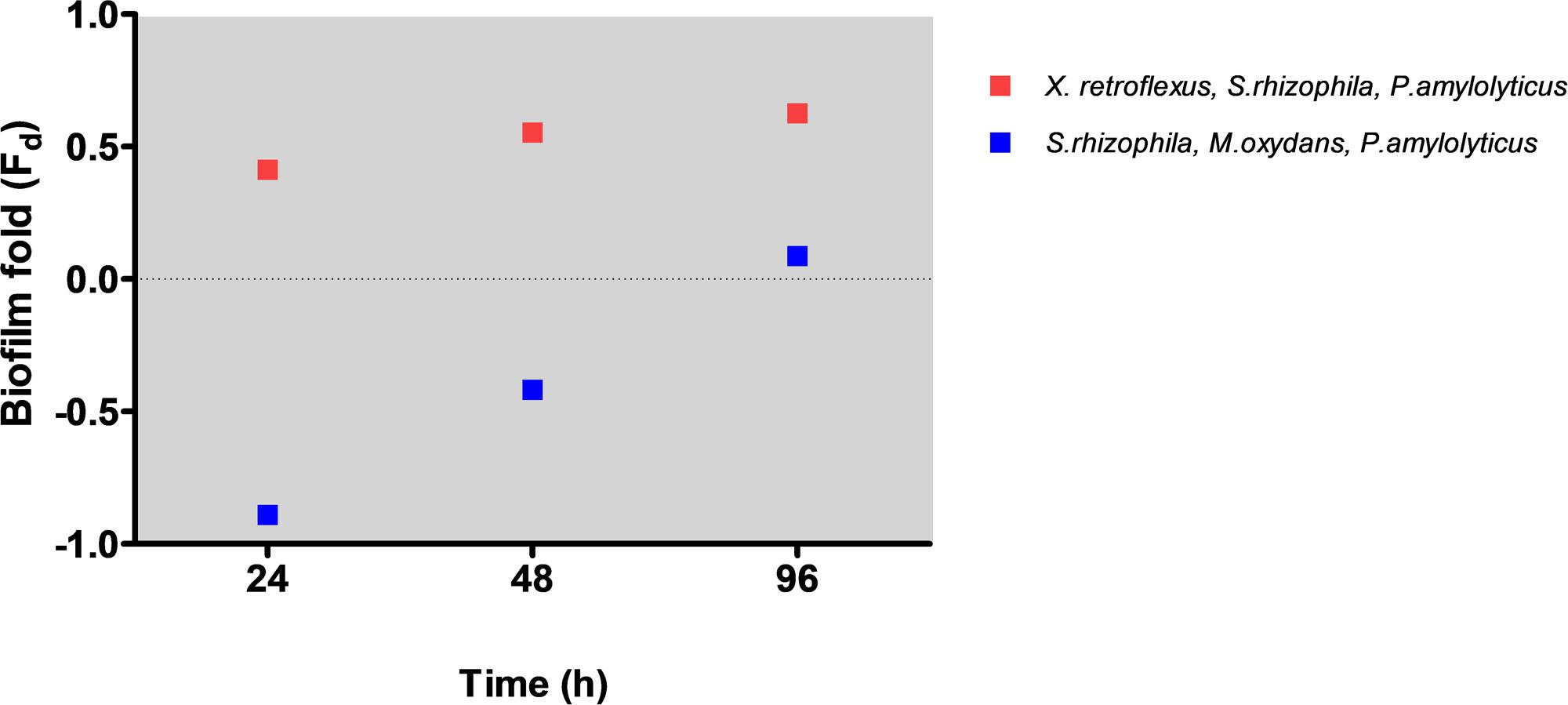
FIGURE 7. Biofilm formation in the presence of T. pyriformis in three-species bacterial consortia. X. retroflexus is vital for biofilm development. Biofilm fold was calculated as the ratio of Abs590 [(three – species biofilm cultured with grazer cells + SEM) – (three – species biofilm as control – SEM)] to Abs590 (three – species biofilm cultured with grazer cells + SEM).
Impact of Grazing on the Population Dynamics of Individual Bacterial Species in Multispecies Biofilm and Planktonic Consortia
In order to determine the cell numbers of the individual species within the multispecies consortium, 16S rRNA gene based q-PCR quantification was applied according to a previously developed protocol (Ren et al., 2014). The results showed that in the multispecies biofilm fraction, cell numbers of X. retroflexus, S. rhizophila, and P. amylolyticus increased in the presence of grazers compared to the control biofilms that were not grazed. The ∼2.5-fold increase in cell numbers of X. retroflexus and P. amylolyticus and 1.7-fold increase in S. rhizophila cell numbers suggest that synergistic interactions between these species were enhanced in the presence of grazing, resulting in increased cell numbers in the biofilm. The cell numbers of M. oxydans in the biofilm remained unaffected either in the presence or absence of grazers (Figure 8A).
