- 1Parco Scientifico e Tecnologico della Sicilia, Catania, Italy
- 2Dipartimento di Agricoltura, Alimentazione e Ambiente, Università degli Studi di Catania, Catania, Italy
- 3Dipartimento di Scienze Agrarie, Alimentari e Forestali, Università degli Studi di Palermo, Palermo, Italy
- 4School of Computing, Federal University of Mato Grosso do Sul, Campo Grande, Brazil
- 5Dipartimento di Agraria, Università degli Studi “Mediterranea” di Reggio Calabria, Reggio Calabria, Italy
- 6Department of Agriculture, School of Agriculture, Food and Nutrition, Technological Educational Institute of Crete, Heraklion, Greece
- 7Department of Biosciences, College of Life and Environmental Sciences, University of Exeter, Exeter, United Kingdom
- 8Institute of Molecular Biology and Biotechnology, Foundation for Research and Technology – Hellas, Heraklion, Greece
Cyclic lipopeptides (CLPs) are considered as some of the most important secondary metabolites in different plant-associated bacteria, thanks to their antimicrobial, cytotoxic, and surfactant properties. In this study, our aim was to investigate the role of the Quorum Sensing (QS) system, PcoI/PcoR, and the LuxR-type transcriptional regulator RfiA in CLP production in the phytopatogenic bacterium, Pseudomonas corrugata based on our previous work where we reported that the pcoR and rfiA mutants were devoid of the CLPs cormycin and corpeptin production. Due to the close genetic link between the QS system and the RfiA (rfiA is co-transcribed with pcoI), it was difficult to ascertain the specific regulatory role in the expression of target genes. A transcriptional approach was undertaken to identify the specific role of the PcoR and RfiA transcriptional regulators for the expression of genes involved in CLP production. The RNA-seq-based transcriptional analysis of the wild-type (WT) strain CFBP 5454 in comparison with GL2 (pcoR mutant) and GLRFIA (rfiA mutant) was performed in cultural conditions favoring CLP production. Differential gene expression revealed that 152 and 130 genes have significantly different levels of expression in the pcoR and rfiA mutants, respectively. Of these, the genes linked to the biosynthesis of CLPs and alginate were positively controlled by both PcoR and RfiA. Blast homology analysis showed that 19 genes in a large CLP biosynthetic cluster involved in the production of three antimicrobial peptides, which span approximately 3.5% of the genome, are strongly over-expressed in the WT strain. Thus, PcoR and RfiA function mainly as activators in the production of bioactive CLPs, in agreement with phenotype analysis of mutants. RNA-seq also revealed that almost all the genes in the structural/biosynthetic cluster of alginate exopolysaccharide (EPS) are under the control of the PcoR–RfiA regulon, as supported by the 10-fold reduction in total EPS yield isolated in both mutants in comparison to the parent strain. A total of 68 and 38 gene expressions was independently regulated by PcoR or RfiA proteins, respectively, but at low level. qPCR experiments suggest that growth medium and plant environment influence the expression of CLP and alginate genes.
Introduction
Pseudomonas corrugata Roberts and Scarlett 1981 is a ubiquitous bacterium in agro-ecosystems. It has been isolated from bulk soils, plant rhizosphere, and either as endophyte or parasite from diverse cultivated plants (Catara, 2007). It was first described in the United Kingdom (Scarlett et al., 1978) as the causal agent of tomato pith necrosis (TPN) and later was reported in association with TPN worldwide (Catara, 2007). It has a very low host range, and along with tomato, it has been sporadically described as a plant pathogen on pepper and chrysanthemum (Catara, 2007). P. corrugata strains have a notable antimicrobial activity against bacteria, chromista, and fungi, and some strains have been successfully tested as biological control agents in different pathosystems (Catara, 2007; Strano et al., 2017). P. corrugata produces several bioactive compounds such as the lipopeptide siderophore corrugatin (Risse et al., 1998), the cyclic lipopeptides (CLPs) cormycin A and corpeptin A and B (Emanuele et al., 1998; Scaloni et al., 2004), and hydrogen cyanide (Strano et al., 2017). Cormycin and corpeptins merit great interest as they have both phytotoxic and antimicrobial properties (Emanuele et al., 1998; Scaloni et al., 2004). CLPs consist of a short oligopeptide that is cyclized to form a lacton ring with a linked fatty acid tail and they may have diverse roles in plant-associated Pseudomonas species, such as motility, biofilm formation, antimicrobial activity, and they also play a key role in virulence of phytopathogenic bacteria (Bender et al., 1999; Raaijmakers et al., 2006).
Cyclic lipopeptides are synthesized with a thiotemplate process by large multifunctional non-ribosomal peptide synthetases (NRPSs) that have a modular structure (Gross and Loper, 2009). Each module serves as a building block for the gradual incorporation of one amino acid in the peptide chain (Finking and Marahiel, 2004; Raaijmakers et al., 2006). CLP biosynthetic loci, organized in clusters which include transporter systems and regulatory genes, have been described in several Pseudomonas spp. (de Bruijn and Raaijmakers, 2009a; Gross and Loper, 2009). Proteins of the LuxR superfamily play an important role in the regulation of CLP production. This superfamily consists of transcriptional regulators containing a DNA-binding helix-turn-helix (HTH) motif in the C-terminal region and the proteins are grouped into different subfamilies based on their domain architecture and activation mechanism (Subramoni and Venturi, 2009; Chen and Xie, 2011; Vaughn and Gross, 2016). Three different LuxR-type regulators are involved in CLP biosynthesis in many Pseudomonas species (Lu et al., 2002; Dubern et al., 2005; Wang et al., 2006a; Berti et al., 2007; de Bruijn and Raaijmakers, 2009b). The first group consists of regulators belonging to a two-component sensory transduction system, activated upon the phosphorylation of a transmembrane kinase as in the GacA/GacS regulatory system, with a key role in syringomycin–syringopeptin, putisolvins, massetolide A, and viscosin production (Scholz-Schroeder et al., 2001; Dubern et al., 2005; de Bruijn et al., 2007, 2008). Mutations disrupting either of the two genes result in impaired CLP production. The second group consists of regulators that contain an autoinducer-binding domain in the N-terminal region, usually activated via binding to an N-acyl homoserine lactone (AHL) in different Pseudomonas spp. (von Bodman et al., 2003; Venturi, 2006; Subramoni and Venturi, 2009). The conjugate serves as a signaling molecule involved in Quorum Sensing (QS). AHL-QS plays a role in CLP production in terms of viscosin and putisolvin biosynthesis in the plant pathogenic P. fluorescens strain 5064 and the saprophytic P. putida strain PCL1445, respectively (Cui et al., 2005; Dubern et al., 2006). The third group of LuxR-type transcriptional regulators harbors the typical C-terminal HTH DNA-binding domain but lacks an N-terminal regulatory domain. They have been found positioned up and downstream of the CLP biosynthesis clusters of different Pseudomonas, playing a crucial role in the production of several CLPs, including syringomycin, syringopeptin, syringafactins, putisolvins, viscosin, massetolide, sessilin, and orfamide (Lu et al., 2002; Dubern et al., 2005; Wang et al., 2006a; Berti et al., 2007; de Bruijn and Raaijmakers, 2009b; Vaughn and Gross, 2016; Olorunleke et al., 2017).
In our previous studies, we demonstrated that two LuxR-type regulators, PcoR and RfiA in P. corrugata, have a role in virulence on tomato, and elicitation of hypersensitive-like response on Nicotiana spp. Neither cormycin nor corpeptins were detected in the culture filtrates of the pcoR and rfiA mutants (Licciardello et al., 2012). However, only in the rfiA mutant was the ability to inhibit fungal growth in dual plate assays greatly reduced (Strano et al., 2017). PcoR is part of a QS system mediated by a set of AHLs, namely N-hexanoyl-L-homoserine lactone (C6-HSL), 3-oxo-C6-HSL, and C8-HSL, and it is synthesized thanks to the AHL synthase PcoI (Licciardello et al., 2007). Unlike PcoR, RfiA lacks a N-terminal regulatory domain but it is directly controlled by QS via positive-feedback regulatory loops, since rfiA is located downstream of pcoI and they are co-transcribed (Licciardello et al., 2009). The 20 kb cosmid insert in which QS genes were identified was also shown to contain an operon designated as pcoABC downstream of rfiA. This operon encodes a tripartite resistance nodulation-cell-division (RND) transporter system. Genes encoding for an ABC-transport system and part of an NRPS are involved in the production of corpeptins, designated as crpCDE (Licciardello et al., 2009; Strano et al., 2015). Hierarchical regulation where the PcoR–AHL complex regulates the pcoI/rfiA operon and, in turn, RfiA activates pcoABC transcription has been demonstrated (Licciardello et al., 2009). Since RfiA does not require AHL to be active, its complementation in trans has also been shown to be sufficient to restore pathogenicity of the pcoR mutant in the absence of AHL (Licciardello et al., 2009). Interestingly the pcoI mutant, which is actually a pcoI-/rfiA-double mutant, has been shown to be as virulent as the wild-type (WT) strain. Thus, it has been suggested a model where either QS regulates the synthesis of RfiA or PcoR regulates virulence independently of the AHL (Licciardello et al., 2009).
Genome analysis has revealed that P. corrugata could putatively produce at least four NR peptides, a polyketide, and a bacteriocin (Licciardello et al., 2014; Trantas et al., 2015). The availability of the genome sequences of a number of P. corrugata strains including our model strain CFBP 5454, isolated from tomato affected by TPN, led us to further investigate both the role of PcoR and RfiA by an RNA-seq approach. Under the experimental conditions proven to induce cormycin and corpeptin production in vitro (Scaloni et al., 2004; Licciardello et al., 2009; Strano et al., 2015) PcoR and RfiA positively regulate the same set of genes involved in the secondary metabolite production of (i) three antimicrobial peptides in a DNA region that spans approximately 3.5% of the P. corrugata genome and (ii) all of the biosynthetic/structural alginate genes. In line with these findings, supported by phenotypic analysis, in this work we further support the previously proposed model, for pcoABC regulation. In this model, QS at a high cellular concentration regulates these important traits for P. corrugata fitness and biology via RfiA. Expression analysis studies on the WT strain also suggest that in comparison to alginate genes, CLP genes present higher expression levels in minimal media, while alginate genes presented higher expression in rich media and in planta.
Materials and Methods
Bacterial and Fungal Strains and Routine Growing Conditions
Pseudomonas corrugata strain CFBP 5454 and the derivative mutants used in this study are listed in Table 1. They were routinely cultured at 28°C on either Nutrient Agar (NA, Oxoid, Milan, Italy) supplemented by 1% D-glucose (NDA), or Luria-Bertani (LB) agar (Oxoid, Milan, Italy) (Table 1). The pcoR-mutant strain, designated GL2 is a Tn5 mutant (pcoR76::Tn5) (Licciardello et al., 2007); the rfiA mutant (GLRFIA strain) was obtained by insertional mutagenesis using the conjugative suicide vector pKNOCK-Km (rfiA::pKnock) (Licciardello et al., 2009). The complemented mutant strains used in phenotypic tests are listed in Table 1.
Antibiotics were added as required in the following final concentrations: tetracycline, 40 μg ml-1; gentamicin, 40 μg ml-1, and kanamycin, 100 μg ml-1.
For transcript profiling by RNA-seq, mid-logarithmic phase cells grown on nutrient broth (NB, Oxoid, Milan, Italy) were used to inoculate Improved Minimal Medium (IMM) (Surico et al., 1988) at an OD600 = 0.05, and incubated in static conditions at 28°C (Scaloni et al., 2004; Licciardello et al., 2012; Strano et al., 2015). In each experiment three separate batch cultivations were performed for each bacterial strain. The Gram-positive bacterium Bacillus megaterium ITM100 and the yeast Rhodotorula pilimanae ATCC 26423 were used as bioindicators of CLP production according to Lavermicocca et al. (1997).
