- 1Department of Biology and Monte L. Bean Life Science Museum, Brigham Young University, Provo, UT, United States
- 2Museum of Evolution, Uppsala University, Uppsala, Sweden
- 3Science and Education, The Field Museum, Chicago, IL, United States
- 4Research School of Chemistry, Australian National University, Canberra, ACT, Australia
- 5Natural History Museum, University of Oslo, Oslo, Norway
- 6Department of Biotechnology, Iranian Research Organization for Science and Technology, Tehran, Iran
- 7Plant Ecology and Systematics, Biology Institute, University of Kaiserslautern, Kaiserslautern, Germany
- 8Department of Botany, Swedish Museum of Natural History, Stockholm, Sweden
Multiple drivers shape the spatial distribution of species, including dispersal capacity, niche incumbency, climate variability, orographic barriers, and plate tectonics. However, biogeographic patterns of fungi commonly do not fit conventional expectations based on studies of animals and plants. Fungi, in general, are known to occur across exceedingly broad, intercontinental distributions, including some important components of biological soil crust communities (BSCs). However, molecular data often reveal unexpected biogeographic patterns in lichenized fungal species that are assumed to have cosmopolitan distributions. The lichen-forming fungal species Psora decipiens is found on all continents, except Antarctica and occurs in BSCs across diverse habitats, ranging from hot, arid deserts to alpine habitats. In order to better understand factors that shape population structure in cosmopolitan lichen-forming fungal species, we investigated biogeographic patterns in the cosmopolitan taxon P. decipiens, along with the closely related taxa P. crenata and P. saviczii. We generated a multi-locus sequence dataset based on a worldwide sampling of these taxa in order to reconstruct evolutionary relationships and explore phylogeographic patterns. Both P. crenata and P. decipiens were not recovered as monophyletic; and P. saviczii specimens were recovered as a monophyletic clade closely related to a number of lineages comprised of specimens representing P. decipiens. Striking phylogeographic patterns were observed for P. crenata, with populations from distinct geographic regions belonging to well-separated, monophyletic lineages. South African populations of P. crenata were further divided into well-supported sub-clades. While well-supported phylogenetic substructure was also observed for the nominal taxon P. decipiens, nearly all lineages were comprised of specimens collected from intercontinental populations. However, all Australian specimens representing P. decipiens were recovered within a single well-supported monophyletic clade consisting solely of Australian samples. Our study supports up to 10 candidate species-level lineages in P. decipiens, based on genealogical concordance and coalescent-based species delimitation analyses. Our results support the general pattern of the biogeographic isolation of lichen-forming fungal populations in Australia, even in cases where closely related congeners have documented intercontinental distributions. Our study has important implications for understanding factors influencing diversification and distributions of lichens associated with BSC.
Introduction
Many symbiotic fungi have distinctive biogeographic patterns that do not conform with conventional expectations based on studies of animals and plants (MacArthur and Wilson, 1967; Galloway, 2008; Peay et al., 2010). Among symbiotic fungi, lichen-forming fungal lineages (Lücking et al., 2016b) are well known for unique and varied biogeographic patterns (Galloway, 2008; Werth, 2011). Species distributions of lichen-forming fungi range from truly widespread, intercontinental species with broad ecological amplitude (Wirtz et al., 2008; Fernández-Mendoza et al., 2011) to those with geographically and ecologically restricted distributions (Lücking et al., 2016a). While factors determining the establishment of populations of cosmopolitan species of lichen-forming fungi are not well understood, dynamic interactions among a variety of historical and ecological factors play important roles in determining species distributions (Leavitt and Lumbsch, 2016), e.g., reproductive strategies (Hilmo et al., 2012), availability of symbiotic partners (Fernández-Mendoza and Printzen, 2013; Werth and Sork, 2014), niche incumbency (Culberson and Culberson, 1967), and historical biogeography (Weber, 2003; Amo de Paz et al., 2012).
The origin of widely disjunct populations of lichen-forming fungi is not well understood for most lineages due to limited information on the temporal scale of divergences and population structure. Some species with disjunct populations may have had more contiguous distributions during the Tertiary period, with connections between North America and Asia, e.g., via Beringia or the North Pole by way of Greenland (Weber, 2003). Alternatively, intercontinental populations may be a result of long-distance dispersal events or migration into ecologically similar, disjunct habitats (Geml et al., 2012).
A number of cosmopolitan lichens occur in biological soil crust communities (BSCs) where they play important ecological roles (Büdel, 2003). The ecological importance of BSC communities is well-documented for arid and semiarid areas, the predominant environments for BSCs, which comprise approximately one third of the Earth’s total land area (Bowker et al., 2008). However, BSCs are also commonly found in temperate, subalpine, alpine, and nival zones (Hansen, 2003; Türk and Gärtner, 2003). In spite of the overall importance of lichens in BSCs and the well-documented degradation of these communities worldwide (Belnap et al., 2001), our understanding of the processes driving biogeographic and diversification patterns for soil crust lichens are poorly understood.
The lichen-forming fungal species Psora decipiens (Hedwig) Hoffm. is a common component of BSCs worldwide and occurs in habitats ranging from hot, arid deserts to alpine tundra/steppes (Timdal, 1984). The ability of P. decipiens to form symbiotic relationships with a broad range of photosynthetic algal partners, including multiple lineages within Asterochloris and Trebouxia, has been proposed as an important factor contributing to the successful establishment of this lichen across diverse habitats (Ruprecht et al., 2014). However, conflicting results suggested that the range of suitable photosynthetic partners for P. decipiens may actually be restricted to only two distinct clades in the green algal genus Myrmecia (Geitler, 1963; Williams et al., 2017). European populations of P. decipiens are comprised of distinct genotypes apparently adapted to specific climactic conditions, which are likely unable to acclimate to the different environmental conditions found within the species range or switch photobiont partners across short time scales (Williams et al., 2017). However, genetic diversity in populations outside of Europe have not been well characterized.
While the occurrence of P. decipiens as disjunct populations in Asia, Europe, and North America may be an artifact of much broader distribution predating the Quaternary (Weber, 2003), the origin of populations in Australia are particularly enigmatic. Australia includes one of the largest arid biomes in the world, supporting a unique, diverse and relatively well-studied biota (Byrne et al., 2008), although the impact of the isolation and aridification of Australia on symbiotic fungal radiations remains largely unexplored. From a biogeographic perspective, lichen-forming fungi in Australia are, in general, well-separated from their closest related congeners (Argüello et al., 2007; Elix et al., 2009; Amo de Paz et al., 2012). The physical origin of Australia’s desert biome is generally well characterized and includes three major events: (i) the rifting of Australia from Antarctica (accelerated ca. 32 Ma); (ii) the advent of the Antarctic Circumpolar Current; and (iii) the onset of severe aridity during the Pliocene (Crisp et al., 2004). The tectonic isolation of Australia led to a transition from an aseasonal-wet biome to the unique Australian sclerophyll biomes dominated by eucalypts, acacias, and casuarinas, accompanied by another major shift in the Australian biota during the late Oligocene and Miocene (Crisp et al., 2004). Dispersal of P. decipiens to Australia likely occurred via long distance dispersal by spores, and BSC-type habitats suitable for P. decipiens may not have been present in Australia before the Pliocene.
Psora decipiens appears to belong to a group of closely related soil dwelling lichens, including P. crenata (Taylor) Reinke and P. saviczii (Tomin) Follmann & A. Crespo. Generally, P. crenata is separated from P. decipiens by the presence of larger squamules with a prominent central depression and the presence of the extrolite norstictic acid. However, morphological and chemical variation in P. decipiens is well known (Timdal, 1984), including populations of P. decipiens with norstictic acid in Australia, the Mediterranean, and some Arctic localities. P. saviczii is morphologically similar to P. decipiens but differs largely based on its occurrence on gypsum-based soils in central Spain, where it has been considered a threatened lichen as defined by IUCN categories (Atienza and Segarra, 2000). Given the absence of fixed diagnostic characters separating these taxa and overlapping geographic and ecological distributions, an alternative view is that P. crenata, P. decipiens, and P. saviczii should be considered a single variable species.
In order to better understand the biogeographic and diversification patterns of cosmopolitan BSC lichens, we sampled Psora specimens (P. crenata, P. decipiens, and P. saviczii) from worldwide populations in Australia, Central Asia, Europe, the Middle East, North America, and South Africa. From this sampling, we generated a multi-locus sequence dataset which we used to reconstruct evolutionary relationships for these three taxa. Our specific research questions were: (i) What are the phylogenetic relationships among P. crenata, P. decipiens, and P. saviczii? (ii) Are P. decipiens populations structured phylogeographically? (iii) How isolated are Australian populations from other P. decipiens populations? and (iv) Can we place the diversification history of this group within a temporal context?
Materials and Methods
Taxon Sampling
Our sampling focused on the cosmopolitan lichen-forming taxon P. decipiens, and closely related species P. crenata and P. saviczii; and a total of 159 specimens were included (Supplementary Table S1). Psora crenata and P. decipiens are broadly distributed, and specimens were opportunistically sampled with the intent to represented a wide range of populations dispersed across broad geographic and ecological distributions. Our sampling of P. crenata included 25 specimens: three from western North America, one from the Middle East (Yemen), and 21 from South Africa. Psora decipiens was represented by 129 specimens, including 16 from Asia [Iran (11); Russia (5)], 35 from Australia, 57 from Europe, and 25 from North America (Figure 1). Specimens representing P. saviczii were sampled from Spain (3) and Russia (1). Latitude and longitude were used to extract bioclimatic variables at a spatial resolution of 2.5 min, using the R (R Core Team, 2015) package raster (Hijmans et al., 2015). All specimens were examined using an Olympus SZH stereomicroscope, and, for selected specimens, thin-layer chromatography (TLC) was performed following the methods of Culberson C.F. (1972), as modified by Menlove (1974) and Culberson and Johnson (1982). A single specimen representing P. tuckermanii R. Anderson ex Timdal was selected as the outgroup based on Ekman and Blaalid (2011) and exploratory analyses.