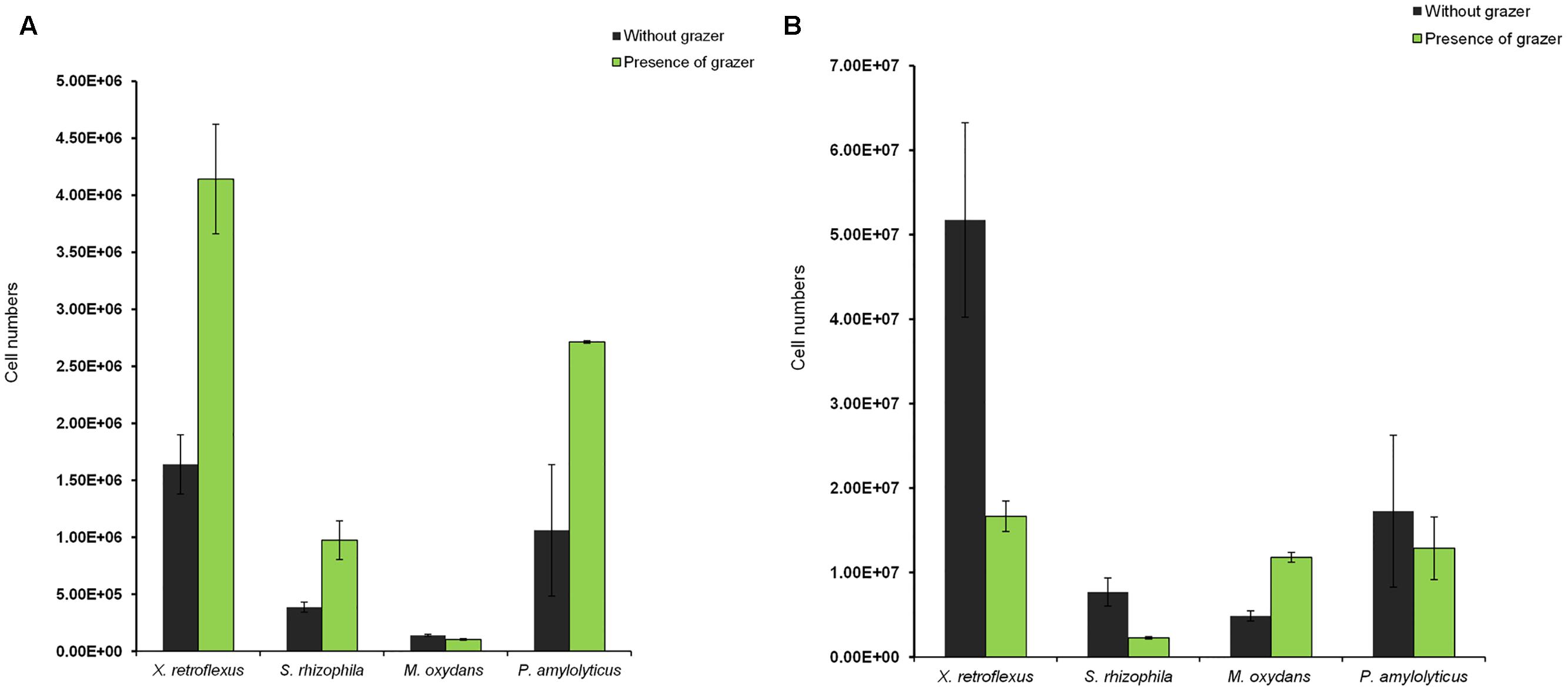
FIGURE 8. Impact of grazing by T. pyriformis on the population dynamics of the individual bacterial species in the multispecies consortia as assessed by qPCR. Cell numbers of the individual bacterial members in (A) the multispecies biofilm fraction and (B) the multispecies planktonic fraction after 24 h of grazing.
In the planktonic fraction without grazing, a similar trend in cell numbers compared to the non-grazed biofilm was seen with X. retroflexus, P. amylolyticus, and S. rhizophila being the dominant species (Figure 8B). However, the planktonic cell numbers of these species decreased in the presence of T. pyriformis indicating an effect of grazing on these planktonic fractions. In contrast, the cell numbers of M. oxydans increased, which possibly can be a result of grazing preference of the ciliate in the mixed communities and/or higher nutrient or space availability for M. oxydans cells as the other members of the consortia were grazed upon.