RNA Isolation
RNA from WT P. corrugata CFBP 5454 as well as GL2 (pcoR76::Tn5) and GLRFIA (rfiA::pKnock) mutants were extracted from cells grown at the early stationary phase (t = 40 h, OD600 = 8.9) in IMM at 28°C. Samples from three replicates of each strain grown on separate days and different batches of medium were collected. The cultures were fixed using RNATM Protect Bacterial Reagent (Qiagen Inc.) in a ratio of 2 ml of reagent per 1 ml of bacterial culture. Centrifugation was used to pellet the cells (5000 rpm, 4°C, 20 min), and RNA was extracted in RNase-/DNase-free water using the RNeasy Mini Kit (Qiagen Inc.). Total RNA was quantified using micro-spectrophotometry (NanodropTM 2000C, Thermo ScientificTM, Waltham, MA, United States). The RNA quality was estimated using an Agilent 2100 Bioanalyzer and RNA samples with an RNA Integrity Number (RIN) above 8.0 were selected.
Library Construction and RNA Sequencing
Libraries were prepared for sequencing according to the manufacturer’s instructions (Illumina). Single-end 51 nucleotide sequence reads were obtained using the Illumina HiSeq2000 system at Parco Tecnologico Padano (Lodi, Italy), processed with Casava version 1.8. Raw sequencing reads were quality controlled using FastQC v.0.10.1 and processed with Trimmomatic v.0.32 to remove sequencing adapters and low-quality bases. High-quality filtered reads were aligned against the P. corrugata genome (ATKI01000000). Bowtie v2.2.2 software was used to perform the alignments and generate the corresponding BAM files.
Aligned reads were processed using HTSeq v0.6.1 to extract read counts over the annotated genes for the genome provided. For all samples, the number of raw reads mapping to each gene was normalized based on the total number of input reads (non-rRNA and non-tRNA reads) for that sample. This normalization procedure enabled gene-expression patterns to be compared across strains, within and between experiments. Reads that partially overlapped a gene contributed to its total raw read value. Only genes that had an average of >10 reads in the three replicates for the WT in comparison with the mutants were considered for further analyses.
The read counts for each sample were imported into R and processed using the Bioconductor package EdgeR. Counts values were normalized using the Trimmed Mean of M-values (TMM) method and statistical comparisons of expression levels across different groups were performed using the EdgeR exact test method. For the further analyses, genes with a false-discovery rate of ≤0.05 were selected. We relied on the top 243 differentially expressed genes without any fold change cut-off.
The RNA-Seq data were submitted to the Sequence Read Archive (SRA) under accession number SRP128274.
RNA Isolation From Inoculated Tomato Plants
RNA was extracted from tomato cv. Bacio plants previously inoculated with P. corrugata CFBP 5454. Tomato plants were grown in nursery flats. After germination and during the trials, plants were maintained in a growth chamber with a 16 h/8 h photoperiod and a temperature of 26°C. Tomato plants were pin-pricked on the stem at the axil of the first true leaf with bacterial cells from 48-h culture on NDA (Licciardello et al., 2007). Four days after inoculation, 5 cm of stem portions including the inoculation site was sampled and stored at -80°C. Pools of four stems for each bacteria-inoculated plant were ground in liquid nitrogen and 100 mg of powder processed for total RNA extraction with the RNeasy Plant minikit (Qiagen Inc.), according to the manufacturer’s instructions.
Primer Design and Quantitative Real-Time PCR Validation
Quantitative Real-Time PCR (qPCR) was performed on 13 genes of the CLP cluster (crpC, grsb_1, grsB_2, dhbF_3, dhbF_4, syrD2, bepE_1, mefA, arpC, crpD, pcoA, pcoB, oprM_3). Three genes belonging to the biosynthetic cluster of alginate (algD, algG, algI) were selected for validation too. Nucleotide FASTA sequences were retrieved from the P. corrugata CFBP 5454 genome and used to design the primer sets useful for qPCR. Primers were designed with Beacon software (Premier Biosoft International Ltd., Palo Alto, CA, United States) and validated by BLAST (Altschul et al., 1990) in order to minimize the mispriming sites in other genomic loci (Supplementary File 1).
After treatment of the RNA samples with DNAse I (Invitrogen, Life Technologies, Italy), 1 μg of total RNA (from three different independent extractions) was used for cDNA synthesis with Superscript III (Invitrogen, Life Technologies, Italy) according to the manufacturer’s protocol. Samples in which reverse transcriptase had not been added were used as negative controls.
Reactions were conducted with the BioRad iQ Cycler and the SYBR® Select Master Mix for CFX (Applied Biosystem, Life Technologies, Italy) according to the manufacturer’s protocols. To correct small differences in template concentration, the 16S rRNA gene was used for normalization (Conte et al., 2006). Analysis of the dissociation curve ensured that a single product was amplified. cDNA synthesis reaction was performed at 95°C for 15 s, and at 58–64°C for 1 min (for annealing temperatures see Supplementary File 1).
Data were analyzed using the comparative Ct method, wherein the Ct values of the samples of interest were compared to the Ct values of a control. All the Ct values were normalized versus the 16S rRNA gene. The relative expression (RE) values were calculated by the formula RE = 2-[ΔCt(Wt) - ΔCt(mutant)] (Livak and Schmittgen, 2001).
Bioinformatics Tools for Genomic and Transcriptomic Data
Genome comparative analysis and gene cluster visualization were performed using the Integrated Microbial Genomes & Microbiomes (IMG/M) system1. The antiSMASH software pipeline (Blin et al., 2013) was used for the automated identification of secondary metabolite biosynthesis clusters. The number of genes differentially regulated was shown in a Venn diagram. Graphical representation of the relationship between intensity (LogCPM) and difference (Log2FC) of transcripts between P. corrugata CFBP 5454 (Wt) versus GL2 and GLRFIA derivatives mutants was done graphically represented using the DEPICTViz, Differential Expression, and Protein InteraCTions Visualization tool (Lima et al., 2016). Functional annotation, which categorizes genes into functional classes, was performed by Gene Ontology (GO) identification developed at the GO Consortium (Ashburner et al., 2000).
In Vitro Bioassay for CLP Production
Antimicrobial activity of 10× concentrated culture filtrates from bacterial strains grown in IMM and NB were assessed after 4 days of incubation in static conditions, using the well diffusion assay according to Licciardello et al. (2009). Two CLP-sensitive bioindicator strains R. pilimanae ATTC26432 and B. megaterium ITM100 previously grown in layers on top of agar potato dextrose agar plates (PDA, Oxoid, Milan, Italy) were used (Licciardello et al., 2009). The in zone for each antimicrobial compound tested was measured. All tests were carried out at least twice in triplicate.
Exopolysaccharide Isolation and Quantification
Total exopolysaccharides (EPSs) were isolated from P. corrugata CFBP 5454 and derivative mutants grown in IMM at 28°C for 4 days. EPSs were also evaluated from WT strain grown on NB. After centrifugation at 16,300 × g for 20 min to remove cells, total EPSs were isolated according to Fett et al. (1996) with slight modifications (Licciardello et al., 2017). Three separate partially purified samples were prepared for each bacterial strain.
Statistical Analysis
Data were analyzed by two-way ANOVA using IBM® SPSS® v20. Mean values were compared using the Student–Newman–Keuls test. Statistical significance was established at P ≤ 0.05 and P ≤ 0.001.
Results
Differential Expression Analysis of the Transcriptome of Pseudomonas corrugata CFBP 5454 Versus pcoR and rfiA Mutants
To investigate the regulatory functions of P. corrugata LuxR transcriptional regulators PcoR and RfiA, expression profiles from RNA-seq data were analyzed. The transcriptome of P. corrugata strain CFBP 5454 was compared to those of the mutant strains GL2 (pcoR mutant) and GLRFIA (rfiA mutant) (Licciardello et al., 2007, 2009) grown to the early stationary phase in IMM which facilitates CLP production (Scaloni et al., 2004; Licciardello et al., 2009, 2012). Libraries derived from single-stranded cDNAs were sequenced and mapped against P. corrugata CFBP 5454 reference genome (ATKI01000000). Genes with increased or decreased expression in the WT strain compared to the mutant strains were considered to be positively or negatively regulated by PcoR or RfiA.
With a false-discovery rate (FDR) correction of 5%, 152 genes (46 increased and 106 decreased) differed significantly in the pcoR mutant, and 130 genes (52 increased and 78 decreased) differed significantly in the rfiA mutant compared to the parent strain CFBP 5454 (Figure 1A). Overall, the expression of 92 genes, which represent 3% of the annotated genes in the CFBP 5454 draft genome, differed significantly in both pcoR and rfiA mutants (Supplementary Files 2, 3). The remaining 60 (out of 152) and 38 (out of 130) genes were independently regulated either by PcoR or RfiA, respectively (Figure 1A) (Supplementary Files 4, 5). The Supplementary Files contain a thorough analysis of the transcripts and their predicted functions found to be associated with the role of PcoR and RfiA (Supplementary Files 2–5). In order to assemble a catalog of functions strongly linked to these transcriptional regulators, differentially expressed genes for both mutants were grouped based on their GO utilizing GO Consortium2. Genes were grouped into 14 functional categories on the basis of PseudoCAP and were plotted with respect to down-regulation and up-regulation (Figure 1B).
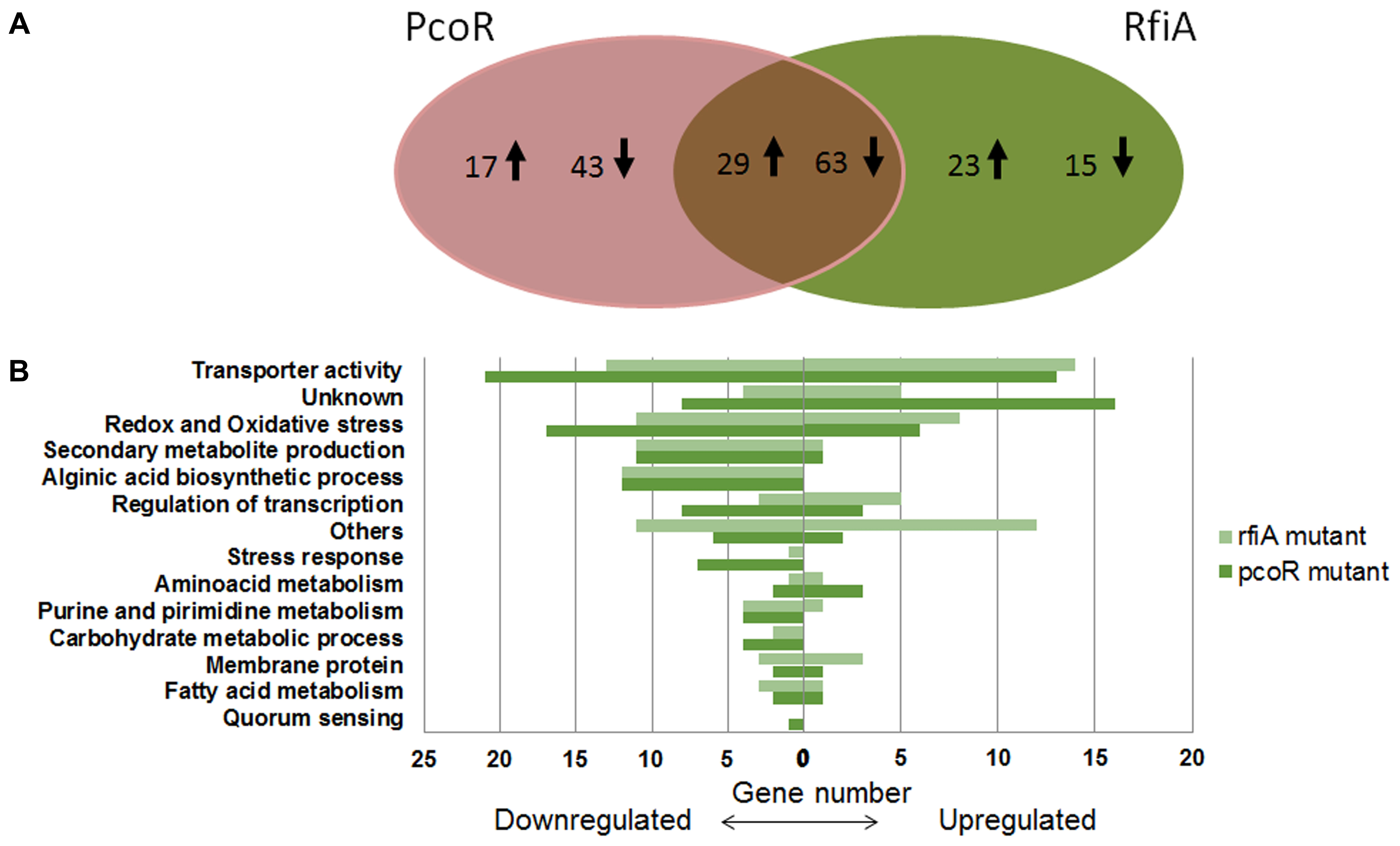
FIGURE 1. Differential expressed genes in Pseudomonas corrugata CFBP 5454 WT strain in comparison to pcoR- and rfiA-derivative mutants by RNA-seq analysis. (A) Venn diagram with up-/down-regulated genes after mutation in pcoR and/or rfiA genes. A total of 180 genes were found to be differentially expressed, 92 of which overlap in the transcriptional profiles of both mutants (in the center of the diagram). Up and down arrows represent genes up- and down-regulated, respectively. (B) Number and functional classification of genes up- and down-regulated in P. corrugata strain CFBP 5454 in comparison with pcoR- and rfiA-derivative mutants. The plot indicates the type of physiological role(s) and the total number of genes with decreased and increased expression within each category.
