- 1First Faculty of Medicine, Institute of Immunology and Microbiology, Charles University, Prague, Czechia
- 2Chemistry Department, Faculty of Science, J. E. Purkinje University, Ústí nad Labem, Czechia
- 3Institute of Microbiology, Academy of Sciences of the Czech Republic, Prague, Czechia
cis-Antisense RNAs (asRNAs) provide very simple and effective gene expression control due to the perfect complementarity between regulated and regulatory transcripts. In Streptomyces, the antibiotic-producing clade, the antisense control system is not yet understood, although it might direct the organism's complex development. Initial studies in Streptomyces have found a number of asRNAs. Apart from this, hundreds of mRNAs have been shown to bind RNase III, the double strand-specific endoribonuclease. In this study, we tested 17 mRNAs that have been previously co-precipitated with RNase III for antisense expression. Our RACE mapping showed that all of these mRNAs possess cognate asRNA. Additional tests for antisense expression uncovered as-adpA, as-rnc, as3983, as-sigB, as-sigH, and as-sigR RNAs. Northern blots detected the expression profiles of 18 novel transcripts. Noteworthy, we also found that only a minority of asRNAs respond to the absence of RNase III enzyme by increasing their cellular levels. Our findings suggest that antisense expression is widespread in Streptomyces, including genes of such important developmental regulators, as AdpA, RNase III, and sigma factors.
Introduction
Bacterial small RNAs are important post-transcriptional regulators that control a variety of cell processes. A large majority of these RNAs acts on target mRNAs via base pairing, an antisense mechanism that leads to positive or negative regulation of the target gene, as reviewed in Thomason (Thomason and Storz, 2010). Such antisense RNAs fall into two groups: cis- (asRNAs) and trans-encoded (sRNAs) (Romby and Charpentier, 2010).
The trans-acting sRNAs are encoded distinctly from their target mRNA(s), which is also the reason why the sRNA-mRNA pair mostly shares reduced complementarity. On the other hand, the sRNAs are usually able to act on multiple targets. The trans-encoded sRNAs have been extensively characterized and discussed in many reviews (Papenfort and Vogel, 2009; Waters and Storz, 2009). The limited base pairing requires the RNA chaperone protein Hfq in a number of bacteria. However, Hfq is either missing or its homolog has not yet been found in several bacterial clades, including Actinomycetes (Sun et al., 2002).
Reported proportions of cis-antisense expression in various bacteria vary from 13% in Bacillus subtilis (Nicolas et al., 2012), 27% in Synechocystis PCC6803 (Mitschke et al., 2011a), 30% in Anabaena (Mitschke et al., 2011b), 46% in Helicobacter pylori (Sharma et al., 2010), and up to 49% in Staphylococcus aureus (Lasa et al., 2011). These transcripts are encoded on the DNA strand opposite to their specific targets. Both asRNA and mRNA are produced by overlapping transcription, thus sharing perfect complementarity (summarized in Lasa et al., 2012). The ability of asRNAs to modulate mRNA levels is not only mediated by post-transcriptional mechanisms but they may also directly impact transcription due to collisions between RNA polymerases traveling in opposite directions [transcription interference, for review see Thomason (Thomason and Storz, 2010)]. The mechanisms of post-transcriptional action of asRNAs may be divided into two groups: (i) asRNA influences the stability of the target mRNA by either tagging for degradation or stabilizing its structure and/or (ii) asRNA affects translation either by blocking or promoting ribosome access to the ribosome binding site (Geissmann et al., 2009; Lasa et al., 2011).
In many cases of negative antisense control, the sense-antisense RNA complex formation results in its rapid cleavage. In many bacteria, two dominant endoribonucleases, RNase E and RNase III, are involved in the degradation process. The RNase E enzyme, as a member of the degradosome complex with Hfq in E. coli, cleaves single-stranded RNA (reviewed in Carpousis et al., 2009). The RNase III enzyme cleaves double-stranded RNA (MacRae and Doudna, 2007). Although RNase III was initially shown to be associated with the processing of ribosomal RNAs (Carpousis et al., 2009; Taverniti et al., 2011), its involvement in the degradation of sense/antisense pairs is being reported in an increasing number of publications (Blomberg et al., 1990; Gerdes et al., 1992; Lasa et al., 2011, 2012; Durand et al., 2012; Lioliou et al., 2012; Lybecker et al., 2014a,b; Le Rhun et al., 2016).
In Staphylococcus aureus, deep sequencing of the short RNA fraction revealed a massive accumulation of 22-nucleotide RNA fragments generated by the RNase III cleavage of paired transcripts (Lasa et al., 2011). More than 75% of the fragments corresponded to the overlapping transcription from most regions of the chromosome. The number of short RNA fragments was significantly decreased in an RNase III-deletion strain. In contrast, such a collection of short RNA fragments was not found when using a similar transcriptome analysis for the Gram-negative bacterium Salmonella enterica (Viegas et al., 2007).
Bacteria of the genus Streptomyces undergo a complex mycelial life cycle. Their growth starts with the germination of spores that develop into a vegetative mycelium of branching hyphae. Subsequent development of aerial hyphae is considered to be a cell response to nutrient depletion (Chater and Losick, 1997). At this stage part of the vegetative mycelium is lysed and can be used as a nutrient source, while the synthesis of antibiotics reaches its maximum presumably to avoid competitive organisms. Eventually, the aerial hyphae are dissected into spores by sporulation septa, producing chains of uninucleoid spores.
The complexity of the morphological and physiological differentiation in Streptomyces can be documented by the existence of more than 900 transcriptional protein regulators that control the metabolic and developmental transitions. Among them, over 60 sigma factors have been identified thus far (Gruber and Gross, 2003). Besides sigma factors, one of the most pleiotropic transcription regulators is AdpA. AdpA is expressed in an A-factor-dependent manner in Streptomyces griseus and acts as a transcriptional repressor as well as an activator thus controlling expression of several hundred genes during Streptomyces development (Higo et al., 2012). In Streptomyces coelicolor, not only could AdpA-mRNA bind purified RNase III in vitro, but, as also shown, AdpA and RNase III coordinated the expression of each other in a posttranscriptional feedback loop (Xu et al., 2010). This finding may rationally explain the rnc (RNase III-deficient) mutant phenotype that affects expression of genes involved in sporulation and antibiotic production.