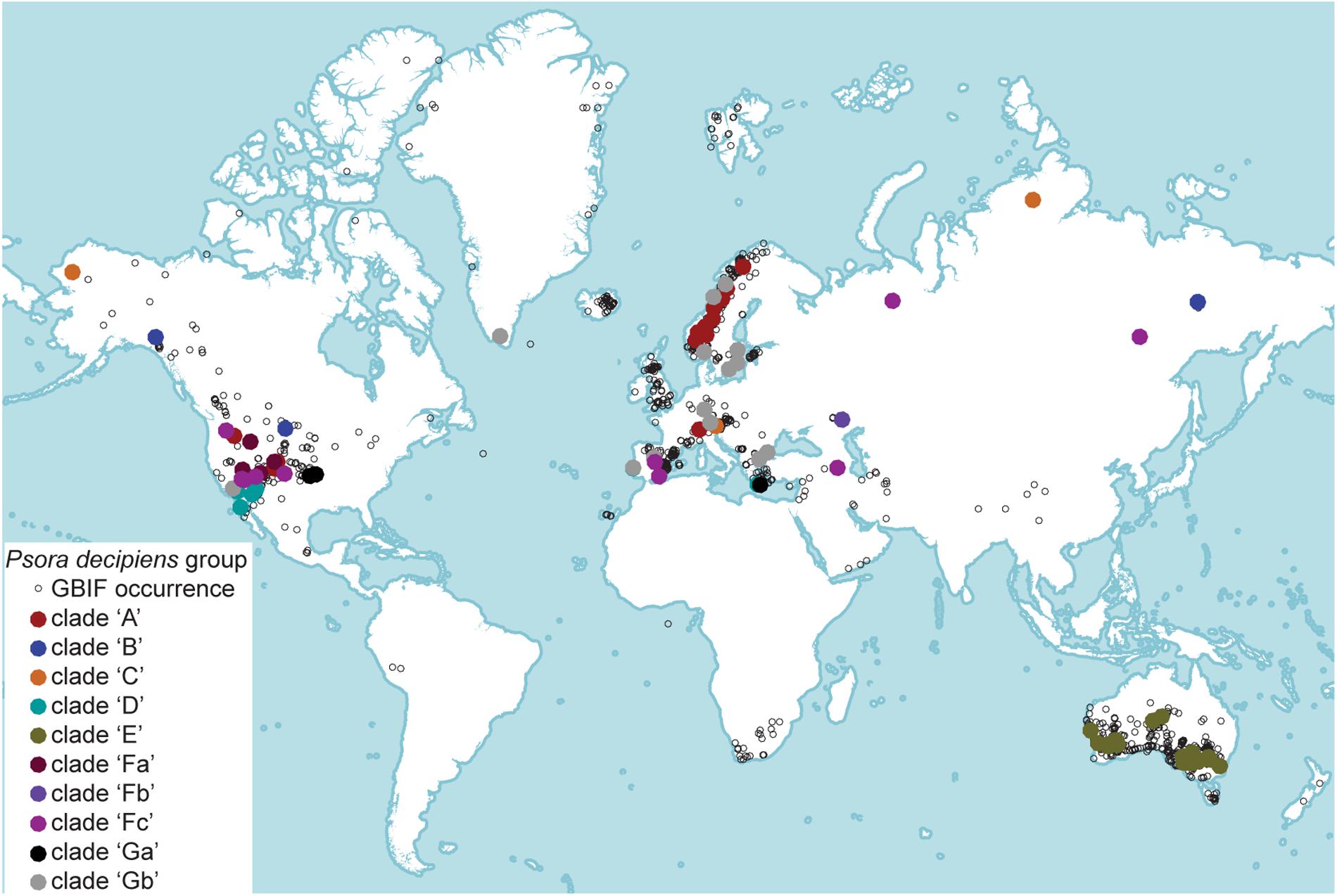
FIGURE 1. The colored, filled circles represent the candidate species. Geographic distribution of Psora decipiens s. lat. Empty, black circles represent records from Global Biodiversity Information Facility (GBIF; https://www.gbif.org). Colored circles represent specimens included in the present study, and different colors correspond to the distinct candidate species circumscribed using molecular sequence data.
DNA Extraction, Amplification, and Sequencing
Total genomic DNA was extracted from specimens using the USB PrepEase Genomic DNA Kit (Affymetrix, Santa Clara, CA, United States – product discontinued), DNeasy Plant MiniKit (Qiagen), or the ZR fungal/bacterial DNA miniprep kit (Zymo Research). We generated molecular sequence data for a total of five markers: two nuclear ribosomal loci were sampled, including the large-subunit (nuLSU) and the internal transcribed spacer region (ITS – including ITS1, 5.8S, and ITS2); a fragment of the mitochondrial small subunit (mtSSU); and fragments from two protein-coding loci, the mini-chromosome maintenance complex component 7 (MCM7), and RNA polymerase II largest subunit (RPB1). Sequences were generated using Sanger sequencing, and primers and conditions for polymerase chain reaction (PCR) amplifications for all loci follow previous studies (Blanco et al., 2004; Schmitt et al., 2009; Leavitt et al., 2013b). PCR amplifications were performed using Ready-To-Go PCR Beads (GE Healthcare, Pittsburgh, PA, United States), with cycling parameters following a 55–50°C touchdown reaction (Lindblom and Ekman, 2006). PCR products were visualized on 1% agarose gel and cleaned using ExoSAP-IT (USB, Cleveland, OH, United States). Complementary strands were sequenced using the same primers used for amplifications, and sequencing reactions were performed using BigDye 3.1 (Applied Biosystems, Foster City, CA, United States). Products were run on an automated sequencer (ABI Prism 377) located in the Molecular Systematic Laboratory at the Swedish Museum of Natural History in Stockholm or an ABI 3730 automated sequencer (Applied Biosystems) at the Pritzker Laboratory for Molecular Systematics and Evolution at the Field Museum, Chicago, IL, United States and the DNA Sequencing Center at Brigham Young University, Provo, UT, United States.
Sequences Assembly, Multiple Sequence Alignments and Estimating Substitution Models
Bi-directional Sanger reads were assembled and edited using the program Sequencher 4.10 (Gene Codes Corporation, Ann Arbor, MI, United States), and sequences were aligned using the program MAFFT 7 (Katoh et al., 2005; Katoh and Toh, 2008). We specified the G-INS-i alignment algorithm and ‘1PAM/K = 2’ scoring matrix, with an offset value of 0.9. The remaining parameters were set to default values for the protein-coding (MCM7 and RPB2) and nuLSU markers. For the mtSSU, we used the same parameters, with the exception of an offset value set to 0.1 rather than 0.9 and ‘unalignlevel’ set to 0.6. Exploratory multiple sequence alignments of the ITS resulted in a substantial proportion of ambiguously aligned regions across the P. crenata, P. decipiens, and P. saviczii groups. Therefore, the ITS data was only aligned for the P. decipiens group, including P. saviczii. Each of subclade within the P. decipiens group (see Results) was aligned individually using the G-INS-I and ‘1PAM/K = 2’ scoring matrix, with an offset value of 0.1, and the remaining parameters were set to default values for the protein-coding. Individual sub-alignments were merged using MAFFT using the same parameters as described above for each individual sub-alignment. For the Bayesian analysis (see below), substitution models for each locus were estimated using jModelTest v.2.1.10 (Darriba et al., 2012).
Phylogenetic Analyses and Divergence Time Estimates
Phylogenetic relationships among the sampled fungal lineages were inferred using both maximum likelihood (ML) and Bayesian inference (BI) methods. Due to ambiguities in an exploratory ITS alignment across all samples, ML topologies were inferred from two concatenated multi-locus data matrices: (1) a four-locus matrix comprised of the nuLSU, MCM7, RPB1, and mtSSU sequences representing the complete taxon sampling – P. tuckermanii (outgroup), P. crenata, P. decipiens, and P. saviczii; and (2) a five-locus matrix comprised of the ITS, nuLSU, MCM7, RPB1, and mtSSU alignments for the P. decipiens and P. saviczii samples only (outgroup and P. crenata excluded). ML phylogenies were inferred using the program RAxML v8.2.1 (Stamatakis, 2006; Stamatakis et al., 2008) in the CIPRES Science Gateway server1, the data matrices were partitioned by locus, and applying the GTRGAMMA’ model. Nodal support was evaluated using 1000 bootstrap pseudo-replicates.
We used the program BEAST v1.8.3 (Drummond and Rambaut, 2007; Heled and Drummond, 2010) to reconstruction relationships within a Bayesian framework. Relevant fossil and rate calibrations are not available for Psoraceae. However, as a hypothesis-generating approach, we used a substitution rate for the RPB1 estimated for the entire Lecanorales, 1.65 substitutions/site/year X-9 (Amo de Paz et al., 2011), to place the diversification of the P. decipiens group within a temporal context. Bayesian topologies were inferred from the two concatenated multi-locus data matrices defined above – the four marker dataset representing the complete taxon sampling and the five-locus dataset representing the focal group, P. decipiens and P. saviczii. Substitution rates were co-estimated for each locus under a uniform prior and relative to the fixed 1.65 substitutions/site/year X-9 rate for the RPB1. The BI analyses were performed using a Yule speciation process prior and with the data matrix partitioned by individual gene regions, and applying the locus-specific models inferred using jModelTest (see section “Results”). Divergence times were estimated under a strict molecular clock (Drummond et al., 2006), and two independent MCMC runs of 25 million generations were performed, sampling every 1000 steps. Chain mixing and convergence were evaluated in Tracer v1.6 (Rambaut and Drummond, 2003), considering ESS values > 200 as good indicators. After excluding the first 25% of sampled trees as burn-in, trees from the two independent runs were combined using the program LogCombiner v1.8.3 (Rambaut and Drummond, 2013), and the final MCC tree was estimated from the combined posterior distribution of trees using TreeAnnotator v1.8.3 (Rambaut and Drummond, 2009).
Delimiting Candidate Species
Well-supported phylogenetic substructure was inferred from the multi-locus data matrices, and we treated distinct, well-supported clades within the nominal species P. crenata and P. decipiens as candidate species-level lineages (see section “Results”). For the P. decipiens group, which included P. saviczii, relationships of candidate species were evaluated among individual ITS, MCM7, and RPB1 topologies to identify lineages that exhibited genealogical exclusivity across multiple loci (Avise and Ball, 1990; Hudson and Coyne, 2002). The presence of the same clades in the majority of single-locus phylogenies is taken as evidence that the clades represent reproductively isolated lineages (Dettman et al., 2003; Pringle et al., 2005). Genealogical concordance provides a conservative approach for robustly delimiting species boundaries among putative lineages, although it may fail to delimit species with more recent diversification histories due to incomplete lineage sorting (Leavitt et al., 2016a). Individual locus topologies were inferred using the program RAxML v8.2.1 in the CIPRES Science Gateway server2. Nodal support was evaluated using 1000 bootstrap pseudo-replicates.
The evolutionary independence of the 10 candidate species representing P. decipiens was validated using program BPP v3.2 (Yang and Rannala, 2010, 2014; Rannala and Yang, 2013). We used the unguided species delimitation analysis ‘A11’ (Yang, 2015), which explores different species delimitation models and different species phylogenies, with fixed specimen assignments to populations. Analysis ‘A00’ (Yang, 2015), a within-model inference, was used to generate the posterior distribution of the parameters theta (𝜃s) and tau (τs) under the multispecies coalescent model (MSC) model to infer a reasonable combination of priors given the data (Rannala, 2015). Based on the results from the ‘A00’ analyses, the gamma prior G for 𝜃 was set to ∼G(1,125), and the gamma prior G for tau (τ) was set to ∼G(1,300). Under the unguided species delimitation model, ‘A11,’ we used two different search algorithms (algorithm 0 or 1), with equal probabilities for the labeled histories, to assign probabilities to the models, rates were allowed to vary among loci (locus rate = 1), and the analyses were set for automatic fine-tune adjustments. The rjMCMC analysis was run for 100,000 generations, sampling every two generations and discarding the first 10% as burn-in. The analysis was run twice to confirm consistency between runs.
Niche Data Comparisons among Candidate Species
Individual bioclimatic variables were compared among candidate species using boxplots. Data are provided in SI. Additionally, a phylogenetic PCA was performed to help visualize each candidate species distribution in multidimensional niche space. The four-gene phylogeny was reduced to include a single representative of each candidate species, and the mean value for each bioclimatic variable was obtained for each candidate species. A phylogenetic PCA using the correlation matrix was then run in the R package phytools (Revell, 2012).
Results
Molecular Sequence Data
New sequences generated in association with this study have been deposited in GenBank under accession numbers MG677156–MG677545; MG783593–MG783845. Both data matrices – the complete taxon sampling (nuLSU, MCM7, RPB1, and mtSSU) and the P. decipiens group (ITS, nuLSU, MCM7, RPB1, and mtSSU) – and associated tree files were submitted to TreeBase (submission ID: 20946). Locus-specific substitution models inferred using jModelTest were: nuLSU, GTR+I+G; ITS, SYM+I+G; MCM7, TrNef+I; RPB1, SYM+G; and mtSSU, TPM1uf.
Phylogenetic Relationships and Estimated Divergence Times
Both the ML and BI phylogenetic reconstructions resulted in similar topologies, providing a generally well-resolved backbone for the P. crenata/P. decipiens clade (Figure 2). Neither P. crenata nor P. decipiens were recovered as monophyletic in analyses of the complete data matrix (Figure 2); and P. saviczii (clade ‘Fb’) was recovered as a monophyletic clade nested among a number of clades comprised of P. decipiens specimens (Figure 2). P. crenata specimens were recovered in three, deeply diverging clades, each corresponding to the three distinct geographic regions sampled for this study: The Middle East (Yemen; one sample), South Africa, and western North America (Figure 2). Furthermore, well-supported phylogenetic substructure was reconstructed for P. crenata specimens collected from South Africa, but with no clear regional biogeographic structuring. Phylogenetic reconstructions of the complete taxon sampling revealed distinct lineages comprised of P. decipiens samples (Figure 2), and seven well-supported clades – ‘A’ through ‘G’– were recovered in analyses of the five-locus dataset representing the P. decipiens core group (Figure 3). Clade ‘F’ was further divided into three subclades, ‘Fa,’ ‘Fb,’ and ‘Fc,’ with clade ‘Fb’ comprised exclusively of all P. saviczii specimens. Two subclades were also recognized in clade ‘G,’ ‘Ga,’ and ‘Gb’ (Figure 3).
