- 1Division of Abdominal Transplantation, Department of Surgery, Stanford University, Stanford, CA, United States
- 2Division of Stem Cell Transplantation, Department of Pediatrics, Stanford University, Stanford, CA, United States
- 3Department of Pathology, Stanford University, Stanford, CA, United States
- 4Division of Cardiology, Department of Pediatrics, Stanford University, Stanford, CA, United States
- 5Stanford Immunology, Stanford University School of Medicine, Stanford, CA, United States
Epstein–Barr virus (EBV) is the etiological agent of acute infectious mononucleosis (IM). Since acute IM is a self-resolving disease with most patients regaining health in 1–3 weeks there have been few studies examining molecular signatures in early acute stages of the disease. MicroRNAs (miRNAs) have been shown, however, to influence immune cell function and consequently the generation of antibody responses in IM. In this study, we performed a comprehensive analysis of differentially expressed miRNAs in early stage uncomplicated acute IM. miRNAs were profiled from patient peripheral blood obtained at the time of IM diagnosis and at subsequent time points, and pathway analysis performed to identify important immune and cell signaling pathways. We identified 215 differentially regulated miRNAs at the most acute stage of infection when the patients initially sought medical help. The number of differentially expressed miRNAs decreased to 148 and 68 at 1 and 2 months post-primary infection, with no significantly changed miRNAs identified at 7 months post-infection. Interferon signaling, T and B cell signaling and antigen presentation were the top pathways influenced by the miRNAs associated with IM. Thus, a dynamic and regulated expression profile of miRNA accompanies the early acute immune response, and resolution of infection, in IM.
Introduction
Epstein–Barr virus is a gamma-herpes family virus that infects over 95% of humans globally and is the etiological agent of many acute and chronic infections as well as human malignancies (Khan and Hashim, 2014). While EBV infection is widespread, the outcome of infection can depend upon the age of the host. Pediatric cases are mainly asymptomatic and the infection subsides due to a vigorous host T cell response with the virus subsequently transitioning to latency in a subset of memory B cells (Jayasooriya et al., 2015; Gantt et al., 2016). However, in adolescents, primary infection can manifest as glandular fever, lymphadenopathy, and a sore throat, termed infectious mononucleosis (IM) (Balfour et al., 2013). IM is associated with a transient proliferation of EBV-infected B cells followed by a considerable expansion of EBV-specific T cells (Sixbey et al., 1984; Kurth et al., 2000; Chaganti et al., 2009; Hadinoto et al., 2009; Balfour et al., 2013). IM is typically self-limiting and resolves over a period of weeks to months with the virus persisting into a latent phase in infected B cells (Hochberg et al., 2004).
MicroRNAs are small 18–25 nt long single-stranded RNA molecules which regulate gene expression at the post-transcriptional level. miRNA target specific mRNAs by complementary base-pairing, leading to cleavage or repression of translation, and can act as major regulators of key cellular processes. We (Harris et al., 2010) and others (Forte et al., 2012; Mansouri et al., 2014) have shown that EBV infection can alter the expression of host miRNAs and thus modulate various arms of the cellular machinery including cell differentiation and death, proliferation, and the immune response. Along these lines, miR-194 is suppressed by EBV and participates in regulation of IL-10 expression in EBV lymphomas (Harris et al., 2010) while two miRNA families, the let-7 family and the miR-200 family, as well as miR-143-3p, act as tumor-suppressor miRNAs and are significantly downregulated in EBV-infected gastric carcinoma cells (Marquitz et al., 2014). Other studies have found that miR-155 and miR-21 were induced in B cells by EBV infection and potentially play a role in viral tumorigenesis (Linnstaedt et al., 2010; Rosato et al., 2012; Anastasiadou et al., 2015). Furthermore, p53-targeted tumor suppressor, miR-34a, is strongly induced by EBV infection in many EBV and Kaposi’s sarcoma-associated herpesvirus-infected lymphoma cell lines and plays a role in B cell transformation (Forte et al., 2012). In addition, miRNAs have been identified that play a critical role in the T cell response to pathogens. For example, miR-155 is essential for the CD8+ effector T cell response to mCMV (Dudda et al., 2013).
In addition to host miRNAs, EBV-expressed miRNAs have also been shown to modulate the function of T and B cells during an immune response. In a study by Albanese et al. (2016), EBV-encoded miRNAs were found to inhibit CD8+ T cell-mediated viral surveillance at multiple levels. The viral miRNAs downregulate TAP-complex and HLA allotype that present TAP-dependent epitopes, reduce IL-12 secretion by infected B cells to diminish EBV-specific CD8 T cells and repress EBNA1, a viral protein responsible for latency (Albanese et al., 2016). EBV encoded miRNAs miR-BART1, miR-BART2, and miR-BHRF1-2 have also been shown to target the host adaptive immune response by repressing IL-12 secretion resulting in reduced differentiation of CD4+ T cells into the Th1 subset and inhibiting lysosomal enzymes involved in MHC class-II peptide processing (Tagawa et al., 2016), while miR-BART16 suppresses Type I IFN signaling (Hooykaas et al., 2017). In the current study, we report the differential expression of host and viral miRNAs in acute IM caused by primary EBV infection. Our findings elucidate the miRNA signature associated with acute IM and demonstrate the dynamic features of this response with the miRNA expression profile reverting to that of healthy individuals within a few months of IM diagnosis in subjects without complications.
Materials and Methods
Patient Samples
Peripheral blood samples were obtained from symptomatic patients (aged 18–24 years) who visited Vaden Student Health Center at Stanford University and were diagnosed with clinical IM. Samples were obtained upon diagnosis (time point 0) and then at, 1, 2, and 7 months. Blood samples were processed using Ficoll-Paque PLUS (Amersham Pharmacia Biotech, Piscataway, NJ, United States) then PBMC frozen and maintained in liquid nitrogen until use. PBMCs were isolated from blood derived from healthy age-matched individuals. This study was carried out in accordance with the recommendations of Institutional Review Board at Stanford University with written informed consent from all subjects. All subjects gave written informed consent in accordance with the Declaration of Helsinki.
RNA Isolation and miRNA Microarray
Patient (n = 16) and healthy control (n = 3) PBMCs were suspended and lysed in TRIzol Reagent (Life Technologies, Foster City, CA, United States) for isolation of total RNA. Glycogen (20 μg) was used for RNA precipitation. Isolated RNA was DNase treated (Qiagen) and clean-up was done on RNeasy spin columns (Qiagen) using a modified protocol which replaced RW1 buffer with RWT buffer for washing. RNA was suspended in RNase free water and stored at -80°C. RNA quantification was done using Nanodrop 2000 Spectrophotometer (Thermo Fisher scientific, Wilmington, DE, United States). Approximately 200 ng RNA was used for microarray analysis using Affymetrix GeneChip miRNA 4.0 arrays (Affymetrix, Santa Clara, CA, United States) through the PAN (Protein and Nucleic acid) facility at Stanford University. Briefly, 130 ng total RNA was assessed for quality using Bioanalyzer 2100 (Agilent), followed by Poly-A tailing and labeling with biotin using a Affymetrix FlashTag Biotin HSR RNA Labeling kit (Affymetrix, Santa Clara, CA, United States) according to the manufacturer’s protocol. The labeled targets are hybridized to the GeneChip® miRNA 4.0 arrays per standard miRNA protocol, which includes 16 h (overnight) hybridization at 48°C at 60 RPM in the Affymetrix GeneChip Hybridization oven 645. The arrays were then washed and stained in the Affymetrix GeneChip Fluidics Station 450. The arrays were scanned using the Affymetrix GeneChip Scanner 3000 7G. The GeneChip® miRNA 4.0 arrays contain 30,424 total mature miRNA probe sets including 2578 mature human miRNAs, 2025 pre-miRNA human probes, 1996 Human snoRNA and scaRNA probe sets and miRNAs of 202 other organisms.