Although RNase III from the Streptomyces genus was recently shown to assist processing of ribosomal RNAs (Jones et al., 2014), it came to light as a global regulator of antibiotic biosynthesis (Adamidis and Champness, 1992; Aceti and Champness, 1998; Huang et al., 2005; Gatewood et al., 2012; Lee et al., 2013; Jones et al., 2014). In Streptomyces coelicolor, the deletion of the gene encoding RNase III [rnc gene, also termed as absB (Adamidis and Champness, 1992)] leads to a severely reduced production of at least four antibiotics (actinorhodin, undecylprodigiosin, CDA, and methylenomycin) (Price et al., 1999; Chang et al., 2005; Sello and Buttner, 2008). Microarray analysis was used to compare the levels of gene expression in the S. coelicolor parental strain and the RNase III mutant strain (Huang et al., 2005). A wide effect of the ribonuclease was found, mainly on genes connected with sporulation and antibiotic production. In the rnc mutant strain, sporulation genes were up-regulated, whereas activators of the antibiotic biosynthetic pathways (e.g., actII-ORF4, redD, redZ, and cdaR) were down-regulated, which is consistent with defects in antibiotic production. However, Strakova (Strakova et al., 2013) revealed that rnc expression is activated from the first hour of germination, suggesting a more general role for RNase III, either in ribosomal RNA or in asRNA processing. Subsequent microarray and co-immunoprecipitation analyses, performed by Gatewood (Gatewood et al., 2012), revealed at least 777 mRNAs bound by the RNase III enzyme. The authors also showed that the absence of the enzyme directly or indirectly affected the levels of hundreds of mRNAs and at least two small RNAs. These very valuable results greatly inspired the work described in this paper.
Confirmation of the expected employment of small RNAs in the regulation of cell processes, including primary metabolism, developmental transitions, antibiotic production, and various stress responses is being increasingly reported (Palecková et al., 2007; Pánek et al., 2008; Swiercz et al., 2008; D'Alia et al., 2010; Mikulík et al., 2014). Hundreds of cis-acting asRNAs were identified using RNA sequencing in two recent studies (Vockenhuber et al., 2011; Moody et al., 2013). Here, we further exploited Gatewood's results to see if there are connections between asRNAs and RNase III, which have not yet been reported in Streptomyces. We selected 17 mRNAs that are bound by the RNase III enzyme in vivo as stated in Gatewood et al. (2012) to check if they possessed an antisense transcript. Surprisingly, the search for asRNAs within the selected group of mRNAs was 100% successful. Moreover, additional analyses revealed antisense transcripts to selected mRNAs that encode RNase III and several transcription regulators (AdpA, SigB, SigH, and SigR).
Although our data did not elucidate the exact role of newly found asRNAs in the RNase III-degradation pathway, the current findings further demonstrate that the antisense mechanism is widely present in Streptomyces and antisense RNAs are possibly involved in developmental and antibiotic synthesis control.
Materials and Methods
Bacterial Strain, Cultivation
In this study, the Streptomyces coelicolor wild-type (wt) strain M145 (Kieser et al., 2000) and its RNase III-deletion strain derivative [rnc, M145 rnc::aac(3)IV (Sello and Buttner, 2008)] were used. 108 spores were inoculated on solid R2YE medium (Kieser et al., 2000) covered by cellophane at 29°C. Bacterial samples were collected after 24, 48, and 72 h of cultivation, where each time point represented a different developmental stage, i.e., vegetative mycelium, aerial mycelium, and spores.
RNA Isolation
Total RNA was isolated using a TRIzol method (Van Dessel et al., 2004). Harvested cells were immediately submerged in TRIzol reagent (Ambion) on ice (1 ml of TRIzol per 50 cm2 of culture dish surface area). Five glass beads (3 mm in diameter) were added to the cell suspension. The cells were disrupted using a Minilys homogenizer (Precellys) twice for 2 min at 3,000 rpm and twice for 2 min at 4,000 rpm, cooled on ice between the cycles. The samples were subsequently centrifuged for 2 min at 10,000 g and purified in TRIzol/chloroform (5:1) and chloroform. For RNA precipitation, the samples were incubated in isopropanol at −20°C overnight and centrifuged for 30 min at 10,000 g. RNA samples were washed in ethanol and resuspended in 30 μl of RNase-free water. Residual DNA in the RNA samples was removed by DNase I treatment (Ambion). Typically, a concentration between 1 and 3.5 μg/μl was obtained. RNA quality was checked on a 1% agarose gel.
5′ and 3′ Race
RNA samples were isolated after 48 h of cultivation of both wt and rnc strains. Antisense RNA expressions were tested by means of the FirstChoice RLM-RACE Kit (Ambion) following the manufacturer's protocol with the several exceptions:
1. Because the uncapped 5′ ends of bacterial RNAs are sensitive to the CIP (calf intestinal phosphatase) enzyme dephosphorylation, the treatment was omitted from the 5′ RACE procedure.
2. A gene-specific primer (see Figure 1) was used instead of random decamers in the 5′RACE. The 5′RACE primers as well as the probes used for Northern blot hybridizations were designed to cover the ribosome binding site and start codon of a cognate mRNA. All of the primers were designed using the Primer3 software (http://sourceforge.net/projects/primer3/) (Untergasser et al., 2012).
3. The PrimeScript (Takara, 100 units per 10 μl of reaction mixture) reverse transcriptase was always included in the experiment. Negative control lacking the enzyme was always attached to the experiment.
4. The reverse transcription proceeded at 42°C for 45 min and 48°C for 10 min.
5. Preceding the 3′RACE, total RNA samples were polyadenylated by 5 units of Poly(A) Polymerase I (New England Biolabs), according the manufacturer's protocol.
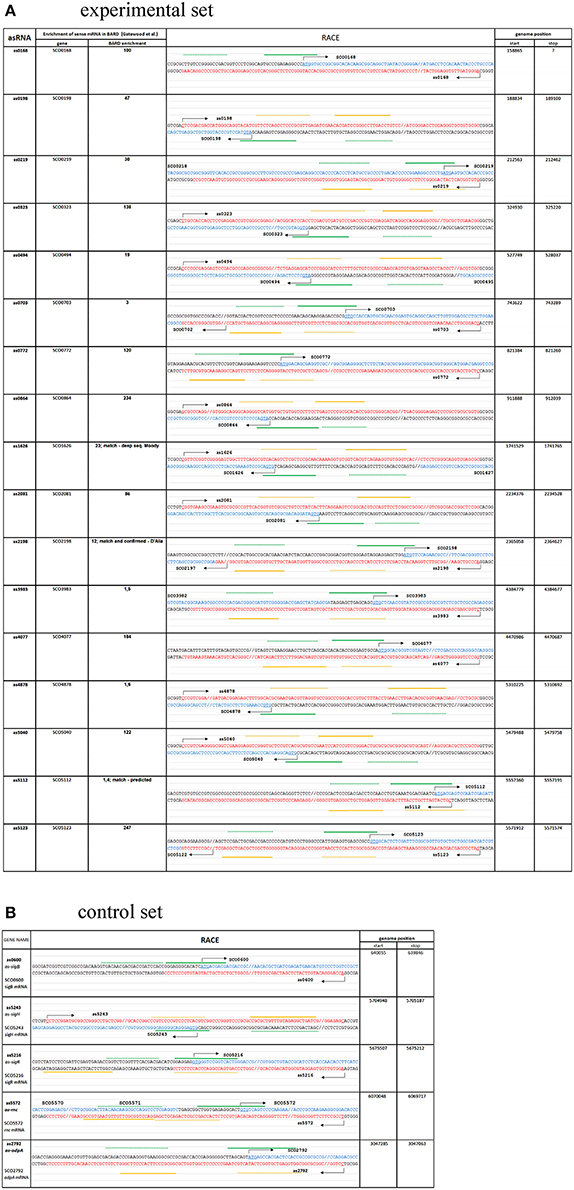
Figure 1. Novel asRNAs revealed by 5′and 3′RACE method, and their genome locations (sequence of asRNA in red, sequence of mRNA in blue). Transcriptional start sites are indicated by arrows. Full green line represents 5′RACE inner primers, dotted green line represents 5′RACE outer primers, full orange line represents 3′RACE inner primers, dotted orange line represents 3′RACE outer primers. (A) Experimental set; (B) Control set; (see text for details).
