- 1Fujian Key Laboratory of Traditional Chinese Veterinary Medicine and Animal Health, Fujian Agriculture and Forestry University, Fuzhou, China
- 2Department of Biosciences, Faculty of Sciences, COMSATS Institute of Information Technology, Islamabad, Pakistan
In this study, microcosms were established to determine the effect of nitrogen (N) and phosphorus (P) on the multidrug resistance and biofilm-forming abilities of Escherichia coli. The expression of biofilm-formation-related genes was detected to establish correlations between genotype and phenotype. Different concentrations of N and P were added to make one control group and four treatment groups. The glass tube method was used to determine biofilm-forming capabilities. Real-time PCR was used to detect the mRNA abundance of six biofilm-formation-related genes in E. coli. No resistant strains were isolated from the control group; meanwhile, multidrug resistance rates were high in the treatment groups. Expression of the biofilm-associated genes luxS, flhD, fliA, motA, and fimH was detected in all treatment groups; however, there was no expression of mqsR. The expression of luxS, flhD, fliA, motA, and fimH significantly correlated with the concentration of N and P, as well as with the appearance and duration of multidrug resistance in different groups. Overall, the results of this study suggest that biofilm-forming ability plays a key role in the formation of multidrug resistance in E. coli after the addition of N and P to a microcosm.
Introduction
Diverse aquatic microorganisms including Escherichia coli population stick to each other to form a colonizing surface which leads to the formation of biofilm (Dang and Lovell, 2016). In these associations, the microbes are exposed to diverse environmental conditions such as nutrient availability, temperature, pH, and so on. One of the main properties of biofilm development is to promote resistance to antibiotics compared with planktonic cells (Soto, 2013). Furthermore, biofilms protect microbes against environmental stresses and result in increased resistance to antimicrobial treatments and disinfectants (Sanchez-Vizuete et al., 2015). The reduced sensitivity of biofilms to antibiotics is a critical problem in the chronic infection treatments. Approximately 65% of bacterial infections are linked with biofilms (Romling and Balsalobre, 2012). Furthermore, biofilm are 100–1,000 times more resistant to antibiotics than planktonic bacteria (Ito et al., 2009).
Biofilm resistance is one of the main modes of transmission of drug resistance in E. coli. It is also the way that most biofilm forming bacteria survive in hostile environments. In drinking water, the biofilm development is dependent on the physiological state of E. coli rather than anaerobic conditions (Farkas et al., 2016). Bjergbæk et al. (2006) found that minimal and complex media may affect E. coli biofilm development in aerobic condition. Furthermore, this bacterium is also capable to develop biofilm in anaerobic conditions. Higher phosphorus (P) concentrations in drinking water promote biofilm and prolonged the survival of E. coli (Juhna et al., 2007). Cerca and Jefferson (2008) found that while the expression of pga (a gene operon of E. coli) and polysaccharide synthesis were induced by ethanol, NaCl, and glucose, however, biofilm formation was only induced by glucose. At a high glucose concentration (1 g⋅L-1), the extreme biofilm volume was achieved after 24 h; similar values were obtained in incubators with orbital diameters of both 50 and 25 mm. At a low glucose concentration (0.5 g⋅L-1), lower biofilm volumes were obtained (Moreira et al., 2013).
Biofilm development within aquatic environment depends on the chemical and physical characteristics of the water and sediments. In the underlying sediments, nutrient, dissolved organic carbon, carbon dioxide, and oxygen concentrations can vary in spatial and temporal regions with respect to temperature, pH, diurnal, and annual changes that lead to horizontal and vertical stratifications of biofilm development. In the sediment, nutrients such as nitrogen (N) and phosphorus (P) play important role in the biofilm development (Ahmad et al., 2017; Shrestha and Afsar, 2017). Previous studies have reported that cornmeal; N and P induced fecal colonization and antibiotic resistance in mesocosm experiments. Mesocosm constructions are useful tools because these approaches allow researchers to simulate realistic environmental conditions, replicates of different treatments, and extended periods of experimentation (Taub, 1997). In recent years, controlled conditions have been used to evaluate the bacterial biofilm development in marine and freshwater, using mesocosm and characterized by taxonomy, microscopy, gravimetry, chemical analysis and molecular biology (Zippel et al., 2007; Ali et al., 2016).
To the best of our knowledge, previous studies have not examined the direct relationship between nutritional factors and biofilm formation and drug resistance, nor dose-effect and time-effect associations. Biofilm formation-related genes play different roles in biofilm formation; it is necessary to determine the expression levels of these genes, as well as the relationship between their expression levels and the level of nutrition and drug resistance.
We have previously studied the impacts on the drug resistance of Enterococcus spp. and E. coli of adding cornmeal, glucose, nitrogen (N), and phosphorus (P) nutrients to mesocosms (Yu et al., 2009, 2012; Xiao et al., 2015; Ali et al., 2016; Zhang et al., 2016). Yu et al. (2009) established mesocosm experiments to study the effects of feed residue on the acquired antibiotic resistance of bacteria in sediment. Furthermore, Yu et al. (2012) examined the antibiotic resistance in a mesocosm containing different concentrations of cornmeal. In the presence of several environmental factors in aquatic environments, biofilm development is one of the best survival strategies for microbes. Previous studies have often focused on bacterial resistance, while little is known about biofilm development in aquatic environments. In this study, the effect of N and P on the multidrug resistance and biofilm-forming abilities of E. coli were examined, as well as the correlations between the expression of biofilm-formation-related genes and different stages of biofilm formation. This provides a deeper understanding of the mechanism of bacterial resistance caused by nutritional factors, improves awareness of environmental pollution, and provides a theoretical basis for improved control of bacterial resistance.
