- Bacterial Genomics and Evolution Laboratory, CSIR-Institute of Microbial Technology, Chandigarh, India
Staphylococcus epidermidis is a prominent commensal member of human skin microbiome and an emerging nosocomial pathogen, making it a good model organism to provide genomic insights, correlating its transition between commensalism and pathogenicity. While there are numerous studies to understand differences in commensal and pathogenic isolates, systematic efforts to understand variation and evolutionary pattern in multiple strains isolated from healthy individuals are lacking. In the present study, using whole genome sequencing and analysis, we report presence of diverse lineages of S. epidermidis isolates in healthy individuals from two geographically diverse locations of India and North America. Further, there is distinct pattern in the distribution of candidate gene(s) for pathogenicity and commensalism. The pattern is not only reflected in lineages but is also based on geographic origin of the isolates. This is evident by the fact that North American isolates under this study are more genomically dynamic and harbor pathogenicity markers in higher frequency. On the other hand, isolates of Indian origin are less genomically dynamic, harbor less pathogenicity marker genes and possess two unique antimicrobial peptide gene clusters. This study provides a basis to understand the nature of selection pressure in a key human skin commensal bacterium with implications in its management as an opportunistic pathogen.
Introduction
Staphylococcus epidermidis is coagulase-negative staphylococcus (CONS) commensal member of human skin microbiota inhabiting skin since millions of years (Rogers et al., 2009). As a key member of skin microbiome, its role in skin homeostasis is widely assumed (Kong et al., 2012; Skabytska and Biedermann, 2016). There are many studies showing its role in immune system modulation (Lai et al., 2009, 2010; Belkaid and Tamoutounour, 2016; Egert et al., 2017). It is also considered crucial for skin microbiota because of its ability to inhibit the growth of notorious skin pathogen, Staphylococcus aureus (Otto et al., 1999; Heikkilä and Saris, 2003; Iwase et al., 2010). It produces antimicrobial peptides, which inhibit the growth of pathogenic strains (Nakatsuji et al., 2017). However, in recent decades, S. epidermidis has emerged as an opportunistic pathogen, as a frequent cause of nosocomial infections (Hidron et al., 2008; Otto, 2009) in immunocompromised patients or patients with indwelling medical devices (Marr et al., 1997; Varani et al., 2009; Percival et al., 2015). Abundance of S. epidermidis on skin enhances the probability of contamination of indwelling devices through formation of biofilms. Presence of biofilms on these medical devices interrupts the proper functioning of the devices and cause bacteremia (Costerton et al., 1999; Mishra et al., 2015; Otto, 2017). Staphylococcus epidermidis is the major causative agent for blood stream infections, vascular graft, central nervous system shunt, prosthetic joint infections, prosthetic valve endocarditis, and other biomedical device-related infections (Widerström et al., 2012a). It has also been reported to play a role in neonatal sepsis (Sgro et al., 2011). Management of S. epidermidis is further challenged by the presence of antibiotic resistance genes (Archer, 1978; Raad et al., 1998; Gill et al., 2005; Dave et al., 2011; Widerström et al., 2012b), thereby contributing to multi-drug resistance forms of the species (Rabaud and Mauuary, 2001; Miragaia et al., 2005). These strains may also transfer them horizontally to its pathogenic relative, S. aureus (Méric et al., 2015). Thus, management of this commensal yet an opportunistic pathogen is crucial in both community and clinical settings.
One of the major challenges in treating pathogenic S. epidermidis is the lack of proper demarcation or markers to differentiate among commensal and pathogenic isolates (Galdbart et al., 2000; Grice and Segre, 2011). Some studies based on MLST analysis and other genetic studies highlights genes among S. epidermidis isolates of hospital environment and from those obtained outside of it (Galdbart et al., 2000). Some genes, including, IS256 (insertion sequence) (Kozitskaya et al., 2004), mecA (methicillin resistance gene) (Lazaris et al., 2017; Qin et al., 2017; Takizawa et al., 2017) and icaA (biofilm biosynthesis cluster ica gene) (Grice and Segre, 2011) are found to be more frequently present in pathogenic strains and are also shown to contribute to their pathogenicity. These genes are also present in pathogenic strain, RP62A and hence, are proposed by some studies as potential markers to delineate pathogenic strains of S. epidermidis (Galdbart et al., 2000; Gu et al., 2005). Some other genes viz. fdh and arsD, encoding for “formate dehydrogenase” “arsenic resistance gene” respectively, are observed to be frequent in commensal strains (Conlan et al., 2012). Higher frequency of these genes in their S. epidermidis isolates based on their origin hints in their ability to resolve the demarcation of commensal and pathogenic S. epidermidis. However, their full scope as markers is yet to be exploited.
There are numerous studies highlighting the clinical importance of this bacterium. However, no studies have been carried out so far emphasizing its phylogenomic aspect that can help in understanding the diversity among multiple isolates of a healthy individual. While earlier studies focused on the differential features among commensal and pathogenic isolates (Harris et al., 2016; Post et al., 2017), this study mainly pinpoints diversity and evolution among commensal isolates of diverse geographic origin.
Since S. epidermidis inhabiting the skin through ages, there might be ongoing selection on isolates within and between healthy individuals. In a recent publication, we reported that S. epidermidis isolates from diverse habitats like plants, rodents and human shown remarkable variation suggesting the ongoing selection in this species (Chaudhry and Patil, 2016). Moreover, there are studies on inter-strain variation, based on pangenome analysis of S. epidermidis isolates from different body sites (Conlan et al., 2012). However, there are no studies on inter-strain variation of S. epidermidis isolates living on healthy individuals from diverse geographic origins, which might be due to ongoing selection on isolates within and between healthy individuals. Advent of next generation sequencing and large-scale genome based studies are allowing us to capture inter-strain variation at unprecedented details.
In the present study, we report whole genome sequencing of twenty-eight S. epidermidis isolates from different body parts of skin of healthy individuals from India, analyzed with twenty-one S. epidermidis isolates from different body parts of skin of healthy individuals from North America (Conlan et al., 2012).
Materials and Methods
Ethics, Consent, and Permissions
The study was approved by the Institutional Ethics Committee (Human), CSIR-Institute of Microbial Technology, Chandigarh (India) [Project no. 12IEC/1/9-2014] and informed consent was obtained from the individuals.
Bacterial Strains
Twenty-eight S. epidermidis isolates (SEI), from different body sites of healthy individuals, viz., retro-auricular crease, nares, antecubital fossa and opisthenar area of hand, from India were isolated from four healthy individuals. Isolations were done as described by Farran et al. (2013). Pre-moistened saline (0.85% NaCl) swabs were rubbed with skin of the healthy individuals and transferred to TSB (Tryptic Soy Broth) for enrichment. Inoculated media was than incubated at 37°C in a shaker ~200 rpm and growth was observed after 16-18 hrs. Overnight grown culture was then streaked onto MSA (mannitol salt agar) plates and was incubated at 37°C for ~36–48 h. Pink and white color colonies were observed on agar plates indicating S. epidermidis and S. aureus populations respectively. Pure cultures of S. epidermidis were obtained and identified by 16S rRNA sequencing using amplification primers 27F (AGAGTTTGATCMTGGCTCAG) and 1492R (GGTTACCTTGTTACGACTT), and further 16S rRNA gene sequence was used for characterization using EZ-taxon server (http://www.ezbiocloud.net/eztaxon; Kim et al., 2012). For preservation, 15% glycerol stocks of the pure culture of each isolate was prepared and maintained at −80°C. Genome sequences of type strain, MTCC3382 (accession number: NZ_LILE01000007) and reference strains, RP62A (accession number: NC_002976), ATCC12228 (accession number: NC_004461) were taken from NCBI for detailed analysis.
Twenty-one isolates of western origin (SEA) used in this study were taken from a similar study where multiple S. epidermidis isolates were extracted from different body sites of several healthy individuals (Table 1; Conlan et al., 2012).
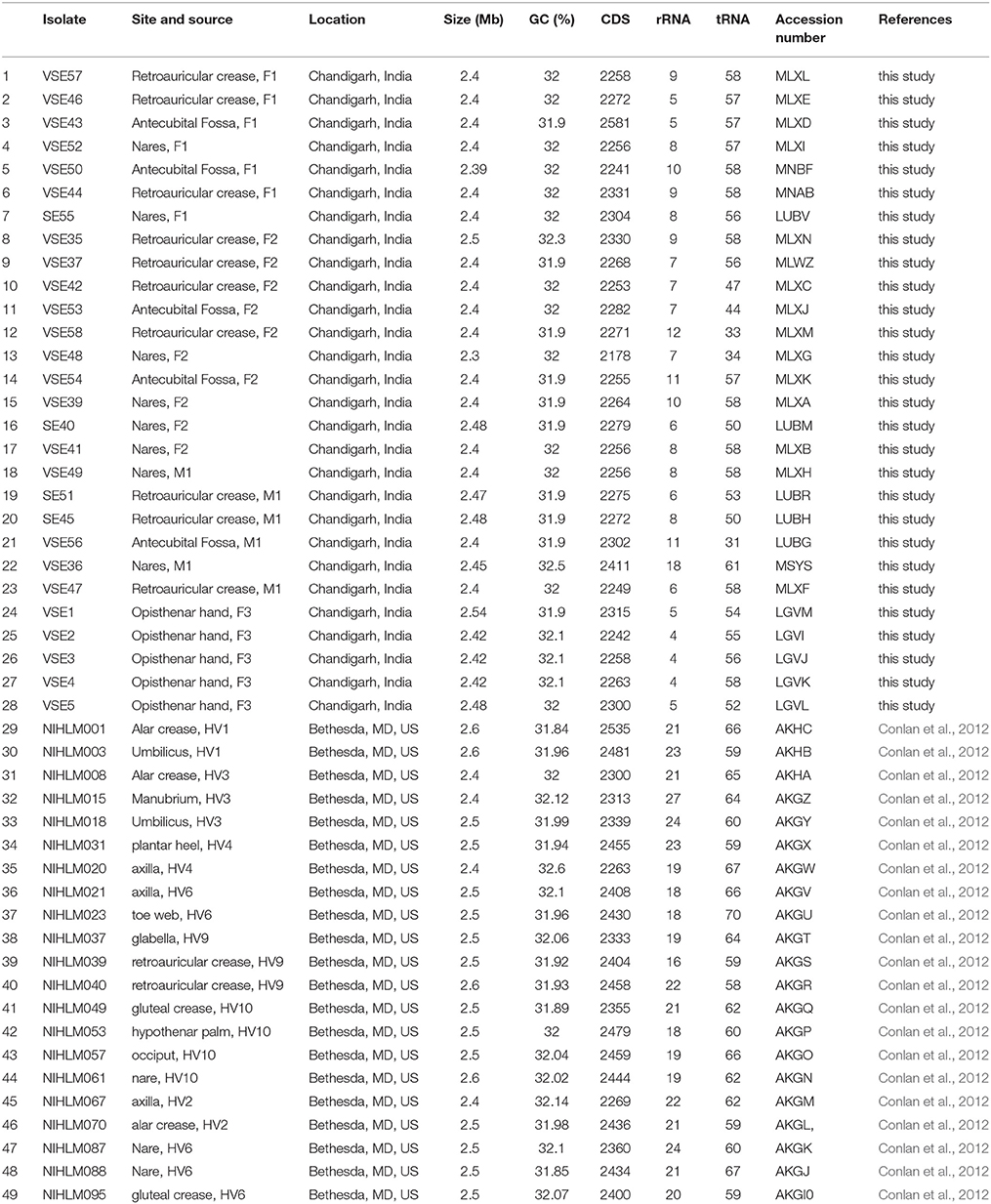
Table 1. Details of the S. epidermidis isolates extracted from different body sites of healthy individuals, of two different geographical locations.
