- 1Department of Parasitology, Institute of Biomedical Sciences, University of São Paulo, São Paulo, Brazil
- 2Department of Entomology, University of Antananarivo, Antananarivo, Madagascar
- 3Centro de Ciências Biológicas e da Saúde, Universidade Federal do Mato Grosso do Sul, Campo Grande, Brazil
- 4Laboratorio de Investigaciones en Parasitología Tropical (LIPT), University of Tolima, Ibagué, Colombia
- 5Department of Parasitology, University of Los Andes, Mérida, Venezuela
- 6Centro de Biotecnologia, Eduardo Mondlane University, Maputo, Mozambique
- 7Department of Veterinary Tropical Diseases, Faculty of Veterinary Science, University of Pretoria, Pretoria, South Africa
This study is about the inter- and intra-specific genetic diversity of trypanosomatids of the genus Angomonas, and their association with Calliphoridae (blowflies) in Neotropical and Afrotropical regions. Microscopic examination of 3,900 flies of various families, mostly Calliphoridae, revealed that 31% of them harbored trypanosomatids. Small subunit rRNA (SSU rRNA) barcoding showed that Angomonas predominated (46%) over the other common trypanosomatids of blowflies of genera Herpetomonas and Wallacemonas. Among Angomonas spp., A. deanei was much more common than the two-other species, A. desouzai and A. ambiguus. Phylogenetic analyses based on SSU rRNA, glycosomal glyceraldehyde-3-phosphate dehydrogenase (gGAPDH) and internal transcribed spacer rDNA (ITS rDNA) sequences revealed a marked genetic diversity within A. deanei, which comprised four infraspecific genotypes (Dea1–Dea4), and four corresponding symbiont genotypes (Kcr1–Kcr4). Host and symbiont phylogenies were highly congruent corroborating their co-divergence, consistent with host-symbiont interdependent metabolism and symbiont reduced genomes shaped by a long coevolutionary history. We compared the diversity of Angomonas/symbionts from three genera of blowflies, Lucilia, Chrysomya and Cochliomyia. A. deanei, A. desouzai, and A. ambiguus were found in the three genera of blowflies in South America. In Africa, A. deanei and A. ambiguus were identified in Chrysomya. The absence of A. desouzai in Africa and its presence in Neotropical Cochliomyia and Lucilia suggests parasite spillback of A. desouzai into Chrysomya, which was most likely introduced four decades ago from Africa into the Neotropic. The absence of correlation between parasite diversity and geographic and genetic distances, with identical genotypes of A. deanei found in the Neotropic and Afrotropic, is consistent with disjunct distribution due to the recent human-mediated transoceanic dispersal of Angomonas by Chrysomya. This study provides the most comprehensive data gathered so far on the genetic repertoires of a genus of trypanosomatids found in flies from a wide geographical range.
Introduction
The family Trypanosomatidae (Euglenozoa, Kinetoplastea) is comprised of flagellates that are parasites of vertebrates, plants, and insects. Insects can host trypanosomatid parasites of their own, but they also allow for the cyclical development and transmission of trypanosomatids to plants and vertebrates (Wallace, 1966, 1979; Camargo, 1999; Maslov et al., 2013; Lukeš et al., 2014). Four genera of insect trypanosomatids harbor bacterial endosymbionts: Angomonas, Strigomonas (Teixeira et al., 2011), Kentomonas (Votýpka et al., 2014), and Novymonas (Kostygov et al., 2016). The first three genera constitute the subfamily Strigomonadinae (Votýpka et al., 2014), henceforward referred as symbiont-harboring trypanosomatids (SHTs). The obligate endosymbionts of Angomonas species belong to the Betaproteobacteria (Teixeira et al., 2011; Alves et al., 2013a). They are known as trypanosomatid proteobacterial endosymbionts (TPEs), and include two Candidatus species, Ca. Kinetoplastibacterium desouzai, and Ca. K. crithidii (Teixeira et al., 2011).
Trypanosomatid proteobacterial endosymbionts are a model study for symbiosis evolution, they live in the trypanosomatid cytoplasm, replicate synchronically with their hosts, re-shape cell structures, and are vertically transmitted (Freymuller and Camargo, 1981; Motta et al., 2010; Morales et al., 2016). The symbiont-trypanosomatid relationship is characterized by mutually dependent due to reciprocal advantages of their metabolic interactions. Symbionts cannot grow outside of the host trypanosomatid reflecting the limitations imposed by genome streamlining resulting from relaxed selection on genes that are superfluous in the intracellular environment. Nevertheless, the gene arsenal of the symbionts renders their trypanosomatid hosts autotrophic for hemin, essential amino acids and vitamins (Alves et al., 2011, 2013a,b; Klein et al., 2013; Motta et al., 2013). Antibiotic selective elimination of symbionts impairs the metabolic capabilities and growth of the trypanosomatid partner, rendering it unable to colonize insects (Roitman and Camargo, 1985; Catta-Preta et al., 2013). The interdependent metabolic association between hosts and symbionts reflects an ancient co-evolutionary history of the SHTs, as suggested by codivergence supported by congruence between SHT and TPE phylogenies (Teixeira et al., 2011).
Angomonas comprises just three species: A. deanei, A. desouzai, and A. ambiguus (Mundim et al., 1974; Fiorini et al., 1989; Teixeira et al., 2011). Despite being one of the most studied insect trypanosomatids, the single isolate of A. deanei analyzed was obtained more than 30 years ago (Mundim et al., 1974). In a previous study, a small sampling of blowflies of Lucilia and Chrysomya captured in different Brazilian biomes was found to harbor A. deanei, A. desouzai, and the new species A. ambiguus (Teixeira et al., 2011).
Calliphorids (blowflies) currently existing in the Neotropical region are either native or invading flies (McDonagh and Stevens, 2011). Until the 1960s, the most abundant calliphorids in Brazil were Lucilia eximia and screwworm flies of Cochliomyia, in addition to the cosmopolitan Lucilia sericata and Lucilia cuprina (Aubertin, 1933; Mello, 1961). Most species of Lucilia are limited to determined continents while very few are cosmopolitan such as L. sericata (Williams et al., 2016). Cochliomyia spp. are endemic to the Americas and two species are common in South America: Cochliomyia hominivorax that was eradicated from North and Central America and Cochliomyia macellaria dispersed from Argentina to Canada (Yusseff-Vanegas and Agnarsson, 2016). In the 1970s, blowflies of Chrysomya arrived in Brazil from Africa (Imbiriba et al., 1978; Guimarães et al., 1978), and shortly dispersed throughout the Latin America reaching North America a few years later (Baumgartner and Greenberg, 1984). Invading blowflies may have transmitted trypanosomatids from their native range to flies of the invaded areas (parasite spillover), and may have become infected with trypanosomatids that already existed in the newly occupied areas (parasite spillback) (Kelly et al., 2009; Wells et al., 2015).
Previous finding of native Lucilia and African Chrysomya captured in Brazil infected with Angomonas spp. (Teixeira et al., 2011), and systematic surveys we have carried out of insect trypanosomatids from wide host species and geographical ranges suggested that Calliphoridae are common hosts of Angomonas spp. In addition, Angomonas spp. was identified in a few flies from other countries (Týč et al., 2013). Our main goals in the present study were to investigate the genetic diversity and host and geographical ranges of Angomonas, to verify the existence of any association between the repertoires of Angomonas and Neotropical and Afrotropical blowflies, and to assess possible dispersal of Angomonas spp. by blowflies. For these purposes, we carried out a broad survey of trypanosomatids in Chrysomya and Lucilia from the Neotropical and Afrotropical Ecozones and the Latin American Cochliomyia using molecular methods sensitive enough to assess interspecific and intra-specific genetic diversity of Angomonas spp., and their respective symbionts.
Materials and Methods
Sampling of Flies and Identification in Africa, and South and Central America
In the last decade, we have systematically surveyed flies for the presence of trypanosomatids in general by culturing and PCR-screening. We examined 3,900 flies collected manually (mostly from 2007 to 2016) of the families Calliphoridae, Muscidae, Syrphidae, and Sarcophagidae. The Calliphoridae constituted most of the sample collected (2,418 flies, ∼ 62%) and they were the focus of this work. The blowflies examined were classified as: Chrysomya (C. putoria, C. albiceps, C. megacephala); Lucilia (L. eximia, L. sericata/L. cuprina) and Cochliomyia (C. hominivorax, C. macellaria). No molecular attempts were made to distinguish L. sericata from L. cuprina, which are morphologically very similar, thus specimens collected in Neotropical regions were classified as L. sericata following the recommended classification (Williams et al., 2016). Species and geographic origin of all flies examined in the present study are in Supplementary Table 1.
In the Afrotropical region, flies were collected in Guinea-Bissau (cities of Bissau and Buba); Mozambique (Beira, Tete, Chupanga, Maputo, Marromeu, and Gorongosa); Madagascar (Andasibe, Antsiranana, Antananarivo, and Moramanga); South Africa (Pretoria); Tanzania (Dar es Salaam and Zanzibar); and Ethiopia (Addis Ababa, Arba Minch, Harar, Hawassa and Lalibela [2,500 m]). In Central America, flies were collected in Panamá city. In South America, sampling of flies extended from northern to southern regions. In Venezuela, flies were collected in Merida and Laguna of Mucubaji (3,500 m) in the Andean Mountains, and in the wetlands of Santa Barbara. In Colombia, flies were collected in Ibagué and near villages between the Central and Oriental Andes Cordilleras. In Ecuador, flies were collected in Ingapirca and Quito (2,850 m). In French Guyana, calliphorids were collected in Cayenne. In Brazil, flies were collected in domestic and sylvatic environments from northern to southern regions across the biomes of the Amazonia, Pantanal, Atlantic Forest, Cerrado, and Caatinga. The locations where flies were collected are shown in Figure 1 and Table 1 (detailed in Supplementary Table 1). Blowflies were identified using morphological parameters, and African and American specimens representative of different species from each country were also identified by cytochrome c oxidase 1 (COI) barcoding (Marinho et al., 2012).