Data Analysis
Raw microarray data were statistically analyzed using Partek Genomics Suite software (Partek Inc.). Normalization was done suing Robust Multi-array Average (RMA) and log2 transformation of data was performed, followed by one-way ANOVA statistical testing to identify significantly differentially expressed miRNA between different groups (P ≤ 0.05). After running ANOVA, the software performs multi-testing correction using Benjamini–Hochberg Step-Up FDR-controlling procedure for all the expressed miRNAs (default FDR Alpha level 0.05). Venn diagram depicting intersection of differentially expressed miRNAs and Unsupervised Hierarchical Clustering analysis was also performed. Raw Fold change and adjusted p-value data was exported to dot excel file and data selection and graphic visualization was done using Power BI software (Microsoft Inc.). Differentially expressed EBV miRNAs were also identified using the same miRNA microarray dataset using the miRNA filter as “EBV,” mRNA expression data from a publicly available GEO dataset (GSE45924) was used to perform miRNA–mRNA integrated analysis. Briefly, SOFT formatted family file (GSE45924-GPL6883_series_matrix.piv.txt.fmt) were downloaded from the GEO Database and imported into Partek Genomics Suite for mRNA “Gene Expression” workflow. Illumina HumanRef-8 v3.0 expression beadchip annotation files were uploaded to the Partek Software to obtain Fold Change Values for mRNA differential expression between comparison groups of Acute IM subjects (PBMC) and control subjects (PBMC). Pre-normalized data was log2 transformed, followed by one-way ANOVA statistical testing to identify significantly differentially expressed mRNAs and multiple-testing correction using Benjamini–Hochberg Step-Up FDR-controlling procedure (default FDR Alpha level 0.05). Fold increases of >2, and fold decreases of >2 were considered significant. The differentially expressed mRNA data was exported into a text file and converted into a suitable format for upload to IPA for further analysis.
Functional and Pathway Enrichment Analysis of Target Genes Controlled by Differentially Expressed miRNAs
Pathway and molecular function enrichment analysis was performed using IPA software (Qiagen, Redwood city, CA, United States). A list of all twofold differentially expressed miRNAs obtained from Partek Genomics Suite software analysis was uploaded into IPA for analysis. miRNA target enrichment was performed using the “miRNA Target Filter” function, generating a list of 181 miRNAs with available targeting information. An mRNA gene expression dataset (GSE45924) obtained from GEO database was added to the “miRNA Target Filter” setting in IPA and “Expression pairing” was performed. An additional filter applied to this list was “Applied filters: confidence = Experimentally Observed OR High (predicted).” Further “Core Analysis” was performed on the target mRNAs based on different experimental time points to determine the affected networks and canonical pathways. Throughout the analysis, a p-value cutoff of 0.05 was applied. The HumanRef-8 v3.0 reference gene list was used to set the population of genes to consider for p-value calculations while performing Core Analysis.
Results
Sample QC and Principal Component Analysis
As a means of quality control to determine the biological separation of the groups based on probe intensities, principal component analysis (PCA) was performed using Partek Genomics software. Two separate groups of samples from IM patients at 0 month (at the time of diagnosis) and healthy controls are clearly observed (Figure 1). The remaining samples from 1, 2, and 7 months are loosely segregated into groups and fall between these two groups on PC1 with the samples obtained at 7 months grouping closely with those from healthy controls. These findings indicate that the differentially expressed miRNAs are maximal at the time of IM diagnosis and return to the healthy control pattern in subsequent months.
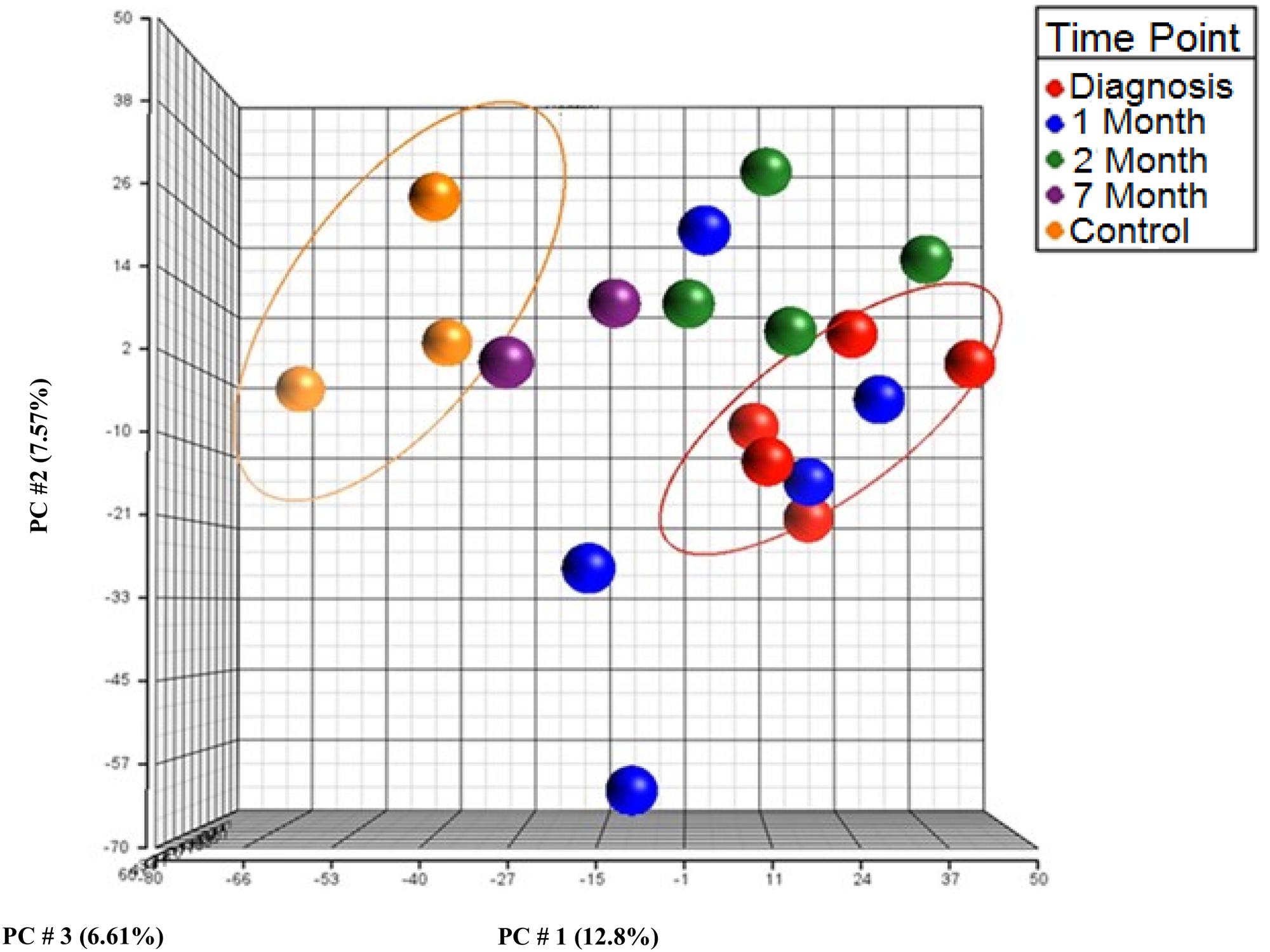
FIGURE 1. Principal component analysis (PCA) on miRNA expression data from human peripheral blood mononuclear cells (PBMCs) from acute infectious mononucleosis (IM) patients and healthy controls using the first three components. Red, at diagnosis; Blue, 1 month; Green, 2 months; Purple, 7 months; Orange, Healthy control.