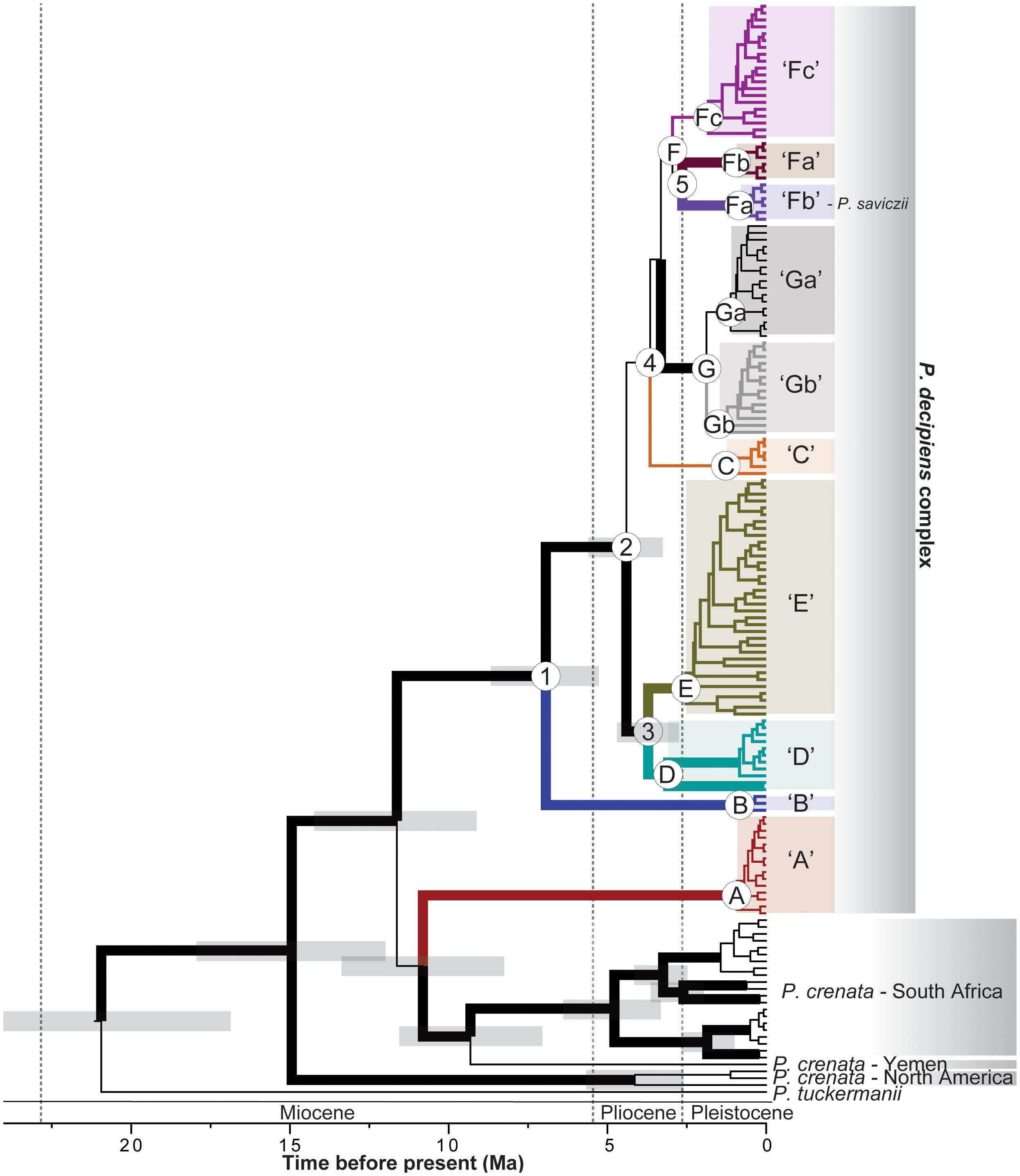
FIGURE 2. A rate-calibrated chronogram for the P. crenata, P. decipiens, and P. saviczii clade. The topology was inferred from a four-marker (nuLSU, MCM7, RPB1, and mtSSU) data matrix using the program BEAST; and divergence times were estimated using a fixed rate for the RPB1 locus (1.65 substitutions/site/year X-9) previously estimated for the lichen-forming fungal order Lecanorales. The Oligocene-Miocene (O/M), Miocene-Pliocene (M/P), and Pliocene-Pleistocene (P/P) boundaries are indicated by dashed vertical lines. Posterior probabilities ≥ 0.95 are indicated with thickened branches. Candidate species from the P. decipiens complex are indicated by distinct colors and codes (‘A’–‘Gb’) and correspond to identical colors in all other figures. Number nodes are referred to in the text.
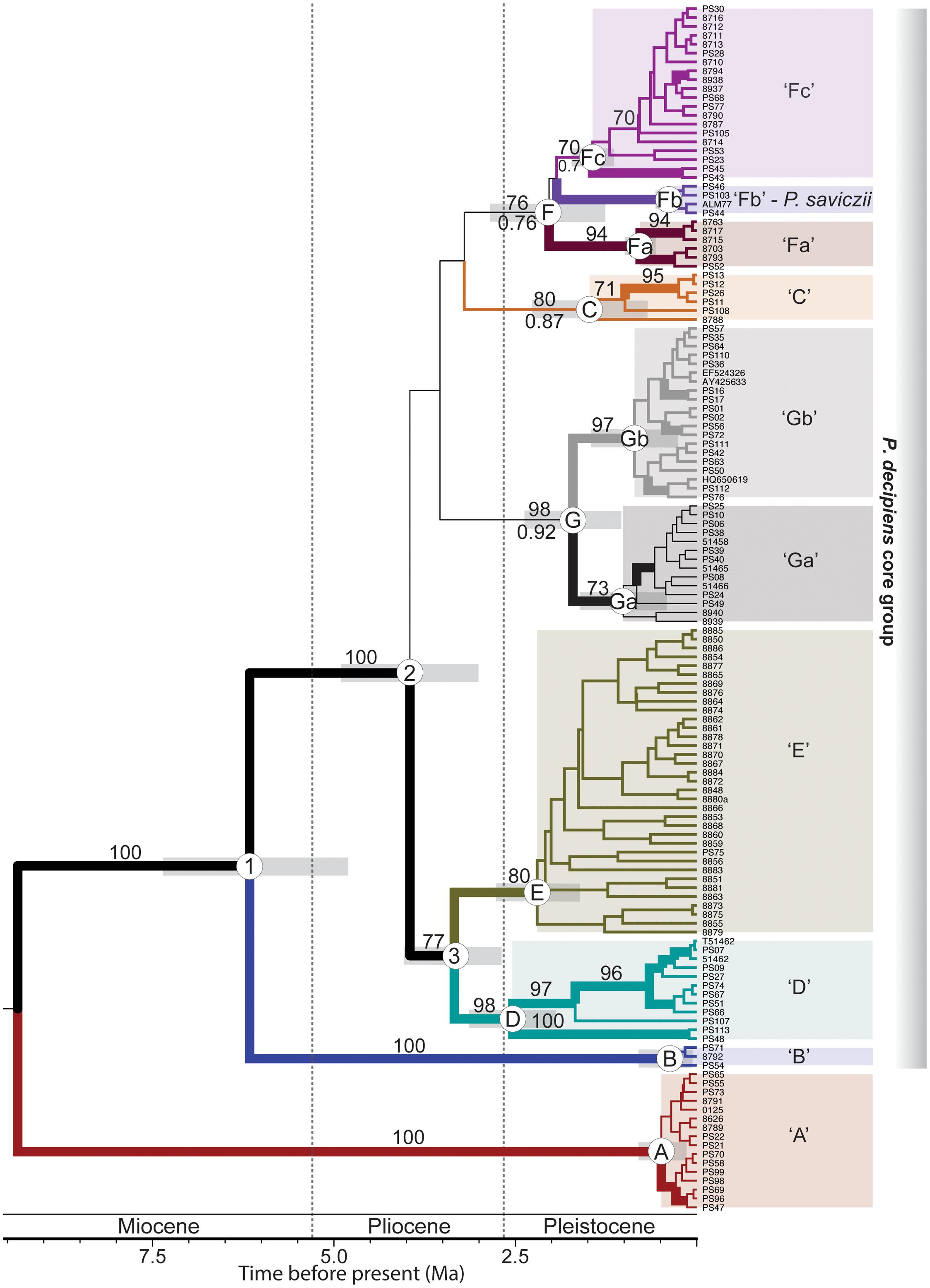
FIGURE 3. A rate-calibrated chronogram for the P. decipiens complex. The topology was inferred from a five-marker (ITS, nuLSU, MCM7, RPB1, and mtSSU) data matrix using the program BEAST; and divergence times were estimated using a fixed rate for the RPB1 locus (1.65 substitutions/site/year X-9) previously estimated for the lichen-forming fungal order Lecanorales. The Miocene-Pliocene and Pliocene-Pleistocene boundaries are indicated by dashed vertical lines. Posterior probabilities ≥ 0.95 are indicated with thickened branches; and maximum likelihood bootstrap is indicated above the branches. Candidate species from the P. decipiens complex are indicated by distinct colors and codes (‘A’–‘Gb’) and correspond to identical colors in all other figures. Number nodes are referred to in the text.
Each clade representing the P. decipiens core group included specimens collected from intercontinental distributions, with the exception of clade ‘E,’ which was comprised exclusively of Australian specimens (Figure 1 and Table 1). Confirmed geographic distributions and habitat preferences for each clade are summarized in Table 1. Specimens recovered in clades ‘A’ and ‘C’ were collected from high altitude/latitude populations in Europe, North America, and Russia, occurring in regions with generally lower temperatures and higher precipitation than those from the other clades (Supplementary Figure S1). However, no bioclimatic variable clearly demarked the ecological preferences of the other clades, although some differences are identifiable at regional scales. For example, the Scandinavian P. decipiens specimens were recovered in two distinct clades: one found in inland populations (clade ‘A’) and the other occurring in more coastal soil crust communities (clade ‘Gb’). Other intercontinental populations of P. decipiens were also recovered in both clades ‘A’ and ‘Gb,’ and not all specimens recovered in clade ‘Gb’ were collected from soil crust communities with a maritime influence, e.g., populations in Germany and Southern California, United States (Figure 1 and Supplementary Table S1). TLC results revealed the presence of multiple chemotypes in a number of P. decipiens lineages (clades ‘D,’ ‘E,’ ‘Fc,’ ‘Ga,’ and ‘Gb’), with Australian specimens, clade ‘E,’ harboring the highest extrolite diversity. No compounds were detected in the sampled specimens recovered in clades ‘A,’ ‘B,’ ‘C,’ ‘Fa,’ and ‘Fb’ (P. saviczii) (Table 1 and Supplementary Table S1).