Materials and Methods
Materials
Microcosm
Sediment: soil without antibiotics was collected from Fuzhou Forest Park, Fujian Province, China. Water: moderately filtered water (single distilled water) was exposed to sunlight for 1 day. Plastic bucket: a white transparent storage box with a volume of 12 L. Tents: 3 m × 3 m × 2 m, surrounded by white transparent plastic film, with top covering of a plastic film. The microcosms were arranged in a randomized complete block design in five blocks. Each block represents different treatment (three replicate microcosms per treatment).
Standard Antibiotics
Ampicillin, cefazolin, cefotaxime, gentamicin, ciprofloxacin, tetracycline, and chloramphenicol were purchased from the China Pharmaceutical and Biological Products Inspection Institute.
Reagents
Premix TaqTM (Takara TaqTM Version 2.0), a PrimeScript RT reagent Kit with gDNA Eraser, SYBR® Premix Ex TaqTM (Tli RNaseH Plus), DL500 Marker, and 6 × Agarose Gel Loading buffer were purchased from Takara (Dalian, China). TAE buffer (5 ×) was purchased from Sangon Biotech Co., Ltd. (Shanghai, China).
Standard Strain
Escherichia coli (ATCC 25922) was purchased from the National Institute for the Control of Pharmaceutical and Biological Products (Beijing, China).
Methods
Microcosm
Autoclaved soil (15 kg) was evenly distributed in 12 L white transparent pails and mixed with 10 L of distilled water and the appropriate standard dilution of E. coli ATCC 25922. The water level of each pail was marked and maintained at a constant volume. The transparent buckets were placed in plastic greenhouses for 30 days, with about 10 cm between buckets.
Sample Collection
Bacterial samples were collected from the microcosm sediments on days 1, 8, 15, 22, 29, 36, 54, and 85 as described previously (Yu et al., 2012). These samples were collected from four random locations of each microcosm (five treatments and three replicates) for further analyses. In order to avoid overlapping, the samples were collected from consistently dispersed points of the entire microcosm. The total nitrogen (TN) and total phosphorus (TP) levels were measured during incubation. Once the system was set up, the water-sediment interface was sampled (0 day). Then, sterilized N (NH4NO3) and P (NaH2PO4) were added at the specified concentrations. Samples were taken at 1, 8, 15, 22, 29, 36, 54, and 85 days. There was one control group and four treatment groups; each group was run in triplicate. Based on preliminary tests (data not shown), the added concentrations of TN and TP in the microcosm were lowered. The final nutrient concentrations of all treatment groups ranged from moderately eutrophic to hypertrophic (Table 1).
Escherichia coli Sensitivity Test
Colonies were suspended in Mueller-Hinton Broth (MHB) for 6 h and quantified using an ultraviolet Spectrophotometer (Thermo Fisher Scientific Inc., Wilmington, DE, United States). The cultures were then diluted with sterilized MHB broth (about 10 times to achieve the absorbance value between 0.08 and0.10). Broth dilution technique was carried out to determine resistance to the antimicrobials (Table 2).
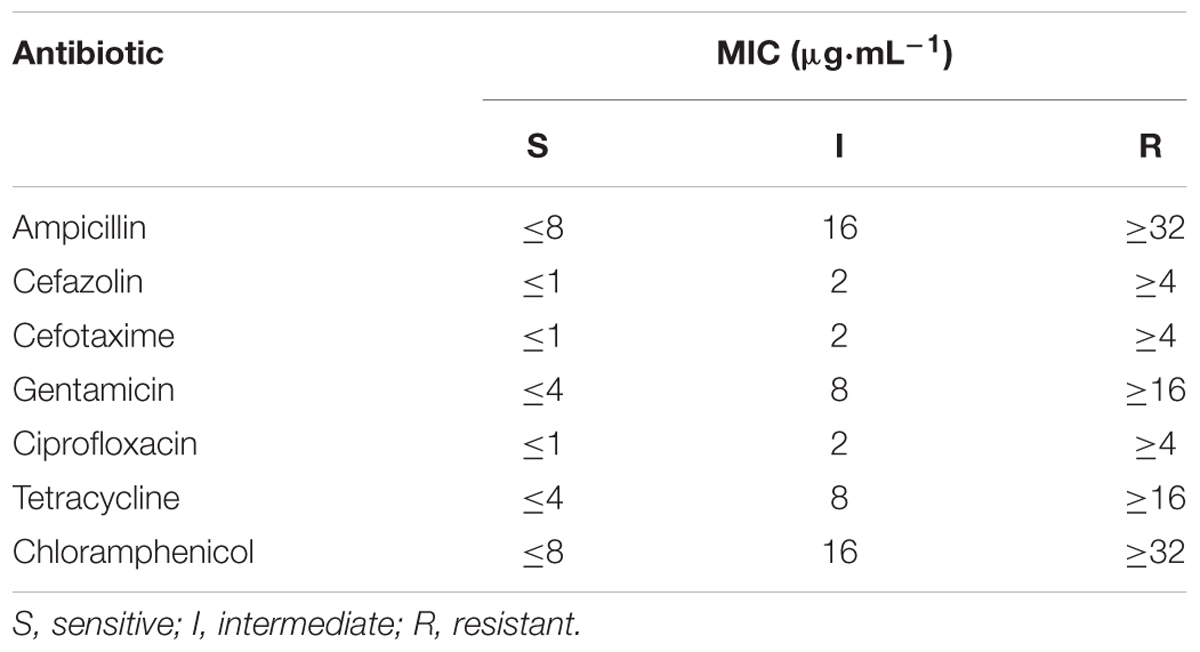
TABLE 2. Standard of equivalent minimal inhibitory concentration (MIC) breakpoints for Escherichia coli.