Differentially Expressed miRNAs
The differential expression of miRNAs were compared between patients at the time of IM diagnosis (0 month), and at 1 and 2 months after diagnosis, compared to age-matched healthy controls (Figure 2A). We identified 215 differentially regulated miRNAs at the most acute stage of infection (diagnosis, 0 month). The number of differentially expressed miRNAs decreased to 148 and 68 during the course of 1 and 2 months post-primary infection, with no significantly changed miRNAs identified at 7 months post-infection (data not shown). The 27 top differentially expressed miRNAs at diagnosis, and 1 and 2 months after diagnosis, show that most of the differentially expressed miRNAs are increased (25 increased, 2 decreased) (Figure 2B).
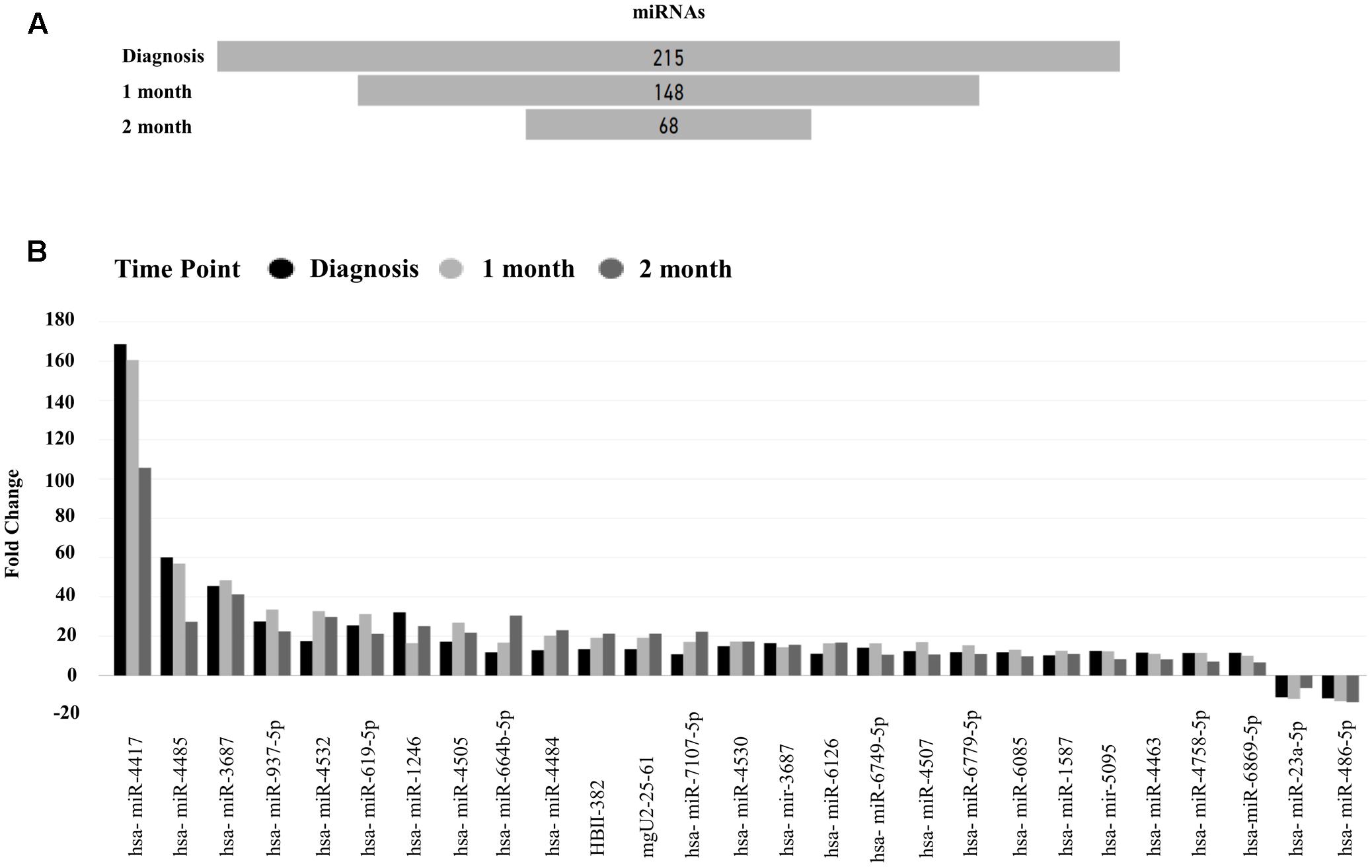
FIGURE 2. Top differentially expressed microRNAs (miRNAs). (A) Differentially expressed miRNAs in IM Patients at Time 0, 1, and 2 months compared to healthy controls, (B) Top differentially expressed miRNAs observed at all three time points, at diagnosis, 1, and 2 months vs. healthy controls [false discovery rate (FDR) 0.05, Absolute Fold change >10].
Unsupervised hierarchical clustering depicted as a heatmap shows the relative intensity for the 215, 148, and 68 differentially expressed miRNAs at diagnosis (Figure 3), 1 month (Supplementary Figure S1) and 2 months (Supplementary Figure S2) as compared to healthy controls, respectively. A fold change of two and FDR adjusted p-value (Benjamini–Hochberg method for multiple corrections) of 0.05 was considered significant in Partek Genomics Suite Software, with black depicting decreased expression levels and blue depicting upregulation. Furthermore, the differentially expressed miRNAs demonstrate a decreasing absolute fold change over time, with the highest fold change generally observed at the time of diagnosis (Figure 4). These 41 miRNAs exhibit a dynamic differential expression pattern that trends toward healthy control levels at 2 months post IM diagnosis. Two miRNAs that are highly upregulated, miR-4417 and miR-4485 (data not shown), exhibit a similar trend of decreasing differential expression from diagnosis to 2 months. A compilation of all statistically significant differentially expressed miRNAs at diagnosis and one, and 2 months versus healthy controls is included in Supplementary Table S1. Supplementary Table S2 provides a list of all differentially expressed miRNAs. A Venn diagram depicts the number of miRNAs in common at IM diagnosis and at 1 and 2 months (Figure 5). The intersection shows that 56 miRNAs are differentially expressed at the three time points, thus providing important information regarding possible disease specific signatures. Interestingly, miRNAs uniquely expressed at diagnosis, 1 and 2 months after diagnosis also decrease in number during the course of the infection toward recovery (79 at diagnosis, 15 at 1 month and 9 at 2 months).