Discussion
In the present study, the impact of grazing by the ciliate T. pyriformis on a previously described synergistic mixed species biofilm model consortium (Ren et al., 2015) was assessed. These bacterial strains were isolated from a single micro-habitat and studies have reported that long-term coexistence within a habitat can stimulate synergistic biofilm development in complex communities (Madsen et al., 2016). Our results showed that co-culturing T. pyriformis with single-species bacterial cultures stimulated biofilm formation in X. retroflexus and S. rhizophila strains but not in M. oxydans and P. amylolyticus (Figure 1). S. rhizophila and P. amylolyticus were most sensitive to grazing (Figure 2). Ciliate abundances reached a maximum in co-culture with these strains over time indicating extensive feeding on these strains (Figures 4B,D and Supplementary Figure S1). The monospecies grazing experiments thus indicate differential bacterial behavior in response to a predator and vice-versa. Similar observations have been reported previously where protozoa regulate the social behavior of the bacteria (Rønn et al., 2002; Scherwass et al., 2016) or where bacteria regulate the protozoan population (Kaminskaya et al., 2007). The specificity of such responses has been reported to vary depending on the selected bacteria and protozoa (Dopheide et al., 2011; Friman et al., 2013).
In the four-species consortia, biofilm formation was enhanced even when compared to the best performing monoculture, suggesting a strong and significant biodiversity effect which was even further enhanced in the presence of grazing (Figure 1 and Supplementary Figure S1). The total cell numbers in mixed biofilm fraction under grazed conditions were increased compared to non-grazed mixed biofilm (Figure 3) and at the same time, qPCR results showed that the bacterial numbers of all strains except M. oxydans increased in comparison with the non-grazed biofilm. Even the grazing sensitive species P. amylolyticus increased in cell numbers in the mixed biofilm during grazing. X. retroflexus dominated the grazed biofilm followed by P. amylolyticus and S. rhizophila, respectively (Figure 8A). This suggests strong synergistic and complex interactions between these species under grazing pressure, resulting in a shared protection against grazing. In contrast, total bacterial numbers in the multispecies planktonic fraction under grazing were reduced for all species, except M. oxydans (Figure 8B). This can be explained by the lowest grazing preference for M. oxydans in monoculture. Protozoan cell numbers in the mixed planktonic cultures (Figure 4E) gradually decreased with time, possibly reflecting a lower availability of the preferred individual prey or co-aggregation of the bacterial consortia members into composite aggregates.
In the mixed-species consortia there was an increase by ∼2.5-fold in total bacterial cell numbers (all four species combined) in the grazed biofilm compared to the non-grazed biofilm, whereas in the planktonic fractions grazing reduced total cell numbers by ∼1.8-fold, emphasizing the protective nature of the biofilm mode of life. Evidence that grazing pressure is positively correlated with the formation of cell clusters has come from both monospecies laboratory biofilm (Matz et al., 2004, 2005) and from natural/semi-natural multispecies biofilm (Wey et al., 2008; Rychert and Neu, 2010; Corno et al., 2015). Grazing induced biofilm formation could reflect either an active defense mechanism (Matz and Kjelleberg, 2005; Friman and Buckling, 2014) or a passive mechanical process where the movement of the protozoan cells drives the bacterial cells to the substratum (Wey et al., 2012). Also, protozoan grazing on the planktonic bacterial population could release nutrients which stimulate the biofilm-associated cells resulting in enhanced levels of biofilm formation (Petropoulos and Gilbride, 2005; Böhme et al., 2009). Additionally, the total bacterial productivity is shown to be influenced under grazing where bacterial aggregates display increased carbon transfer and uptake (Corno et al., 2013, 2015). Discrepancies found in the literature with respect to the protective nature of biofilms against grazing (Jackson and Jones, 1991; Huws et al., 2005; Weitere et al., 2005) could be attributed to the type of protozoa used, their feeding mechanism and the growth conditions. Studies have shown feeding traits of grazers to influence grazing resistance in bacterial biofilms (Seiler et al., 2017) and surface associated bacteria can be even more consumed when exposed to a specialized grazer (Rogerson and Laybourn-Parry, 1992). Therefore, more studies with different gazers are needed for a comprehensive understanding on the effect of grazing by protozoan on bacterial biofilm. In this study, we determined the grazing effect on a four species biofilm using a single pelagic grazer, the precise mechanisms that confer grazing resistance to individual species remains unknown. However, the biofilm formation was enhanced in a more diverse biofilm composed of four species, beyond the expected biofilm forming capacities of all monocultures, especially under grazed conditions. Thus, in a multispecies biofilm, the observed protection due to biofilm formation could be seen as a result of synergistic interactions or complementarity within the mixed cultures.