Final PCR products were separated on a 1.2% agarose gel. Products that were found in samples but absent in negative controls were excised and purified using the Qiagen MinElute PCR purification kit. The purified products were cloned into the TOPO vector using the TOPO TA Cloning (Invitrogen) and transformed into E. coli One Shot TOP10F' competent cells (Invitrogen). Plasmids containing the cDNA inserts were extracted using the QIAprep Miniprep kit, and sequenced to map 5′ and 3′ ends of RNAs reverse-transcribed.
Reverse Transcription and PCR
Experiments were performed according to the reverse transcription and PCR protocols described in the FirstChoice RLM-RACE Kit using gene specific DNA probes (details in Results and discussion). The PrimeScript (Takara, 100 units per 10 μl of reaction mixture) was used as a reverse transcriptase. A negative control PCR reaction used the original RNA sample as a template.
Northern Blot Analysis
RNA samples (30 μg) were denatured for 10 min at 70°C in RNA loading buffer (95% formamide, 0.1% bromophenol blue, 0.1% xylene cyanol, 10 mM EDTA) and separated in a 1% agarose gel containing formaldehyde, provided by the NorthernMax Kit (Ambion). Separated samples were transferred onto positively charged nylon membranes (ZetaProbe, Bio-Rad) by electroblotting at 240 mA for 45 min. The nylon membrane was UV-crosslinked.
Oligonucleotides were radioactively labeled on their 5′ ends by γ-32P-ATP using T4 polynucleotide kinase (Thermo Scientific) and purified (QIAquick Nucleotide Removal Kit, Qiagen). Hybridization was performed in ULTRAhyb hybridization buffer (Ambion) overnight at 37–42°C. The membranes were then washed twice with 2xSSC, 0.1% SDS (NorthernMax kit) at room temperature and once with 0.1xSSC, 0.1% SDS (NorthernMax kit) at 42°C. The membranes were dried and exposed in a BAS cassette on the imaging plate (Fuji-Film) for 4 days. The signals were visualized using a Phosphorimager FX (Bio-Rad) and quantified using QuantityOne analysis software (Bio-Rad), where the signals were standardized proportionally to the 5S RNA levels. Each northern blot was performed at least twice with samples from separate cultivations in the same conditions.
Results
Using the RNA-seq approach, Gatewood (Gatewood et al., 2012) compared gene expression between the S. coelicolor M145 wild type strain and the JSE1880 rnc-mutant strain. The authors found that approximately 10% of all mRNAs from the vegetative state of growth were directly or indirectly affected by RNase III. In addition, they applied RNA immunoprecipitation to detect mRNAs targeted by the enzyme (referred to in their paper as BARD, bead-antibody-RNA-D70A strain). However, the necessity of involvement of other transcripts for the binding of the double-strand-specific enzyme to the mRNAs is still unknown in the case of Streptomyces. In vitro assays showed that the RNase III digests mRNA transcripts SCO3982 to SCO3988 and SCO5737 unattended (i.e., without asRNA) (Gatewood et al., 2012), whereas another unattended transcript, SCO0762, was not cleaved (Xu et al., 2008). Although Streptomyces as GC rich bacteria form highly structured RNAs, naturally occurring stem-loop structures of most mRNAs are too short to be bound by the RNase III enzyme that requires a minimum of approximately 20 bp of double stranded RNA for binding in vivo (Robertson, 1982).
RNase III-Binding mRNAs and Small RNAs Genome Vicinities
Here, to probe if the antisense RNA expression occurs in the vicinity of the RNase III-binding mRNAs (or alternatively, the binding is not complexed with asRNA), we firstly analyzed the genes whose transcripts are affected by RNase III (i.e., those mRNAs that are increased by more than two-fold in the JSE1880 rnc-mutant or detected in BARD as listed in the Table S2 in Gatewood; Gatewood et al., 2012). We asked whether in the proximity of these genes lies a gene encoding for any of 1713 small RNAs found or predicted in S. coelicolor up-to-date (Pánek et al., 2008; Swiercz et al., 2008; D'Alia et al., 2010; Vockenhuber et al., 2011; Moody et al., 2013) that could act on the messenger by the antisense mechanism. We found out that from the 153 mRNAs increased in JSE1880 (at a single experimental time point), 45 neighbor with one of the 1713 small RNAs that could thus theoretically act as an asRNA. From these 45 mRNAs, 21 mRNAs were listed in the BARD, i.e., they bind RNase III. These data, summarized in Table 1, encouraged us to search for novel asRNAs expressed in the opposite direction to other RNase III-binding mRNAs.
Experimental Search for Novel cis-Antisense Transcripts
Genes for the experimental analyses had been selected independently of the in silico search above. We altogether tested 30 genes for the possible novel antisense expression. The initial experimental set consisted of 17 exemplar genes which we selected out of a total of 37 genes whose mRNAs were enriched in the BARD, i.e., they co-precipitated with RNase III (listed in Table 2, see also Gatewood's Table 2 and Table S3 in Gatewood et al., 2012). Within this set, only as1625, as2198, and as5112 genes have been predicted before (see Table 1). The control set consisted of three other genes—SCO5737, adpA, and rnc (the gene of RNase III) that had been previously shown to be directly targeted by RNase III in vitro (Xu et al., 2008, 2010; Gatewood et al., 2012). Additionally, to determine whether the existence of antisense transcripts involves only those messengers bound by RNase III or is even more widespread, we decided to add into the control set the genes whose transcripts have not been shown to bind RNase III. For these tests, we selected 10 genes encoding sigma factors (HrdA, HrdB, HrdC, HrdD, SigB, SigD, SigE, SigH, SigR, and WhiG) as important transcriptional regulators that govern gene expression, controlling cell development and/or responses to various stresses (Bobek et al., 2014).