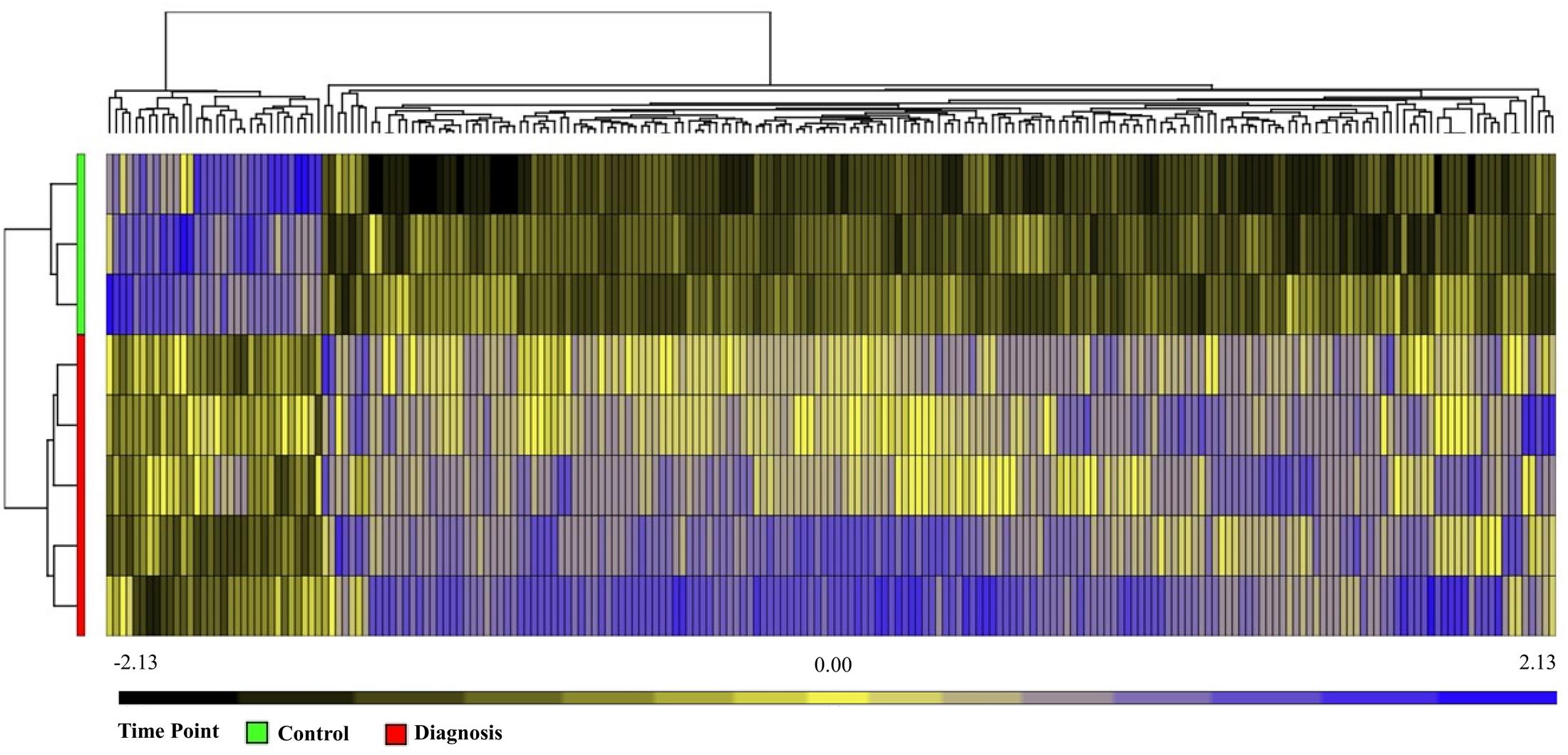
FIGURE 3. Unsupervised Hierarchical Clustering Heatmap showing relative intensity for 215 miRNAs in Patients at diagnosis versus healthy controls. Analysis using Partek Genomics suite, step up FDR 0.05. Black shows downregulation and blue shows upregulation. Healthy controls (green), IM at diagnosis (red).
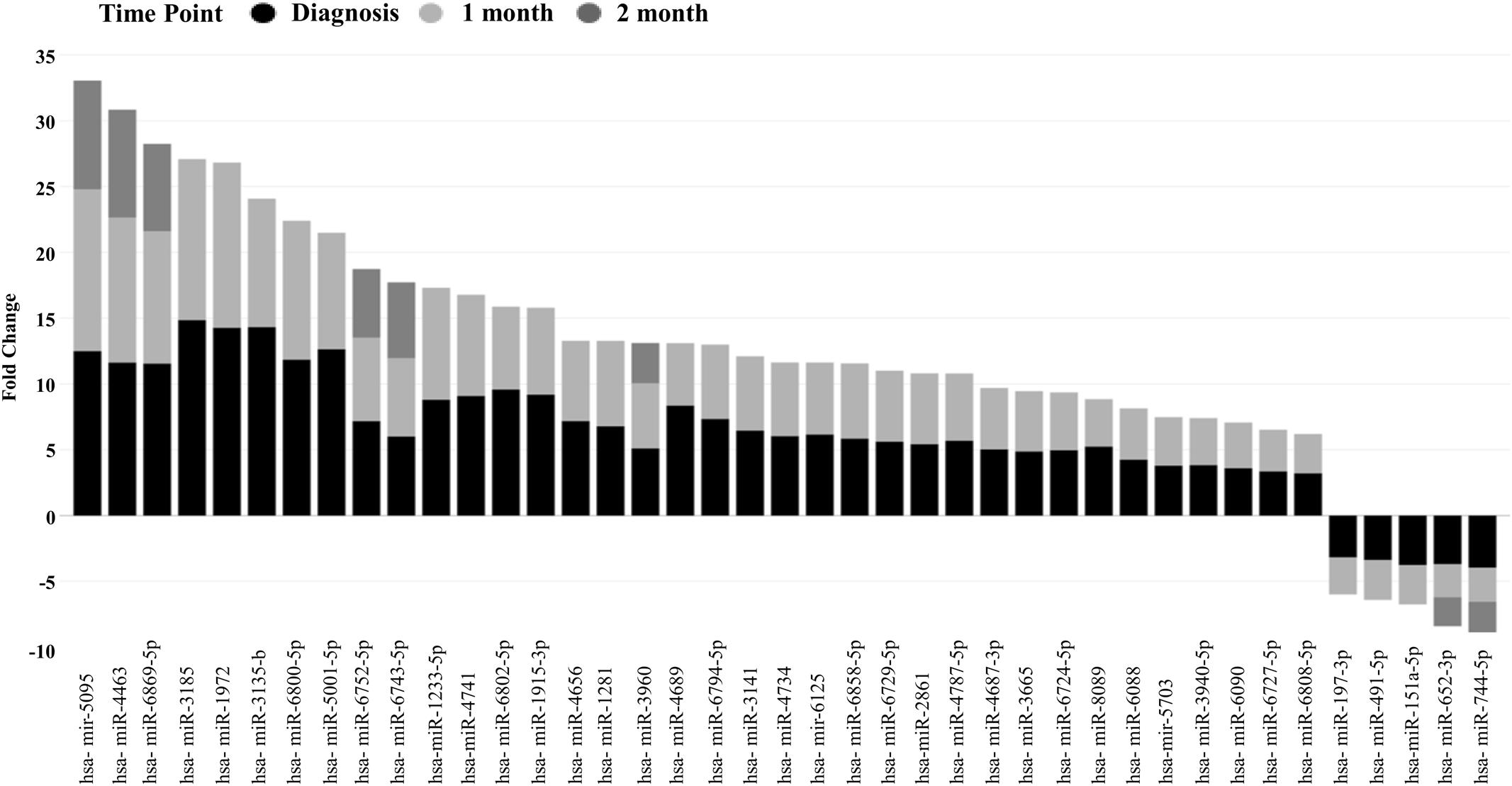
FIGURE 4. Differentially expressed miRNAs at three time points, at Diagnosis, 1 and 2 months showing a trend toward decreasing absolute fold change (FDR 0.05).
