- 1Department of Infectious Diseases, Sir Run Run Shaw Hospital, School of Medicine, Zhejiang University, Hangzhou, China
- 2Department of Clinical Laboratory, Zhejiang Provincial People’s Hospital, People’s Hospital of Hangzhou Medical College, Hangzhou, China
- 3Department of Clinical Laboratory, Sir Run Run Shaw Hospital, School of Medicine, Zhejiang University, Hangzhou, China
- 4Institute of Hygiene, Zhejiang Academy of Medical Sciences, Hangzhou, China
Tigecycline is one of the last resort treatments for carbapenem-resistant Klebsiella pneumoniae (CRKP) infections. Tigecycline resistance often occurs during the clinical treatment of CRKP, yet its mechanism has still not been clearly elucidated. This study presents an analysis of a tigecycline resistance mechanism that developed in clinical isolates from a 56-year-old female patient infected with CRKP during tigecycline treatment. Consecutive clonal consistent K. pneumoniae isolates were obtained during tigecycline treatment. Whole genome sequencing of the isolates was performed, and putative single nucleotide polymorphisms and insertion and deletion mutations were analyzed in susceptible and resistant isolates. The identified gene of interest was examined through experiments involving transformations and conjugations. Four isolates, two of which were susceptible and two resistant, were collected from the patient. All of the isolates belonged to Sequence Type 11 (ST11) and were classified as extensively drug resistant (XDR). One amino acid substitution S251A in TetA was identified in the tigecycline-resistant isolates. Subsequent transformation experiments confirmed the contribution of the TetA variant (S251A) to tigecycline resistance. The transfer capacity of tigecycline resistance via this mutation was confirmed by conjugation experiments. Using southern blot hybridization and PCR assays, we further proved that the tetA gene was located on a transferable plasmid of ca. 65 kb in an Escherichia coli EC600 transconjugant. Our results provide direct in vivo evidence that evolution in the tetA gene can lead to tigecycline treatment failure in CRKP clinical strains that carry tetA. Moreover, the transfer capacity of tigecycline resistance mediated by mutated tetA is a threat.
Introduction
Carbapenem-resistant Klebsiella pneumoniae (CRKP) infections have emerged worldwide as a serious challenge to public health (Pitout et al., 2015). K. pneumoniae resistance to carbapenem is mainly due to the acquisition of carbapenemase, and the carbapenem-hydrolyzing K. pneumoniae carbapenemase (KPC) has been the enzyme most commonly identified in K. pneumoniae in China (Zhang et al., 2017). K. pneumoniae can cause various types of infections, including abdominal and urinary tract infections, liver abscesses, bacteremia, and pneumonia (He et al., 2015). The management of infections caused by CRKP is complicated (Pitout et al., 2015). In fact, carbapenemase-producing K. pneumoniae often carry multiple drug resistance genes that enable the strains to exhibit resistance to almost all antibiotics except colistin and tigecycline (da Silva et al., 2012; Pitout et al., 2015).
Tigecycline is one of the last treatment options remaining for carbapenem-resistant Enterobacteriaceae (Alhashem et al., 2017; Iovleva and Doi, 2017). A semisynthetic derivative of minocycline, tigecycline is a recent antibiotic that belongs to the glycylcycline class and can overcome the mechanisms of tetracycline resistance (Pankey, 2005). Tigecycline is effective against most carbapenemase-producing bacteria, including K. pneumoniae, and has been approved in recent years for clinical use in China (He et al., 2015). However, resistance to tigecycline has emerged since its approval (Hoban et al., 2005). The mechanism for tigecycline resistance has not yet been clearly elucidated. In Gram-negative bacteria, the enhanced expression of resistance–nodulation–cell division (RND)-type efflux pumps was associated with decreased tigecycline susceptibility (Osei Sekyere et al., 2016). Over-expression of the RND efflux pumps, AcrAB and OqxAB, is one of the mechanisms reported most frequently in the Enterobacteriaceae species, and several global transcriptional regulators of the AraC family, namely, RamA, MarA, SoxS, and RarA, participate in tigecycline resistance via efflux pump activation (Ruzin et al., 2005; Bratu et al., 2009; Veleba and Schneiders, 2012; Veleba et al., 2012). Nielsen et al. (2014) also reported a new efflux pump operon, kpgABC, and identified an insertion element whose presence correlated with the in vivo development of tigecycline non-susceptibility in K. pneumoniae. The ribosomal S10 protein is also a general target (via the mutation of rpsJ) that has been described for its ability to cause decreased tigecycline susceptibility in both Gram-negative and Gram-positive bacteria (Cannatelli et al., 2014; Beabout et al., 2015).