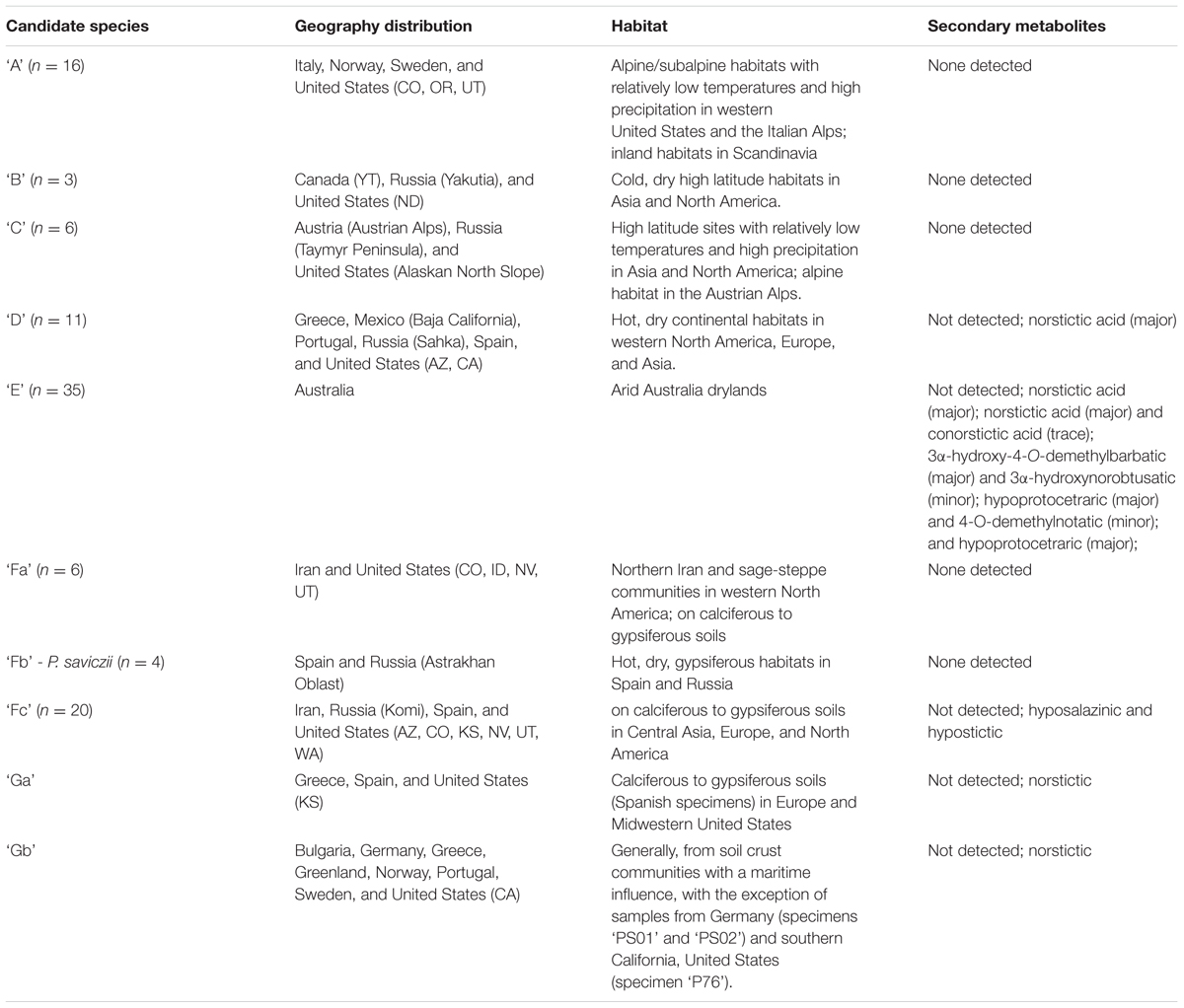
TABLE 1. Generalized information summarized for candidate species circumscribed in this study, including geographic distributions, habitat preferences, and secondary metabolites.
Our estimates suggest a most recent common ancestor (MRCA) for the P. crenata/P. decipiens clade at ca. 15 Ma [95% Highest Posterior Density (HPD) = 12.1–18.1 Ma] and a MRCA for the core P. decipiens group at ca. 7 Ma (95% HPD = 5.4–8.7 Ma) (node ‘1,’ Figure 2). Our estimates of divergence times for the P. crenata/P. decipiens clade support a Miocene-dominated diversification history for the major lineages within this group and a Pliocene-dominated history for the core group of P. decipiens lineages (clades ‘B’ – ‘G’) (Figure 2). Similar divergent times were inferred from the 5-locus matrix restricted to the P. decipiens core group, which included the ITS, nuLSU, MCM7, RPB1, and mtSSU alignments (Figure 3). The MRCA for each candidate species-level lineage circumscribed here most likely occurred in the Quaternary (Figure 3). For the analysis of the P. decipiens core group, substitution rates co-estimated relative to the 1.65 substitutions/site/year X-9 for the RPB1 locus were: nuLSU = 1.22 X-9 s/s/y (95% HPD interval = 0.83–1.65 X-9 s/s/y); ITS = 5.38 X-9 s/s/y (95% HPD interval = 4.11–6.82 X-9 s/s/y); MCM7 = 2.27 X-9 s/s/y (95% HPD interval = 1.63–2.97 X-9 s/s/y); and mtSSU = 0.31 X-9 s/s/y (95% HPD interval = 0.16–0.48 X-9-9 s/s/y).
Candidate Species
Individual locus topologies showed a general pattern of genealogical concordance for the 10-candidate species in the P. decipiens core group that were initially delimited based on well-supported phylogenetic structure (Figure 4). These species-level lineages were further supported by high speciation probabilities (>0.99) in the coalescent-based validation method BPP. All candidate species delimited in the P. decipiens core group reflected high genetic variability, with the exception of clade ‘A’ (Table 2).
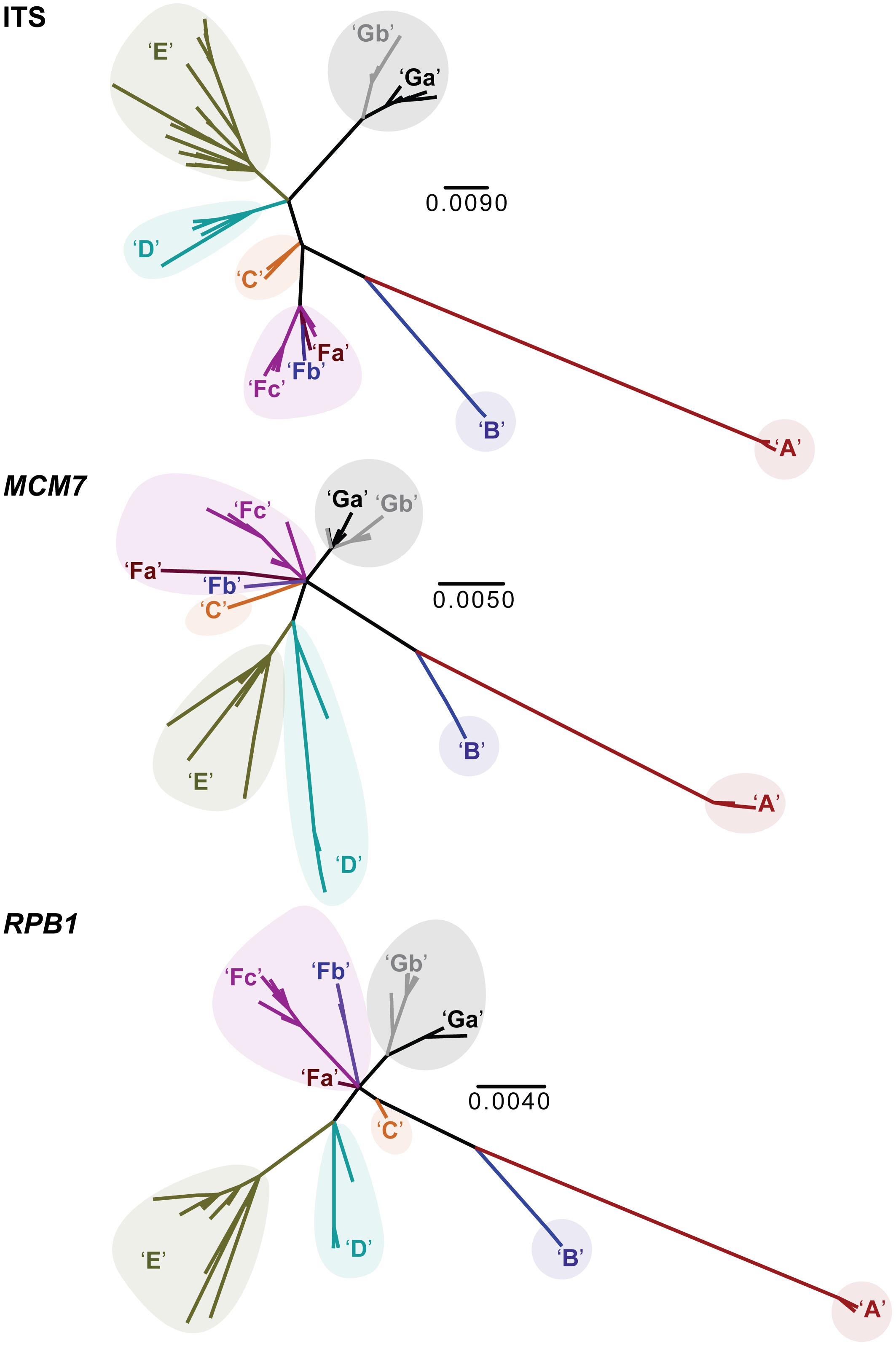
FIGURE 4. Unrooted individual locus topologies for the ITS, MCM7, and RPB1 markers. Candidate species are indicated by color and code (‘A’–‘Gb’), corresponding to identical colors in all other figures. Scale bar in each panel represents substitutions per site.
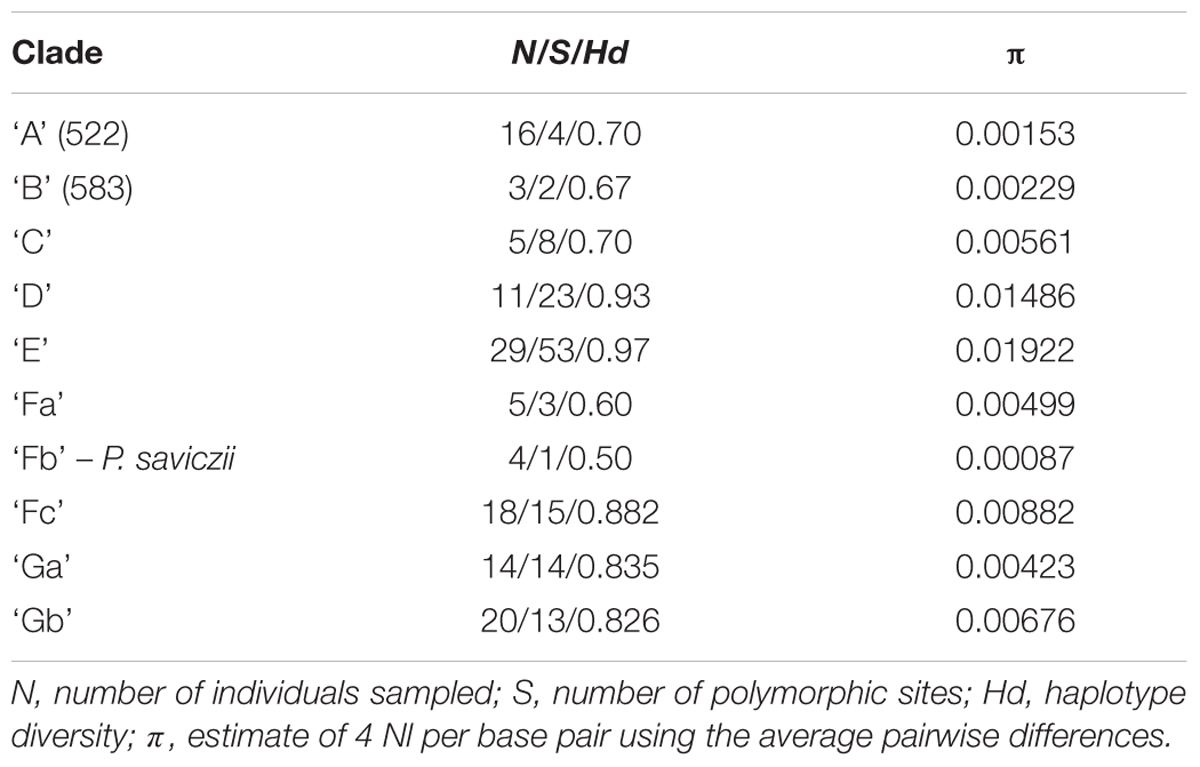
TABLE 2. Polymorphism statistics for the internal transcribed spacer region (ITS) in candidate species within the P. decipiens complex.