Antibiotic resistance testing was performed according to the broth microdilution method (Clinical and Laboratory Standards Institute [CLSI], 2015). Strains with an MIC less than or equal to the break points were considered susceptible; those higher than the break points were considered resistant. Susceptibility test results were interpreted using Clinical and Laboratory Standards Institute [CLSI] (2006) breakpoint criteria (Table 2).
Glass Tube Test
A tube containing 1 mL of liquid medium was inoculated with 10 μL of E. coli and incubated at 37°C for 17 h. Then, the medium was removed and the cells were stained with 1.5 mL of 1% crystal violet at 18–20°C (room temperature) for 30 min. The crystal violet staining solution was removed by washing with clear water until colorless water was obtained; then, the cells were dried at room temperature. Visual observation was performed to observe the junction of the liquid level and color of the glass tube wall. Pictures were taken to record the results.
Real-Time PCR
Total RNA was extracted from 45 samples using TRIzol method (Invitrogen) and cDNA was obtained by reverse transcription kit (Takara Dalian, China). Real-time PCR was performed according to the conditions described previously (Maeda et al., 2003). Seven sets of PCR primers (Table 3) were used (synthesized by Sangon Biotech). To ensure primer specificity, agarose gels with the PCR product were run and product melting was assessed at the end of the reaction to verify the specificity of the reaction (Supplementary Figure S1). Standards for all seven genes (gapA, luxS, mqsR, flhD, fliA, motA, and fimH) were prepared and were serially diluted over five or six orders of magnitude to generate a standard curve (Supplementary Figure S2). The PCR conditions were: pre-denaturation at 95°C for 2 min, then 39 cycles of denaturation at 95°C for 15 s, annealing at 62°C for 15 s, and extension at 72°C for 20 s. After the cycling program, a melting curve was generated by cooling the samples to 65°C for 2 min and then slowly increasing the temperature to 95°C with a slope of 0.2°C/s while measuring fluorescence continuously.
Statistical Analysis
Quantification cycle (Cq) values were obtained after completion of the fluorescence quantitative PCR reaction. The relative abundance of the target gene in each sample and calibrator (blank group) was calculated using the Cq value of the internal reference gene gapA as a control and the relative expression level of the target gene = 2-ΔΔCq (where ΔCq = Cq(target gene) - Cq(reference gene), and ΔΔCq = ΔCq(test) - ΔCq(calibrator)) as described previously (Livak and Schmittgen, 2001). Statistical analysis was performed using SPSS software (version 10.0).
Results
Multidrug Resistance in E. coli
In the control group, no resistant E. coli strains were obtained (Table 4). The multidrug resistance rate was highest in the medium N and P dose group; the rate was second highest in the high-dose group. The resistance rate was lowest in the lowest- and low-dose groups. After 1 day, high multidrug resistance rates were found in the lowest, medium, and high dose groups. In the medium dose group, there were multiple multidrug-resistant E. coli strains isolated on selected days after the addition of N and P. In all treatment groups, there were high levels of multidrug-resistant strains from day 29 to the end of the experiment.
Biofilm Formation of E. coli in Different Dose Groups Detected by Glass Tube Method
There was no biofilm formation in the control group or at day 0 in any of the treatment groups. After adding N and P nutrients, biofilm formation in the medium-dose group was the highest, followed by the high-dose and two low-dose groups (Figure 1). The biofilm that formed on the glass tube of the middle-dose group was visually apparent from the 1st day onward; meanwhile, the biofilm in the other groups was visually apparent from the 29th day onward. There was a positive correlation between the multidrug resistance rate and biofilm formation (Figure 2).