The largest group consisted of enzymes associated with transport systems, 34 of which were differentially expressed in the WT strain compared with expression in pcoR mutant and 27 with rfiA mutant. The second largest group were the genes involved in redox and oxidative stress, most of which were down-regulated in both pcoR (17 genes) and rfiA (11 genes) mutants. Genes predicted to be related with alginic acid biosynthesis (12 genes) and secondary metabolite production (11 genes) were well represented among the over-expressed genes in the WT strains in comparison to mutants, thus revealing the predominantly positive control of both PcoR and RfiA in the biosynthesis of these molecules. Transcriptional regulator genes account for a significant number of transcripts affected by pcoR and rfiA mutations, including up- (8 genes) and down- (11 genes) regulated genes that show a wide-ranging control through a cascade of other regulators. Other gene categories affected are involved in carbohydrate metabolic processes, fatty acids, amino acids, and purine and pyrimidine metabolisms.
CLP Biosynthesis Clusters Are Part of the PcoR–RfiA Regulon
RNA-seq analysis showed that among the transcripts differentially expressed in both pcoR and rfiA mutants, there are 21 genes putatively involved in CLP production, as ascertained by homology BLAST analysis. The genome of P. corrugata CFBP 5454 was assembled into 156 contigs and NRP genes were located in at least 10 different contigs (Licciardello et al., 2014; Trantas et al., 2015). Therefore, for the in-silico reconstruction of P. corrugata secondary metabolite clusters, we used the annotated sequence of strain LMG 2172T (also known as BS3649, Genbank accession NZ_LT629798.1) which shared an average nucleotide identity (ANI) of 99.53% with strain CFBP 5454. Using combined AntiSMASH and BLAST analyses, we found that the differentially expressed genes were located in a large HSL-NRPS cluster accounting for approximately 3.4% of the LMG 2172T genome (Figure 2A, Table 2 and Supplementary File 6).
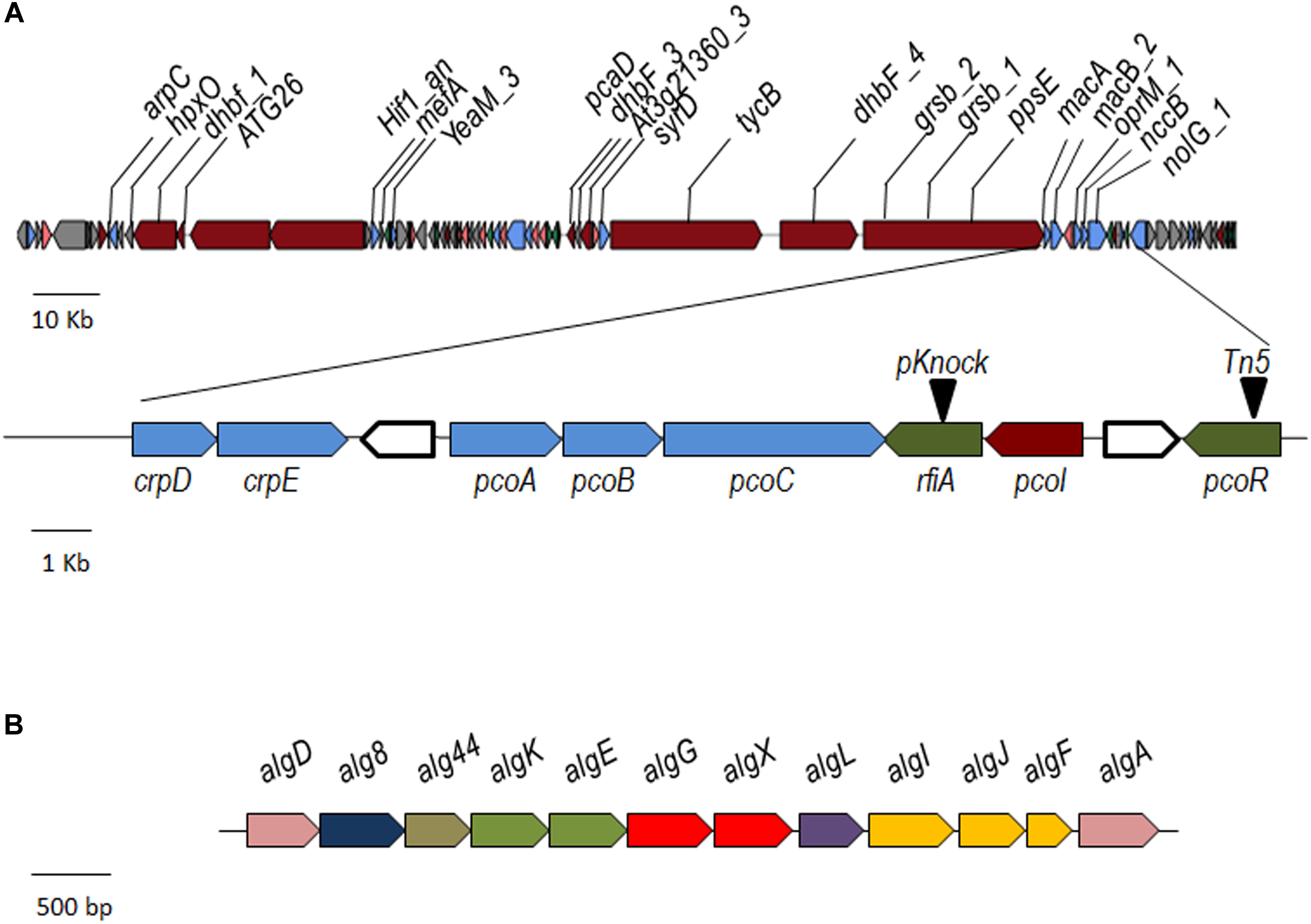
FIGURE 2. The P. corrugata gene clusters involved in the biosynthesis of cyclolipopeptides (A) and alginate (B). (A) Genes over-expressed in P. corrugata CFBP 5454 in comparison with both pcoR- and rfiA-derivative mutants are labeled in the upper part of the graph. In the enlarged frame, genes for PcoI/PcoR QS system, RfiA, PcoABC, and CrpDE transporter system are labeled. Triangles indicate the positions of insertional mutagenesis. pcoI was over-expressed in the parent strain only in comparison to the pcoR-derivative mutant. Core biosynthetic genes (dark red), transport-related genes (light blue), regulatory genes (dark green), additional biosynthetic gene (pink), and other genes (gray) are represented. (B) Genes of the alginate structural/biosynthetic cluster differentially expressed in both pcoR and rfiA mutants; sugar production (pink), subunit polymerization (dark blue), c-di-GMP (gray), outer membrane secretion protein (light green), epimerase/modification (red), lyase (violet), and O-acetylation (yellow).
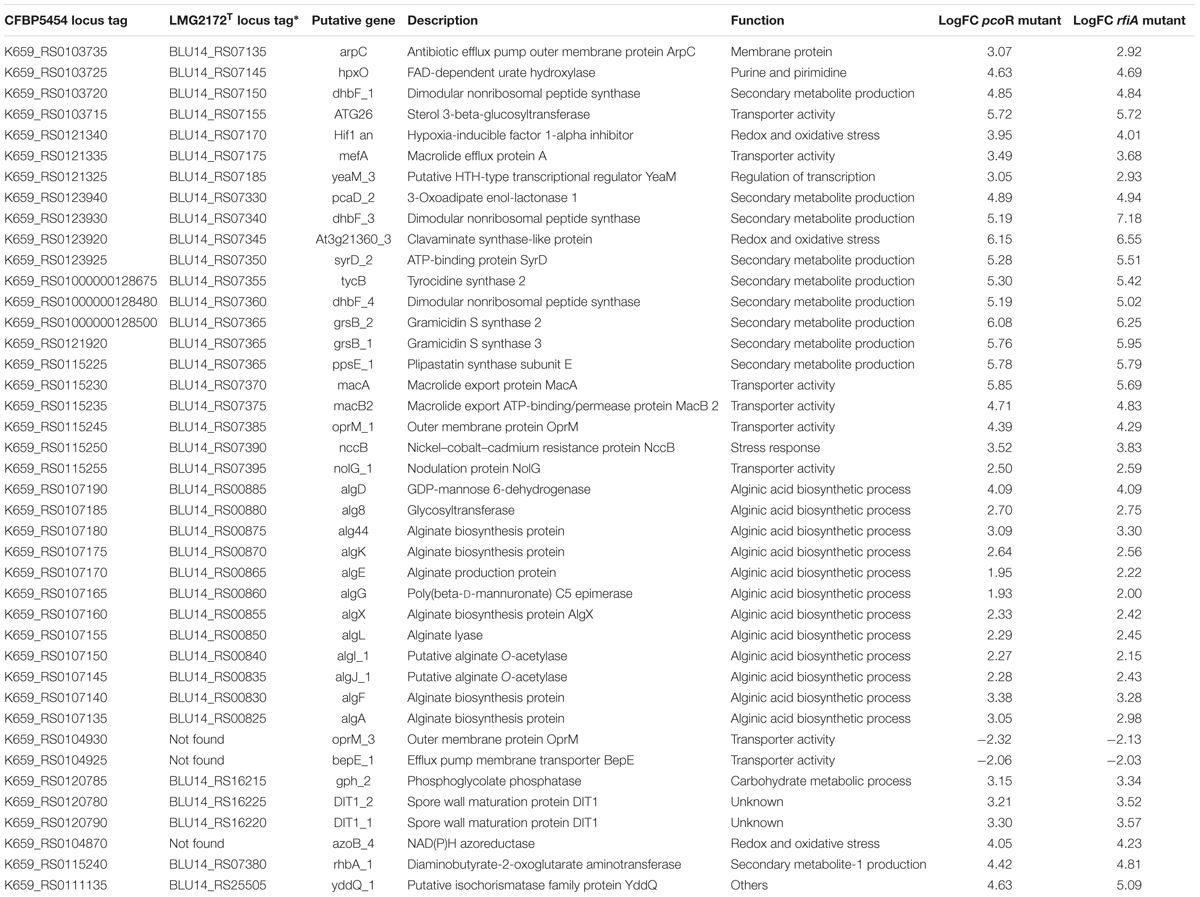
TABLE 2. Locus tag of significantly differentially expressed genes in P. corrugata strain CFBP 5454 derivative mutants pcoR and rfiA and correspondent loci in strain LMG 2172T.
