- 1Key Laboratory of Pollution Ecology and Environmental Engineering, Institute of Applied Ecology, Chinese Academy of Sciences, Shenyang, China
- 2University of Chinese Academy of Sciences, Beijing, China
- 3School of Biological and Chemical Engineering, Liaoning Institute of Science and Technology, Benxi, China
Elevated carbon dioxide (eCO2), a much-discussed topic in global warming, influences development and functions of mycorrhizal fungi and plants. However, due to the inconsistent results reported in various publications, the response patterns of symbionts associated with the arbuscular mycorrhizal (AM) or with ectomycorrhizal (ECM) fungi to eCO2 remains still unclear. Therefore, we performed a meta-analysis to identify how eCO2 affected mycorrhizal fungi and if there is a significant different response between AM and ECM symbionts. Our results demonstrated that eCO2 increased mycorrhizal plants biomass (+26.20%), nutrient contents [+2.45% in nitrogen (N), and +10.66% in phosphorus (P)] and mycorrhizal fungal growth (+22.87% in extraradical hyphal length and +21.77% in mycorrhizal fungal biomass), whereas plant nutrient concentrations decreased (−11.86% in N and −12.01% in P) because the increase in plant biomass was greater than that in nutrient content. The AM plants exhibited larger increases in their biomass (+33.90%) and in their N (+21.99%) and P contents (+19.48%) than did the ECM plants (+20.57% in biomass, −4.28% in N content and −13.35% in P content). However, ECM fungi demonstrated increased responses of mycorrhizal fungal biomass (+29.98%) under eCO2 compared with AM fungi (+6.61%). These data indicate different patterns in the growth of AM and ECM symbionts under eCO2: AM symbionts contributed more to plant growth, while ECM symbionts were more favorable to mycorrhizal fungal growth. In addition, the responses of plant biomass to eCO2 showed no significant difference between short-term and long-term groups, whereas a significant difference in the responses of mycorrhizal fungal growth was found between the two groups. The addition of N increased plant growth but decreased mycorrhizal fungal abundance, and P addition increased total plant biomass and extraradical hyphal length, but shoot biomass largely increased in low P conditions. Mixtures of mycorrhizal fungi affected the total plant and root biomasses more than a single mycorrhizal fungus. Clarifying the different patterns in AM and ECM symbionts under eCO2 would contribute to a better understanding of the interactions between mycorrhizal fungi and plant symbionts under the conditions of global climate change as well as of the coevolution of flora with Earth's environment.
Introduction
The rising level of carbon dioxide (CO2) in the atmosphere is a major concern worldwide and could cause many changes in plant physiology and metabolism (Leakey et al., 2009; McGrath and Lobell, 2013). Individual studies have been conducted to assess the effects of elevated CO2 (eCO2) levels on plant growth, including nutrient absorption (Feng et al., 2015), the net assimilation rate, stomatal conductance (Augé et al., 2015), transpiration, water-use efficiency and sugar accumulation in leaves (Drake et al., 1997). It has been reported that eCO2 has changed the ecosystem element cycles, and many earth system models (ESMs) have been established to predict the future carbon (C), nitrogen (N), and phosphorus (P) cycles, as well as their interactions (Zaehle et al., 2014; Reed et al., 2015). In addition, excessive fertilizer input would further complicate the C-N-P interactions under eCO2 conditions. Moreover, mycorrhizal fungi, probably the most widespread symbionts in nature, exist in approximately 80% of terrestrial plant species (Baum et al., 2015). Extensive interactions exist between mycorrhizal fungi and plant symbionts: mycorrhizal fungi transfer N and P to plants, while plants supply organic carbon (C) to mycorrhizal fungi (Smith and Smith, 2012). This phenomenon improves plant growth, nutrient absorption, and water-use efficiency (Smith and Smith, 2012). By altering stomatal conductance, making osmotic adjustments (Augé et al., 2014) and regulating related gene expression (Porcel et al., 2016), among other mechanisms, the symbionts alleviate the harmful effects of drought and salinity stress on their plant hosts (Augé et al., 2015). Furthermore, mycorrhizal fungi can contribute to the alleviation of heavy metal pollution (Curaqueo et al., 2014; Yang et al., 2015), heat stress (Prasad et al., 2008; Cabral et al., 2016), ozone stress (Cui et al., 2013), and soil aggregation (Leifheit et al., 2014; Rillig et al., 2015). Therefore, discussing the responses of plants to eCO2, their interactions with mycorrhizal fungi must be considered (Grover et al., 2015; Simonin et al., 2017).
Arbuscular mycorrhizal (AM) and ectomycorrhizal (ECM) fungi employ two different nutrient acquisition strategies: AM fungi scavenge for nutrients released by saprotrophic microbes, whereas ECM fungi mineralize nutrients from organic matter and can thus access some forms of organic N directly (Phillips et al., 2013). It is therefore expected that AM and ECM might respond differently to eCO2 levels (Treseder and Allen, 2000; Alberton et al., 2005). However, inconsistent results of AM colonization were reported, including positive effects (Becklin et al., 2016; Jakobsen et al., 2016), negative effects (Goicoechea et al., 2014) and no effect (Tang et al., 2006), and similar inconsistent results were found for ECM (Walker et al., 1998; Garcia et al., 2008; Wang et al., 2015). Mycorrhizal fungal biomass has been reported to show positive or negative effects in different AM species (Langley et al., 2003), and this is also the case in ECM (Gutknecht et al., 2012). AM and ECM trees are also expected to respond differently to global change factors due to their different adaptations and distribution patterns (Phillips et al., 2013). In previous studies, it was reported that AM trees exhibited positive responses and ECM more often demonstrated negative responses (Boggs et al., 2005; Quinn Thomas et al., 2009). Additionally, the fungal community is tightly linked to fine root production in plants under eCO2 (Lipson et al., 2014), and the characteristics of the plant type and relevant physicochemical factors induced by eCO2 may be important key factors in structuring the response of the microbial community to environmental change (Lee et al., 2015). N and P additions reportedly affect the growth of both mycorrhizal fungi and plants under eCO2 (Staddon et al., 2004; Lee et al., 2015; Ekblad et al., 2016; Jakobsen et al., 2016). Overall, it is difficult to draw a consistent conclusion and determine the magnitude of the effect without a statistical analysis due to the above mentioned inconsistent results in individual studies. Meta-analysis is a quantitative statistical method that integrates the results of numerous individual studies and can be used to extract a general trend from numerous individual results in a precise statistical manner. Therefore, in recent studies, meta-analysis was widely used to assess the overall summary effect of variables.
Plant responses to eCO2 have been thoroughly researched through meta-analyses, and plant growth has been shown to increase to different extents under eCO2 (Curtis and Wang, 1998; Poorter and Pérez-Soba, 2001; Jablonski et al., 2002; Ainsworth and Long, 2005; De Graaff et al., 2006; Duval et al., 2012). Nevertheless, the interactions between mycorrhizal fungi and plants under eCO2 were not considered in these previous studies, and only a few meta-analyses have addressed mycorrhizal fungi under eCO2 (Treseder, 2004; Alberton et al., 2005; Terrer et al., 2016). Treseder (2004) showed a greater increase in AM abundance compared to ECM abundance under eCO2 but did not assess the difference between AM and ECM plant responses. Alberton et al. (2005) reported higher response in ECM growth compared to AM fungal growth and a slightly but not significantly higher response in ECM compared with AM plant growth using a mixed parameters method. Terrer et al. (2016) showed a higher increase in biomass in ECM plants than in AM plants under both N-limiting and non-N-limiting conditions. Since inconsistent patterns of AM and ECM plants symbionts were reported in current meta-analysis studies, it is difficult to reach a conclusion of how eCO2 affects mycorrhizal plants and fungal growth in AM and ECM symbionts, Therefore, we conducted a meta-analysis to determine the different effects of eCO2 on AM and ECM symbionts.
