- 1College of Biotechnology and Bioengineering, Zhejiang University of Technology, Hangzhou, China
- 2Institute of Microbiology and College of Life Sciences, Zhejiang University, Hangzhou, China
- 3College of Life Sciences, Nanchang University, Nanchang, China
Production of chromosome-encoded β-lactamases confers resistance to β-lactams in many Gram-negative bacteria. Some inducible β-lactamases, especially the class C β-lactamase AmpC in Enterobacteriaceae, share a common regulatory mechanism, the ampR-ampC paradigm. Induction of ampC is intimately linked to peptidoglycan recycling, and the LysR-type transcriptional regulator AmpR plays a central role in the process. However, our previous studies have demonstrated that the expression of class D β-lactamase gene blaA in Shewanella oneidensis is distinct from the established paradigm since an AmpR homolog is absent and major peptidoglycan recycling enzymes play opposite roles in β-lactamase expression. Given that lytic transglycosylases (LTs), a class of peptidoglycan hydrolases cleaving the β-1,4 glycosidic linkage in glycan strands of peptidoglycan, can disturb peptidoglycan recycling, and thus may affect induction of blaA. In this study, we investigated impacts of such enzymes on susceptibility to β-lactams. Deletion of three LTs (SltY, MltB and MltB2) increased β-lactam resistance, while four other LTs (MltD, MltD2, MltF, and Slt2) seemed dispensable to β-lactam resistance. The double LT mutants ΔmltBΔmltB2 and ΔsltYΔmltB2 had β-lactam resistance stronger than any of the single mutants. Deletion of ampG (encoding permease AmpG) and mrcA (encoding penicillin binding protein 1a, PBP1a) from both double LT mutants further increased the resistance to β-lactams. Notably, all increased β-lactam resistance phenotypes were in accordance with enhanced blaA expression. Although significant, the increase in β-lactamase activity after inactivating LTs is much lower than that produced by PBP1a inactivation. Our data implicate that LTs play important roles in blaA expression in S. oneidensis.
Introduction
Beta-lactam antibiotics are the most widely used group of antibiotics, they target the penicillin-binding proteins (PBPs), eventually disrupting the peptidoglycan synthesis. To combat these antibiotics, microorganisms have evolved multiple resistance mechanisms, including the direct inactivation or modification of antibiotics, protection of antibiotic targets, overexpression of drug efflux pumps, and reduction of permeability of the outer membrane (Blair et al., 2015). In Gram-negative bacteria, the production of β-lactamases is the predominant strategy of resistance to β-lactams. These enzymes, which resemble PBPs structurally and mechanistically, have ability to rapidly hydrolyze the β-lactams (Nicholas and Davies, 2012).
A great number of chromosome- and plasmid-mediated β-lactamases have been characterized. Expression of β-lactamases encoded by the chromosome is often inducible by β-lactam antibiotics. The regulatory mechanisms for β-lactamase production are intensively studied, especially for class C β-lactamase AmpC in several members of family Enterobacteriaceae (such as Enterobacter cloacae, Citrobacter freundii) (Lindberg et al., 1985; Jacobs et al., 1994, 1997) and Pseudomonas aeruginosa (Juan et al., 2006, 2017; Moya et al., 2009; Ropy et al., 2015). The induction of ampC is intimately linked to peptidoglycan recycling through LysR-type transcriptional regulator AmpR (the ampC-ampR paradigm). The peptidoglycan fragments, usually GlcNAc-1,6-anhMurNAc peptides, enter the cytoplasm across the inner membrane via permease AmpG. In the cytoplasm, these fragments are further processed by NagZ and AmpD, and then participate in peptidoglycan biosynthesis again. Two cytoplasmic peptidoglycan intermediates can act as regulatory ligands for ampC induction by binding to AmpR, resulting in either activation or repression of ampC expression.
In contrast to the ampC-ampR paradigm, our previous studies demonstrated that the regulatory mechanism for β-lactamase induction is distinct in Shewanella oneidensis MR-1, the most intensively studied strain in Shewanella genus (Yin et al., 2014). This genus belongs to the order “Alteromonadales” within the class “γ-proteobacteria”. Shewanella species are widely distributed in marine and freshwater environments and well-known for their diverse metabolic capabilities and versatile electron-accepting capacities (Fredrickson et al., 2008). Recently, Shewanella species are increasingly being implicated as human pathogens (Janda and Abbott, 2012; Cimmino et al., 2016). More importantly, bacteria of the genus Shewanella are regarded as a reservoir for antibiotic resistance, since several β-lactamases and Qnr-type quinolone resistance determinants have been isolated and characterized from this genus (Poirel et al., 2004). In S. oneidensis MR-1, the production of class D β-lactamase BlaA (encoded by the blaA gene) confers resistance to penicillins and the expression of blaA is induced by ampicillin (AMP) (Yin et al., 2013). However, a homolog of AmpR is absent in S. oneidensis and major peptidoglycan recycling enzymes (AmpG, NagZ and AmpD) have opposite effects for β-lactamase expression when compared to the ampC-ampR paradigm (Yin et al., 2014). Inactivation of PBP1a and its lipoprotein cofactor (LpoA) results in constitutive expression of blaA (Yin et al., 2015). Therefore, S. oneidensis contains an AmpG-independent, but PBP1a-dependent inducible pathway for blaA expression.
A common feature for the induction of β-lactamase genes is the involvement of peptidoglycan recycling. In contrast to the peptidoglycan recycling event in the cytoplasm, how the precursors of regulatory ligands are produced in the periplasm remains unclear. At least three classes of enzymes are responsible for peptidoglycan hydrolysis, including low-molecular-weight PBPs (LMW PBPs), N-acetylmuramoyl-L-alanine amidases and lytic transglycosylases (LTs) (van Heijenoort, 2011). Among them, LMW PBPs possess endopeptidase and/or carboxypeptidase activities, responsible for controlling the extent of cross-linking. In P. aeruginosa, inactivation of LMW PBPs increased ampC expression and β-lactam resistance (Moya et al., 2009; Ropy et al., 2015). N-acetylmuramoyl-L-alanine amidases liberate the peptides from the carboxyl of the lactyl moiety of MurNAc. LTs cleave the β-1,4 glycosidic linkage between GlcNAc and MurNAc resides, leading to the formation of GlcNAc-1,6-anhMurNAc peptides. The regulatory ligands for ampC induction are very likely derived from the function of LTs (Kraft et al., 1999). However, Gram-negative bacteria harbor multiple LTs that may be functionally redundant. For example, Escherichia coli encodes eight LTs (Yunck et al., 2016), while P. aeruginosa possesses 11 LTs (Lee et al., 2017). Based on the sequence similarities and identified consensus motifs, LTs are classified into families 1–4 (Blackburn and Clarke, 2001); two forms of LTs exist in bacteria, including soluble (sLTs) and membrane-bound (mLTs) LTs. Recent studies demonstrated that loss of SltB1 or MltB increases β-lactam resistance, whereas loss of Slt decreases resistance in P. aeruginosa (Cavallari et al., 2013; Lamers et al., 2015). In Stenotrophomonas maltophilia, inactivation of mltD1 confers a partial basal-level β-lactamase derepression phenotype (Huang et al., 2015). Besides, very little research has been focused on LTs and β-lactam resistance in other bacteria. The effect of LTs on blaA expression in S. oneidensis remains unknown.