We reasoned that many asRNAs overlap the ribosome binding site and the start codon of their target, possibly leading to negative translational control. Following this, all of the DNA primers used here to find antisense transcripts have been designed accordingly (Figure 1). To demonstrate expression of antisense transcripts, 5′ RACE analyses were performed using the primers. Sequence(s) extended from the primer was/were PCR amplified and sequenced. To avoid false positive results, each reverse transcription was accompanied with a negative control sample lacking the reverse transcriptase in the reaction. The resulting electrophoretograms of both the experimental and the negative control samples were compared to exclude non-unspecific fragments in the experimental sample from sequencing.
From the total of 30 samples tested (involving both the experimental and the control set), 22 revealed a novel antisense transcript in cis (5′ end(s) detected; see Table 2).
From the 17 samples of the experimental set (i.e., our selection from those mRNAs enriched in the BARD), 17 cognate asRNAs were detected (three of them have been predicted previously as could be seen in Table 1), signifying a 100% outcome within this group (Figure 1A). The 3′ RACE revealed 16 3′ ends within the experimental set (scr0168 unsuccessful, northern blot was not performed).
In the control set (Figure 1B), our RACE data detected transcripts antisense to adpA (as-adpA), rnc (as-rnc), and three sigma factor genes (as-sigB, as-sigH, as-sigR). Seven sigma factor genes and the transcript SCO5737, which is bound by RNase III in vitro, did not reveal antisense expression.
Due to our primer design, we did not detect such antisense transcripts acting on messenger's 3′ end [in Moody (Moody et al., 2013) termed as cutoRNAs]. Also the experimental approach did not allow identification of trans-encoded sRNAs, although similar complementary sequence on the genome could be found (as examples, novel RNAs as0772, as0600, as3983, and as5216 could theoretically act on other targets throughout the genome).
Sometimes the RACE analysis may produce false positive results due to the RNA self-priming during the reverse transcription which could be theoretically caused by stem-loop structures formation. In order to confirm the existence of the newly found cis-antisense transcripts and to compare their expression during Streptomyces cell development (24, 48, and 72 h at standard growth conditions, see methods), we performed northern blot analyses. From the total of 22 analyzed, 18 asRNAs revealed an apparent signal (Figure 2; for the raw northern blot images see Presentation 1 in Supplementary Material). The signals of the remaining four were too weak or undetectable.
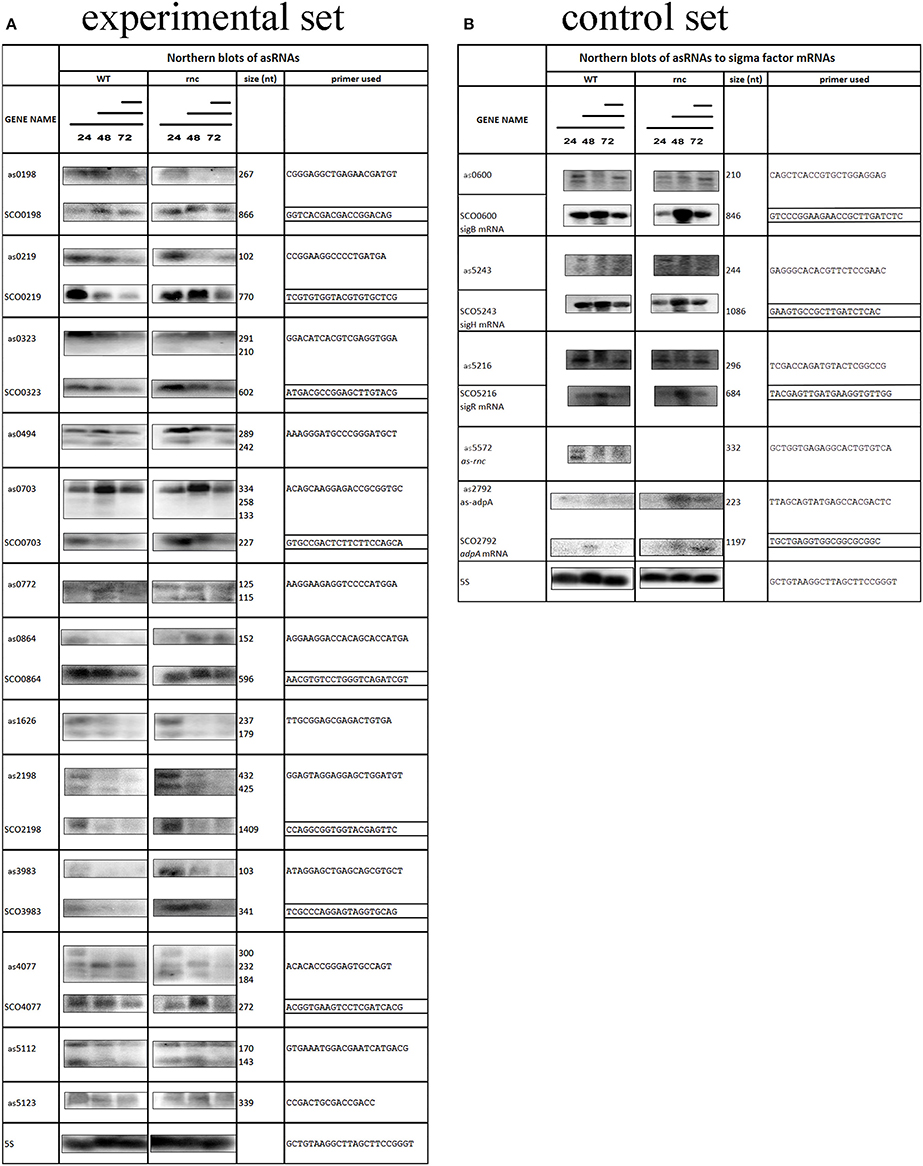
Figure 2. Differential expression analyses of novel asRNAs and their target mRNAs in WT and rnc strains. Three black lines (from long to short) represent RNA samples from vegetative mycelium (24 h), aerial mycelium (48 h), and spores (72 h), respectively. To enable comparison of the expression profiles, all asRNA-mRNA pairs from both WT and rnc strains were analyzed on the same blot. Sizes of the products well corresponded to those obtained by RACE. Primers used are shown on the right. The 5S loading control is included below. The signal quantification is presented in Table S2. (A) Experimental set; (B) Control set; (see text for details).
Details describing expression profiles of asRNAs are summarized in Table S1 and signal quantification is presented in Table S2 online, where the listed relative values proportional to the 5S RNA controls correspond with intracellular levels of the analyzed transcripts.
