- 1Department of Plant and Environmental Sciences, University of Copenhagen, Frederiksberg, Denmark
- 2Pcovery ApS, Copenhagen, Denmark
- 3Medical Research Council Centre for Medical Mycology, University of Aberdeen, Aberdeen Fungal Group, Aberdeen, United Kingdom
Zinc is an essential micronutrient, required for a range of zinc-dependent enzymes and transcription factors. In mammalian cells, zinc serves as a second messenger molecule. However, a role for zinc in signaling has not yet been established in the fungal kingdom. Here, we used the intracellular zinc reporter, zinbo-5, which allowed visualization of zinc in the endoplasmic reticulum and other components of the internal membrane system in Candida albicans. We provide evidence for a link between cyclic AMP/PKA- and zinc-signaling in this major human fungal pathogen. Glucose stimulation, which triggers a cyclic AMP spike in this fungus resulted in rapid intracellular zinc mobilization and this “zinc flux” could be stimulated with phosphodiesterase inhibitors and blocked via inhibition of adenylate cyclase or PKA. A similar mobilization of intracellular zinc was generated by stimulation of cells with extracellular zinc and this effect could be reversed with the chelator EDTA. However, zinc-induced zinc flux was found to be cyclic AMP independent. In summary, we show that activation of the cyclic AMP/PKA pathway triggers intracellular zinc mobilization in a fungus. To our knowledge, this is the first described link between cyclic AMP signaling and zinc homeostasis in a human fungal pathogen.
Introduction
Zinc is an essential micronutrient for all living organisms and maintaining precise zinc homeostasis is vital for cell survival. Indeed, in eukaryotes, 9% of the proteome requires zinc (Andreini et al., 2009). Zinc proteins can be categorized in four groups: enzymes (47%), transcription factors (44%), storage and transport (5%), and cell signaling (3%). Zinc is used as a co-factor or structural component in more than 300 enzymes within all 6 classes (Bozym et al., 2006).
Candida albicans is an opportunistic fungal pathogen and a major cause of invasive fungal infections, where mortality can reach 40% (Kullberg and Arendrup, 2015), and of vulvovaginal candidiasis, which affects 75% of women of childbearing age (Brown et al., 2012).
Metals, such as zinc, play important roles in the control of microbial infections. Depending on the host niche, the immune system can attempt to restrict microbial access to zinc, or to expose them to potentially toxic levels. Collectively these processes are known as “nutritional immunity” (Hood and Skaar, 2012). The mammalian protein calprotectin is used to decorate neutrophil extracellular traps where it elicits anti-Candida activity via zinc chelation (Urban et al., 2009). On the other hand, C. albicans can produce its own secreted zinc-binding protein Pra1 in order to scavenge this metal from host tissue (Citiulo et al., 2012).
In mammalian cells zinc is emerging as an important intracellular second messenger (Yamasaki et al., 2007). Zinc signals can be classified as either early, which occur within minutes, or late signals, which are transcription dependent (Fukada et al., 2011). The early signals are dependent on release of zinc from proteins or via transporter-mediated release from intracellular organelles. Eukaryotes, including both fungi and metazoans have two known families of zinc-transporters. The Zrt/Irt-like protein (ZIP) family imports zinc from outside of the cell or from within organelles into the cytoplasm. Although the first ZIP structure has recently been reported (Zhang et al., 2017), the precise transport mechanism remains unclear (Eide, 2006). In contrast, members of the cation diffusion facilitator (CDF) family can transport zinc from the cytosol, either sequestering it within organelles or exporting to the extracellular environment. These CDF or ZnT transporters functions as H+ or K+ antiporters (Eide, 2006). In mammalian cells, a phenomenon called the zinc wave has been described whereby activation of the FcεRI receptor leads to zinc release from the endoplasmic reticulum (ER) (Yamasaki et al., 2007). This ER export is mediated by the zinc transporter ZIP7, which is activated via phosphorylation by Protein Kinase 2 (CK2) (Taylor et al., 2012).
A role for zinc has been identified in several signaling cascades, such as in gastrula cells in zebra fish where STAT3 activation leads to activation of ZIP6, which increases the cytoplasmic zinc concentration. This leads to activation of the zinc finger transcription factor Snail, which regulates cell movement (Yamashita et al., 2004; Fukada et al., 2011). Additionally, in mast cells, Protein Kinase C (PKC) is regulated by zinc that is imported to the Golgi by the zinc transporter ZnT5 (Nishida et al., 2009; Fukada et al., 2011) and this PKC activation then leads to NF-κB mediated cytokine production.
Although zinc has not yet been described as an intracellular signaling molecule within the fungal kingdom, an ER zinc pool has been reported in several fungal species. In Saccharomyces cerevisiae two transporters belonging to the CDF family, Msc2 and Zrg17, have been shown to localize to the ER, where they function as heterodimers (Li and Kaplan, 2001; Ellis et al., 2005). Both Msc2 and zinc have been shown to be required for normal ER function, as low zinc or deletion of MSC2 leads to the unfolded protein response (UPR), which is a hallmark of ER stress (Ellis et al., 2004). Deletion of the CDF family zinc transporter ZHF located to the ER in Schizosaccharomyces pombe leads to hypersensitivity to zinc and cobalt (Clemens et al., 2002).
Fluorescent probes have frequently been used to assess intracellular pools of zinc (Lim et al., 2004; Dean et al., 2012). Different probes can be used to study zinc in different intracellular compartments. The zinc probe zinquin has revealed the presence of so-called zincosomes in S. cerevisiae (Devirgiliis et al., 2004). These are vesicular-like bodies containing zinc, but their function remains unclear (Eide, 2006). Zinquin is able to interact with ligand bound zinc and is therefore most likely reporting on zinc bound to various zincosomal proteins (Wellenreuther et al., 2009). Vacuolar zinc in S. cerevisiae has been detected using the zinc probe FuraZin-1 (MacDiarmid et al., 2003). Zinbo-5, which is used in this study, has also previously been used to track changes in labile (free) zinc in yeast in response to compounds which alter zinc homeostasis (Simm et al., 2011). The localization of zinbo-5 in this previous study, however, was not examined.
Here we observed that zinbo-5 in C. albicans reports on non-vacuolar zinc, including the ER pool. Using this probe we have identified rapid and dynamic changes in intracellular zinc in response to stimulation with extracellular zinc as well as cyclic AMP/PKA pathway activation.
Materials and Methods
Strains and Growth Conditions
The laboratory C. albicans strain BWP17 + CIp30 (Mayer et al., 2012) was used for all experiments and maintained on YPD agar (1% yeast extract, 2% peptone, 2% glucose, 2% agar). For experiments, cells were grown overnight in liquid YPD or in SD medium [0.171% yeast nitrogen base (Sunrise Science Products), 2% glucose and 0.5% NH4SO4] as indicated.
Microscopy
Cells from an overnight culture in YPD-media were washed in PBS buffer and diluted to an OD600 of 0.01. One hundred and fifty microliters cell plus 150 μL of 2× RPMI-1640 media (R6504, Sigma) was transferred to wells of an 8-well slide (80826, Ibidi). The cells were grown for 3.5–4.5 h at 37°C before washing three times in PBS buffer.
Cell staining was performed in 200 μL PBS within the 8-well slide. For zinbo-5 (sc-222425, Santa Cruz), 5 μM for 15 min; DiOC6(3) (D273, Thermo Fisher), 2 μM for 3 min; Syto13 (S7575, Thermo Fisher) 2 μM for 5 min; DAPI (D1306, Thermo Fisher) 5 μM for 10 min. For Syto13, cells were first incubated overnight in PBS at 37°C prior to staining. After staining, cells were washed in PBS. For pulse-chase staining of the fungal vacuole, cells were incubated in RPMI for 2 h, incubated with 5 μM FM4-64 in RPMI for 2 h (pulse), washed with PBS, incubated for a further 1 h in RPMI in the absence of dye (chase), washed and imaged. An inverted confocal laser microscope (Leica SP5, Leica Microsystems) equipped with a 63× water-immersion objective was used for obtaining fluorescent images of the stained cells. The following laser settings was used: zinbo-5 and DAPI, 405 nm excitation, 415–470 nm detection; DiOC6(3), 488 nm excitation, 495–620 nm detection; FM4-64, 513 nm excitation, 650–750 nm detection; Syto13, 488 nm excitation, 495–510 nm detection. The pictures were analyzed in the open source program ImageJ.