Previous studies have shown that tigecycline can overcome the mechanisms of tetracycline resistance (Pankey, 2005; Jenner et al., 2013). However, Akiyama et al. (2013) found that the tetA gene carried by Salmonella species, which imparts their tetracycline resistance and also causes reduced susceptibility to tigecycline. Linkevicius et al. (2016) discovered that evolutionary changes in TetA, TetM, and TetX can cause tigecycline resistance in Escherichia coli in vitro, and they predicted that TetX might become the most problematic future Tet determinant because it did not have adverse effects on resistance toward other tetracyclines. Most recently, Chiu et al. (2017) reported that widespread mutated tetA genes are concerning due to the possible dissemination of increased tigecycline resistance in K. pneumoniae.
In this study, consecutive clonal consistent K. pneumoniae isolates were collected from a 56-year-old female patient with duodenal papillary carcinoma who was undergoing tigecycline treatment. In the early stages of her tigecycline treatment, the isolates were susceptible to tigecycline (MIC = 1 mg/L); 13 days later, we isolated the first resistant strain with a tigecycline MIC of 32 mg/L. To the best of our knowledge, this study is the first report to present consecutive clonal consistent K. pneumoniae isolates while they evolved high-level resistance to tigecycline during in vivo treatment. Whole genome sequencing (WGS) of these isolates was completed using the Illumina HiSeq X Ten platform, and subsequent bioinformatics analyses were performed to explore the tigecycline resistance mechanism of these isolates.
Materials and Methods
The Patient and Isolates
A 56-year-old female patient was diagnosed with duodenal papillary carcinoma and admitted to the hospital for a Whipple operation. Following surgery, the patient contracted an abdominal infection. Initially, the patient received multiple antimicrobial treatments, including cefotaxime, levofloxacin, and imipenem. Isolates were cultured from the patient during her hospitalization. KPC-2 producing extensively drug resistant (XDR) K. pneumoniae susceptible only to colistin and tigecycline were isolated from both blood and ascites samples 23 days subsequent to the Whipple surgery. The antibiotic therapeutic strategy was then adjusted to that of intravenous tigecycline (a 100 mg loading dose followed by 50 mg every 12 h). Thirteen days later, the first tigecycline-resistant isolate was detected from an ascites sample (QJJ49) with a tigecycline MIC of 32 mg/L; 19 days later, another tigecycline resistant isolate was detected from a blood sample (QJJ51) with a tigecycline MIC of 32 mg/L. Tigecycline therapy lasted for 19 days, until the resistant phenotype appeared. At the end of the treatment period, the patient expired from multiple organ dysfunction syndrome (MODS).
Bacterial Isolates
All of the isolates were preliminarily identified using the VITEK 2 system (bioMérieux, France) and were further confirmed by 16S rRNA gene sequencing. Multilocus sequence typing (MLST) of the isolate was analyzed using the BacWGSTdb server with the entire genome sequence (Ruan and Feng, 2016).
Antimicrobial Susceptibility Test
The MICs of tigecycline and colistin were determined using standard broth microdilution tests with fresh (<12 h) Mueller–Hinton broth (Cation-adjusted, Oxoid LTD, Basingstoke, Hampshire, England). E. coli ATCC 25922 was used for quality control (He et al., 2015). The MICs of other antimicrobial agents were determined using the Etest method. All tests were performed according to the guidelines of the Clinical and Laboratory Standards Institute (CLSI). Antimicrobial susceptibility was determined using the breakpoints approved by the CLSI, with the U.S. Food and Drug Administration (FDA) breakpoints used for tigecycline (≤2.0 mg/L, susceptible; 4.0 mg/L, intermediate; and ≥8.0 mg/L, resistant).
Whole Genome Sequencing and Analysis
Genomic DNA was extracted using a QIAamp DNA MiniKit (Qiagen, Valencia, CA, United States) following the manufacturer’s instructions. The genomes were sequenced using the Illumina HiSeqTM X Ten platform (Illumina, Inc., San Diego, CA, United States) following the paired-end 2 bp × 150 bp protocol. The whole genome sequences were assembled using CLC Genomics Workbench 9.0 software (Qiagen, Valencia, CA, United States). Resistance-related genes were analyzed using ResFinder 3.0 with a 90% threshold for gene identification and a 60% minimum length (Zankari et al., 2012).