Genome Sequencing, Assembly and Annotation
Cells were revived from −80°C stocks and DNA was isolated using ZR Fungal/Bacterial DNA isolation kit (Zymo Research Corporation, Orange, CA, USA) as per the instruction manual. The purity, quality and quantity of purified genomic DNA were assessed using Nanodrop (Thermo Scientific, MA, USA), agarose gel electrophoresis and Qubit 2.0 Fluorometer (Invitrogen, Carlsbad, CA, USA) respectively. Illumina DNA sequencing libraries was prepared by using Nextera XT sample preparation kit (Illumina, Inc., San Diego, CA, USA) with dual indexing adapters, as per the instruction manual and prepared library was sequenced on in-house Illumina MiSeq platform (Illumina, Inc., San Diego, CA, USA) using paired end sequencing kits. Adapter trimming was carried out automatically by MiSeq Control Software (MCS) and additional adapter contamination identified by NCBI server was removed by manual trimming. De novo assembly of the sequences was carried out using CLC genomic workbench v7.5 (CLC Bio-Qiagen, Aarhus, Denmark). 16S rRNA sequences were extracted from the assembled genomes using RNAmmer 1.2 server (Lagesen et al., 2007) and were characterized by Ez-taxon server. tRNA was calculated by tRNAScan-SE (Lowe and Eddy, 1997). Sequences were annotated using NCBI Prokaryotic Genome Annotation Pipeline (http://www.ncbi.nlm.nih.gov/genome/annotation_prok/) and Rapid Annotation System Technology (RAST) pipeline (Aziz et al., 2008). Genome sequences of all 28 isolates sequenced under this study are submitted in NCBI GenBank and are summarized into Table 1.
Genome Based Taxonomy and Phylogenomics
Phylogenetic analysis of the isolates was carried out by constructing a tree based on 400 universal protein by PhyloPhlAn version 0.99 (Segata et al., 2013) and was represented by interactive tree of life (iTOL) version 3 (Letunic and Bork, 2006). For genome similarity assessment, BLAST-based average nucleotide identity (ANIb) values were calculated by using JSpecies (Richter and Rosselló-Móra, 2009).
Multilocus Sequence Typing (MLST)
Sequence type (ST) and consecutive allelic profile of all genomes was elucidated from an online platform by Center for genomic epidemiology (Larsen et al., 2012; http://cge.cbs.dtu.dk/services/MLST). This tool utilizes the prescribed MLST scheme of seven genes. Two genes, gtr and tpi, were not detected in genomes, VSE3, VSE43 and VSE48, VSE50, respectively. Reads for these genomes were re-assembled in Spades version 3.11 (Bankevich et al., 2012), required genes were extracted and ST was analyzed. Generated STs were confirmed in MLST database (Aanensen and Spratt, 2005; http://www.mlst.net). Novel allele profile was submitted to PubMLST database (Jolley et al., 2004; http://pubmlst.org/sepidermidis/) to obtain the unique and novel ST information.
Comparative Genome Analysis
Comparative subsystem analysis of all isolates was carried out by comparative examination of their RAST annotations. Atypical genomic regions were extracted by using whole genome based Blast based Ring Image Generator (BRIG) tool version 0.95 (Alikhan et al., 2011). All genomes were tried as reference in BRIG analysis, to extract out most significant atypical regions. Observed atypical genomic regions were taken out by Artemis comparisons (Carver et al., 2012). Pangenome analysis was carried for all 49 genomes, out by BPGA v1.3 (Chaudhari et al., 2016). A minimum identity of 50% was used as the cut-off. Number of combinations of genomes considered for analysis were chosen to be 50, which signifies the maximum number of possible clusters for a USEARCH run. USERACH is an integral dependency of BPGA used for clustering. Flower plot diagram used to represent the core and unique gene was constructed using a python script of Matplotlib (Hunter, 2007).
Genome Mining
In silico search of candidate virulence genes was carried out in RAST annotated genomes (Aziz et al., 2008) and was further confirmed by BLASTN (Johnson et al., 2008) to analyze genes for commensalism and pathogenicity.
Genomes were mined in antiSMASH tool version 2.0 (Medema et al., 2011) and BAGEL version 3 (van Heel et al., 2013) web tool for examining gene clusters for secondary metabolites, bacteriocin, or lantibiotics. Individual genes of clusters were mapped manually using BLASTN. Prophages were checked through web server PHAST (Phage search tool) (Zhou et al., 2011).
Results
Genome Sequencing and Annotation
Twenty-eight S. epidermidis (SE) isolates from different body sites of healthy individuals of Indian origin (SEI) were sequenced (Table S1) and high quality data was obtained with coverage >50x and number of contigs in range, 52–176. Annotation of all isolates revealed CDS ranging from 2,178 to 2,535. Sequencing and annotation details of SEI isolates and 21 S. epidermidis isolates of American origin (SEA) from another study (Conlan et al., 2012) are provided in Table 1.
Phylogenomic and Phylogeographic Analysis of SEI and SEA Isolates
Phylogenetic relationship among 49 SE isolates (Table S2) was elucidated by constructing a phylogenetic tree based on 400 conserved protein sequences (Figure 1). The tree branched into two major clades, “A” and “B.” Majority of SEI isolates were present in “A” clade while SEA isolates were divided uniformly among the two clades.
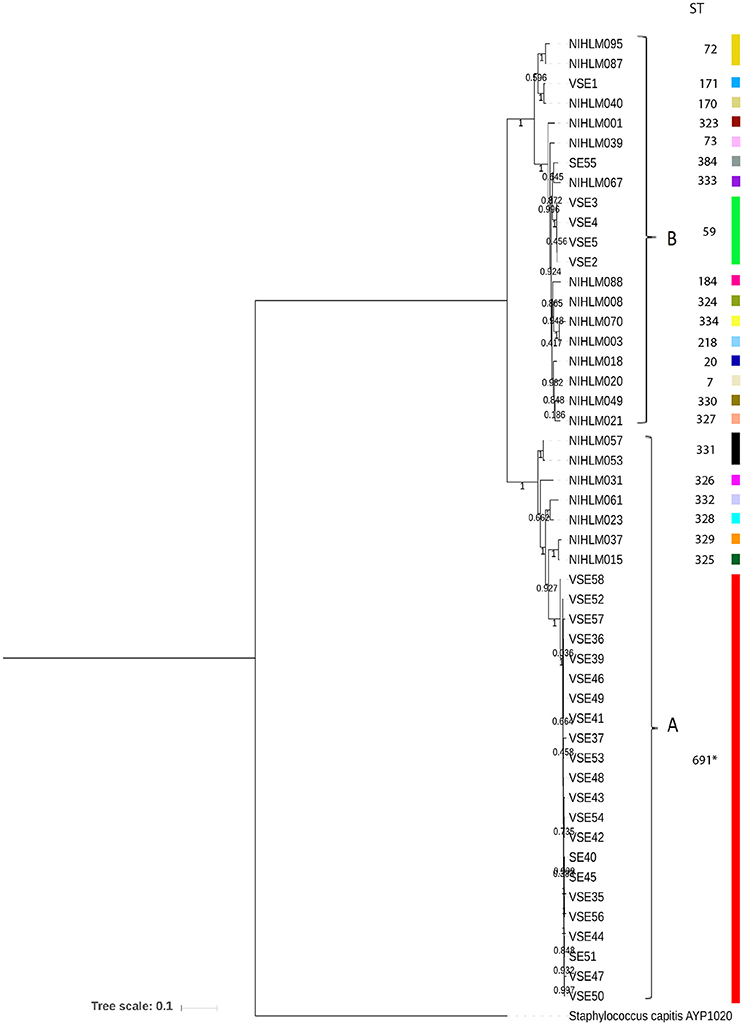
Figure 1. Phylogenetic tree among 49 Staphylococcus epidermidis isolates, based on protein sequence of 400 universal housekeeping genes, using MEGA7.10.18. S. capitis AYP1020 was used as outgroup of the tree and bootstrap values are highlighted on the branches. STs of all isolates are mentioned against them and isolates with similar STs or clones are represented with same color-coding.