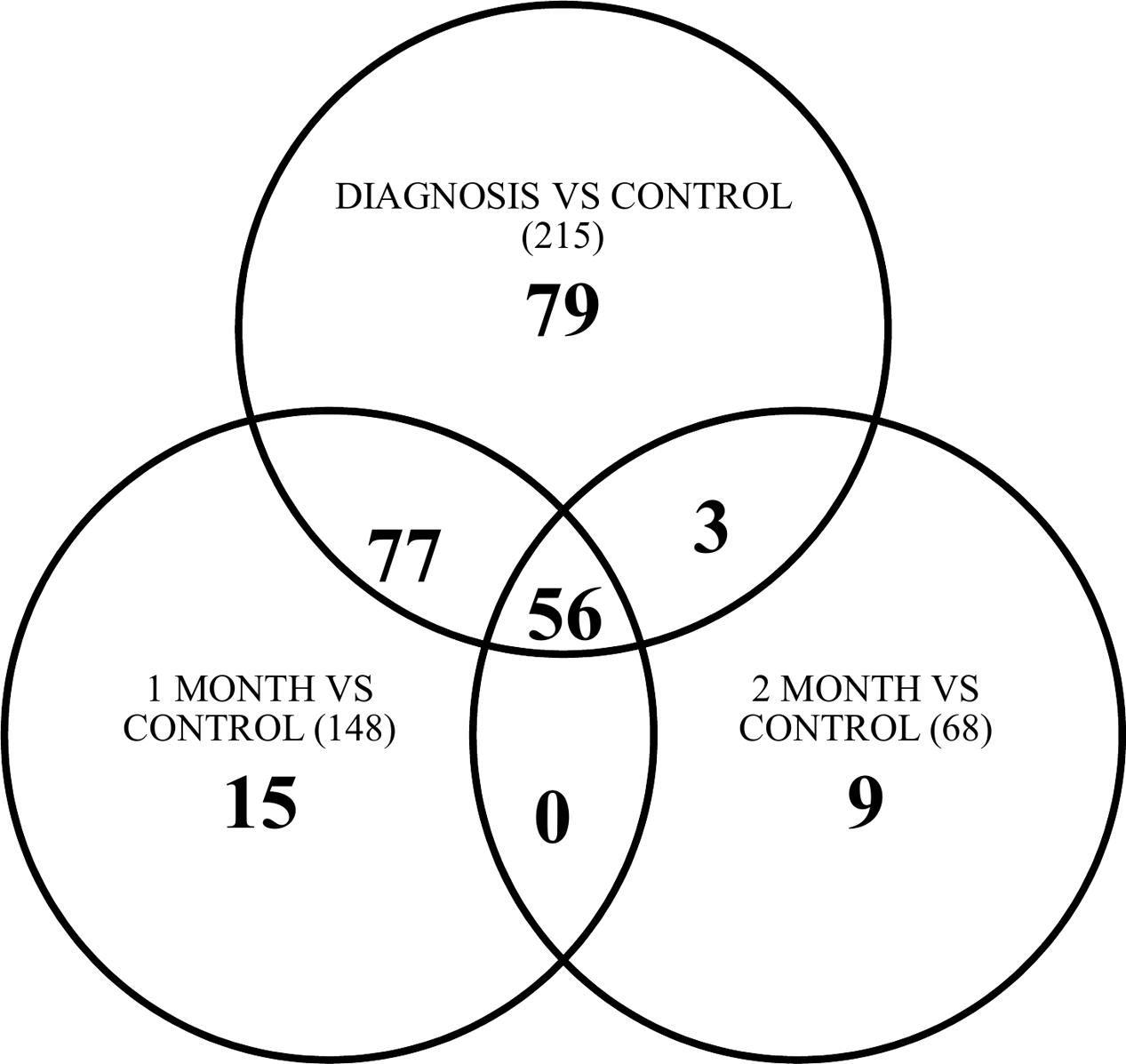
FIGURE 5. Venn diagram depicting number of miRNAs common between point of diagnosis, 1 and 2 months versus healthy control. Number in brackets () is the total number of differentially expressed miRNAs at that time point vs. control.
Pathways Altered by IM
Expression pairing of miRNA data, from our study and gene expression data from GEO set GSE45924 returned 46 miRNAs targeting 17 mRNAs (Figure 6A). Comparative analysis of mRNA targets highlighted the most canonical pathways as well as disease and biological functions (Figures 6B,C). Furthermore, functional pathway analysis in IPA using the list of differentially expressed miRNAs at 0 month suggests the most impacted networks include antigen presentation, infectious disease, humoral immune response, protein synthesis, cell cycle, and cell death mechanisms. Networks have been shown in addition to their scores and number of mRNA targets (including particular molecules that pass our set filter criterion) (Table 1). Further analysis in IPA for top canonical pathways targeted by our list of differentially expressed miRNAs at IM diagnosis and based on the Illumina HumanRef-8 v3.0 gene superset shows candidates including interferon signaling, altered T and B cell signaling, primary immunodeficiency signaling, antigen presentation and communication between innate and adaptive immune cells as the major affected canonical pathways (Supplementary Figure S3).