In addition, X. retroflexus dominated the multispecies biofilm while M. oxydans was the least preferred prey in monoculture. However, both these species have been shown to confer synergy and shared protection (Ren et al., 2015; Hansen et al., 2017; Liu et al., 2017). Different three-species co-cultures, set up to investigate the role of these two bacteria in the communal protection observed in the multispecies biofilm, showed that biofilm formation was enhanced by 3.5-folds in the three-species biofilm composed of X. retroflexus, S. rhizophila, and P. amylolyticus in the presence of grazers; but that the synergy was hampered when X. retroflexus was substituted by M. oxydans (Figure 7). From these results, it can be deduced that the intricate interactions between X. retroflexus and the other two members is vital for enhanced biofilm formation and communal grazing resistance. Grazing-sensitive members (S. rhizophila and P. amylolyticus) are more susceptible to grazing in the absence of key biofilm producers such as X. retroflexus. These results demonstrate that synergistic interactions within the multispecies communities are further enhanced under grazing pressure, as also observed by (Corno et al., 2015), and the multispecies biofilm architecture provided grazing sensitive members with improved protection (Burmølle et al., 2014). This emergent property of multispecies biofilms could serve as a public goods strategy, as previously reported for antimicrobials (Lee et al., 2014), and can thus act as a major driver for synergistic cooperative behavior.
Our findings support previous findings (Ren et al., 2015; Madsen et al., 2016) that bacteria can increase their fitness by engaging in the formation of multispecies biofilms. We showed that in multispecies consortium under grazing pressure, cell numbers of free floating bacteria decrease while biofilm cell numbers increase. Our findings thus suggest that synergy in biofilm formation could have evolved from the selective pressures under stressful environmental conditions such as grazing.
Author Contributions
PR, SS, MB, and KH designed the study. PR performed the experiments. PR and WL analyzed the data. PR, WL, KS, KH, MB, and SS revised the manuscript. KH, KS, MB, and SS provided the final approval to publish.
Funding
This study was funded by grants from The Danish Council for Independent Research; ref no: DFF-1335-00071, ref no: DFF-1323-00235 (SIMICOM) and BOF Special Research Fund, Belgium: 01SF1614.
Conflict of Interest Statement
The authors declare that the research was conducted in the absence of any commercial or financial relationships that could be construed as a potential conflict of interest.
Acknowledgment
The authors thank Karin Vestberg and Anette Hørdum Løth for their technical assistance during the experiments.
Supplementary Material
The Supplementary Material for this article can be found online at: https://www.frontiersin.org/articles/10.3389/fmicb.2017.02649/full#supplementary-material
References
Amann, R. I. (1995). “In situ identification of micro-organisms by whole cell hybridization with rRNA-targeted nucleic acid probes,” in Molecular Microbial Ecology Manual, eds A. D. L. Akkermans, J. D. Van Elsas, and F. J. De Bruijn (Dordrecht: Springer), 331–345.
Arndt, H., Schmidt-Denter, K., Auer, B., and Weitere, M. (2003). “Protozoans and biofilms,” in Fossil and Recent Biofilms: A Natural History of Life on Earth, eds W. E. Krumbein, D. M. Paterson, and G. A. Zavarzin (Dordrecht: Springer), 161–179. doi: 10.1007/978-94-017-0193-8_10
Battin, T. J., Kaplan, L. A., Denis Newbold, J., and Hansen, C. M. E. (2003). Contributions of microbial biofilms to ecosystem processes in stream mesocosms. Nature 426, 439–442. doi: 10.1038/nature02152
Besemer, K., Peter, H., Logue, J. B., Langenheder, S., Lindstrom, E. S., Tranvik, L. J., et al. (2012). Unraveling assembly of stream biofilm communities. ISME J. 6, 1459–1468. doi: 10.1038/ismej.2011.205
Böhme, A., Risse-Buhl, U., and Kusel, K. (2009). Protists with different feeding modes change biofilm morphology. FEMS Microbiol. Ecol. 69, 158–169. doi: 10.1111/j.1574-6941.2009.00710.x
Bonnet, J. L., Guiraud, P., Dusser, M., Kadri, M., Laffosse, J., Steiman, R., et al. (2005). Assessment of anthracene toxicity toward environmental eukaryotic microorganisms: Tetrahymena pyriformis and selected micromycetes. Ecotoxicol. Environ. Saf. 60, 87–100. doi: 10.1016/j.ecoenv.2003.10.001