Further, genetic relatedness among all 49 isolates was deduced by whole genome based, ANI heatmap tree using type strain, MTCC 3382 (T). Biofilm forming strain, RP62A and non-biofilm forming strain, ATCC12228 were also used as reference strains. All 49 isolates demarcated themselves in two major phylogenetic lineages, PG1 and PG2, and a minor group of four isolates, independent of the habitat, individual or body site from which they were isolated (Figure 2). PG1 lineage of ANI tree corroborated with clade “A” of phylogenetic tree, consisting of the same isolates. PG1 lineage mainly consists of SEI isolates, having ANI > 99% among themselves, forming a single clonal group, while some SEA isolates of the same lineages were comparatively more diverse. PG2 lineage consists of five SEI isolates along with type strain, MTCC 3382 (T), reference strains, ATCC12228, RP62A and other SEA isolates. The minor group represented by four isolates, appeared as a transitional lineage, having intermediate ANI values of PG1 and PG2 lineages.
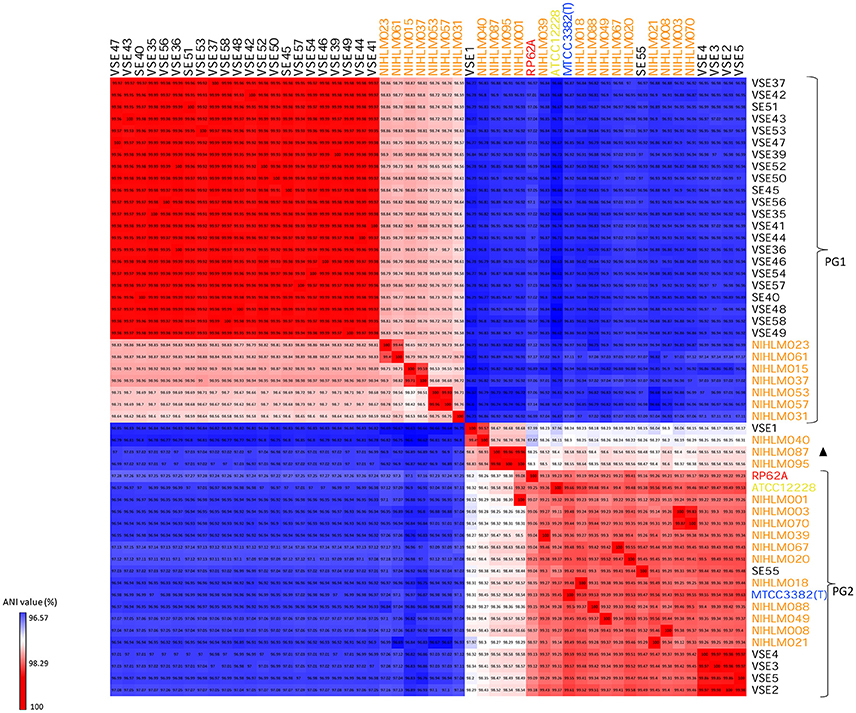
Figure 2. ANI heatmap of SEI and SEA isolates. PG1 and PG2 demonstrates two kind of lineages, phylogenetic group 1 and phylogenetic group 2; Blank triangle represents transitional phase. Orange color text isolates are SEA isolates. Type strain, MTCC 3382 (T) [In blue], pathogenic strain RP62A [In red] and commensal strain, ATCC12228 [In yellow].
Multilocus Sequence Type (ST) Analysis of SEI and SEA Genomes
Nineteen Sequence Types (ST) of 21 SEA genomes is already mentioned in the earlier study (Conlan et al., 2012) and ST of SEI isolates were determined in this study (Figure 1). Four STs were observed in SEI isolates. Isolates VSE2-VSE5 belong to ST59, isolate SE55 belong to ST384, and isolate VSE1 belong to ST171. Interestingly all the SEI isolates of PG1 lineage or clade “A,” i.e., VSE35-VSE58 belong to a novel ST691 (Table S3). All the STs are lineage or clade specific and large majority (16 out of 23 STs) belong to clade “B” or PG2 lineage. MLST typing revealed that PG1 lineage isolates are less diverse than PG2 lineage corroborating finding from core genome tree and phylogenomic analysis.
Phylogenomic and Phylogeographic Variation in Pathogenicity and Commensal Candidate Genes(s) in S. epidermidis Isolates
Commensal strains marker gene, “fdh” was seen to be present in all isolates (SEI and SEA) of lineage PG1. While another gene, arsD, was absent from all SEI isolates of PG1 and was present in SEA isolates of the same lineage. Distribution of this gene in PG2 and transitional lineage is uneven and inconsistent with both SEA as well as SEI isolates (Figure 3A).
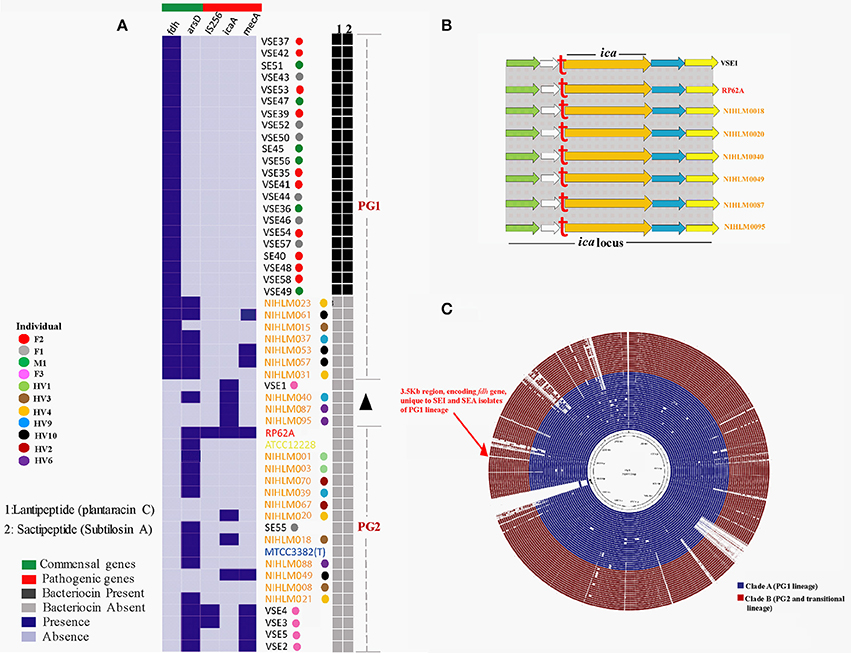
Figure 3. (A) In-silico mining of potential commensal and pathogenic markers in isolates and type strain. Blue color indicates presence of the corresponding gene, which is estimated as 100% coverage of the gene with identity value >80% and Light blue color indicates absence of the gene, which is estimated by no identity, e-value >0 or coverage <5. Different color-coding is representation for different individuals. Details of the individuals are provided in metadata in Table S2. Bacteriocins, lantipeptide and sactipeptide are denoted by 1 and 2 respectively. Their presence or absence is marked by Dark gray and Light gray respectively. (B) Complete ica cluster along with upstream downstream regions in icaA positive isolates. A red “t” indicates presence of tRNA in all isolates. The whole cluster along with downstream, upstream genes is exactly similar in all isolates. Green: MFS transporter, Blank: Hypothetical gene, Blue: Lipase, Yellow: N-acetyltransferase. (C) Blast based comparative analysis of 49 S. epidermidis isolates by BRIG, taking VSE35 as reference, blue color represents PGI isolates and red color represents PG2 and transitional lineages isolates, irrespective of geographic origin.
IS256, an insertion sequence, is a marker for pathogenicity (Kozitskaya et al., 2004) in S. epidermidis was absent from all isolates of PG1 and transitional lineage and was present only in two SEI isolates of lineage PG2. While mecA gene (methicillin resistance) was absent from SEI isolates of lineage PG1 and present in 4 SEI isolates of PG2. On the other hand, three SEA isolates of lineage PG1 and one from PG2 were observed having mecA resistance gene.
Biofilm forming, ica gene cluster consists of 5 genes, icaR, icaA, icaD, icaB, and icaC. Most important gene of this cluster is icaA, which is considered crucial for biofilm formation and was searched in all genomes. The gene was present in all isolates of transitional lineage and only in 2 isolates of PG2 lineage. Whole cluster of the ica was in silico mined in all seven genomes along with their loci, which were found to be in complete synteny to each other (Figure 3B).
Comparative Genomics of SEA and SEI Isolates
Pangenome Evolution and Diversification
To study the genetic repertoire of the commensal S. epidermidis isolates', pan-genome analysis of 49 SEA and SEI genomes was performed using BPGA. The results of core pan curve showed that pan genome size increase with sequential addition of the new genome and reached a plateau state. The power-law regression model depicted “b” parameter value as 0.155528, suggesting an open pan genome, which may be closed soon. The analysis revealed that 49 compared Staphylococcus epidermidis strains possess 2178-2535 CDSs. Of these, 1,302 are core to all genomes in comparison (Figure 4A). However, nearly 700–1,000 CDSs proteins make up the accessory fraction, which include unique gene content to each genome among the 49 compared strains. Figure 4B depicts polar plot of pan-genome study with predicted number of core and unique genes across the 49 strains. It shows that, 32/49 strains are having a strain specific unique gene. The predicted genes (core, accessory and unique) in all 49 strains were further classified based on COG and KEGG categories. Functional classification according to COG category showed that the core gene pool was found to be abundant in all cell metabolism classes, ‘energy production, conversion, and translation, ribosomal structure and biogenesis. However, the unique gene pool of all the strains showed significant abundance of subclasses that belonged to transcription and replication, recombination and repair followed by cell motility and defense mechanisms compared to core (Figure 4C). As per KEGG category analysis (Figure 4D), the genome of all the strains depicted higher fraction of core genes under “metabolism” class whereas the unique gene pool were found high in number for drug resistance, immune system, infectious diseases, terpenoids and polyketides apart from amino acid, carbohydrate, xenobiotics metabolism and replication and repair genes.