Discussion
Characterizing evolutionary processes and biogeographic patterns in widely distributed components of BSCs has important implications, ranging from management and conservation to biodiversity inventories to providing an improved framework for ecological and evolutionary research. Our study of the cosmopolitan lichen-forming taxon P. decipiens, and closely related species P. crenata and P. saviczii, reveals complex biogeographic patterns, previously unrecognized species-level diversity, and a diversification history spanning millions of years for this important clade of biological soil crust lichens. Phylogenies inferred from multi-locus sequence data provide a generally well-supported hypothesis of relationships and novel insight into diversity and distributions of the sampled taxa and worldwide populations (Figure 2). Neither P. crenata nor P. decipiens were recovered as monophyletic and multiple species-level lineages were circumscribed in both nominal taxa based on molecular data. Strikingly, our results revealed multiple widespread, intercontinental candidate species comprised of P. decipiens specimens, in contrast to geographically restricted species-level lineages representing P. crenata populations. However, all Australian populations representing the nominal taxon P. decipiens belonged to a singled species-level clade that was not sampled outside of Australia. Below we discuss the implications of our study for better understanding the diversification of lichen-forming fungi in BSCs commonly associated with and arid and semi-arid habitats.
Contrasting Patterns of Geographically Restricted and Intercontinental Species-Level Lineages
Floristic similarities in widely disjunct geographic regions have long fascinated biologists and are well-known in lichen-forming fungi (Culberson W.L., 1972; Galloway, 2008). However, due to limited information on genetic population structure and the temporal scale of divergences, the origin of widely disjunct populations remains unclear for most cosmopolitan species. Two general explanations of disjunct lichen distributions prevail: (1) species with disjunct populations had more contiguous distributions during the Tertiary period; or the alternative explanation that (2) disjunct populations are a result of long-distance dispersal events or migration into ecologically similar, disjunct regions (Weber, 2003). For example, in the case of the former, Weber (2003) characterized the Rocky Mountain flora as “a microcosm of its rich Middle Asiatic counterpart,” with the present disjunctions representing relict populations of a once widely distributed Oroboreal flora. However, more recent studies suggest a more nuanced perspective into the temporal and spatial components of diversification in cosmopolitan lichen-forming fungi species, highlighting the role of long-distance dispersal during the Quaternary for many species (Buschbom, 2007; Geml et al., 2010; Otálora et al., 2010; Fernández-Mendoza and Printzen, 2013; Leavitt et al., 2013a).
Our study of P. decipiens, based on data from worldwide populations, also supports the idea of effective long-distance dispersal by soil crust lichens. In fact, nine of the 10 candidate species circumscribed from our cosmopolitan sampling of P. decipiens s. lat. occurred in disjunct, intercontinental populations (Table 1). While the MRCA for the core P. decipiens group was estimated to be during the late Miocene (Node ‘1,’ Figure 3), the MRCA for specimens comprising each putative species within this group was inferred to have occurred during the Pleistocene. If disjunct P. decipiens populations were relicts of past continuous distributions predating the Pleistocene we would expect to observe biogeographically structured clades with deeper divergence times, rather than intercontinental lineages with similar haplotypes shared across disjunct populations. In spite of the capacity of P. decipiens s. lat. for effective long-distance dispersal across Asia, Europe and North America, our results suggest all extant populations in Australia resulted from only a single dispersal event. The Australian clade, clade ‘E,’ shares a MRCA with its sister clade, clade ‘D’ during the mid-Pliocene (node ‘3,’ Figure 3), a time of increasing aridity worldwide (Crisp et al., 2004). In southwestern Australia, the prevailing mild wet climate that prevailed into the Pliocene was replaced by the extreme wet–dry glacial cycles typical of the present climatic system that began approximately 2.9 Ma (Dodson and Macphail, 2004); likely resulting in suitable habitat and environmental conditions for successful establishment of BSC communities.
In contrast to the core P. decipiens group, P. crenata s. lat. populations showed strong phylogeographic patterns, with populations in North American, South Africa, and The Middle East forming three distinct lineages, with deeper divergence times than the core P. decipiens group (Figure 2). In spite of the widespread, intercontinental distributions of many lichens species, South African and Australian populations of nominal taxa with intercontinental distributions are commonly found to belong to distinct evolutionary lineages (Argüello et al., 2007; Hodkinson and Lendemer, 2011). While we were unable to obtain fresh specimens representing P. crenata from Australia, we would predict that these specimens would likely be recovered as a fourth major lineage representing this nominal taxon.
Both global cooling and mountain uplift have been proposed as playing important roles in the long-term aridification during the Mid–Late Miocene (Miao et al., 2012), and our divergence estimates for the P. crenata/P. decipiens clade generally coincide with this pattern of aridification (Figure 2). The Miocene also appears to be a major epoch of diversifications for other lineages of lichen-forming fungi, including many lineages in the hyper-diverse lichen-forming fungal family Parmeliaceae (Amo de Paz et al., 2011; Kraichak et al., 2015), as well as others in Lecanoraceae (Leavitt et al., 2016b). Our results suggest that the expansion of open habitats during the last 5 million years (Cerling et al., 1997; Fortelius et al., 2006) played a major role in diversification in the P. decipiens core group (Figure 3). Similar patterns have been observed for other organisms adapted to open habitats, including falcons (Fuchs et al., 2015), C4 grasslands (Edwards et al., 2010), other lichen-forming fungi (Amo de Paz et al., 2012; Leavitt et al., 2013b), and humans (Bobe and Behrensmeyer, 2004).
In spite of the interesting correlations between major global climatic shifts and divergence times estimated for the P. crenata/P. decipiens clades, we emphasize that our divergence estimates must be interpreted with caution. There are significant limitations to the rate-calibrated approach implemented here and based on an order-wide estimate for a single locus (Amo de Paz et al., 2011). While recent studies highlight the promise of incorporating fossil evidence into divergence dating of lichen-forming fungi (Divakar et al., 2015; Kaasalainen et al., 2015), to our knowledge, no relevant fossil evidence is available for the placing the diversification of the genus Psora into a temporal framework. Similarly, more accurate estimates of substitution rates are not available for Psoraceae. Therefore, we strongly caution against over-interpreting our divergence estimates and only include them as a hypothesis-generating approach for considering the temporal context of diversification in this group.
Potential for Ecological Specialization in the Psora decipiens Core Group
The results of this study indicate that P. decipiens specimens occurring across diverse ecological habitats are commonly found in distinct, species-level clades (Table 1). In some cases, ecological differences, rather than geographic proximity may be a better predictor of clade membership. Regions with ecologically diverse habitats, such as the southwestern portion of the United States, harbor a wide range candidate species, while populations that are isolated across thousands of kilometers may belong to a single species-level lineage, e.g., geographically isolated populations in Russia belong to the same species-level lineage ‘Fc’ (Figure 1). However, based on limitations of our current sampling (small sample sizes for a number of lineages, limited environmental data at collecting sites, etc.), robustly characterizing ecological differences among the candidate species is beyond the scope of this study. Results from previously published studies and the evidence presented here highlight the importance of additional research into ecological specialization in the P. decipiens group.
Williams et al. (2017) identified four distinct clades representing European populations of P. decipiens and demonstrated that different genotypes were unable to acclimate to different environmental conditions within the species’ range. Additional evidence suggests that differences among these clades may be related to the regulation of thallus water content, with some clades specifically adapted to conditions found in semi-arid habitats, while others are better adapted to seasonally wet, alpine habitats (Colesie et al., 2017). Our additional sampling supports this distinction. Specifically, clade ‘C’ (this study) corresponds to the ‘Austria’ clade [Figure 5 in Williams et al. (2017)]; and all specimens comprising this clade were collected for alpine sites characterized by relatively lower temperatures and higher precipitation (Supplementary Figure S1). However, our sampling also revealed another alpine-adapted lineage of P. decipiens s. lat, clade ‘A’ (Figure 3 and Supplementary Figure S1), with a somewhat different geographic distribution relative to clade ‘C’ (Figure 1 and Table 1). A third clade appears to be restricted to relatively cold habitats in North America, clade ‘B,’ but differs from clades ‘A’ and ‘C’ by its occurrence in habitats with much lower precipitation (Supplementary Figure S1). Additional studies are required to more explicitly characterize potential ecological differences among these cold-adapted candidate species, ‘A,’ ‘B,’ and ‘C.’ However, the phylogenetic PCA, corroborated these results, with temperature (BIOCLIM variables 1–3, 5–11), precipitation (BIOCLIM variables 12–19), and seasonality (BIOCLIM variables 4 and 7) generally correlating with candidate species-level lineages circumscribed here (Supplementary Figure S2).
The remaining candidate species representing P. decipiens s. lat. – ‘D,’ ‘E,’ ‘Fa,’ ‘Fb’ (P. saviczii), ‘Fc,’ ‘Gb,’ and ‘Gc’ – are found in warmer, drier habitats (Table 1 and Supplementary Figure S1). Clades ‘D,’ ‘Fc,’ and ‘Gb’ correspond to the ‘Spain,’ ‘Germany,’ and ‘Germany/Sweden’ clades, respectively, in Williams et al. (2017; Figure 5). In North America, the majority of the species-level lineages occur on calciferous soil in open habitats ranging from deserts to coniferous forests. Similarly, these lineages are found widely distributed in Asia, Europe, and Central Asia, with the exception of clade ‘E,’ which occurs exclusively in Australia. Additional sampling, coupled with ecological niche modeling (Austin, 2007), will likely provide important insights into the role of ecological specialization in the P. decipiens group. In addition to looking at bioclimatic variables that are commonly used to infer niche envelopes (Araújo and Peterson, 2012), investigating the role of physical properties of substrates may provide important insight into differences in distributions among these candidate species. P. decipiens s. lat. generally occurs generally on calciferous to gypsiferous soils, including P. saviczii (clade ‘Fb’) which occurs exclusively on highly gypsiferous soils and may co-occur with other P. decipiens s. lat. lineages. Whether differences in soil properties are related to species distributions in the P. decipiens groups remains untested. Availability of suitable photosynthetic algae (photobionts) may be another factor influencing the distribution of these lineages. Although previous studies suggested high photobiont diversity associating with the P. decipiens group (Ruprecht et al., 2014), a more recent study suggests that P. decipiens s. lat. may associate with a much narrower range of photobionts than previously thought (Williams et al., 2017). Additional research is required to elucidate the role of selection and specificity in structuring P. decipiens distributions.