Brown, M. R. W., and Barker, J. (1999). Unexplored reservoirs of pathogenic bacteria: protozoa and biofilms. Trends Microbiol. 7, 46–50. doi: 10.1016/S0966-842X(98)01425-5
Burmølle, M., Kjøller, A., and Sørensen, S. J. (2011). “Biofilms in soil,” in Encyclopedia of Agrophysics, eds J. Gliński, J. Horabik, and J. Lipiec (Dordrecht: Springer), 70–75. doi: 10.1007/978-90-481-3585-1_260
Burmølle, M., Ren, D., Bjarnsholt, T., and Sørensen, S. J. (2014). Interactions in multispecies biofilms: do they actually matter? Trends Microbiol. 22, 84–91. doi: 10.1016/j.tim.2013.12.004
Caron, D. A. (1987). Grazing of attached bacteria by heterotrophic microflagellates. Microb. Ecol. 13, 203–218. doi: 10.1007/BF02024998
Cateau, E., Delafont, V., Hechard, Y., and Rodier, M. H. (2014). Free-living amoebae: what part do they play in healthcare-associated infections? J. Hosp. Infect. 87, 131–140. doi: 10.1016/j.jhin.2014.05.001
Chavatte, N., Bare, J., Lambrecht, E., Van Damme, I., Vaerewijck, M., Sabbe, K., et al. (2014). Co-occurrence of free-living protozoa and foodborne pathogens on dishcloths: implications for food safety. Int. J. Food Microbiol. 191, 89–96. doi: 10.1016/j.ijfoodmicro.2014.08.030
Corno, G., Salka, I., Pohlmann, K., Hall, A. R., and Grossart, H. P. (2015). Interspecific interactions drive chitin and cellulose degradation by aquatic microorganisms. Aquat. Microb. Ecol. 76, 27–37. doi: 10.3354/ame01765
Corno, G., Villiger, J., and Pernthaler, J. (2013). Coaggregation in a microbial predator–prey system affects competition and trophic transfer efficiency. Ecology 94, 870–881. doi: 10.1890/12-1652.1
Costerton, J. W. (eds). (2007). “The biofilm primer,” in Control of all Biofilm Strategies and Behaviours (Berlin: Springer), 85–97. doi: 10.1007/b136878
Daims, H. (2009). Use of fluorescence in situ hybridization and the daime image analysis program for the cultivation-independent quantification of microorganisms in environmental and medical samples. Cold Spring Harb. Protoc. 2009:pdb.prot5253. doi: 10.1101/pdb.prot5253
Darby, C., Hsu, J. W., Ghori, N., and Falkow, S. (2002). Caenorhabditis elegans: plague bacteria biofilm blocks food intake. Nature 417, 243–244. doi: 10.1038/417243a
DePas, W. H., Syed, A. K., Sifuentes, M., Lee, J. S., Warshaw, D., Saggar, V., et al. (2014). Biofilm formation protects Escherichia coli against killing by Caenorhabditis elegans and Myxococcus xanthus. Appl. Environ. Microbiol. 80, 7079–7087. doi: 10.1128/AEM.02464-14
Donlan, R. M. (2002). Biofilms: microbial life on surfaces. Emerg. Infect. Dis. 8, 881–890. doi: 10.3201/eid0809.020063
Dopheide, A., Lear, G., Stott, R., and Lewis, G. (2011). Preferential feeding by the ciliates Chilodonella and Tetrahymena spp. and effects of these protozoa on bacterial biofilm structure and composition. Appl. Environ. Microbiol. 77, 4564–4572. doi: 10.1128/AEM.02421-10
Ekelund, F., and Rønn, R. (1994). Notes on protozoa in agricultural soil with emphasis on heterotrophic flagellates and naked amoebae and their ecology. FEMS Microbiol. Rev. 15, 321–353. doi: 10.1111/j.1574-6976.1994.tb00144.x
Ekelund, F., Rønn, R., and Griffiths, B. S. (2001). Quantitative estimation of flagellate community structure and diversity in soil samples. Protist 152, 301–314. doi: 10.1078/1434-4610-00069
Flemming, H.-C., Wingender, J., Szewzyk, U., Steinberg, P., Rice, S. A., and Kjelleberg, S. (2016). Biofilms: an emergent form of bacterial life. Nat. Rev. Microbiol. 14, 563–575. doi: 10.1038/nrmicro.2016.94
Foissner, W. (1999). Soil protozoa as bioindicators: pros and cons, methods, diversity, representative examples. Agric. Ecosyst. Environ. 74, 95–112. doi: 10.1016/S0167-8809(99)00032-8
Friman, V.-P., and Buckling, A. (2014). Phages can constrain protist predation-driven attenuation of Pseudomonas aeruginosa virulence in multienemy communities. ISME J. 8, 1820–1830. doi: 10.1038/ismej.2014.40
Friman, V.-P., Diggle, S. P., and Buckling, A. (2013). Protist predation can favour cooperation within bacterial species. Biol. Lett. 9:20130548. doi: 10.1098/rsbl.2013.0548
Gittleson, S. M., and Ganapathy, M. (2011). Cell Counting with the Sedgewick-Rafter Chamber and Whipple Micrometer Disc. Available at: http://www.protocol-online.org/prot/Protocols/Cell-Counting-with-the-Sedgewick-Rafter-Chamber-and-Whipple-Micrometer-Disc-4315.html [accessed November 15, 2016].