The genomic sequence of the initial isolate QJJ29 was used as the reference genome. The reads from the other isolates were mapped against this reference genome using CLC Genomics Workbench 9 software. Basic variants such as single nucleotide polymorphisms (SNPs) and insertion and deletion mutations were predicted by CLC software and the potential mutations were confirmed by PCR and Sanger sequencing with the primers listed in Supplementary Table S1. Potential large fragment insertions were also searched by CLC software, but not verified by PCR and sequencing.
Transformation and Conjugation Experiment
DNA fragments carrying the wild-type tetA and mutated tetA genes were amplified from the tigecycline-susceptible isolate QJJ29 and the tigecycline-resistant isolate QJJ51, respectively, with the primers listed in Supplementary Table S1. Following amplification, the amplimer was cloned into plasmid pCR2.1 (Invitrogen, United States) behind the T7 promoter. E. coli ATCC 25922 was used for transformation. The effect of the tetA mutation on the tigecycline MIC was examined using standard broth microdilution tests.
The transfer capacity of tigecycline resistance was investigated by conjugation experiments (Fu et al., 2012). We used the rifampicin-resistant E. coli EC600 as a recipient and the rifampicin-susceptible isolates QJJ29 and QJJ51 as donors. Transconjugants were selected on MH agar plates supplemented with tetracycline (32 mg/L) and rifampicin (800 mg/L). The tigecycline MIC of the transconjugants was examined using standard broth microdilution tests. The tetA gene was analyzed in the EC600 transconjugants by PCR and Sanger sequencing with the primers tetA-Com-F and tetA-Com-R (Supplementary Table S1).
S1-PFGE and Southern Blot Hybridization
Genomic DNA was digested with the S1 enzyme following the protocol of Barton et al. (1995).
The DNA fragments were transferred to a positively charged nylon membrane (Millipore, United States), hybridized with a DIG-labeled specific probe and detected with an NBT/BCIP color detection kit (Roche, Germany). The hybridization probe was designed to bind within the middle portion of the tetA gene and was synthesized using the primers listed in Supplementary Table S1.
Nucleotide Sequence Accession Numbers
The Whole Genome Shotgun projects for isolates QJJ29 (Accession No. POUY00000000), QJJ36 (Accession No. POWK00000000), QJJ49 (Accession No. POUX00000000), and QJJ51 (Accession No. POWJ00000000) have been deposited at DDBJ/EMBL/GenBank.
Results
Isolate Characterizations
Four K. pneumoniae isolates were isolated from a patient during tigecycline treatment at Sir Run Run Shaw hospital in Zhejiang, China, in 2016. All of the isolates belong to Sequence Type 11 (ST11) and were classified as XDR. According to Illumina sequencing and subsequent mapping, the mapping percentages among the isolates all exceeded 99.6% (i.e., they are clonally consistent). The MICs of the isolates to different antibiotics are presented in Table 1. The initial isolate, QJJ29, was collected from a blood sample on April 23, 2016, the same day tigecycline treatment was initiated. QJJ29 was resistant to carbapenem but susceptible to tigecycline. Six days later, on April 28, 2016, the second isolate, QJJ36, was collected. QJJ36 was also susceptible to tigecycline. Thirteen days after the commencement of tigecycline treatment, on May 5, 2016, the first tigecycline-resistant isolate, QJJ49 with a tigecycline MIC of 32 mg/L, was collected from an ascites sample. On May 11, 2016, the second tigecycline-resistant isolate, QJJ51, was collected from a blood sample. The clinical data of the isolates are listed in Table 2.
Distribution of Resistance Genes
The resistance genes present in the genomes of the isolates are presented in Table 3. All isolates harbored multiple resistance genes. We identified the aminoglycoside resistance gene rmtB; the beta-lactam resistance genes blaTEM-1B, blaCTX-M-65, blaSHV -2, and blaKPC-2; the fluoroquinolone resistance gene qnrS1; the fosfomycin resistance genes fosA and fosA3; the lincosamide resistance gene lnu(A); the phenicol resistance gene catA2; the sulphonamide resistance gene sul2; the trimethoprim resistance gene dfrA14; and the tetracycline resistance gene tetA. Isolates QJJ51 and QJJ49 had an additional lnu(A) gene that was not present in QJJ29 and QJJ36, and isolate QJJ49 lacked the blaKPC-2 gene and was susceptible to imipenem and meropenem.