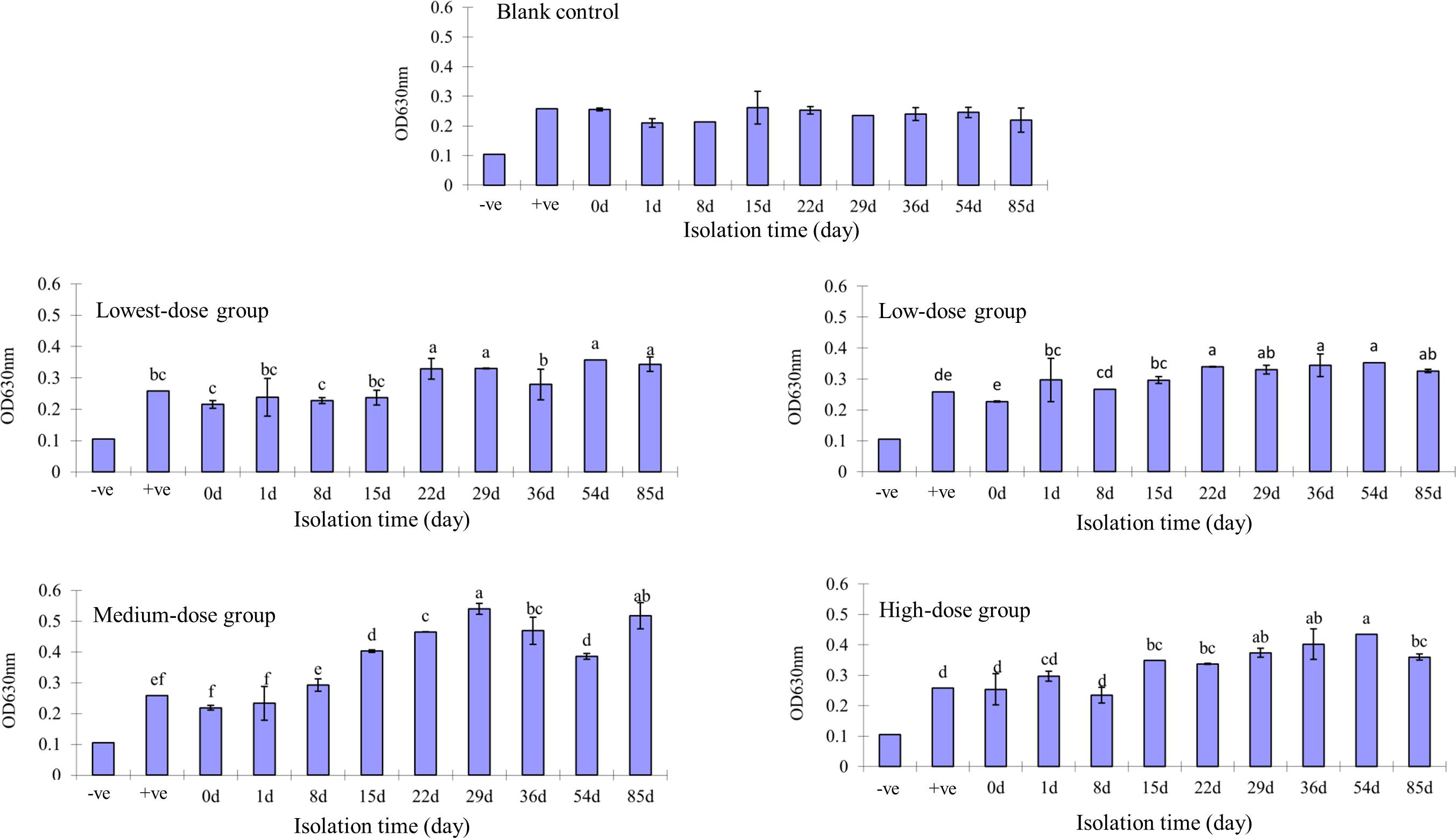
FIGURE 1. Escherichia coli biofilm developed by different-dose groups at different time points. Different lowercase letters indicate significant differences (P < 0.05); marked with the same lowercase letters indicate no significant difference (P > 0.05).
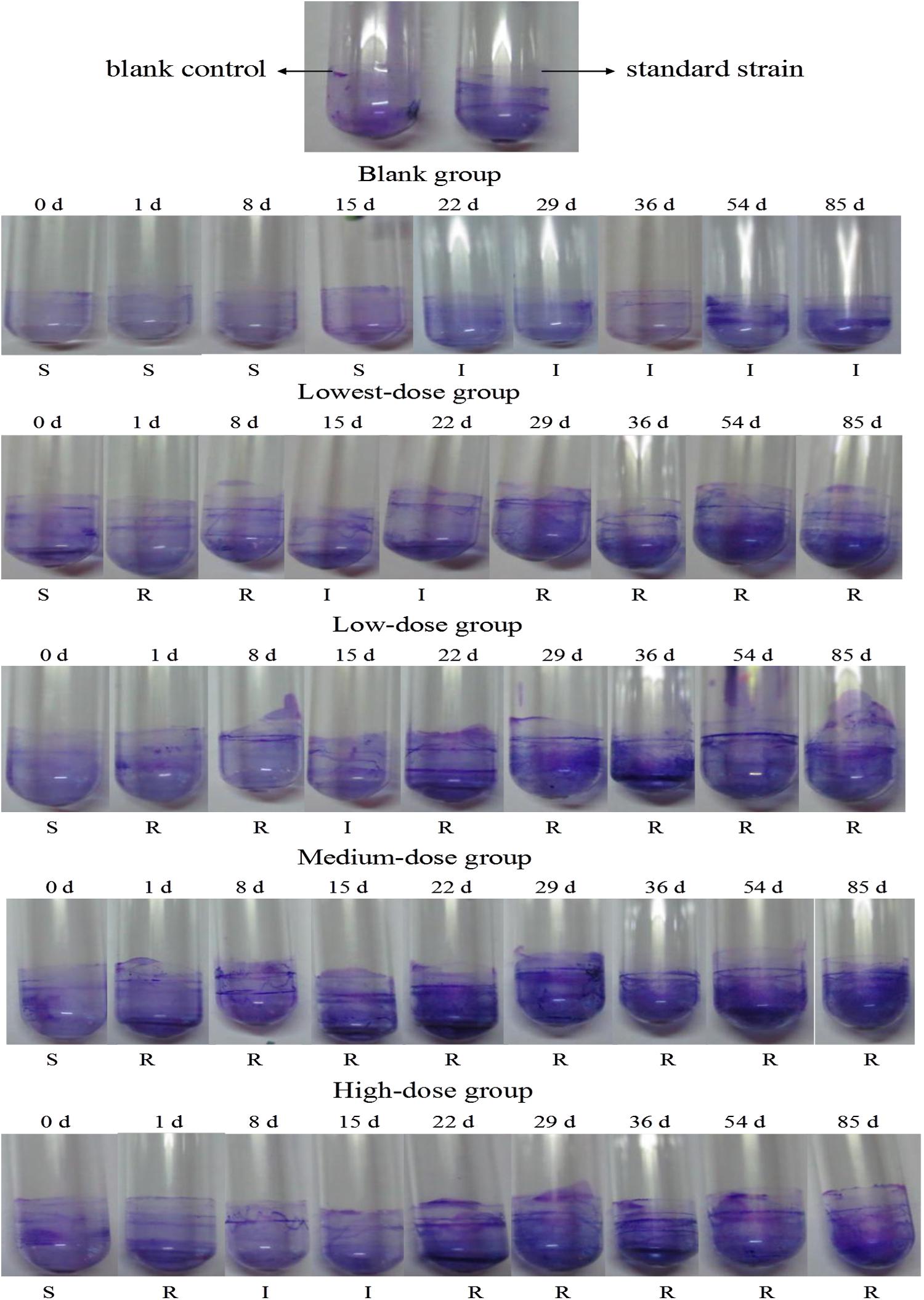
FIGURE 2. Escherichia coli biofilm developed by different-dose groups in test tubes. R, resistant; I, intermediate; S, sensitive.