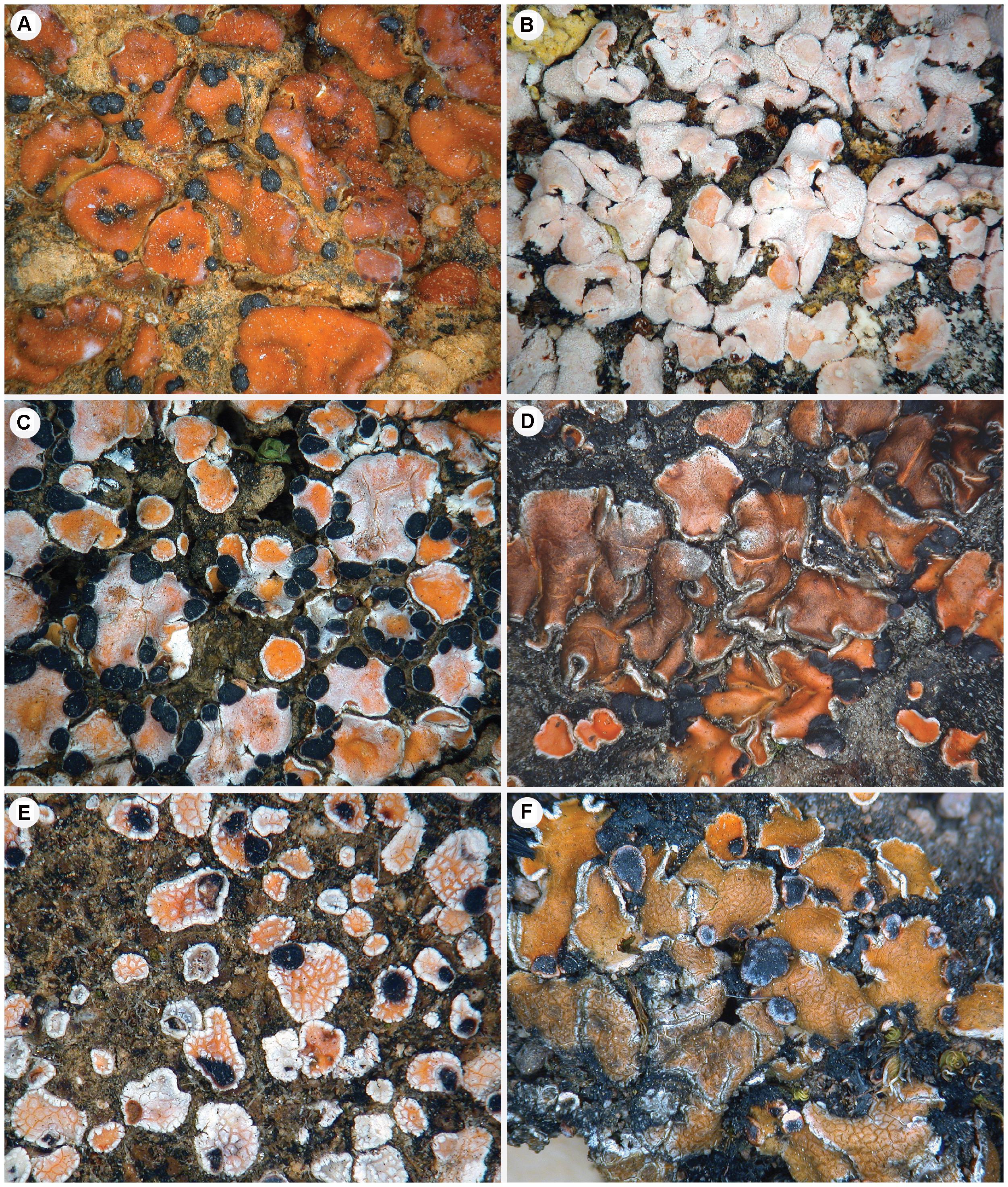
FIGURE 5. Morphological variation in the P. crenata s. lat. and P. decipiens s. lat. group. (A) P. crenata from the North Cape of South Africa (specimen ‘PS87’ [F268261 - S]). (B) P. saviczii from Spain, clade ‘Fb’ (specimen ‘PS46’ [F283532 - S]). (C) P. decipiens s. lat. from Spain, clade ‘D’ (specimen ‘PS09’ [Westberg SCIN077 - S]); (D) P. decipiens s. lat. from Austria, clade ‘C’ (specimen ‘PS12’ [Westberg HOCH042 - S]). (E) P. decipiens s. lat. from Spain, clade ‘Ga’ (specimen ‘PS06’ [Westberg SCIN071 -S]). (F) P. decipiens s. lat. from Sweden, clade ‘Gb’ (specimen ‘PS19’ [Westberg GYN051 - S]).
Taxonomic Implications
While both P. crenata and P. decipiens were traditionally thought to be widespread taxa within broad, intercontinental distributions (Timdal, 1984), our results show that a number of species-level lineages have been masked within these nominal taxa. Based on our results, specimens identified as P. crenata are clearly distinct from P. decipiens s. lat. However, what has traditionally been considered P. crenata, likely comprises at least three species-level lineages with distinct biogeography distributions – western North America, South Africa, and the Middle East (Figure 2). Given the deep divergence among these lineages and distinct phylogeographic patterns, there is convincing evidence that these represent valid species. While our current sampling did not include Australian populations of P. crenata, the chemistry in specimens in Australia and South Africa is highly variable. Future work investigating anatomical and morphological features, chemistry, and additional specimen sampling will be important to resolve the taxonomy and evolutionary relationships within this nominal taxon. In the meantime, we do not plan to make any formal taxonomic revisions for P. crenata s. lat., but encourage additional research into this group. Furthermore, we emphasize the importance of future studies to investigate patterns of gene flow and/or genetic population structure in this nominal taxon in order to better understand the evolutionary independence of these biogeographically structured lineages.
Similar to P. crenata, we also present convincing evidence that P. decipiens is comprised of up to 10 species-level lineages, some of which have distinct geographic and ecological distributions (Williams et al., 2017). However, pending detailed ecological and morphological studies of these candidate species, we refrain from making any formal taxonomic recommendations. In spite of the high degree of morphological variability in P. crenata s. lat. and P. decipiens s. lat. populations (Figure 5), exploratory morphological and chemical comparisons failed to reveal fixed diagnostic characters separating species-level lineages circumscribed here. Further complicating formal taxonomic revisions in P. crenata and P. decipiens is the fact that more than 10 epithets are available (Zahlbruckner, 1934), and linking candidate species circumscribed here with available epithets is beyond the scope of this project.
Our results reveal that a simplistic approach using the presence or absence of distinct secondary metabolites will fail to distinguish species for most groups circumscribed here, as a number of lineages were found to be chemically polymorphic and or included specimens in which no extrolites were detected (Table 1). In a few cases, such as the hyposalazinic/hypostictic acid chemotypes in P. decipiens s. lat. clade ‘Fc,’ distinct secondary metabolites appear to be restricted to specific species-level clades, although some acid-deficient specimens were also recovered in this clade.
Future investigations into the candidate species circumscribed in this study should be complemented with other lines of evidence in an integrative taxonomic approach (Dayrat, 2005; Fujita et al., 2012). From an evolutionary perspective, is not prerequisite that observable morphological differences coincide with evolutionarily independent lineages, although phenotypic traits have traditionally been used as proxies to infer evolutionary independence. In some cases, corroborating phenotypic characters have supported lichen-forming fungal species circumscribed using molecular sequence data (Divakar et al., 2005; Schneider et al., 2016), but in others, no diagnostic phenotypic traits segregating species have been observed.
Potential Directions for Future Studies
Global aridification and development of open habitats during the Neogene have been proposed to play important roles in diversification of lichen-forming fungal components of BSC. However, in spite of the fact that Australia includes one of the largest arid biomes in the world, much less is known regarding the timing and potential factors influencing diversification of symbiotic fungi in Australia in general (Amo de Paz et al., 2012). Additional studies investigating the temporal component of fungal diversification in Australia, especially in comparison to other arid regions, will likely provide key insights into the processes of diversification in this unique continent. Specifically, studying other groups of lichenized fungi will provide insight into whether or not there are similar underlying factors that have shaped fungal diversity in Australia.
Southern Africa is another fascinating region in terms of lichen biogeography (Hale, 1990). In this study, striking phylogenetic diversity was revealed in the nominal taxon P. crenata, with at least three candidate species revealed in South Africa alone. However, we were unable to sample populations representing P. decipiens from South Africa, and the relationship of these populations with P. crenata populations, and other P. decipiens, remains unknown. P. crenata and P. decipiens are morphological variable in South Africa, and intermediates forms not easily identifiable to either taxa are commonly encountered. Additional collections from South Africa will be required to elucidate the relationships of these populations to the lineages recovered in this study. Based on our limited sampling of Psora populations, it appears that intercontinental dispersal and long-distance dispersal may be less frequent in the Southern hemisphere when compared to similar populations in the Northern Hemisphere (e.g., North America, Europe, and Central Asia). Additional research will be required to adequately test this hypothesis and assess potential limitations to dispersal.
The relationship of P. decipiens populations in a number of other major regions have not yet been assessed within a molecular phylogenetic context, including South America (Peru), Tibet Plateau/Himalayas, Middle East, and Central Asia (Figure 1). Although P. saviczii (clade ‘Fb’) is known from a relatively small geographic distribution in hot, dry, gypsiferous habitats in Spain and Russia, and this taxon was represented by only four specimens. Sampling additional populations throughout the species range will be essential to confirm that P. saviczii is, in fact, monophyletic.
Our sampling of P. crenata was relatively sparse in comparison to P. decipiens. Representing additional populations throughout the range of this taxon will likely reveal novel biogeographic insight and may influence subsequent phylogenetic inference and interpretations.
Author Contributions
SL and MarW designed and implemented this study. MN, LS, LW, MatW, ET, and HL made substantial contributions to the conceptual design of this work. SL, MarW, MS, JE, ET, LS, and MatW were central to the acquisition of specimens and other data. SL led the analytical component of this study, and all co-authors made substantial contributions to the interpretation of data. SL drafted the manuscript, with significant contributions from all co-authors.
Funding
This research was funded, in part, by a start-up grant from BYU College of Life Sciences to SL; MarW’s and MatW’s work was done within the European Soil Crust Project SCIN (Büdel et al., 2014) funded by the ERA-Net BiodivERsA program, with the national funder The Swedish Research Council for Environment, Agricultural Sciences and Spatial Planning (FORMAS).
Conflict of Interest Statement
The authors declare that the research was conducted in the absence of any commercial or financial relationships that could be construed as a potential conflict of interest.
Acknowledgments
We express our gratitude to Roger Rosentreter, Ted Esslinger, Bettina Weber, Burkhard Büdel, Alica Košuthová, Ulrike Ruprecht, Roman Türk, and Laura Concostrina for generously providing specimens for this study. We also appreciate the thoughtful comments from the reviewers that improved this manuscript.
Supplementary Material
The Supplementary Material for this article can be found online at: https://www.frontiersin.org/articles/10.3389/fmicb.2018.00283/full#supplementary-material
FIGURE S1 | Bioclimatic data for 19 Bioclim variables for candidate species. Latitude and longitude were used to extract bioclimatic variables at a spatial resolution of 2.5 min, using the R (R Core Team, 2015) package raster (Hijmans et al., 2015).
FIGURE S2 | Niche ecospace as described by PC1 and PC2, derived from a phylogenetic PCA on the mean candidate species values for 19 bioclim variables. Candidate species are plotted by name, and eigenvectors as arrows. Together, PC1 (66.4%) and PC2 (20.2%) account for account for >86% of the variation. Temperature and precipitation variables are associated with PC1, while temperature seasonality and annual range are associated with PC2. Abbreviations for individual bioclim variables correspond to the following: bio1 = Annual Mean Temperature; bio2 = Mean Diurnal Range [Mean of monthly (max temperature - min temperature)]; bio3 = Isothermality (bio2/bio7) (∗ 100); bio4 = Temperature Seasonality (standard deviation ∗100); bio5 = Max Temperature of Warmest Month; bio6 = Min Temperature of Coldest Month; bio7 = Temperature Annual Range (bio5-bio6); bio8 = Mean Temperature of Wettest Quarter; bio9 = Mean Temperature of Driest Quarter; bio10 = Mean Temperature of Warmest Quarter; bio11 = Mean Temperature of Coldest Quarter; bio12 = Annual Precipitation; bio13 = Precipitation of Wettest Month; bio14 = Precipitation of Driest Month; bio15 = Precipitation Seasonality (Coefficient of Variation); bio16 = Precipitation of Wettest Quarter; bio17 = Precipitation of Driest Quarter; bio18 = Precipitation of Warmest Quarter; bio19 = Precipitation of Coldest Quarter.
TABLE S1 | List of specimens included in this study.