In this meta-analysis, the effect sizes of 27 individual variables (Table 1) were calculated from 434 observations from 1987 to 2016 to quantify the effects of the individual variables on mycorrhizal fungal and plant growth under eCO2. Additionally, the meta-analysis aimed to answer the following questions: (1) what are the different patterns of mycorrhizal plant biomass and nutrients and of mycorrhizal fungal growth in AM and ECM symbionts under eCO2? and (2) how do other factors (species richness, experimental duration and fertilization) affect mycorrhizal plant and fungal biomass, and do they exhibit any interesting patterns under eCO2? Determining the patterns of mycorrhizal plant and fungal growth under eCO2 would help to improve our understanding of the interactions between mycorrhizal plant and fungal symbionts during the current global climate change involving eCO2.
Materials and Methods
Data Collection
Publications were searched using the ISI Web of Science search tool (Thompson Reuters). On December 12, 2016, we conducted a search using the terms “mycorrhiz*” and “CO2” or “mycorrhiz*” and “carbon dioxide,” and the search resulted in 1,140 publications. Papers were included when they met the following criteria: mycorrhizal fungi can be clearly identified as AM or ECM; at least one of the 27 variables was given; and means and sample sizes were reported. For each paper, data resulting from studies with different mycorrhizal fungal species, host plant species, experimental durations and nutrients levels were considered independent studies. When multifactorial studies appeared, only data of control groups and eCO2 groups were used. When papers reported the same data, we selected one of them (Kohler et al., 2009, 2010). We obtained 434 observations from 112 papers (Appendix S1) by using the above criteria and removing the duplicates.
The mycorrhizal fungal and plant species richness, experimental durations, fertilization conditions (Figure 2) and 27 variables (Table 1) were collected for each study. Engauge software was used to extract data that were provided in graphical form. When observations lacked total plant biomass, shoot biomass, root biomass or shoot-to-root ratio, the missing parameters were calculated using the following formulas: total biomass = shoot biomass + root biomass and shoot-to-root ratio = shoot biomass/root biomass. Standard deviations (SDs) were calculated when only standard errors (SEs) were reported by using the equation SD = SE × sqrt(n). Unidentified error bars were assumed to represent SEs. For the studies that did not report SDs, we calculated the average coefficient of variation (CV) within each dataset and then approximated the missing SD by multiplying the reported mean by the average CV. The number of treatments listed in the text was replicates of a treatment rather than the sample size per treatment. When the value of n was given as a range, the smallest value was taken.
Moderators
Each mycorrhizal fungal type was grouped into either the AM or the ECM category. Plant and mycorrhizal fungal species richness was grouped into the “single” and “mixture” categories. Treatment durations spanned from 5 days to 14 years and were grouped into two experimental durations: ≤1 year and >1 year. The fertilization conditions included two groups: N addition (high N and low N) and P addition (high P and low P). The ambient CO2 levels ranged from 336 to 400 ppm, and the eCO2 levels ranged from 550 to 1,000 ppm except in four studies that reported eCO2 concentrations greater than 1,000 ppm (1,500, 3,360, and 10,000 ppm in two studies), which were included in our meta-analysis.
All 27 variables (Table 1) except the mycorrhizal fungal biomass were used individually in the meta-analysis according to Augé et al. (2014). Alberton et al. (2005) tested whether mycorrhizal plants and mycorrhizal fungi responded similarly under eCO2. To extract as much information as possible from the scarce papers available at the time, Alberton et al. provided an order of measurements to ensure that the plant and fungal responses were maximally different and noted the possibility that not all parameters were unbiased. The meta-analysis of mycorrhizal fungal biomass in our study was conducted according to the method described by Alberton et al. (2005) because there were not enough observations representing the allocation of mycorrhizal fungal biomass.
The data used for calculating the mycorrhizal fungal biomass responses included the dry weight of extraradical mycelia, extramatrical hyphae mass, hyphal biomass, fungal biomass in soil, total fungal biomass, ECM tip biomass, mycorrhizal mass, fungal biomass in root, specific phospholipid fatty acid (PLFA) content, neutral lipid fatty acid (NLFA) content, and ergosterol content according to the rank-order method described by Alberton et al. (2005).
Meta-Analysis
The response ratio (R), which was defined as the “effect size,” was calculated as the ratio of the values in the eCO2 treatment group (Xt) to those in the control group (Xc) (Hedges et al., 1999). We performed a log transformation on the response ratio R to develop a better statistical understanding as follows (Hedges et al., 1999):
The variance of loge R was calculated using the following formula:
where st and sc represent the SDs of the treatment and control groups, respectively. In addition, nt and nc are the sample sizes of the treatment and control groups, respectively.
The variance, vn, was adjusted by the number of observations (n) in each study and was calculated by the following formula:
where n represents the number of observations from the same publication.
The final weighted effect sizes, loge Ri′, and the mean effect sizes, were calculated by MetaWin 2.0 software using the loge Ri′ and vn values.
To obtain a clearer understanding, the percentage changes of the mean effect sizes were transformed with the following formula:
These four equations were described in Bai et al. (2013).
Heterogeneity was estimated with the Q statistic in MetaWin 2.0 software. Total heterogeneity (Qt) was composed of the difference between group cumulative effect sizes (Qm) and residual error (Qe) (Rosenberg et al., 2000). I2 is an index that assesses the ratio of true heterogeneity to the total heterogeneity across the observed mean effect sizes and is calculated as: (Qt – df)/Qt, where the degrees of freedom (df) represent the expected variation and (Qt – df) represents the true heterogeneity (Rosenberg et al., 2000; Higgins and Thompson, 2002; Huedo-Medina et al., 2006; Borenstein et al., 2009). The value of I2 ranges from 0 to 100%. The value of 0% indicates no heterogeneity exists among the variable dataset. The larger the value of I2, the larger the dataset's true heterogeneity. Some studies suggested that assumptions of heterogeneity were considered invalid when p-values were less than 0.1 (Higgins et al., 2003; Wilson et al., 2005; Allen et al., 2008). In this study, we used a random-effect model for all of the variables in the meta-analysis. When significant heterogeneities were identified among studies, the sources of true heterogeneity were investigated with moderator or subgroup analysis.
The 95% confidence intervals (CIs) of the weighted effect sizes were obtained using the bootstrapping (9,999 iterations) function in MetaWin software. The weighted mean effect size of a variable was considered significant when the 95% CI did not overlap zero and the p-value was less than 0.05 (Borenstein et al., 2009). A random-effect model was used to test the relationships between the weighted mean effect sizes of all the variables, experimental duration and the CO2 fold change using MetaWin software.
Publication bias was estimated with the Egger test function in Stata 12.0 software, and the estimates were obtained from the mean effects and variance. A p-value < 0.05 indicated a potential publication bias was present (Egger et al., 1997; Sterne et al., 2000; Deeks et al., 2005). The trim and fill method was used when there was a publication bias in the variable dataset (Duval and Tweedie, 2000; Peters et al., 2007).
Results
Overall Summary Effects
A total of 112 papers were included in this meta-analysis. Egger test was conducted to assess publication bias on the mean effect sizes of the 27 variables mentioned in the Data Collection section of Materials and Methods. Seventeen of the 27 variables exhibited no publication bias (p > 0.05) in their mean effect sizes. The datasets of 10 variables showed potential publication biases (p < 0.05), but seven of these sets showed no publication bias with the unchanged mean effect sizes after a “trim and fill” correction (Table S1).
The raw mean effect sizes of two variables (root with arbuscules and P concentration in the leaf) with true publication bias were slightly overestimated, but no subversive changes were found, and corrections were performed with the trim and fill method (Figure 1). Only the mean effect size of the P content in shoot, which was +0.126 and −0.066 before and after (Figure 1A) the adjustment, respectively, was changed subversively after trim and fill corrections.