Genomic Analysis
According to the mapping analysis, the QJJ36, QJJ49, and QJJ51 isolates all exhibited greater than 99.6% similarity to the reference genome (QJJ29). Three, five, and six mutation sites were confirmed in QJJ36, QJJ49, and QJJ51, respectively (Table 2). The three mutations in QJJ36 (QJJ29 contig 1: 189918; QJJ29 contig 19: 47896; and QJJ29 contig 22: 133) were all present in QJJ49 and QJJ51. The tigecycline MIC of QJJ36 was the same as that of QJJ29. One point mutation in tetA (QJJ29 contig 79: 1641) was found in both QJJ49 and QJJ51. This mutation (T751G) results in an amino acid substitution (S251A). One, six, and four potential large fragment insertions were found in QJJ36, QJJ49, and QJJ51, respectively. Most of them were located in intergenic region. Only one gene rmpA (in isolate QJJ49) was truncated by the insertion (Supplementary Table S2).
Effects of the tetA Mutation on Tigecycline Resistance
The results of the complementation experiment are presented in Table 4. When E. coli ATCC 25922 was transformed with the mutated tetA gene (25922/pCR2.1-tetA+), its tigecycline MIC increased from 0.125 to 8 mg/L, its tetracycline MIC increased from 1 to 128 mg/L, and its minocycline MIC increased from 1 to 16 mg/L. When 25922 was transformed with the wild-type tetA gene (25922/pCR2.1-tetA), its tigecycline MIC increased only from 0.125 to 0.5 mg/L, while its tetracycline MIC increased from 1 to 64 mg/L and its minocycline MIC increased from 1 to 4 mg/L. No MIC change was found in 25922 when it was transformed with the empty pCR2.1 vector (25922/pCR2.1).
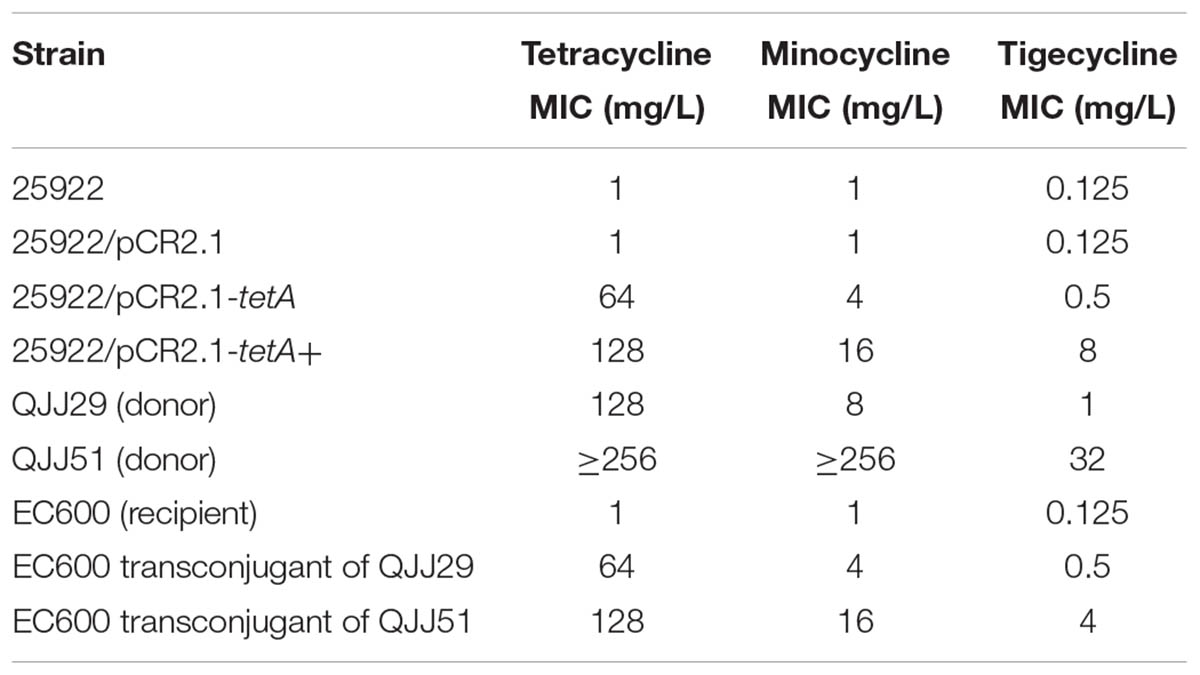
TABLE 4. Changes in the tetracycline, minocycline, and tigecycline MICs during the transformation and conjugation experiments.