Results of Real-Time PCR
There was no mqsR gene expression observed throughout the study (samples collected on day 0 as controls). Five genes (i.e., the self-inducible gene luxS, motif flhD, fliA, motA, and fimH) were not expressed in the control group.
The gene expression level and duration trends of flhD, fliA, motA, and fimH in different treatment groups were consistent. The order of duration and expression level from highest to lowest was: the medium-dose group, the high-dose group, the low-dose group, and the lowest-dose group. The expression levels of the genes peaked after 8 days in the medium-dose group and high-dose group, and reached minima at the 15th and the 22nd day, respectively. The expression levels of the genes in the low-dose group and lowest-dose group peaked after 15 days, and reached minima at the 29th and 36th day, respectively. In the lowest-, low-, and medium-dose groups, fliA had the highest expression level, followed by motA, fimH, and flhD. The overall expression levels of the four genes were similar in the high-dose group.
The luxS expression levels were similar in all groups except the high-dose group (Figure 3) and the hierarchy of luxS expression duration and level in the other three groups was the same as for the other genes tested. We found that luxS began to be expressed in the medium- and high-dose groups after 1 and 8 days, respectively; the expression level was significantly higher than that of the other four genes. Meanwhile, luxS was expressed on the 1st day in the lowest-dose group at a higher level than all of the other genes except fliA.
Discussion
Response of Drug Resistance to Environment
Thevenon et al. (2012) investigated multiple antibiotic resistance (MAR) of the fecal indicator bacteria (FIB) E. coli and Enterococcus spp. from sediments of Lake Geneva (Switzerland) over multiple decades. Their study showed that after 1970 sediments had deposited much higher levels of FIB with MAR than those before 1970. This is due to the fact that, after 1970, eutrophication greatly increased around Lake Geneva. Thus, the trophic state of the lake and municipal wastewater treatment plants had substantial effects on FIB and on the distribution of multidrug resistance genes in the sediments. In addition, certain areas of the bay constitute a reservoir of FIB and FIB with MAR in the contaminated sediments. Therefore, the suspension of FIB and FIB with MAR can impact health risks and water quality during recreational activities.
Our previous studies also found that FIB from microcosms quickly acquired resistance when subjected to different concentrations of cornmeal, glucose, or N and P (Yu et al., 2009, 2012). Here, the concentrations of N and P were lowered; however, they were still equivalent to a medium eutrophication level (the N and P levels in the control group were equivalent to a mesotrophic level). We have found that even small additions of any type of nutrient salt to a microcosm can alter the resistance characteristics of FIB (Ali et al., 2016; Zhang et al., 2016). The resistance mechanism(s) of FIB seem to be sensitive to environmental changes, and we believe that these resistance mechanisms play a key role in bacterial self-defense systems in changing conditions, and are even more important than antibiotics themselves (Rochelle-Newall et al., 2015). Thus, it would be of great interest to develop a new system capable of detecting the no observed effect concentration (NOEC) of glucose or N and P on the formation of resistance in FIB. Furthermore, these NOECs will be critical in evaluating the threat of FIB resistance in water sources.
Relationship Between Biofilm-Forming Genes and MAR
Real-time fluorescent quantitative PCR showed that E. coli mqsR was not expressed (Figure 3). Although studies have shown that mqsR can regulates the motility master regulon flhD and play an important role in biofilm development (González Barrios et al., 2006), our results show that the formation of E. coli biofilms was not related to mqsR expression following the addition of low levels of N and P.
Quantitative fluorescence PCR showed that expression levels of luxS, flhD, fliA, motA, and fimH, genes involved in biofilm formation, increased to a certain extent in each treatment group (Figure 3). This provides further confirmation that E. coli uses biofilm formation to adapt to changes in the external environment, in this case, in response to the addition of low levels of N and P.