Hansen, L. B. S., Ren, D., Burmølle, M., and Sørensen, S. J. (2017). Distinct gene expression profile of Xanthomonas retroflexus engaged in synergistic multispecies biofilm formation. ISME J. 11, 300–303. doi: 10.1038/ismej.2016.107
Huws, S. A., McBain, A. J., and Gilbert, P. (2005). Protozoan grazing and its impact upon population dynamics in biofilm communities. J. Appl. Microbiol. 98, 238–244. doi: 10.1111/j.1365-2672.2004.02449.x
Iriberri, J., Ayo, B., Santamaria, E., Barcina, I., and Egea, L. (1995). Influence of bacterial density and water temperature on the grazing activity of two freshwater ciliates. Freshw. Biol. 33, 223–231. doi: 10.1111/j.1365-2427.1995.tb01163.x
Jackson, S., and Jones, E. (1991). Interactions within biofilms: the disruption of biofilm structure by protozoa. Kieler Meeresforsch. Sonderh. 8, 264–268. doi: 10.1016/j.ijpara.2016.11.010
Jezbera, J., Horňák, K., and Šimek, K. (2005). Food selection by bacterivorous protists: insight from the analysis of the food vacuole content by means of fluorescence in situ hybridization. FEMS Microbiol. Ecol. 52, 351–363. doi: 10.1016/j.femsec.2004.12.001
Joubert, L.-M., Wolfaardt, G. M., and Botha, A. (2006). Microbial exopolymers link predator and prey in a model yeast biofilm system. Microb. Ecol. 52, 187–197. doi: 10.1007/s00248-006-9063-7
Jürgens, K., and Güde, H. (1994). The potential importance of grazing-resistant bacteria in planktonic systems. Mar. Ecol. Prog. Ser. 112, 169–188. doi: 10.3354/meps112169
Jürgens, K., and Matz, C. (2002). Predation as a shaping force for the phenotypic and genotypic composition of planktonic bacteria. Antonie Van Leeuwenhoek 81, 413–434. doi: 10.1023/A:1020505204959
Kaminskaya, A., Pushkareva, V., Moisenovich, M., Stepanova, T., Volkova, N., Romanova, J., et al. (2007). Stimulation of biofilm formation by insertion of Tetrahymena pyriformis wells within Burkholderia cenocepacia biofilms. Mol. Genet. Microbiol. Virol. 22, 186–194. doi: 10.3103/S0891416807040088
Lambrecht, E., Baré, J., Chavatte, N., Bert, W., Sabbe, K., and Houf, K. (2015). Protozoan cysts act as a survival niche and protective shelter for foodborne pathogenic bacteria. Appl. Environ. Microbiol. 81, 5604–5612. doi: 10.1128/AEM.01031-15
Lee, K. W. K., Periasamy, S., Mukherjee, M., Xie, C., Kjelleberg, S., and Rice, S. A. (2014). Biofilm development and enhanced stress resistance of a model, mixed-species community biofilm. ISME J. 8, 894–907. doi: 10.1038/ismej.2013.194
Liu, W., Russel, J., Røder, H. L., Madsen, J. S., Burmølle, M., and Sørensen, S. J. (2017). Low-abundant species facilitates specific spatial organization that promotes multispecies biofilm formation. Environ. Microbiol. 19, 2893–2905. doi: 10.1111/1462-2920.13816
Logares, R., Audic, S. S., Santini, S. S., Pernice, M. C., de Vargas, C., and Massana, R. (2012). Diversity patterns and activity of uncultured marine heterotrophic flagellates unveiled with pyrosequencing. ISME J. 6, 1823–1833. doi: 10.1038/ismej.2012.36
Loreau, M., and Hector, A. (2001). Partitioning selection and complementarity in biodiversity experiments. Nature 412, 72–76. doi: 10.1038/35083573
Madsen, J. S., Røder, H. L., Russel, J., Sørensen, H., Burmølle, M., and Sørensen, S. J. (2016). Coexistence facilitates interspecific biofilm formation in complex microbial communities. Environ. Microbiol. 18, 2565–2574. doi: 10.1111/1462-2920.13335
Matz, C. (2011). “Competition, communication, cooperation: molecular crosstalk in multi-species biofilms,” in Biofilm Highlights, eds H.-C. Flemming, J. Wingender, and U. Szewzyk (Berlin: Springer), 29–40.