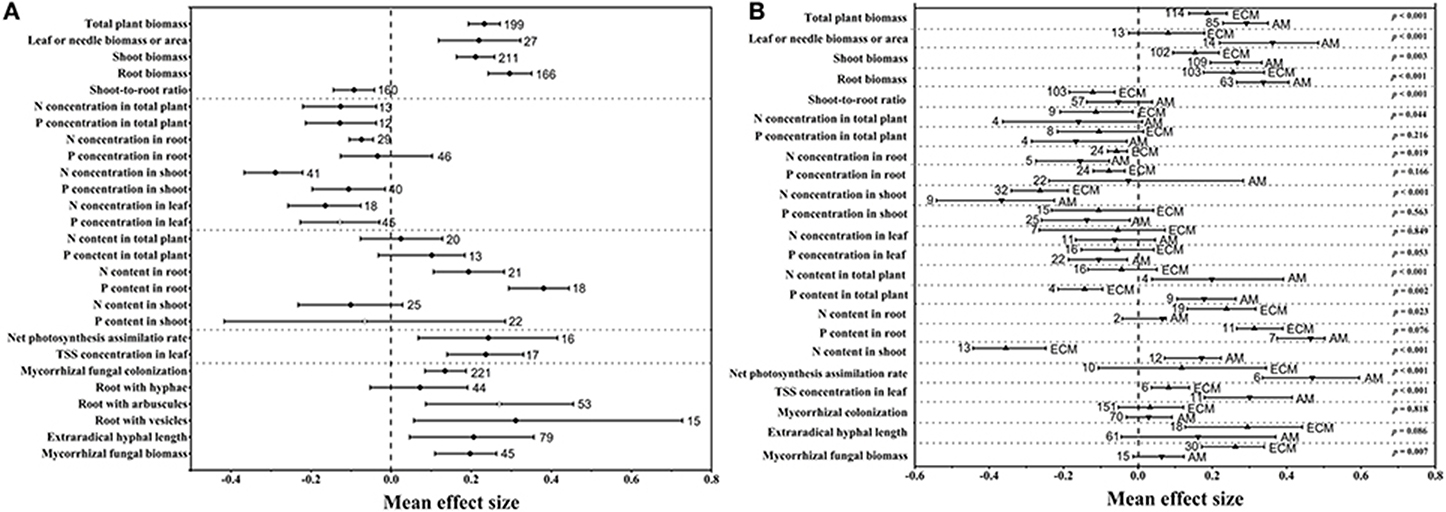
Figure 1. Mean effect sizes of eCO2 on 27 variables related to mycorrhizal fungi and plants in all groups (A) and in the AM and ECM (B) groups. TSS, total soluble sugar. Error bars represent 95% CIs. Open points are effect sizes that were corrected by the trim and fill method. The dashed line shows where the mean effect sizes are equal to zero. The effect size of eCO2 was considered significant when the 95% CI of the effect size did not contain zero. The sample size for each variable is shown next to the corresponding point.
As illustrated in Figure 1A, the mycorrhizal plant biomass responded positively to rising CO2 concentrations. The mean effect size of the total plant biomass was +0.233. The mean effect sizes of the leaf or needle biomass or area, shoot biomass and root biomass were +0.219, +0.211, and +0.296, respectively. However, there was a bias in the allocation of total biomass to the shoots and roots under eCO2, which led to a shoot-to-root ratio mean effect size of −0.093 (Figure 1A). Their 95% CIs did not overlap 0, indicating a significantly positive effect of the eCO2 atmosphere on plant biomass. Regarding mycorrhizal fungal development, CO2 enrichment significantly and positively affected extraradical hyphal length, with a mean effect size of +0.206 (Figure 1A). However, there was no significant difference in extraradical hyphal length between AM and ECM fungi (Figure 1B), although eCO2 significantly affected ECM extraradical hyphal length but had an insignificant effect on AM fungi. The mycorrhizal fungal biomass increased by +21.77% (Figure 1A), which was significant. Mycorrhizal fungal colonization, a variable associated with plants and mycorrhizal fungi, was significantly increased under eCO2. Moreover, the mean effect sizes of eCO2 on roots with hyphae, arbuscules and vesicles were +0.072, +0.270, and +0.311, respectively (Figure 1A). The AM plants displayed significantly larger effect sizes on biomass and nutrient contents than the ECM plants (Figure 1B). However, the ECM fungi showed a greater mycorrhizal fungal biomass response than the AM fungi (Figure 1B). The N and P concentrations were significantly decreased in all organs under eCO2 except for the P concentrations in root (Figure 1A). CO2 enrichment had a significantly positive effect on the N and P contents in root, whereas nonsignificant effects were found on the total plant and shoot N and P contents (Figure 1A). The N (p < 0.001) and P contents (p = 0.002) showed greater responses in whole AM plants than in whole ECM plants (Figure 1B). Significantly positive effects of eCO2 on the net photosynthesis assimilation rate (Pn) and total soluble sugar (TSS) concentration in the leaf were also found in this study (Figure 1A), and both responses were increased more in AM plants than in ECM plants (Figure 1B).
Subgroup Moderator Analysis
To test for interesting patterns, a subgroup analysis was conducted with six variables (total plant biomass, shoot biomass, root biomass, mycorrhizal fungal colonization, extraradical hyphal length, and mycorrhizal fungal biomass) that directly reflect the mycorrhizal fungi and plant growth and five moderators (plant species richness, mycorrhizal fungal species richness, experimental duration, N addition, and P addition).
Species Richness of Mycorrhizal Fungi and Plants
Species richness affected the responses of mycorrhizal fungi and plants differently. Specifically, the plant species richness significantly affected the shoot biomass (p = 0.001), mycorrhizal fungal colonization (p < 0.001) and extraradical hyphal length (p < 0.001), whereas the mycorrhizal fungal species richness significantly influenced the total plant biomass (p < 0.001), shoot biomass (p < 0.001) and fungal colonization (p < 0.001). The responses of mycorrhizal fungal colonization (Figure 2D) and extraradical hyphal length (Figure 2E) were greater in single plant than in mixtures of plants. The total plant biomass (Figure 2A), shoot biomass (Figure 2B) and mycorrhizal fungal colonization (Figure 2D) showed greater increases in mixtures of fungi than in single fungus.
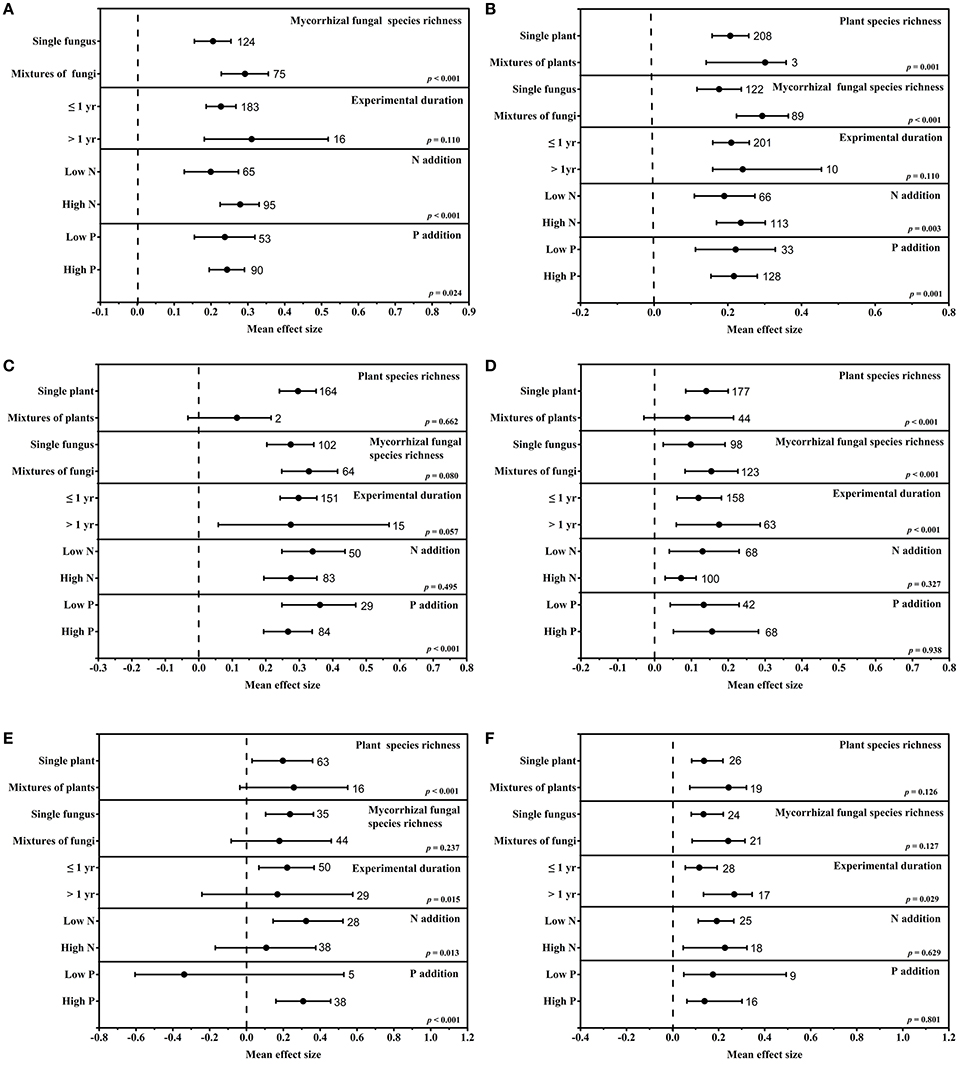
Figure 2. Subgroup moderator analysis of the mean effect sizes of eCO2 on the total plant biomass (A), shoot biomass (B), root biomass (C), mycorrhizal colonization (D), extraradical hyphal length (E) and mycorrhizal fungal biomass (F). Variables were subgrouped into the following moderators: species richness (plants and mycorrhizal fungi), experimental durations and fertilization conditions (N addition and P addition). Missing subgroup moderators are absent due to inadequate observations involving those moderators in the meta-analysis. The dashed line shows where the mean effect size is equal to zero. The effect size of eCO2 was considered significant when the 95% CI of the effect size did not contain zero. The sample size for each moderator is shown next to the corresponding point.