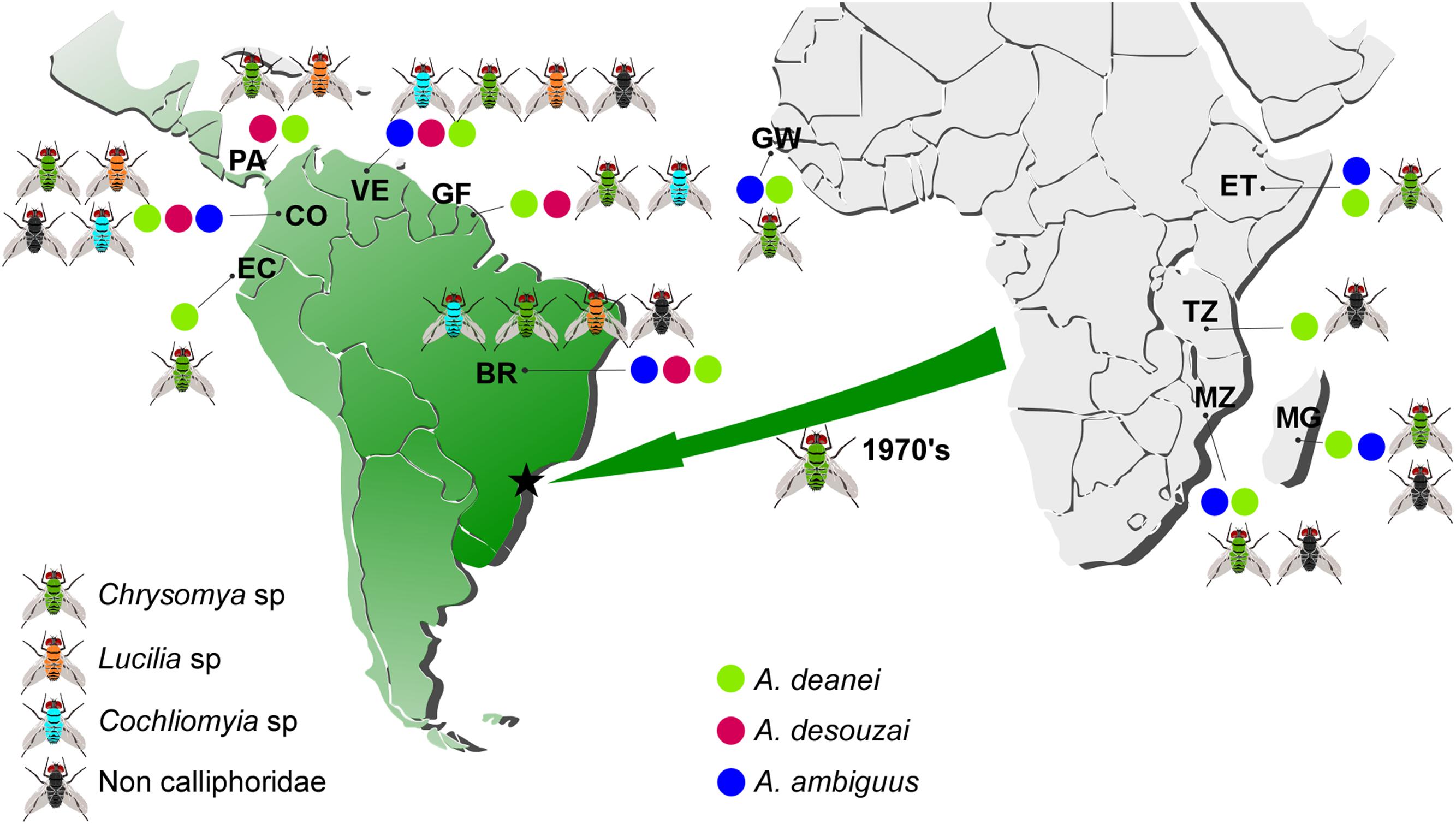
FIGURE 1. Map indicating the collecting sites of flies and distribution of Angomonas species in Afrotropical and Neotropical regions. Blowflies of genera Chrysomya, Lucilia, and Cochliomyia were found infected with Angomonas deanei, A. desouzai, and A. ambiguus. The green arrow indicates the radiation of the African Chrysomya, and the green gradient its dissemination from Southern Brazil to across South and Central America. The black star indicates the site of the first reported Chrysomya in the Neotropic. BR, Brazil; VE, Venezuela; CO, Colombia; EC, Ecuador; GF, French Guiana; PA, Panama; GW, Guinea Bissau; ET, Ethiopia; TZ, Tanzania; MZ, Mozambique; MG, Madagascar.
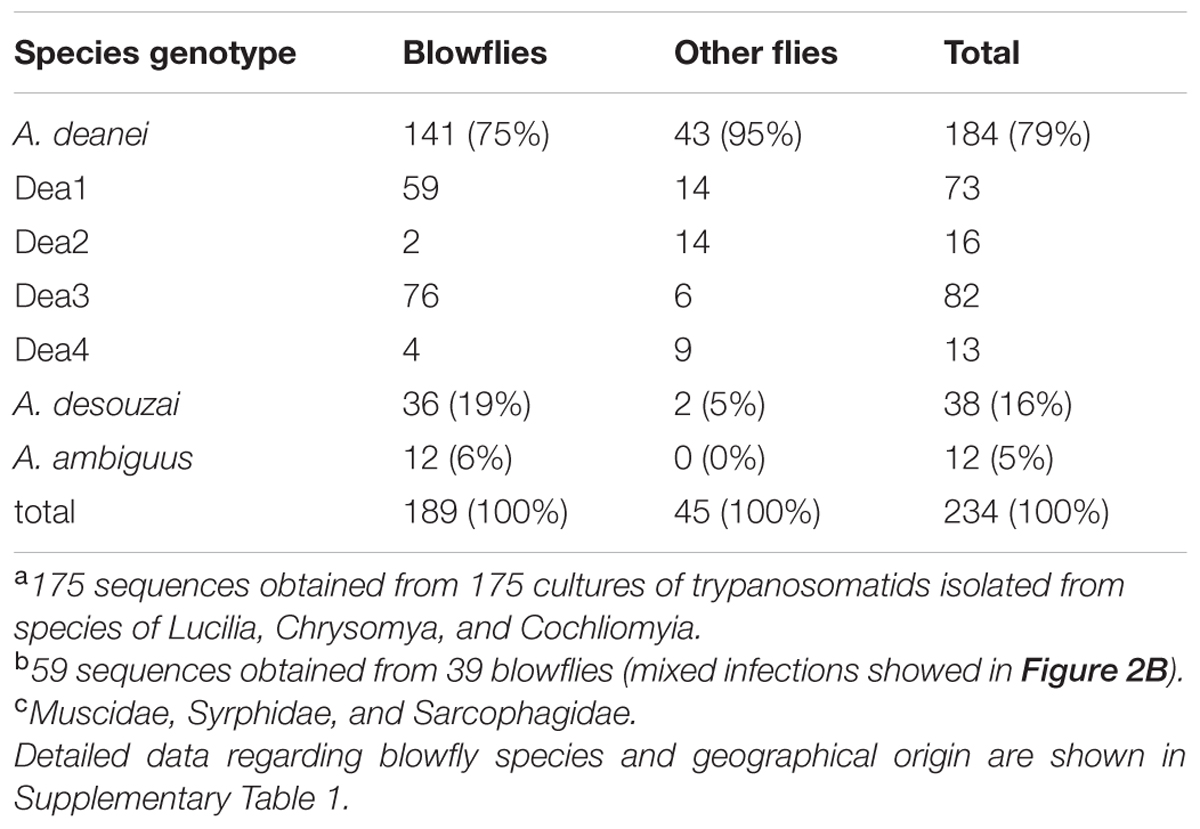
TABLE 1. Identification of Angomonas species and genotypes in culturesa and guts of blowfliesb and other fliesc based on V7V8 SSU rRNA plus gGAPDH and/or symbiont-GAPDH sequences.
Surveys of Flies Carrying Trypanosomatids and the Selection of Angomonas spp.
The gut contents of dissected flies were microscopically examined for trypanosomatids. Samples that were microscopically positive for trypanosomatids were inoculated in culture tubes containing TC100 medium (=Grace’s medium) containing 20 μg/ml of gentamicin, and incubated at 25°C. Positive cultures cleared of bacteria and fungi were cloned and re-cloned using agar plates (TC100 medium with 2% agar base) (Sbravate et al., 1989; Borghesan et al., 2013). Then, well-separated colonies were transferred to liquid medium, and flagellates checked regarding clonality by sequencing of V7V8 SSU rRNA and/or gGAPDH genes. Cryopreserved cultures, ethanol preserved gut samples, and whole flies were deposited in the Trypanosomatid Culture Collection of the University of São Paulo (TCC-USP). With the aim of detecting Angomonas, DNA from guts of blowflies that were positive for trypanosomatids were submitted to V7V8 SSU rRNA barcoding using primers and PCR conditions described previously (Borghesan et al., 2013), and sequences obtained employed for Angomonas identification (Figure 2).
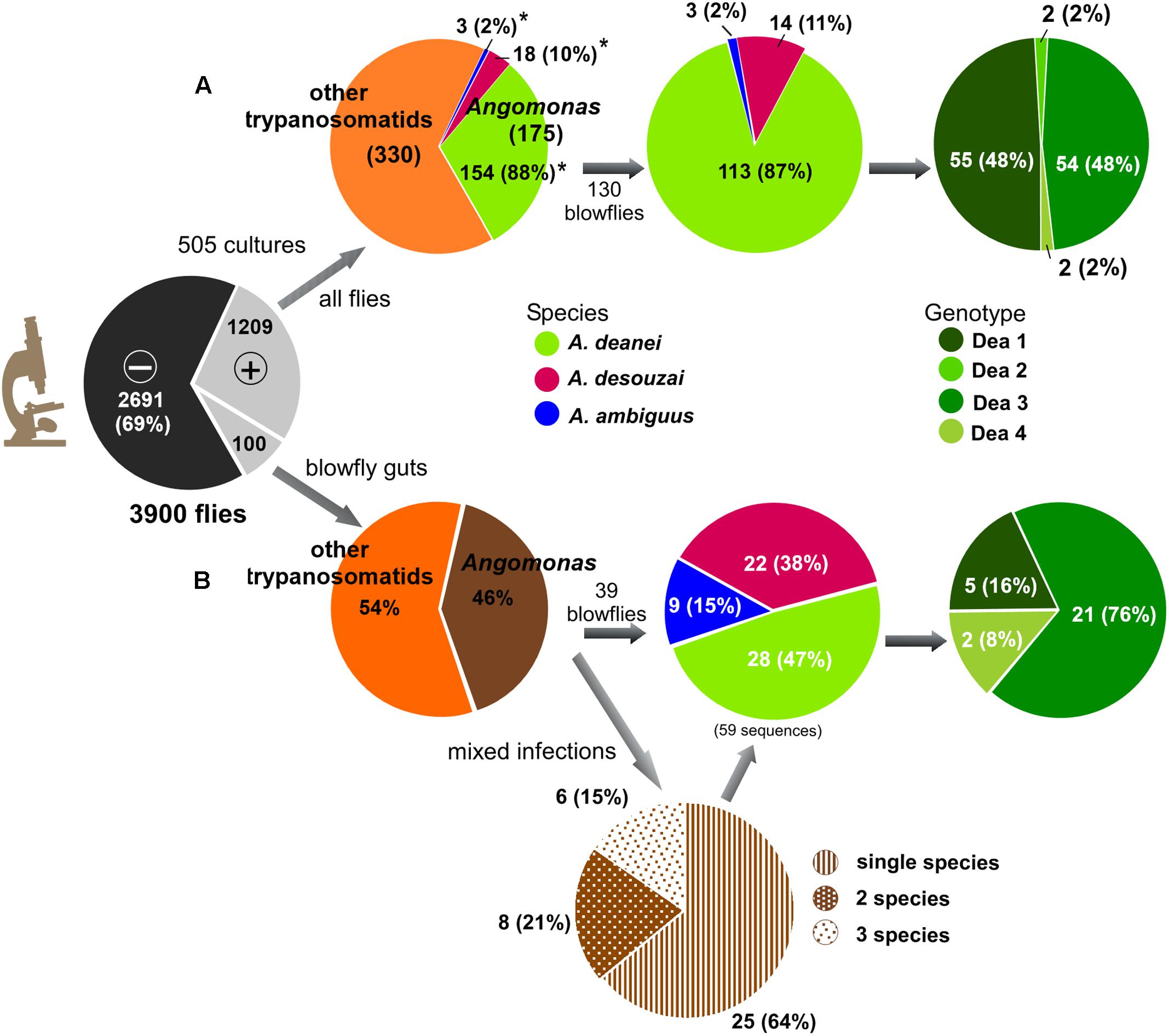
FIGURE 2. Genetic diversity of Angomonas in flies collected in Africa and Latin America. Surveys of trypanosomatids carried out by culturing on 3,900 flies followed by molecular analyses unveiled A. deanei, A. desouzai, and A. ambiguus, and four genotypes (Dea1–Dea4) of A. deanei in: (A) 505 cultures obtained from 1,209 fly guts microscopically positive (+) for trypanosomatids: 175 cultures were identified as Angomonas spp. by V7V8 SSU rRNA barcoding, and 130 cultures were further characterized using GAPDH sequences; (B) 100 blowfly microscopically positive guts surveyed by PCR-screening based on symbiont-GAPDH sequences. Sou, A. desouzai; Amb, A. ambiguus; Dea1–Dea4, A. deanei genotypes.