In this study, our goal is to explore the roles of LTs in β-lactam resistance in S. oneidensis MR-1. S. oneidensis possesses seven genes that are predicted to encode LTs. Inactivation of three LTs [MltB(SO1166), SO1194, and SltY(SO2040)] increased the expression of blaA, resulted in improved resistance to β-lactams. Further studies suggested that these three LTs have additive effects for the expression of blaA and β-lactam resistance. Our results implicated that these LTs are involved in blaA expression and β-lactam resistance in S. oneidensis.
Materials and Methods
Bacterial Strains, Plasmids, and Culture Conditions
All bacterial strains and plasmids used in this study are listed in Table 1. All primers were synthesized by Sangon Biotech (Shanghai, China) and listed in Table S1. For normal growth, S. oneidensis and E. coli were cultivated aerobically in Luria-Bertani (LB) medium (Difco, Detroit, MI) at 30°C and 37°C, respectively. Where needed, the growth medium was supplemented with chemicals at the following concentrations: 2,6-diaminopimelic acid (DAP), 0.3 mM; ampicillin (AMP), 100 μg/mL; kanamycin (Km), 50 μg/mL; and gentamicin (Gm), 15 μg/mL. All chemicals were purchased from Sigma-Aldrich (Shanghai, China) unless otherwise noted.
In-Frame Deletion Mutagenesis and Complementation
In-frame deletion strains of S. oneidensis MR-1 were constructed by the att-based fusion PCR method (Jin et al., 2013). In brief, two fragments flanking the targeted gene were amplified by PCR with primers containing attB sequence or gene-specific sequence, and then joined together by an overlapping PCR method. The fusion fragments were introduced into plasmid pHGM01 by site-specific recombination, using BP Clonase (Invitrogen), and then transformed into E. coli WM3064 strain (DAP auxotroph). The resulting recombinant plasmids were transferred from E. coli WM3064 into the appropriate S. oneidensis strains via conjugation. Integration of the recombinant plasmid into the chromosome was selected by resistance to gentamicin and verified by PCR. The correct transconjugants were grown in LB broth in the absence of NaCl and plated onto LB medium supplemented with 10% sucrose. Gentamicin-sensitive and sucrose-resistant colonies were screened by PCR for deletion of the targeted gene. The deletion mutations were then verified by sequencing.
Plasmid pHG101 was used for genetic complementation of mutants (Wu et al., 2011). A fragment containing the gene of interest together with its native promoter was amplified by PCR and cloned into pHG101. The correct recombinant plasmids were transferred into its corresponding mutant strains via conjugation. The presence of recombinant plasmid was confirmed by plasmid purification and restriction enzyme digestion.
Growth of S. oneidensis
For measuring growth of different S. oneidensis strains, Overnight cultures were diluted 1:100 in 3 mL fresh LB medium and incubated at 200 rpm 30°C. The optical density at 600 nm (OD600) was recorded every 1 h. Then the generation times (G) were calculated according to the OD600 at the exponential stage.
Antibiotic Susceptibility Assay
Antibiotic susceptibility of S. oneidensis was determined with both liquid and solid cultures. Liquid cultures were utilized to determine the minimum inhibitory concentration (MIC) of the antibiotics (Yin et al., 2014). Antibiotics used in the MICs assay were AMP, cefotaxime (CTX), and imipenem (IPM). All MICs were determined at least in triplicate. Susceptibility assays on plates were also used to compare differences in AMP resistance among S. oneidensis strains. In this case, decimal dilution series were prepared. Three μL of each dilution was placed onto LB plates supplemented with AMP at different concentrations. The plates were incubated for 18 h at 30°C and then photographed.
Promoter Activity Assay
The activity of the promoter for each LT and blaA genes was determined by a markerless integrative lacZ reporter system, which we used to measure the activity of the blaA promoter (Yin et al., 2014, 2015). In brief, the wild type strains harboring different transcriptional fusion vector were cultivated to the exponential-phase ([OD600] of ≈ 0.4), then harvested by centrifugation at 4°C. After washed with phosphate-buffered saline (PBS) and treated with lysis buffer [0.25 M Tris-HCl (pH = 7.5), 0.5% Triton-X-100]. β-Galactosidase activity was determined by monitoring optical density at 420 nm using a Infinite M200 Pro microplate reader (Tecan) as previously described (Yin et al., 2014, 2015).
BlaA β-lactamase Activity Assay
The β-lactamase activity was measured directly by nitrocefin hydrolysis method, as described previously (Yin et al., 2014). In brief, bacterial cultures were cultivated to the early-exponential-phase [(OD600) of ≈ 0.2], and then 0 or 200 μg/mL AMP was added for blaA induction. The cultures were incubated for an additional 2 h at 30°C and 200 rpm. After incubation, 1 mL culture was centrifuged at 2,500 × g for 5 min, washed once with 1 mL 50 mM phosphate buffer (pH 7.0), and resuspended in 1 mL of the same buffer. Crude cell extracts were prepared by sonication (pulse on, 3 s; pulse off, 4 s; 10 times). The protein content of the crude extracts was determined using a Bradford assay with BSA as a standard (Bio-Rad). The nitrocefin hydrolysis assays were performed in 200 μL reaction mixtures containing 8 μg total protein and 4 μg nitrocefin (Calbiochem, San Diego, CA). Nitrocefin hydrolysis was measured every minute for 10 min at 25°C by absorbance at 486 nm. The specific BlaA β-lactamase activity was expressed in nanomoles of nitrocefin hydrolyzed per minute per milligram of protein. All experiments were performed in triplicate, and the results presented are averages for the three experiments.
Other Analyses
The experimental values were subjected to statistical analyses and are presented as means ± standard deviations. Student's t-test was performed for pairwise comparisons of groups.