Fluorescence Kinetics
Cells were grown overnight in SD media containing 10 μM ZnSO4, unless stated otherwise. The cells were washed in PBS buffer (D8537, Sigma) three times by centrifugation at 2000 g for 2 min and re-suspended to an OD600 of 1.8. One hundred microliters cells plus 50 μl of 20 μM zinbo-5 was mixed in a 96 well black plate and fluorescence measured on a plate reader (SpectraMax M2, Molecular Devices). Measurements was performed every 1 min with excitation at 405 nm, emission at 443 nm and emission filter at 420 nm. Twenty-five microliter zinc and EDTA were added to the final concentrations indicated. A four-parameter variable slope equation was performed in GraphPad Prism 6 to calculate the EC50 in the zinc titration experiment. Experiments with chemical inhibitors/activators of zinc fluxes were performed in 0.5 M MOPS-tris buffer pH 7.0 containing 5 μM EDTA to prevent changes in pH. The following compounds were used: 25 μl of dithiothreitol (DTT, D0632, Sigma), theophylline (T1633, Sigma), caffeine (C8960, Sigma), dibutyryl cAMP (D0260, Sigma), MDL12330 (sc-3537, Santa Cruz,) or myristoylated PKI (14–22) (myr-PKI, sc-471154, Santa Cruz,). Fifty micromolar clioquinol (33931, Sigma) was used as control for minimum zinbo-5 fluorescence.
For determination of metal selectivity, the following metals were added to a final concentration of 23 μM: ZnSO4, CuSO4, NiCl2, CoCl2, FeSO4, CdCl2, MgSO4, CaCl2.
P-values were calculated in one- or two-way analysis of variance (ANOVA) followed by Tukey (one-way) or Dunnett (two-way) post hoc testing in GraphPad Prism 6.
Zinc Uptake Assay
Zinc uptake was measured either following overnight incubation in SD + 10 μM Zn2+ media (∼18 h) or after addition of a zinc pulse to cells washed and re-suspended in PBS buffer to OD600 of 1.0. For the zinc pulse, 5 μM ZnSO4 was added for 20 min at room temperature. Cells were pelleted and 25 μl supernatant transferred to 75 μl of 0.5 M MOPS-tris buffer pH 7.0 containing 0.2 M KCl and 0.66 μM cell impermeant FluoZin-3 (F24194, Thermo Fisher). The fluorescence was measured on a plate reader (SpectraMax M2, Molecular Devices) with excitation at 485 nm and emission at 525 nm. A standard curve of ZnSO4 was prepared in PBS and included with every experiment. The amount of zinc in the supernatant was found to fit a second order polynomial equation in Excel (Microsoft) from the ZnSO4 standard curves of the FluoZin-3 signal.
Results
Zinbo-5 Localizes to the Internal Membrane System Including the ER in C. albicans
The cellular localization of the zinc probe zinbo-5 was first determined using fluorescence microscopy. Co-staining of C. albicans with the vacuolar membrane stain FM4-64 demonstrated that zinbo-5 is excluded from the vacuole (Figure 1A). The fungal vacuole can also be observed as an apparent indentation by differential interference contrast (DIC) (Figure 1A). Instead, zinbo-5 appeared to localize to non-vacuolar intracellular membrane structures. Figure 1B shows co-localization of zinbo-5 with DiOC6(3). At the concentrations used here, DiOC6(3) has been reported to specifically stain the nuclear envelope and ER in S. cerevisiae (Koning et al., 1993).
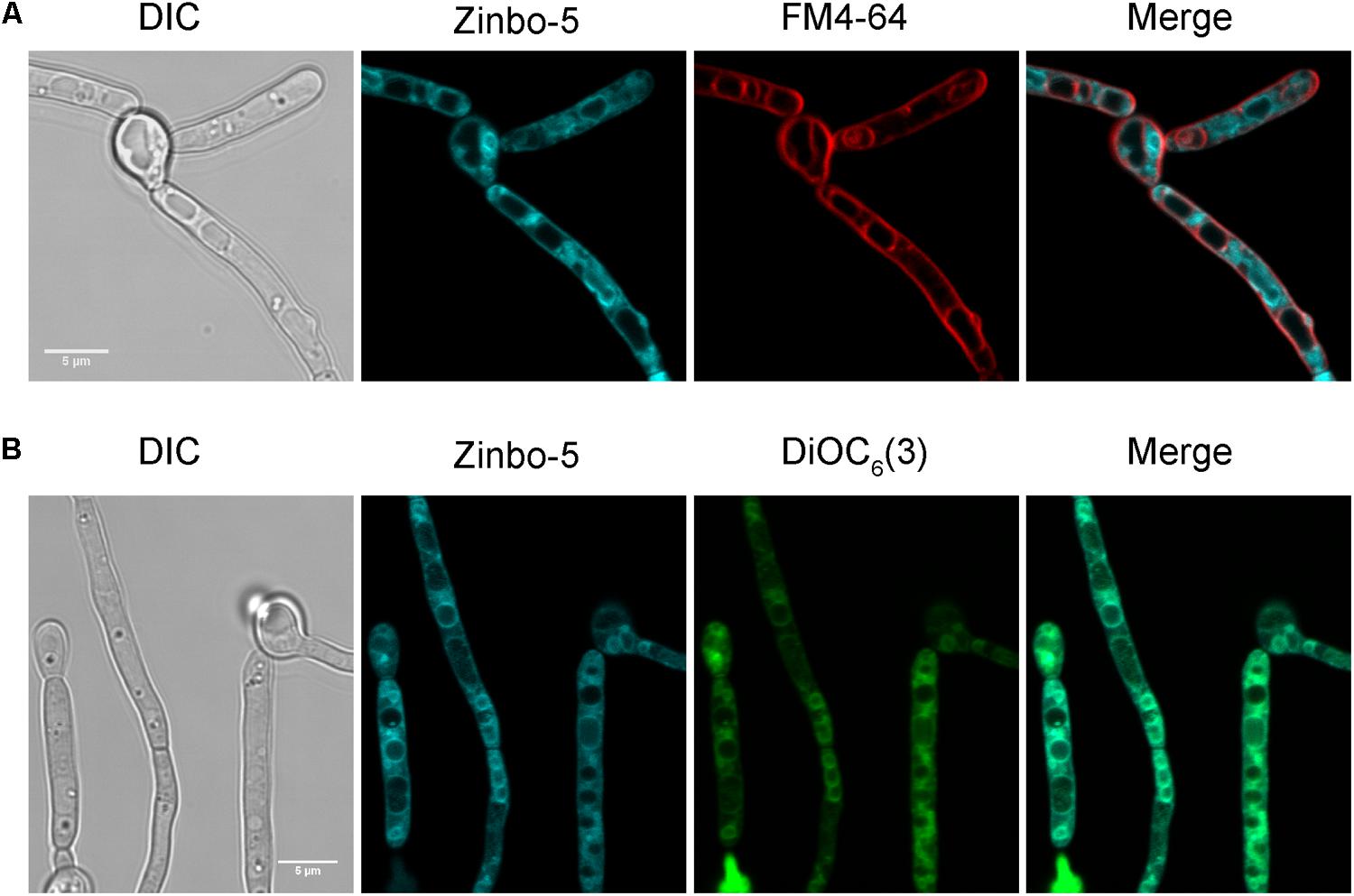
FIGURE 1. Zinbo-5 is excluded from the vacuole and localizes to the internal membrane system in Candida albicans. (A) Cells stained with zinbo-5 and FM4-64, which, under these conditions stains the plasma membrane and vacuolar membrane. (B) Co-localization of zinbo-5 and DiOC6(3) (internal membranes).
We therefore investigated whether zinbo-5 associates with the nucleus in C. albicans using the nuclear dyes Syto13 and DAPI (Figures 2A,B). In order to stain the fungal nucleus with Syto13, cells were first incubated overnight in PBS. Interestingly, this incubation period altered zinbo-5 localization, possibly due to changes in the cells’ internal membrane system or redistribution of the intracellular zinc pool during this extensive period in buffer. Under these conditions, zinbo-5 fluorescence localized as a ring surrounding the nucleus, likely to be the perinuclear ER (Figure 2A), as did DiOC6(3) (Figure 2B). Therefore, in C. albicans zinbo-5 appears to stain the internal membrane system, including the perinuclear ER and most likely also other parts of the ER.