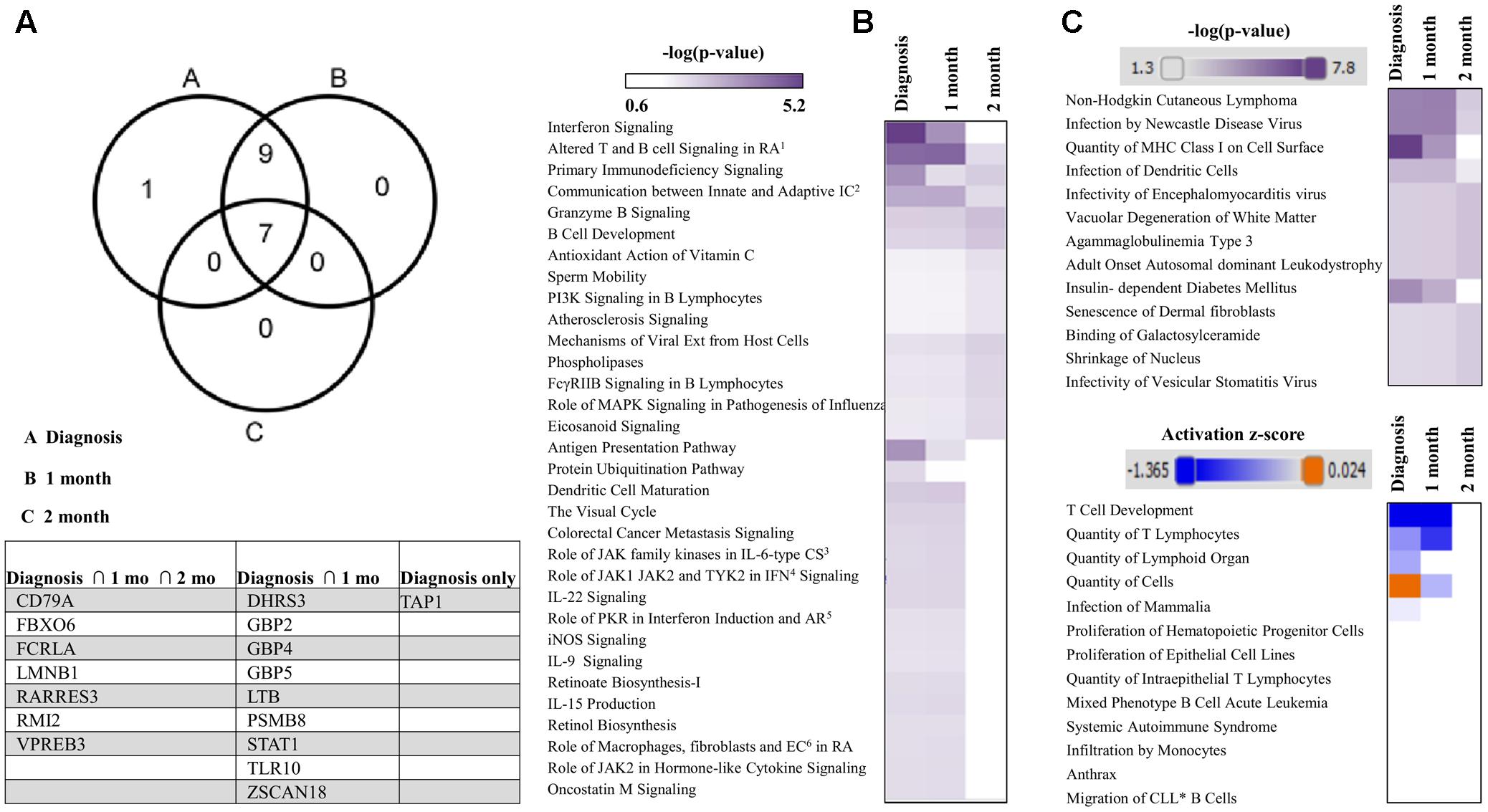
FIGURE 6. mRNA targets and associated pathways of the differentially expressed miRNAs. (A) Venn diagram and table comparison of mRNA targets of differentially expressed miRNAs at Diagnosis, 1 and 2 months. (B) Comparison of canonical pathways, (C) Comparison of Diseases and Bio Functions; mRNA targets were obtained from miRNA–mRNA expression pairing of differentially expressed miRNAs and GEO dataset GSM1119621–GSM1119636 in ingenuity pathway analysis (IPA).
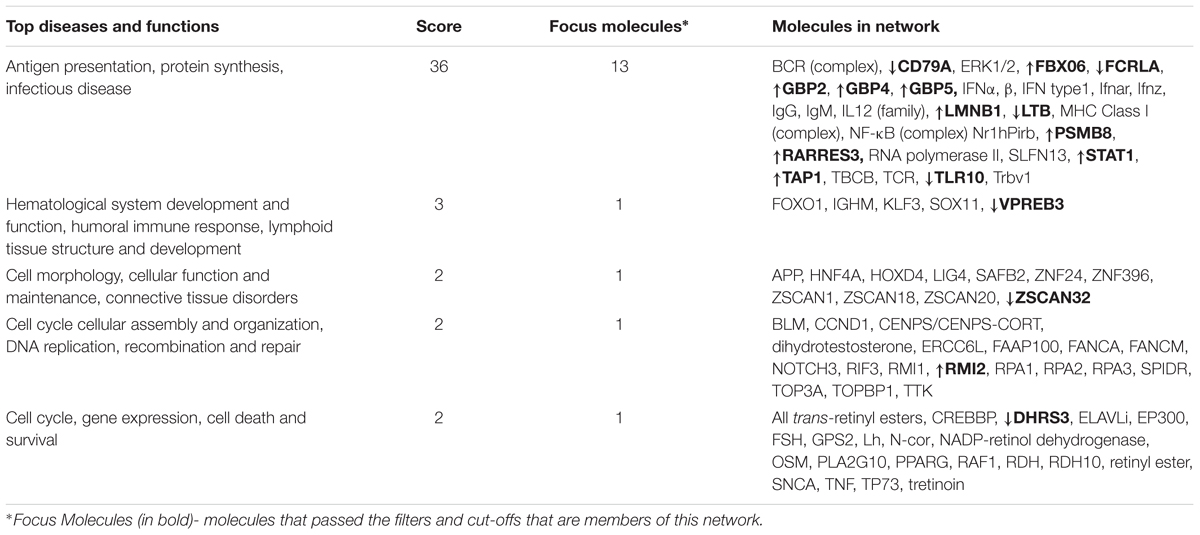
TABLE 1. List of top five networks with their respective scores obtained from ingenuity pathway analysis (IPA): Data are indicative of networks regulated by mRNA targets of microRNAs (miRNAs) differentially expressed at the time of infectious mononucleosis (IM) diagnosis.
Differentially Expressed EBV miRNAs
Interestingly, when the EBV miRNAs are analyzed using specific filtering criteria in Partek, only ebv-miR-BART-16 and ebv-miR-BART5-3p are uniquely upregulated at IM diagnosis and at 1 and 2 months as compared to healthy controls (ANOVA 0.05, unadjusted p-value) (Table 2). It is notable that no differential expression of any EBV miRNAs is observed at 7 months post-infection, which is expected due to resolution of the symptoms in the acute phase and establishment of EBV latency. Thus, of the more than 40 known EBV miRNAs only two, miR-BART5-3p and miR-BART-16 are expressed during acute IM (Skinner et al., 2017).

TABLE 2. Differentially expressed EBV miRNAs in patients at diagnosis, 1 and 2 months versus healthy controls, ANOVA 0.05, unadjusted p-value.
Discussion
This study provides the first miRNA expression profile of sequential, peripheral blood samples from college-age patients with acute IM. Our results clearly indicate that primary EBV disease is accompanied by marked expression changes of multiple host and viral miRNA, and that the number of differentially expressed miRNAs decreases substantially after the initial diagnosis. It is noteworthy that there were no differentially expressed miRNAs observed at 7 months as compared to healthy controls, indicating that there is no identifiable miRNA signature that can distinguish between normal college students and those that have resolved IM. Since none of the patients studied had any complications after IM, it remains possible that expression of the differentially expressed host miRNAs would remain dysregulated if IM were not completely resolved.
We determined that 43 of the host miRNAs, including miR-197-3p, miR-491-5p, miR-151a-5p, miR-652-3p, and miR-744-5p, decreased from the levels found at diagnosis over the subsequent 2 months. While the specific function of these miRNA in IM is unknown, other reports have linked these miRNA to disease processes. Recently, miR-197 was found to act synergistically with EBV-BART6-3p to reduce the expression of IL-6R, thereby compromising host immune defense in EBV-positive Burkitt Lymphoma (Zhang et al., 2017). miR-625-3p has been shown to be a potential biomarker for a number of cancerous conditions including malignant pleural mesothelioma (Kirschner et al., 2012). miR-625-3p targets p38 MAPK activator MAP2K6 and induces oxaliplatin resistance by abrogating MAP2K6-p38-regulated apoptosis and cell cycle control networks (Rasmussen et al., 2016). A recent study also shows that miR-625-3p is upregulated in CD8+ T cells during early immune reconstitution following allogeneic stem cell transplantation (Verma et al., 2017). An interesting study with highly virulent H5N2 strain of mouse-adapted avian influenza virus found miR-151a-p as one of the significantly upregulated miRNAs, whose inhibition resulted in a 70% reduction in mortality of inoculated mice (Choi et al., 2014).