Conjugation of the Tigecycline Resistance Factor
The transfer capacity of tigecycline resistance was confirmed by a conjugation experiment. The tigecycline MIC increased 32-fold in the EC600 transconjugant of QJJ51 compared with that of the parental strain EC600 (from 0.125 to 4 mg/L); furthermore, the tetracycline MIC increased from 1 to 128 mg/L and the minocycline MIC increased from 1 to 16 mg/L (Table 4). Conversely, in the EC600 transconjugant of QJJ29, the tigecycline MIC increased only from 0.125 to 0.5 mg/L while the tetracycline MIC increased from 1 to 64 mg/L and the minocycline MIC increased from 1 to 4 mg/L compared with the parental strain EC600.
Southern Blot Hybridization of the tetA Gene
The tetA gene was determined to be transferred by conjugation by southern blot hybridization and PCR assays. The tetA gene was located on a transferable plasmid with a size of ca. 65 kb in all the isolates detected (Figure 1). From the PCR and Sanger sequencing results, the EC600 transconjugant of QJJ51 harbored the same mutant-type tetA gene as isolate QJJ51.
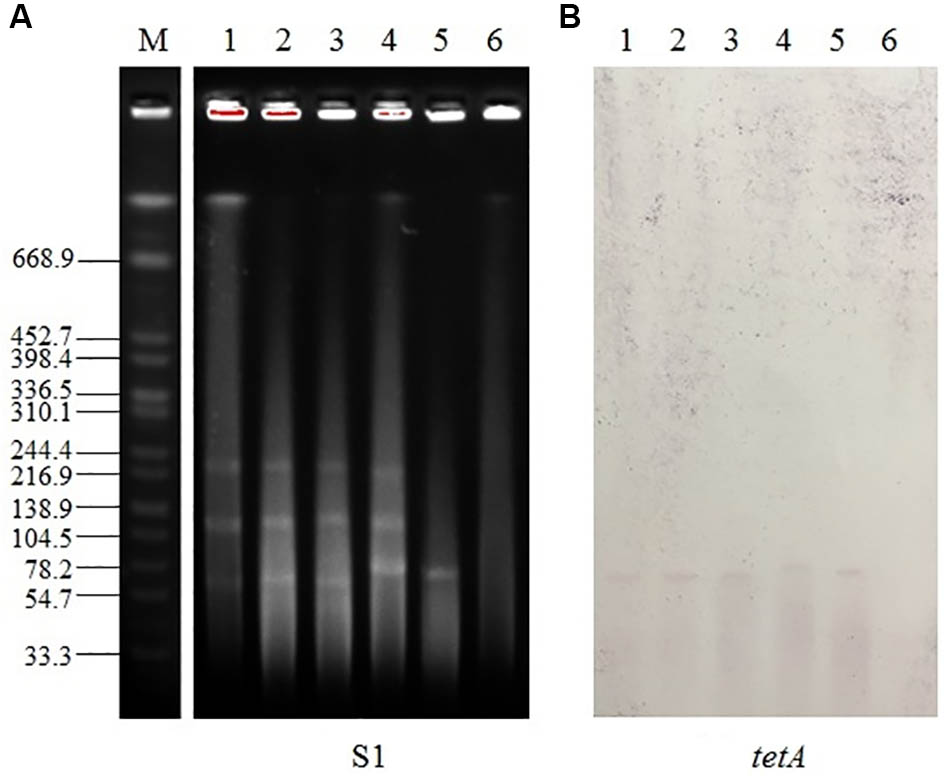
FIGURE 1. Analysis of tetA localization using S1-PFGE and southern blot hybridization. (A) S1-PFGE profiles and (B) southern blot hybridization with a tetA probe. M, Salmonella enterica H9812 DNA digested with XbaI, used as a molecular marker (kb); lanes 1–6, QJJ29, QJJ36, QJJ49, QJJ51, EC600 transconjugant of QJJ51, and EC600, respectively.