Vice Versa: scr2101 Small RNAs Found in BARD (Gatewood et al., 2012) is Antisense to the SCO2100-SCO2101 Transcript
In Gatewood's work, expression of two small RNAs, scr6925 and scr2101, was enriched in the rnc strain (scr = small S. coelicolor RNA). These data imply their antisense role and subsequent processing by the RNase III enzyme. The expression of scr2101 was previously revealed by Swiercz (Swiercz et al., 2008). Gatewood et al. (2012) showed that the molecule is up-regulated in the JSE1880 strain (lacking the RNase III), where its level was 7-fold higher compared to that in the wild type strain. scr2101 was also shown to be bound by the enzyme (presented in the BARD, although its enrichment was only 0.57). As the RNA's gene lies on the opposite strand between SCO2100 and SCO2101, we raised the question whether these two protein-coding genes form an operon, producing one common transcript targeted by the scr2101 antisense RNA. The potential SCO2100-SCO2101 common transcript was used here as a template for reverse transcription using a DNA probe complementary to the 5′ end of the SCO2101 mRNA (TGTCCCGGCTGCTCCAGGGA). The second DNA primer, used for the following PCR amplification, was identical to the 3′ end of the SCO2100 mRNA sequence (CGTAGGTCCCCGCCCGCT), thus forming a 635-nt product, which was indeed produced. Use of a negative control PCR reaction, where the original RNA was used instead of a template, eliminated the risk of false positive results (Figure S1). This finding suggests that the antisense function of scr2101 (which thus should be termed as as2101) targets the region between two open reading frames. Although the binding experiments are outside the scope of this paper, our findings raise demand to expand the RNase III-binding analyses to the unannotated sRNA transcriptome.
Discussion
Novel asRNAs to mRNAs That Bind RNase III in Vitro
The double stranded stems of stem-loop mRNA structures are thought to be too short to be digested by the RNase III enzyme (Robertson, 1982). On the other hand, one may argue that the stem-loop structures on several mRNAs are the only possible targets for RNase III activity. Indeed, the enzyme is involved in pre-rRNA, tRNA, and polycistronic RNA processing (Conrad and Rauhut, 2002; Drider and Condon, 2004), where such stem-loop structures are present and possibly long enough to be cleaved by the enzyme. Gatewood clearly showed that some lone mRNAs are targeted and cleaved by RNase III in vitro (SCO5737, or the SCO3982 to SCO3988 mRNA region). In accordance to the first example, our experiments did not reveal any antisense transcript to the SCO5737 (which encodes a polynucleotide phosphorylase). Its mRNA transcript is 2,220 nt in length, and thus might be capable of forming several longer double-stranded regions observed by RNAfold (http://rna.tbi.univie.ac.at/cgi-bin/RNAWebSuite/RNAfold.cgi; fig. not shown), which are most likely targeted by the RNase III enzyme in vitro.
On the other hand, we show that at least one member of the SCO3982-SCO3988 operon – SCO3983 possesses an antisense gene. Its transcript, as3983, is 103 nt long and exhibited a strongest expression signal in vegetative cells (24 h) of the rnc strain. The mRNA also had a strong signal at 24 h and 48 h, even elevated in the rnc strain. Moreover, we observed that the as3983 sequence is nearly identical to the region adjacent to SCO3268 and theoretically may thus also act on this transcript in trans.
In addition to the identified as3983 RNA, we have found antisense transcripts to two other mRNAs targeted by RNase III in vitro. These two mRNAs code important developmental regulators that influence antibiotic production—AdpA and the RNase III itself.
According to Gatewood's results, the adpA mRNA was enriched 22-fold in the BARD. Therefore, we asked whether AdpA expression is controlled by an antisense mechanism. Indeed, our RACE analysis revealed a 223-nt long antisense transcript termed as-adpA (Figure 1B) and although subsequent northern blot weakly detected the transcript in the wt strain (faint signal at 72 h stage of growth, Figure 2B), it was clearly found in the rnc strain at later stages, peaking at the 48 h old mycelium.
The rnc gene (SCO5572) that encodes the RNase III enzyme is the last member of a three gene operon, about 2,000 nt in length, that also encodes a hypothetical protein SCO5570 and a ribosomal protein L32 (SCO5571). A point mutation at amino acid position 120 of the RNase III protein causes deficiency in the ribonucleolytic activity of the enzyme, whereas its ability to bind double stranded RNAs remains intact (Huang et al., 2005). Observations that the rnc mRNA abundance is increased in these mutants led to discovery that RNase III cleaves among others its own transcript (Xu et al., 2008). Consistently, our northern blot analyses did not detect the rnc transcript in the wt strain, though we clearly confirmed the as-rnc RNA, which was detectable during the time course. Hence the antisense mechanism is probably involved in gene expression control of regulatory proteins such as the RNase III enzyme or the AdpA transcription regulator. Our future effort will be focused on more detailed characterization of the involvement of these two novel asRNAs in Streptomyces development.
as2198 RNA as an Example from the Experimental Set
as2198 is an asRNA to the glnA gene (SCO2198, encoding a glutamine synthase) and was previously independently shown to be expressed, termed cnc2198.1 (D'Alia et al., 2010). The authors performed a detailed functional analysis and revealed that overexpression of cnc2198.1 affects growth rate and antibiotic production. In the overexpression strain, the intracellular level of the targeted GlnA protein was decreased by 40%. The authors speculated that the glnA-cnc2198.1 RNA complex blocks glnA translation, which may lead to its subsequent degradation. We further hypothesize that the complex is degraded by the RNase III enzyme, as our northern blot revealed that the asRNA level is increased in the rnc mutant. Our RACE mapping estimated the size of the antisense transcript to be 432 nt, nearly four times longer than the 121 nt transcript described by D'Alia et al. (2010). Moreover, the detected 3′ end overlapped the adjacent SCO2197, revealing that as2198 is in fact the 5′UTR of the SCO2197 mRNA. The fact that our northern blot showed a second, weaker fragment of 425 nt in size, could mean that the SCO2197 gene possesses another promoter or its transcript is further processed. Both of the detected fragments, as well as the cognate SCO2198 mRNA, had the strongest expression signals in samples from vegetative 24-h old cells, even strengthened in the rnc strain.
Are the Sense-Antisense Transcripts Cleaved by RNase III?