The majority of studies to date examining the impact of EBV infection or pathogenesis on miRNA expression have focused on the miRNA profile within the virally infected cell and the implications for tumorigenesis. In contrast, we analyzed the impact of EBV infection on peripheral lymphoid cells that typically participate in the immune response to EBV. A single previous study did examine the cellular miRNAs from pre-adolescent children with IM in China over the first 14 days after primary infection (Gao et al., 2015). They determined the host miRNA expression in B cells and CD8+ T cells and EBV miRNAs in plasma and B cells. Interestingly, some miRNAs such as miR-155 and miR-142 were increased in B cells and decreased in CD8+ T cells, thus, changes would not be reflected in unfractionated PBMC. Our analysis of miRNAs in PBMC demonstrated a different set of host miRNAs as differentially expressed. These distinct patterns are most likely attributed to different methodologies, since our study took an unbiased approach and performed microarray analysis using Affymetrix GeneChip miRNA 4.0 arrays (over 30,000 mature miRNAs) on unfractionated PBMC, whereas the Gao group specifically examined a small subset of 84 miRNAs in sorted B and T cells via PCR. Further, the ages of the subjects were different and there could be other differences including environmental factors and variant strains of EBV. EBV expresses more miRNAs than other characterized human viruses, with at least 44 EBV miRNAs identified to date (Skinner et al., 2017). We determined that ebv-miR-BART16 and ebv-miR-BART5-3p were increased in PBMC as compared to healthy controls. The specific function of these viral miRNA in IM is unknown, but it is interesting that a recent report indicates that BART16 suppresses IFN signaling (Hooykaas et al., 2017). Notably, the EBV miRNA profile we identified in acute IM is distinct from the EBV miRNA profile identified in plasma samples of patients with chronic active EBV infection (Kawano et al., 2013).
In addition to EBV-induced miRNA regulation in infected B cells, others have focused on understanding the EBV pathogenesis and host responses by transcriptomic (mRNA microarray, Illumina) analysis on PBMCs of human subjects in a prospective study of naturally acquired primary EBV infection (Dunmire et al., 2014). The authors found a distinct gene expression profile in acute but not latent EBV infection. Moreover, upon gene expression profiling in sorted CD8+ T cells, NK cells, monocytes, and B cells, it was found that CD8+ T cells and monocytes showed upregulation of key gene groups (Type I interferon regulated genes (IRGs), type II IRGs, and cell cycle/metabolism genes.) during acute infection. This pathway analysis agrees with the pathways predicted to be altered by our miRNA profile during acute IM.
Conclusion
We have defined the host immune cell and EBV miRNA profile elicited by primary EBV infection during acute IM. This profile is indicative of a response that is accompanied by complete resolution of disease. Thus, it may be useful in predicting which individuals that present with IM will have an uncomplicated course and ultimately may shed insight into the host immune response to acute EBV infection.
Author Contributions
VK designed and performed experiments, analyzed the data, and wrote the manuscript. KW obtained the samples and contributed to development of study design. SB contributed to development of study design and critically revised the manuscript. DB contributed to development of study design. CE contributed to development of study design. OM contributed to study design, provided critical review of the experimental design, wrote, and provided critical review of the manuscript. SK designed the study, analyzed the data, wrote, and critically reviewed the manuscript. All authors reviewed and approved the final manuscript.
Funding
VK was supported by fellowship from the Transplant and Tissue Engineering Center of Excellence at Lucile Packard Children’s Hospital. Funding was also obtained from the Lucile Packard Children’s Hospital Heart Center Research Fund (DB, KW, and SB). OM was partially funded by AI115313, SK was partially funded by AI119686, KW, SB, DB, CE, OM, SK were partially supported by AI104342.
Conflict of Interest Statement
The authors declare that the research was conducted in the absence of any commercial or financial relationships that could be construed as a potential conflict of interest.
Acknowledgment
The authors would like to acknowledge Natalia V. Kosovilka from Stanford PAN facility for help with microarray experiments.
Supplementary Material
The Supplementary Material for this article can be found online at: https://www.frontiersin.org/articles/10.3389/fmicb.2017.02666/full#supplementary-material
Abbreviations
EBV: Epstein–Barr virus; FDR, false discovery rate; IPA, ingenuity pathway analysis; miRNA, microRNA; PBMC, peripheral blood mononuclear cells.
References
Albanese, M., Tagawa, T., Bouvet, M., Maliqi, L., Lutter, D., Hoser, J., et al. (2016). Epstein-Barr virus microRNAs reduce immune surveillance by virus-specific CD8+ T cells. Proc. Natl. Acad. Sci. U.S.A. 113, E6467–E6475. doi: 10.1073/pnas.1605884113
Anastasiadou, E., Garg, N., Bigi, R., Yadav, S., Campese, A. F., Lapenta, C., et al. (2015). Epstein-Barr virus infection induces miR-21 in terminally differentiated malignant B cells. Int. J. Cancer 137, 1491–1497. doi: 10.1002/ijc.29489
Balfour, H. H., Odumade, O. A., Schmeling, D. O., Mullan, B. D., Ed, J. A., Knight, J. A., et al. (2013). Behavioral, virologic, and immunologic factors associated with acquisition and severity of primary Epstein-Barr virus infection in university students. J. Infect. Dis. 207, 80–88. doi: 10.1093/infdis/jis646
Chaganti, S., Heath, E. M., Bergler, W., Kuo, M., Buettner, M., Niedobitek, G., et al. (2009). Epstein-Barr virus colonization of tonsillar and peripheral blood B-cell subsets in primary infection and persistence. Blood 113, 6372–6381. doi: 10.1182/blood-2008-08-175828
Choi, E.-J., Kim, H. B., Baek, Y. H., Kim, E.-H., Pascua, P. N. Q., Park, S.-J., et al. (2014). Differential microRNA expression following infection with a mouse-adapted, highly virulent avian H5N2 virus. BMC Microbiol. 14:252. doi: 10.1186/s12866-014-0252-0
Dudda, J. C., Salaun, B., Ji, Y., Palmer, D. C., Monnot, G. C., Merck, E., et al. (2013). MicroRNA-155 is required for effector CD8+ T cell responses to virus infection and cancer. Immunity 38, 742–753. doi: 10.1016/j.immuni.2012.12.006