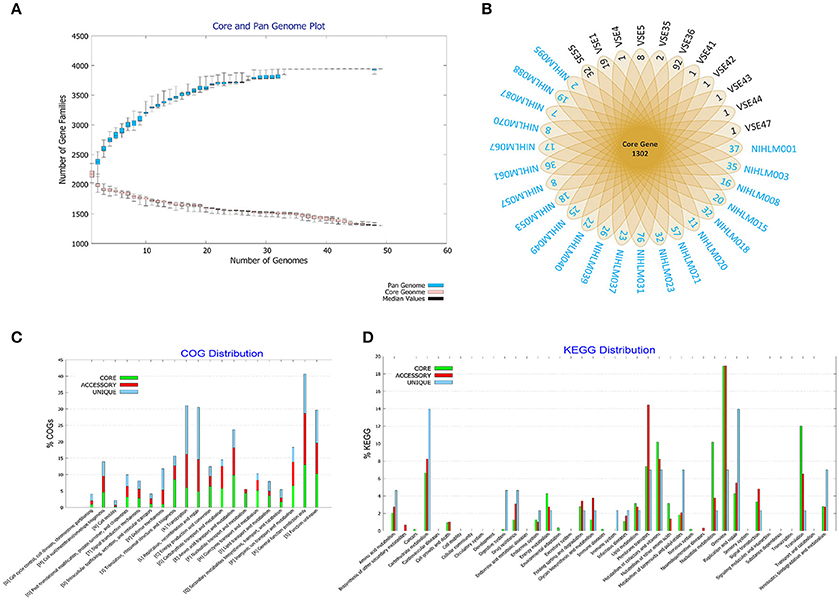
Figure 4. Pangenome profile analysis of 49 S. epidermidis isolates. Indian origin isolates are highlighted in black, while American origin isolates are marked in blue (A) Pan-genome curve generated by plotting total number of gene families in the pan and core genome for all isolates. (B) Polar diagram showing isolates having unique number of genes. 32 isolates out of 49 possess unique genes, represented in outer edge of the diagram. (C) Collective distribution of core, accessory and unique genes of all 49 isolates in COG and (D) KEGG analysis.
Comparative Analysis of Functional Gene Classes
Functional comparisons of subsystems are similar in all the isolates, except variations in “phage, prophage, transposable elements, and plasmids” and “production of secondary metabolites” (Figure 5). Most of the SEI isolates of PG1 lineage showed fewer number of phages, prophages, transposable elements and plasmids as compared to SEA isolates of the same lineage while SEI isolates of PG2 lineage had higher number of phages, prophages, transposable elements and plasmids. However, phage presence could only affect the functionality of the genomes, if they are intact. Hence, an in silico analysis was carried out to figure out the exact intact phages in these genomes (Table S4). SEI isolates of lineage PG1, corroborates with the previous findings demonstrating absence of phages, except VSE35 and VSE36. Since, functional comparisons also revealed variations in secondary metabolite production, genomes were analyzed for the same. Presence of a lantibiotic, having similarity to “Epilancin 15x” and a sactipeptide, subtilosin A was detected in SEI isolates of PG1 lineage (Figure 3A, Table S5).
Mining Atypical Regions by Genome Wide Comparisons
Whole genome comparisons were made to extract out atypical regions among genomes, taking different genomes as references. Three genomic regions (Region A, Region B, and Region C) were found to be unique to SEI isolates of PG1 lineage, against SE51 as reference (Figure 6).
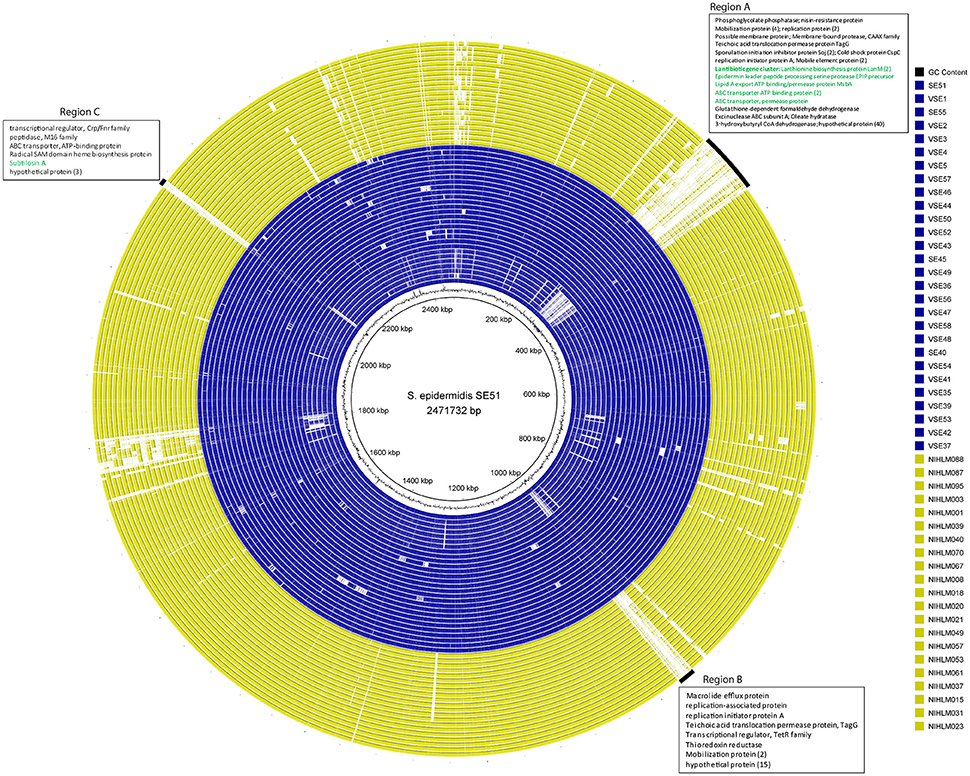
Figure 6. Blast based whole genome comparison of SEI and SEA isolates, taking Indian commensal, SE51 as reference. Blue color represents SEI isolates and yellow color represents SEA isolates. SEI isolates of PG1 lineage were seen to have three large dynamic regions with annotation indicated within boxes as region A, B, and C.
Interestingly, region A, which was largest of all regions is about 65 kb with more than 70 predicted ORFs and 28.29% GC content, encoding for genes responsible for membrane proteins, mobile element proteins, antimicrobial peptide biosynthetic gene cluster, and large number of hypothetical proteins. Further annotation of this region revealed the presence of a lantibiotic peptide (49 aa), having 28.5% GC content and surrounded by LanM (lantibiotic biosynthesis enzyme) encoding genes (Table S6). BlastP of the peptide revealed its 100% similarity to “plantaracin C family lantibiotic” of Bacillus subtilis. Secondary metabolite prediction tools confirmed the presence of lantibiotic peptide and predicted a putative lantibiotic biosynthetic gene cluster having 18% homology to “Epilancin 15X biosynthetic gene cluster” (Velásquez et al., 2011). Putative lantibiotic biosynthetic gene cluster having the lantibiotic peptide and other associated genes were manually mapped (Figure 7A).
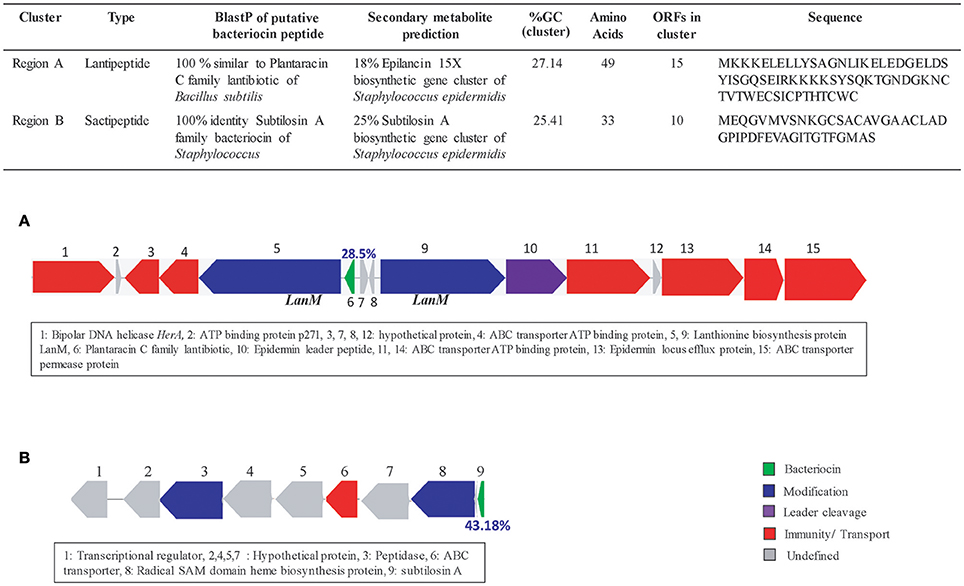
Figure 7. Bacteriocin details of Indian isolates from PG1 lineage. (A) Putative bacteriocin cluster from a “plantaracin C family” lantibiotic and (B) Putative bacteriocin, “subtilosin A” sactipeptide biosynthetic gene cluster.
A comparatively smaller (19 kbp) Region B having 24 ORFs has a GC content of 30%. Most of the genes of this region are hypothetical proteins (15). However, one “macrolide efflux protein” gene was seen specific to the region (Table S7).