Detection of Trypanosomatid Proteobacterial Endosymbionts of Angomonas
To specifically detect the TPEs of Angomonas spp. using DNA obtained from fly guts, we designed primers based on GAPDH sequences retrieved from published genomes of A. deanei and A. desouzai symbionts: GAPDH.ENDO.F (5′AAGAGCTCATTATGAAGGTGG3′) and GAPDH.ENDO.R (5′TGGAATCATAYTCATGGTTGC3′). PCR reaction mixtures (25 μl) contained 50–100 ng of DNA, 100 ng of each primer, 200 μM of each dNTP, 1.5 mM of MgCl2, and 2.5 U of Taq DNA polymerase. PCR reactions were carried out with 30 cycles of 3 min at 94°C, 2 min at 58°C, and 2 min at 72°C, and a final extension for 10 min at 72°C. PCR-screening based on GAPDH sequences of TPEs were used to select fly samples containing Angomonas for further analyses. All TPE sequences obtained herein were aligned with GenBank available sequences and submitted to phylogenetic inferences (parsimony, as described below). This approach allows identification of known and novel TPEs of Angomonas.
Barcoding and Phylogeny of Angomonas Based on V7V8 SSU rRNA and gGAPDH Sequences
PCR-sequencing of the variable V7V8 SSU rRNA and gGAPDH from Angomonas cultures and fly gut samples were performed as described previously (Borghesan et al., 2013). Sequences obtained were screened for chimera using the RDP4 package (Martin et al., 2015), and aligned with sequences from species of several genera representative of the main clades of Trypanosomatidae using ClustalX. Three alignments, were used for phylogenetic inferences. Alignment A1 comprised V7V8 SSU rRNA gene (810 characters) from SHTs, and was submitted to parsimony analysis. A2 included concatenated V7V8 SSU rRNA and gGAPDH sequences (1,957 characters) from representatives of all Angomonas spp., other species of SHTs, and various trypanosomatid genera; analyses were performed by maximum likelihood (ML) and Bayesian inference (BI) methods. A3 contained gGAPDH sequences (864 characters) representative of all species and genotypes of Angomonas using species of the genus Strigomonas as outgroups, and submitted to parsimony. Parsimony and bootstrap analyses were performed using PAUP∗ version 4.0b10 software (Swofford, 2002) with 500 random sequence replicates followed by branch swapping (RAS-TBR). ML analyses were performed using the general time reversible (GTR)-gamma-I model in RAxML v.7.0.4 (Stamatakis, 2006) with 1,000 maximum parsimony-starting trees. Model parameters were estimated using the randomized accelerated maximum likelihood (RAxML) model over the duration of the tree search. Bootstrap support was calculated by including 1,000 replicates in the RAxML model using the rapid bootstrapping algorithm, and maximum parsimony as starting trees. Bayesian analysis was performed using MrBayes v3.1.2, the tree searches employed GTR-gamma-I, and the first 25% of the trees from 1,000,000 generations were discarded as burn-in. Default values were used for the remaining parameters. Sequence divergences were calculated using distance matrices (uncorrected p-distance) that were constructed using the program PONTOS (1). Sequences of V7V8 SSU rRNA and gGAPDH genes representative of all Angomonas species and genotypes, from different flies and geographical origin, determined in the present study were deposited in GenBank. The accession numbers of these sequences, and those from previously sequenced SHTs are shown in Supplementary Table 2.
To evaluate whether SHTs and TPEs have undergone parallel diversification, the significance of topological congruence was assessed using the program TreeMap 3.0β, a pairwise distance correlation test of topological congruence that allowed for graphic representation (tanglegrams) of coevolutionary, host-switching, and other events (Charleston and Robertson, 2002). TreeMap uses reconciled trees to compute the fit between the host and parasite phylogenies, and includes a statistical testing that generated random trees to assess whether co-divergence is significantly higher than chance alone.
Polymorphism Analysis of Internal Transcribed Spacer-1 of rDNA from Angomonas spp.
Sequences of PCR-amplified internal transcribed spacer-1 (ITS1) of rDNA were determined using primers and PCR reaction described previously (Borghesan et al., 2013). Sequences obtained in the present study were aligned with those described previously for Angomonas spp. (from GenBank), and employed for phylogenetic analysis using parsimony as described above. Secondary structures of ITS rDNA were inferred using the Unafold package (thermodynamically predicted models) with the energy minimization of paired and unpaired regions at 37°C folding temperatures (Markham and Zuker, 2008), as described previously for trypanosomatids of the genus Herpetomonas (Borghesan et al., 2013). The secondary structures were visualized by using the VARNA program (Darty et al., 2009). All ITS1 rDNA sequences employed in this study are deposited in GenBank, and the accession numbers are shown in Supplementary Table 2.
Statistical Analyses
Analyses of genetic structures of Angomonas populations were tested using AMOVA implemented in Arlequin v. 3.5.1.2 (Excoffier and Lischer, 2010), and the significance was calculated using 20,000 permutations. Statistical analyses were done using Epi Info public domain site. The correlation between the geographic and genetic distance matrices was investigated with the Mantel test version 2.0 (Liedloff, 1999), with 1,000 iterations for the calculation of the random distribution. Fisher’s Test, a standard procedure for measuring the statistical association between two discrete variables, were used to assess the significance of associations between Angomonas species/genotypes and geographical origin or host species.
Results
Identification of Angomonas spp. from Cultures by V7V8 SSU rRNA Barcoding
Microscopy revealed the presence of trypanosomatids in 1,209 out of 3,900 flies from the families Calliphoridae, Sarcophagidae, Muscidae, and Syrphidae, yielding an estimated overall prevalence of 31%. Gut contents of all microscopically positive flies were inoculated in culture, and 505 cultures of different genera of trypanosomatids were obtained, yielding a 42% rate of culturing success. In the last 15 years, V7V8 SSU rRNA barcoding of trypanosomatids from a range of fly families has been systematically carried out in our laboratory. Barcoding of 505 cultures of fly trypanosomatids allowed to identify 175 (34%) cultures harboring Angomonas spp.: 154 A. deanei (88%), 18 (10%) A. desouzai, and 3 (2%) A. ambiguus. Barcoding of trypanosomatids from non-cloned cultures and guts from blowflies revealed the coexistence of Angomonas spp., and other species that require further phylogenetic analyses. Among 130 cloned cultures of Angomonas from blowflies, 113 (87%) were identified as A. deanei, 14 (11%) as A. desouzai, and 3 (2%) as A. ambiguus (Figure 2A).
Phylogenetic analysis of V7V8 SSU rRNA sequences from cultured Angomonas spp. showed three strongly supported clades corresponding to the three species, A. deanei, A. desouzai, and A. ambiguus (Figure 3A). Phylogeny including all know species of the different genera of SHT supported three separate clades, Angomonas, Strigomonas, and Kentomonas (Figure 3A), which together form the subfamily Strigomonadinae (Votýpka et al., 2014). V7V8 SSU rRNA sequences failed to disclose infraspecific diversity within Angomonas spp. Most V7V8 SSU rRNA sequences employed in this analysis were determined in the present study, and sequences representative of each species/genotypes were deposited in Genbank (Supplementary Table 2).
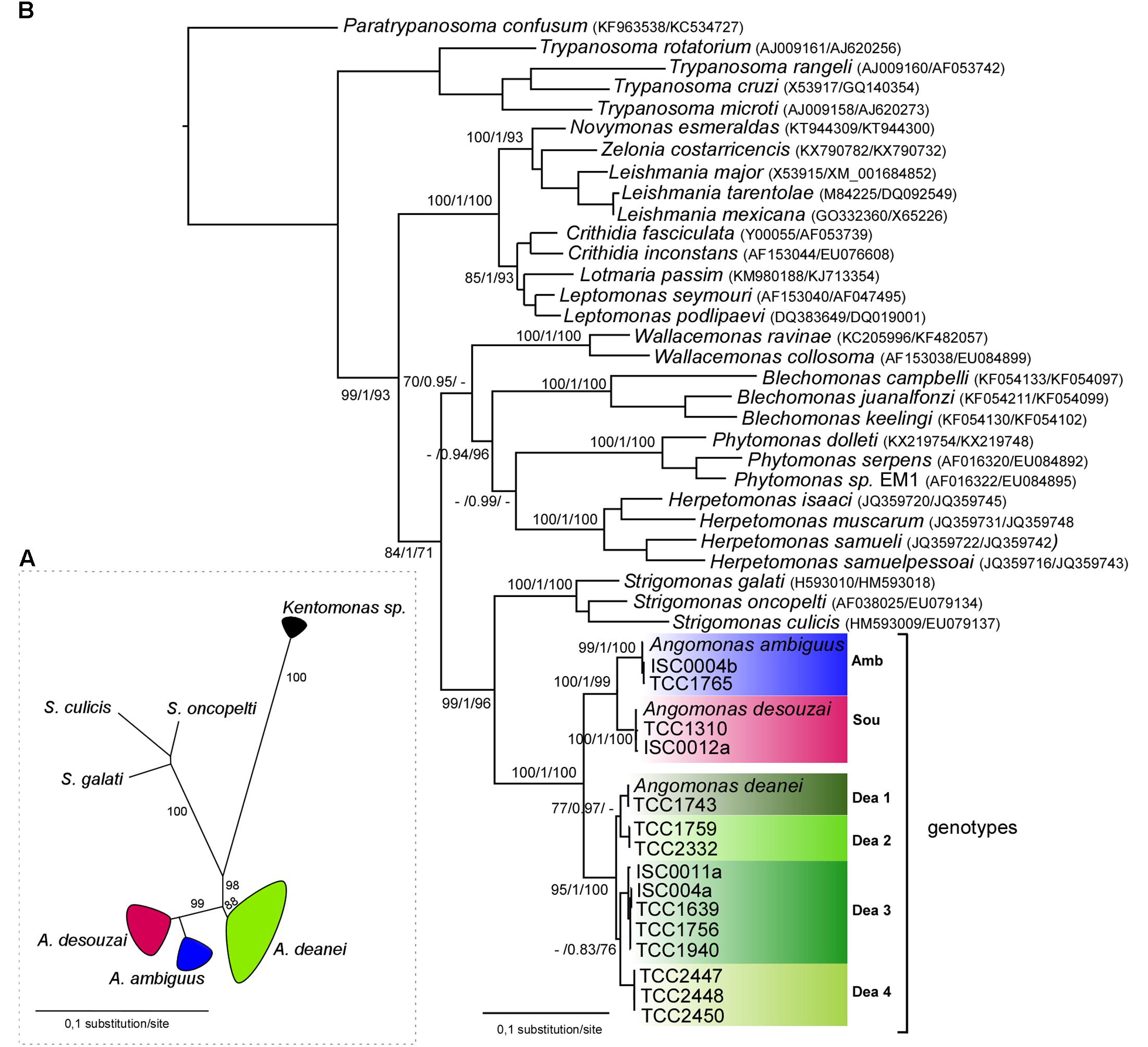
FIGURE 3. Phylogenetic analysis of Angomonas species and genotypes. (A) Dendrogram (ML) of V7V8 SSU rRNA sequences (barcodes) from 175 (174 from blowflies) cultures of A. deanei (Dea), A. desouzai (Amb), and A. ambiguus (Sou), and species of the other genera of symbiont harboring trypanosomatids: Strigomonas culicis, Strigomonas oncopelti, Strigomonas galati, and Kentomonas sorsogonicus. Numbers at the nodes are ML support values (>50%) from 500 replicates. (B) Positioning of representatives of each the three Angomonas species and four genotypes of A. deanei (Dea1–Dea4) were showed in the phylogenetic tree of Trypanosomatidae based on concatenated gGAPDH and V7V8 SSU rRNA gene sequences, inferred by maximum likelihood (–Ln = –16564.401081) and Bayesian analyses. Numbers at the nodes correspond, respectively, to ML/BI/P support values (>50%) from 500 replicates. GenBank accession numbers of V7V8 SSU rRNA and gGAPDH sequences are shown in Supplementary Table 2.