Discussion
In this study, tigecycline resistance was monitored in a 56-year-old female patient infected with CRKP during tigecycline treatment. At the outset of her treatment, the strain was found to be susceptible to tigecycline; 13 days later, the first tigecycline resistant isolate was detected, and it possessed a high tigecycline MIC (32 mg/L).
Over-expression of RND efflux pumps and mutations in the ribosomal S10 protein (rpsJ) have been the widely accepted mechanisms that result in decreased tigecycline susceptibility in K. pneumoniae (Ruzin et al., 2005, 2008; Bratu et al., 2009; Roy et al., 2013; Villa et al., 2014; Beabout et al., 2015; He et al., 2015). However, the tigecycline resistant isolates (QJJ49 and QJJ51) collected in this study had neither over-expressed RND efflux pumps AcrAB and OqxAB (the expression levels of the efflux pump genes were detected by qRT-PCR, Supplementary Table S3) nor rpsJ gene mutations. One mutation in the tetA gene (S251A) was uncovered through WGS and analysis. Subsequent transformation and conjugation experiments revealed that the tetA mutation was responsible for the development of tigecycline resistance in these isolates.
The wild-type tetA gene was observed to slightly decrease the sensitivity to tigecycline in Salmonella enterica clinical isolates by Akiyama et al. (2013). In our study, transformation of the wild-type tetA gene into E. coli ATCC 25922 increased the tigecycline MIC from 0.125 to 0.5 mg/L (Table 4). This phenomenon coincides with that of Akiyama’s.
Linkevicius et al. (2016) reported that tetA evolution causes decreased sensitivity to tigecycline in E. coli in vitro. They created libraries of mutagenized tetA sequences through separate, error-prone PCR assays, which were then cloned into the pBAD30 vector and electroporated into E. coli NEB5α. Subsequently, the researchers selected 53 independent mutants with elevated tigecycline MICs. The most common amino acid substitution identified was G300E. Other frequent changes were identified more than once in the independent mutants including S251A. In another report, Chiu et al. (2017) also frequently isolated mutated tetA genes in tigecycline-resistant K. pneumoniae.
The current study is the first to provide direct in vivo evidence that changes in the tetA gene can lead to tigecycline treatment failure in patients infected with CRKP clinical strains. Distinct from Linkevicius’s study, we discovered that the S251A mutation resulted in high-level tigecycline resistance in CRKP strains and E. coli ATCC 25922, and their tetracycline and minocycline MICs also increased (Tables 1, 4). Furthermore, our conjugation experiment confirmed the transfer capacity of tigecycline resistance, which was found to be mediated by the mutated tetA through a transferable plasmid of ca. 65 kb. Tigecycline is regarded as a last resort treatment for CRKP infections. This finding serves as a therapeutic warning as the tetA gene is frequently carried by CRKP strains. Under selective pressure from tigecycline, the tetA mutation may occur and lead to treatment failure. This transfer capacity is also a threat to the service life of tigecycline; the more the tetA mutates and evolves toward tigecycline resistance, the more likely it will become a horizontally transferrable resistance gene for tigecycline resistance.
Author Contributions
XD and FH analyzed the genomic data and drafted the manuscript. FH, QS, FZ, JX, and YF performed the experiments. XD and YY designed the study and drafted the manuscript. All authors read and approved the final manuscript.
Funding
This study was supported by grants from the National Natural Science Foundation of China (81702042 and 81471986), the Zhejiang Provincial Key Research and Development Plan (2015C03046), and the Zhejiang Provincial Medical and Health Science and Technology Plan (2018KY344).
Conflict of Interest Statement
The authors declare that the research was conducted in the absence of any commercial or financial relationships that could be construed as a potential conflict of interest.