Results
LTs in S. oneidensis MR-1
According to genome annotation, seven genes [mltB (SO1166), SO1994, sltY (SO2040), SO2564, mltF (SO3288), mltD (SO4017), and SO4660] are predicted to encode LTs in S. oneidensis MR-1. Except for SO4660, all LTs have a homolog in E. coli and P. aeruginosa (Table 2). SltY and MltF share 29 and 41% sequence identity with E. coli Slt70 and MltF, respectively. Interestingly, both MltB and SO1994 are E. coli MltB homologs (36 and 35% identity respectively); both SO2564 and MltD are homologous with E. coli MltD (34 and 33% identity respectively). These results indicate that S. oneidensis may produce two MltB and MltD isozymes. SO4660 is a protein of 239 amino acids (aa), which contains a transglycosylase SLT domain at the C-terminal region (115–217 aa). Blastp results demonstrate that SO4660 shares 37% identity and 51% similarity with P. aeruginosa Slt only at the C-terminal region (Query cover: 52%; E-value: 6e-17). The amino acids of SltY and SO4660 do not include a predicted lipoprotein process site, suggesting that they are soluble LTs. However, the rest LTs contain a predicted lipidation site [L(A/S)(G/A)C], a hallmark for lipoprotein (Tokuda and Matsuyama, 2004), implicating that these enzymes are membrane-bound lipoprotein. E. coli Slt70, MltF, MltB, MltD, and P. aeruginosa Slt belong to family 1A, 1E, 3, 1D, and 1A, respectively (Blackburn and Clarke, 2001). The consensus motifs of each family are also found in S. oneidensis counterparts (Figure S1). Therefore, based on sequence similarities and consensus motifs, we designated SO1994, SO2564, and SO4660 of S. oneidensis as mltB2, mltD2, and slt2, respectively. In total, S. oneidensis produces seven LTs, two from families 1A (SltY, Slt2), 1D (MltD and MltD2), and 3 (MltB and MltB2), one from family 1E (MltF).
To determine whether all these LTs are expressed, a markerless integrative lacZ-reporter system was employed to measure the promoter activity of each LT genes (Fu et al., 2014; Figure 1). Compared to the control, the expression of the β-galactosidase driven by each promoter was detectable in the wild type background. Among these, the promoter of mltD2 (PmltD2) had the highest activity to induce the expression of lacZ, while the promoter of mltF (PmltF) had the lowest activity. These results suggest that all LT genes are effectively expressed in our tested conditions.
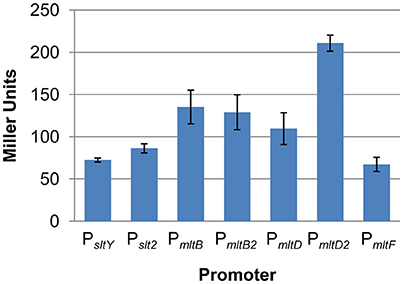
Figure 1. All LTs are expressed in S. oneidensis. Promoter activity of each LT gene was determined by measuring β-galactosidase (in Miller Units) using the markerless integrative lacZ reporter system in the wild type strain. Results are averages for at least three replicates, and the error bars represent standard derivation (SD).
Effects of LTs on Cell Growth
To understand the roles of LTs in S. oneidensis, strains lacking each gene were constructed individually. Firstly, we measured growth of each mutant in LB broth medium (Figure 2). Deletion of the mltB, mltD, mltD2, and slt2 genes had little impact on cell growth. In contrast, the other three mutants (the ΔsltY, ΔmltF, and ΔmltB2 strains) displayed obviously defects when grown in LB broth. Compared to the wild type, the generation time of the three mutants increased about 20 percent. Genetic complementation was then carried out with the multicopy plasmid pHG101 harboring a copy of sltY, mltF, or mltB2 under the control of their native promoters (Wu et al., 2011). The growth defect of the three mutants was fully recovered by expression of the corresponding genes in trans (Figure 2), confirming that growth defects observed in the three mutants were due to the mutation per se.
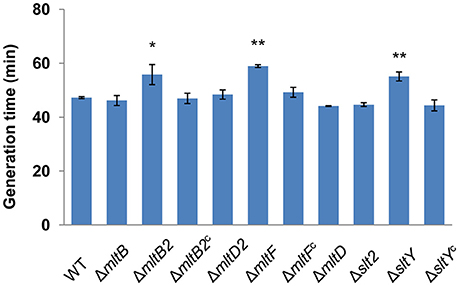
Figure 2. Effects of LTs on cell growth. The generation times for different strains were calculated from the OD600 values. ΔmltB2c, ΔmltFc and ΔsltYc represent ΔmltB2, ΔmltF and ΔsltY that were complemented with pHG101 in trans, respectively. Results are averages for at least three replicates, and the error bars represent standard derivation (SD) (*P < 0.05 and **P < 0.01, respectively; two-tailed t-test).
To assess whether growth defects are involved in impaired cell wall integrity, we observed cell shape and determined the susceptibility to hyposmolality. S. oneidensis is a typical rod-shaped bacterium and can grow normally in LB minus NaCl. Both cell shape and susceptibility to hyposmolality in all tested mutants were similar to those in the wild type (Figure S2). These results suggest that loss of single LT does not affect the cell wall integrity, growth defects of the ΔsltY, ΔmltF, and ΔmltB2 strains are result from other unknown reasons.
Deletion of sltY, mltB, and mltB2 Increases β-Lactam Resistance
Our previous study showed that major peptidoglycan recycling enzymes are involved in β-lactamase BlaA expression and β-lactam resistance in S. oneidensis (Yin et al., 2014). To study whether LTs are also play important roles in this process, the resistance to β-lactams in these LTs mutants were determined. Deletion of the mltF, mltD, mltD2 and slt2 genes did not affect the susceptibility to AMP. In contrast, the ΔsltY, ΔmltB2, and ΔmltB strains displayed significantly improved resistance to AMP (Figure 3A). Compared to the wild type, the ΔsltY, ΔmltB2, and ΔmltB strains had 8-, 8-, and 4-fold increases in resistance to AMP, respectively. Besides, the ΔsltY and ΔmltB2 strains had 2-fold increases in resistance to another two tested β-lactams, ceftotaxime (CTX) and imipenem (IMP) (Table 3).