Because the sense mRNAs of the antisense transcripts found here are targeted by RNase III (or at least, their expression was negatively affected in the presence of the enzyme), we speculated that the RNase III enzyme is the most likely candidate for the paired transcript degradations. The 18 northern blot positive results proved the existence of novel asRNAs. However, only five of those asRNAs were increased in the rnc strain when compared to the expression in the wild type strain. These included as0494, as0864, as2198, as3983, and the as-adpA. On the other hand, in two other cases, our northern blots suggest even a positive effect of the presence of RNase III enzyme on the cellular level of asRNAs (as0323 and as5112, see below). Consistently, Gatewood (Gatewood et al., 2012) showed that the majority of known sRNAs, detected in their RNA-seq analysis, do not exhibit significant expression differences between the wild-type and the rnc-deletion mutant. As another example of sense-antisense RNA pair with an undistinguishable or even a positive effect of the enzyme on its stability in Streptomyces is the scr4677-SCO4676 complex (Hindra et al., 2014). The possibility that the RNase III enzyme does not always post-transcriptionally degrade sense-antisense complexes is also inferred from a study on sRNA degradation by three different RNases (RNase Y, J1, and III) in another Gram-positive bacterial model, Bacillus subtilis (Durand et al., 2012). In Bacillus, RNase III depletion has little effect on antisense RNAs observed by high-resolution tiling arrays. Although several RNAs showed increased abundance in the RNase III mutant, their half-lives were not affected by the enzyme, as observed by northern blot analysis. The authors concluded that the role of RNase III in Bacillus subtilis lies more likely in indirect transcriptional control rather than post-transcriptional RNA turnover. One may argue that the function of the enzyme might be substituted by other ribonucleases in rnc mutants. As the RNase III-binding mRNAs may serve as a fruitful source for novel antisense transcript discoveries, we can assume that the mRNA-asRNA pairs are targeted by the enzyme but not always degraded. An involvement of some other RNA-binding proteins, such as RNA helicase or Hfq-like protein which has not been found in Streptomyces yet, could be expected (Gerhart Wagner, Uppsala University, Sweden, personal communication). On the other hand, the possibility that in other cases the antisense transcripts might protect their mRNA targets against the RNase III cleavage should be taken in mind. Clarification of the exact role of RNase III enzyme in Streptomyces remains to be established.
asRNAs as a Potential Part of the Gene Expression Control System in Streptomyces
The genus Streptomyces can be presented as a model bacterial group lying on the top of prokaryotic cellular complexity. Their 8–10 Mbp long genome encodes all the developmental stages, including morphological changes (spore formation and germination, vegetative branching hyphae, aerial twisting mycelium), secondary metabolite production (antibiotics and a variety of other bioactive compounds, siderophores, pigments, etc.) and a capacity to respond to all possible environmental changes (diverse stresses) that are encountered. Developmental transitions and environmental intricacy require advanced regulatory networks that involve a concerted action of more than 900 transcription regulatory proteins known thus far. Here we unveiled 22 novel antisense transcripts, out of which 18 were confirmed by northern blot analyses. These results suggest an equivalent role and possibly even bigger number of non-protein-coding RNA regulators. The cis-antisense transcripts are efficient gene expression modulators with minimal space requirements on their genome (Georg and Hess, 2011), with a theoretical capability to act on nearly all genes. Moreover, the mode of their action (whether based on co-transcriptional collision or post-transcriptional 100% complementarity) is simple and effective. Our work suggests that antisense transcription is widespread in Streptomyces and somehow connected with the function of RNase III, although the absence of the rnc gene did not greatly influence the majority of the transcripts. It is noteworthy that the majority of antisense transcripts found here escaped previous sRNA predictions and/or whole genome searches, suggesting that their expression level is often low and may be lost during statistical background subtraction. Nevertheless, the results in this work confirm the extensiveness of the antisense transcripts and raise the demand for elucidation of their role in gene expression control in Streptomyces.
Author Contributions
DŠ, PP, IM, and KŠ conceived the RACE and northern blot experiments, JB performed the in silico analysis and provided the project design, analyzed the results and wrote the manuscript. All authors reviewed the manuscript.
Conflict of Interest Statement
The authors declare that the research was conducted in the absence of any commercial or financial relationships that could be construed as a potential conflict of interest.
Acknowledgments
Authors are very grateful to Prof. Beatrix Suess, Dr. Michael Vockenhuber, and Dr. Marie Elliot for checking their RNA-Seq expression data to verify the results presented here. This work was supported by a project of J. E. Purkinje University: UJEP-SGS-173-07-01 to JB, PP, IM, a project of Charles University in Prague: Progres Q26/LF1 to JB, SVV260369 to DŠ and KŠ, by the Czech research infrastructure for systems biology C4SYS (project no. LM2015055) to JB, and a grant of the Grant Agency of the Charles University (www.cuni.cz/UK-33.html) under contract no. 160214 to KŠ and 290215 to DŠ.
Supplementary Material
The Supplementary Material for this article can be found online at: https://www.frontiersin.org/articles/10.3389/fmicb.2017.02693/full#supplementary-material
Figure S1. SCO2100-SCO2101 RT-PCR. RNA samples were isolated from both wt (lane 1) and rnc (lane 2) 48 h old cultures from the R2YE medium. The first probe TGTCCCGGCTGCTCCAGGGA primed the reverse transcription reaction and together with the second probe CGTAGGTCCCCGCCCGCT was used in the subsequent PCR. Additional samples originated from both strains but lacking reverse transcriptase in the reaction were used as negative controls (lanes 3 and 4).
Table S1. Description of the expression profiles of detected asRNAs.
Table S2. Quantification of the northern blot signals presented in Figure 2.
Presentation 1. Raw northern blot images.
References
Aceti, D. J., and Champness, W. C. (1998). Transcriptional regulation of Streptomyces coelicolor pathway-specific antibiotic regulators by the absA and absB loci. J. Bacteriol. 180, 3100–3106.
Adamidis, T., and Champness, W. (1992). Genetic analysis of absB, a Streptomyces coelicolor locus involved in global antibiotic regulation. J. Bacteriol. 174, 4622–4628. doi: 10.1128/jb.174.14.4622-4628.1992
Blomberg, P., Wagner, E. G., and Nordström, K. (1990). Control of replication of plasmid R1: the duplex between the antisense RNA, CopA, and its target, CopT, is processed specifically in vivo and in vitro by RNase III. EMBO J. 9, 2331–2340.
Bobek, J., Strakova, E., Zikova, A., and Vohradsky, J. (2014). Changes in activity of metabolic and regulatory pathways during germination of S. coelicolor. BMC Genomics 15:1173. doi: 10.1186/1471-2164-15-1173
Carpousis, A. J., Luisi, B. F., and McDowall, K. J. (2009). Endonucleolytic initiation of mRNA decay in Escherichia coli. Prog. Mol. Biol. Transl. Sci. 85, 91–135. doi: 10.1016/S0079-6603(08)00803-9
Chang, S. A., Bralley, P., and Jones, G. H. (2005). The absB gene encodes a double strand-specific endoribonuclease that cleaves the read-through transcript of the rpsO-pnp operon in Streptomyces coelicolor. J. Biol. Chem. 280, 33213–33219. doi: 10.1074/jbc.M503440200
Chater, K., and Losick, R. (1997). “Mycelial life style of Streptomyces coelicolor A3 (2) and its relatives,” in Bacteria as Multicellular Organisms, eds K. F. Chater, R. Losick, and American Society for Microbiology (New York, NY: Oxford University Press), 149–182.