Supplementary material
The Supplementary Material for this article can be found online at: https://www.frontiersin.org/articles/10.3389/fmicb.2018.00648/full#supplementary-material
References
Akiyama, T., Presedo, J., and Khan, A. A. (2013). The tetA gene decreases tigecycline sensitivity of Salmonella enterica isolates. Int. J. Antimicrob. Agents 42, 133–140. doi: 10.1016/j.ijantimicag.2013.04.017
Alhashem, F., Tiren-Verbeet, N. L., Alp, E., and Doganay, M. (2017). Treatment of sepsis: What is the antibiotic choice in bacteremia due to carbapenem resistant Enterobacteriaceae? World J. Clin. Cases 5, 324–332. doi: 10.12998/wjcc.v5.i8.324
Barton, B. M., Harding, G. P., and Zuccarelli, A. J. (1995). A general method for detecting and sizing large plasmids. Anal. Biochem. 226, 235–240. doi: 10.1006/abio.1995.1220
Beabout, K., Hammerstrom, T. G., Perez, A. M., Magalhaes, B. F., Prater, A. G., Clements, T. P., et al. (2015). The ribosomal S10 protein is a general target for decreased tigecycline susceptibility. Antimicrob. Agents Chemother. 59, 5561–5566. doi: 10.1128/AAC.00547-15
Bratu, S., Landman, D., George, A., Salvani, J., and Quale, J. (2009). Correlation of the expression of acrB and the regulatory genes marA, soxS and ramA with antimicrobial resistance in clinical isolates of Klebsiella pneumoniae endemic to New York City. J. Antimicrob. Chemother. 64, 278–283. doi: 10.1093/jac/dkp186
Cannatelli, A., Giani, T., D’andrea, M. M., Di Pilato, V., Arena, F., Conte, V., et al. (2014). MgrB inactivation is a common mechanism of colistin resistance in KPC-producing Klebsiella pneumoniae of clinical origin. Antimicrob. Agents Chemother. 58, 5696–5703. doi: 10.1128/AAC.03110-14
Chiu, S. K., Huang, L. Y., Chen, H., Tsai, Y. K., Liou, C. H., Lin, J. C., et al. (2017). Roles of ramR and tet(A) mutations in conferring tigecycline resistance in carbapenem-resistant Klebsiella pneumoniae clinical isolates. Antimicrob. Agents Chemother. 61:e00391-17. doi: 10.1128/AAC.00391-17
da Silva, R. M., Traebert, J., and Galato, D. (2012). Klebsiella pneumoniae carbapenemase (KPC)-producing Klebsiella pneumoniae: a review of epidemiological and clinical aspects. Expert Opin. Biol. Ther. 12, 663–671. doi: 10.1517/14712598.2012.681369
Fu, Y., Du, X., Ji, J., Chen, Y., Jiang, Y., and Yu, Y. (2012). Epidemiological characteristics and genetic structure of blaNDM-1 in non-baumannii Acinetobacter spp. in China. J. Antimicrob. Chemother. 67, 2114–2122. doi: 10.1093/jac/dks192
He, F., Fu, Y., Chen, Q., Ruan, Z., Hua, X., Zhou, H., et al. (2015). Tigecycline susceptibility and the role of efflux pumps in tigecycline resistance in KPC-producing Klebsiella pneumoniae. PLoS One 10:e0119064. doi: 10.1371/journal.pone.0119064
Hoban, D. J., Bouchillon, S. K., Johnson, B. M., Johnson, J. L., and Dowzicky, M. J. (2005). In vitro activity of tigecycline against 6792 Gram-negative and Gram-positive clinical isolates from the global Tigecycline Evaluation and Surveillance Trial (TEST Program, 2004). Diagn. Microbiol. Infect. Dis. 52, 215–227. doi: 10.1016/j.diagmicrobio.2005.06.001
Iovleva, A., and Doi, Y. (2017). Carbapenem-resistant Enterobacteriaceae. Clin. Lab. Med. 37, 303–315. doi: 10.1016/j.cll.2017.01.005
Jenner, L., Starosta, A. L., Terry, D. S., Mikolajka, A., Filonava, L., Yusupov, M., et al. (2013). Structural basis for potent inhibitory activity of the antibiotic tigecycline during protein synthesis. Proc. Natl. Acad. Sci. U.S.A. 110, 3812–3816. doi: 10.1073/pnas.1216691110
Linkevicius, M., Sandegren, L., and Andersson, D. I. (2016). Potential of tetracycline resistance proteins to evolve tigecycline resistance. Antimicrob. Agents Chemother. 60, 789–796. doi: 10.1128/AAC.02465-15