Niu et al. (2013) proved that luxS influences E. coli biofilm development independently of autoinducer-2 and can assist in adaptation to varied conditions. In our study, the expression of luxS was induced rapidly in response to the addition of nutrient salts; it was detected in the medium- and high-concentration groups on day one. Pratt and Kolter (1998) used E. coli as a model organism to examine the initiation of biofilm development. Their study showed that motility is critical for normal biofilm development. Furthermore, it has been presented that type I pili (harboring the fimH gene) is required for initial surface adhesion (Pratt and Kolter, 1998). Niu et al. (2013) also showed that fimbriae may promote surface colonization and other aspects of initial biofilm development by E. coli.
Based on the role of pili and kinetic (or motility) genes in biofilm formation, as well as the results of this study, we infer that the lack of flhD, fliA, motA, and fimH expression suggests that the mature biofilm has begun to form. After completion of the adhesion and microcolony formation stages, in which the flagella and pili play an important role, the bacteria begin to gradually enter the mature biofilm stage (Berne et al., 2015). The shorter the duration of gene expression and maintenance of these four genes, the earlier E. coli enter the mature biofilm forming stage.
We showed that the expression of the four genes was maintained for the shortest time in the medium-dose group, followed by the high-dose group, the lowest-dose group, and the low-dose group. Thus, the medium-dose group was the earliest to form a mature biofilm; this agrees with previous analyses of biofilm formation abilities (Abberton et al., 2016). In addition, judging from the overall gene expression levels, gene expression is positively correlated over time, indicating that the medium-dose group was the best-adapted to the external environment. It formed a mature and stable biofilm in the shortest time and at the lowest gene expression levels, which ensured the survival of the bacteria in a changing environment.
The Expression Level of Biofilm Forming Genes and Their Relationship
The expression levels and trends of the three motor genes and pilus were consistent (Figure 3). The expression level of fliA was the highest, followed by motA, fimH, and flhD. In E. coli, flg, flh, and fli are the flagellum biosynthetic genes, and mot are motor genes (Chen and Helmann, 1992). The fimH adhesin is the tip protein of E. coli type I fimbriae (Lee et al., 2005). At the top of the hierarchy is the flhD master operon, which is consists of the flhD and flhC genes: expression of this regulon is essential for the expression of all of the remaining genes (Supplementary Figure S3). Class II operons consist of operons under direct control of the master operon. The genes contained in these operons encode a flagellum-specific sigma factor (σ28 or FliA) that is required for the transcription of class III operons, many structural components assembled in the early and middle stages of flagella synthesis, and some proteins of unknown function. Class IIIa regulons are under the dual control of fliA and flhD/flhC; meanwhile, class IIIb operons (such as motA) are under the control of fliA alone (Liu and Matsumura, 1994). Claret et al. (2007) showed that sigma factor FliA, transcriptional activator FlhD2C2, and flagella regulators are involved in the coordination of flagella and type I pilus synthesis. FliA is also a key regulatory component linking synthesis of the flagella and type I pili; its effect on type I pili is mediated via a c-di-GMP-dependent signaling pathway. Additionally, fliA, the key gene connecting flagella and pili, had the highest overall expression in our experiments, followed by motA, the motor gene (Belas, 2014). This is important since motility plays a critical role in surface contact and biofilm expansion during the initial stages of biofilm formation.
Author Contributions
XC, LA, JR, L-YW, CL, QH, and DY conceived and designed the study. XC and LA performed the experiments. XC, LA, CG, SB, and YR analyzed the data and wrote the paper. All authors contributed to the editing of the manuscript.
Funding
This work was supported by the National Natural Science Foundation of China (Grant No. 31272606) and grants from the State Key Development Program during the 13th Five-Year Plan period (Grant No. 2016YFD0501310) and the Research Center of Water Sources and Safety, Fujian Agriculture and Forestry University, Fujian Province, China.
Conflict of Interest Statement
The authors declare that the research was conducted in the absence of any commercial or financial relationships that could be construed as a potential conflict of interest.
Supplementary Material
The Supplementary Material for this article can be found online at: https://www.frontiersin.org/articles/10.3389/fmicb.2018.00367/full#supplementary-material
FIGURE S1 | (A) Electrophoresis result of six targeted genes “M” is DL500 marker; 1, 2, 3, 4, 5 and 6 is luxS, mqsR, flhD, fliA, motA, and fimH PCR products, respectively. (B) Melting peak of luxS, mqsR, flhD, fliA, motA, and fimH gene.
FIGURE S2 | Standard curve of (A) gapA, (B) luxS, (C) mqsR, (D) flhD, (E) fliA, (F) motA, and (G) fimH gene.
FIGURE S3 | The relationships between biofilm-forming genes are used with permission of (Liu and Matsumura, 1994).