Identification of Angomonas spp. from Blowfly Guts by PCR-Amplification of Symbiont GAPDH Sequences
Comparison of symbiont-GAPDH sequences from all Angomonas spp. discovered relevant polymorphisms among sequences obtained for 113 cultures of A. deanei, whereas sequences from A. desouzai and A. ambiguus were more homogeneous. Analysis of symbiont-GAPDH sequences allowed to identify four genotypes of A. deanei: Dea1, 55 cultures (48%); Dea3, 54 cultures (48%); Dea2 and Dea4, two cultures each (2%) (Figure 2A).
PCR-amplification of symbiont-GAPDH sequences from gut content of 100 blowflies revealed 46 flies (46%) harboring Angomonas spp. Further analysis of 39 flies revealed infections with a single species in 25 (64%) flies, and mixed infections with two and three species in 8 (21%) and 6 (15%) flies, respectively, yielding a total of 59 symbiont-GAPDH sequences. These sequences showed a predominance of A. deanei (28 sequences, 47%) followed by A. desouzai (22 sequences, 38%), and A. ambiguus (9 sequences, 15%) (Figure 2B). The results reveal that A. ambiguus and A. desouzai have a higher prevalence (7 and 3.5 times, respectively) in blowfly gut samples than in cultures (p < 0.01), confirming experimental observation that culturing favors the growth of A. deanei over A. desouzai and A. ambiguus. In addition, the genotype Dea3 (76%) prevails over Dea1 (16%) in blowfly guts, thus differing from cultures showing 48% prevalence rates for both Dea1 and Dea3 genotypes. Notably, not a single species or even genotype of Angomonas distinct from those found in cultures were unveiled by direct examination of blowfly gut samples.
Altogether, the identification of Angomonas spp. among trypanosomatids of cultures and blowfly guts by the combination of SSU rRNA barcoding plus symbiont-GAPDH genotyping revealed A. deanei in 184 flies (141 blowflies), A. desouzai in 38 (36 blowflies), and A. ambiguus in 12 blowflies (Figures 2A,B and Table 1).
Phylogenetic Relationships and Genetic Repertoire of Angomonas Inferred by SSU rRNA and gGAPDH Phylogenetic Analyses
Analyses of gGAPDH sequences of Angomonas spp. corroborated species identification based on V7V8 SSU rRNA, and genotyping based on symbiont-GAPDH sequences. Phylogenetic analysis using V7V8 SSU rRNA and gGAPDH sequences strongly supported three clades corresponding to the three species of Angomonas as showed in the phylogenetic tree inferred using isolates representative of each species/genotypes (Figures 3A,B). In addition, this analysis strongly support the partition of A. deanei in two main lineages, with infra-lineage diversity (moderately supported) suggesting the existence of four genotypes of this species (Figure 3B).
In contrast with highly conserved SSU rRNA sequences, the more polymorphic gGAPDH sequences from isolates of A. deanei showed relevant sequence divergences supporting the four (Dea1–Dea4) genotypes of this species previously uncovered by comparison of symbiont-GAPDH sequences. Among the 113 cultures of A. deanei examined, 55 (48%) were identified as Dea1, 54 (48%) as Dea 3, 2 (2%) as Dea 2, and 2 (2%) as Dea4 (Figures 2, 3). Phylogenetic relationships based on gGAPDH sequences of a larger number of isolates of A. deanei strongly supported the Dea1-Dea4 genotypes (Figure 4). In addition, the four genotypes of A. deanei were strongly supported in the analyses restricted to Angomonas based on ITS rDNA (Figure 5),
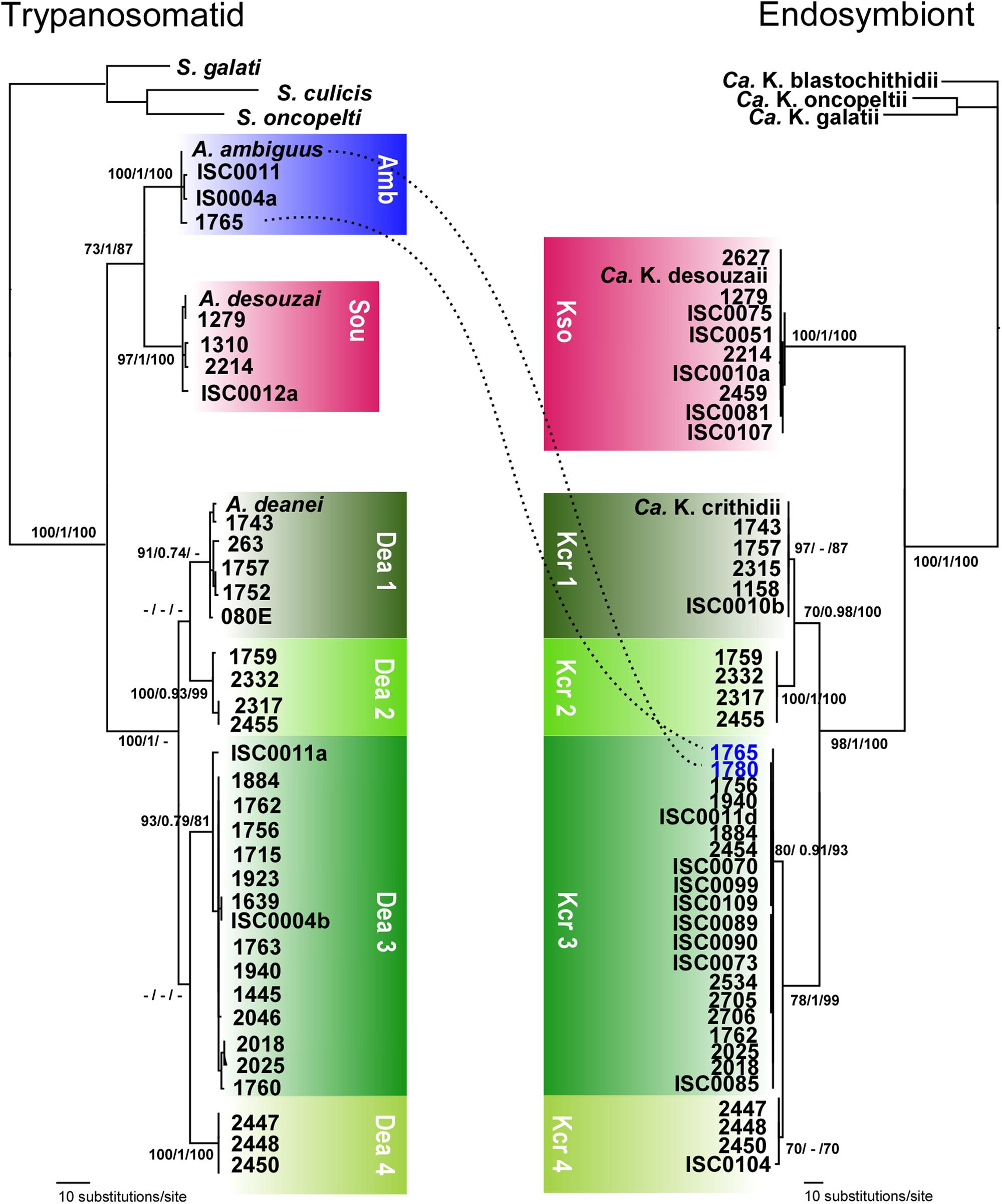
FIGURE 4. Congruence between Angomonas spp. and endosymbiont phylogenies. Parity analysis between phylogenies inferred using sequences of gGAPDH from the trypanosomatid hosts and GAPDH from their respective symbionts. Host and corresponding symbiont genotypes are represented by matching colors. The dotted line indicates the presence of Kcr3 symbiont of A. deanei in A. ambiguus. Amb, A. ambiguus; Sou, A. desouzai; Dea1–Dea4, A. deanei genotypes. Kso, Candidatus Kinetoplastibacterium desouzaii symbiont of A. desouzai; Kcr1–Kcr4, genotypes of Candidatus Kinetoplastibacterium crithidii symbiont of A. deanei. Numbers at the nodes correspond, respectively, to P/ML/BI support values (>50%) from 500 replicates. GenBank accession numbers of sequences employed in this analysis are shown in Supplementary Table 2.
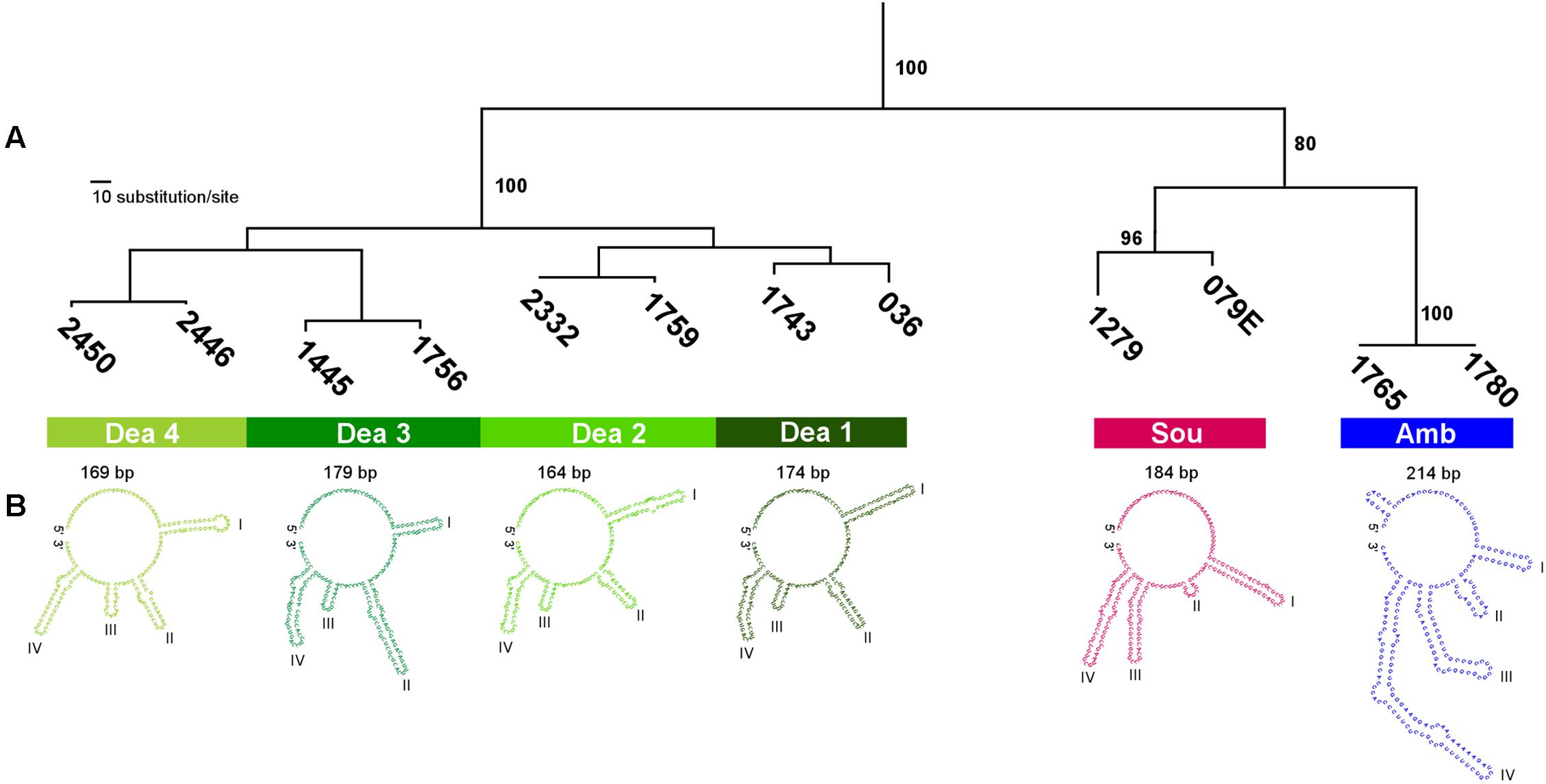
FIGURE 5. Analyses of ITS1 rDNA sequences from Angomonas species and genotypes. (A) Dendrogram (parsimony) of ITS1 rDNA sequences from A. ambiguus, A. desouzai, and genotypes (Dea1–Dea4) of A. deanei. The numbers at the node correspond to bootstrap values (>50%) from 500 replicates. (B) Predicted secondary structures of ITS1 rDNA sequences from Angomonas species and genotypes showing an open loop and four main helices that varied in length according to species and main genotypes of A. deanei. The estimated values of thermodynamic energy of modelled structures (ΔG Kcal/mol) for each species were: A. deanei (–54.4), A. desouzai (–69), and A. ambiguus (–83.3). Sou, A. desouzai; Amb, A. ambiguus; Dea1–Dea4, A. deanei genotypes. GenBank accession numbers of ITS1 rDNA sequences included in this analysis are shown in Supplementary Table 2.