Matz, C., Bergfeld, T., Rice, S. A., and Kjelleberg, S. (2004). Microcolonies, quorum sensing and cytotoxicity determine the survival of Pseudomonas aeruginosa biofilms exposed to protozoan grazing. Environ. Microbiol. 6, 218–226. doi: 10.1111/j.1462-2920.2004.00556.x
Matz, C., and Jürgens, K. (2005). High motility reduces grazing mortality of planktonic bacteria. Appl. Environ. Microbiol. 71, 921–929. doi: 10.1128/AEM.71.2.921-929.2005
Matz, C., and Kjelleberg, S. (2005). Off the hook - How bacteria survive protozoan grazing. Trends Microbiol. 13, 302–307. doi: 10.1016/j.tim.2005.05.009
Matz, C., McDougald, D., Moreno, A. M., Yung, P. Y., Yildiz, F. H., and Kjelleberg, S. (2005). Biofilm formation and phenotypic variation enhance predation-driven persistence of Vibrio cholerae. Proc. Natl. Acad. Sci. U.S.A. 102, 16819–16824. doi: 10.1073/pnas.0505350102
Mielich-Süss, B., and Lopez, D. (2015). Molecular mechanisms involved in Bacillus subtilis biofilm formation. Environ. Microbiol. 17, 555–565. doi: 10.1111/1462-2920.12527
Niu, C., and Gilbert, E. S. (2004). Colorimetric method for identifying plant essential oil components that affect biofilm formation and structure. Appl. Environ. Microbiol. 70, 6951–6956. doi: 10.1128/AEM.70.12.6951-6956.2004
Paisie, T. K., Miller, T. E., and Mason, O. U. (2014). Effects of a ciliate protozoa predator on microbial communities in pitcher plant Sarracenia purpurea leaves. PLOS ONE 9:e113384. doi: 10.1371/journal.pone.0113384
Parry, J. D. (2004). Protozoan grazing of freshwater biofilms. Adv. Appl. Microbiol. 54, 167–196. doi: 10.1016/S0065-2164(04)54007-8
Pernthaler, J. (2005). Predation on prokaryotes in the water column and its ecological implications. Nat. Rev. Microbiol. 3, 537–546. doi: 10.1038/nrmicro1180
Petropoulos, P., and Gilbride, K. A. (2005). Nitrification in activated sludge batch reactors is linked to protozoan grazing of the bacterial population. Can. J. Microbiol. 51, 791–799. doi: 10.1139/w05-069
Pfister, G., Auer, B., and Arndt, H. (2002). Pelagic ciliates (Protozoa, Ciliophora) of different brackish and freshwater lakes—a community analysis at the species level. Limnologica 32, 147–168. doi: 10.1016/S0075-9511(02)80005-6
Ren, D., Madsen, J. S., de la Cruz-Perera, C. I., Bergmark, L., Sørensen, S. J., and Burmølle, M. (2014). High-throughput screening of multispecies biofilm formation and quantitative PCR-based assessment of individual species proportions, useful for exploring interspecific bacterial interactions. Microb. Ecol. 68, 146–154. doi: 10.1007/s00248-013-0315-z
Ren, D., Madsen, J. S., Sørensen, S. J., and Burmølle, M. (2015). High prevalence of biofilm synergy among bacterial soil isolates in cocultures indicates bacterial interspecific cooperation. ISME J. 9, 81–89. doi: 10.1038/ismej.2014.96
Rivera, F., Ramirez, E., Bonilla, P., Calderon, A., Gallegos, E., Rodriguez, S., et al. (1993). Pathogenic and free-living amoebae isolated from swimming pools and physiotherapy tubs in Mexico. Environ. Res. 62, 43–52. doi: 10.1006/enrs.1993.1087
Roberts, E. C., Legrand, C., Steinke, M., and Wootton, E. C. (2011). Mechanisms underlying chemical interactions between predatory planktonic protists and their prey. J. Plankton Res. 33, 833–841. doi: 10.1093/plankt/fbr005
Rogerson, A., and Laybourn-Parry, J. (1992). The abundance of marine amoebae in the water column of the Clyde estuary. Estuar. Coast. Shelf Sci. 34, 187–196. doi: 10.1016/S0272-7714(05)80104-0
Rønn, R., McCaig, A. E., Griffiths, B. S., and Prosser, J. I. (2002). Impact of protozoan grazing on bacterial community structure in soil microcosms. Appl. Environ. Microbiol. 68, 6094–6105. doi: 10.1128/AEM.68.12.6094-6105.2002
Rowe, M. T., and Grant, I. R. (2006). Mycobacterium avium ssp. paratuberculosis and its potential survival tactics. Lett. Appl. Microbiol. 42, 305–311. doi: 10.1111/j.1472-765X.2006.01873.x
Rychert, K., and Neu, T. R. (2010). Protozoan impact on bacterial biofilm formation. Biol. Lett. 47, 3–10. doi: 10.2478/v10120-009-0017-x
Scherwass, A., Erken, M., and Arndt, H. (2016). “Grazing effects of ciliates on microcolony formation in bacterial biofilms,” in Microbial Biofilms - Importance and Applications, eds D. Dhanasekaran and N. Thajuddin (Rijeka: InTech).