Dunmire, S. K., Odumade, O. A., Porter, J. L., Reyes-Genere, J., Schmeling, D. O., Bilgic, H., et al. (2014). Primary EBV infection induces an expression profile distinct from other viruses but similar to hemophagocytic syndromes. PLOS ONE 9:e85422. doi: 10.1371/journal.pone.0085422
Forte, E., Salinas, R. E., Chang, C., Zhou, T., Linnstaedt, S. D., Gottwein, E., et al. (2012). The Epstein-Barr virus (EBV)-induced tumor suppressor microRNA MiR-34a is growth promoting in EBV-infected B cells. J. Virol. 86, 6889–6898. doi: 10.1128/JVI.07056-11
Gantt, S., Orem, J., Krantz, E. M., Morrow, R. A., Selke, S., Huang, M.-L., et al. (2016). Prospective characterization of the risk factors for transmission and symptoms of primary human herpesvirus infections among ugandan infants. J. Infect. Dis. 214, 36–44. doi: 10.1093/infdis/jiw076
Gao, L., Ai, J., Xie, Z., Zhou, C., Liu, C., Zhang, H., et al. (2015). Dynamic expression of viral and cellular microRNAs in infectious mononucleosis caused by primary Epstein-Barr virus infection in children. Virol. J. 12:208. doi: 10.1186/s12985-015-0441-y
Hadinoto, V., Shapiro, M., Sun, C. C., and Thorley-Lawson, D. A. (2009). The dynamics of EBV shedding implicate a central role for epithelial cells in amplifying viral output. PLOS Pathog. 5:e1000496. doi: 10.1371/journal.ppat.1000496
Harris, A., Krams, S. M., and Martinez, O. M. (2010). MicroRNAs as immune regulators: implications for transplantation. Am. J. Transplant. 10, 713–719. doi: 10.1111/j.1600-6143.2010.03032.x
Hochberg, D., Souza, T., Catalina, M., Sullivan, J. L., Luzuriaga, K., and Thorley-Lawson, D. A. (2004). Acute infection with Epstein-Barr virus targets and overwhelms the peripheral memory B-cell compartment with resting, latently infected cells. J. Virol. 78, 5194–5204. doi: 10.1128/JVI.78.10.5194-5204.2004
Hooykaas, M. J. G., van Gent, M., Soppe, J. A., Kruse, E., Boer, I. G. J., van Leenen, D., et al. (2017). EBV MicroRNA BART16 suppresses Type I IFN signaling. J. Immunol. 198, 4062–4073. doi: 10.4049/jimmunol.1501605
Jayasooriya, S., de Silva, T. I., Njie-jobe, J., Sanyang, C., Leese, A. M., Bell, A. I., et al. (2015). Early virological and immunological events in asymptomatic Epstein-Barr virus infection in African children. PLOS Pathog. 11:e1004746. doi: 10.1371/journal.ppat.1004746
Kawano, Y., Iwata, S., Kawada, J.-I., Gotoh, K., Suzuki, M., Torii, Y., et al. (2013). Plasma viral microRNA profiles reveal potential biomarkers for chronic active Epstein-Barr virus infection. J. Infect. Dis. 208, 771–779. doi: 10.1093/infdis/jit222
Khan, G., and Hashim, M. (2014). Global burden of deaths from Epstein-Barr virus attributable malignancies 1990-2010. Infect. Agent. Cancer 9:38. doi: 10.1186/1750-9378-9-38
Kirschner, M. B., Cheng, Y. Y., Badrian, B., Kao, S. C., Creaney, J., Edelman, J. J. B., et al. (2012). Increased circulating miR-625-3p: a potential biomarker for patients with malignant pleural mesothelioma. J. Thorac. Oncol. 7, 1184–1191. doi: 10.1097/JTO.0b013e3182572e83
Kurth, J., Spieker, T., Wustrow, J., Strickler, G. J., Hansmann, L. M., Rajewsky, K., et al. (2000). EBV-infected B cells in infectious mononucleosis: viral strategies for spreading in the B cell compartment and establishing latency. Immunity 13, 485–495. doi: 10.1016/S1074-7613(00)00048-0
Linnstaedt, S. D., Gottwein, E., Skalsky, R. L., Luftig, M. A., and Cullen, B. R. (2010). Virally induced cellular microRNA miR-155 plays a key role in B-cell immortalization by Epstein-Barr virus. J. Virol. 84, 11670–11678. doi: 10.1128/JVI.01248-10
Mansouri, S., Pan, Q., Blencowe, B. J., Claycomb, J. M., and Frappier, L. (2014). Epstein-Barr virus EBNA1 protein regulates viral latency through effects on let-7 microRNA and dicer. J. Virol. 88, 11166–11177. doi: 10.1128/JVI.01785-14
Marquitz, A. R., Mathur, A., Chugh, P. E., Dittmer, D. P., and Raab-Traub, N. (2014). Expression profile of microRNAs in Epstein-Barr virus-infected AGS gastric carcinoma cells. J. Virol. 88, 1389–1393. doi: 10.1128/JVI.02662-13
Rasmussen, M. H., Lyskjær, I., Jersie-Christensen, R. R., Tarpgaard, L. S., Primdal-Bengtson, B., Nielsen, M. M., et al. (2016). miR-625-3p regulates oxaliplatin resistance by targeting MAP2K6-p38 signalling in human colorectal adenocarcinoma cells. Nat. Commun. 7:12436. doi: 10.1038/ncomms12436
Rosato, P., Anastasiadou, E., Garg, N., Lenze, D., Boccellato, F., Vincenti, S., et al. (2012). Differential regulation of miR-21 and miR-146a by Epstein–Barr virus-encoded EBNA2. Leukemia 26, 2343–2352. doi: 10.1038/leu.2012.108
Sixbey, J. W., Nedrud, J. G., Raab-Traub, N., Hanes, R. A., and Pagano, J. S. (1984). Epstein–Barr virus replication in oropharyngeal epithelial cells. N. Engl. J. Med. 310, 1225–1230. doi: 10.1056/NEJM198405103101905
Skinner, C. M., Ivanov, N. S., Barr, S. A., Chen, Y., and Skalsky, R. L. (2017). An Epstein-Barr virus microRNA blocks IL-1 signaling by targeting the interleukin-1 receptor 1. J. Virol. 91:e00530-17. doi: 10.1128/JVI.00530-17
Tagawa, T., Albanese, M., Bouvet, M., Moosmann, A., Mautner, J., Heissmeyer, V., et al. (2016). Epstein-Barr viral miRNAs inhibit antiviral CD4+ T cell responses targeting IL-12 and peptide processing. J. Exp. Med. 213, 2065–2080. doi: 10.1084/jem.20160248
Verma, K., Jyotsana, N., Buenting, I., Luther, S., Pfanne, A., Thum, T., et al. (2017). miR-625-3p is upregulated in CD8+ T cells during early immune reconstitution after allogeneic stem cell transplantation. PLOS ONE 12:e0183828. doi: 10.1371/journal.pone.0183828
Keywords: RNA, acute infectious mononucleosis, Epstein–Barr virus, immunology
Citation: Kaul V, Weinberg KI, Boyd SD, Bernstein D, Esquivel CO, Martinez OM and Krams SM (2018) Dynamics of Viral and Host Immune Cell MicroRNA Expression during Acute Infectious Mononucleosis. Front. Microbiol. 8:2666. doi: 10.3389/fmicb.2017.02666
Received: 04 October 2017; Accepted: 21 December 2017;
Published: 15 January 2018.
Edited by:
Yves Renaudineau, University of Western Brittany, FranceReviewed by:
Mario M. D’Elios, University of Florence, ItalyHaitao Guo, University of North Carolina at Chapel Hill, United States
Copyright © 2018 Kaul, Weinberg, Boyd, Bernstein, Esquivel, Martinez and Krams. This is an open-access article distributed under the terms of the Creative Commons Attribution License (CC BY). The use, distribution or reproduction in other forums is permitted, provided the original author(s) or licensor are credited and that the original publication in this journal is cited, in accordance with accepted academic practice. No use, distribution or reproduction is permitted which does not comply with these terms.
*Correspondence: Sheri M. Krams, c21rcmFtc0BzdGFuZm9yZC5lZHU= Olivia M. Martinez, b21tQHN0YW5mb3JkLmVkdQ==
†Co-senior authors