Genotype Dea1 includes the holotype of A. deanei, TCC 036E (Teixeira et al., 2011). There were no gGAPDH divergences greater than 0.1% among isolates of the same genotype of A. deanei. The gGAPDH sequence divergences among the four Dea genotypes ranged from 2.0 to 3.0%. For comparison, gGAPDH divergences of A. deanei from A. desouzai and A. ambiguus were about 7%, whereas divergences between A. desouzai and A. ambiguus were 4.0%. New isolates of A. desouzai and A. ambiguus diverged less than 1.0% from the respective holotypes, thus lacking relevant polymorphisms that would justify their separation in infraspecific genotypes.
Inter- and Intra-specific Diversity of Angomonas spp. Unveiled by ITS1 rDNA Sequences
Aiming at improving the assessment on infraspecific genetic diversity within the species of SHTs, we determined sequences of the highly polymorphic ITS rDNA sequences (Teixeira et al., 2011). ITS rDNA phylogenetic analysis confirmed the clustering of sequences according to species and genotypes (Figure 5A). Extensive polymorphisms, with divergences of ∼ 50% in ITS rDNA sequences, separate the cluster containing A. deanei genotypes from those formed by A. desouzai and A. ambiguus (which diverge ∼ 35% from each other), and corroborated the high genetic diversity among A. deanei genotypes: ∼ 12% between Dea1and Dea2, and ∼20% between Dea1 and Dea3/Dea4. Sequence divergences within a given genotype were small, varying from 1.0% (within Dea3) to 6.0% (within Dea1). The maximum ITS rDNA sequence divergence among clones of a given isolate was 2.0%. Despite relevant polymorphisms among species and genotypes, the relationships inferred using ITS rDNA sequences (Figure 5) corroborated V7V8 SSU rRNA + gGAPDH (Figure 3B) and gGAPDH (Figure 4A) inferred phylogenetic relationships.
Angomonas spp. exhibited the same general features of ITS rDNA secondary structure of trypanosomatids in general, consisting of an open loop with four main helices. The putative secondary structures exhibited four helices that varied in length, shape and position, which allowed for the species of Angomonas to be clearly distinguished. The structures of the most divergent Dea1 and Dea3 genotypes were distinguishable, while Dea1 and Dea 2 shared very similar structures (Figure 5B). In every case, isolates of the same genotype yielded identical overall structure, which added support to the consistency of secondary structures as one valuable taxonomic parameter to distinguish species and even genotypes of trypanosomatids (Borghesan et al., 2013).
Parallel Evolution of Symbionts and Their Angomonas Hosts Supported by Congruent Phylogenies
Accompanying the genetic heterogeneity of gGAPDH sequences of Angomonas spp. hosts (Figure 4A), GAPDH sequences of Angomonas symbionts also exhibited marked heterogeneity. In the inferred phylogenies, sequences of the symbionts of A. deanei were split into two main clusters, each one comprising two well-supported genotypes (three genotypes were herein reported for the first time). We refer to these four genotypes of Candidatus Kinetoplastibacterium crithidii as Kcr1, Kcr2, Kcr3, and Kcr4 (Figure 4B). The Dea clusters are congruent with the Kcr clusters as supported by ML, P, and BI analyses: Kcr1 associated exclusively with Dea1, Kcr2 with Dea2, Kcr3 with Dea3, and Kcr4 with Dea4. Similarly, isolates of A. desouzai paired only with Ca. K. desouzaii. The only exception was the lack of congruence between A. ambiguus and its symbiont; this species harbors the symbiont Kcr3 of A. deanei Dea3 (Figures 4A,B).
To assess whether SHTs and TPEs have undergone parallel diversification, the significance of topological congruence between the phylogenies of the SHT and TPE species and genotypes was assessed by TreeMap. The tanglegram inferred by TreeMap strongly supported (p < 0.002) codivergence events between SHTs and TPEs, and a single event of host switch represented by A. ambiguus carrying the symbiont Kcri3 of A. deanei Dea3 (Figure 6).
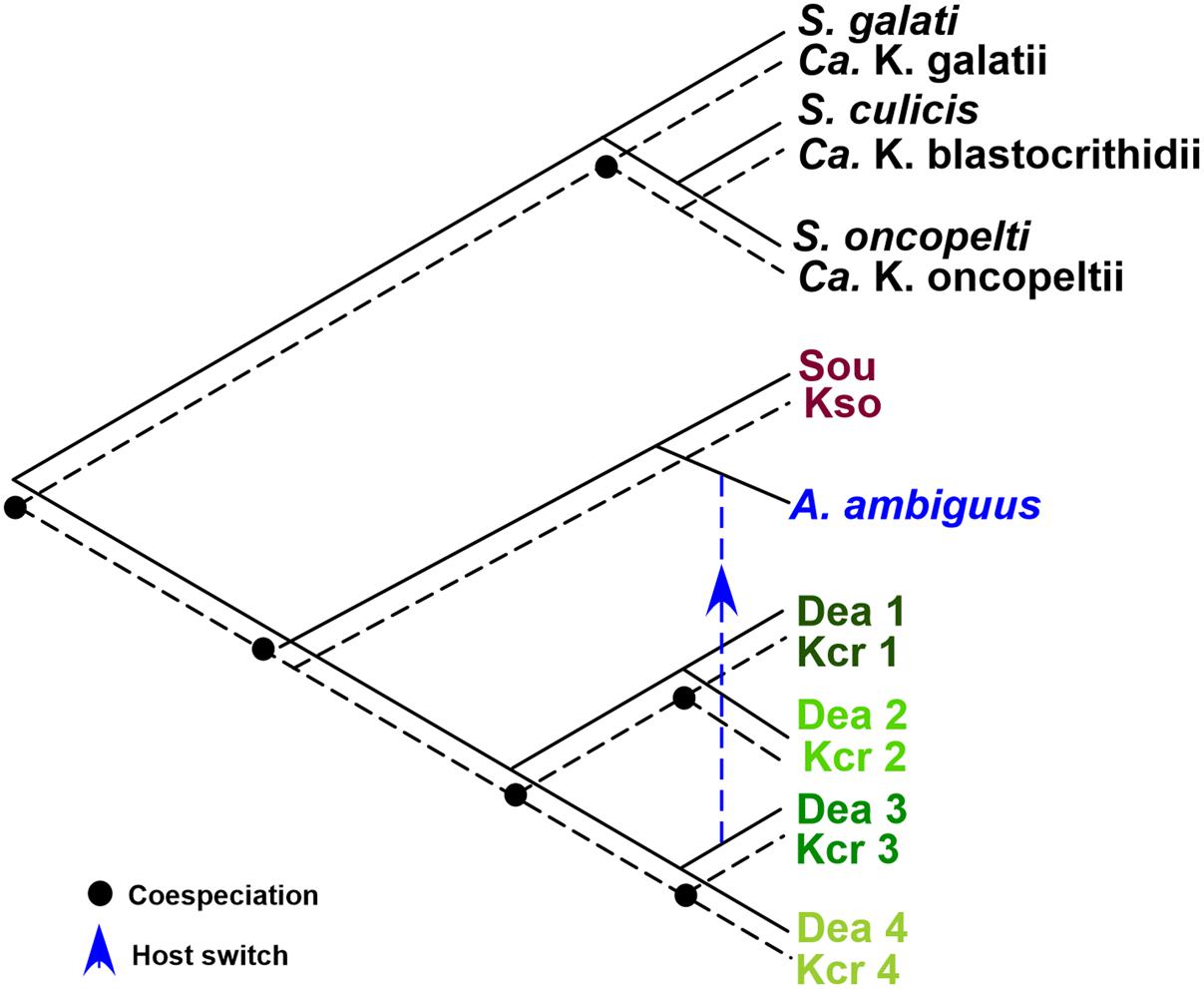
FIGURE 6. Tanglegram of coevolutionary patterns between Angomonas hosts (SHTs) and symbionts (TPEs), strongly supporting (p < 0.002) codivergence between species/genotypes of SHTs and species/genotypes of TPEs. The only exception was a single host switch between A. ambiguus and the symbiont Kcri3 of A. deanei Dea3. Tanglegram generated by TreeMap using the MP topologies inferred with gGAPDH sequences from SHTs and GAPDH from TPEs (showed in Figure 5).
Tripartite Association of Angomonas, Endosymbionts and Calliphorids throughout Their Geographic Ranges
Keeping in mind that Cochliomyia spp. and Lucilia eximia are New World flies and that, until the 1970’s, Chrysomya was absent in the Americas, we scored the presence of Angomonas genotypes, and their association with these three genera of Calliphoridae, in the Neotropical and Afrotropical realms (Figures 1, 7). A. deanei predominated in all examined genera of blowflies, from Neotropic and Afrotropic, regardless whether the trypanosomatids were analyzed from cultures or directly from fly guts. C. putoria and C. megacephala as well as in L. sericata and L. eximia were the commonest hosts of A. deanei. A. desouzai was identified exclusively in the Neotropical blowflies of the three calliphorid genera examined; however, most isolates of this species were detected in L. eximia followed by C. putoria. The absence of A. desouzai in Chrysomya in Africa contrasts with very high significance (p < 0.01) with its presence in the introduced Chrysomya spp. A. ambiguus was found in the Afrotropic and Neotropic, mainly in C. putoria and sporadically in C. megacephala, C. albiceps, and L. eximia from the New World (Figures 1, 7). Interestingly, this species was not detected in Cochliomyia, even in searches carried out directly by PCR using DNA from fly guts to avoid favored culturing of other species. To date, C. hominivorax and C. macellaria were found infected with A. deanei and A. desouzai (Figures 2B, 7 and Table 1).