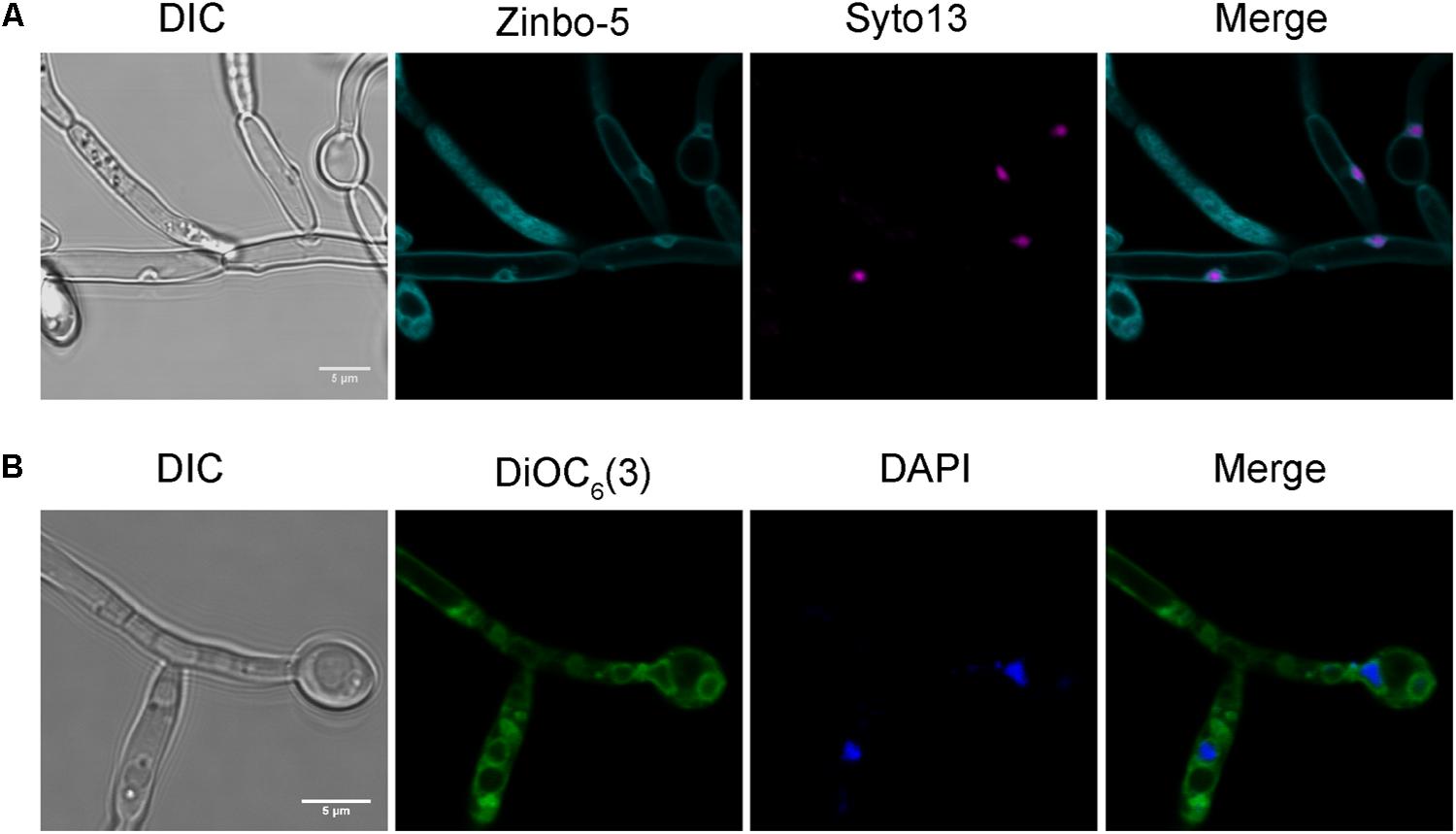
FIGURE 2. Zinbo-5 and DiOC6(3) localizes to the perinuclear endoplasmic reticulum. (A) Cells stained with zinbo-5 and the nuclear stain Syto13. Cells were first incubated in PBS buffer overnight at 37°C prior to staining with this dye. This incubation period also changed the zinbo-5 staining pattern to the plasma membrane and the nucleus. (B) Cells stained with DiOC6(3) and the nuclear stain DAPI.
Intracellular Zinc Is Highly Dynamic and Responds to Extracellular Zinc
In mammalian cells, it has been demonstrated that stimulation with extracellular zinc results in the release of zinc from intracellular stores. Using zinbo-5 as a reporter, we tested whether a similar phenomenon occurs in fungi. C. albicans cells were pre-grown in minimal medium (SD) supplemented with 0, 1, 10, or 100 μM Zn2+. Figure 3 shows that the initial zinbo-5 signal intensified with increased zinc in the culture medium. Cells were then stimulated with 5.7 μM exogenous Zn2+ for 20 min. Interestingly, cells pre-cultured in the presence of (≥10 μM) Zn2+ exhibited a rapid loss of zinbo-5 fluorescence (Figure 3). Extracellular Zn2+ was then sequestered via addition of the chelator EDTA for 15 min. This treatment resulted in an increase in zinbo-5 signal for all samples. Cells that were not stimulated with Zn2+ did not respond to EDTA treatment (Figure 3). These data suggest that the zinbo-5 cellular zinc pool is responsive to extracellular zinc levels. The regenerated zinc pool created by EDTA treatment remained responsive to a second zinc challenge (Supplementary Figure S1).
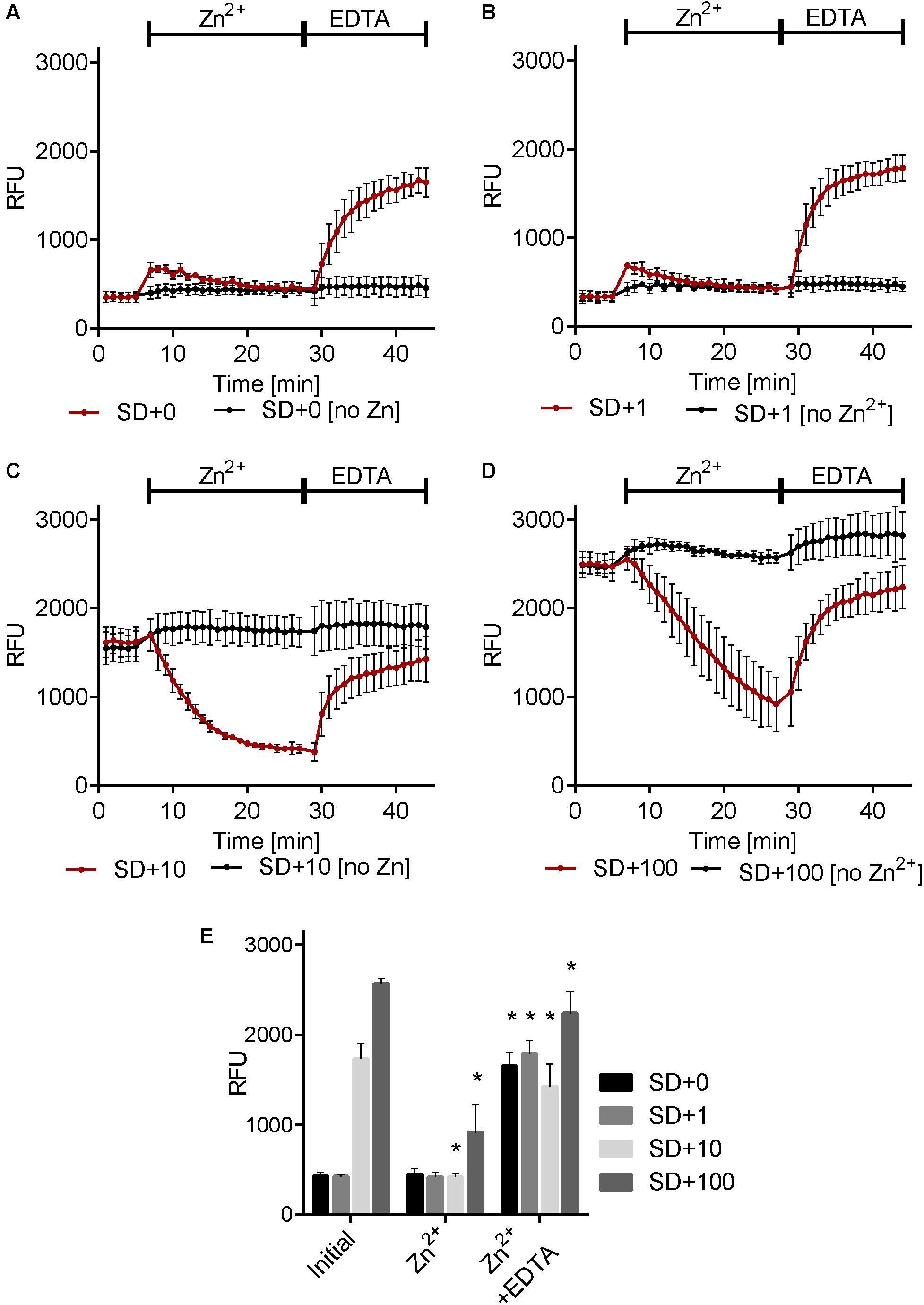
FIGURE 3. Environmental Zn2+ induces dynamic changes in the cellular zinc pool. Cells were grown in SD media containing 0 μM Zn2+ [SD+0 (A)], 1 μM Zn2+ [SD+1 (B)], 10 μM Zn2+ [SD+10 (C)] or 100 μM Zn2+ [SD+100 (D)]. Zinbo-5 was added and the zinbo-5 fluorescence determined following 10 min of incubation with probe (initial), followed by 20 min incubation with 5.7 μM zinc (Zn2+) and following a subsequent 15 min incubation with 50 μM EDTA (Zn2+ + EDTA). (E) Shows a composite of single measurements of the different conditions from A–D at the beginning of the experiment (initial), and following 20 min zinc treatment (Zn2+), followed by 15 EDTA treatment (Zn2+ + EDTA). Error bars indicate standard deviation for n = 3. “∗” indicate p < 0.01 in two-way ANOVA test for the treatment compared to before treatment (‘Zn2+’ compared to ‘initial’ and ‘Zn2+ + EDTA’ compared to ‘Zn2+’).