The smallest region (7.5 kb) having GC content of 25.42%, encodes for nine ORFs only, one of them which is for “subtilosin A” (Table S8). Performing BLASTN of the gene revealed its 100% identity to “serine protease” of S. epidermidis isolates and BlastP shows its identity to subtilosin A to Staphylococcus. Secondary metabolite prediction tools confirmed the presence of the gene by predicting a sactipeptide biosynthetic cluster having 25% homology to “subtilosinA biosynthetic gene cluster.” Putative sactipeptide biosynthetic gene cluster having the sactipeptide and its biosynthetic genes were manually mapped (Figure 7B). Complete details of all the genes of lantibiotic and sactipeptide clusters are present in Tables S9, S10 respectively.
Mapped putative lantibiotic and sactipeptide clusters were observed to be present only in SEI isolates of PG1 lineage. However, NIHLM040, a SEA was observed to have another lantipeptide of around 29 Kbp with high sequence identity of 90% to a lantipeptide isolated from “Staphylococcus gallinarum,” known as “Gallidermin” (Kellner et al., 1988). Clade specific comparative analysis revealed no significant cluster or gene, specific to any of the group, A or B. However, a small, 3.5 Kb region is specific to group A or lineage PG1 (Figure 3C) irrespective of geographic origin of the isolates. Annotation of this region revealed “formate dehydrogenase alpha subunit” i.e., fdh, followed by 2 hypothetical proteins (Table S11).
Discussion
Microbial diversity is greatly affected by the environment and selection pressure. S. epidermidis isolates of Indian origin (SEI), used in this study may be diverse at genomic level but all are from a similar habitat. However, to depict the trend in their diversity S. epidermidis isolates from a completely diverse environment or niche are required. Therefore, taking advantage of an already existing dataset of 21 isolates of a study performed in North America i.e., at a different geographic location (Conlan et al., 2012), along with our own generated data. In this regard, we performed comparative genomic and phylogenomic analysis with multiple S. epidermidis North American isolates (SEA) collected from different body sites of healthy individuals.
Phylogenetic analysis depicts simultaneous diversification of the two lineages from a common ancestor. SEI and SEA isolates cluster themselves in two lineages, PG1 and PG2, independent of individual or site of isolation and geographic location. The ANI value difference of PG1 lineage isolates and PG2 lineage isolates varies considerably i.e., from 2 to 3%, suggesting that lineages are divergent from each other and their divergence is not recent. Presence of SEI isolates of PG1 lineage in a single group depicting their clonal nature, inspite of the fact that they were isolated from different individuals. However, SEI isolates of PG2 lineages are comparatively diverse. Based on ANI values, a minor third kind of population or transitional lineage highlighting SEI isolate VSE1, with three SEA isolates, depicts presence of three type of populations in commensal pool of S. epidermidis, at genomic level. Isolates collected from a single individual can cluster into both PG1 as well as PG2 lineages, suggesting that skin microbiota of a healthy individual generally can consists of 2-3 kinds of population irrespective of their geographical differences. However, there can also be individuals that harbor isolates from only one type of lineage or ST. Interestingly, individuals harboring isolates from only one lineage or ST are of Indian origin and not American origin. Clonal nature of SEI isolates of PG1 lineage is evident in their allelic profile, which was exactly same for all the isolates (Table S3). Presence of no exact matches for STs for these isolates in entire database of MLST, speculate presence of a single but novel ST in SEI isolates of PG1 lineage, which is might be unique to Indian population. In addition, presence of 19 STs in 21 SEA isolates signifies diversity of these isolates as compared to Indian ones (SEI).
Revisiting Commensal Status of S. epidermidis Isolates from Healthy Individuals
One of the biggest challenge of clinicians is to differentiate among pathogenic and commensal S. epidermidis due to lack of proper markers. Comparative analysis in some of the studies has highlighted that on the basis of their overall frequency in commensal and pathogenic isolates, some of the genes have the potential for being the discriminatory markers (Conlan et al., 2012). However, the scope of these genes is yet to be fully exploited and, hence, a clear and evident declaration of markers among the two kinds of S. epidermidis is lacking. In this study, we tried to evaluate the trend of these genes in two diverse kinds of population, i.e., Indian and western. The ecology, habitat, economical status and environment of these geographic populations is completely diverse. Trend of these genes in two different kinds of population will help in understanding the adaptation of these two different kinds of isolates and will throw light on the differentiating ability of these genes. Five potential marker genes: fdh (formate dehydrogenase), arsD (arsenic resistance cluster gene), IS256 (insertion sequence), icaA (biofilm biosynthesis cluster ica gene), and mecA (methicillin resistant) were investigated for this purpose. Among these, fdh and arsD were found to be more common in commensal isolates while IS256, icaA and mecA were more frequent in clinical isolates (Conlan et al., 2012).
Presence of fdh gene in all isolates of PG1 lineage and their complete absence from that of PG2, reflects that distribution of this gene is lineage dependent, as reported by Conlan and co-workers, but lineage specific distribution is independent of geographic origin of the isolates, in our case SEA or SEI. Hence, it can be restated that “fdh” gene is associated with commensal strains of S. epidermidis. While its absence from pathogenic RP62A coincides with the hypothesis, its absence from non-biofilm forming ATCC12228 is counterintuitive. However, the commensal status of ATCC12228 strain is not clear as it harbors some of the virulence genes like “Delta haemolysin and beta haemolysin” and reported to have slight virulence in mouse and rats (Zhang et al., 2003). Another gene, arsD is also connected to commensalism. However, its absence from SEI isolates of lineage PG1 and inconsistent distribution among isolates of other lineages, contradicts its status as a demarking marker of commensal and pathogenic isolates. Since all isolates understudy are from healthy individuals, absence of IS256 from majority of the isolates of all lineages is not surprising. Its presence in only two isolates could be a case of horizontal gene transfer, since both the isolates belong to the same individual. Antibiotic resistance is another important challenge in treating S. epidermidis infections. Methicillin resistant S. aureus (MRSA) is already known and studied extensively. Recent studies have reported many methicillin resistant S. epidermidis isolates (MRSE), further complicating the disease management. Pathogenic strain, RP62A is also known to harbor mecA gene that encodes for methicillin resistance. Presence of this gene in SEI isolates of PG2 lineage may be due to high antibiotic load in India (Laxminarayan and Chaudhury, 2016). Hence, presence of mecA gene in S. epidermidis in healthy individuals from India is a matter of concern. 3 SEA isolates of PG1 lineage, carrying the mecA gene, belongs to the same individual from which SEA isolate, NIHLM047 was isolated and similarly, all 4 SEI isolates from PG2 lineage carrying the mecA gene belongs to a single individual suggesting presence of this gene due to individual specific antibiotic resistance rather than overall load. One important factor required for pathogenicity is the ability to form biofilms. The ica cluster is found responsible for biofilm formation in pathogenic reference strain, RP62A (Li et al., 2005). The ica cluster (Heilmann et al., 1996), is followed by a tRNA in RP62A genome and has a low GC content (29.80%), suggesting that the cluster might have been acquired by the strain through horizontal gene transfer events. However, the whole cluster is fully conserved in all seven isolates of PG2 lineage, along with their locus. This implies that gain of the cluster is prior diversification of the two lineages. Occurrence of non-biofilm forming strain, ATCC12228 with other S. epidermidis isolates in lineage PG2 is not surprising as all are commensal bacteria. However, presence of pathogenic strain, RP62A in the same clade is unexpected, hinting at minor differences in commensal S. epidermidis isolates and RP62A at genomic level.
Secondary Metabolites and Commensalism
Commensal S. epidermidis produces antimicrobials to inhibit the progression of pathogenic species, like S. aureus (Otto et al., 1999; Heikkilä and Saris, 2003; Iwase et al., 2010) and secondary metabolites for skin homeostasis (Lai et al., 2009; Belkaid and Segre, 2014; Wang et al., 2014) and stress tolerance (Gallo and Nakatsuji, 2011; Naik et al., 2012).
In fact, production of secondary metabolites is often related as a defined task of “being commensal” (Brestoff and Artis, 2013; Sharon et al., 2014). Presence of virulent or commensal genes focuses on the existences of two diverse phylogenetic lineages in commensal pool of S. epidermidis. However, presence of gene clusters for two antimicrobial peptides, a putative lantibiotic and sactipeptide, unique to SEI isolates on PG1 lineage, not only suggests commensal status of these isolates but also highlights diversity among SEA and SEI isolates belonging to same phylogenetic lineage but isolated from geographical locations. It is possible that the clusters have been acquired by horizontal gene transfer in recent past but after separation of these populations. One of the putative lantipeptide cluster showed 18% similarity to already identified lantipeptide from a clinical isolate of S. epidermidis, “Epilancin 15x,” which might have required the lantibiotic to survival and success. Presence of similar lantibiotic in commensal isolates of Indian origin, might play a role in ecological fitness of the isolates and their adaptation to stressed conditions like, humidity, high temperature etc. of the region. Existence of multiple secondary metabolite biosynthetic gene clusters is also supported by the fact that SEI isolates of PG1 lineage are clonal, which might maintains their clonality in order to conserve these metabolite gene clusters. Diverse SEA isolates might have lost these clusters due to frequent genome dynamics. In this context, it is surprising to note that a gene encoding macrolide-efflux protein is present only in SEI isolates from PG1 lineage. Further studies will allow us to understand selection pressure that has led to presence an antimicrobial resistance gene along with two antimicrobial peptide biosynthetic gene clusters in SEI isolates of PG1 lineage.
Presence of commensal specific marker, fdh in lineage PG1 and absence of virulent factors, icaA and IS256 from all isolates from PG1, suggests lineage PG1 is comprised of “true commensals.” Non-biofilm forming strain, ATCC12228, present in PG2, is also devoid of commensal associated fdh gene. Though ATCC12228 is a non-biofilm producing strain and was isolated from a healthy individual, it does have many virulence factors (Zhang et al., 2003) and seems to have compromised commensal status. Presence of pathogenic RP62A strain in PG2 lineage, frequency of virulence factors and absence of commensal associated gene, “fdh” from transitional and PG2 lineage, suggests that these isolates are of varying pathogenicity, might have potential to turn into a pathogenic strain. In addition, where the study reasserts “fdh” as a putative marker to recognize a true commensal among pool of numerous and geographically diverse skin isolates, it restricts the use of “arsD” gene for the same purpose. In fact, absence of “fdh” gene, with presence of any one virulence gene can be considered sufficient to redefine that isolate as a “potential pathogenic strain.”