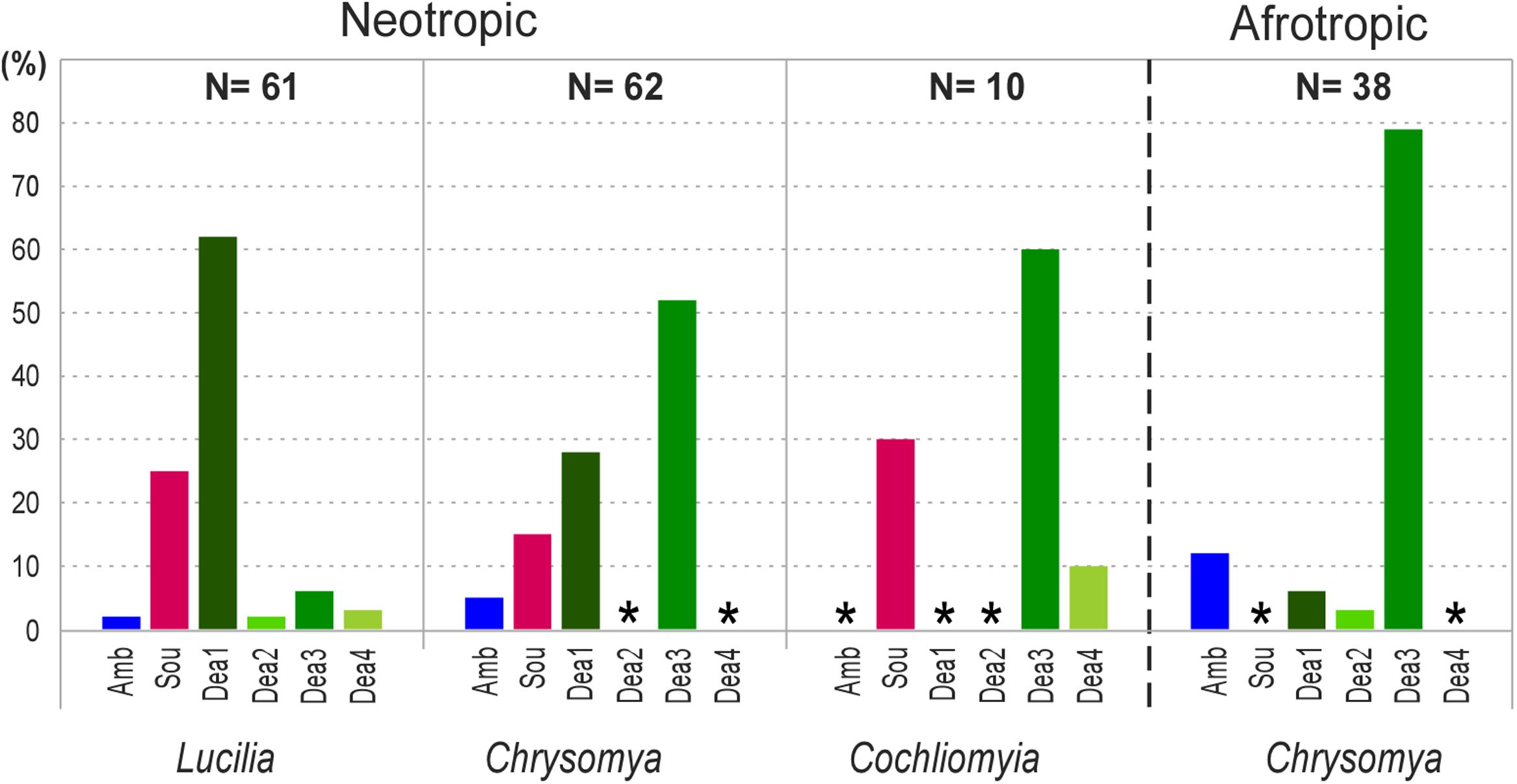
FIGURE 7. Infection rates and geographical distribution of Angomonas species and genotypes in blowflies from the Afrotropic and Neotropic. A. ambiguus (Amb), A. desouzai (Sou), and A. deanei (Dea1–Dea4 genotypes) detected in calliphorids of the genera Chrysomya, Lucilia, and Cochliomyia. N = number of infected blowflies. The asterisk indicates absence. Angomonas was not detected in Lucilia from the Afrotropic. Detailed data from each fly genera are shown in Table 1, and result obtained from each fly culture and gut sample are shown in Supplementary Table 1.
Regarding genotypes of A. deanei, Dea3 was predominant in Chrysomya spp. from the Afrotropic and Neotropic, collected either in native or invaded regions (p < 0.01). Notably, this genotype was also common in Cochliomyia. In contrast, Dea1 was the predominant genotype infecting South American Lucilia, either the Neotropical L. eximia or exotic L. cuprina/L. sericata. Compared to Dea3, Dea1 was rarer in African Chrysomya spp., whereas it is worth noting the presence of Dea2 exclusively in African Chrysomya (Figure 7). The repertoire of A. deanei uncovered in C. hominivorax and C. macellaria collected in Brazil, Colombia, Venezuela and French Guiana revealed A. deanei of the genotypes Dea3 and Dea4 (Figures 1, 7). In addition, these flies were the major hosts of A. desouzai, suggesting (p < 0.01) that this species may be endemic to the New Word. Altogether, our data revealed a distinct repertoire of Angomonas species and genotypes for each calliphorid genus examined (Figure 7). Apparently, Dea1 and Dea3 genotypes have preferences for Chrysomya and Lucilia, respectively, with very high significance estimated by Fisher’s Test (p < 0.01).
Lack of Genetic Distances between African and American Populations of Angomonas spp.
Despite the widespread distribution of A. deanei and A. ambiguus, divergences of gGAPDH sequences of isolates from distant areas in South America and Africa were always smaller than 1.0%. Similar results were obtained for isolates of A. desouzai from distant collecting sites of South America. We used Mantel’s test to assess the correlation between geographic and genetic distances among the main genotypes Dea1 and Dea3 that infect blowflies in the Afrotropic and Neotropic. We found no significant correlation (r = –0.0353) between the two matrices, with a standard normal variate (g) value of –0.4359 for our dataset (for a significance of 0.05, the critical value is 1.645). Overall, 34.6% of the random permutations exhibited a better correlation between matrices than that of collected data. Thus, no correlation was found between geographic and genetic distances of Dea1 and Dea3 genotypes from Africa and South America what clearly indicates the recentness of their geographical separation.
We also analyzed the genetic structure of A. deanei genotypes by comparing gGAPDH sequences from the Afrotropical and Neotropical genotypes Dea1 and Dea3. Using the AMOVA test, results revealed a diversity of 2.4% between the African and South American genotypes, and 97.4% in each population, which supports the lack of spatial genetic structure for both Neotropical and Afrotropical parasites (фST 0.024, p < 0.003). Regarding the endosymbiont, the analysis of Kcr1 and Kcr3 TPEs confirmed the somewhat higher degree of genetic structure of symbionts. Divergence between gGAPDH sequences amounted to 6.1% between African and South American TPEs, with ∼ 85% divergence within each population (фST 0.15).
Discussion
Here, we provided the most comprehensive data gathered so far regarding the genetic diversity, and ranges of host species and geographical distribution of a genus of trypanosomatids exclusive of insects, mainly flies. The results demonstrated that regardless of their hosts and geographic origin, the populations of Angomonas consisted of species and genotypes that clustered together in three strongly supported phylogenetic lineages. Each lineage corresponds to one of three species, A. deanei (four genotypes), A. desouzai, and A. ambiguus. There is a consistent congruence between the phylogenies of these flagellates and their respective symbionts both at species and genotype levels. The only exception is A. ambiguus that harbors the symbiont of the Dea3 genotype of A. deanei, confirming the ambiguity of A. ambiguus as previously demonstrated based on analysis of TPE ITS rDNA and 16S sequences (Teixeira et al., 2011)
It is not known when the Angomonas–symbiont association was first established in geologic times. Provisional estimates (Du et al., 1994) suggested that a single event of symbiont acquisition by the SHT ancestor may have occurred by the end of the Cretaceous. However, phylogenetic analyses supported that all TPEs have a common Betaproteobacteria ancestor (Alves et al., 2011, 2013a,b; Klein et al., 2013). The existence of an extinct, therefore, inferable common symbiont ancestor is supported by the overall genomic similarity among Angomonas symbionts, and broad genomic synteny (Alves et al., 2013b). Regardless of the timing of the event, SHTs and their symbionts initiated a joint journey of coevolution long ago, which seems to have been influenced by their strong association with flies, and by the cohabitating in the insect guts with a diversity of bacteria. The tightly associated pairs of host–symbiont genotypes suggest coevolution, with long-shared evolutionary histories consistent with interdependence between host and symbiont genomes (Moran, 2006; Moran et al., 2008; Alves et al., 2011, 2013a).
This study brought information about the tripartite association of Angomonas, endosymbionts and calliphorids throughout their geographic ranges. A. deanei and A. ambiguus seem to be cosmopolitan because in addition to Neotropical and Afrotropical realms, they have been also reported in Papua New Guinea, while A. deanei has also been found in Europe (Týč et al., 2013). Noticeable was the absence of A. desouzai in the Afrotropic, suggesting Neotropical endemicity of this species, and spillback of A. desouzai from resident Neotropical hosts to Chrysomya in South America. The finding of A. desouzai in sarcophagid flies from Ecuador (Týč et al., 2013) corroborated the hypothesis that A. desouzai may be endemic to the New Word, but also suggests that flies other than blowflies may be the preferred hosts of this species.
The three-known species of Angomonas have been recovered from blowflies, with high prevalence rates, from both hemispheres, from sea level to 3,500 m of altitude in the Andes Cordillera, from 9° north (Panama) to 25° south (South Brazil), and from 47° east (Madagascar) to 77° west (Panama). In Brazil, Angomonas spp. Occurred in calliphorid flies from the Amazonian Rainforest, the Pantanal wetlands, Cerrado (savanna), Atlantic Forest, and semiarid Caatinga. Nothing is known about the behavioral and pathogenic implications to blowflies of the highly prevalent Angonomas infections. Blowflies of Chrysomya, Cochliomyia, and Lucilia exhibiting different parasite repertoires were many times collected while feeding together, and it is well known that the spread of insect trypanosomatids occurs by coprophagy (Wallace, 1966; Hupperich et al., 1992). This finding together with different repertoires of Angomonas exhibited by each calliphorid genus examined suggested the existence of a certain degree of association between species/genotypes of Angomonas and genera/species of Calliphoridae flies.
Geographic distribution of A. deanei genotypes (Dea1–Dea4) in Chrysomya partially agrees with the general rule that parasite diversity in a determined host is larger in the native area of the invader than in the invaded area (Poulin and Mouillot, 2003; Torchin et al., 2003). Accordingly, a great diversity of genotypes has been uncovered in Chrysomya in the Afrotropic (Dea2 was found exclusively in Africa), suggesting a possible bottleneck effect of A. deanei genotypes, concordant with the bottleneck of Chrysomya when introduced in the New World (Junqueira et al., 2016). However, Chrysomya spp. in Afrotropical habitats carried mostly A. deanei and sporadically A. ambiguus, whereas in the Neotropic, these flies carried the three species of Angomonas.
For the first time, the repertoire of trypanosomatids was described in C. hominivorax and C. macellaria,which are the two most widespread species of this Neotropical genus of blowfly (Yusseff-Vanegas and Agnarsson, 2016). Cochliomyia spp. collected for the present study in Brazil, Colombia, Venezuela and French Guiana were found infected with the widespread genotype Dea3, and the rare genotype Dea4. The genotype Dea 3 of A. deanei can be associated to Chrysomya spp. of both the Neotropic and Afrotropic, whereas Dea1 was present only in South American Lucilia spp. It is important to notice the absence in our study of Angomonas in Lucilia collected in Africa. Apparently, Dea2 and Dea 4 occur preferentially in Muscidae, and their scarcity may be due to our survey focusing on Calliphoridae.
Mitogenomics support the origin of Calliphoridae at the boundary of Oligocene and Miocene, ∼ 22 million years ago (Junqueira et al., 2016), when the ancestors of Angomonas were likely parasitizing flies other than calliphorids. There are fossil records showing trypanosomatids associated with insects since the early Cretaceous (Poinar, 2007), but no fossil exists of blowflies harboring these flagellates. Regardless of the dating, results from the present study, and studies currently being done at our laboratories on various fly and hemipteran families, are showing that the extant tripartite association calliphorid–Angomonas–symbiont is tightly linked, and that Angomonas stands out among the preferred trypanosomatid inquiline of the Calliphoridae. However, whether Angomonas spp. prefers the Calliphoridae flies requires additional studies because there are more than one hundred dipteran families, and we examined only four of them.
Although highly prevalent in flies, A. deanei was originally recovered from a predator reduviid (Carvalho, 1973). Before that, nothing was published about flies harboring SHTs. However, there are drawings of fly trypanosomatids more than one century old (Roubaud, 1908, 1911a,b) showing opisthomorphs, which are characteristic of SHTs (Teixeira et al., 2011), thus suggesting the presence of Angomonas and other SHTs in African calliphorids at least one century ago.