As shown in Figure 3 cells pre-cultured in the presence of zinc store this cation internally, whilst cells pre-cultured in the presence of low zinc exhibit low initial zinbo-5 fluorescence following overnight culture. Therefore we next assessed zinc assimilation by C. albicans. Table 1 shows that, following 18 h culture in the presence of 10 μM Zn2+, C. albicans take up half of the zinc from the culture medium. However, when these Zn2+-rich cells were subsequently pulsed with 5 μM Zn2+ for 20 min, they assimilated only 0.2 μM (±0.3 μM) Zn2+. In contrast, cells pre-cultured under lower Zn2+ conditions (SD + 1 μM Zn2+) assimilated more than half (2.7 μM) of exogenous Zn2+ during the 20 min pulse. These assimilation data indicate that cells from a zinc-rich preculture predominantly mobilize intracellular zinc reserves, as very little if any extracellular zinc is taken up during the treatment period. On the other hand, it would appear that cells from a zinc-poor preculture assimilate zinc during the 20 min treatment period, and that this freshly acquired zinc pool is subsequently mobilized to the ER following EDTA treatment.
In order to test the specificity of this response, cells from an SD + 1 μM Zn2+ culture were pre-treated with Zn2+, Cu2+, Ni2+, Co2+, Fe2+, Cd2+, Mg2+, or Ca2+, and then subsequently challenged with EDTA. Supplementary Figure S2 shows that only Zn2+ pretreatment resulted in a subsequent EDTA-induced increase in zinbo-5 signal. Therefore, of the divalent cations tested, our observed intracellular zinc fluxes are specific to Zn2+.
The above data show that exogenous Zn2+ and EDTA can, respectively, stimulate the release and reentry of Zn2+ from and to the zinbo-5 compartment. It is possible that these events may be analogous to the stimulated release of ER zinc observed in mammalian cells (Taylor et al., 2012). Therefore the effect of ER stress on zinc release was tested. Cells were treated with the ER stress inducer DTT for 20 min. This treatment itself decreased zinbo-5 fluorescence by 27%, compared to 10% for the control (Figure 4). Subsequent addition of Zn2+ to the DTT-treated cells only decreased the zinbo-5 fluorescence to 50% of the initial signal, while control cells decreased by 78%. These data show that ER perturbation impacts the intracellular Zn2+ pool and supports our microscopy observations that zinbo-5 reports on ER-associated zinc (Figures 1, 2).
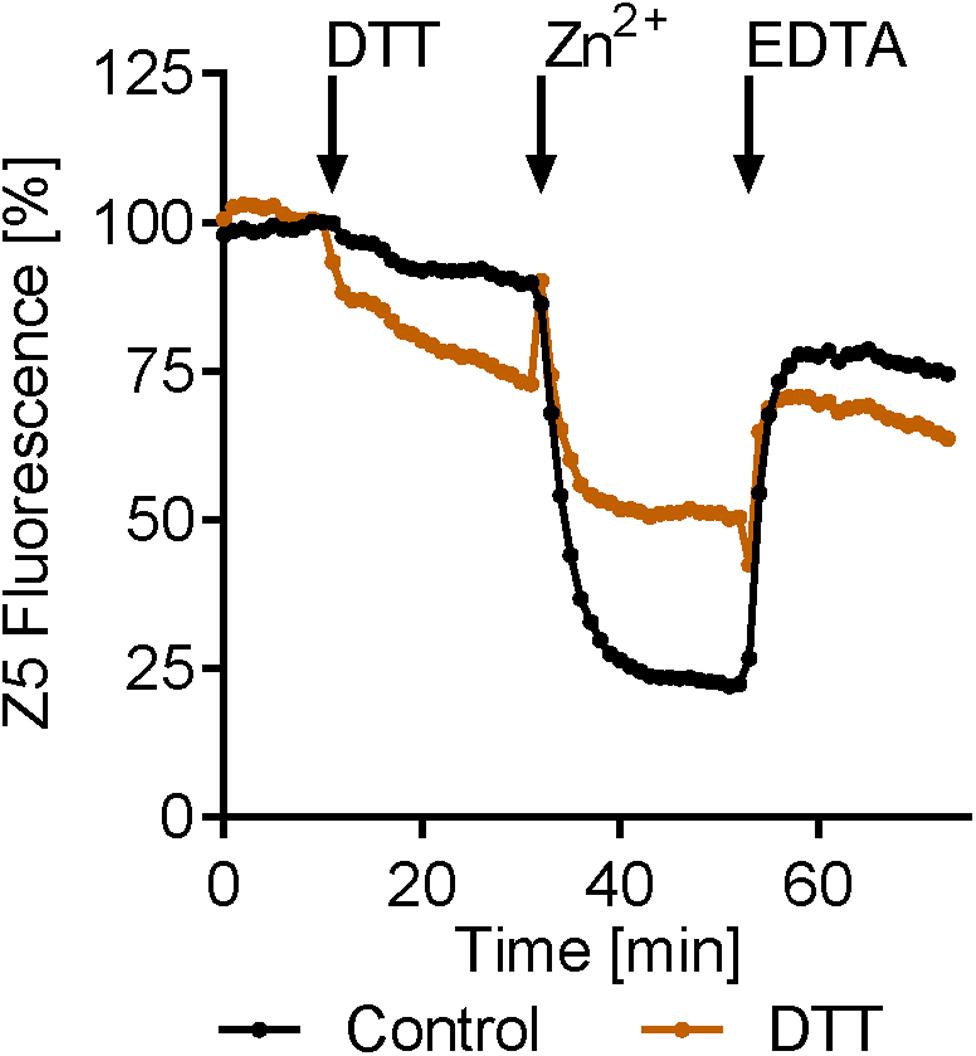
FIGURE 4. Endoplasmic reticulum (ER) stress modifies intracellular zinc release. Zinbo-5 (Z5) fluorescence was measured in cells pre-cultured in SD media containing 10 μM Zn2+. DTT was added for 20 min (stimuli) before 20 μM zinc was added for 20 min (Zn2+). Finally EDTA (100 μM) was added for 20 min n = 3.
As ER-released zinc serves as an intracellular second messenger in mammalian cells (Yamasaki et al., 2007), we next questioned the robustness of the response in fungal cells. Cells were repeatedly challenged with increasing concentrations of Zn2+ and EDTA. Figure 5A shows that the zinbo-5 zinc pool remained responsive to three sequential treatments. As C. albicans is both a human commensal and pathogen, we next sought to determine whether or not the observed intracellular zinc fluxes were physiologically relevant. To determine how much extracellular zinc is required to initiate intracellular zinc release, a dilution series of Zn2+ was applied to cells pre-grown in SD + 100 μM Zn2+ media. The rate of zinbo-5 decline within the first 10 min was then calculated and the EC50 value was found to be 0.53 μM (±0.19 μM) (Figure 5B). Therefore, C. albicans intracellular zinc release is responsive to physiologically relevant concentrations of this cation, which have been reported to be 12–18 μM in plasma (Strachan, 2010).