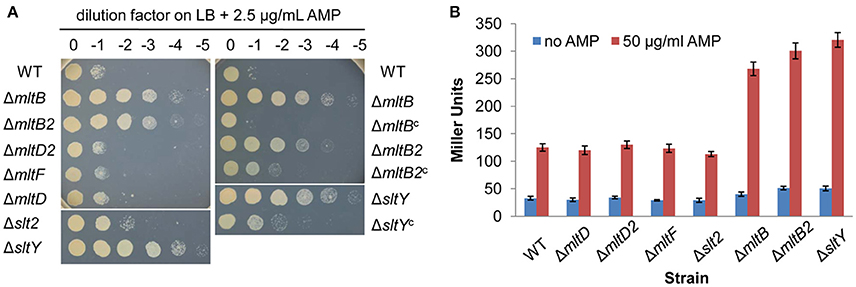
Figure 3. Inactivation of MltB, MltB2 and SltY increased the resistance to AMP. (A) AMP susceptibility assay for S. oneidensis strains lacking LTs. Cells of late-logarithmic phase (OD600 ≈ 0.6) were used to prepare a decimal dilution series. Three μL of each dilution was spotted onto LB plates supplemented with 2.5 μg/mL AMP. ΔmltBc, ΔmltB2c, and ΔsltYc represent ΔmltB, ΔmltB2, and ΔsltY that were complemented with pHG101 in trans, respectively. (B) Improved AMP resistance in ΔmltB, ΔmltB2, and ΔsltY strains is dependent on the expression of blaA. Promoter activity of the blaA gene (PblaA) was determined by measuring β-galactosidase (in Miller Units) using the PblaA-lacZ reporter system in the wild type and single LT gene deletion strains. Results are averages for at least three replicates, and the error bars represent standard derivation (SD).
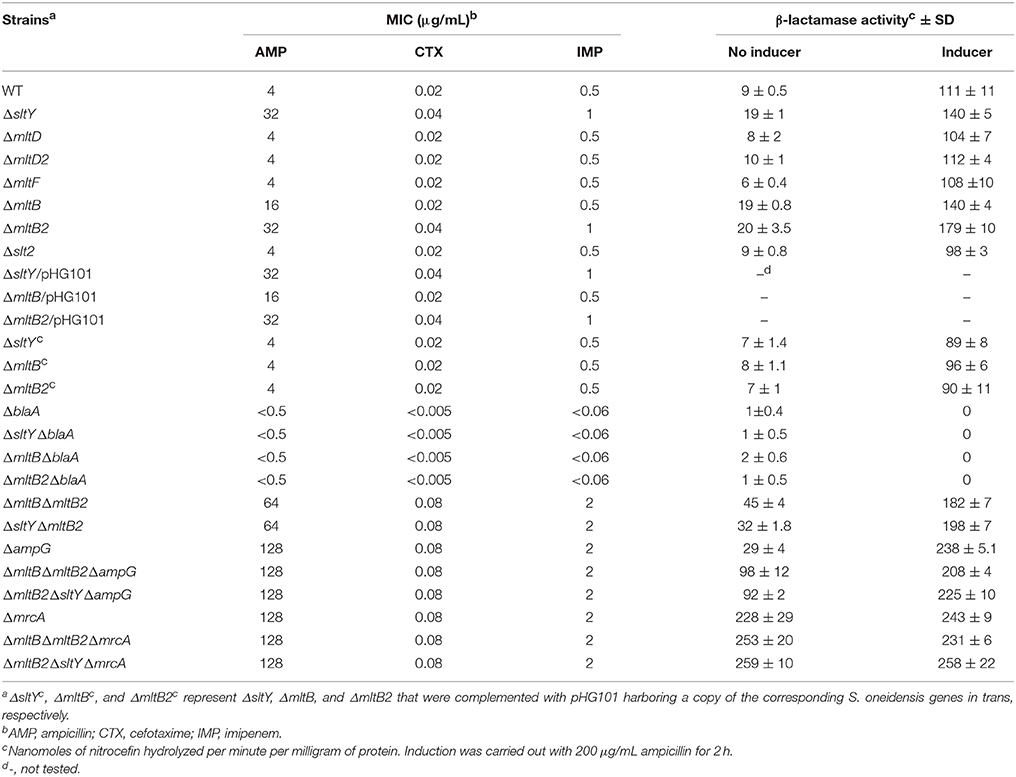
Table 3. MICs of β-lactams and specific activities of BlaA in S. oneidensis wild type and its derivate strains.
More importantly, expression of the sltY, mltB2, and mltB genes under the control of their native promoters recovered all β-lactam susceptibility to the level of the wild type, indicating that the phenotypes observed in these mutants was due to the intended mutations (Figure 3A and Table 3). These data, collectively, indicate that certain LTs (SltY, MltB2 and MltB) are important players mediating the β-lactam resistance in S. oneidensis.
Improved β-Lactam Resistance Is Dependent on BlaA
Production of β-lactamase BlaA confers S. oneidensis with natural resistance to certain β-lactams (Yin et al., 2013). In strains lacking AmpG or NagZ, two major peptidoglycan recycling enzymes, BlaA expression is significantly increased, leading to improved β-lactam resistance (Yin et al., 2014). It is reasonable that LTs-mediated β-lactam resistance is also dependent on BlaA.
To test this possibility, the lacZ reporter system was employed to determine the activity of the blaA promoter (PblaA) (Yin et al., 2014, 2015). As shown in the Figure 3B, the expression levels of the β-galactosidase driven by the PblaA in the ΔsltY, ΔmltB2 and ΔmltB strains were substantially higher than that in the wild type under all tested conditions. To confirm, the BlaA activity in all LTs mutants was measured by the nitrocefin hydrolysis method (Table 3; Yin et al., 2014). Our previously study found that β-lactamase activity was hardly detected in the ΔblaA strain under all tested conditions, implicating that nitrocefin assay is specific for BlaA in S. oneidensis. As expected, deletion of the mltF, mltD, mltD2, and slt2 genes did not affect the BlaA activities under all tested conditions. However, the ΔsltY, ΔmltB2, and ΔmltB strains had higher levels of BlaA activity. Compared to the wild type, the basal levels of BlaA activity of all these three mutants were increased ~2-fold, while the induced levels were increased 1.2~1.6-fold. Notably, expression of the sltY, mltB2 and mltB in the corresponding mutants significantly decreased the BlaA activities under all tested conditions.
In addition, the blaA gene was deleted from the ΔsltY, ΔmltB2, and ΔmltB strains. Similar to the ΔblaA strain, loss of BlaA completely abolished the β-lactam resistance and BlaA activities of the three LTs mutants (Table 3). Based on these results, we can conclude that the improved β-lactam resistance in the ΔsltY, ΔmltB2, and ΔmltB strains is dependent on BlaA.