Surveys of blowflies (field surveys and museum collections) revealed the predominance of species of Lucilia and Cochliomyia, and the absence of Chrysomya among Brazilian calliphorids in the 1970s (Mello, 1961; Guimarães et al., 1978, 1979). The Afrotropical Chrysomya putoria (=C. chloropyga) was for the first time reported in the Neotropic in 1976 in the vicinities of a Southern Brazilian seaport harboring ships from Angola and Mozambique (Imbiriba et al., 1978; Guimarães et al., 1978). Shortly after that, C. albiceps and C. megacephala were also reported in southeastern Brazil (Guimarães et al., 1979). Then, African blowflies rapidly expanded through the New World (Guimarães et al., 1978, 1979; Richard and Gerrish, 1983; Baumgartner and Greenberg, 1984; Wells, 1991). The growing Chrysomya population most likely overwhelmed the population of Cochliomyia, which became mainly restricted to more preserved areas of central and northern South America (Batista-da-Silva et al., 2011). Genetic population structure supporting recent introduction of Chrysomya blowflies into the New World have been well-documented (Godoy et al., 1997; Rodrigues et al., 2009; McDonagh and Stevens, 2011; Junqueira et al., 2016).
Available data do not permit the establishment of the timeframe and place in which Angomonas spp. originated and dispersed to the Neotropic and Afrotropic. After Columbus, any fly hosting Angomonas spp. could have been carried in both directions between the Old and New Worlds due to the intensive commerce of people, livestock, and goods (Wells, 1991; McDonagh and Stevens, 2011; Junqueira et al., 2016; Williams et al., 2016). Apparently, Chrysomya only became established in the Americas in the last 40 years. The description of A. deanei in Brazil before the arrival of Chrysomya (Carvalho, 1973; Carvalho and Deane, 1974; Mundim et al., 1974) plus the fact that flies of many families including resident Lucilia spp. are hosts of Angomonas, may indicate the existence of these flagellates in the American continent before the introduction of Chrysomya. Nevertheless, the recent dispersion of the highly prolific African Chrysomya spp., and their prompt adaption to diverse environments, particularly anthropogenic habitats, likely played a fundamental role in the rapid dispersion of Angomonas spp. throughout South and Central America. The genotypes of Angomonas that are present in the Neotropic are indistinguishable from their Afrotropical counterparts, consistent with the recent, and most likely still ongoing, dispersal of Chrysomya spp. throughout the world. These findings highlight the current disjunct distribution of Angomonas spp., and support its trans-oceanic dispersal (de Queiroz, 2014) through man-mediated spreading of flies harboring Angomonas–TPE pairs.
The very comprehensive data gathered in the present study, and results from two previous smaller surveys (Teixeira et al., 2011; Týč et al., 2013), demonstrated that Angomonas occurs in the Neotropic, Afrotropic, Palearctic, Indomalaya, and Australasia ecozones. The findings herein reported on the genetic repertoire of Angomonas spp. in calliphorid flies in the Neotropic and Afrotropic is the first step toward a broader phylogeography of the tripartite Angomonas–symbiont–blowfly association. In this study, we examined just the more prevalent species of three Calliphoridae genera from the Neotropic and Afrotropic. Our findings underscore the need to extend data collection of blowflies, and many other flies from wider geographical range to better understand the repertoire composition of Angomonas-TPE species and genotypes, and their dispersal and evolutionary dynamics throughout the world. Notably, SHTs of the genus Strigomonas were not detected in blowflies, and to date species of Kentomonas were reported only in Sarcophagidae from the Philippines (Votýpka et al., 2014). A better knowledge on the host-species and geographical ranges of these genera is crucial to the understanding of the evolutionary history of the whole Strigomonadinae subfamily.
Author Contributions
TB, MC, and TM are co-first authors; TB and TM performed the sequencing and phylogenetic analyses; MC was responsible for the isolation and culturing of parasites; OE, VR, FP, JC, NA, and LN coordinated the field works, and help collection, dissection and microscopical examination of flies; EC and MT designed the study, organized the data set, drafted the manuscript, and share co-last authorship. All authors contributed with data analysis, share the responsibility related to the accuracy of the work, revised the manuscript, and approved its final version.
Funding
This work was funded by the PROAFRICA, INCT-EPIAMO, and PROSUL programs of CNPq, PNIPB of Capes, and FAPESP (Process 2016/07487-0). CAPEs (PNPD) granted a postdoctoral scholarship to TB.
Conflict of Interest Statement
The authors declare that the research was conducted in the absence of any commercial or financial relationships that could be construed as a potential conflict of interest.
Acknowledgments
The authors are grateful to many students and local people for their valuable help collecting and examining flies in Africa and South America. They are indebted to Lala H. Ravaomanarivo from the Antananarivo University for lab facilities and the students Andry Z. K. Rakatomalala, Marie E. Rabotoson, and Tahina E. Rajaonera for help collecting and dissecting flies. They are grateful to Gladys H. Crisante for collecting insects in Venezuela. They also thank, for logistic support, José and Carmen Martins, and Carlos L. Pereira, in Mozambique, Desie M. Sheferaw in Ethiopia, and Benoit de Thoisy in French Guyana. They are grateful to Silvio Nihei (IB-USP) and Ana M. A. Espin (UNICAMP) for fly identification, João M. P. Alves and Henrique Krieger for help in statistical analyses, Carmen S. A. Takata for DNA sequencing assistance, and Manoel Perez for TCC-USP CryoBank maintenance.
Supplementary Material
The Supplementary Material for this article can be found online at: https://www.frontiersin.org/articles/10.3389/fmicb.2018.00131/full#supplementary-material
Footnotes
References
Alves, J. M., Klein, C. C., da Silva, F. M., Costa-Martins, A. G., Serrano, M. G., Buck, G. A., et al. (2013a). Endosymbiosis in trypanosomatids: the genomic cooperation between bacterium and host in the synthesis of essential amino acids is heavily influenced by multiple horizontal gene transfers. BMC Evol. Biol. 13:190. doi: 10.1186/1471-2148-13-190
Alves, J. M., Serrano, M. G., Maia da Silva, F., Voegtly, L. J., Matveyev, A. V., Teixeira, M. M. G., et al. (2013b). Genome evolution and phylogenomic analysis of Candidatus Kinetoplastibacterium, the betaproteobacterial endosymbionts of Strigomonas and Angomonas. Genome Biol. Evol. 5, 338–350. doi: 10.1093/gbe/evt012
Alves, J. M., Voegtly, L., Matveyev, A. V., Lara, A. M., da Silva, F. M., Serrano, M. G., et al. (2011). Identification and phylogenetic analysis of heme synthesis genes in trypanosomatids and their bacterial endosymbionts. PLOS ONE 6:e23518. doi: 10.1371/journal.pone.0023518
Aubertin, D. (1933). Revision of the genus Lucilia R.-D. (Diptera. Calliphoridae). Zool. J. Linn. Soc. 38, 389–436. doi: 10.1111/j.1096-3642.1933.tb00991.x
Batista-da-Silva, J. A., Moya-Borja, G. E., Pinto de Mello, R., and Queiroz, M. M. (2011). Abundance and richness of Calliphoridae (Diptera) of public health importance in the Tinguá Biological Reserve, Nova Iguaçu (RJ), Brazil. Entomotropica 26, 137–142.
Baumgartner, D. L., and Greenberg, B. (1984). The genus Chrysomya (Diptera: Calliphoridae) in the new world. J. Med. Entomol. 21, 105–113. doi: 10.1093/jmedent/21.1.105
Borghesan, T. C., Ferreira, R. C., Takata, C. S., Campaner, M., Borda, C. C., Paiva, F., et al. (2013). Molecular phylogenetic redefinition of Herpetomonas (Kinetoplastea, Trypanosomatidae), a genus of insect parasites associated with flies. Protist 164, 129–152. doi: 10.1016/j.protis.2012.06.001
Camargo, E. P. (1999). Phytomonas and other trypanosomatid parasites of plants and fruit. Adv. Parasitol. 42, 29–112. doi: 10.1016/S0065-308X(08)60148-7
Carvalho, A. L., and Deane, M. P. (1974). Trypanosomatidae isolated from Zelus leucogrammus (Perty, 1834) (Hemiptera, Reduviidae), with a discussion on flagellates of insectivorous bugs. J. Protozool. 21, 5–8. doi: 10.1111/j.1550-7408.1974.tb03605.x
Carvalho, A. L. M. (1973). Estudos sobre a posição sistemática, a biologia ea transmissão de tripanosomatídeos encontrados em Zelus leucogrammus (Perty, 1834) (Hemiptera, Reduviidae). Rev. Pat. Trop. 2, 223–274.
Catta-Preta, C. M. C., Nascimento, M. T. C., Garcia, M. C. F., Saraiva, E. M., Motta, M. C., and Meyer-Fernandes, J. R. (2013). The presence of a symbiotic bacterium in Strigomonas culicis is related to differential ecto-phosphatase activity and influences the mosquito-protozoa interaction. Int. J. Parasitol. 43, 571–577. doi: 10.1016/j.ijpara.2013.02.005
Charleston, M. A., and Robertson, D. L. (2002). Preferential host switching by primate lentiviruses can account for phylogenetic similarity with the primate phylogeny. Sys. Biol. 51, 528–535. doi: 10.1080/10635150290069940
Darty, K., Denise, A., and Ponty, Y. (2009). VARNA: interactive drawing and editing of the RNA secondary structure. Bioinformatics 25, 1974–1975. doi: 10.1093/bioinformatics/btp250
de Queiroz, A. (2014). The Monkey’s Voyage: How Improbable Journeys Shaped the History of Life. New York, NY: Basic Books.
Du, Y., Maslov, D. A., and Chang, K. P. (1994). Monophyletic origin of beta-division proteobacterial endosymbionts and their coevolution with insect trypanosomatid protozoa Blastocrithidia culicis and Crithidia spp. Proc. Natl. Acad. Sci. U.S.A. 91, 8437–8441. doi: 10.1073/pnas.91.18.8437
Excoffier, L., and Lischer, H. E. (2010). Arlequin suite ver 3.5: a new series of programs to perform population genetics analyses under Linux and Windows. Mol. Ecol. Resour. 10, 564–567. doi: 10.1111/j.1755-0998.2010.02847.x
Fiorini, J. E., Silva, P. M. F., Soares, M. J., and Brazil, R. P. (1989). Três novas espécies de tripanosomatídeos de insetos isolados em Alfenas, Minas Gerais, Brasil. Mem. Inst. Oswaldo Cruz 84, 69–74. doi: 10.1590/S0074-02761989000100013
Freymuller, E., and Camargo, E. P. (1981). Ultrastructural differences between species of trypanosomatids with and without endosymbionts. J. Protozool. 28, 175–182. doi: 10.1111/j.1550-7408.1981.tb02829.x
Godoy, W. A. C., von Zuben, C. J., dos Reis, S. F., and von Zuben, F. J. (1997). The spatial dynamics of native and introduced blowflies (Dipt., Calliphorodae). J. Appl. Entomol. 121, 305–309. doi: 10.1111/j.1439-0418.1997.tb01410.x
Guimarães, J. H., Prado, A. P., and Buralli, G. M. (1979). Dispersal and distribution of three newly introduced species of Chrysomya Robineau-Desvoidy in Brazil (Diptera: Calliphoridae). Rev. Bras. Zool. 23, 245–255.
Guimarães, J. H., Prado, A. P., and Linhares, A. X. (1978). Three newly introduced blowfly species in Southern Brazil (Diptera: Calliphoridae). Rev. Bras. Zool. 22, 53–60.