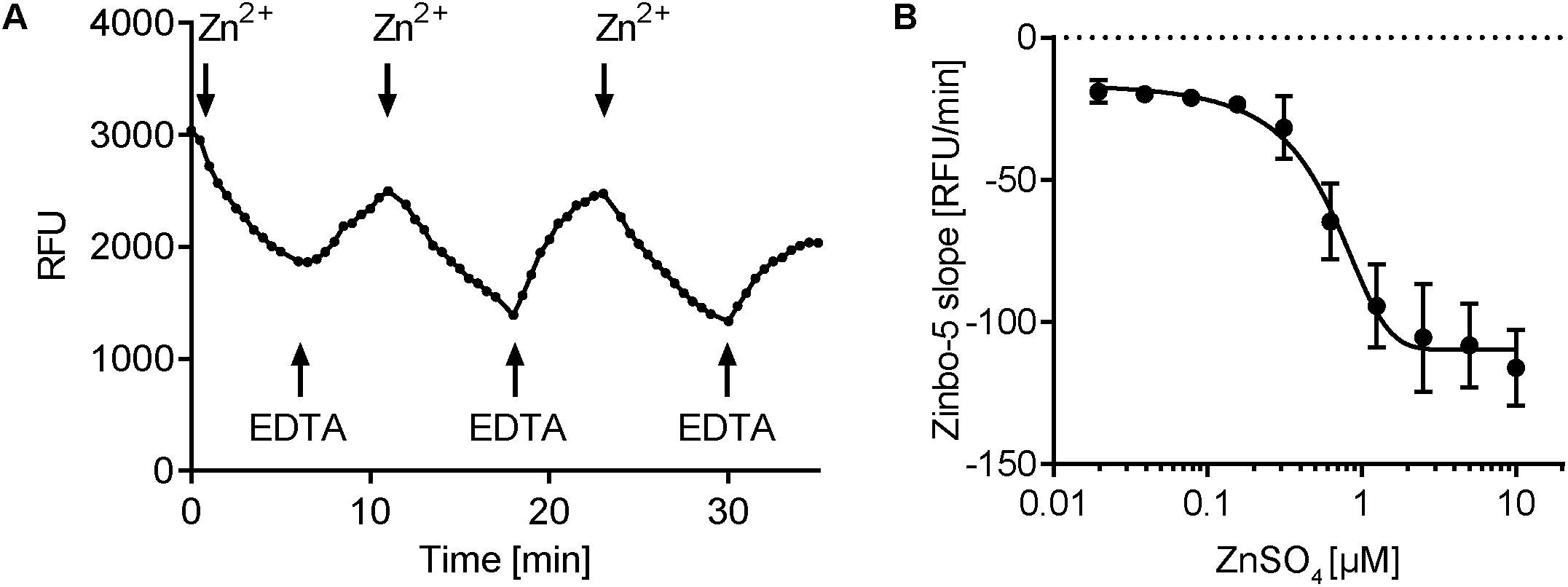
FIGURE 5. Zinbo-5 fluorescence changes upon extracellular zinc addition and chelation. (A) zinbo-5 fluorescence within cells in response to addition of Zn2+ and EDTA. Addition at the indicated arrows in the following order: 5 μM Zn2+, 10 μM EDTA, 20 μM Zn2+, 40 μM EDTA, 80 μM Zn2+, and 160 μM EDTA. (B) Titration of extracellular addition of ZnSO4. The slope was calculated in the first 10 min after zinc addition. Error bars indicate standard deviation. The EC50 value was calculated to 0.53 ± 0.19 μM ZnSO4 (n = 3).
Cyclic AMP Pathway Activation Induces Intracellular Zinc Mobilization
Our data suggest that, analogous to mammalian cells, the fungus C. albicans also triggers rapid intracellular zinc flux in response to extracellular zinc. We next questioned whether other environmental signals could also trigger intracellular zinc release. The cyclic AMP/protein kinase A (cAMP/PKA) pathway integrates multiple nutrient signals; moreover, we have previously shown that C. albicans rapidly activates that cAMP pathway in response to glucose, and that this cAMP spike is negatively regulated by the phosphodiesterase Pde1 (Wilson et al., 2010). We therefore pre-cultured C. albicans in SD + 10 μM Zn2+ to generate the intracellular zinc pool and then stimulated the cells with glucose. Figure 6 shows that glucose stimulation also resulted in a rapid decrease in zinbo-5 fluorescence. In contrast, galactose stimulation had no effect. To test whether this effect was cAMP/PKA dependent, we simultaneously treated the cells with the cAMP agonist glucose and the broad spectrum phosphodiesterase (PDEase) inhibitors theophylline or caffeine. These combinations of cAMP/PKA pathway activation resulted in a much stronger decrease in zinbo-5 fluorescence. Finally, the cell permeable cAMP analog dibutyryl cAMP also triggered zinc release at levels comparable to PDEase inhibition (Figure 6B). These data indicate that cAMP/PKA pathway activation triggers intracellular zinc release in C. albicans.
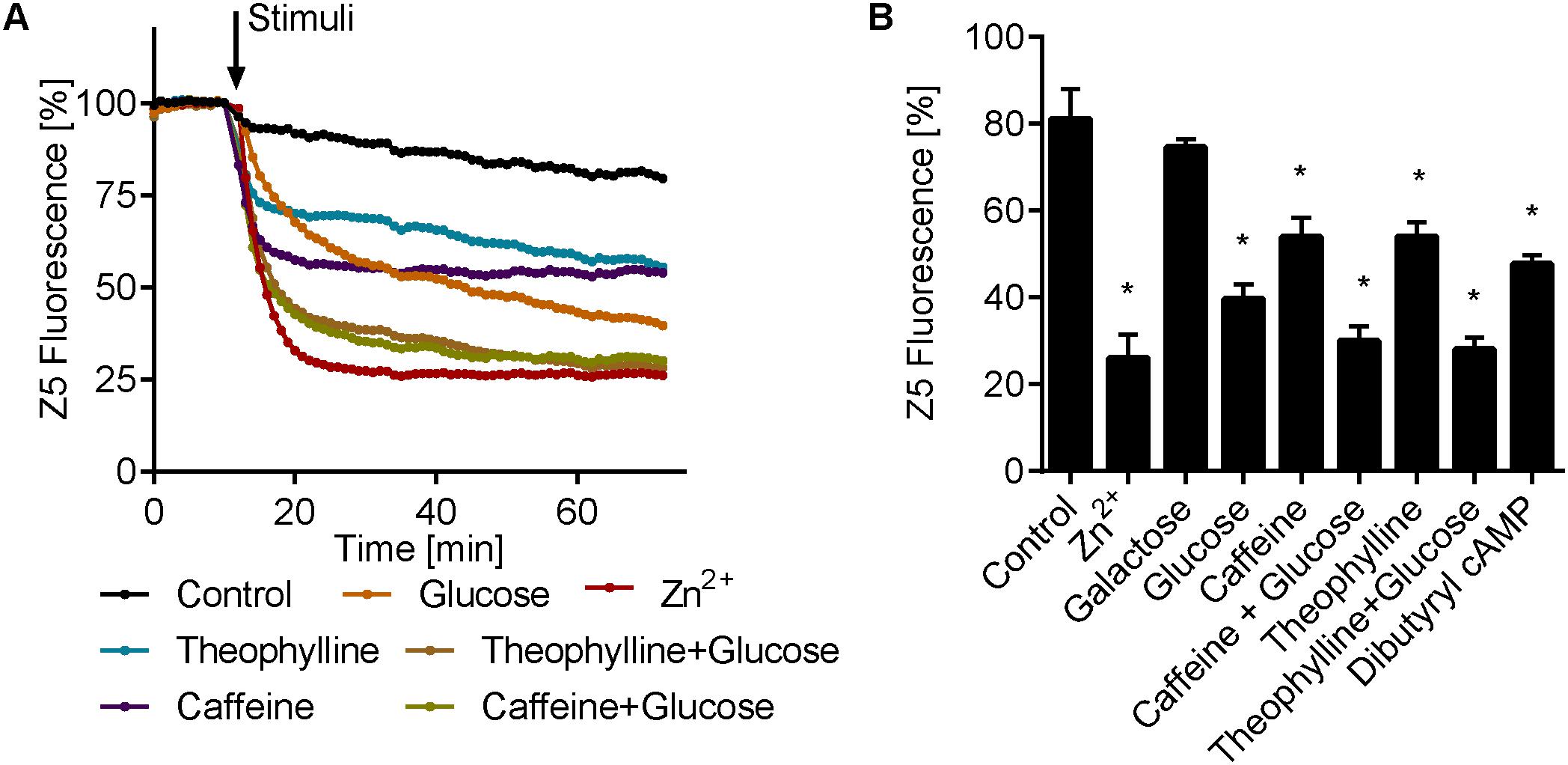
FIGURE 6. cAMP activation induce intracellular zinc release. (A) Time course of zinbo-5 (Z5) fluorescence following addition of cAMP pathway activators to cells grown in SD+10 media. (B) Same data as A showing the final zinbo-5 fluorescence after 1 h incubation with stimuli. Error bars represent standard deviation for n = 3–5. “∗” indicate p < 0.01 in one-way ANOVA compared to control. The following concentrations were used: 25 μM Zn2+, 0.25% glucose and galactose, 10 mM caffeine, 9.5 mM theophylline and 10 mM dibuturyl cAMP.