Footnotes
References
Amo de Paz, G., Cubas, P., Crespo, A., Elix, J. A., and Lumbsch, H. T. (2012). Transoceanic dispersal and subsequent diversification on separate continents shaped diversity of the Xanthoparmelia pulla group (Ascomycota). PLoS One 7:e39683. doi: 10.1371/journal.pone.0039683
Amo de Paz, G., Cubas, P., Divakar, P. K., Lumbsch, H. T., and Crespo, A. (2011). Origin and diversification of major clades in parmelioid lichens (Parmeliaceae, Ascomycota) during the Paleogene inferred by Bayesian analysis. PLoS One 6:e28161. doi: 10.1371/journal.pone.0028161
Araújo, M. B., and Peterson, A. T. (2012). Uses and misuses of bioclimatic envelope modeling. Ecology 93, 1527–1539. doi: 10.1890/11-1930.1
Argüello, A., Del Prado, R., Cubas, P., and Crespo, A. (2007). Parmelina quercina (Parmeliaceae, Lecanorales) includes four phylogenetically supported morphospecies. Biol. J. Linn. Soc. 91, 455–467. doi: 10.1111/j.1095-8312.2007.00810.x
Atienza, V., and Segarra, J. G. (2000). Preliminary red list of the lichens of the Valencian community (eastern Spain). For. Snow Landsc. Res. 75, 391–400.
Austin, M. (2007). Species distribution models and ecological theory: a critical assessment and some possible new approaches. Ecol. Model. 200, 1–19. doi: 10.1016/j.ecolmodel.2006.07.005
Avise, J. C., and Ball, R. M. (1990). Principles of genealogical concordance in species concepts and biological taxonomy. Oxford Surv. Evol. Biol. 7, 45–67.
Belnap, J., Kaltenecker, J. H., Rosentreter, R., Williams, J., Leonard, S., and Eldridge, D. (2001). Biological Soil Crusts: Ecology and Management. Denver, CO: US Department of the Interior, 110.
Blanco, O., Crespo, A., Elix, J. A., Hawksworth, D. L., and Lumbsch, H. T. (2004). A molecular phylogeny and a new classification of parmelioid lichens containing Xanthoparmelia-type lichenan (Ascomycota: Lecanorales). Taxon 53, 959–975. doi: 10.2307/4135563
Bobe, R., and Behrensmeyer, A. K. (2004). The expansion of grassland ecosystems in Africa in relation to mammalian evolution and the origin of the genus Homo. Palaeogeogr. Palaeoclimatol. Palaeoecol. 207, 399–420. doi: 10.1016/j.palaeo.2003.09.033
Bowker, M. A., Miller, M. E., Belnap, J., Sisk, T. D., and Johnson, N. C. (2008). Prioritizing conservation effort through the use of biological soil crusts as ecosystem function indicators in an arid region. Conserv. Biol. 22, 1533–1543. doi: 10.1111/j.1523-1739.2008.01036.x
Büdel, B. (2003). “Synopsis: comparative biogeography of soil-crust biota,” in Biological Soil Crusts: Structure, Function, and Management, eds J. Belnap and O. L. Lange (Berlin: Springer), 141–152.
Büdel, B., Colesie, C., Green, T. G. A., Grube, M., Lázaro Suau, R., Loewen-Schneider, K., et al. (2014). Improved appreciation of the functioning and importance of biological soil crusts in Europe: the Soil Crust International Project (SCIN). Biodivers. Conserv. 23, 1639–1658. doi: 10.1007/s10531-014-0645-2
Buschbom, J. (2007). Migration between continents: geographical structure and long-distance gene flow in Porpidia flavicunda (lichen-forming Ascomycota). Mol. Ecol. 12, 957–968. doi: 10.1111/j.1365-294X.2007.03258.x
Byrne, M., Yeates, D. K., Joseph, L., Kearney, M., Bowler, J., Williams, M. A. J., et al. (2008). Birth of a biome: insights into the assembly and maintenance of the Australian arid zone biota. Mol. Ecol. 17, 4398–4417. doi: 10.1111/j.1365-294X.2008.03899.x
Cerling, T. E., Harris, J. M., Macfadden, B. J., Leakey, M. G., Quade, J., Eisenmann, V., et al. (1997). Global vegetation change through the Miocene/Pliocene boundary. Nature 389, 153–158. doi: 10.1007/s00114-008-0500-y
Colesie, C., Williams, L., and Büdel, B. (2017). Water relations in the soil crust lichen Psora decipiens are optimized via anatomical variability. Lichenologist 49, 483–492. doi: 10.1017/S0024282917000354
Crisp, M., Cook, L., and Steane, D. (2004). Radiation of the Australian flora: what can comparisons of molecular phylogenies across multiple taxa tell us about the evolution of diversity in present-day communities? Philos. Trans. R. Soc. Lond. B Biol. Sci. 359, 1551–1571. doi: 10.1098/rstb.2004.1528
Culberson, C. F. (1972). Improved conditions and new data for identification of lichen products by standardized thin-layer chromatographic method. J. Chromatogr. A 72, 113–125. doi: 10.1016/0021-9673(72)80013-X
Culberson, C. F., and Johnson, A. (1982). Substitution of methyl tert.-butyl ether for diethyl ether in the standardized thin-layer chromatographic method for lichen products. J. Chromatogr. A 238, 483–487. doi: 10.1016/S0021-9673(00)81336-9
Culberson, W. L. (1972). Disjunctive distributions in the lichen-forming fungi. Ann. Mo. Bot. Gard. 59, 165–173. doi: 10.2307/2394751
Culberson, W. L., and Culberson, C. F. (1967). Habitat selection by chemically differentiated races of lichens. Science 158, 1195–1197. doi: 10.1126/science.158.3805.1195
Darriba, D., Taboada, G. L., Doallo, R., and Posada, D. (2012). jModelTest 2: more models, new heuristics and parallel computing. Nat. Methods 9:772. doi: 10.1038/nmeth.2109
Dayrat, B. (2005). Towards integrative taxonomy. Biol. J. Linn. Soc. 85, 407–415. doi: 10.1111/j.1095-8312.2005.00503.x
Dettman, J. R., Jacobson, D. J., and Taylor, J. W. (2003). A multilocus genealogical approach to phylogenetic species recognition in the model eukaryote Neurospora. Evolution 57, 2703–2720. doi: 10.1111/j.0014-3820.2003.tb01514.x
Divakar, P. K., Crespo, A., Wedin, M., Leavitt, S. D., Hawksworth, D. L., Myllys, L., et al. (2015). Evolution of complex symbiotic relationships in a morphologically derived family of lichen-forming fungi. New Phytol. 208, 1217–1226. doi: 10.1111/nph.13553
Divakar, P. K., Molina, M. C., Lumbsch, H. T., and Crespo, A. (2005). Parmelia barrenoae, a new lichen species related to Parmelia sulcata (Parmeliaceae) based on molecular and morphological data. Lichenologist 37, 37–46. doi: 10.1017/S0024282904014641
Dodson, J. R., and Macphail, M. K. (2004). Palynological evidence for aridity events and vegetation change during the Middle Pliocene, a warm period in Southwestern Australia. Glob. Planet. Change 41, 285–307. doi: 10.1016/j.gloplacha.2004.01.013
Drummond, A., Ho, S., Phillips, M., and Rambaut, A. (2006). Relaxed phylogenetics and dating with confidence. PLoS Biol. 4:e88. doi: 10.1371/journal.pbio.0040088
Drummond, A., and Rambaut, A. (2007). BEAST: Bayesian evolutionary analysis by sampling trees. BMC Evol. Biol. 7:214. doi: 10.1186/1471-2148-7-214
Edwards, E. J., Osborne, C. P., Strömberg, C. A. E., and Smith, S. A. (2010). The origins of C4 grasslands: integrating evolutionary and ecosystem science. Science 328, 587–591. doi: 10.1126/science.1177216
Ekman, S., and Blaalid, R. (2011). The devil in the details: interactions between the branch-length prior and likelihood model affect node support and branch lengths in the phylogeny of the Psoraceae. Syst. Biol. 60, 541–561. doi: 10.1093/sysbio/syr022
Elix, J. A., Corush, J., and Lumbsch, H. T. (2009). Triterpene chemosyndromes and subtle morphological characters characterise lineages in the Physcia aipolia group in Australia (Ascomycota). Syst. Biodivers. 7, 479–487. doi: 10.1017/S1477200009990223
Fernández-Mendoza, F., Domaschke, S., García, M. A., Jordan, P., Martin, M. P., and Printzen, C. (2011). Population structure of mycobionts and photobionts of the widespread lichen Cetraria aculeata. Mol. Ecol. 20, 1208–1232. doi: 10.1111/j.1365-294X.2010.04993.x
Fernández-Mendoza, F., and Printzen, C. (2013). Pleistocene expansion of the bipolar lichen Cetraria aculeata into the Southern hemisphere. Mol. Ecol. 22, 1961–1983. doi: 10.1111/mec.12210
Fortelius, M., Eronen, J., Liu, L., Pushkina, D., Tesakov, A., Vislobokova, I., et al. (2006). Late Miocene and Pliocene large land mammals and climatic changes in Eurasia. Palaeogeogr. Palaeoclimatol. Palaeoecol. 238, 219–227. doi: 10.1016/j.palaeo.2006.03.042
Fuchs, J., Johnson, J. A., and Mindell, D. P. (2015). Rapid diversification of falcons (Aves: Falconidae) due to expansion of open habitats in the Late Miocene. Mol. Phylogenet. Evol. 82(Pt A), 166–182. doi: 10.1016/j.ympev.2014.08.010
Fujita, M. K., Leache, A. D., Burbrink, F. T., Mcguire, J. A., and Moritz, C. (2012). Coalescent-based species delimitation in an integrative taxonomy. Trends Ecol. Evol. 9, 480–488. doi: 10.1016/j.tree.2012.04.012
Galloway, D. J. (2008). “Lichen biogeography,” in Lichen Biology, 2nd Edn, ed. T. H. Nash (New York, NY: Cambridge University Press), 315–335. doi: 10.1017/CBO9780511790478.017
Geitler, L. (1963). Über haustorien bei flechten und über Myrmecia biatorellae in Psora globifera. Österreichische Bot. Z. 110, 270–280. doi: 10.1007/BF01373133
Geml, J., Kauff, F., Brochmann, C., Lutzoni, F., Laursen, G. A., Redhead, S. A., et al. (2012). Frequent circumarctic and rare transequatorial dispersals in the lichenised agaric genus Lichenomphalia (Hygrophoraceae, Basidiomycota). Fungal Biol. 116, 388–400. doi: 10.1016/j.funbio.2011.12.009
Geml, J., Kauff, F., Brochmann, C., and Taylor, D. L. (2010). Surviving climate changes: high genetic diversity and transoceanic gene flow in two arctic–alpine lichens, Flavocetraria cucullata and F. nivalis (Parmeliaceae, Ascomycota). J. Biogeogr. 37, 1529–1542. doi: 10.1111/j.1365-2699.2010.02287.x
Hale, M. E. (1990). A Synopsis of the Lichen Genus Xanthoparmelia (Vainio) Hale (Ascomycotina, Parmeliaceae). Washington, DC: Smithsonian Institution Press. doi: 10.5962/bhl.title.123253
Hansen, E. S. (2003). “Lichen-rich soil crusts of arctic Greenland,” in Biological Soil Crusts: Structure, Function, and Management, eds J. Belnap and O. L. Lange (Berlin: Springer), 57–65.
Heled, J., and Drummond, A. J. (2010). Bayesian inference of species trees from multilocus data. Mol. Biol. Evol. 27, 570–580. doi: 10.1093/molbev/msp274
Hijmans, R., Phillips, S., Leathwick, J., and Elith, J. (2015). dismo: Species Distribution Modeling. R Package Version 1.0-12. Available at: http://CRAN.R-project.org/package=dismo
Hilmo, O., Lundemo, S., Holien, H., Stengrundet, K., and Stenøien, H. K. (2012). Genetic structure in a fragmented Northern Hemisphere rainforest: large effective sizes and high connectivity among populations of the epiphytic lichen Lobaria pulmonaria. Mol. Ecol. 21, 3250–3265. doi: 10.1111/j.1365-294X.2012.05605.x
Hodkinson, B. P., and Lendemer, J. C. (2011). Molecular analyses reveal semi-cryptic species in Xanthoparmelia tasmanica. Bibl. Lichenol. 106, 108–119.