Additive Effects of sltY, mltB, and mltB2 on β-Lactam Resistance
Given that different LTs appear to play functionally redundant roles in peptidoglycan degradation, we supposed that LTs may have additive effects on blaA expression. To confirm, the ΔmltBΔmltB2 and ΔsltYΔmltB2 strains were constructed and subjected to β-lactam susceptibility testing. As shown in Figure 4 and Table 3, both two strains had increased β-lactam resistance compared to the single mutants (ΔmltB, ΔmltB2, and ΔsltY). When compared to the ΔmltB and ΔmltB2 strains, the MICs of the ΔmltBΔmltB2 strain for all three β-lactams (AMP, CTX, and IMP) were increased 4- and 2-fold, respectively. The ΔsltYΔmltB2 strain had 2-fold increases in resistance to these β-lactams compared to the single mutants.
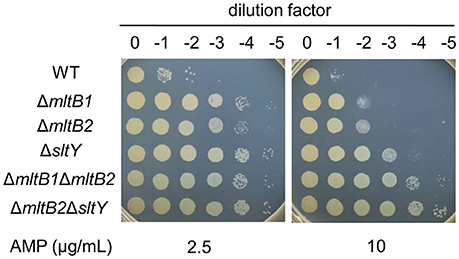
Figure 4. LTs have additive effects on AMP resistance. Cells of late-logarithmic phase (OD600 ≈ 0.6) were used to prepare a decimal dilution series. Three μL of each dilution was spotted onto LB plates supplemented with 2.5 or 10 μg/mL AMP.
Consistently, both the basal and induced levels of BlaA activity were increased in the two double mutants. The ΔmltBΔmltB2 and ΔsltYΔmltB2 strains had basal levels of BlaA activity ~2.2- and ~1.7-fold higher than either of its single-mutation parent strains, respectively. By induction, the BlaA activity of the ΔmltBΔmltB2 strain was 1.3-fold higher than that of the ΔmltB strain. Similarly, the BlaA activity of the ΔsltYΔmltB2 strain was ~1.4-fold higher than that of the ΔsltY strain. These results suggest that SltY, MltB and MltB2 have additive effect on blaA expression and β-lactam resistance.
Loss of AmpG Enhances LTs-Mediated BlaA Activity at Basal Levels
In S. oneidensis, loss of AmpG increases the basal level of blaA expression, implying that intermediate products transported across AmpG permease are likely to be the repressors rather than inducers for blaA expression (Yin et al., 2014). To evaluate the effects of AmpG on LTs-mediated blaA expression, the ΔmltBΔmltB2ΔampG and ΔsltYΔmltB2ΔampG strains were constructed for antibiotic susceptibility testing (Table 3). The two strains, together with the ΔampG strain, displayed similar levels of resistance to all tested β-lactams, which had 2-fold increases in resistance to AMP when compared to the strains lacking two LTs (ΔmltBΔmltB2 and ΔsltYΔmltB2). Notably, deletion of ampG from the two double mutants increased the basal levels of BlaA activity. Therefore, loss of AmpG further increases the basal levels of blaA expression and β-lactam resistance in strains lacking LTs.
PBP1a-Mediated Induction of blaA Expression Is Independent of the LTs
Our previous study demonstrated that deletion of mrcA (encoding PBP1a) drastically enhances blaA expression in S. oneidensis even in the absence of β-lactams, which is independent of AmpG (Yin et al., 2015). Although significant, the increase in β-lactamase activity after inactivating LTs is much lower than that produced by PBP1a inactivation. To explore the involvement of LTs in PBP1a-mediated blaA expression, we deleted the mrcA gene from both ΔmltBΔmltB2 and ΔsltYΔmltB2 strains and performed the antibiotic susceptibility testing (Table 3). The resistance to β-lactams of both the ΔmltBΔmltB2ΔmrcA and ΔsltYΔmltB2ΔmrcA strains was identical to those of the ΔmrcA strain. Compared to the strains lacking two LTs, both the two triple mutants had 2-fold increases in resistance to AMP. Consistently, deletion of mrcA significantly increased the basal levels of BlaA activity of the strains lacking two LTs. Both the basal and induced levels of BlaA activity of the triple mutants were comparable to those of the ΔmrcA strain (Table 3). These results suggest that PBP1a-mediated blaA expression is independent of LTs. In other words, PBP1a functionally overrides LTs in blaA expression.
Discussion
The regulatory mechanism for blaA induction in S. oneidensis is distinct from the ampR-ampC paradigm. Nonetheless, our previous studies demonstrated that the expression of blaA is also related to peptidoglycan maintenance, turnover and recycling (Yin et al., 2013, 2014, 2015). In contrast to the ampR-ampC paradigm, inactivation of major peptidoglycan recycling enzymes, AmpG and NagZ, improved the blaA expression and subsequent β-lactam resistance. Here we evaluated whether LTs are involved in β-lactam resistance in S. oneidensis. This bacterium harbors seven putative LTs. All these LTs are effectively expressed in the wild type background. Our results demonstrated that only family 1 LT, SltY and family 3 LTs, MltB and MltB2, are related to the blaA expression. Loss of these LTs improves the BlaA activities under basal and induced conditions, resulting in increased β-lactam resistances.
S. oneidensis LTs also play different roles in bacterial cell growth. Strains lacking SltY, MltB2 or MltF displayed a certain growth defect, while other LTs have no effects on growth. Our results suggest that S. oneidensis LTs have different physiological functions, although they appear to be redundant in cleavage of glycan strains. Notably, MltB and MltB2 are both family 3 LTs, their roles in cell growth are also different. Consistently, the distinct roles of LTs have been observed in other bacteria. For example, Salmonella enterica serovar Typhimurium contains seven putative family 1 LTs, whereas only two of them, MltE and MltC, are specifically involved in the regulation of biofilm formation (Monteiro et al., 2011). At least three reasons might contribute to the distinct roles of LTs. Firstly, the expression of LTs may be changed by different growth phase and physiological conditions. Secondly, two forms of LTs (soluble and membrane-bound) may carry out either exolytic or endolytic reaction. In addition, it is possible that LTs have different substrates preferences, which have been observed in E. coli (Lee et al., 2013).