Experimental Durations
The experimental duration significantly affected the mycorrhizal fungi but had no significant effects on mycorrhiza-associated plants. The extraradical hyphal length was more affected under short-term conditions than under long-term conditions, whereas the colonization and fungal biomass exhibited greater effects under long-term conditions (Figures 2D,E).
Fertilization Conditions
The addition of fertilizers (N or P) to the soil changed the mycorrhizal fungal and plant responses to eCO2. Plant biomass was enhanced more under high-nutrient conditions (Figure 2A). P addition significantly increased the shoot biomass, whereas N addition did not significantly affect the shoot biomass (Figures 2B,C). There was no significant difference in effect the on mycorrhizal fungal colonization and fungal biomass under eCO2 between N enriched and low N conditions (Figures 2D,F). The extraradical hyphal length showed a greater response under low N or high P conditions than under other conditions (Figure 2E).
The sources of true heterogeneity were calculated using the Q statistic method. Two of the 27 parameters in our study had an I2-value of zero, whereas the other 25 parameters had positive I2 values. Twenty of these 25 parameters had significant heterogeneity (Phetero < 0.1) (Table 1), which indicated true variation among these results; random-effect models were then used to assess the final mean effect sizes. True heterogeneity indicates that certain moderators significantly influence the variables' responses to eCO2. Therefore, a subgroup analysis was conducted to investigate the sources of true heterogeneity in datasets of only five variables (Figures 2A–E) due to the limited number of observations. As illustrated in Figure 2, the plant species richness significantly affected the responses of shoot biomass, mycorrhizal fungal colonization, and extraradical hyphal length to eCO2. The mycorrhizal fungal species richness and P addition significantly affected the responses of all five variables except the extraradical hyphal length to eCO2. The experimental duration significantly affected the responses of mycorrhizal fungal colonization and extraradical hyphal length to eCO2. In addition, N addition significantly affected the responses of total plant biomass, mycorrhizal fungal colonization and extraradical hyphal length to eCO2.
Meta-Regression Analysis
The mycorrhizal fungal biomass was significantly correlated with the experimental duration (Table 2). It increased with increasing experimental duration but exhibited a relatively small slope (+0.0032). The N and P concentrations in shoot and the N and P concentrations in root were significantly correlated with the CO2 fold change. The N (slope of approximately −0.063) and P concentrations in shoot (slope of −0.083) and the N (slope of −0.090) and P concentrations in root (slope of −0.075) decreased as the CO2 fold change increased. The extraradical hyphal length was significantly correlated with the CO2 fold change, with a slope of +1.100.
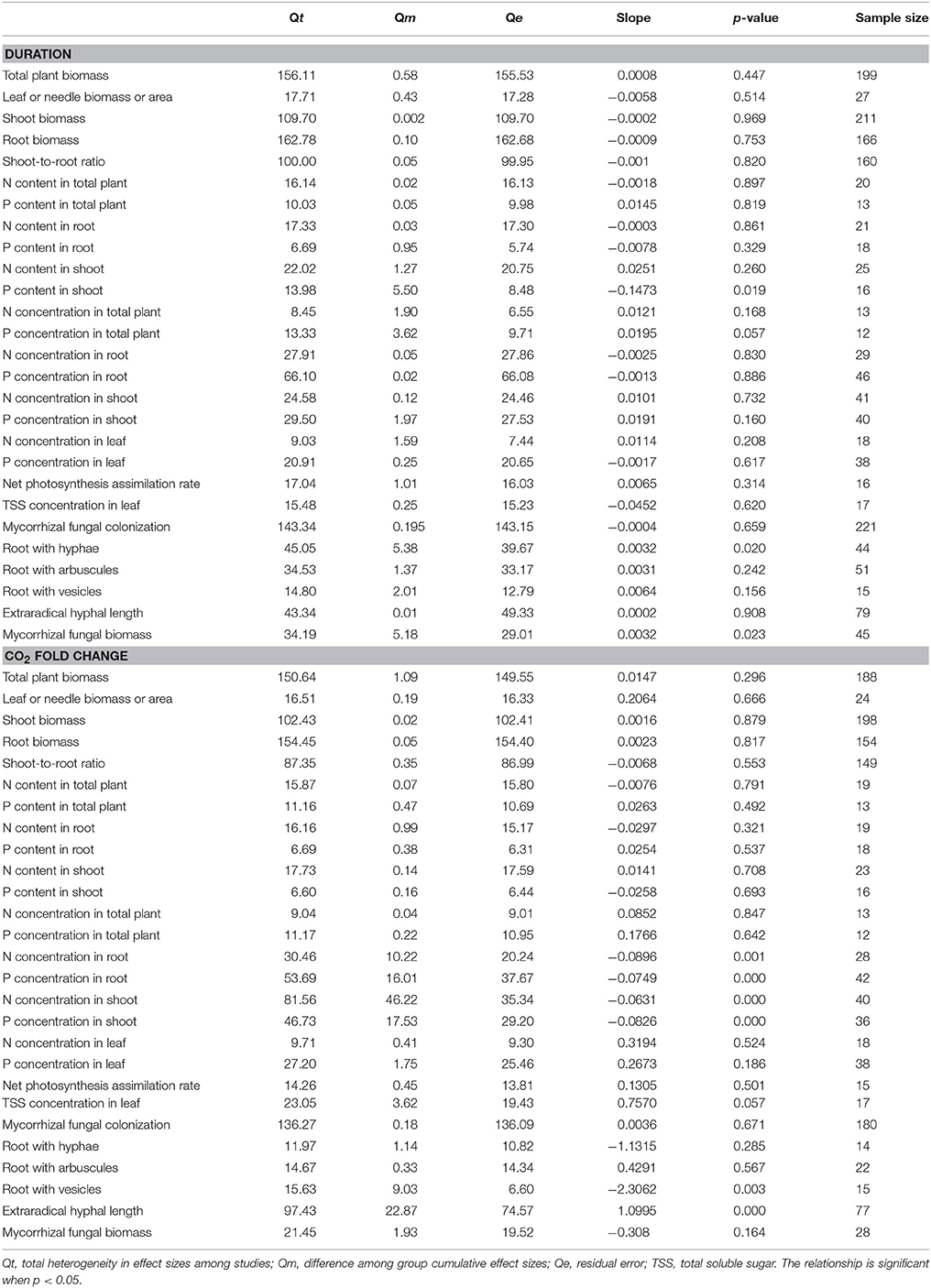
Table 2. Relationships between the effect sizes of eCO2 on mycorrhizal fungal and plant development parameters and the experimental duration and CO2 fold change.