Inhibition of the cAMP Pathway Inhibits Glucose-Induced Intracellular Zinc Release
Next we tested the effect of cAMP/PKA pathway inhibition on intracellular zinc release. MDL12330 and myr-PKI inhibit the adenylate cyclase (AC) and PKA, respectively (Castilla et al., 1998; Jain et al., 2003). Both compounds resulted in a modest increase in zinbo-5 signal, in line with PKA activity negatively regulating the intracellular zinc pool (Figures 7A,B). Interestingly, cAMP/PKA inhibition fully blocked glucose- (Figure 7A), but not Zn2+-induced (Figure 7B) zinc release. However, treatment with myr-PKI moderately inhibited Zn2+ release upon treatment with extracellular Zn2+ and both myr-PKI and MDL12330 enhanced subsequent EDTA-induced Zn2+ reentry to the zinbo-5 compartment (Figure 7B). These results indicate that glucose activates zinc release through cAMP signaling, while Zn2+ stimulation can at least partially bypass the cyclic AMP/PKA pathway.
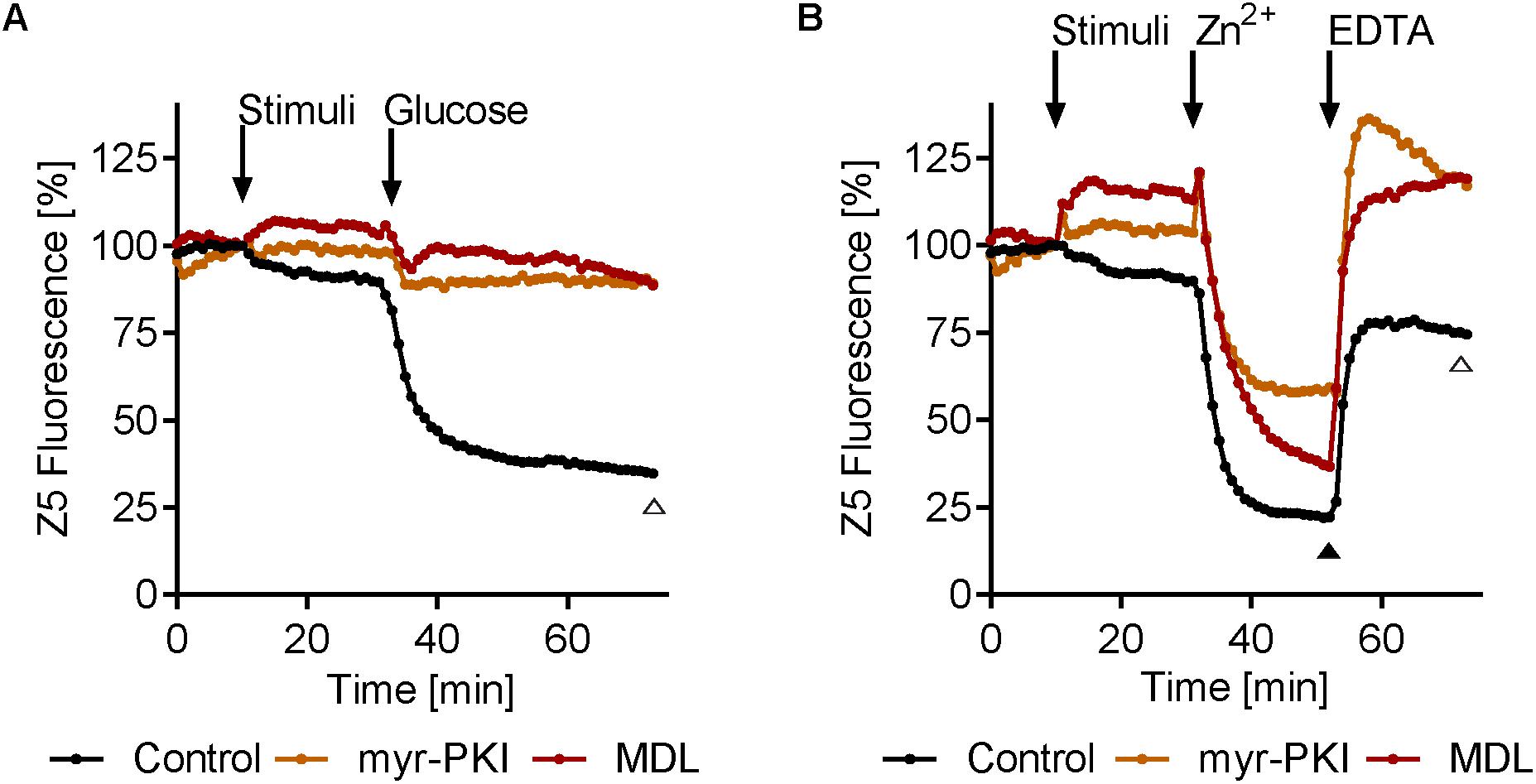
FIGURE 7. Inhibition of cAMP signaling inhibits glucose-induced zinc mobilization. (A) Adenylate cyclase (AC) inhibitor MDL12330 (MDL, 417 μM) or PKA inhibitor myr-PKI (25 μM) was added for 20 min (stimuli) before addition of 0.25% glucose. (B) MDL12330 (MDL, 417 μM) and myr-PKI (25 μM) was added for 20 min (stimuli) before 20 μM zinc was added for 20 min (Zn2+). Finally 100 μM EDTA was added for 20 min. Open triangle (Δ) indicate p < 0.01 in one-way ANOVA test for both myr-PKI and MDL compared to control at the specified time point, while closed triangle () indicate p ≤ 0.01 in one-way ANOVA test for only myr-PKI n = 3–6.
Discussion
In humans, zinc acts as both an intercellular (e.g., neurotransmission) (Sensi et al., 2009) and an intracellular signaling molecule, analogous to well-known second messengers such as Ca2+ and cyclic AMP (Fukada et al., 2011).
While its role as an essential micronutrient in fungi is relatively well established, a function for zinc in cell signaling has not, to the best of our knowledge, been described in the fungal kingdom. The only well-described zinc sensor in fungi is the transcription factor Zap1. In the model yeast, S. cerevisiae, Zap1 positively regulates the expression of zinc uptake genes as cellular zinc becomes limited (Wilson and Bird, 2016) and the C. albicans ortholog (Zap1/Csr1) appears to have a similar role (Ballou and Wilson, 2016).
Here we demonstrate rapid changes in fungal intracellular zinc pools in response to two, apparently distinct environmental signals. When cells were cultured overnight in the presence of (≥10 μM) Zn2+, they assimilated (Table 1) and stored the cation in the internal membrane system, including the ER – the zinbo-5 detectable zinc pool (Figures 1–3). When stimulated with external zinc, these cells immediately began to mobilize this intracellular pool (Figure 3), yet did not assimilate significant levels of zinc from the medium over the 20 min treatment (Table 1). It is possible that the rapid drop in zinbo-5 signal observed here represents direct release of zinc into the cytoplasm, which may act in itself as a second messenger, as has been observed in mammalian cells (Yamasaki et al., 2007).
This indicates that the observed intracellular response to extracellular zinc is likely to be Zap1-independent. Primarily, mobilization occurred immediately following zinc stimulation (Figure 3), which is too rapid to be the result of transcriptional changes; secondly, virtually none of the added zinc actually entered the fungal cell (Table 1). Finally, when these cells were treated with the cell impermeable zinc chelator EDTA, zinc was rapidly re-compartmentalized within the ER (Figure 3). These three lines of evidence suggest the existence of an extracellular zinc sensing system in C. albicans.
Animals encode a plasma membrane G-protein coupled plasma membrane receptor called Gpr39/ZnR, which in humans has been reported to sense extracellular zinc and trigger intracellular signaling events (Hershfinkel et al., 2001; Sunuwar et al., 2017). Although fungi do not share an ortholog, it is interesting to note that Gpr39-mediated signaling was also responsive to micromolar levels of extracellular zinc and did not involve zinc import (Hershfinkel et al., 2001).
Recently, the S. cerevisiae plasma membrane protein Zrt1 has been identified as a fungal transceptor, that in addition to its well-defined role in high affinity zinc assimilation, can also sense extracellular zinc. Intriguingly, addition of zinc to zinc starved cells resulted in rapid (1–2 min) Zrt1-dependent trehalase activation – a classical target of the PKA pathway in yeast (Schothorst et al., 2017).
At this stage it is unclear whether C. albicans responds to extracellular zinc via a GPCR like in animals, or via a transceptor like S. cerevisiae or an as yet unknown mechanism (Figure 8).