References
Abberton, C. L., Bereschenko, L., van der Wielen, P. W. J. J., and Smith, C. J. (2016). Survival, biofilm formation, and growth potential of environmental and enteric Escherichia coli strains in drinking water microcosms. Appl. Environ. Microbiol. 82, 5320–5331. doi: 10.1128/AEM.01569-16
Ahmad, M., Liu, S., Mahmood, N., Mahmood, A., and Ali, M. (2017). Effects of porous carrier size on biofilm development, microbial distribution and nitrogen removal in microaerobic bioreactors. Bioresour. Technol. 234, 360–369. doi: 10.1016/j.biortech.2017.03.076
Ali, L., Wang, Y. Q., Zhang, J., Ajmal, M., Xiao, Z., Wu, J., et al. (2016). Nutrient-induced antibiotic resistance in Enterococcus faecalis in the eutrophic environment. J. Glob. Antimicrob. Resist. 7, 78–83. doi: 10.1016/j.jgar.2016.07.014
Belas, R. (2014). Biofilms, flagella, and mechanosensing of surfaces by bacteria. Trends Microbiol. 22, 517–527. doi: 10.1016/j.tim.2014.05.002
Berne, C., Ducret, A., Hardy, G. G., and Brun, Y. V. (2015). Adhesins involved in attachment to abiotic surfaces by Gram-negative bacteria. Microbiol. Spectr. 3, doi: 10.1128/microbiolspec.MB-0018-2015
Bjergbæk, L. A., Haagensen, J. A. J., Reisner, A., Molin, S., and Roslev, P. (2006). Effect of oxygen and growth medium on in vitro biofilm formation by Escherichia coli. Biofilms 3, 1–10. doi: 10.1017/S1479050507002074
Cerca, N., and Jefferson, K. K. (2008). Effect of growth conditions on poly-N-acetylglucosamine expression and biofilm formation in Escherichia coli. FEMS Microbiol. Lett. 283, 36–41. doi: 10.1111/j.1574-6968.2008.01142.x
Chen, Y. F., and Helmann, J. D. (1992). Restoration of motility to an Escherichia coli fliA flagellar mutant by a Bacillus subtilis sigma factor. Proc. Natl Acad. Sci. U.S.A. 89, 5123–5127. doi: 10.1073/pnas.89.11.5123
Claret, L., Miquel, S., Vieille, N., Ryjenkov, D. A., Gomelsky, M., and Darfeuille-Michaud, A. (2007). The flagellar sigma factor FliA regulates adhesion and invasion of Crohn disease-associated Escherichia coli via a cyclic dimeric GMP-dependent pathway. J. Biol. Chem. 282, 33275–33283. doi: 10.1074/jbc.M702800200
Clinical and Laboratory Standards Institute [CLSI] (2006). Performance Standards for Antimicrobial Susceptibility Testing; Sixteenth Informational Supplement Document M100-S16. Wayne, PA: CLSI.
Clinical and Laboratory Standards Institute [CLSI] (2015). Performance Standards for Antimicrobial Susceptibility Testing; Twenty-Fifth Informational Supplement Document M100-S25. Wayne, PA: CLSI.
Congrui, Z., Mingxu, Z., Jie, T., and Guoqiang, Z. (2013). Cloning and sequencing of housekeeping gene gapA from different originated Escherichia coli. China Poult. 35, 21–23. doi: 10.3969/j.issn.1004-6364.2013.17.007
Dang, H., and Lovell, C. R. (2016). Microbial surface colonization and biofilm development in marine environments. Microbiol. Mol. Biol. Rev. 80, 91–138. doi: 10.1128/MMBR.00037-15
Farkas, A., Craciunas, C., Chiriac, C., Szekeres, E., Coman, C., and Butiuc-Keul, A. (2016). Exploring the role of coliform bacteria in class 1 integron carriage and biofilm formation during drinking water treatment. Microb. Ecol. 72, 773–782. doi: 10.1007/s00248-016-0758-0
González Barrios, A. F., Zuo, R., Hashimoto, Y., Yang, L., Bentley, W. E., and Wood, T. K. (2006). Autoinducer 2 controls biofilm formation in Escherichia coli through a novel motility quorum-sensing regulator (MqsR, B3022). J. Bacteriol. 188, 305–316. doi: 10.1128/JB.188.1.305-316.2006
Ito, A., Taniuchi, A., May, T., Kawata, K., and Okabe, S. (2009). Increased antibiotic resistance of Escherichia coli in mature biofilms. Appl. Environ. Microbiol. 75, 4093–4100. doi: 10.1128/AEM.02949-08
Juhna, T., Birzniece, D., and Rubulis, J. (2007). Effect of phosphorus on survival of Escherichia coli in drinking water biofilms. Appl. Environ. Microbiol. 73, 3755–3758. doi: 10.1128/AEM.00313-07
Lee, J., Shin, S., Teng, C.-H., Hong, S. J., and Kim, K. S. (2005). FimH adhesin of Escherichia coli K1 type 1 fimbriae activates BV-2 microglia. Biochem. Biophys. Res. Commun. 334, 917–923. doi: 10.1016/j.bbrc.2005.06.180
Liu, X., and Matsumura, P. (1994). The FlhD/FlhC complex, a transcriptional activator of the Escherichia coli flagellar class II operons. J. Bacteriol. 176, 7345–7351. doi: 10.1128/jb.176.23.7345-7351.1994
Livak, K. J., and Schmittgen, T. D. (2001). Analysis of relative gene expression data using real-time quantitative PCR and the 2-ΔΔCT method. Methods 25, 402–408. doi: 10.1006/meth.2001.1262
Maeda, H., Fujimoto, C., Haruki, Y., Maeda, T., Kokeguchi, S., Petelin, M., et al. (2003). Quantitative real-time PCR using TaqMan and SYBR Green for Actinobacillus actinomycetemcomitans, Porphyromonas gingivalis, Prevotella intermedia, tetQ gene and total bacteria. FEMS Immunol. Med. Microbiol. 39, 81–86. doi: 10.1016/S0928-8244(03)00224-4