Conrad, C., and Rauhut, R. (2002). Ribonuclease III: new sense from nuisance. Int. J. Biochem. Cell Biol. 34, 116–129. doi: 10.1016/S1357-2725(01)00112-1
D'Alia, D., Nieselt, K., Steigele, S., Müller, J., Verburg, I., and Takano, E. (2010). Noncoding RNA of glutamine synthetase I modulates antibiotic production in Streptomyces coelicolor A3(2). J. Bacteriol. 192, 1160–1164. doi: 10.1128/JB.01374-09
Drider, D., and Condon, C. (2004). The continuing story of endoribonuclease III. J. Mol. Microbiol. Biotechnol. 8, 195–200. doi: 10.1159/000086700
Durand, S., Gilet, L., Bessières, P., Nicolas, P., and Condon, C. (2012). Three essential ribonucleases-RNase Y, J1, and III-control the abundance of a majority of Bacillus subtilis mRNAs. PLoS Genet. 8:e1002520. doi: 10.1371/journal.pgen.1002520
Gatewood, M. L., Bralley, P., Weil, M. R., and Jones, G. H. (2012). RNA-Seq and RNA immunoprecipitation analyses of the transcriptome of Streptomyces coelicolor identify substrates for RNase III. J. Bacteriol. 194, 2228–2237. doi: 10.1128/JB.06541-11
Geissmann, T., Marzi, S., and Romby, P. (2009). The role of mRNA structure in translational control in bacteria. RNA Biol. 6, 153–160. doi: 10.4161/rna.6.2.8047
Georg, J., and Hess, W. R. (2011). cis-antisense RNA, another level of gene regulation in bacteria. Microbiol. Mol. Biol. Rev. 75, 286–300. doi: 10.1128/MMBR.00032-10
Gerdes, K., Nielsen, A., Thorsted, P., and Wagner, E. G. (1992). Mechanism of killer gene activation. Antisense RNA-dependent RNase III cleavage ensures rapid turn-over of the stable hok, srnB and pndA effector messenger RNAs. J. Mol. Biol. 226, 637–649. doi: 10.1016/0022-2836(92)90621-P
Gruber, T. M., and Gross, C. A. (2003). Multiple sigma subunits and the partitioning of bacterial transcription space. Annu. Rev. Microbiol. 57, 441–466. doi: 10.1146/annurev.micro.57.030502.090913
Higo, A., Hara, H., Horinouchi, S., and Ohnishi, Y. (2012). Genome-wide distribution of AdpA, a global regulator for secondary metabolism and morphological differentiation in Streptomyces, revealed the extent and complexity of the AdpA regulatory network. DNA Res. 19, 259–273. doi: 10.1093/dnares/dss010
Hindra Moody, M. J., Jones, S. E., and Elliot, M. A. (2014). Complex intra-operonic dynamics mediated by a small RNA in Streptomyces coelicolor. PLoS ONE 9:e85856. doi: 10.1371/journal.pone.0085856
Huang, J., Shi, J., Molle, V., Sohlberg, B., Weaver, D., Bibb, M. J., et al. (2005). Cross-regulation among disparate antibiotic biosynthetic pathways of Streptomyces coelicolor. Mol. Microbiol. 58, 1276–1287. doi: 10.1111/j.1365-2958.2005.04879.x
Jones, S. E., Leong, V., Ortega, J., and Elliot, M. A. (2014). Development, antibiotic production, and ribosome assembly in Streptomyces venezuelae are impacted by RNase J and RNase III deletion. J. Bacteriol. 196, 4253–4267. doi: 10.1128/JB.02205-14
Kieser, T. B. M., Buttner, M. J., Chater, K. F., and Hopwood, D. A. (2000). Practical Streptomyces Genetics, 2nd Edn. Norwich: John Innes Foundation.
Lasa, I., Toledo-Arana, A., Dobin, A., Villanueva, M., de los Mozos, I. R., Vergara-Irigaray, M., et al. (2011). Genome-wide antisense transcription drives mRNA processing in bacteria. Proc. Natl. Acad. Sci. U.S.A. 108, 20172–20177. doi: 10.1073/pnas.1113521108
Lasa, I., Toledo-Arana, A., and Gingeras, T. R. (2012). An effort to make sense of antisense transcription in bacteria. RNA Biol. 9, 1039–1044. doi: 10.4161/rna.21167
Lee, J. H., Gatewood, M. L., and Jones, G. H. (2013). RNase III is required for actinomycin production in Streptomyces antibioticus. Appl. Environ. Microbiol. 79, 6447–6451. doi: 10.1128/AEM.02272-13
Le Rhun, A., Beer, Y. Y., Reimegård, J., Chylinski, K., and Charpentier, E. (2016). RNA sequencing uncovers antisense RNAs and novel small RNAs in Streptococcus pyogenes. RNA Biol. 13, 177–195. doi: 10.1080/15476286.2015.1110674
Lioliou, E., Sharma, C. M., Caldelari, I., Helfer, A. C., Fechter, P., Vandenesch, F., et al. (2012). Global regulatory functions of the Staphylococcus aureus endoribonuclease III in gene expression. PLoS Genet. 8:e1002782. doi: 10.1371/journal.pgen.1002782
Lybecker, M., Bilusic, I., and Raghavan, R. (2014a). Pervasive transcription: detecting functional RNAs in bacteria. Transcription 5:e944039. doi: 10.4161/21541272.2014.944039
Lybecker, M., Zimmermann, B., Bilusic, I., Tukhtubaeva, N., and Schroeder, R. (2014b). The double-stranded transcriptome of Escherichia coli. Proc. Natl. Acad. Sci. U.S.A. 111, 3134–3139. doi: 10.1073/pnas.1315974111
MacRae, I. J., and Doudna, J. A. (2007). Ribonuclease revisited: structural insights into ribonuclease III family enzymes. Curr. Opin. Struct. Biol. 17, 138–145. doi: 10.1016/j.sbi.2006.12.002
Mikulík, K., Bobek, J., Zídková, J., and Felsberg, J. (2014). 6S RNA modulates growth and antibiotic production in Streptomyces coelicolor. Appl. Microbiol. Biotechnol. 98, 7185–7197. doi: 10.1007/s00253-014-5806-4
Mitschke, J., Georg, J., Scholz, I., Sharma, C. M., Dienst, D., Bantscheff, J., et al. (2011a). An experimentally anchored map of transcriptional start sites in the model cyanobacterium Synechocystis sp. PCC6803. Proc. Natl. Acad. Sci. U.S.A. 108, 2124–2129. doi: 10.1073/pnas.1015154108
Mitschke, J., Vioque, A., Haas, F., Hess, W. R., and Muro-Pastor, A. M. (2011b). Dynamics of transcriptional start site selection during nitrogen stress-induced cell differentiation in Anabaena sp. PCC7120. Proc. Natl. Acad. Sci. U.S.A. 108, 20130–20135. doi: 10.1073/pnas.1112724108
Moody, M. J., Young, R. A., Jones, S. E., and Elliot, M. A. (2013). Comparative analysis of non-coding RNAs in the antibiotic-producing Streptomyces bacteria. BMC Genomics 14:558. doi: 10.1186/1471-2164-14-558
Nicolas, P., Mäder, U., Dervyn, E., Rochat, T., Leduc, A., Pigeonneau, N., et al. (2012). Condition-dependent transcriptome reveals high-level regulatory architecture in Bacillus subtilis. Science 335, 1103–1106. doi: 10.1126/science.1206848
Palecková, P., Felsberg, J., Bobek, J., and Mikulík, K. (2007). tmRNA abundance in Streptomyces aureofaciens, S. griseus and S. collinus under stress-inducing conditions. Folia Microbiol. (Praha) 52, 463–470. doi: 10.1007/BF02932105
Pánek, J., Bobek, J., Mikulík, K., Basler, M., and Vohradský, J. (2008). Biocomputational prediction of small non-coding RNAs in Streptomyces. BMC Genomics 9:217. doi: 10.1186/1471-2164-9-217
Papenfort, K., and Vogel, J. (2009). Multiple target regulation by small noncoding RNAs rewires gene expression at the post-transcriptional level. Res. Microbiol. 160, 278–287. doi: 10.1016/j.resmic.2009.03.004
Price, B., Adamidis, T., Kong, R., and Champness, W. (1999). A Streptomyces coelicolor antibiotic regulatory gene, absB, encodes an RNase III homolog. J. Bacteriol. 181, 6142–6151.