Seiler, C., van Velzen, E., Neu, T. R., Gaedke, U., Berendonk, T. U., and Weitere, M. (2017). Grazing resistance of bacterial biofilms: a matter of predators’ feeding trait. FEMS Microbiol. Ecol. 93:fix112. doi: 10.1093/femsec/fix112
Sibbald, M. J., and Albright, L. J. (1988). Aggregated and free bacteria as food sources for heterotrophic microflagellates. Appl. Environ. Microbiol. 54, 613–616.
Singh, T., and Coogan, M. M. (2005). Isolation of pathogenic Legionella species and legionella-laden amoebae in dental unit waterlines. J. Hosp. Infect. 61, 257–262. doi: 10.1016/j.jhin.2005.05.001
Taylor, M., Ross, K., and Bentham, R. (2009). Legionella, protozoa, and biofilms: interactions within complex microbial systems. Microb. Ecol. 58, 538–547. doi: 10.1007/s00248-009-9514-z
Thomas, J. M., and Ashbolt, N. J. (2011). Do free-living amoebae in treated drinking water systems present an emerging health risk? Environ. Sci. Technol. 45, 860–869. doi: 10.1021/es102876y
Vanelslander, B., De Wever, A., Van Oostende, N., Kaewnuratchadasorn, P., Vanormelingen, P., Hendrickx, F., et al. (2009). Complementarity effects drive positive diversity effects on biomass production in experimental benthic diatom biofilms. J. Ecol. 97, 1075–1082. doi: 10.1111/j.1365-2745.2009.01535.x
Weitere, M., Bergfeld, T., Rice, S. A., Matz, C., and Kjelleberg, S. (2005). Grazing resistance of Pseudomonas aeruginosa biofilms depends on type of protective mechanism, developmental stage and protozoan feeding mode. Environ. Microbiol. 7, 1593–1601. doi: 10.1111/j.1462-2920.2005.00851.x
Wen, Z. T., Yates, D., Ahn, S.-J., and Burne, R. A. (2010). Biofilm formation and virulence expression by Streptococcus mutans are altered when grown in dual-species model. BMC Microbiol. 10:111. doi: 10.1186/1471-2180-10-111
Wey, J., Scherwass, A., Norf, H., Arndt, H., and Weitere, M. (2008). Effects of protozoan grazing within river biofilms under semi-natural conditions. Aquat. Microb. Ecol. 52, 283–296. doi: 10.3354/ame01236
Keywords: synergy, multispecies biofilm, Tetrahymena pyriformis, grazing, species protection
Citation: Raghupathi PK, Liu W, Sabbe K, Houf K, Burmølle M and Sørensen SJ (2018) Synergistic Interactions within a Multispecies Biofilm Enhance Individual Species Protection against Grazing by a Pelagic Protozoan. Front. Microbiol. 8:2649. doi: 10.3389/fmicb.2017.02649
Received: 07 August 2017; Accepted: 19 December 2017;
Published: 09 January 2018.
Edited by:
Frank Schreiber, Bundesanstalt für Materialforschung und -Prüfung (BAM), GermanyReviewed by:
Efstathios D. Giaouris, University of the Aegean, GreeceHans-Peter Grossart, Leibniz-Institut für Gewässerökologie und Binnenfischerei, Germany
Copyright © 2018 Raghupathi, Liu, Sabbe, Houf, Burmølle and Sørensen. This is an open-access article distributed under the terms of the Creative Commons Attribution License (CC BY). The use, distribution or reproduction in other forums is permitted, provided the original author(s) or licensor are credited and that the original publication in this journal is cited, in accordance with accepted academic practice. No use, distribution or reproduction is permitted which does not comply with these terms.
*Correspondence: Søren J. Sørensen, c2pzQGJpby5rdS5kaw==