Moreira, J. M. R., Gomes, L. C., Araújo, J. D. P., Miranda, J. M., Simões, M., Melo, L. F., et al. (2013). The effect of glucose concentration and shaking conditions on Escherichia coli biofilm formation in microtiter plates. Chem. Eng. Sci. 94, 192–199. doi: 10.1016/j.ces.2013.02.045
Niu, C., Robbins, C. M., Pittman, K. J., Osborn, J. L., Stubblefield, B. A., Simmons, R. B., et al. (2013). LuxS influences Escherichia coli biofilm formation through autoinducer-2-dependent and autoinducer-2-independent modalities. FEMS Microbiol. Ecol. 83, 778–791. doi: 10.1111/1574-6941.12034
Pratt, L. A., and Kolter, R. (1998). Genetic analysis of Escherichia coli biofilm formation: roles of flagella, motility, chemotaxis and type I pili. Mol. Microbiol. 30, 285–293. doi: 10.1046/j.1365-2958.1998.01061.x
Rochelle-Newall, E., Nguyen, T. M. H., Le, T. P. Q., Sengtaheuanghoung, O., and Ribolzi, O. (2015). A short review of fecal indicator bacteria in tropical aquatic ecosystems: knowledge gaps and future directions. Front. Microbiol. 6:308. doi: 10.3389/fmicb.2015.00308
Romling, U., and Balsalobre, C. (2012). Biofilm infections, their resilience to therapy and innovative treatment strategies. J. Intern. Med. 272, 541–561. doi: 10.1111/joim.12004
Sanchez-Vizuete, P., Orgaz, B., Aymerich, S., Le Coq, D., and Briandet, R. (2015). Pathogens protection against the action of disinfectants in multispecies biofilms. Front. Microbiol. 6:705. doi: 10.3389/fmicb.2015.00705
Shrestha, S. R., and Afsar, A. (2017). Mutation in flrA and mshA genes of Vibrio cholerae inversely involved in vps-independent biofilm driving bacterium toward nutrients in lake water. Front. Microbiol. 8:1770. doi: 10.3389/fmicb.2017.01770
Soto, S. M. (2013). Role of efflux pumps in the antibiotic resistance of bacteria embedded in a biofilm. Virulence 4, 223–229. doi: 10.4161/viru.23724
Taub, F. B. (1997). Unique information contributed by multispecies systems: examples from the standardized aquatic microcosm. Ecol. Appl. 7, 1103–1110. doi: 10.1890/1051-0761(1997)007[1103:UICBMS]2.0.CO;2
Thevenon, F., Adatte, T., Wildi, W., and Pote, J. (2012). Antibiotic resistant bacteria/genes dissemination in lacustrine sediments highly increased following cultural eutrophication of Lake Geneva (Switzerland). Chemosphere 86, 468–476. doi: 10.1016/j.chemosphere.2011.09.048
Xiao, Z., Yu, D., Ali, L., Lin, J., Wang, Y., and Zhang, J. (2015). Glucose-induced resistance to ciprofloxacin and erythromycin in Enterococci. Enliven J. Anesthesiol. Crit. Care Med. 2, 24–29. doi: 10.1016/j.chemosphere.2012.04.014
Yu, D., Yi, X., Ma, Y., Yin, B., Zhuo, H., Li, J., et al. (2009). Effects of administration mode of antibiotics on antibiotic resistance of Enterococcus faecalis in aquatic ecosystems. Chemosphere 76, 915–920. doi: 10.1016/j.chemosphere.2009.04.057
Yu, D. J., Lai, B. S., Li, J., Ma, Y. F., Yang, F., Li, Z., et al. (2012). Cornmeal-induced resistance to ciprofloxacin and erythromycin in enterococci. Chemosphere 89, 70–75. doi: 10.1016/j.chemosphere.2012.04.014
Zhang, J., Wang, Y.-Q., Li, J., Ma, Y.-F., Lin, J., Xiao, Z.-R., et al. (2016). Phenotypic and genotypic analysis of vancomycin-resistant Enterococci strains isolated from different water sources. Afr. J. Bacteriol. Res. 8, 8–13.
Keywords: microcosm, nitrogen, phosphorus, Escherichia coli, antibiotic resistance, biofilm, biofilm-forming related genes, no observed effect concentration (NOEC)
Citation: Chen XP, Ali L, Wu L-Y, Liu C, Gang CX, Huang QF, Ruan JH, Bao SY, Rao YP and Yu D (2018) Biofilm Formation Plays a Role in the Formation of Multidrug-Resistant Escherichia coli Toward Nutrients in Microcosm Experiments. Front. Microbiol. 9:367. doi: 10.3389/fmicb.2018.00367
Received: 22 October 2017; Accepted: 16 February 2018;
Published: 02 March 2018.
Edited by:
Jean-Christophe Avarre, Institut de Recherche pour le Développement (IRD), FranceReviewed by:
Josée Harel, Université de Montréal, CanadaFohad Mabood Husain, King Saud University, Saudi Arabia
Copyright © 2018 Chen, Ali, Wu, Liu, Gang, Huang, Ruan, Bao, Rao and Yu. This is an open-access article distributed under the terms of the Creative Commons Attribution License (CC BY). The use, distribution or reproduction in other forums is permitted, provided the original author(s) and the copyright owner are credited and that the original publication in this journal is cited, in accordance with accepted academic practice. No use, distribution or reproduction is permitted which does not comply with these terms.
*Correspondence: DaoJin Yu, eXVkYW9qaW5AeWVhaC5uZXQ=
†These authors have contributed equally to this work.