Discussion
A meta-analysis, which is a statistical analysis method that combines data from independent studies, is used to determine whether the effect of a variable is consistent across a dataset and to check the potential variance in the effects within the dataset (Huque, 1988; Gurevitch and Hedges, 2001; Borenstein et al., 2009). Biases, including both publication and research bias, are important and complicating issues in a meta-analysis. Publication bias commonly results in overestimated mean effect sizes and significance (Gurevitch and Hedges, 2001) and is generally caused when studies with negative results are less frequently accepted than studies with positive results. The trim and fill method is used to correct the publication bias in a meta-analysis (Duval and Tweedie, 2000). In our study, the publication bias among the 27 variable datasets was taken into consideration, and the mean effect sizes with publication bias were corrected using the trim and fill method according to the study of Duval and Tweedie (2000) (Table 1, Figure 1A). Research bias is a more troublesome issue in meta-analysis since it originates from a variety of sources (Gurevitch and Hedges, 1999). For instance, the objects of the studies, the methods and the experimental conditions are subjectively selected by researchers, potentially resulting in research bias. The selection of the parameters to investigate could also result in research bias. For example, fractional colonization was not the most suitable parameter for assessing the performance of mycorrhizal fungi under eCO2 but was used frequently in previous studies because it is relatively easy to assess (Alberton et al., 2005). Klironomos et al. (2005) demonstrated that the magnitude of changes in fungal species richness and function was smaller in response to gradually increasing CO2 than in response to abruptly rising CO2, indicating a possible overestimation of the effects of enriched CO2 in studies using abruptly rising atmospheric CO2. Until now, however, quantifying research bias has been difficult because no formula or model could be fitted to predict research bias.
Positive effects were found in the responses of mycorrhizal plants and fungi to eCO2 in our study. The increases in total plant biomass (+26.20%) was consistent with the increases observed in other meta-analyses [+28.8% in the study conducted by Curtis and Wang (1998), +47% in that performed by Poorter and Pérez-Soba (2001), and +31% in the analysis conducted by Jablonski et al. (2002)]. These above previous studies did not consider the mycorrhizal fungal interaction, whereas our study only included plants associated with mycorrhizal fungi. In addition, in our study, the increases in mycorrhizal fungal colonization length and mycorrhizal fungal biomass (+14.88 and +22.23%, respectively) were smaller than the increases in mycorrhizal fungal abundance and colonization (+47 and +36%, respectively) reported by Treseder (2004). This difference may be because the meta-analysis of Treseder (2004) included only field studies and the smaller number of observations (14) compared to the number of observations in our study (434). As shown in Figure 1A, eCO2 increased the plant biomass and led to a smaller shoot-to-root ratio, resulting in a larger increase in root biomass than shoot biomass under eCO2. The shoot-to-root ratio depends on the partitioning of photosynthesis products, which might be affected by environmental and nutrient conditions (Rogers et al., 1995). Previous studies have elucidated that the partitioning of dry matter into shoots and roots is determined by the internal balance between labile N and C in the shoot and root systems (Ericsson, 1995). The N:C ratios in the mycorrhizal plant shoots and roots were found to be decreased in our study. An overall negative effect (−0.144) on the root-to-shoot ratios of AM-colonized plants was detected in the meta-analysis conducted by Veresoglou et al. (2012), a result opposite to the effect on the shoot-to-root ratio observed in our study (−0.052). Moreover, factors other than the nutrient supply rates that decrease growth rates have been reported to increase the N:C and P:C ratios (Sterner et al., 2002). Decreased N:C and P:C ratios were found in our study. Various mechanisms that could explain the decreased N and P concentrations in plants under eCO2 include the following: (1) the N or P content of plant organs is diluted by the enhanced photosynthetic assimilation of C (Kuehny et al., 1991; Gifford et al., 2000) and secondary compounds (Gifford et al., 2000); (2) decreased transpiration leads to decreased N uptake (McDonald et al., 2002; Del Pozo et al., 2007); and (3) N is incrementally lost (Pang et al., 2006) and the mycorrhization status decreases (BassiriRad et al., 2001; Alberton et al., 2005). The decreased N:C and P:C ratios observed in our study may be explained by the first mechanism described above, i.e., that the enhanced photosynthetic assimilation of C dilutes the N and P contents in plants. Plants generally maintain a homeostatic N:P ratio that is sensitive to environmental changes (Loladze and Elser, 2011). However, significant different responses detected in mycorrhizal plants and mycorrhizal fungi (Alberton et al., 2005), which may result in more complicating changes in roots under eCO2 since the root is a dual organ. We further deduced that the different responses in mycorrhizal fungi and plants may result in a more imbalanced N:P ratio in roots. Our study demonstrated that the increase in the P content (+13.48%) under eCO2 was greater than that in the N content (+2.45%) and also found a +26.20% increase in total plant biomass. The enhanced total plant biomass and lower N:P ratio observed in our study could be explained by the growth rate hypothesis, which assumes a low plant N:P ratio when the growth rates are enhanced (Sterner et al., 2002; Elser et al., 2010). In addition, the meta-regression results demonstrated that the decreased N and P concentrations in shoots and roots were significantly correlated with the amount of CO2 level change. This correlation indicated that the N and P concentrations in plant organs were influenced more strongly by the CO2 concentration than by the exposure period (Table 2).
Previous studies showed no significant difference in the responses of ECM plants (+1.26) and AM plants (+1.25) (Alberton et al., 2005) or showed a larger effect in ECM plants (+33 ± 4%) than in AM plants (+20 ± 6%) (Terrer et al., 2016). However, our study demonstrated that AM plants showed larger increases in their biomasses (+33.90%) and their N (+21.99%) and P contents (+19.48%) than the ECM plants (changes of +20.57% in biomass, −4.28% in N content and −13.35% in P content). The above data indicating opposite results in our study compared to previous studies, and this may be explained by the different parameters used and different number of observations in these meta-analyses. For instance, Alberton et al. (2005) conducted the meta-analysis using mixed parameters rather than single parameters in our study. Terrer et al. (2016) assessed the responses of plant biomass using a smaller dataset (27 and 56 observations in AM and ECM plants, respectively) than that in our study (85 and 114 observations in AM and ECM plants, respectively). The larger total plant biomass observed in AM plants (+33.90%) than in ECM plants (+20.57%) in our study may be the result of the larger increase in the net photosynthesis assimilation rate in AM plants (+59.86%) than in ECM plants (+12.50%). This possibility was supported by the higher gross and net plant primary production (GPP and NPP) in AM-dominated ecosystems reported by Averill et al. (2014) and Vargas et al. (2010). Regarding to mycorrhizal fungal growth in our study, ECM fungi demonstrated stronger responses in terms of mycorrhizal fungal biomass (+29.98%) under eCO2 compared with AM fungi (+6.61%) (Figure 3). The larger biomass in ECM fungi observed in our study could be explained by the following mechanisms: it is reported that approximately 30% of the total photoassimilation products are used to maintain fungal growth (Nehls and Hampp, 2000), and ECM plants typically allocate more C to their fungal partner than do AM plants (Gehring et al., 2006; Orwin et al., 2011; Soudzilovskaia et al., 2015). Furthermore, one proposed framework postulated that forests dominated by AM trees have an inorganic nutrient economy, whereas forests dominated by ECM trees have an organic nutrient economy (Phillips et al., 2013). The framework in Phillips et al. (2013) speculate that the slow decomposition of litter in these soils results in a greater accumulation of soil organic matter (SOM). Thus, the large proportion of C allocating belowground is used by ECM fungi to acquire N and P from SOM. For different patterns in AM and ECM plants, the larger ECM plant biomass reported in a previous study (Terrer et al., 2016) was explained by the following mechanism: AM trees have a faster leaf litter decomposition rate than ECM trees, this rapid decomposition of AM leaf litter results in the formation of stable mineral-associated organic matter in AM-symbiont soil systems (Sulman et al., 2014; Cotrufo et al., 2015) that cannot be absorbed by AM fungi and plants (Cornelissen et al., 2001; Read and Perez-Moreno, 2003). The above-described evidence indicated that ECM fungi could provide more available N for plants. However, the significantly higher N and P contents in AM plants than in ECM plants obtained in our study may be explained by the possibility that the uptake of plant nutrients might depend heavily on the high C accumulation from photosynthesis under eCO2, which was lower in ECM plants than in AM plants. The results in our study corroborate the results described by Phillips et al. (2013), who found a smaller N:C ratio in ECM-dominated plots than in AM-dominated plots. The above data indicated that AM symbionts contributed more to plant growth than ECM symbionts, whereas ECM symbionts contributed more to mycorrhizal fungal growth.