The effects of LTs on β-lactam resistance have been evaluated in some other ampR-ampC harboring Gram-negative bacteria. In P. aeruginosa, loss of family 3 LTs SltB1 and MltB increases β-lactam resistance, while loss of Slt decreases β-lactam resistance. However, inactivation of these LTs did not affect the uninduced and induced AmpC activities (Cavallari et al., 2013; Lamers et al., 2015). On the contray, deletion of mltD1 increased the uninduced β-lactamase activity in Stenotrophomonas maltophilia, which results from mltB1 and mltD2 upexpression and depends on the ampG-nagZ-ampR regulatory circuit (Huang et al., 2015; Wu et al., 2016). Notably, inactivation of LTs in these bacteria compromise cell envelope integrity, results in susceptibility to β-lactams and other antibiotics (Lamers et al., 2015; Wu et al., 2016).
Our studies on S. oneidensis showed that deletion of three LTs (MltB, MltB2 and SltY) increase β-lactam resistance. However, the underlying mechanism is quite different from that in P. aeruginosa or S. maltophilia. The upregulation of blaA contributes to LTs-mediated β-lactam resistance in S. oneidensis. The regulatory mechanism for blaA induction is unique because of the lack of an AmpR homolog (Yin et al., 2014). More importantly, S. oneidensis contains an AmpG-NagZ-dependent repressible pathway and AmpG-independent inducible pathway for blaA expression, since loss of AmpG increases the basal level of BlaA activity and remains inducible (Figure 5; Yin et al., 2014). Peptidoglycan degradation fragments transported by AmpG might function as repressors (rather than inducers for the ampR-ampC paradigm) for blaA induction. LTs are responsible for the cleavage of glycan strands and production of GlcNAc-1,6-anhMurNAc peptides. It is reasonable that the repressors for blaA induction are derived from these LTs. Although the AmpR homolog is absent in S. oneidensis, genome annotation demonstrated that this bacterium possesses at least 22 putative LysR-type transcriptional regulators. Unlike AmpR, certain regulators could bind to these repressors, thus inhibiting β-lactam expression in the absence of inducer. In general, the AmpG-NagZ-dependent repressible pathway for blaA expression is also LTs dependent.
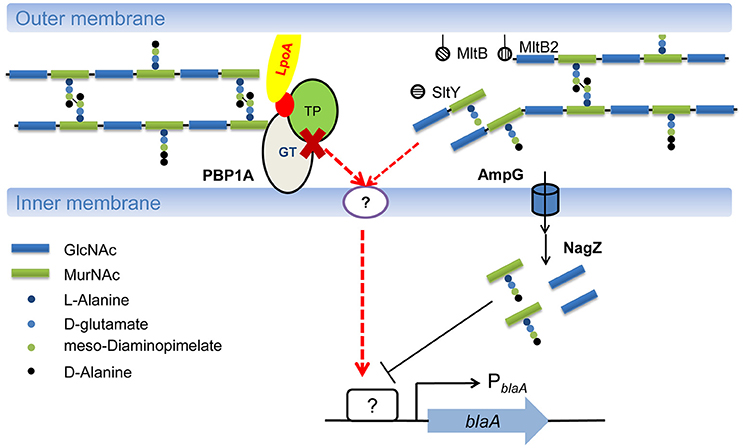
Figure 5. Model of regulatory mechanism for blaA induction in S. oneidensis. S. oneidensis possesses two pathways for blaA regulation. One is an AmpG-NagZ-dependent repressible pathway, which is also LT dependent. Another one is AmpG-NagZ-independent inducible pathway, which is dependent on PBP1a. PBP1a is responsive to the presence of antibiotics in the periplasm, impaired peptidoglycan production by PBP1a compromises cell envelope integrity, and then activates an unknown TCS, eventually results in expression of blaA in S. oneidensis. Moreover, it cannot be excluded that LTs inactivation disturbs peptidoglycan homeostasis, which is then monitored an unknown TCS to induce the expression of blaA.
Given that inactivation of mrcA (encoding PBP1a) derepresses the expression of blaA in the strain lacking AmpG, the AmpG-independent inducible pathway for blaA expression may be dependent on PBP1a (Yin et al., 2015). In this study, we found that PBP1a also functionally overrides LTs in blaA expression, implicating that PBP1a-depedent inducible pathway is LTs independent. As the primary target for β-lactams, PBP1a is responsive to the presence of antibiotics in the periplasm. In P. aeruginosa, inactivation of a nonessential PBP triggers the activation of CreBC two-component system (TCS), which in turn play an important role in β-lactam resistance (Zamorano et al., 2014). In parallel, BlrAB, a homolog of CreBC, are involved in β-lactamase expression and β-lactam resistance in Aeromonas spp. (Niumsup et al., 2003). Recently, TCSs that sense β-lactams (VbrKR) or cell wall damage (WigKR) have been characterized in Vibrio, which are responsible for β-lactam resistance or tolerance (Dörr et al., 2016; Li et al., 2016). However, the VbrKR and CreBC/BlrAB homologs are absent in S. oneidensis. It is notable that S. oneidensis possesses a large number of TCSs, including Cpx and Rcs (Laubacher and Ades, 2008; Weatherspoon-Griffin et al., 2010), two systems that monitor cell envelope stress. It is likely that impaired peptidoglycan production by PBP1a compromises cell envelope integrity, and then activates an unknown TCS, eventually results in expression of blaA in S. oneidensis (Figure 5).
A recent study showed that inactivation of LTs alters the outer membrane permeability in S. maltophilia (Wu et al., 2016). Although our results suggested that deletion of LTs in S. oneidensis did not affect cell wall integrity, it cannot be excluded that LT inactivation disturbs peptidoglycan homeostasis, which is then monitored an unknown TCS to induce the expression of blaA (Figure 5).
It has been proposed that the combination of LT inhibitors and β-lactams can be used to treat ampR-ampC harboring pathogens (Kraft et al., 1999). However, the LTs in different bacteria may play different roles in β-lactam resistance. Especially, loss of three LTs in S. oneidensis increased the expression of class D β-lactamase BlaA, which is regarded as progenitor of carbapenem-hydrolyzing oxacillinase in clinically relevant Gram-negative pathogens (Poirel et al., 2004). Therefore, it is importance to understand the roles of LTs in β-lactam resistance in bacteria that are phylogenetically more diverse. In addition, the species specific effects of LT inhibitors should be carefully considered.
Author Contributions
JY, ZY, JQ, and HG analyzed data, conceived the idea and designed the project. JY, YYS, and YJS carried out the experiments. JY and HG wrote the paper.
Conflict of Interest Statement
The authors declare that the research was conducted in the absence of any commercial or financial relationships that could be construed as a potential conflict of interest.