Genome Dynamics Role in Adaptation of S. epidermidis
Phage attack is one of the most important factor bringing out diversity in genomes and contributing to genomic evolution (Wei et al., 2008; Rodriguez-Valera et al., 2009; Stern and Sorek, 2011). Phage, prophage, transposable elements and plasmids not only bring genomic diversity but they are also the important source of antibiotic resistance genes and other virulence factors. Limited intensity of these in SEI isolates and their variation in the two lineages indicate mobilomes acquisition in S. epidermidis isolates, which is lineage as well as geography dependent. Isolates in PG2 and transitional lineage are commensals and presence of virulence factors might be an outcome of phage acquisitions.
Pangenome analysis revealing open pangenome suggest ongoing variation and selection in S. epidermidis strains. These results were in agreement with the previous pan genomic study on S. epidermidis (Conlan et al., 2012), which also showed the role of the above stated gene classes as evolutionary force that shape S. epidermidis genomes (Conlan et al., 2012). Further, core and unique genes were analyzed for KEGG pathway, which showed that high fraction of core belongs to metabolic pathways that are required for the normal functioning of the cell (Conlan et al., 2012). Further, unique gene pool were associated with antibiotic resistance, diseases, secondary metabolites apart from important metabolic pathways and, replication and repair processes. Hence, the genomic evidence explains the dual role of commensal S. epidermidis in human skin protection and as well as its conversion as a skin pathogen in disease. These findings provide new perspectives on the intra-strain diversity from phylogenomics point of view of important skin commensals in the human health and disease management. Unique gene analysis could not reveal any significant or identifiable region, gene or cluster unique to a special population but can be in a large sub-population, as seen in SEI of PG1 lineage. This suggests that may be evolution of both population is geographically independent and is rather undergoing parallel evolution, diversifying as two lineages.
Phylogenomic study comparison has revealed two diverse lineages of S. epidermidis in healthy individuals, depicting distinct pattern in geographically diverse S. epidermidis isolates with respect to candidate and novel genes. These genes may have a possible role in virulence, commensalism and adaptation to skin. In addition, a putative antimicrobial gene cluster was seen present in one type of population and strikingly absent in another, signifying the importance of systematic ongoing evolution of this organism having an important role in human health and disease. Though formation of two major lineages seems independent of individual and body site, isolates VSE2-VSE5, present in PG2 lineage are from a single individual F3 and are isolates from opisthenar part of the hand. None of the isolates from this site was observed to be present in PG1 lineage and all of them harbored one or more pathogenic markers. This implies precise yet noteworthy role of body site in pathogenicity of S. epidermidis. Isolates of this site might have been undergoing comparatively large selection pressure as compared to other body sites, as they are far more exposed in different adverse environmental conditions. Interestingly, the study highlighted two geographically different populations that are following similar evolution trend, diversified into different lineages, one of which consists of true commensals (PG1) and other consists of potential pathogenic strains (PG2). Potential marker genes trend in both lineages and conserved locus of ica gene cluster, highlights that both PG1 and PG2 lineage isolates are evolved from a single ancestor having all these marker genes. True commensals isolates lost the pathogenic genes and retained the commensal marker gene, fdh, while PG2 isolates, lost the commensal markers and retained the pathogenic ones. Since, similar evolution trend is observed in both Indian and American isolates; it suggests an ongoing parallel evolution in S. epidermidis (Bailey et al., 2017).
Occurrence of some pathogenic strains in the otherwise commensal species, S. epidermidis, pose it as an accidental pathogen, which might have gained some of the virulence factors by horizontal gene transfer. However, presence of multiple lineages in S. epidermidis isolates collected from healthy individuals, independent of geography, individual or site of isolation, forces us to revisit the current hypothesis. The study mentions two major lineages, one of which can be regarded as “true commensal”; since it is devoid of any pathogenic, genes and another one contain strains which might hold the potential to be pathogenic. This suggests that occurrence of some pathogenic strains in this species might not be accidental and rather, is a result of ongoing negative frequency selection, shaping a diverse, disease-causing lineage among the commensal pool of S. epidermidis. Similar kind of results has been reported in many recent studies (Takeuchi et al., 2015; Corander et al., 2017). However, a detailed analysis including a large dataset, including global isolates and multiple factors (geography, site of isolation) is required to actually validate this hypothesis.
Conclusion
Our phylogeographic study has revealed that the S. epidermidis population harbored by healthy individuals consist of at least two phylogenomically divergent lineages, suggesting parallel evolution of a disease causing or opportunistic lineage. This can be seen in the distinct pattern in distribution of candidate gene(s) for pathogenicity and commensalism. This is reflected in the fact the SEA isolates understudy are more genomically dynamic and harbored pathogenicity markers in higher frequency On the other hand, isolates of Indian origin are less genomically dynamic, harbored less pathogenicity marker gene and have multiple unique antimicrobial peptide biosynthetic gene clusters. Further studies to understand the selection pressure for such systematic variation, having implication in S. epidermidis lifestyle, as an evolving pathogen or beneficial skin probiotic is required, to explore its potential in disease management.
Author Contributions
SS and VC carried out isolation of strains. SS and VC are involved in genome sequencing, submission and analysis. SS, VC and SK were involved in insilico analysis of the data. VC, SS, and PP participated in conception, design of the study, interpretation of data and drafting of the manuscript. PP applied for funding. All authors reviewed and approved the manuscript.
Conflict of Interest Statement
The authors declare that the research was conducted in the absence of any commercial or financial relationships that could be construed as a potential conflict of interest.
Acknowledgments
SS is supported by senior research Fellowship from University Grant Commission (UGC). VC gratefully acknowledges support from DST-SERB (SERB-National Post-Doctoral Fellowship), New Delhi, India. We acknowledge CSIR network project, Man as a Super-organism Understanding the Human Microbiome (HUM- BSC0119) and Microbiome Based Consortia as Therapeutics for Sepsis (OLP0601-20) for financial support to PP. We thank Director, IMTECH, for encouragement and support.
Supplementary Material
The Supplementary Material for this article can be found online at: https://www.frontiersin.org/articles/10.3389/fmicb.2018.00333/full#supplementary-material
References
Aanensen, D. M., and Spratt, B. G. (2005). The multilocus sequence typing network: mlst. net. Nucleic Acids Res. 33, W728–W733. doi: 10.1093/nar/gki415
Alikhan, N.-F., Petty, N. K., Zakour, N. L. B., and Beatson, S. A. (2011). BLAST Ring Image Generator (BRIG): simple prokaryote genome comparisons. BMC Genomics 12:402. doi: 10.1186/1471-2164-12-402
Archer, G. L. (1978). Antimicrobial susceptibility and selection of resistance among Staphylococcus epidermidis isolates recovered from patients with infections of indwelling foreign devices. Antimicrob. Agents chemother. 14, 353–359. doi: 10.1128/AAC.14.3.353
Aziz, R. K., Bartels, D., Best, A. A., DeJongh, M., Disz, T., Edwards, R. A., et al. (2008). The RAST Server: rapid annotations using subsystems technology. BMC Genomics 9:75. doi: 10.1186/1471-2164-9-75
Bailey, S. F., Blanquart, F., Bataillon, T., and Kassen, R. (2017). What drives parallel evolution?. Bioessays 39, 1–9. doi: 10.1002/bies.201600176
Bankevich, A., Nurk, S., Antipov, D., Gurevich, A. A., Dvorkin, M., Kulikov, A. S., et al. (2012). SPAdes: a new genome assembly algorithm and its applications to single-cell sequencing. J. Comput. Biol. 19, 455–477. doi: 10.1089/cmb.2012.0021