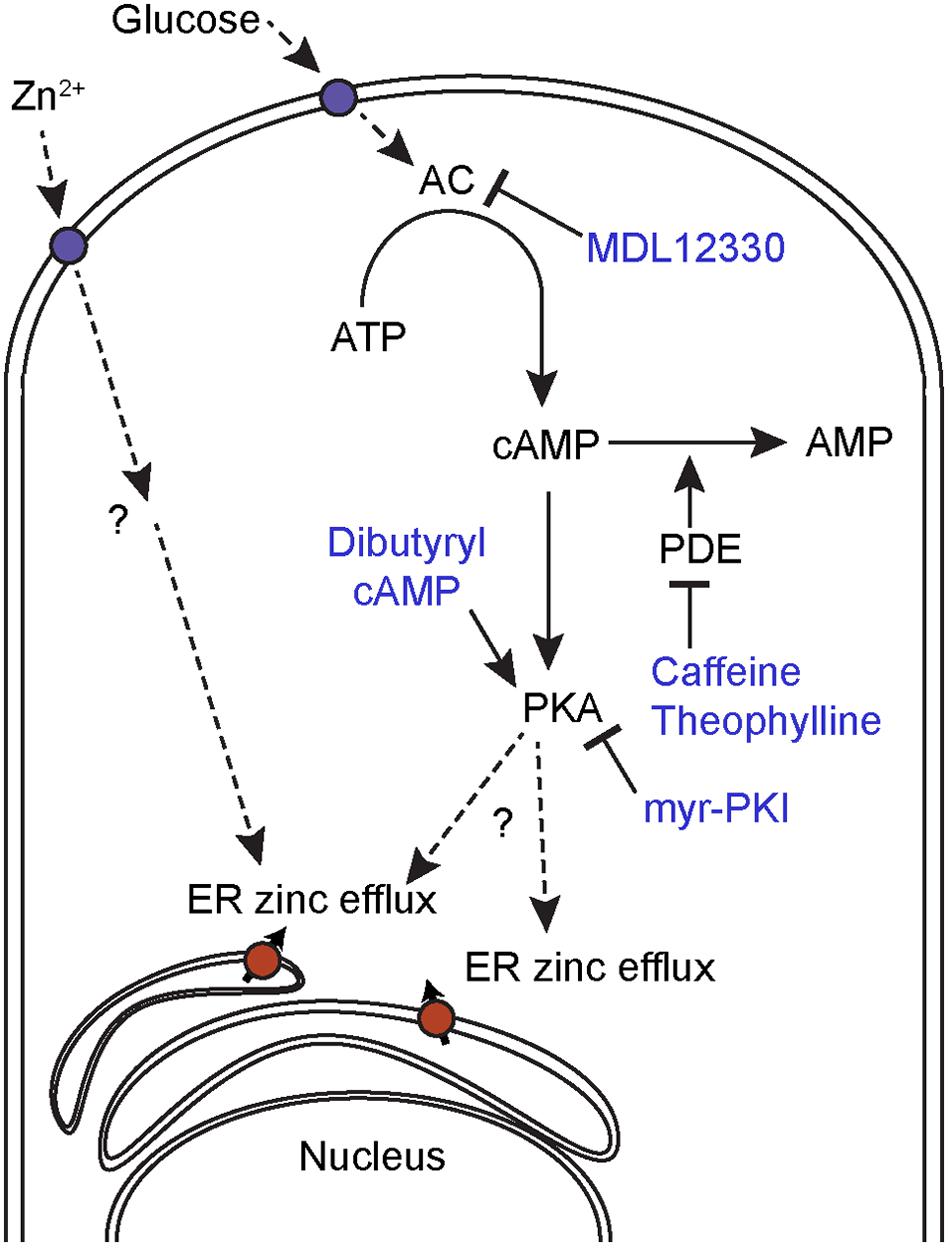
FIGURE 8. Proposed mechanism for intracellular zinc mobilization. Glucose induces cAMP-PKA-dependent intracellular zinc release and can be inhibited by the AC inhibitor MDL12330 or the PKA inhibitor myr-PKI. The cAMP analog, dibutyryl cAMP and PDE inhibitors, also induces intracellular zinc release. Extracellular Zn2+ induces ER zinc efflux through a cAMP-PKA independent mechanism.
Release of intracellular pools in response to stimulation with extracellular zinc has been observed previously. Taylor et al. (2012) reported that treatment of mammalian cells with extracellular zinc or with epidermal growth factor resulted in ER zinc efflux. However, subsequent studies suggest that the zinc flux observed may have been due to ionophore-mediated uptake of zinc from the culture medium (Hessels and Merkx, 2015). We did not use an ionophore in the current study.
In addition to extracellular zinc, we also observed cAMP/PKA-dependent changes in the intracellular zinc pool. Stimulation of cells with the cAMP/PKA agonist glucose resulted in rapid mobilization, and this effect was amplified by the addition of PDEase inhibitors. PDEase inhibitors stimulate the PKA pathway by preventing the hydrolysis of cAMP. Likewise, treatment of cells with the cAMP analog, dibutyryl cAMP had a similar effect. In contrast, inhibition of AC (MDL12330) or PKA activity (myr-PKI) prevented glucose-induced zinc flux. These four lines of evidence suggest that cAMP/PKA activation generates rapid changes in intracellular zinc localization. At this stage it is not clear whether the released zinc acts as second messenger in fungi, as has been shown for mammalian cells.
Interestingly, it would appear that extracellular zinc and cAMP/PKA pathway activation represent distinct mediators of intracellular zinc flux as the zinc-induced flux was not blocked by AC or PKA inhibitors. We therefore propose a model whereby glucose induce ER zinc efflux to the cytoplasm mediated by cAMP/PKA signaling, whereas exogenous zinc induce ER zinc efflux in a cAMP/PKA independent manner (Figure 8).
In summary, this study is the first report of agonist-induced changes in intracellular zinc distribution in a fungal species. Intriguingly, this intracellular zinc flux is triggered by changes in either environmental zinc or glucose and we define a novel role for cyclic AMP/PKA signaling in controlling the intracellular zinc pool.
Author Contributions
LK, A-MW, DW, and AF conceived the study, acquired (LK), analyzed and interpreted the data, drafted the manuscript and critically revised the manuscript. All authors approved the final submitted version and agreed to accountability for all aspects of the work.
Funding
LK was supported by Innovation Fund Denmark, Denmark (4019-00019B). Pcovery ApS received funding from Wellcome Trust, Research Councils, United Kingdom (100480/Z/12), Novo Seeds, Denmark, and Boehringer Ingelheim Venture Fund, Germany. DW was supported by a Sir Henry Dale Fellowships jointly funded by the Wellcome Trust and The Royal Society (102549/Z/13/Z), the Medical Research Council and University of Aberdeen (MR/N006364/1), and received support from a Wellcome Trust Strategic Award for Medical Mycology and Fungal Immunology (097377/Z/11/Z).
Conflict of Interest Statement
LK and A-MW are or were employees of Pcovery ApS.
The other authors declare that the research was conducted in the absence of any commercial or financial relationships that could be construed as a potential conflict of interest.