Acknowledgments
This research was supported by the National Natural Science Foundation of China (31600041, 41476105), the Natural Science Foundation of Jiangxi Province of China (20161BAB214156) and the Department of Education of JiangXi Province (GJJ150075).
Supplementary Material
The Supplementary Material for this article can be found online at: https://www.frontiersin.org/articles/10.3389/fmicb.2018.00013/full#supplementary-material
References
Blackburn, N. T., and Clarke, A. J. (2001). Identification of four families of peptidoglycan lytic transglycosylases. J. Mol. Evol. 52, 78–84. doi: 10.1007/s002390010136
Blair, J. M., Webber, M. A., Baylay, A. J., Ogbolu, D. O., and Piddock, L. J. (2015). Molecular mechanisms of antibiotic resistance. Nat. Rev. Microbiol. 13, 42–51. doi: 10.1038/nrmicro3380
Cavallari, J. F., Lamers, R. P., Scheurwater, E. M., Matos, A. L., and Burrows, L. L. (2013). Changes to its peptidoglycan-remodeling enzyme repertoire modulate β-lactam resistance in Pseudomonas aeruginosa. Antimicrob. Agents Chemother. 57, 3078–3084. doi: 10.1128/AAC.00268-13
Cimmino, T., Olaitan, A. O., and Rolain, J. M. (2016). Whole genome sequence to decipher the resistome of Shewanella algae, a multidrug-resistant bacterium responsible for pneumonia, Marseille, France. Expert Rev. Anti Infect. Ther. 14, 269–275. doi: 10.1586/14787210.2016.1106936
Dörr, T., Alvarez, L., Delgado, F., Davis, B. M., Cava, F., and Waldor, M. K. (2016). A cell wall damage response mediated by a sensor kinase/response regulator pair enables beta-lactam tolerance. Proc. Natl. Acad. Sci. U.S.A. 113, 404–409. doi: 10.1073/pnas.1520333113
Fredrickson, J. K., Romine, M. F., Beliaev, A. S., Auchtung, J. M., Driscoll, M. E., Gardner, T. S., et al. (2008). Towards environmental systems biology of Shewanella. Nat. Rev. Microbial. 6, 592–603. doi: 10.1038/nrmicro1947
Fu, H., Jin, M., Ju, L., Mao, Y., and Gao, H. (2014). Evidence for function overlapping of CymA and the cytochrome bc1 complex in the Shewanella oneidensis nitrate and nitrite respiration. Environ. Microbiol. 16, 3181–3195. doi: 10.1111/1462-2920.12457
Huang, Y. W., Wu, C. J., Hu, R. M., Lin, Y. T., and Yang, T. C. (2015). Interplay among membrane-bound lytic transglycosylase D1, the CreBC two-component regulatory system, the AmpNG-AmpDI-NagZ-AmpR regulatory circuit, and L1/L2 β-lactamase expression in Stenotrophomonas maltophilia. Antimicrob. Agents Chemother. 59, 6866–6872. doi: 10.1128/AAC.05179-14
Jacobs, C., Frère, J. M., and Normark, S. (1997). Cytosolic intermediates for cell wall biosynthesis and degradation control inducible beta-lactam resistance in gram-negative bacteria. Cell 88, 823–832. doi: 10.1016/S0092-8674(00)81928-5
Jacobs, C., Huang, L. J., Bartowsky, E., Normark, S., and Park, J. T. (1994). Bacterial cell wall recycling provides cytosolic muropeptides as effectors for beta-lactamase induction. EMBO J. 13, 4684–4694.
Janda, J. M., and Abbott, S. L. (2012). The genus Shewanella: from the briny depths below to human pathogen. Crit. Rev. Microbiol. 40, 293–312. doi: 10.3109/1040841X.2012.726209
Jin, M., Jiang, Y., Sun, L., Yin, J., Fu, H., Wu, G., et al. (2013). Unique organizational and functional features of the cytochrome c maturation system in Shewanella oneidensis. PLoS ONE 8:e75610. doi: 10.1371/journal.pone.0075610
Juan, C., Moyá, B., Pérez, J. L., and Oliver, A. (2006). Stepwise upregulation of the Pseudomonas aeruginosa chromosomal cephalosporinase conferring high-level β-lactam resistance involves three AmpD homologues. Antimicrob. Agents Chemother. 50, 1780–1787. doi: 10.1128/AAC.50.5.1780-1787.2006
Juan, C., Torrens, G., González-Nicolau, M., and Oliver, A. (2017). Diversity and regulation of intrinsic β-lactamases from non-fermenting and other Gram-negative opportunistic pathogens, FEMS Microbiol. Rev. 41, 781–815. doi: 10.1093/femsre/fux043
Kraft, A. R., Prabhu, J., Ursinus, A., and Höltje, J. V. (1999). Interference with murein turnover has no effect on growth but reduces beta-lactamase induction in Escherichia coli. J. Bacteriol. 181, 7192–7198.
Lamers, R. P., Nguyen, U. T., Ylan, N., Buensuceso, R. N. C., and Burrows, L. L. (2015). Loss of membrane-bound lytic transglycosylases increases outer membrane permeability and β-lactam sensitivity in Pseudomonas aeruginosa. Microbiologyopen 4, 879–895. doi: 10.1002/mbo3.286
Laubacher, M. E., and Ades, S. E. (2008). The Rcs phosphorelay is a cell envelope stress response activated by peptidoglycan stress and contributes to intrinsic antibiotic resistance. J. Bacteriol. 190, 2065–2074. doi: 10.1128/JB.01740-07
Lee, M., Hesek, D., Dik, D. A., Fishovitz, J., Lastochkin, E., Boggess, B., et al. (2017). From genome to proteome to elucidation of reactions for all eleven known lytic transglycosylases from Pseudomonas aeruginosa. Angew. Chem. Int. Ed. 56, 2735–2739. doi: 10.1002/anie.201611279
Lee, M., Hesek, D., Llarrull, L. I., Lastochkin, E., Pi, H., Boggess, B., et al. (2013). Reactions of all E. coli lytic transglycosylases with bacterial cell wall. J. Am. Chem. Soc. 135, 3311–3314. doi: 10.1021/ja309036q
Li, L., Wang, Q., Zhang, H., Yang, M., Khan, M. I., and Zhou, X. (2016). Sensor histidine kinase is a β-lactam receptor and induces resistance to β-lactam antibiotics. Proc. Natl. Acad. Sci. U.S.A. 113, 1648–1653. doi: 10.1073/pnas.1520300113
Lindberg, F., Westman, L., and Normark, S. (1985). Regulatory components in Citrobacter freundii ampC beta-lactamase induction. Proc. Natl. Acad. Sci. U.S.A. 82, 4620–4624. doi: 10.1073/pnas.82.14.4620
Monteiro, C., Fang, X., Ahmad, I., Gomelsky, M., and Römling, U. (2011). Regulation of biofilm components in Salmonella enterica Serovar Typhimurium by lytic transglycosylases involved in cell wall turnover. J. Bacteriol. 193, 6443–6451. doi: 10.1128/JB.00425-11
Moya, B., Dötsch, A., Juan, C., Blázquez, J., Zamorano, L., Haussler, S., et al. (2009). β-lactam resistance response triggered by inactivation of a nonessential penicillin-binding protein. PLoS Pathog. 5:e1000353. doi: 10.1371/journal.ppat.1000353
Nicholas, R. A., and Davies, C. (2012). “Structural mechanisms of β-lactam antibiotic resistance in penicillin-binding proteins” in Antibiotic Discovery and Development, ed T. J. Dougherty and M. J. Pucci (New York, NY: Springer US), 397–425.