Belkaid, Y., and Segre, J. A. (2014). Dialogue between skin microbiota and immunity. Science 346, 954–959. doi: 10.1126/science.1260144
Belkaid, Y., and Tamoutounour, S. (2016). The influence of skin microorganisms on cutaneous immunity. Nat. Rev. Immunol. 16, 353–366. doi: 10.1038/nri.2016.48
Brestoff, J. R., and Artis, D. (2013). Commensal bacteria at the interface of host metabolism and the immune system. Nat. Immunol. 14, 676–684. doi: 10.1038/ni.2640
Carver, T., Harris, S. R., Berriman, M., Parkhill, J., and McQuillan, J. A. (2012). Artemis: an integrated platform for visualization and analysis of high-throughput sequence-based experimental data. Bioinformatics 28, 464–469. doi: 10.1093/bioinformatics/btr703
Chaudhari, N. M., Gupta, V. K., and Dutta, C. (2016). BPGA-an ultra-fast pan-genome analysis pipeline. Sci. Rep. 6:24373. doi: 10.1038/srep24373
Chaudhry, V., and Patil, P. B. (2016). Genomic investigation reveals evolution and lifestyle adaptation of endophytic Staphylococcus epidermidis. Sci. Rep. 6:19263. doi: 10.1038/srep19263
Conlan, S., Mijares, L. A., Becker, J., Blakesley, R. W., Bouffard, G. G., Brooks, S., et al. (2012). Staphylococcus epidermidis pan-genome sequence analysis reveals diversity of skin commensal and hospital infection-associated isolates. Genome Biol. 13:R64. doi: 10.1186/gb-2012-13-7-r64
Corander, J., Fraser, C., Gutmann, M. U., Arnold, B., Hanage, W. P., Bentley, S. D., et al. (2017). Frequency-dependent selection in vaccine-associated pneumococcal population dynamics. Nat. Ecol. Evol. 1, 1950-1960. doi: 10.1038/s41559-017-0337-x
Costerton, J. W., Stewart, P. S., and Greenberg, E. P. (1999). Bacterial biofilms: a common cause of persistent infections. Science 284, 1318–1322. doi: 10.1126/science.284.5418.1318
Dave, S. B., Toma, H. S., and Kim, S. J. (2011). Ophthalmic antibiotic use and multidrug-resistant staphylococcus epidermidis: a controlled, longitudinal study. Ophthalmology 118, 2035–2040. doi: 10.1016/j.ophtha.2011.03.017
Egert, M., Simmering, R., and Riedel, C. U. (2017). The association of the skin microbiota with health, immunity, and disease. Clin. Pharmacol. Ther. 102, 62–69. doi: 10.1002/cpt.698
Farran, C. E., Sekar, A., Balakrishnan, A., Shanmugam, S., Arumugam, P., and Gopalswamy, J. (2013). Prevalence of biofilm-producing Staphylococcus epidermidis in the healthy skin of individuals in Tamil Nadu, India. Indian J. Med. Microbiol. 31, 19-23. doi: 10.4103/0255-0857.108712
Galdbart, J.-O., Allignet, J., Tung, H.-S., Rydèn, C., and El Solh, N. (2000). Screening for Staphylococcus epidevmidis markers discriminating between skin-flora strains and those responsible for infections of joint prostheses. J. Infect. Dis. 182, 351–355. doi: 10.1086/315660
Gallo, R. L., and Nakatsuji, T. (2011). Microbial symbiosis with the innate immune defense system of the skin. J. Invest. Dermatol. 131, 1974–1980. doi: 10.1038/jid.2011.182
Gill, S. R., Fouts, D. E., Archer, G. L., Mongodin, E. F., DeBoy, R. T., Ravel, J., et al. (2005). Insights on evolution of virulence and resistance from the complete genome analysis of an early methicillin-resistant Staphylococcus aureus strain and a biofilm-producing methicillin-resistant Staphylococcus epidermidis strain. J. Bacteriol. 187, 2426–2438. doi: 10.1128/JB.187.7.2426-2438.2005
Grice, E. A., and Segre, J. A. (2011). The skin microbiome. Nat. Rev. Microbiol. 9, 244–253. doi: 10.1038/nrmicro2537
Gu, J., Li, H., Li, M., Vuong, C., Otto, M., Wen, Y., et al. (2005). Bacterial insertion sequence IS256 as a potential molecular marker to discriminate invasive strains from commensal strains of Staphylococcus epidermidis. J. Hosp. Infect. 61, 342–348. doi: 10.1016/j.jhin.2005.04.017
Harris, L. G., Murray, S., Pascoe, B., Bray, J., Meric, G., Magerios, L., et al. (2016). Biofilm morphotypes and population structure among Staphylococcus epidermidis from commensal and clinical samples. PLoS ONE 11:e0151240. doi: 10.1371/journal.pone.0151240
Heikkilä, M., and Saris, P. (2003). Inhibition of Staphylococcus aureus by the commensal bacteria of human milk. J. Appl. Microbiol. 95, 471–478. doi: 10.1046/j.1365-2672.2003.02002.x
Heilmann, C., Schweitzer, O., Gerke, C., Vanittanakom, N., Mack, D., and Götz, F. (1996). Molecular basis of intercellular adhesion in the biofilm-forming Staphylococcus epidermidis. Mol. Microbiol. 20, 1083–1091. doi: 10.1111/j.1365-2958.1996.tb02548.x
Hidron, A. I., Edwards, J. R., Patel, J., Horan, T. C., Sievert, D. M., Pollock, D. A., et al. (2008). Antimicrobial-resistant pathogens associated with healthcare-associated infections: annual summary of data reported to the National Healthcare Safety Network at the Centers for Disease Control and Prevention, 2006–2007. Infect. Control Hosp. Epidemiol. 29, 996–1011. doi: 10.1086/591861
Hunter, J. D. (2007). Matplotlib: a 2D graphics environment. Comput. Sci. Eng. 9, 90–95. doi: 10.1109/MCSE.2007.55
Iwase, T., Uehara, Y., Shinji, H., Tajima, A., Seo, H., Takada, K., et al. (2010). Staphylococcus epidermidis Esp inhibits Staphylococcus aureus biofilm formation and nasal colonization. Nature 465, 346–349. doi: 10.1038/nature09074
Johnson, M., Zaretskaya, I., Raytselis, Y., Merezhuk, Y., McGinnis, S., and Madden, T. L. (2008). NCBI BLAST: a better web interface. Nucleic Acids Res. 36, W5–W9. doi: 10.1093/nar/gkn201
Jolley, K. A., Chan, M. S., and Maiden, M. C. (2004). mlstdbNet–distributed multi-locus sequence typing (MLST) databases. BMC Bioinformatics 5:86. doi: 10.1186/1471-2105-5-86
Kellner, R., Jung, G., Hörner, T., Zähner, H., Schnell, N., Entian, K. D., and Götz, F. (1988). Gallidermin: a new lanthionine-containing polypeptide antibiotic. FEBS J. 177, 53–59.
Kim, O. S., Cho, Y. J., Lee, K., Yoon, S. H., Kim, M., Na, H., et al. (2012). Introducing EzTaxon-e: a prokaryotic 16S rRNA gene sequence database with phylotypes that represent uncultured species. Int. J. Syst. Evol. Microbiol. 62, 716–721. doi: 10.1099/ijs.0.038075-0
Kong, H. H., Oh, J., Deming, C., Conlan, S., Grice, E. A., Beatson, M. A., et al. (2012). Temporal shifts in the skin microbiome associated with disease flares and treatment in children with atopic dermatitis. Genome Res. 22, 850–859. doi: 10.1101/gr.131029.111
Kozitskaya, S., Cho, S.-H., Dietrich, K., Marre, R., Naber, K., and Ziebuhr, W. (2004). The bacterial insertion sequence element IS256 occurs preferentially in nosocomial Staphylococcus epidermidis isolates: association with biofilm formation and resistance to aminoglycosides. Infect. Immun. 72, 1210–1215. doi: 10.1128/IAI.72.2.1210-1215.2004
Lagesen, K., Hallin, P., Rødland, E. A., Stærfeldt, H.-H., Rognes, T., and Ussery, D. W. (2007). RNAmmer: consistent and rapid annotation of ribosomal RNA genes. Nucleic Acids Res. 35, 3100–3108. doi: 10.1093/nar/gkm160
Lai, Y., Cogen, A. L., Radek, K. A., Park, H. J., MacLeod, D. T., Leichtle, A., et al. (2010). Activation of TLR2 by a small molecule produced by Staphylococcus epidermidis increases antimicrobial defense against bacterial skin infections. J. Invest. Dermatol. 130, 2211–2221. doi: 10.1038/jid.2010.123
Lai, Y., Di Nardo, A., Nakatsuji, T., Leichtle, A., Yang, Y., Cogen, A. L., et al. (2009). Commensal bacteria regulate Toll-like receptor 3–dependent inflammation after skin injury. Nat. Med. 15, 1377–1382. doi: 10.1038/nm.2062
Larsen, M. V., Cosentino, S., Rasmussen, S., Friis, C., Hasman, H., Marvig, R. L., et al. (2012). Multilocus sequence typing of total-genome-sequenced bacteria. J. Clin. Microbiol. 50, 1355–1361. doi: 10.1128/JCM.06094-11
Laxminarayan, R., and Chaudhury, R. R. (2016). Antibiotic resistance in India: drivers and opportunities for action. PLoS Med. 13:e1001974. doi: 10.1371/journal.pmed.1001974
Lazaris, A., Coleman, D. C., Kearns, A. M., Pichon, B., Kinnevey, P. M., Earls, M. R., et al. (2017). Novel multiresistance cfr plasmids in linezolid-resistant methicillin-resistant Staphylococcus epidermidis and vancomycin-resistant Enterococcus faecium (VRE) from a hospital outbreak: co-location of cfr and optrA in VRE. J. Antimicrob. Chemother. 72, 3252–3257. doi: 10.1093/jac/dkx292
Letunic, I., and Bork, P. (2006). Interactive Tree Of Life (iTOL): an online tool for phylogenetic tree display and annotation. Bioinformatics 23, 127–128. doi: 10.1093/bioinformatics/btl529
Li, H., Xu, L., Wang, J., Wen, Y., Vuong, C., Otto, M., et al. (2005). Conversion of Staphylococcus epidermidis strains from commensal to invasive by expression of the ica locus encoding production of biofilm exopolysaccharide. Infect. Immun. 73, 3188–3191. doi: 10.1128/IAI.73.5.3188-3191.2005
Lowe, T. M., and Eddy, S. R. (1997). tRNAscan-SE: a program for improved detection of transfer RNA genes in genomic sequence. Nucleic Acids Res. 25, 955–964. doi: 10.1093/nar/25.5.0955