This large cluster includes six NRPS genes most of which were putatively attributed to two closed CLP biosynthetic clusters for the synthesis of corpeptins, 22 amino acid lipopeptides, and the nonapeptide cormycin A. A similar topology was observed for nunapeptins and nunamycin in P. fluorescens In5 (Michelsen et al., 2015; Hennessy et al., 2017) and for thanapeptins, and thanamycin of Pseudomonas sp. SH-C52 (Mendes et al., 2011; Van Der Voort et al., 2015). In addition, in close proximity to the cormycin cluster, a biosynthetic cluster of five genes was identified, which was highly homologous to the CLP brabantamide cluster described in Pseudomonas sp. SH-C52 (Schmidt et al., 2014; Van Der Voort et al., 2015). This biosynthetic cluster is also present in P. fluorescens In5 (Hennessy et al., 2017). BLAST analysis also revealed that 3 of the 21 genes differentially expressed were within the same open-reading frame (ppsE_1; grsB_1; grsB_2). These include all the three putative corpeptin NRPS genes (tycB, dhbF_4, ppsE_1; Table 2) and the two downstream genes coding for an ABC transporter system (macA, macB2; Table 2 and Figure 2A). The genes ppsE_1, macA, and macB have been demonstrated to be part of the same transcriptional unit known as crpCDE (Strano et al., 2015). Insertional mutants in crpC and crpD were no longer able to produce corpeptins, but still produced cormycin (Strano et al., 2015). CrpC was significantly upregulated in the WT strain compared to the pcoR and rfiA mutants by 5.78 and 5.79 Log-fold changes (LogFC), respectively (Table 2 and Supplementary Files 2, 3).
None of the putative cormycin NRPS genes were detected among the differentially expressed genes. However, two genes coding for an ABC transporter system and a gene annotated as syrD_2 were over-expressed in the WT CFBP 5454 strain in comparison with pcoR- and rfiA-mutant strains (5.28- and 5.51-fold, respectively). In addition, among the differentially expressed genes, we identified the yeaM gene coding for an AraC family transcriptional regulator, in proximity of the putative cormycin NRPS genes, overexpressed 3.05- and 2.93-fold in WT compared to the pcoR- and rfiA-mutant strains, respectively. Four out five genes of the putative brabantamide biosynthesis cluster were differentially expressed. Although the production of this metabolite has not yet been described in P. corrugata, it could be argued that previous experimental conditions prevented it from being detected (Emanuele et al., 1998; Scaloni et al., 2004).
Cell-free culture filtrates of the pcoI- and rfiA-mutant strains grown on IMM medium for all RNA extractions didn’t show antimicrobial activity against the two CLP bioindicators, the yeast R. pilimanae ATCC26423 and the Gram-positive bacterium B. megaterium ITM100 (Figure 3A) as opposed to the parent strain. The antagonistic activity was complemented at the same levels as those of the CFBP 5454 strain by expression in trans of the pcoR and rfiA genes into the respective mutant strains. In addition, the expression in trans of rfiA was sufficient to restore the antagonistic activity of the culture filtrate of the pcoR mutant (Figure 3A).
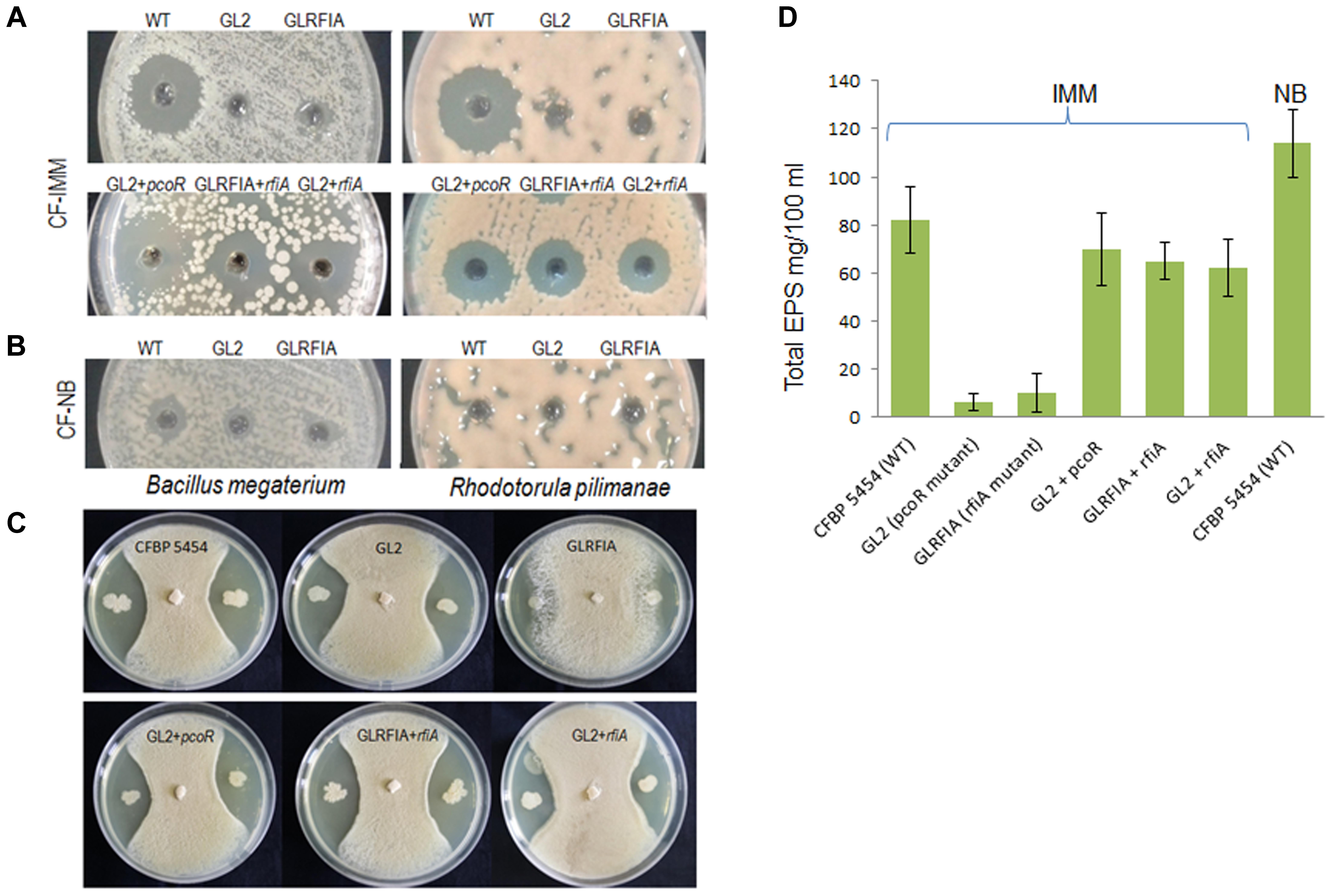
FIGURE 3. Mutational phenotypes of P. corrugata CFBP 5454 and derivatives mutants. (A) Antimicrobial activity of cell culture filtrates (10×) obtained in IMM (CF-IMM) of WT, GL2 (pcoR mutant), and GLRFIA (rfiA mutant) against CLP bioindicators B. megaterium and R. pilimanae. No activity was detected in pcoR and rfiA mutants. Complementation of pcoR (GL2+pcoR), rfiA (GLRFIA+rfiA), and of rfiA in the pcoR mutant (GL2+rfiA) restored antimicrobial activity. (B) Antimicrobial activity of cell culture filtrates (10×) obtained in NB (CF-NB). (C) Antimicrobial activity of bacterial cells of P. corrugata CFBP 5454, pcoR, and rfiA mutants and complemented mutants against Penicillium digitatum. (D) Total EPS produced after 4 days of incubation in IMM by the parent strain, pcoR, and rfiA mutants and complemented mutants and NB (only P. corrugata CFBP 5454).
Figure 3C shows the antimicrobial activity of the living cells of strain CFBP 5454 and also the derivative mutants. It is worth noting that living cells of the two regulatory mutants still demonstrate antimicrobial activity against P. digitatum although to a different extent.
Positive Regulation of the PcoR–RfiA Regulon on the Alginate Biosynthetic Cluster
RNA-seq analysis revealed that 12 genes putatively involved in the EPS alginate biosynthesis were upregulated in strain CFBP 5454 compared to both luxR derivative mutants with LogFC ranging from 4.09 (algD) to 1.93 (algG) in comparison with the pcoR-mutant strain, and from 4.09 (algD) to 2 (algG) to the rfiA-mutant strain (Table 2 and Supplementary Files 2, 3).
In the P. corrugata CFBP 5454 genome, these genes were located in an 18 kb region constituting most of the core structural/biosynthetic cluster (contig38, Figure 2B) except for algC which was located elsewhere (contig86) and is not differentially expressed. This cluster encodes the biosynthetic enzymes and membrane-associated polymerization, modifications, and exports proteins necessary for the alginate production. The order and arrangement of the alginate structural gene cluster in P. corrugata is similar to those already described for P. aeruginosa and P. syringae (Fialho et al., 1990; Shankar et al., 1995; Peñaloza-Vázquez et al., 1997). The expression of other genes implicated in alginate regulation and switching phenotype and dispersed in other parts of the genome were not altered in these mutants (data not shown). No other EPS clusters in Pseudomonas strains, i.e., the pel and psl clusters described in P. aeruginosa (Franklin et al., 2011), the epm cluster, responsible for the production of an alginate-like EPS in P. alkyphenolia (Lee et al., 2014) or levane, were detected in the P. corrugata genome, as assessed by BLAST analysis. Total EPSs were isolated after isopropanol precipitation from the supernatant of the pcoR and rfiA mutant and complemented strains growing in IMM and compared to the P. corrugata parent strain CFBP 5454 (Figure 3D). An approximate 10-fold reduction of EPS yield was recorded in both mutants. The production of EPS was almost restored after complementation of the pcoR and rfiA (P ≤ 0.01) (Figure 3D). As P. corrugata has been demonstrated by Fett et al. (1996) to produce alginate as polymannuronic acid and not levan as EPSs, PcoR, and RfiA would seem to play a role in alginate production regulation.
Other Genes Differentially Expressed in the Two luxR Mutants
In addition to the genes described above, there are eight genes whose expression was significantly modified in both the pcoR and rfiA mutants. Only two of them, oprM_3 and bepE_1, are significantly down-expressed (LogFC ≥-2 and P-value ≤ 0.005) in the WT in comparison with mutant strains and are predicted to codify for multidrugs efflux systems. The ompM_3 gene encodes a putative outer membrane protein and bepE_1 an efflux pump membrane transporter (Table 2). Although they are located adjacently in the same genomic region, there was no evidence of their function. No gene homologs were found in the genome of the LMG 2172T. Three adjacently located genes (DIT1_1, gph_2, and DIT1_2) were over-expressed in the WT and showed more than a three-LogFC in transcript abundances compared to both pcoR and rfiA mutants. Analysis of the DIT1_2 putative protein revealed the presence of a DIT1_PvcA superfamily conserved domain, common to pyoverdine/dityrosine biosynthesis proteins. Blastx analysis showed a 40% homology with PvcA protein of P. aeruginosa, involved in the biosynthesis of the paerucumarin, a new metabolite described as an isonitrile functionalized cumarin (Clarke-Pearson and Brady, 2008). DIT1_1 differed from DIT1_2 in terms of an additional conserved domain belonging to the CAS-like superfamily, responsible for clavaminic acid biosynthesis. The gph_2 gene encodes a putative phosphoglycolate phosphatase. Among the most differentially expressed genes (LogFC > 4 and P-value ≤ 0.005), rhbA_1 also needs mentioning. This gene is a putative diaminobutyrate-2-oxoglutarate aminotransferase located in the ornicorrugatin gene cluster of P. fluorescens SBW25 and in histicorrugatin of P. thivervalensis (Cheng et al., 2013; Matthijs et al., 2016).