Robertson, H. D. (1982). Escherichia coli ribonuclease III cleavage sites. Cell 30, 669–672. doi: 10.1016/0092-8674(82)90270-7
Romby, P., and Charpentier, E. (2010). An overview of RNAs with regulatory functions in gram-positive bacteria. Cell. Mol. Life Sci. 67, 217–237. doi: 10.1007/s00018-009-0162-8
Sello, J. K., and Buttner, M. J. (2008). The gene encoding RNase III in Streptomyces coelicolor is transcribed during exponential phase and is required for antibiotic production and for proper sporulation. J. Bacteriol. 190, 4079–4083. doi: 10.1128/JB.01889-07
Sharma, C. M., Hoffmann, S., Darfeuille, F., Reignier, J., Findeiss, S., Sittka, A., et al. (2010). The primary transcriptome of the major human pathogen Helicobacter pylori. Nature 464, 250–255. doi: 10.1038/nature08756
Strakova, E., Bobek, J., Zikova, A., Rehulka, P., Benada, O., Rehulkova, H., et al. (2013). Systems insight into the spore germination of Streptomyces coelicolor. J. Proteome Res. 12, 525–536. doi: 10.1021/pr300980v
Sun, X., Zhulin, I., and Wartell, R. M. (2002). Predicted structure and phyletic distribution of the RNA-binding protein Hfq. Nucleic Acids Res. 30, 3662–3671. doi: 10.1093/nar/gkf508
Swiercz, J. P., Hindra Bobek, J., Bobek, J., Haiser, H. J., Di Berardo, C., et al. (2008). Small non-coding RNAs in Streptomyces coelicolor. Nucleic Acids Res. 36, 7240–7251. doi: 10.1093/nar/gkn898
Taverniti, V., Forti, F., Ghisotti, D., and Putzer, H. (2011). Mycobacterium smegmatis RNase J is a 5'-3' exo-/endoribonuclease and both RNase J and RNase E are involved in ribosomal RNA maturation. Mol. Microbiol. 82, 1260–1276. doi: 10.1111/j.1365-2958.2011.07888.x
Thomason, M. K., and Storz, G. (2010). Bacterial antisense RNAs: how many are there, and what are they doing? Annu. Rev. Genet. 44, 167–188. doi: 10.1146/annurev-genet-102209-163523
Untergasser, A., Cutcutache, I., Koressaar, T., Ye, J., Faircloth, B. C., Remm, M., et al. (2012). Primer3–new capabilities and interfaces. Nucleic Acids Res. 40:e115. doi: 10.1093/nar/gks596
Van Dessel, W., Van Mellaert, L., Geukens, N., Lammertyn, E., and Anné, J. (2004). Isolation of high quality RNA from Streptomyces. J. Microbiol. Methods 58, 135–137. doi: 10.1016/j.mimet.2004.03.015
Viegas, S. C., Pfeiffer, V., Sittka, A., Silva, I. J., Vogel, J., and Arraiano, C. M. (2007). Characterization of the role of ribonucleases in Salmonella small RNA decay. Nucleic Acids Res. 35, 7651–7664. doi: 10.1093/nar/gkm916
Vockenhuber, M. P., Sharma, C. M., Statt, M. G., Schmidt, D., Xu, Z., Dietrich, S., et al. (2011). Deep sequencing-based identification of small non-coding RNAs in Streptomyces coelicolor. RNA Biol. 8, 468–477. doi: 10.4161/rna.8.3.14421
Waters, L. S., and Storz, G. (2009). Regulatory RNAs in bacteria. Cell 136, 615–628. doi: 10.1016/j.cell.2009.01.043
Xu, W., Huang, J., and Cohen, S. N. (2008). Autoregulation of AbsB (RNase III) expression in Streptomyces coelicolor by endoribonucleolytic cleavage of absB operon transcripts. J. Bacteriol. 190, 5526–5530. doi: 10.1128/JB.00558-08
Keywords: cis-antisense RNA, RNase III, Streptomyces, antibiotics, gene expression control
Citation: Šetinová D, Šmídová K, Pohl P, Musić I and Bobek J (2018) RNase III-Binding-mRNAs Revealed Novel Complementary Transcripts in Streptomyces. Front. Microbiol. 8:2693. doi: 10.3389/fmicb.2017.02693
Received: 30 August 2017; Accepted: 26 December 2017;
Published: 15 January 2018.
Edited by:
Dirk Tischler, Freiberg University of Mining and Technology, GermanyReviewed by:
Francis Repoila, Institut National de la Recherche Agronomique (INRA), FranceGerd M. Seibold, University of Ulm, Germany
Edgardo Sepulveda, Center for Scientific Research and Higher Education at Ensenada, Mexico
Copyright © 2018 Šetinová, Šmídová, Pohl, Musić and Bobek. This is an open-access article distributed under the terms of the Creative Commons Attribution License (CC BY). The use, distribution or reproduction in other forums is permitted, provided the original author(s) or licensor are credited and that the original publication in this journal is cited, in accordance with accepted academic practice. No use, distribution or reproduction is permitted which does not comply with these terms.
*Correspondence: Jan Bobek, amFuLmJvYmVrQGxmMS5jdW5pLmN6