Niumsup, P., Simm, A. M., Nurmahomed, K., Walsh, T. R., Bennett, P. M., and Avison, M. B. (2003). Genetic linkage of the penicillinase gene, amp, and blrAB, encoding the regulator of beta-lactamase expression in Aeromonas spp. J. Antimicrob. Chemother. 51, 1351–1358. doi: 10.1093/jac/dkg247
Poirel, L., Héritier, C., and Nordmann, P. (2004). Chromosome-encoded Ambler class D β-lactamase of Shewanella oneidensis as a progenitor of carbapenem-hydrolyzing oxacillinase. Antimicrob. Agents Chemother. 48, 348–351. doi: 10.1128/AAC.48.1.348-351.2004
Ropy, A., Cabot, G., SánchezDiener, I., Aguilera, C., Moya, B., Ayuan, J. A., et al. (2015). Role of Pseudomonas aeruginosa low-molecular-mass penicillin-binding proteins in AmpC expression, β-lactam resistance, and peptidoglycan structure. Antimicrob. Agents Chemother. 59, 3925–3934. doi: 10.1128/AAC.05150-14
Tokuda, H., and Matsuyama, S. I. (2004). Sorting of lipoproteins to the outer membrane in E. coli. Biochim. Biophys. Acta 1693, 5–13. doi: 10.1016/j.bbamcr.2004.02.005
van Heijenoort, J. (2011). Peptidoglycan hydrolases of Escherichia coli. Microbiol. Mol. Biol. Rev. 75, 636–663. doi: 10.1128/MMBR.00022-11
Weatherspoon-Griffin, N., Zhao, G., Kong, W., Kong, Y., Morigen, M., Andrewspolymenis, H., et al. (2010). The CpxR/CpxA two-component system upregulates two Tat-dependent peptidoglycan amidases to confer bacterial resistance to antimicrobial peptide. J. Biol. Chem. 286, 5529–5539. doi: 10.1074/jbc.M110.200352
Wu, C. J., Huang, Y. W., Lin, Y. T., and Yang, T. C. (2016). Inactivation of lytic transglycosylases increases susceptibility to aminoglycosides and macrolides by altering the outer membrane permeability of Stenotrophomonas maltophilia. Antimicrob. Agents Chemother. 60, 3236–3239. doi: 10.1128/AAC.03026-15
Wu, L., Wang, J., Tang, P., Chen, H., and Gao, H. (2011). Genetic and molecular characterization of flagellar assembly in Shewanella oneidensis. PLoS ONE 6:e21479. doi: 10.1371/journal.pone.0021479
Yin, J., Mao, Y., Ju, L., Jin, M., Sun, Y., Jin, S., et al. (2014). Distinct roles of major peptidoglycan recycling enzymes in beta-lactamase production in Shewanella oneidensis. Antimicrob. Agents Chemother. 58, 6536–6543. doi: 10.1128/AAC.03238-14
Yin, J., Sun, L., Dong, Y., Chi, X., Zhu, W., Qi, S. H., et al. (2013). Expression of blaA underlies unexpected ampicillin-induced cell lysis of Shewanella oneidensis. PLoS ONE 8:e60460. doi: 10.1371/journal.pone.0060460
Yin, J., Sun, Y., Mao, Y., Jin, M., and Gao, H. (2015). PBP1a/LpoA but not PBP1b/LpoB are involved in regulation of the major β-lactamase gene blaA in Shewanella oneidensis. Antimicrob. Agents Chemother. 59, 3357–3364. doi: 10.1128/AAC.04669-14
Yunck, R., Cho, H., and Bernhardt, T. G. (2016). Identification of MltG as a potential terminase for peptidoglycan polymerization in bacteria. Mol. Microb. 99, 700–718. doi: 10.1111/mmi.13258
Zamorano, L., Moyà, B., Juan, C., Mulet, X., Blázquez, J., and Oliver, A. (2014). The Pseudomonas aeruginosa CreBC two-component system plays a major role in the response to β-lactams, fitness, biofilm growth, and global regulation. Antimicrob. Agents Chemother. 58, 5084–5095. doi: 10.1128/AAC.02556-14
Keywords: lytic transglycosylases, β-lactamase, peptidoglycan, Shewanella oneidensis, resistance mechanisms
Citation: Yin J, Sun Y, Sun Y, Yu Z, Qiu J and Gao H (2018) Deletion of Lytic Transglycosylases Increases Beta-Lactam Resistance in Shewanella oneidensis. Front. Microbiol. 9:13. doi: 10.3389/fmicb.2018.00013
Received: 01 September 2017; Accepted: 05 January 2018;
Published: 22 January 2018.
Edited by:
Jose L. Martinez, Consejo Superior de Investigaciones Científicas (CSIC), SpainReviewed by:
Antonio Oliver, Hospital Universitario Son Dureta, SpainAlexander J. F. Egan, Newcastle University, United Kingdom
Copyright © 2018 Yin, Sun, Sun, Yu, Qiu and Gao. This is an open-access article distributed under the terms of the Creative Commons Attribution License (CC BY). The use, distribution or reproduction in other forums is permitted, provided the original author(s) or licensor are credited and that the original publication in this journal is cited, in accordance with accepted academic practice. No use, distribution or reproduction is permitted which does not comply with these terms.
*Correspondence: Jianhua Yin, amlhbmh1YXlAemp1dC5lZHUuY24=
Haichun Gao, aGFpY2h1bmdAemp1LmVkdS5jbg==