Genes Regulated Independently by PcoR and RfiA
A total of 60 and 38 differentially expressed genes were identified in either the pcoR- or rfiA-mutant strains, respectively, mainly associated with transport systems, transcriptional regulation, and redox and oxidative stress. Of these, six transcriptional regulators were over-expressed in the WT in comparison to the pcoR mutant, although at low LogFC (0.77–1.10). Three of them belong to the HTH transcriptional regulator family, whose role to the best of our knowledge has not been investigated. Compared to the rfiA mutant in the WT strain, only one HTH regulator was over-expressed and two were down-expressed (LogFC 1) (Supplementary File 4). We observed that most of these genes had a very low LogFC < 1; thus, we decided to focus only genes with a minimum two LogFC. We found a strong overexpression of the traI gene coding for the AHL synthase (pcoI by Licciardello et al., 2007) in the WT compared to pcoR mutant. Without PcoR, the AHL-QS would not be able to work, since it is strictly dependent on the PcoR–AHL complex, and pcoI is only expressed at the basal level. Three genes involved in copper metabolism are among the most over-expressed in the WT compared to the pcoR mutant (Supplementary File 4). The Cyp4d2 gene, which codes for a cytochrome P450 involved in redox and oxidative stress, was differentially expressed only in the pcoR mutant. It is down-regulated in the WT with a LogFC of -2.4 (Supplementary Files 4, 5).
Validation of the RNA-Seq Expression Patterns by Quantitative Real-Time PCR
Thirteen genes among those co-regulated by PcoR and RfiA, putatively involved in biosynthesis secondary metabolites (six genes) and transport (seven genes), and three genes putatively responsible of alginate biosynthesis, were selected to validate RNA-seq results. qPCR was carried out with gene-specific primers (listed in Supplementary File 1) and the gene expression of WT versus the mutant strains was analyzed. Although there was a difference in the fold change estimated by the two methods (RNA-seq and qPCR), the expression pattern was the same (Figure 4A). A close correlation (Pearson’s R2 = 0.796) was observed between LogFC measured by RNA-seq and qPCR.
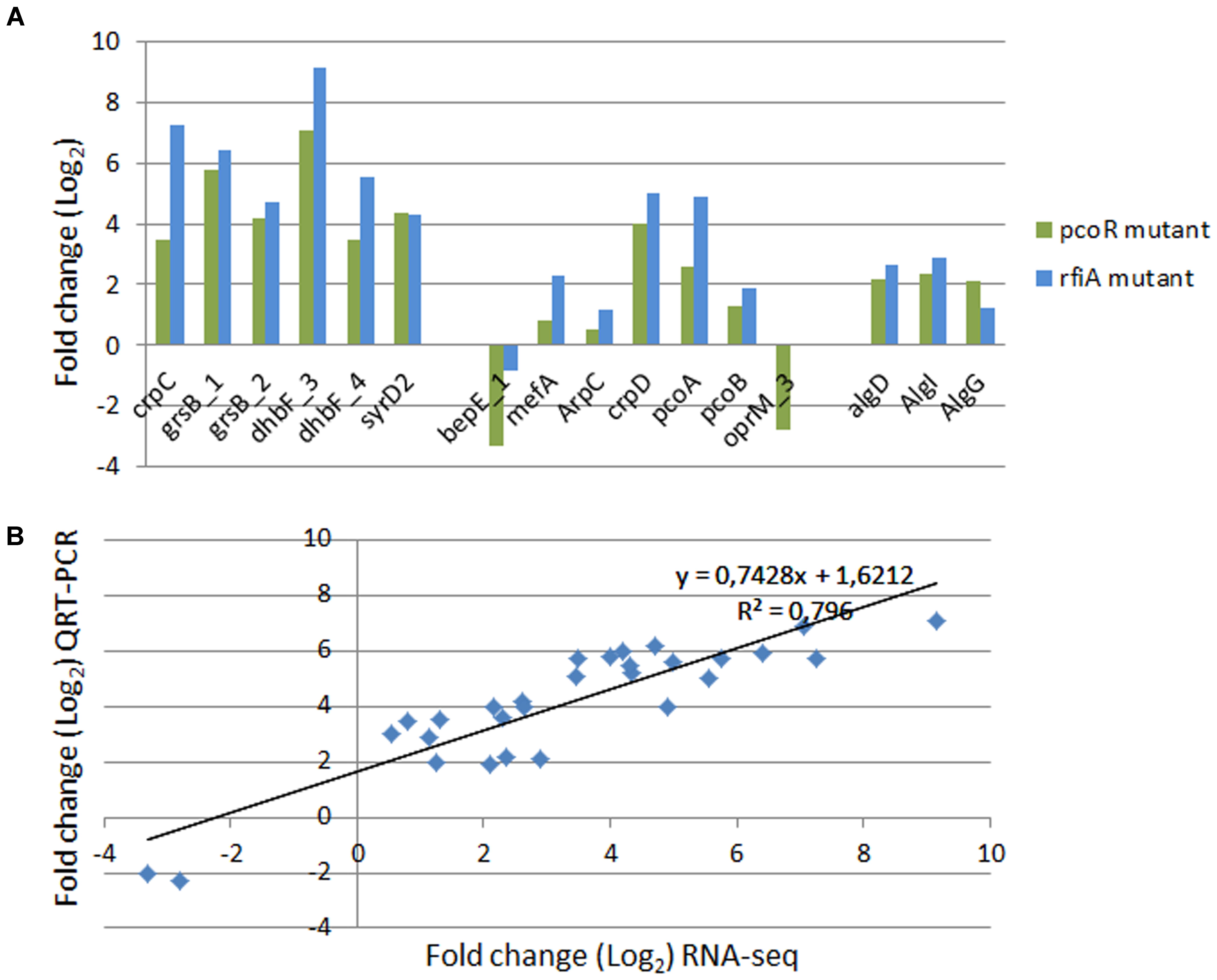
FIGURE 4. Validation by quantitative RT-PCR (qRT-PCR) of transcriptional patterns of randomly selected genes involved in CLPs and alginate production. (A) Transcriptional expression by qPCR in P. corrugata WT strain in comparison with GL2 and GLRFIA mutants grown on IMM for 40 h at the early stationary phase. The expression levels of all genes were standardized to the level of the constitutively expressed housekeeping 16S rDNA and normalized to expression in WT. The results represent the means of three independent experiments. (B) Correlation of estimates fold changes of differentially expressed transcripts between RNA-seq and qPCR analysis.
The data confirmed the positive regulation of PcoR and RfiA of all the selected genes, and the negative regulation of bepE_1 and opmR_3, which were down-regulated in the WT compared to both mutants, in accordance with RNA-seq data.
CLP and Alginate Gene Expression Analysis in Different Media and in Planta by qPCR
Since the RNA-seq experiment relied on conditions known to stimulate CLP production, we investigated the expression of NRP and alginate genes in the P. corrugata CFBP 5454 strain grown in complex undefined medium (NB) and in planta. The results demonstrated that genes involved in NRP biosynthesis and transport were activated two to sixfold more in minimal medium compared to NB, and two to five in planta compared to NB medium (Figure 5). Thus, cell culture filtrates of CFBP 5454 grown on NB showed very little or no activity against B. megaterium and R. piliminae, respectively (Figure 3B). AlgG gene in P. corrugata CFBP 5454 was upregulated both in NB and in planta compared to IMM. Total EPS production was higher in NB (114 ± 14 mg/100 ml) compared to IMM (82 ± 14 mg/100 ml), showing that rich medium provides better conditions for EPS production (Figure 3D).
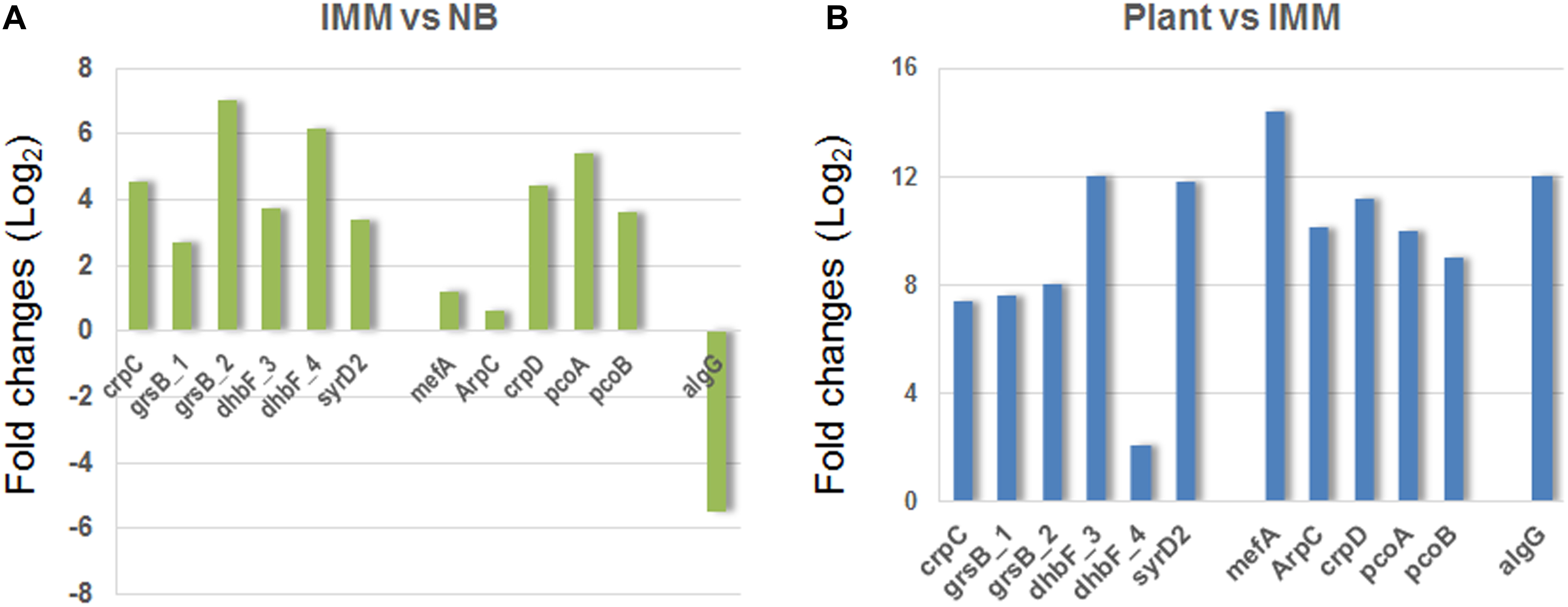
FIGURE 5. Relative expression of a subset of randomly selected genes from those already validated involved in CLPs and alginate production in P. corrugata CFBP 5454 grown in a rich medium, i.e., Nutrient broth (NB) (A) and in inoculated tomato plantlets (B) in comparison with growth in IMM by qPCR.
Discussion
In this study, we performed an in vitro transcriptome study to investigate the role of PcoR and RfiA LuxR-type transcriptional regulators in P. corrugata secondary metabolite production. Based on our previous results, we speculated that the two regulators play a pivotal role in the regulation of CLP biosynthetic loci, since cormycin and corpeptin production was impaired in P. corrugata CFBP 5454 derivative mutants (Licciardello et al., 2012). The overlapping of the RNA-seq data showed that approximately 50% of the genes cataloged in this study (approximately 3% of the annotated genes in the CFBP 5454 genome) were differentially expressed both in the pcoR- and rfiA-mutant strains compared to the P. corrugata CFBP 5454 parent strain. PcoR is the cognate receptor of AHLs, synthesized by PcoI in the P. corrugata QS system (Licciardello et al., 2007). In line to our previous results, the PcoR–AHL complex directly activates the transcriptional regulator gene rfiA since it is co-transcribed with pcoI (Licciardello et al., 2009). Thus, it is conceivable that this set of genes is co-regulated by both the LuxR-type transcriptional regulators.