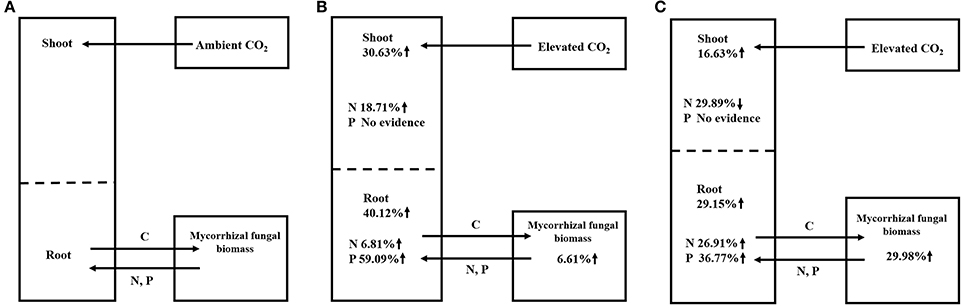
Figure 3. Summary of mycorrhizal plant-fungal symbiont responses to aCO2 (A), AM symbiont responses to eCO2 (B), and ECM symbiont responses to eCO2 (C).
In our study, the responses of mycorrhizal fungi to eCO2 differed significantly between the short-term group and the long-term group. However, the responses of mycorrhizal plants to eCO2 did not differ significantly between the short-term and long-term groups, indicating a reduced positive effect as the experimental duration was extended. Many mechanisms could explain this positive acclimation effect in plant biomass responses under eCO2. Prolonged exposure to eCO2 generally reduces the initial stimulation of photosynthesis in many species (Long and Drake, 1991; Ziska et al., 1991) and frequently suppresses photosynthesis (Couture et al., 2014; Kostiainen et al., 2014). These plant responses are further attributed to secondary responses that are related to either excess carbohydrate accumulation or decreased N content rather than directly to eCO2 (Chapin et al., 1987; Makino and Mae, 1999). First, increased photosynthesis in response to eCO2 could result in excess carbohydrates in plants, as described above, which would subsequently downregulate photosynthesis. Second, as shown in our study, the N in plants was diluted in the absence of an increased nutrient supply in the soil, resulting in decreased photosynthesis and hence a gradual decrease in the beneficial effect. A reduced positive effect might also be found for progressive nitrogen limitation (PNL) in long-term experiments. PNL describes the notion that the stimulation of plant growth by eCO2 results in increased N sequestration in plants, litter and SOM, eventually leading to a progressive decline in soil N availability for plant growth over time (Luo et al., 2004). The reduced N availability, in turn, constrains the eCO2 fertilization effect on plant growth over longer timescales, and the mycorrhizal plant partners cause mycorrhizal fungal-induced PNL, reducing the positive effect in plants (Alberton et al., 2007; Liang et al., 2016). A primary mechanism driving this response is the rapid rate of N immobilization by plants and microbes under eCO2, which depletes the soil N content and causes slower rates of N mineralization (Finzi et al., 2006). Both AM (Hodge and Fitter, 2010) and ECM (Franklin et al., 2014) fungi can therefore immobilize substantial amounts of N in their tissues, and an increased fungal response to eCO2 could result in what has been described as the PNL, as argued by Alberton et al. (2007). The significantly different responses of mycorrhizal fungi to eCO2 in the short-term and long-term groups in our study indicate that the PNL has little effect on mycorrhizal fungi.
Larger significant effects on the total plant and shoot biomass were observed in the high N addition experiments in our study. Two mechanisms might explain the improved plant biomass observed with high N addition under eCO2 as follows: 1) the addition of N increases the N:C ratio and thereby relieves the negative photosynthesis feedback caused by N limitation, and 2) the increased N input could relieve mycorrhiza-induced PNL (Alberton and Kuyper, 2009) and offset the PNL by increasing the ecosystem N capital under eCO2 (Luo et al., 2004). Phillips et al. (2009) reported evidence that the increased allocation of C to root exudates might be a mechanism that delays PNL in forested ecosystems. The lack of a significant difference in the effect sizes of root biomass between low N (+0.340) and high N (+0.275) conditions observed in our study might provide supporting evidence for this hypothesis. One hypothesis assumes that plants would invest more C in mycorrhizal fungi when N or P limited plant growth, since mycorrhizal fungi contribute to the nutrient uptake of plants (Mosse and Phillips, 1971). This report opposite to the idea that the increased N supply might suppress the abundance of mycorrhizal fungi (Treseder, 2004). Plants in nutrient-rich or well-fertilized (high N, high P) soils tend to be less frequently colonized by AM fungi (Staddon et al., 2004), and our study showed that changes in mycorrhizal fungal colonization depend heavily on N sufficiency rather than P sufficiency. Jakobsen et al. (2016) reported that the plant growth responses to elevated atmospheric CO2 are increased by a sufficient P supply rather than by arbuscular mycorrhizae. Plant P acquisition is increased by extensive root development and is therefore determined by the C status of plants; notably, the P status influences plant photosynthesis and growth rates, leading to multiple C-P interactions (Jakobsen et al., 2016). Our study showed a slightly higher increase in plant biomass under high P conditions than under low P conditions, and the decreased total plant N:P ratio in our study might indicate potential shifts from P limitation to N limitation in plants. Furthermore, the increased C status of pines under eCO2 might facilitate the uptake of limiting P in native ecosystems (Delucia et al., 1997). Integrating nutrient dynamics into terrestrial C cycle models, particularly the limitations on plant growth imposed by N and P availability, has suggested that the land C sink is overestimated in models without these limitations (Wang and Houlton, 2009; Wang et al., 2010; Zhang et al., 2014). A larger increase in the extraradical hyphal length under high P conditions was found in our study, and this finding might be explained by the need for longer extraradical hyphae to obtain more N. While the N addition relieved PNL in plants, larger C increases resulted in positive feedback in mycorrhizal fungi. However, the addition of N to P-rich soils decreased the AM fungal biomass and the mycorrhizal benefits for plant growth (Blanke et al., 2005), whereas N fertilization of P-limited soils increased the fungal biomass and plant growth benefits (Johnson et al., 2003). This finding was consistent with the larger mycorrhizal fungal biomass observed in our study under high N and low P conditions.
The difference between mixtures of different plants and single species of plants had no significant effects on root biomass, indicating that plant species richness did not significantly affect the root biomass under eCO2 in our study. Furthermore, a previous study reported that eCO2 reduced the loss of plant diversity caused by N deposition (Reich, 2009), and afterward, the whole ecosystem responded to eCO2 changes by a feedback effect caused by the plant community shift (Langley and Megonigal, 2010). The mycorrhizal fungal species composition can also be changed by eCO2 (Denef et al., 2007; Cotton et al., 2015; Godbold et al., 2015). Several studies have reported that eCO2 alters the fungal communities, and positive responses in terms of fungal species richness are rare (Lipson et al., 2014). Our study showed that mixtures of mycorrhizal fungi had greater effects on total plant biomass, root biomass and mycorrhizal fungal colonization under eCO2. Rillig et al. (1999) suggested that finding direct relationships between structural data and functional changes is difficult, although a previous study (Klironomos et al., 1998) demonstrated shifts in the mycorrhizal fungal composition. The manner in which functional diversity changes with alterations in the mycorrhizal fungal species richness is not well understood. Klironomos et al. (2005) reported that eCO2 leads to a loss of the most C-demanding AM fungi, whereas Kohler et al. (2010) showed decreased fungal species richness unaccompanied by changes in functional diversity. Additional studies should be conducted to assess the functional changes that result from shifts in the mycorrhizal fungal species composition.