Nielsen, L. E., Snesrud, E. C., Onmus-Leone, F., Kwak, Y. I., Aviles, R., Steele, E. D., et al. (2014). IS5 element integration, a novel mechanism for rapid in vivo emergence of tigecycline nonsusceptibility in Klebsiella pneumoniae. Antimicrob. Agents Chemother. 58, 6151–6156. doi: 10.1128/AAC.03053-14
Osei Sekyere, J., Govinden, U., Bester, L. A., and Essack, S. Y. (2016). Colistin and tigecycline resistance in carbapenemase-producing Gram-negative bacteria: emerging resistance mechanisms and detection methods. J. Appl. Microbiol. 121, 601–617. doi: 10.1111/jam.13169
Pitout, J. D., Nordmann, P., and Poirel, L. (2015). Carbapenemase-producing Klebsiella pneumoniae, a key pathogen set for global nosocomial dominance. Antimicrob. Agents Chemother. 59, 5873–5884. doi: 10.1128/AAC.01019-15
Roy, S., Datta, S., Viswanathan, R., Singh, A. K., and Basu, S. (2013). Tigecycline susceptibility in Klebsiella pneumoniae and Escherichia coli causing neonatal septicaemia (2007-10) and role of an efflux pump in tigecycline non-susceptibility. J. Antimicrob. Chemother. 68, 1036–1042. doi: 10.1093/jac/dks535
Ruan, Z., and Feng, Y. (2016). BacWGSTdb, a database for genotyping and source tracking bacterial pathogens. Nucleic Acids Res. 44, D682–D687. doi: 10.1093/nar/gkv1004
Ruzin, A., Immermann, F. W., and Bradford, P. A. (2008). Real-time PCR and statistical analyses of acrAB and ramA expression in clinical isolates of Klebsiella pneumoniae. Antimicrob. Agents Chemother. 52, 3430–3432. doi: 10.1128/AAC.00591-08
Ruzin, A., Visalli, M. A., Keeney, D., and Bradford, P. A. (2005). Influence of transcriptional activator RamA on expression of multidrug efflux pump AcrAB and tigecycline susceptibility in Klebsiella pneumoniae. Antimicrob. Agents Chemother. 49, 1017–1022. doi: 10.1128/AAC.49.3.1017-1022.2005
Veleba, M., Higgins, P. G., Gonzalez, G., Seifert, H., and Schneiders, T. (2012). Characterization of RarA, a novel AraC family multidrug resistance regulator in Klebsiella pneumoniae. Antimicrob. Agents Chemother. 56, 4450–4458. doi: 10.1128/AAC.00456-12
Veleba, M., and Schneiders, T. (2012). Tigecycline resistance can occur independently of the ramA gene in Klebsiella pneumoniae. Antimicrob. Agents Chemother. 56, 4466–4467. doi: 10.1128/AAC.06224-11
Villa, L., Feudi, C., Fortini, D., Garcia-Fernandez, A., and Carattoli, A. (2014). Genomics of KPC-producing Klebsiella pneumoniae sequence type 512 clone highlights the role of RamR and ribosomal S10 protein mutations in conferring tigecycline resistance. Antimicrob. Agents Chemother. 58, 1707–1712. doi: 10.1128/AAC.01803-13
Zankari, E., Hasman, H., Cosentino, S., Vestergaard, M., Rasmussen, S., Lund, O., et al. (2012). Identification of acquired antimicrobial resistance genes. J. Antimicrob. Chemother. 67, 2640–2644. doi: 10.1093/jac/dks261
Keywords: tigecycline resistance, carbapenem-resistant Klebsiella pneumoniae, efflux pump, tetA, KPC-2
Citation: Du X, He F, Shi Q, Zhao F, Xu J, Fu Y and Yu Y (2018) The Rapid Emergence of Tigecycline Resistance in blaKPC–2 Harboring Klebsiella pneumoniae, as Mediated in Vivo by Mutation in tetA During Tigecycline Treatment. Front. Microbiol. 9:648. doi: 10.3389/fmicb.2018.00648
Received: 14 November 2017; Accepted: 20 March 2018;
Published: 05 April 2018.
Edited by:
Yuji Morita, Aichi Gakuin University, JapanCopyright © 2018 Du, He, Shi, Zhao, Xu, Fu and Yu. This is an open-access article distributed under the terms of the Creative Commons Attribution License (CC BY). The use, distribution or reproduction in other forums is permitted, provided the original author(s) and the copyright owner are credited and that the original publication in this journal is cited, in accordance with accepted academic practice. No use, distribution or reproduction is permitted which does not comply with these terms.
*Correspondence: Yunsong Yu, eXZ5czExOUB6anUuZWR1LmNu; eXZ5czExOUAxNjMuY29t
†These authors have contributed equally to this work.