Only 42 out of the 92 genes showed high log-fold-change values and were positively regulated, and only two genes were negatively regulated. Interestingly, almost all of the genes positively regulated are putatively involved in secondary metabolite production, namely genes involved in the biosynthesis of antimicrobial CLPs and in the production of alginic acid. As hypothesized, among these genes we found some whose involvement in the production of CLPs was clear and others whose involvement was likely.
Like many other biologically active secondary metabolites, CLPs are synthesized by multifunctional NRPSs (Raaijmakers et al., 2006). It is estimated that approximately 3 kb of DNA are required to code each amino acid activation module (Gross and Loper, 2009). Thus, due to the incomplete nature of the P. corrugata strain CFBP 5454 genome, the large CLP NRPs are divided into different contigs (Licciardello et al., 2014; Trantas et al., 2015). By conducting a BLAST analysis of genes down-regulated in P. corrugata CFBP 5454 pcoR and rfiA mutants and by genome mining the whole-genome sequence of strain LMG 2172T in the GenBank repository, we ascertained that 19 differentially regulated genes were located in a large cluster which accounted for approximately 3.4% of the genome. This large DNA region includes putative gene clusters for cormycin and corpeptin and a brabantamide-like metabolite. This is consistent with the gene organization present in the biocontrol strain Pseudomonas SH-C52 for the thanapeptins, thanamycin, and brabantamide (Van Der Voort et al., 2015). In addition, P. syringae pv. syringae and P. fluorescens In5 produce both CLPs characterized by long peptide chains and smaller nonapeptides. Their biosynthesis clusters are adjacently located in the genome as in the case of syringopeptins and syringomycin (Scholz-Schroeder et al., 2001) and nunamycin and nunapeptins, respectively (Hennessy et al., 2017).
PcoR and RfiA regulate the genes for the three NRPSs necessary for the biosynthesis of corpeptins and the downstream located ABC transporter (crpDE). Some of these genes were described by Strano et al. (2015) who named them crpCDE, i.e., genes that are transcriptionally joined and which code for an NRPS and ABC efflux system. The introduction of a mutation in crpC yielded a P. corrugata strain, PCONRPS, which failed to produce corpeptins, thus demonstrating that crpC is part of the corpeptin biosynthesis locus. Gene disruption of crpD also affected the presence of corpeptins in the culture filtrates of P. corrugata CFBP 5454, supporting the assumption that CrpDE is the transport system involved in corpeptin export (Strano et al., 2015). Although the pcoR and rfiA mutants grown in the same conditions also failed to produce cormycin (Licciardello et al., 2012), we found none of the cormycin NRPS genes among the differentially expressed genes. Nevertheless, more genes that may be putatively involved in cormycin production were positively controlled by PcoR and RfiA. These included both a putative ABC transporter system, which is highly homologous to trasporters for the nonapeptides, syringomycin, and thanamycin in P. syringae B301D, B728A, and in Pseudomonas SHC52, and a gene annotated as syrD_2 coding an ATP-binding protein (Kang and Gross, 2005; Van Der Voort et al., 2015; Vaughn and Gross, 2016). SyrD flanks the corpeptin NRPS genes in P. corrugata and the syringopeptin gene cluster in P. syringae B301D. In the latter species syrD forms an operon with sypA and sypB NRPS genes; however, it is necessary for the secretion of both syringomycin and syringopeptin (Quigley et al., 1993; Wang et al., 2006a,b). In line with the possible similar organization of the transcriptional units, similar values of differential expression for putative syrD, crpA, and crpB (LogFC 5.19–5.30) were observed.
In previous works (Licciardello et al., 2007, 2009, 2012; Strano et al., 2015, 2017), we demonstrated that the expression of the cosmid pLC3.34 in pcoR mutant and of the plasmid pBBRRfia in rfiA mutant could complement the relative mutations, by restoring the virulence in tomato, the hypersensitivity response on Nicotiana spp., and the antimicrobial activity. The expression in trans of rfiA in the pcoR mutant was able to restore the virulence of the mutant at a similar level to the parent strain (Licciardello et al., 2009). We thus showed that the culture filtrates of the replicates of the mutant strains grown in IMM used for RNA-seq and qPCR were depleted in antimicrobial activity against CLP bioindicator strains. The activity is restored by complementation. Based on these results, RfiA is sufficient to also restore the investigated phenotypes in the pcoR mutant.
We previously demonstrated that the pcoABC operon, which is located in the CLP large cluster, is positive regulated by RfiA and, indirectly, by the PcoI/R system. RNA-seq data corroborated by qPCR validation data and phenotype complementation suggest that the regulation of P. corrugata genes in the PcoR–RfiA regulon may occur according to a hierarchical model. When a sufficient AHL signal has accumulated in the surrounding environment, it binds to PcoR and the complex upregulates pcoI gene in a positive feedback loop and consequently rfiA. RfiA, in turn, may activate the transcription of a number of genes either directly or indirectly. CLP biosynthesis clusters in Pseudomonas are flanked by multiple genes coding for LuxR transcriptional regulators (reviewed in Raaijmakers et al., 2010). Until recently the presence of a LuxR regulator directly linked to an AHL-QS system by gene cotranscription with acyl-homoserine lactone synthase gene has only been described for P. corrugata, P. mediterranea, and Pseudomonas sp. strain DF41 (Licciardello et al., 2009, 2012; Berry et al., 2014). Genes of the QS-RfiA system have been found to be conserved in P. corrugata and P. mediterranea (Trantas et al., 2015). This system is also conserved in other P. corrugata, P. mediterranea strains, and in Pseudomonas sp. SC-H52. P. corrugata CFBP 5454 PcoI, PcoR, and RfiA showed 100% protein homologies with the corresponding proteins in P. corrugata strain LMG 2172T, 85%, 95%, and 94% with strain Pseudomonas sp. SHC52, and of 84%, 95%, and 92% with P. mediterranea DSM16733T, respectively (data not shown).
The conservation of the QS system in this group of taxonomically related bacteria could have a biological significance. All of them have biocontrol properties mediated by the production of antimicrobial peptides. However, P. corrugata and P. mediterranea have been isolated as plant pathogens and are widespread pathogens in tomatoes (Catara, 2007). No type III secretion system or type III effectors are present in their genomes and the only relevant information regarding their interaction with plants is based on the pivotal role of QS and RfiA in virulence and in the hypersensitivity response in a non-host plant species and the putative/deduced role of CLPs in this interaction (Licciardello et al., 2009, 2014; Strano et al., 2015; Trantas et al., 2015). The transcriptomic data enlarge the number of secondary metabolites which are under the control of PcoR and RfiA in P. corrugata and for which a role in planta interaction needs to be further investigated. The high-density injection of P. corrugata cells in the stems of plant species belonging to different families led to pith necrosis. However, in nature the disease is widespread essentially in tomato (Siverio et al., 1993; Catara et al., 1997, 2002; Sutra et al., 1997; Catara, 2007). It is therefore conceivable that only in tomato can the bacterium reach the “quorum” cellular concentration required for the hierarchical activation of the genes under the QS control via RfiA including the metabolites with a phytotoxic activity that lead to the necrotrofic colonization of the plant resulting in TPN.
The role of plant signals in triggering the production of syringomycin and syringopeptins in the phytopathogen P. syringae pv. syringae via the sensor kinase GacS and the LuxR-type transcriptional regulators SalA and SyrF has been demonstrated (Mo and Gross, 1991; Wang et al., 2006a). As already reported for other Pseudomonas, the integration and networking of additional regulatory circuits may help P. corrugata to interact with environmental and metabolic signals in order to define the timing of the cell-based activation of QS (Fuqua et al., 1996; Venturi, 2006; Uzelac et al., 2017). In Pseudomonas, sp. strain DF41 AHL production and pdfI expression are under the positive control of the Gac/Rsm system (Berry et al., 2014).
Although CLPs produced by P. corrugata have a strong antimicrobial activity it seems that several other metabolites that are not regulated by PcorR and RfiA are involved in biocontrol activity. In fact, the cell-free culture filtrates of PcoR and rfiA mutants grown in IMM did not contain corpeptins and cormycin (Licciardello et al., 2012). In line with this result the two mutants did not have antimicrobial activity against the bioindicator strains even at 10-fold concentrations. The complementation of rfiA in the pcoR mutant is sufficient to restore antimicrobial activity thus demonstrating that at least its presence is necessary for the production of CLPs. Nevertheless, tests using bacterial cells suggest that more antimicrobial metabolites still have to be produced by the two mutant bacterial strains that are not regulated by PcoR and RfiA. Genome mining highlighted the presence of clusters for other metabolites, including the siderophore corrugatin, which could be involved in antimicrobial activity (Trantas et al., 2015). In addition, the role of volatile compounds in antagonistic activity has already been demonstrated (Trivedi et al., 2008; Strano et al., 2015).
PcoR and RfiA positively influence alginic acid biosynthesis gene expression. We found that almost all the genes belonging to the structural/biosynthetic cluster of the EPS alginate were upregulated in the WT compared to the pcoR and rfiA mutants. The production of alginate and not of levan has been demonstrated in a number of P. corrugata strains (Fett et al., 1996). Further characterization has shown that alginate consists solely of uronic acid (100% w/v) and mannose (Fett et al., 1996). Our analysis of the P. corrugata CFBP 5454 genome revealed that, similarly to other Pseudomonas spp. that belong to the rRNA homology group I (Fett et al., 1992), alginate biosynthesis and regulatory genes are widely distributed over three clusters namely, structural/biosynthetic, regulatory, and genetic switching genes (data not shown). A similar cluster has been found in the closely related species P. mediterranea (Licciardello et al., 2017) and Pseudomonas sp. SH-C52 genomes (Van Der Voort et al., 2015). Total EPSs were reduced in the two mutant strains analyzed in this study.
According to Fett et al. (1992, 1996) only alginate is produced by P. corrugata because there is no evidence of other gene clusters responsible for the synthesis of other EPS in other Pseudomonas species (Bradbury, 1986; Franklin et al., 2011). Alginate production is regulated by AHL in diverse Pseudomonas, including the phytopathogen P. syringae B728a in which it contributes to epiphytic fitness and resistance to desiccation, and increases resistance to oxidative stress (Quinones et al., 2005; Venturi, 2006). However, no significant effect of the salA mutation on alginate gene expression has been observed in P. syringae B301D (Wang et al., 2006b).
In addition, P. corrugata CFBP 5454 strain expression of some selected genes of the biosynthetic cluster for CLPs as investigated by qPCR was upregulated when the bacterium was grown in minimal medium compared to a rich complex undefined medium (NB). The opposite was observed for the expression of alginate gene algG. Genes coding for both secondary metabolites are overexpressed in planta compared to in vitro growth. These include genes for the biosynthesis of corpeptins, which are known to play a role in virulence in tomato (Strano et al., 2015).
Our results suggest that the QS-RfiA system in P. corrugata regulates hierarchically important secondary metabolites production at a high cell concentration. We focused on these metabolites since they play a pivotal role in the bacterial fitness of plant-associated bacteria in the interaction with other microorganisms as well as plants. RNA-seq generated a considerable amount of data, which merit future attention. However, it will be difficult to define the role of those genes for which differential values of expression are very low. Although their regulation is likely to depend on more intricate regulation networks influencing the fitness of the bacterium.