Hupperich, K., Camargo, E. P., and Milder, R. (1992). Ultrastructural study of the host-parasite relationship of trypanosomatids in the housefly. Parasitol. Res. 78, 48–55. doi: 10.1007/BF00936181
Imbiriba, A. S., Izutami, T., Milhoreto, I. T., and Luz, E. (1978). Introdução de Chrysomya chloropyga (Wiedeman, 1818) na região neotropical. Arq. Biol. Technol. 20, 35–39.
Junqueira, A. C., Azeredo-Espin, A. M., Paulo, D. F., Marinho, M. A., Tomsho, L. P., Drautz-Moses, D. I., et al. (2016). Large-scale mitogenomics enables insights into Schizophora (Diptera) radiation and population diversity. Sci. Rep. 6:21762. doi: 10.1038/srep21762
Kelly, D. W., Paterson, R. A., Townsend, C. R., Poulin, R., and Tompkins, D. M. (2009). Parasite spillback: a neglected concept in invasion ecology? Ecology 90, 2047–2056.
Klein, C. C., Alves, J. M., Serrano, M. G., Buck, G. A., Vasconcelos, A. T., Sagot, M. F., et al. (2013). Biosynthesis of vitamins and cofactors in bacterium-harbouring trypanosomatids depends on the symbiotic association as revealed by genomic analyses. PLOS ONE 8:e79786. doi: 10.1371/journal.pone.0079786
Kostygov, A. Y., Dobáková, E., Grybchuk-Ieremenko, A., Váhala, D., Maslov, D. A., Votıpka, J., et al. (2016). Novel trypanosomatid-bacterium association: evolution of endosymbiosis in action. mBio 7:e01985-15. doi: 10.1128/mBio.01985-15
Liedloff, A. C. (1999). Mantel Nonparametric Test Calculator. Version 2.0. School of Natural Resource Sciences. Brisbane, QLD: Queensland University of Technology.
Lukeš, J., Skalickı, T., Tıè, J., Votıpka, J., and Yurchenko, V. (2014). Evolution of parasitism in kinetoplastid flagellates. Mol. Biochem. Parasitol. 195, 115–122. doi: 10.1016/j.molbiopara.2014.05.007
Marinho, M. A., Junqueira, A. C., Paulo, D. F., Esposito, M. C., Villet, M. H., and Azeredo-Espin, A. M. (2012). Molecular phylogenetics of Oestroidea (Diptera: Calyptratae) with emphasis on Calliphoridae: insights into the inter-familial relationships and additional evidence for paraphyly among blowflies. Mol. Phylogenet. Evol. 65, 840–854. doi: 10.1016/j.ympev.2012.08.007
Markham, N. R., and Zuker, M. (2008). UNAFold: software for nucleic acid folding and hybridization. Methods Mol. Biol. 453, 3–31. doi: 10.1007/978-1-60327-429-6_1
Martin, D. P., Murrell, B., Golden, M., Khoosal, A., and Muhire, B. (2015). RDP4: detection and analysis of recombination patterns in virus genomes. Virus Evol. 1:vev003. doi: 10.1093/ve/vev003
Maslov, D. A., Votýpka, J., Yurchenko, V., and Lukeš, J. (2013). Diversity and phylogeny of insect trypanosomatids: all that is hidden shall be revealed. Trends Parasitol. 29, 43–52. doi: 10.1016/j.pt.2012.11.001
McDonagh, L. M., and Stevens, J. R. (2011). The molecular systematics of blowflies and screwworm flies (Diptera: Calliphoridae) using 28S rRNA, COX1 and EF-1α: insights into the evolution of dipteran parasitism. Parasitology 138, 1760–1777. doi: 10.1017/S0031182011001089
Mello, R. P. (1961). Contribuição ao estudo do gênero Phaenicia (Díptera: Calliphoridae). Mem. Inst. Oswaldo Cruz 59, 259–277. doi: 10.1590/S0074-02761961000300002
Morales, J., Kokkori, S., Weidauer, D., Chapman, J., Goltsman, E., Rokhsar, D., et al. (2016). Development of a toolbox to dissect host-endosymbiont interactions and protein trafficking in the trypanosomatid Angomonas deanei. BMC Evol. Biol. 16:247. doi: 10.1186/s12862-016-0820-z
Moran, N. A., McCutcheon, J. P., and Nakabachi, A. (2008). Genomics and evolution of heritable bacterial symbionts. Annu. Rev. Genet. 42, 165–190. doi: 10.1146/annurev.genet.41.110306.130119
Motta, M. C., Catta-Preta, C. M., Schenkman, S., de Azevedo Martins, A. C., Miranda, K., de Souza, W., et al. (2010). The bacterium endosymbiont of Crithidia deanei undergoes coordinated division with the host cell nucleus. PLOS ONE 5:e12415. doi: 10.1371/journal.pone.0012415
Motta, M. C., Martins, A. C., de Souza, S. S., Catta-Preta, C. M., Silva, R., Klein, C. C., et al. (2013). Predicting the proteins of Angomonas deanei, Strigomonas culicis and their respective endosymbionts reveals new aspects of the trypanosomatidae family. PLOS ONE 8:e60209. doi: 10.1371/journal.pone.0060209
Mundim, M. H., Roitman, I., Hermans, M. A., and Kitajima, E. W. (1974). Simple nutrition of Crithidia deanei, a reduviid trypanosomatid with an endosymbiont. J. Protozool. 21, 518–521. doi: 10.1111/j.1550-7408.1974.tb03691.x
Poinar, G. Jr. (2007). Early Cretaceous trypanosomatids associated with fossil sand fly larvae in Burmese amber. Mem. Inst. Oswaldo Cruz 102, 635–637. doi: 10.1590/S0074-02762007005000070
Poulin, R., and Mouillot, D. (2003). Host introductions and the geography of parasite taxonomic diversity. J. Biogeogr. 30, 837–845.
Richard, R. D., and Gerrish, R. R. (1983). The first confirmed field case of myiasis produced by Chrysomya sp. (Diptera: Calliphoridae) in the continental United States. J. Med. Entomol. 20:685. doi: 10.1093/jmedent/20.6.685
Rodrigues, R. A., de Azeredo-Espin, A. M., and Torres, T. T. (2009). Microsatellite markers for population genetic studies of the blowfly Chrysomya putoria (Diptera: Calliphoridae). Mem. Inst. Oswaldo Cruz 104, 1047–1050. doi: 10.1590/S0074-02762009000700020
Roitman, I., and Camargo, E. P. (1985). Endosymbionts of trypanosomatidae. Parasitol. Today 1, 143–144. doi: 10.1016/0169-4758(85)90060-2
Roubaud, E. (1908). Sur un nouveau flagellé parasite de líntestin des muscides au Congo français. C. R. Sc. Soc. Biol. 64, 1106–1108.
Roubaud, E. (1911a). Cystotrypanosoma intestinalis n. sp.; trypanosome vrai a reproduction kystique, de l’intestin dês mouches vertes (Lucilies) de L’Afrique tropicale. C. R. Sc. Soc. Biol. 71, 306–308.
Roubaud, E. (1911b). Sur un type nouveau de leptomonades intestinales des muscides, Leptomonas soudanensis n. sp., parasite des pycnosomes Africains. C. R. Sc. Soc. Biol. 71, 570–573.
Sbravate, C., Campaner, M., Camargo, L. E. A., Conchon, I., Teixeira, M. M. G., and Camargo, E. P. (1989). Culture and generic identification of trypanosomatids of phytophagous hemiptera in Brazil. J. Protozool. 36, 543–547. doi: 10.1111/j.1550-7408.1989.tb01093.x
Stamatakis, A. (2006). RAxML-VI-HPC: maximum likelihood-based phylogenetic analyses with thousands of taxa and mixed models. Bioinformatics 22, 2688–2690. doi: 10.1093/bioinformatics/btl446
Swofford, D. L. (2002). PAUP: Phylogenetic Analysis Using Parsimony (∗and other Methods), Version 4.0b 10. Sunderland: Sinauer.
Teixeira, M. M. G., Borghesan, T. C., Ferreira, R. C., Santos, M. A., Takata, C. S., and Campaner, M. (2011). Phylogenetic validation of the genera Angomonas and Strigomonas of trypanosomatids harboring bacterial endosymbionts with the description of new species of trypanosomatids and of proteobacterial symbionts. Protist 162, 503–524. doi: 10.1016/j.protis.2011.01.001
Torchin, M. E., Lafferty, K. D., Dobson, A. P., McKenzie, V. J., and Kuris, A. M. (2003). Introduced species and their missing parasites. Nature 421, 628–630. doi: 10.1038/nature01346
Týč, J., Votýpka, J., Klepetková, H., Suláková, H., Jirkù, M., and Lukeš, J. (2013). Growing diversity of trypanosomatid parasites of flies (Diptera: Brachycera): frequent cosmopolitism and moderate host specificity. Mol. Phylogenet. Evol. 69, 255–264. doi: 10.1016/j.ympev.2013.05.024
Votýpka, J., Kostygov, A. Y., Kraeva, N., Grybchuk-Ieremenko, A., Tesarova, M., Grybchuk, D., et al. (2014). Kentomonas gen. n., a new genus of endosymbiont-containing trypanosomatids of Strigomonadinae subfam. n. Protist 165, 825–838. doi: 10.1016/j.protis.2014.09.002
Wallace, F. G. (1966). The trypanosomatid parasites of insects and arachnids. Exp. Parasitol. 18, 124–193. doi: 10.1016/0014-4894(66)90015-4
Wallace, F. G. (1979). “Biology of the kinetoplastida of arthropods,” in Biology of the Kinetoplastida, eds W. H. R. Lumsden and D. A. Evans (London: Academic Press), 213–240.
Wells, J. D. (1991). Chrysomya megacephala (Diptera: Calliphoridae) has reached the continental United States: review of its biology, pest status, and spread around the world. J. Med. Entomol. 28, 471–473. doi: 10.1093/jmedent/28.3.471
Wells, K., O’Hara, R. B., Morand, S., Lessard, J. P., and Ribas, A. (2015). The importance of parasite geography and spillover effects for global patterns of host–parasite associations in two invasive species. Diversity Distrib. 21, 477–486. doi: 10.1111/ddi.12297
Williams, K. A., Lamb, J., and Villet, M. H. (2016). Phylogenetic radiation of the greenbottle flies (Diptera, Calliphoridae, Luciliinae). Zookeys 568, 59–86. doi: 10.3897/zookeys.568.6696
Keywords: endosymbiont, reduced genomes, fly parasites, transoceanic dispersal, co-evolution, phylogeography, parasite spillover, DNA barcoding
Citation: Borghesan TC, Campaner M, Matsumoto TE, Espinosa OA, Razafindranaivo V, Paiva F, Carranza JC, Añez N, Neves L, Teixeira MMG and Camargo EP (2018) Genetic Diversity and Phylogenetic Relationships of Coevolving Symbiont-Harboring Insect Trypanosomatids, and Their Neotropical Dispersal by Invader African Blowflies (Calliphoridae). Front. Microbiol. 9:131. doi: 10.3389/fmicb.2018.00131
Received: 06 July 2017; Accepted: 19 January 2018;
Published: 07 February 2018.
Edited by:
Michael Thomas-Poulsen, University of Copenhagen, DenmarkReviewed by:
David William Waite, The University of Queensland, AustraliaEnrique Medina-Acosta, State University of Norte Fluminense, Brazil
Copyright © 2018 Borghesan, Campaner, Matsumoto, Espinosa, Razafindranaivo, Paiva, Carranza, Añez, Neves, Teixeira and Camargo. This is an open-access article distributed under the terms of the Creative Commons Attribution License (CC BY). The use, distribution or reproduction in other forums is permitted, provided the original author(s) and the copyright owner are credited and that the original publication in this journal is cited, in accordance with accepted academic practice. No use, distribution or reproduction is permitted which does not comply with these terms.
*Correspondence: Marta M. G. Teixeira, bW1ndGVpeEBpY2IudXNwLmJy Erney P. Camargo, ZXJuZXlAdXNwLmJy
†These authors have contributed equally to this work.