In summary, we observed some new findings by our meta-analysis, and the different patterns between AM and ECM symbionts under eCO2 in our study could be overall described as follows: the net photosynthesis assimilation rate increased more in AM plants than in ECM plants under eCO2, which led to a larger biomass in AM plants than in ECM plants. Subsequently, the total plant nutrient contents (N and P) increased. Larger contents of N in AM plant shoots resulted in positive feedback to the net photosynthesis assimilation rate in the short-term. In addition, lower N:P ratios in AM plants resulted in a larger biomass in AM plants, according to the growth rate hypothesis. However, as the experimental duration increased, the excessive accumulation of photosynthates and the reduced N and P concentrations reduced the positive effect on plant biomass, which in turn resulted in a lower rate for the increase in plant biomass in the long-term experiments. Thus, eCO2 strengthened the relationship between mycorrhizal plants and fungi, which led to a greater increase in mycorrhizal fungal biomass, and ECM benefited more from this increase. The exogenous input of N significantly improved plant biomass while inhibiting extraradical hyphae extension.
Conclusions
Our study demonstrated distinctly different patterns between AM and ECM symbionts under eCO2: AM symbionts exhibit greater plant growth, whereas ECM symbionts show greater fungal growth. In addition, the species richness, experimental duration, and fertilization were found to influence the responses of mycorrhizal fungal-plant symbionts to eCO2.
Figuring out these different patterns in the responses of mycorrhizal plant-fungal symbionts to eCO2 will aid the identification of trends in the development of mycorrhizal plants and fungi under eCO2.
Author Contributions
YD, HS, WY, and HX planned and designed the research. YD and HS collected and analyzed the data and wrote the manuscript, and YD and ZW organized the manuscript structure. WY, ZW, and HX polished the English to improve the quality of this manuscript. All authors reviewed and approved the final manuscript.
Conflict of Interest Statement
The authors declare that the research was conducted in the absence of any commercial or financial relationships that could be construed as a potential conflict of interest.
Acknowledgments
This work was supported by the Non-profit Research Foundation for Agriculture (201103039).
Supplementary Material
The Supplementary Material for this article can be found online at: https://www.frontiersin.org/articles/10.3389/fmicb.2018.01248/full#supplementary-material
Table S1. Results of the Egger test for publication bias for 27 variables and of the trim and fill analysis for variables with potential publication bias.
Appendix S1. References used in this meta-analysis.
References
Ainsworth, E. A., and Long, S. P. (2005). What have we learned from 15 years of free-air CO2 enrichment (FACE)? A meta-analytic review of the responses of photosynthesis, canopy properties and plant production to rising CO2. New Phytol. 165, 351–372. doi: 10.1111/j.1469-8137.2004.01224.x
Alberton, O., and Kuyper, T. W. (2009). Ectomycorrhizal fungi associated with Pinus sylvestris seedlings respond differently to increased carbon and nitrogen availability: implications for ecosystem responses to global change. Global Change Biol. 15, 166–175. doi: 10.1111/j.1365-2486.2008.01714.x
Alberton, O., Kuyper, T. W., and Gorissen, A. (2005). Taking mycocentrism seriously: mycorrhizal fungal and plant responses to elevated CO2. New Phytol. 167, 859–868. doi: 10.1111/j.1469-8137.2005.01458.x
Alberton, O., Kuyper, T. W., and Gorissen, A. (2007). Competition for nitrogen between Pinus sylvestris and ectomycorrhizal fungi generates potential for negative feedback under elevated CO2. Plant Soil 296, 159–172. doi: 10.1007/s11104-007-9306-5
Allen, N. C., Bagade, S., McQueen, M. B., Ioannidis, J. P., Kavvoura, F. K., Khoury, M. J., et al. (2008). Systematic meta-analyses and field synopsis of genetic association studies in schizophrenia: the SzGene database. Nat. Genet. 40, 827–834. doi: 10.1038/ng.171
Augé, R. M., Toler, H. D., and Saxton, A. M. (2014). Arbuscular mycorrhizal symbiosis and osmotic adjustment in response to NaCl stress: a meta-analysis. Front. Plant Sci. 5:562. doi: 10.3389/fpls.2014.00562
Augé, R. M., Toler, H. D., and Saxton, A. M. (2015). Arbuscular mycorrhizal symbiosis alters stomatal conductance of host plants more under drought than under amply watered conditions: a meta-analysis. Mycorrhiza 25, 13–24. doi: 10.1007/s00572-014-0585-4
Averill, C., Turner, B. L., and Finzi, A. C. (2014). Mycorrhiza-mediated competition between plants and decomposers drives soil carbon storage. Nature 505, 543–545. doi: 10.1038/nature12901
Bai, E., Li, S., Xu, W., Li, W., Dai, W., and Jiang, P. (2013). A meta-analysis of experimental warming effects on terrestrial nitrogen pools and dynamics. New Phytol. 199, 441–451. doi: 10.1111/nph.12252
BassiriRad, H., Gutschick, P. V., and Lussenhop, J. (2001). Root system adjustments: regulation of plant nutrient uptake and growth responses to elevated CO2. Oecologia 126, 305–320. doi: 10.1007/s004420000524
Baum, C., El-Tohamy, W., and Gruda, N. (2015). Increasing the productivity and product quality of vegetable crops using arbuscular mycorrhizal fungi: a review. Sci. Hortic. 187, 131–141. doi: 10.1016/j.scienta.2015.03.002
Becklin, K. M., Mullinix, G. W. R., and Ward, J. K. (2016). Host plant physiology and mycorrhizal functioning shift across a glacial through future CO2 gradient. Plant Physiol. 172, 789–801. doi: 10.1104/pp.16.00837
Blanke, V., Renker, C., Wagner, M., Füllner, K., Held, M., Kuhn, A. J., et al. (2005). Nitrogen supply affects arbuscular mycorrhizal colonization of Artemisia vulgaris in a phosphate-polluted field site. New Phytol. 166, 981–992. doi: 10.1111/j.1469-8137.2005.01374.x
Boggs, J. L., McNulty, S. G., Gavazzi, M. J., and Myers, J. M. (2005). Tree growth, foliar chemistry, and nitrogen cycling across a nitrogen deposition gradient in southern Appalachian deciduous forests. Can. J. For. Res. 35, 1901–1913. doi: 10.1139/x05-128
Borenstein, M., Hedges, L., Higgins, J., and Rothstein, J. (2009). Introduction to Meta-Analysis. John Wiley and Sons.
Cabral, C., Ravnskov, S., Tringovska, I., and Wollenweber, B. (2016). Arbuscular mycorrhizal fungi modify nutrient allocation and composition in wheat (Triticum aestivum L.) subjected to heat-stress. Plant Soil 408, 385–399. doi: 10.1007/s11104-016-2942-x
Chapin, F. S., Bloom, A. J., Field, C. B., and Waring, R. H. (1987). Plant responses to multiple environmental factors. BioScience 37, 49–57. doi: 10.2307/1310177
Cornelissen, J., Aerts, R., Cerabolini, B., Werger, M., and van der Heijden, M. (2001). Carbon cycling traits of plant species are linked with mycorrhizal strategy. Oecologia 129, 611–619. doi: 10.1007/s004420100752
Cotrufo, M. F., Soong, J. L., Horton, A. J., Campbell, E. E., Haddix, M. L., Wall, D. H., et al. (2015). Formation of soil organic matter via biochemical and physical pathways of litter mass loss. Nat. Geosci. 8, 776–779. doi: 10.1038/ngeo2520
Cotton, T. E., Fitter, A. H., Miller, R. M., Dumbrell, A. J., and Helgason, T. (2015). Fungi in the future: interannual variation and effects of atmospheric change on arbuscular mycorrhizal fungal communities. New Phytol. 205, 1598–1607. doi: 10.1111/nph.13224
Couture, J. J., Holeski, L. M., and Lindroth, R. L. (2014). Long-term exposure to elevated CO2 and O3 alters aspen foliar chemistry across developmental stages. Plant Cell Environ. 37, 758–765. doi: 10.1111/pce.12195
Cui, X. C., Hu, J. L., Lin, X. G., Wang, F. Y., Chen, R. R., Wang, J. H., et al. (2013). Arbuscular mycorrhizal fungi alleviate ozone stress on nitrogen nutrition of field wheat. J. Agric. Sci. Technol. 15, 1043–1052.