Hudson, R. R., and Coyne, J. A. (2002). Mathematical consequences of the genealogical species concept. Evolution 56, 1557–1565. doi: 10.1111/j.0014-3820.2002.tb01467.x
Kaasalainen, U., Heinrichs, J., Krings, M., Myllys, L., Grabenhorst, H., Rikkinen, J., et al. (2015). Alectorioid morphologies in Paleogene lichens: new evidence and re-evaluation of the fossil Alectoria succini Mägdefrau. PLoS One 10:e0129526. doi: 10.1371/journal.pone.0129526
Katoh, K., Kuma, K.-I., Toh, H., and Miyata, T. (2005). MAFFT version 5: improvement in accuracy of multiple sequence alignment. Nucleic Acids Res. 33, 511–518. doi: 10.1093/nar/gki198
Katoh, K., and Toh, H. (2008). Recent developments in the MAFFT multiple sequence alignment program. Brief. Bioinform. 9, 286–298. doi: 10.1093/bib/bbn013
Kraichak, E., Divakar, P. K., Crespo, A., Leavitt, S. D., Nelsen, M. P., Lücking, R., et al. (2015). A tale of two hyper-diversities: diversification dynamics of the two largest families of lichenized fungi. Sci. Rep. 5:10028. doi: 10.1038/srep10028
Leavitt, S. D., Divakar, P. K., Crespo, A., and Lumbsch, H. T. (2016a). A matter of time — understanding the limits of the power of molecular data for delimiting species boundaries. Herzogia 29, 479–492. doi: 10.13158/heia.29.2.2016.479
Leavitt, S. D., Fernández-Mendoza, F., Pérez-Ortega, S., Sohrabi, M., Divakar, P. K., Vondrák, J., et al. (2013a). Local representation of global diversity in a cosmopolitan lichen-forming fungal species complex (Rhizoplaca, Ascomycota). J. Biogeogr. 40, 1792–1806. doi: 10.1111/jbi.12118
Leavitt, S. D., Kraichak, E., Vondrak, J., Nelsen, M. P., Sohrabi, M., Perez-Ortega, S., et al. (2016b). Cryptic diversity and symbiont interactions in rock-posy lichens. Mol. Phylogenet. Evol. 99, 261–274. doi: 10.1016/j.ympev.2016.03.030
Leavitt, S. D., and Lumbsch, H. T. (2016). “2 ecological biogeography of lichen-forming fungi,” in Environmental and Microbial Relationships, eds C. P. Kubicek and I. S. Druzhinina (Berlin: Springer), 15–37.
Leavitt, S. D., Lumbsch, H. T., Stenroos, S., and Clair, L. L. S. (2013b). Pleistocene speciation in North American lichenized fungi and the impact of alternative species circumscriptions and rates of molecular evolution on divergence estimates. PLoS One 8:e85240. doi: 10.1371/journal.pone.0085240
Lindblom, L., and Ekman, S. (2006). Genetic variation and population differentiation in the lichen-forming ascomycete Xanthoria parietina on the island Storfosna, central Norway. Mol. Ecol. 15, 1545–1559. doi: 10.1111/j.1365-294X.2006.02880.x
Lücking, R., Dal Forno, M., Moncada, B., Coca, L. F., Vargas-Mendoza, L. Y., Aptroot, A., et al. (2016a). Turbo-taxonomy to assemble a megadiverse lichen genus: seventy new species of Cora (Basidiomycota: Agaricales: Hygrophoraceae), honouring David Leslie Hawksworth’s seventieth birthday. Fungal Divers. 84, 139–207. doi: 10.1007/s13225-016-0374-9
Lücking, R., Hodkinson, B. P., and Leavitt, S. D. (2016b). The 2016 classification of lichenized fungi in the Ascomycota and Basidiomycota – Approaching one thousand genera. Bryologist 119, 361–416. doi: 10.1639/0007-2745-119.4.361
MacArthur, R. H., and Wilson, E. O. (1967). The Theory of Island Biogeography, Monographs in Population Biology, Vol. 1. Princeton, NJ: Princeton University Press.
Menlove, J. E. (1974). Thin-layer chromatography for the identification of lichen products. Bull. Br. Lich. Soc. 34, 3–5.
Miao, Y., Herrmann, M., Wu, F., Yan, X., and Yang, S. (2012). What controlled Mid–Late Miocene long-term aridification in Central Asia? — Global cooling or Tibetan Plateau uplift: a review. Earth Sci. Rev. 112, 155–172. doi: 10.1016/j.earscirev.2012.02.003
Otálora, M. A. G., Martínez, I., Aragón, G., and Molina, M. C. (2010). Phylogeography and divergence date estimates of a lichen species complex with a disjunct distribution pattern. Am. J. Bot. 97, 216–223. doi: 10.3732/ajb.0900064
Peay, K. G., Bidartondo, M. I., and Elizabeth Arnold, A. (2010). Not every fungus is everywhere: scaling to the biogeography of fungal–plant interactions across roots, shoots and ecosystems. New Phytol. 185, 878–882. doi: 10.1111/j.1469-8137.2009.03158.x
Pringle, A., Baker, D. M., Platt, J. L., Wares, J. P., Latgé, J. P., and Taylor, J. W. (2005). Cryptic speciation in the cosmopolitan and clonal human pathogenic fungus Aspergillus fumigatus. Evolution 59, 1886–1899. doi: 10.1111/j.0014-3820.2005.tb01059.x
R Core Team (2015). R: A Language and Environment for Statistical Computing [Internet]. Vienna: R Foundation for Statistical Computing. Available at: https://www.r-project.org
Rambaut, A., and Drummond, A. (2003). Tracer [Computer Program]. Available at: http://tree.bio.ed.ac.uk
Rambaut, A., and Drummond, A. (2013). LogCombiner v1. 8.0. Available at: http://beast.bio.ed.ac.uk/
Rambaut, A., and Drummond, A. J. (2009). TreeAnnotator. Version 1.6.1. Available at: http://beast.community/treeannotator
Rannala, B. (2015). The art and science of species delimitation. Curr. Zool. 61, 846–853. doi: 10.1093/czoolo/61.5.846
Rannala, B., and Yang, Z. (2013). Improved reversible jump algorithms for Bayesian species delimitation. Genetics 194, 245–253. doi: 10.1534/genetics.112.149039
Revell, L. J. (2012). phytools: an R package for phylogenetic comparative biology (and other things). Methods Ecol. Evol. 3, 217–223. doi: 10.1111/j.2041-210X.2011.00169.x
Ruprecht, U., Brunauer, G., and Türk, R. (2014). High photobiont diversity in the common European soil crust lichen Psora decipiens. Biodivers. Conserv. 23, 1771–1785. doi: 10.1007/s10531-014-0662-1
Schmitt, I., Crespo, A., Divakar, P. K., Fankhauser, J. D., Herman-Sackett, E., Kalb, K., et al. (2009). New primers for promising single-copy genes in fungal phylogenies and systematics. Persoonia 23, 35–40. doi: 10.3767/003158509X470602
Schneider, K., Resl, P., and Spribille, T. (2016). Escape from the cryptic species trap: lichen evolution on both sides of a cyanobacterial acquisition event. Mol. Ecol. 25, 3453–3468. doi: 10.1111/mec.13636
Stamatakis, A. (2006). RAxML-VI-HPC: maximum likelihood-based phylogenetic analyses with thousands of taxa and mixed models. Bioinformatics 22, 2688–2690. doi: 10.1093/bioinformatics/btl446
Stamatakis, A., Hoover, P., and Rougemont, J. (2008). A rapid bootstrap algorithm for the RAxML web servers. Syst. Biol. 57, 758–771. doi: 10.1080/10635150802429642
Timdal, E. (1984). The delimitation of Psora (Lecideaceae) and related genera, with notes on some species. Nord. J. Bot. 4, 525–540. doi: 10.1111/j.1756-1051.1984.tb02059.x
Türk, R., and Gärtner, G. (2003). “Biological soil crusts of the subalpine, alpine, and Nival areas in the alps,” in Biological Soil Crusts: Structure, Function, and Management, eds J. Belnap and O. L. Lange (Berlin: Springer), 67–73.
Weber, W. A. (2003). The middle Asian element in the southern Rocky Mountain flora of the western United States: a critical biogeographical review. J. Biogeogr. 30, 649–685. doi: 10.1046/j.1365-2699.2003.00864.x
Werth, S. (2011). “Biogeography and phylogeography of lichen fungi and their photobionts,” in Biogeography of Microscopic Organisms: Is Everything Small Everywhere?, ed. D. Fontaneto (Cambridge: Cambridge University Press), 191–208. doi: 10.1017/CBO9780511974878.011
Werth, S., and Sork, V. L. (2014). Ecological specialization in Trebouxia (Trebouxiophyceae) photobionts of Ramalina menziesii (Ramalinaceae) across six range-covering ecoregions of western North America. Am. J. Bot. 101, 1127–1140. doi: 10.3732/ajb.1400025
Williams, L., Colesie, C., Ullmann, A., Westberg, M., Wedin, M., and Büdel, B. (2017). Lichen acclimation to changing environments: photobiont switching vs. climate-specific uniqueness in Psora decipiens. Ecol. Evol. 7, 2560–2574. doi: 10.1002/ece3.2809
Wirtz, N., Printzen, C., and Lumbsch, H. T. (2008). The delimitation of Antarctic and bipolar species of neuropogonoid Usnea (Ascomycota, Lecanorales): a cohesion approach of species recognition for the Usnea perpusilla complex. Mycol. Res. 112, 472–484. doi: 10.1016/j.mycres.2007.05.006
Yang, Z. (2015). The BPP program for species tree estimation and species delimitation. Curr. Zool. 61, 854–865. doi: 10.1093/czoolo/61.5.854
Yang, Z., and Rannala, B. (2010). Bayesian species delimitation using multilocus sequence data. Proc. Natl. Acad. Sci. U.S.A. 107, 9264–9269. doi: 10.1073/pnas.0913022107
Yang, Z., and Rannala, B. (2014). Unguided species delimitation using DNA sequence data from multiple loci. Mol. Biol. Evol. 31, 3125–3135. doi: 10.1093/molbev/msu279
Keywords: biogeography, biological soil crusts (BSC), cryptic species, disjunct populations, long-distance dispersal, Psora, semi-arid, South Africa
Citation: Leavitt SD, Westberg M, Nelsen MP, Elix JA, Timdal E, Sohrabi M, St. Clair LL, Williams L, Wedin M and Lumbsch HT (2018) Multiple, Distinct Intercontinental Lineages but Isolation of Australian Populations in a Cosmopolitan Lichen-Forming Fungal Taxon, Psora decipiens (Psoraceae, Ascomycota). Front. Microbiol. 9:283. doi: 10.3389/fmicb.2018.00283
Received: 29 April 2017; Accepted: 07 February 2018;
Published: 23 February 2018.
Edited by:
Carlos Pelleschi Taborda, University of São Paulo, BrazilReviewed by:
Birinchi Kumar Sarma, Banaras Hindu University, IndiaRam Prasad, Amity University, India
Stefano Ghignone, Istituto per la Protezione Sostenibile delle Piante (CNR), Italy
Luiz H. Rosa, Universidade Federal de Minas Gerais, Brazil
Copyright © 2018 Leavitt, Westberg, Nelsen, Elix, Timdal, Sohrabi, St. Clair, Williams, Wedin and Lumbsch. This is an open-access article distributed under the terms of the Creative Commons Attribution License (CC BY). The use, distribution or reproduction in other forums is permitted, provided the original author(s) and the copyright owner are credited and that the original publication in this journal is cited, in accordance with accepted academic practice. No use, distribution or reproduction is permitted which does not comply with these terms.
*Correspondence: Steven D. Leavitt, c3RldmVfbGVhdml0dEBieXUuZWR1