Author Contributions
GL and VC conceived the study, and contributed to its design and coordination, and drafted the manuscript. AC contributed to the design and execution of RNA-seq data elaboration, performed and analyzed the RT-PCR experiments and phenotypic analysis. PB, CS, and AA contributed to mutants analysis by molecular and phenotypic assays. AC, PS, ET, GL, VC, NA, and RG contributed to the transcript data elaboration, genome comparison, and bioinformatics analysis. NA and RG contributed materials and bioinformatic tools. All authors contributed to the writing and editing of the manuscript and approved the final version of it.
Conflict of Interest Statement
The authors declare that the research was conducted in the absence of any commercial or financial relationships that could be construed as a potential conflict of interest.
Acknowledgments
GL and VC were supported by the Ministry of Education, University and Research (MIUR) by means of the National Program PON R&C 2007–2013, co-funded by EU, project “PolyBioPlast – Technologies and processes for the production of diversely functionalised sheets based on microbial biopolymers and biosurfactants (PON01_1377)”.
Supplementary Material
The Supplementary Material for this article can be found online at: https://www.frontiersin.org/articles/10.3389/fmicb.2018.00521/full#supplementary-material
Footnotes
References
Altschul, S. F., Gish, W., Miller, W., Myers, E. W., and Lipman, D. J. (1990). Basic local alignment search tool. J. Mol. Biol. 215, 403–410. doi: 10.1016/S0022-2836(05)80360-2
Ashburner, M., Ball, C. A., Blake, J. A., Botstein, D., Butler, H., Cherry, J. M., et al. (2000). Gene ontology: tool for the unification of biology. The Gene Ontology Consortium. Nat. Genet. 25, 25–29. doi: 10.1038/75556
Bender, C. L., Alarcon-Chaidez, F., and Gross, D. C. (1999). Pseudomonas syringae phytotoxins: mode of action, regulation, and biosynthesis by peptide and polyketide synthetases. Microbiol. Mol. Biol. Rev. 63, 266–292.
Berry, C., Nandi, M., Manuel, J., Brassinga, A. K. C., Fernando, W. G. D., Loewen, P. C., et al. (2014). Characterization of the Pseudomonas sp. DF41 quorum sensing locus and its role in fungal antagonism. Biol. Control 69, 82–89. doi: 10.1016/j.biocontrol.2013.11.005
Berti, A. D., Greve, N. J., Christensen, Q. H., and Thomas, M. G. (2007). Identification of a biosynthetic gene cluster and the six associated lipopeptides involved in swarming motility of Pseudomonas syringae pv. tomato DC3000. J. Bacteriol. 189, 6312–6323. doi: 10.1128/JB.00725-07
Blin, K., Medama, M. H., Kazempour, D., Fischbach, M. A., Breitling, R., Takano, E., et al. (2013). antiSMASH 2.0—a versatile platform for genome mining of secondary metabolite producers. Nucleic Acids Res. 41, W204–W212. doi: 10.1093/nar/gkt449
Catara, V. (2007). Pseudomonas corrugata: plant pathogen and/or biological resource? Mol. Plant Pathol. 8, 233–244. doi: 10.1111/j.1364-3703.2007.00391.x
Catara, V., Gardan, L., and Lopez, M. M. (1997). Phenotypic heterogeneity of Pseudomonas corrugata strains from southern Italy. J. Appl. Microbiol. 83, 576–586. doi: 10.1046/j.1365-2672.1997.00268.x
Catara, V., Sutra, L., Morineeau, A., Achouak, W., Christan, R., and Gardan, L. (2002). Phenotypic and genomic evidence for the revision of Pseudomonas corrugata and proposal of Pseudomonas mediterranea species sp. nov. Int. J. Syst. Evol. Microbiol. 52, 1749–1758.
Chen, J., and Xie, J. (2011). Role and regulation of bacterial LuxR-like regulators. J. Cell. Biochem. 112, 2694–2702. doi: 10.1002/jcb.23219
Cheng, X., de Bruijn, I., van der Voort, M., Loper, J. E., and Raaijmakers, J. M. (2013). The Gac regulon of Pseudomonas fluorescens SBW25. Environ. Microbiol. Rep. 5, 608–619. doi: 10.1111/1758-2229.12061
Clarke-Pearson, M. F., and Brady, S. F. (2008). Paerucumarin, a new metabolite produced by the pvc gene cluster from Pseudomonas aeruginosa. J. Bacteriol. 190, 6927–6930. doi: 10.1128/JB.00801-08
Conte, E., Catara, V., Greco, S., Russo, M., Alicata, R., Strano, L., et al. (2006). Regulation of polyhydroxyalkanoate synthases (phaC1 and phaC2) gene expression in Pseudomonas corrugata. Appl. Microbiol. Biotechnol. 72, 1054–1062. doi: 10.1007/s00253-006-0373-y
Cui, X., Harling, R., Mutch, P., and Darling, D. (2005). Identification of N-3-hydroxyoctanoyl-homoserine lactone production in Pseudomonas fluorescens 5064, pathogenic to broccoli, and controlling biosurfactant production by quorum sensing. Eur. J. Plant Pathol. 111, 297–308. doi: 10.1007/s10658-004-4171-z
de Bruijn, I., de Kock, M. J. D., de Waard, P., van Beek, T. A., and Raaijmakers, J. M. (2008). Massetolide A biosynthesis in Pseudomonas fluorescens. J. Bacteriol. 190, 2777–2789. doi: 10.1128/JB.01563-07
de Bruijn, I., de Kock, M. J. D., Yang, M., de Waard, P., van Beek, T. A., and Raaijmakers, J. M. (2007). Genome-based discovery, structure prediction and functional analysis of cyclic lipopeptide antibiotics in Pseudomonas species. Mol. Microbiol. 63, 417–428. doi: 10.1111/j.1365-2958.2006.05525.x
de Bruijn, I., and Raaijmakers, J. M. (2009a). Diversity and functional analysis of LuxR-type transcriptional regulators of cyclic lipopeptide biosynthesis in Pseudomonas fluorescens. Appl. Environ. Microbiol. 75, 4753–4761. doi: 10.1128/AEM.00575-09
de Bruijn, I., and Raaijmakers, J. M. (2009b). Regulation of cyclic lipopeptide biosynthesis in Pseudomonas fluorescens by the ClpP protease. J. Bacteriol. 191, 1910–1923. doi: 10.1128/JB.01558-08
Dubern, J. F., Lagendijk, E. L., Lugtenberg, B. J. J., and Bloemberg, G. V. (2005). The heat shock genes dnaK, dnaJ, and grpE are involved in regulation of putisolvin biosynthesis in Pseudomonas putida PCL1445. J. Bacteriol. 187, 5967–5976. doi: 10.1128/JB.187.17.5967-5976.2005
Dubern, J. F., Lugtenberg, B. J. J., and Bloemberg, G. V. (2006). The ppuI-rsaL-ppuR quorum-sensing system regulates biofilm formation of Pseudomonas putida PCL1445 by controlling biosynthesis of the cyclic lipopeptides putisolvins I and II. J. Bacteriol. 188, 2898–2906. doi: 10.1128/JB.188.8.2898-2906.2006
Emanuele, M. C., Scaloni, A., Lavermicocca, P., Jacobellis, N. S., Camoni, L., Di Giorgio, D., et al. (1998). Corpeptins, new bioactive lipodepsipeptides from cultures of Pseudomonas corrugata. FEBS Lett. 433, 317–320. doi: 10.1016/S0014-5793(98)00933-8
Fett, W. F., Cescutti, P., and Wijey, C. (1996). Exopolysaccharides of the plant pathogens Pseudomonas corrugata and Ps. flavescens and the saprophyte Ps. chlororaphis. J. Appl. Bacteriol. 81, 181–187. doi: 10.1111/j.1365-2672.1996.tb04497.x
Fett, W. F., Wijey, C., and Lifson, E. R. (1992). Occurrence of alginate gene sequences among members of the pseudomonad rRNA homology groups I-IV. FEMS Microbiol. Lett. 78, 151–157.
Fialho, A. M., Zielinski, N. A., Fett, W. F., Chakrabarty, A. M., and Berry, A. (1990). Distribution of alginate gene sequences in the Pseudomonas rRNA homology group I-Azomonas-Azotobacter lineage of superfamily B procaryotes. Appl. Environ. Microbiol. 56, 436–443.
Finking, R., and Marahiel, M. A. (2004). Biosynthesis of nonribosomal peptides 1. Annu. Rev. Microbiol. 58, 453–488. doi: 10.1146/annurev.micro.58.030603.123615
Franklin, M. J., Nivens, D. E., Weadge, J. T., and Lynne Howell, P. (2011). Biosynthesis of the Pseudomonas aeruginosa extracellular polysaccharides, alginate, Pel, and Psl. Front. Microbiol. 2:167. doi: 10.3389/fmicb.2011.00167
Fuqua, C., Winans, S. C., and Greenberg, E. P. (1996). Census and consensus in bacterial ecosystems: the LuxR-LuxI family of quorum-sensing transcriptional regulators. Annu. Rev. Microbiol. 50, 27–751. doi: 10.1146/annurev.micro.50.1.727
Gross, H., and Loper, J. E. (2009). Genomics of secondary metabolite production by Pseudomonas spp. Nat. Prod. Rep. 26, 1408–1446. doi: 10.1039/b817075b
Hennessy, R. C., Glaring, M. A., Olsson, S., and Stougaard, P. (2017). Transcriptomic profiling of microbe–microbe interactions reveals the specific response of the biocontrol strain P. fluorescens In5 to the phytopathogen Rhizoctonia solani. BMC Res. Notes. 10:376. doi: 10.1186/s13104-017-2704-8
Kang, H., and Gross, D. C. (2005). Characterization of a resistance nodulation cell division transporter system associated with the syr syp genomic island of Pseudomonas syringae pv. syringae. Appl. Environ. Microbiol. 71, 5056–5065. doi: 10.1128/AEM.71.9.5056-5065.2005
Lavermicocca, P., Iacobellis, N. S., Sinunaco, M., and Graniti, A. (1997). Biological properties and spectrum of activity of Pseudomonas syringae pv. syringae toxins. Physiol. Mol. Plant Pathol. 50, 129–140. doi: 10.1006/pmpp.1996.0078
Lee, K., Lim, E. J., Kim, K. S., Huang, S. L., Veeranagouda, Y., and Rehm, B. H. (2014). An alginate-like exopolysaccharide biosynthesis gene cluster involved in biofilm aerial structure formation by Pseudomonas alkylphenolia. Appl. Microbiol. Biotechnol. 98, 4137–4148. doi: 10.1007/s00253-014-5529-6
Licciardello, G., Bertani, I., Steindler, L., Bella, P., Venturi, V., and Catara, V. (2007). Pseudomonas corrugata contains a conserved N acyl homoserine lactone quorum sensing system; its role in tomato pathogenicity and tobacco hypersensitivity response. FEMS Microbiol. Ecol. 61, 222–234. doi: 10.1111/j.1574-6941.2007.00338.x
Licciardello, G., Bertani, I., Steindler, L., Bella, P., Venturi, V., and Catara, V. (2009). The transcriptional activator rfiA is quorum-sensing regulated by cotranscription with the luxI homolog pcoI and is essential for plant virulence in Pseudomonas corrugata. Mol. Plant Microbe Interact. 22, 1514–1522. doi: 10.1094/MPMI-22-12-1514
Licciardello, G., Ferraro, R., Russo, M., Strozzi, F., Catara, A., Bella, P., et al. (2017). Transcriptome analysis of Pseudomonas mediterranea and P. corrugata plant pathogens during accumulation of medium-chain-length PHAs by glycerol bioconversion. New Biotechnol. 37, 39–47. doi: 10.1016/j.nbt.2016.07.006
Licciardello, G., Jackson, R. W., Bella, P., Strano, C. P., Catara, A. F., Arnold, D. L., et al. (2014). Draft genome sequence of Pseudomonas corrugata, a phytopathogenic bacterium with potential industrial applications. J. Biotechnol. 175, 65–66. doi: 10.1016/j.jbiotec.2014.02.003
Licciardello, G., Strano, C. P., Bertani, I., Bella, P., Fiore, A., Fogliano, V., et al. (2012). N-acyl-homoserine-lactone quorum sensing in tomato phytopathogenic Pseudomonas spp. is involved in the regulation of lipodepsipeptide production. J. Biotechnol. 159, 274–282. doi: 10.1016/j.jbiotec.2011.07.036
Lima, R., Nishibe, C., Raiol, T., and Almeida, N. F. (2016). DEPICTViz – Differential Expression and Protein InteraCTions Visualization Tool. Buenos Aires: ISCB-Latin America.