Supplementary Material
The Supplementary Material for this article can be found online at: https://www.frontiersin.org/articles/10.3389/fmicb.2018.00502/full#supplementary-material
References
Andreini, C., Bertini, I., and Rosato, A. (2009). Metalloproteomes: a bioinformatic approach. Acc. Chem. Res. 42, 1471–1479. doi: 10.1021/ar900015x
Ballou, E. R., and Wilson, D. (2016). The roles of zinc and copper sensing in fungal pathogenesis. Curr. Opin. Microbiol. 32, 128–134. doi: 10.1016/j.mib.2016.05.013
Bozym, R. A., Thompson, R. B., Stoddard, A. K., and Fierke, C. A. (2006). Measuring picomolar intracellular exchangeable zinc in PC-12 cells using a ratiometric fluorescence biosensor. ACS Chem. Biol. 1, 103–111. doi: 10.1021/cb500043a
Brown, G. D., Denning, D. W., Gow, N. A., Levitz, S. M., Netea, M. G., and White, T. C. (2012). Hidden killers: human fungal infections. Sci. Transl. Med. 4:165rv113. doi: 10.1126/scitranslmed.3004404
Castilla, R., Passeron, S., and Cantore, M. L. (1998). N-acetyl-D-glucosamine induces germination in Candida albicans through a mechanism sensitive to inhibitors of cAMP-dependent protein kinase. Cell. Signal. 10, 713–719. doi: 10.1016/S0898-6568(98)00015-1
Citiulo, F., Jacobsen, I. D., Miramon, P., Schild, L., Brunke, S., Zipfel, P., et al. (2012). Candida albicans scavenges host zinc via Pra1 during endothelial invasion. PLoS Pathog. 8:e1002777. doi: 10.1371/journal.ppat.1002777
Clemens, S., Bloss, T., Vess, C., Neumann, D., Nies, D. H., and Zur Nieden, U. (2002). A transporter in the endoplasmic reticulum of Schizosaccharomyces pombe cells mediates zinc storage and differentially affects transition metal tolerance. J. Biol. Chem. 277, 18215–18221. doi: 10.1074/jbc.M201031200
Dean, K. M., Qin, Y., and Palmer, A. E. (2012). Visualizing metal ions in cells: an overview of analytical techniques, approaches, and probes. Biochim. Biophys. Acta 1823, 1406–1415. doi: 10.1016/j.bbamcr.2012.04.001
Devirgiliis, C., Murgia, C., Danscher, G., and Perozzi, G. (2004). Exchangeable zinc ions transiently accumulate in a vesicular compartment in the yeast Saccharomyces cerevisiae. Biochem. Biophys. Res. Commun. 323, 58–64. doi: 10.1016/j.bbrc.2004.08.051
Eide, D. J. (2006). Zinc transporters and the cellular trafficking of zinc. Biochim. Biophys. Acta 1763, 711–722. doi: 10.1016/j.bbamcr.2006.03.005
Ellis, C. D., MacDiarmid, C. W., and Eide, D. J. (2005). Heteromeric protein complexes mediate zinc transport into the secretory pathway of eukaryotic cells. J. Biol. Chem. 280, 28811–28818. doi: 10.1074/jbc.M505500200
Ellis, C. D., Wang, F., MacDiarmid, C. W., Clark, S., Lyons, T., and Eide, D. J. (2004). Zinc and the Msc2 zinc transporter protein are required for endoplasmic reticulum function. J. Cell Biol. 166, 325–335. doi: 10.1083/jcb.200401157
Fukada, T., Yamasaki, S., Nishida, K., Murakami, M., and Hirano, T. (2011). Zinc homeostasis and signaling in health and diseases. J. Biol. Inorg. Chem. 16, 1123–1134. doi: 10.1007/s00775-011-0797-4
Hershfinkel, M., Moran, A., Grossman, N., and Sekler, I. (2001). A zinc-sensing receptor triggers the release of intracellular Ca2+ and regulates ion transport. Proc. Natl. Acad. Sci. U.S.A. 98, 11749–11754. doi: 10.1073/pnas.201193398
Hessels, A. M., and Merkx, M. (2015). Genetically-encoded FRET-based sensors for monitoring Zn2+ in living cells. Metallomics 7, 258–266. doi: 10.1039/c4mt00179f
Hood, M. I., and Skaar, E. P. (2012). Nutritional immunity: transition metals at the pathogen-host interface. Nat. Rev. Microbiol. 10, 525–537. doi: 10.1038/nrmicro2836
Jain, P., Akula, I., and Edlind, T. (2003). Cyclic AMP signaling pathway modulates susceptibility of Candida species and Saccharomyces cerevisiae to antifungal azoles and other sterol biosynthesis inhibitors. Antimicrob. Agents Chemother. 47, 3195–3201. doi: 10.1128/AAC.47.10.3195-3201.2003
Koning, A. J., Lum, P. Y., Williams, J. M., and Wright, R. (1993). DiOC6 staining reveals organelle structure and dynamics in living yeast cells. Cell Motil. Cytoskeleton 25, 111–128. doi: 10.1002/cm.970250202
Kullberg, B. J., and Arendrup, M. C. (2015). Invasive candidiasis. N. Engl. J. Med. 373, 1445–1456. doi: 10.1056/NEJMra1315399
Li, L., and Kaplan, J. (2001). The yeast gene MSC2, a member of the cation diffusion facilitator family, affects the cellular distribution of zinc. J. Biol. Chem. 276, 5036–5043. doi: 10.1074/jbc.M008969200
Lim, N. C., Freake, H. C., and Brückner, C. (2004). Illuminating zinc in biological systems. Chemistry 11, 38–49. doi: 10.1002/chem.200400599
MacDiarmid, C. W., Milanick, M. A., and Eide, D. J. (2003). Induction of the ZRC1 metal tolerance gene in zinc-limited yeast confers resistance to zinc shock. J. Biol. Chem. 278, 15065–15072. doi: 10.1074/jbc.M300568200
Mayer, F. L., Wilson, D., Jacobsen, I. D., Miramon, P., Grosse, K., and Hube, B. (2012). The novel Candida albicans transporter Dur31 Is a multi-stage pathogenicity factor. PLoS Pathog. 8:e1002592. doi: 10.1371/journal.ppat.1002592
Nishida, K., Hasegawa, A., Nakae, S., Oboki, K., Saito, H., Yamasaki, S., et al. (2009). Zinc transporter Znt5/Slc30a5 is required for the mast cell-mediated delayed-type allergic reaction but not the immediate-type reaction. J. Exp. Med. 206, 1351–1364. doi: 10.1084/jem.20082533
Schothorst, J., Zeebroeck, G. V., and Thevelein, J. M. (2017). Identification of Ftr1 and Zrt1 as iron and zinc micronutrient transceptors for activation of the PKA pathway in Saccharomyces cerevisiae. Microb. Cell 4, 74–89. doi: 10.15698/mic2017.03.561
Sensi, S. L., Paoletti, P., Bush, A. I., and Sekler, I. (2009). Zinc in the physiology and pathology of the CNS. Nat. Rev. Neurosci. 10, 780–791. doi: 10.1038/nrn2734
Simm, C., Luan, C. H., Weiss, E., and O’Halloran, T. (2011). High-throughput screen for identifying small molecules that target fungal zinc homeostasis. PLoS One 6:e25136. doi: 10.1371/journal.pone.0025136
Strachan, S. (2010). Trace elements. Curr. Anaesth. Crit. Care 21, 44–48. doi: 10.1016/j.cacc.2009.08.004
Sunuwar, L., Gilad, D., and Hershfinkel, M. (2017). The zinc sensing receptor, ZnR/GPR39, in health and disease. Front. Biosci. (Landmark Ed.) 22, 1469–1492. doi: 10.2741/4554
Taylor, K. M., Hiscox, S., Nicholson, R. I., Hogstrand, C., and Kille, P. (2012). Protein kinase CK2 triggers cytosolic zinc signaling pathways by phosphorylation of zinc channel ZIP7. Sci. Signal. 5:ra11. doi: 10.1126/scisignal.2002585
Urban, C. F., Ermert, D., Schmid, M., Abu-Abed, U., Goosmann, C., Nacken, W., et al. (2009). Neutrophil extracellular traps contain calprotectin, a cytosolic protein complex involved in host defense against Candida albicans. PLoS Pathog. 5:e1000639. doi: 10.1371/journal.ppat.1000639
Wellenreuther, G., Cianci, M., Tucoulou, R., Meyer-Klaucke, W., and Haase, H. (2009). The ligand environment of zinc stored in vesicles. Biochem. Biophys. Res. Commun. 380, 198–203. doi: 10.1016/j.bbrc.2009.01.074
Wilson, D., Fiori, A., Brucker, K. D., Dijck, P. V., and Stateva, L. (2010). Candida albicans Pde1p and Gpa2p comprise a regulatory module mediating agonist-induced cAMP signalling and environmental adaptation. Fungal Genet. Biol. 47, 742–752. doi: 10.1016/j.fgb.2010.06.006
Wilson, S., and Bird, A. J. (2016). Zinc sensing and regulation in yeast model systems. Arch. Biochem. Biophys. 611, 30–36. doi: 10.1016/j.abb.2016.02.031
Yamasaki, S., Sakata-Sogawa, K., Hasegawa, A., Suzuki, T., Kabu, K., Sato, E., et al. (2007). Zinc is a novel intracellular second messenger. J. Cell Biol. 177, 637–645. doi: 10.1083/jcb.200702081
Yamashita, S., Miyagi, C., Fukada, T., Kagara, N., Che, Y. S., and Hirano, T. (2004). Zinc transporter LIVI controls epithelial-mesenchymal transition in zebrafish gastrula organizer. Nature 429, 298–302. doi: 10.1038/nature02545
Keywords: cAMP, zinc, Candida albicans, signaling, ER
Citation: Kjellerup L, Winther A-ML, Wilson D and Fuglsang AT (2018) Cyclic AMP Pathway Activation and Extracellular Zinc Induce Rapid Intracellular Zinc Mobilization in Candida albicans. Front. Microbiol. 9:502. doi: 10.3389/fmicb.2018.00502
Received: 27 November 2017; Accepted: 05 March 2018;
Published: 21 March 2018.
Edited by:
Dominique Sanglard, Université de Lausanne, SwitzerlandReviewed by:
Sascha Brunke, Hans-Knöll-Institut, GermanyMalcolm Whiteway, Concordia University, Canada
Copyright © 2018 Kjellerup, Winther, Wilson and Fuglsang. This is an open-access article distributed under the terms of the Creative Commons Attribution License (CC BY). The use, distribution or reproduction in other forums is permitted, provided the original author(s) and the copyright owner are credited and that the original publication in this journal is cited, in accordance with accepted academic practice. No use, distribution or reproduction is permitted which does not comply with these terms.
*Correspondence: Anja T. Fuglsang, YXRmQHBsZW4ua3UuZGs=
†These authors have contributed equally to this work.