Marr, K. A., Sexton, D. J., Conlon, P. J., Corey, G. R., Schwab, S. J., and Kirkland, K. B. (1997). Catheter-related bacteremia and outcome of attempted catheter salvage in patients undergoing hemodialysis. Ann. Intern. Med. 127, 275–280. doi: 10.7326/0003-4819-127-4-199708150-00003
Medema, M. H., Blin, K., Cimermancic, P., de Jager, V., Zakrzewski, P., Fischbach, M. A., et al. (2011). antiSMASH: rapid identification, annotation and analysis of secondary metabolite biosynthesis gene clusters in bacterial and fungal genome sequences. Nucleic Acids Res. 39, W339–W346. doi: 10.1093/nar/gkr466
Méric, G., Miragaia, M., de Been, M., Yahara, K., Pascoe, B., Mageiros, L., et al. (2015). Ecological overlap and horizontal gene transfer in Staphylococcus aureus and Staphylococcus epidermidis. Genome Biol. Evol. 7, 1313–1328. doi: 10.1093/gbe/evv066
Miragaia, M., Couto, I., and Lencastre, H. D. (2005). Genetic diversity among methicillin-resistant Staphylococcus epidermidis (MRSE). Microb. Drug Resist. 11, 83–93. doi: 10.1089/mdr.2005.11.83
Mishra, S. K., Basukala, P., Basukala, O., Parajuli, K., Pokhrel, B. M., and Rijal, B. P. (2015). Detection of biofilm production and antibiotic resistance pattern in clinical isolates from indwelling medical devices. Curr. Microbiol. 70, 128–134. doi: 10.1007/s00284-014-0694-5
Naik, S., Bouladoux, N., Wilhelm, C., Molloy, M. J., Salcedo, R., Kastenmuller, W., et al. (2012). Compartmentalized control of skin immunity by resident commensals. Science 337, 1115–1119. doi: 10.1126/science.1225152
Nakatsuji, T., Chen, T. H., Narala, S., Chun, K. A., Yun, T., Shafiq, F., et al. (2017). Antimicrobials from human skin commensal bacteria protect against Staphylococcus aureus and are deficient in atopic dermatitis. Science Transl. Med. 9:eaah4680. doi: 10.1126/scitranslmed.aah4680
Otto, M. (2009). Staphylococcus epidermidis—the'accidental'pathogen. Nat. Rev. Microb. 7, 555–567. doi: 10.1038/nrmicro2182
Otto, M. (2017). Staphylococcus epidermidis: a major player in bacterial sepsis? Future Microbiol. 12, 1031–1033. doi: 10.2217/fmb-2017-0143
Otto, M., Süßmuth, R., Vuong, C., Jung, G., and Götz, F. (1999). Inhibition of virulence factor expression in Staphylococcus aureus by the Staphylococcus epidermidis agr pheromone and derivatives. FEBS Lett. 450, 257–262. doi: 10.1016/S0014-5793(99)00514-1
Percival, S. L., Suleman, L., Vuotto, C., and Donelli, G. (2015). Healthcare-associated infections, medical devices and biofilms: risk, tolerance and control. J. Med. Microbiol. 64, 323–334. doi: 10.1099/jmm.0.000032
Post, V., Harris, L. G., Morgenstern, M., Mageiros, L., Hitchings, M. D., Méric, G., et al. (2017). Comparative genomics study of Staphylococcus epidermidis isolates from orthopedic-device-related infections correlated with patient outcome. J. Clin. Microbiol. 55, 3089–3103. doi: 10.1128/JCM.00881-17
Qin, L., Da, F., Fisher, E. L., Tan, D. C., Nguyen, T. H., Fu, C.-L., et al. (2017). Toxin mediates sepsis caused by methicillin-resistant Staphylococcus epidermidis. PLoS Pathog. 13:e1006153. doi: 10.1371/journal.ppat.1006153
Raad, I., Alrahwan, A., and Rolston, K. (1998). Staphylococcus epidermidis: emerging resistance and need for alternative agents. Rev. Infect. Dis. 26, 1182–1187. doi: 10.1086/520285
Rabaud, C., and Mauuary, G. (2001). Infection and/or colonization by methicillin-resistant Staphylococcus epidermidis (MRSE). Pathol. Biol. 49, 812–814. doi: 10.1016/S0369-8114(01)00221-8
Richter, M., and Rosselló-Móra, R. (2009). Shifting the genomic gold standard for the prokaryotic species definition. Proc. Natl. Acad. Sci. U.S.A. 106, 19126–19131. doi: 10.1073/pnas.0906412106
Rodriguez-Valera, F., Martin-Cuadrado, A.-B., Rodriguez-Brito, B., Pašić, L., Thingstad, T. F., Rohwer, F., et al. (2009). Explaining microbial population genomics through phage predation. Nat. Rev. Microbiol. 7, 828–836. doi: 10.1038/nrmicro2235
Rogers, K. L., Fey, P. D., and Rupp, M. E. (2009). Coagulase-negative staphylococcal infections. Infect. Dis. Clin. North Am. 23, 73–98. doi: 10.1016/j.idc.2008.10.001
Segata, N., Börnigen, D., Morgan, X. C., and Huttenhower, C. (2013). PhyloPhlAn is a new method for improved phylogenetic and taxonomic placement of microbes. Nat. Commun. 4:2304. doi: 10.1038/ncomms3304
Sgro, M., Shah, P. S., Campbell, D., Tenuta, A., Shivananda, S., Lee, S. K., et al. (2011). Early-onset neonatal sepsis: rate and organism pattern between 2003 and 2008. J. Perinatol. 31, 794–798 doi: 10.1038/jp.2011.40
Sharon, G., Garg, N., Debelius, J., Knight, R., Dorrestein, P. C., and Mazmanian, S. K. (2014). Specialized metabolites from the microbiome in health and disease. Cell Metab. 20, 719–730. doi: 10.1016/j.cmet.2014.10.016
Skabytska, Y., and Biedermann, T. (2016). Staphylococcus epidermidis sets things right again. J. Invest. Dermatol. 136, 559–560. doi: 10.1016/j.jid.2015.11.016
Stern, A., and Sorek, R. (2011). The phage-host arms race: shaping the evolution of microbes. Bioessays 33, 43–51. doi: 10.1002/bies.201000071
Takeuchi, N., Cordero, O. X., Koonin, E. V., and Kaneko, K. (2015). Gene-specific selective sweeps in bacteria and archaea caused by negative frequency-dependent selection. BMC Biol. 13:20. doi: 10.1186/s12915-015-0131-7
Takizawa, T., Tsutsumimoto, T., Yui, M., and Misawa, H. (2017). Surgical site infections caused by methicillin-resistant Staphylococcus epidermidis after spinal instrumentation surgery. Spine 42, 525–530. doi: 10.1097/BRS.0000000000001792
van Heel, A. J., de Jong, A., Montalban-Lopez, M., Kok, J., and Kuipers, O. P. (2013). BAGEL3: automated identification of genes encoding bacteriocins and (non-) bactericidal posttranslationally modified peptides. Nucleic Acids Res. 41, W448–W453. doi: 10.1093/nar/gkt391
Varani, S., Stanzani, M., Paolucci, M., Melchionda, F., Castellani, G., Nardi, L., et al. (2009). Diagnosis of bloodstream infections in immunocompromised patients by real-time PCR. J. Infect. 58, 346–351. doi: 10.1016/j.jinf.2009.03.001
Velásquez, J. E., Zhang, X., and Van Der Donk, W. A. (2011). Biosynthesis of the antimicrobial peptide epilancin 15X and its N-terminal lactate. Chem. Biol. 18, 857–867. doi: 10.1016/j.chembiol.2011.05.007
Wang, Y., Kuo, S., Shu, M., Yu, J., Huang, S., Dai, A., et al. (2014). Staphylococcus epidermidis in the human skin microbiome mediates fermentation to inhibit the growth of Propionibacterium acnes: implications of probiotics in acne vulgaris. Appl. Microbiol. Biotechnol. 98, 411–424. doi: 10.1007/s00253-013-5394-8
Wei, W., Davis, R. E., Jomantiene, R., and Zhao, Y. (2008). Ancient, recurrent phage attacks and recombination shaped dynamic sequence-variable mosaics at the root of phytoplasma genome evolution. Proc. Natl. Acad. Sci. U.S.A. 105, 11827–11832. doi: 10.1073/pnas.0805237105
Widerström, M., McCullough, C. A., Coombs, G. W., Monsen, T., and Christiansen, K. J. (2012a). A multidrug-resistant Staphylococcus epidermidis clone (ST2) is an ongoing cause of hospital acquired infection in a Western Australian hospital. J. Clin. Microbiol. 50, 2147-2151. doi: 10.1128/JCM.06456-11
Widerström, M., Wiström, J., Sjöstedt, A., and Monsen, T. (2012b). Coagulase negative staphylococci: update on the molecular epidemiology and clinical presentation, with a focus on Staphylococcus epidermidis and Staphylococcus saprophyticus. Euro. J. Clin. Microbiol. Infect. Dis. 31, 7–20. doi: 10.1007/s10096-011-1270-6
Zhang, Y. Q., Ren, S. X., Li, H. L., Wang, Y. X., Fu, G., Yang, J., et al. (2003). Genome-based analysis of virulence genes in a non-biofilm-forming Staphylococcus epidermidis strain (ATCC 12228). Mol. Microbiol. 49, 1577–1593. doi: 10.1046/j.1365-2958.2003.03671.x
Keywords: Staphylococcus epidermidis, commensal, geographic, markers, pathogen, phylogeographic, evolution, phylogenomics
Citation: Sharma S, Chaudhry V, Kumar S and Patil PB (2018) Phylogenomic Based Comparative Studies on Indian and American Commensal Staphylococcus epidermidis Isolates. Front. Microbiol. 9:333. doi: 10.3389/fmicb.2018.00333
Received: 15 November 2017; Accepted: 12 February 2018;
Published: 27 February 2018.
Edited by:
Yasir Muhammad, King Abdulaziz University, Saudi ArabiaReviewed by:
Sandip Paul, Indian Institute of Chemical Biology (CSIR), IndiaBen Pascoe, University of Bath, United Kingdom
Copyright © 2018 Sharma, Chaudhry, Kumar and Patil. This is an open-access article distributed under the terms of the Creative Commons Attribution License (CC BY). The use, distribution or reproduction in other forums is permitted, provided the original author(s) and the copyright owner are credited and that the original publication in this journal is cited, in accordance with accepted academic practice. No use, distribution or reproduction is permitted which does not comply with these terms.
*Correspondence: Prabhu B. Patil, cGJwYXRpbEBpbXRlY2gucmVzLmlu
†These authors have contributed equally to this work.