Livak, K. J., and Schmittgen, T. D. (2001). Analysis of relative gene expression data using real-time quantitative PCR and the 2(-ΔΔCT) method. Methods 25, 402–408. doi: 10.1006/meth.2001.1262
Lu, S. E., Scholz-Schroeder, B. K., and Gross, D. C. (2002). Characterization of the salA, syrF, and syrG regulatory genes located at the right border of the syringomycin gene cluster of Pseudomonas syringae pv. syringae. Mol. Plant Microbe Interact. 15, 43–53. doi: 10.1094/MPMI.2002.15.1.43
Matthijs, S., Brandt, N., Ongena, M., Achouak, W., Meyer, J. M., and Budzikiewicz, H. (2016). Pyoverdine and histicorrugatin-mediated iron acquisition in Pseudomonas thivervalensis. Biometals 29, 467–485. doi: 10.1007/s10534-016-9929-1
Mendes, R., Kruijt, M., DeBruijn, I., Dekkers, E., Van Der Voort, M., Schneider, J. H., et al. (2011). Deciphering the rhizosphere microbiome for disease-suppressive bacteria. Science 332, 1097–1100. doi: 10.1126/science.1203980
Michelsen, C. F., Watrous, J., Glaring, M. A., Kersten, R., Koyama, N., Dorrestein, et al. (2015). Nonribosomal peptides, key biocontrol components for Pseudomonas fluorescens In5, isolated from a Greenlandic suppressive soil. MBio 6:e00079-15. doi: 10.1128/mBio.00079-15
Mo, Y. Y., and Gross, D. C. (1991). Plant signal molecules activate the syrB gene, which is required for syringomycin production by Pseudomonas syringae pv. syringae. J. Bacteriol. 173, 5784–5792. doi: 10.1128/jb.173.18.5784-5792.1991
Olorunleke, F. E., Kieu, N. P., De Waele, E., Timmerman, M., Ongena, M., and Höfte, M. (2017). Coregulation of the cyclic lipopeptides orfamide and sessilin in the biocontrol strain Pseudomonas sp. CMR12a. Microbiologyopen 2017:e499. doi: 10.1002/mbo3.499
Peñaloza-Vázquez, A., Kidambi, S. P., Chakrabarty, A. M., and Bender, L. (1997). Characterization of the alginate biosynthetic gene cluster in Pseudomonas syringae pv. syringae. J. Bacteriol. 179, 4464–4472. doi: 10.1128/jb.179.14.4464-4472.1997
Quigley, N. B., Mo, Y. Y., and Gross, D. C. (1993). SyrD is required for syringomycin production by Pseudomonas syringae pathovar syringae and is related to a family of ATP-binding secretion proteins. Mol. Microbiol. 9, 787–801. doi: 10.1111/j.1365-2958.1993.tb01738.x
Quinones, B., Dulla, G., and Lindow, S. E. (2005). Quorum sensing regulates exopolysaccharide production, motility, and virulence in Pseudomonas syringae. Mol. Plant Microbe Interact. 18, 682–693. doi: 10.1094/MPMI-18-0682
Raaijmakers, J. M., de Bruijn, I., and De Kock, M. J. D. (2006). Cyclic lipopeptide production by plant-associated Pseudomonas spp.: diversity, activity, biosynthesis, and regulation. Mol. Plant Microbe Interact. 19, 699–710. doi: 10.1094/MPMI-19-0699
Raaijmakers, J. M., de Bruijn, I., Nybroe, O., and Ongena, M. (2010). Natural functions of lipopeptides from Bacillus and Pseudomonas: more than surfactants and antibiotics. FEMS Microbiol. Rev. 34, 1037–1062. doi: 10.1111/j.1574-6976.2010.00221.x
Risse, D., Beiderbeck, H., Taraz, K., Budzikiewicz, H., and Gustine, D. (1998). Bacterial constituents part LXXVII. Corrugatin, a lipopeptide siderophore from Pseudomonas corrugata. Z Naturforsch. C 53, 295–304.
Scaloni, A., Dalla Serra, M., Amodeo, P., Mannina, L., Vitale, R. M., Segre, A. L., et al. (2004). Structure, conformation and biological activity of a novel lipodepsipeptide from Pseudomonas corrugata: cormycin A. Biochem. J. 384, 25–36. doi: 10.1042/BJ20040422
Scarlett, C. M., Fletcher, J. T., Roberts, P., and Lelliott, R. A. (1978). Tomato pith necrosis caused by Pseudomonas corrugata n. sp. Ann. Appl. Biol. 88, 105–114. doi: 10.1111/j.1744-7348.1978.tb00684.x
Schmidt, Y., Van Der Voort, M., Crusemann, M., Piel, J., Josten, M., Sahl, H. G., et al. (2014). Biosynthetic origin of the antibiotic cyclocarbamate brabantamide A (SB-253514) in plant-associated Pseudomonas. Chem. Biochem. 15, 259–266.
Scholz-Schroeder, B. K., Soule, J. D., Lu, S. E., Grgurina, I., and Gross, D. C. (2001). A physical map of the syringomycin and syringopeptin gene clusters localized to an approximately 145-kb DNA region of Pseudomonas syringae pv. syringae strain B301D. Mol. Plant Microbe Interact. 14, 1426–1435. doi: 10.1094/MPMI.2001.14.12.1426
Shankar, S., Ye, R. W., Schlictman, D., and Chakrabarty, A. M. (1995). Exopolysaccharide alginate synthesis in Pseudomonas aeruginosa: enzymology and regulation of gene expression. Adv. Enzymol. Relat. Areas Mol. Biol. 70, 221–255. doi: 10.1002/9780470123164.ch4
Siverio, F., Cambra, M., Gorris, M. T., Corzo, J., and Lopez, M. M. (1993). Lipopolysaccharides as determinants of serological variability in Pseudomonas corrugata. Appl. Environ. Microbiol. 59, 1805–1812.
Strano, C. P., Bella, P., Licciardello, G., Caruso, A., and Catara, V. (2017). Role of secondary metabolites in the biocontrol activity of Pseudomonas corrugata and Pseudomonas mediterranea. Eur. J. Plant Pathol. 149, 103–111. doi: 10.1007/s10658-017-1169-x
Strano, C. P., Bella, P., Licciardello, G., Fiore, A., Lo Piero, A. R., Fogliano, V., et al. (2015). Pseudomonas corrugata crpCDE is part of the cyclic lipopeptide corpeptin biosynthetic gene cluster and is involved in bacterial virulence in tomato and in hypersensitive response in Nicotiana benthamiana. Mol. Plant Pathol. 16, 495–506. doi: 10.1111/mpp.12207
Subramoni, S., and Venturi, V. (2009). LuxR-family “solos”: bachelor sensors/regulators of signalling molecules. Microbiology 155, 1377–1385. doi: 10.1099/mic.0.026849-0
Surico, G., Lavermicocca, P., and Iacobellis, N. S. (1988). Produzione di siringomicina e di siringotossina in colture di Pseudomonas syringae pv. syringae. Phytopathol. Mediterr. 27, 163–168.
Sutra, L., Siverio, F., Lopez, M. M., Hanault, G., Bollet, C., and Gardan, L. (1997). Taxonomy of Pseudomonas strains isolated from tomato pith necrosis: emended description of Pseudomonas corrugata and proposal of three unnamed fluorescent Pseudomonas genomospecies. Int. J. System Bacteriol. 47, 1020–1033. doi: 10.1099/00207713-47-4-1020
Trantas, E. A., Licciardello, G., Almeida, N. F., Witek, K., Strano, C. P., Duxbury, Z., et al. (2015). Comparative genomic analysis of multiple strains of two unusual plant pathogens: Pseudomonas corrugata and Pseudomonas mediterranea. Front. Microbiol. 6:811. doi: 10.3389/fmicb.2015.00811
Trivedi, P., Pandey, A., and Palni, L. M. S. (2008). In vitro evaluation of antagonistic properties of Pseudomonas corrugata. Microbiol. Res. 163, 329–336. doi: 10.1016/j.micres.2006.06.007
Uzelac, G., Patel, H. K., Devescovi, G., Licastro, D., and Venturi, V. (2017). Quorum sensing and RsaM regulons of the rice pathogen Pseudomonas fuscovaginae. Microbiology 163, 765–777. doi: 10.1099/mic.0.000454
Van Der Voort, M., Meijer, H. J. G., Schmidt, Y., Watrous, J., Dekkers, E., Mendes, R., et al. (2015). Genome mining and metabolic profiling of the rhizosphere bacterium Pseudomonas sp. SH-C52 for antimicrobial compounds. Front. Microbiol. 6:693. doi: 10.3389/fmicb.2015.00693
Vaughn, V. L., and Gross, D. C. (2016). Characterization of salA, syrF, and syrG genes and attendant regulatory networks involved in plant pathogenesis by Pseudomonas syringae pv. syringae B728a. PLoS One 11:e0150234. doi: 10.1371/journal.pone.0150234
Venturi, V. (2006). Regulation of quorum sensing in Pseudomonas. FEMS Microbiol. Rev. 30, 274–291. doi: 10.1111/j.1574-6976.2005.00012.x
von Bodman, S. B., Bauer, W. D., and Coplin, D. L. (2003). Quorum sensing in plant-pathogenic bacteria. Annu. Rev. Phytopathol. 41, 455–482. doi: 10.1146/annurev.phyto.41.052002.095652
Wang, N., Lu, S. E., Records, A. R., and Gross, D. C. (2006a). Characterization of the transcriptional activators SalA and SyrF, which are required for syringomycin and syringopeptin production by Pseudomonas syringae pv. syringae. J. Bacteriol. 188, 3290–3298.
Keywords: cyclic lipopeptides, RNA-seq, non-ribosomal peptides, transcriptional analysis, exopolysaccarides
Citation: Licciardello G, Caruso A, Bella P, Gheleri R, Strano CP, Anzalone A, Trantas EA, Sarris PF, Almeida NF and Catara V (2018) The LuxR Regulators PcoR and RfiA Co-regulate Antimicrobial Peptide and Alginate Production in Pseudomonas corrugata. Front. Microbiol. 9:521. doi: 10.3389/fmicb.2018.00521
Received: 17 October 2017; Accepted: 08 March 2018;
Published: 23 March 2018.
Edited by:
Jesús Mercado-Blanco, Instituto Agricultura Sostenible (CSIC), SpainReviewed by:
María A. Llamas, Experimental Station of Zaidín (CSIC), SpainCarmen R. Beuzón, Universidad de Málaga, Spain
Brian H. Kvitko, University of Georgia, United States
Copyright © 2018 Licciardello, Caruso, Bella, Gheleri, Strano, Anzalone, Trantas, Sarris, Almeida and Catara. This is an open-access article distributed under the terms of the Creative Commons Attribution License (CC BY). The use, distribution or reproduction in other forums is permitted, provided the original author(s) and the copyright owner are credited and that the original publication in this journal is cited, in accordance with accepted academic practice. No use, distribution or reproduction is permitted which does not comply with these terms.
*Correspondence: Vittoria Catara, dmNhdGFyYUB1bmljdC5pdA==
†These authors have contributed equally to this work.