Curaqueo, G., Schoebitz, M., Borie, F., Caravaca, F., and Roldán, A. (2014). Inoculation with arbuscular mycorrhizal fungi and addition of composted olive-mill waste enhance plant establishment and soil properties in the regeneration of a heavy metal-polluted environment. Environ. Sci. Pollut. Res. 21, 7403–7412. doi: 10.1007/s11356-014-2696-z
Curtis, S. P., and Wang, X. (1998). A meta-analysis of elevated CO2 effects on woody plant mass, form, and physiology. Oecologia 113, 299–313. doi: 10.1007/s004420050381
Deeks, J. J., Macaskill, P., and Irwig, L. (2005). The performance of tests of publication bias and other sample size effects in systematic reviews of diagnostic test accuracy was assessed. J. Clin. Epidemiol. 58, 882–893. doi: 10.1016/j.jclinepi.2005.01.016
De Graaff, M.-A., Van Groenigen, K.-J., Six, J., Hungate, B., and Van Kessel, C. (2006). Interactions between plant growth and soil nutrient cycling under elevated CO2: a meta-analysis. Global Change Biol. 12, 2077–2091. doi: 10.1111/j.1365-2486.2006.01240.x
Del Pozo, A., Pérez, P., Gutiérrez, D., Alonso, A., Morcuende, R., and Martínez-Carrasco, R. (2007). Gas exchange acclimation to elevated CO2 in upper-sunlit and lower-shaded canopy leaves in relation to nitrogen acquisition and partitioning in wheat grown in field chambers. Environ. Exp. Bot. 59, 371–380. doi: 10.1016/j.envexpbot.2006.04.009
Delucia, E. H., Callaway, R. M., Thomas, E. M., and Schlesinger, W. H. (1997). Mechanisms of phosphorus acquisition for ponderosa pine seedlings under high CO2 and temperature. Ann. Bot. 79, 111–120. doi: 10.1006/anbo.1996.0320
Denef, K., Bubenheim, H., Lenhart, K., Vermeulen, J., Van Cleemput, O., Boeckx, P., et al. (2007). Community shifts and carbon translocation within metabolically-active rhizosphere microorganisms in grasslands under elevated CO2. Biogeosciences 4, 769–779. doi: 10.5194/bg-4-769-2007
Drake, B. G., Gonzàlez-Meler, M. A., and Long, S. P. (1997). More efficient plants: a consequence of rising atmospheric CO2? Ann. Rev. Plant Physiol. Plant Mol. Biol. 48, 609–639.
Duval, B. D., Blankinship, J. C., Dijkstra, P., and Hungate, B. A. (2012). CO2 effects on plant nutrient concentration depend on plant functional group and available nitrogen: a meta-analysis. Plant Ecol. 213, 505–521. doi: 10.1007/s11258-011-9998-8
Duval, S., and Tweedie, R. (2000). A Non Parametric Trim and Fill Method of Accounting for Publication Bias in Meta-Analysis. Alexandria, VA: ETATS-UNIS: American Statistical Association.
Egger, M., Davey Smith, G., Schneider, M., and Minder, C. (1997). Bias in meta-analysis detected by a simple, graphical test. BMJ 315, 629–634.
Ekblad, A., Mikusinska, A., Ågren, G. I., Menichetti, L., Wallander, H., Vilgalys, R., et al. (2016). Production and turnover of ectomycorrhizal extramatrical mycelial biomass and necromass under elevated CO2 and nitrogen fertilization. New Phytol. 211, 874–885. doi: 10.1111/nph.13961
Elser, J. J., Fagan, W. F., Kerkhoff, A. J., Swenson, N. G., and Enquist, B. J. (2010). Biological stoichiometry of plant production: metabolism, scaling and ecological response to global change. New Phytol. 186, 593–608. doi: 10.1111/j.1469-8137.2010.03214.x
Ericsson, T. (1995). “Growth and shoot: root ratio of seedlings in relation to nutrient availability,” in Nutrient Uptake and Cycling in Forest Ecosystems: Proceedings of the CEC/IUFRO Symposium Nutrient Uptake and Cycling in Forest Ecosystems Halmstad, Sweden, June, 7–10, 1993, eds L. O. Nilsson, R. F. Hüttl and U. T. Johansson (Dordrecht: Springer Netherlands), 205–214.
Feng, Z., Rütting, T., Pleijel, H., Wallin, G., Reich, P. B., Kammann, C. I., et al. (2015). Constraints to nitrogen acquisition of terrestrial plants under elevated CO2. Global Change Biol. 21, 3152–3168. doi: 10.1111/gcb.12938
Finzi, A. C., Moore, D. J. P., DeLucia, E. H., Lichter, J., Hofmockel, K. S., Jackson, R. B., et al. (2006). Progressive nitrogen limitation of ecosystem processes under elevated CO2 in a warm-temperate forest. Ecology 87, 15–25. doi: 10.1890/04-1748
Franklin, O., Näsholm, T., Högberg, P., and Högberg, M. N. (2014). Forests trapped in nitrogen limitation – an ecological market perspective on ectomycorrhizal symbiosis. New Phytol. 203, 657–666. doi: 10.1111/nph.12840
Garcia, M. O., Ovasapyan, T., Greas, M., and Treseder, K. K. (2008). Mycorrhizal dynamics under elevated CO2 and nitrogen fertilization in a warm temperate forest. Plant Soil 303, 301–310. doi: 10.1007/s11104-007-9509-9
Gehring, C. A., Mueller, R. C., and Whitham, T. G. (2006). Environmental and genetic effects on the formation of ectomycorrhizal and arbuscular mycorrhizal associations in cottonwoods. Oecologia 149, 158–164. doi: 10.1007/s00442-006-0437-9
Gifford, R. M., Barrett, D. J., and Lutze, J. L. (2000). The effects of elevated CO2 on the C:N and C:P mass ratios of plant tissues. Plant Soil 224, 1–14. doi: 10.1023/A:1004790612630
Godbold, D., Vašutová, M., Wilkinson, A., Edwards-Jonášová, M., Bambrick, M., Smith, A., et al. (2015). Elevated atmospheric CO2 affects ectomycorrhizal species abundance and increases sporocarp production under field conditions. Forests 6:1256. doi: 10.3390/f6041256
Goicoechea, N., Baslam, M., Erice, G., and Irigoyen, J. J. (2014). Increased photosynthetic acclimation in alfalfa associated with arbuscular mycorrhizal fungi (AMF) and cultivated in greenhouse under elevated CO2. J. Plant Physiol. 171, 1774–1781. doi: 10.1016/j.jplph.2014.07.027
Grover, M., Maheswari, M., Desai, S., Gopinath, K. A., and Venkateswarlu, B. (2015). Elevated CO2: plant associated microorganisms and carbon sequestration. Appl. Soil Ecol. 95, 73–85. doi: 10.1016/j.apsoil.2015.05.006
Gurevitch, J., and Hedges, L. (2001). “Meta-analysis: combining the results of independent experiments,” in Design and Analysis of Ecological Experiments 2nd edn., eds S. M. Scheiner and J. Gurevitch. (Oxford, UK: Oxford University Press).
Gurevitch, J., and Hedges, L. V. (1999). Statistical issues in ecological meta-analyses. Ecology 80, 1142–1149. doi: 10.1890/0012-9658(1999)080[1142:SIIEMA]2.0.CO;2
Gutknecht, J. L. M., Field, C. B., and Balser, T. C. (2012). Microbial communities and their responses to simulated global change fluctuate greatly over multiple years. Global Change Biol. 18, 2256–2269. doi: 10.1111/j.1365-2486.2012.02686.x
Hedges, L. V., Gurevitch, J., and Curtis, P. S. (1999). The meta-analysis of response ratios in experimental ecology. Ecology 80, 1150–1156. doi: 10.1890/0012-9658(1999)080[1150:TMAORR]2.0.CO;2
Higgins, J. P., and Thompson, S. G. (2002). Quantifying heterogeneity in a meta-analysis. Statist. Med. 21, 1539–1558. doi: 10.1002/sim.1186
Higgins, J. P. T., Thompson, S. G., Deeks, J. J., and Altman, D. G. (2003). Measuring Inconsistency in Meta-Analyses. London: British Medical Association.
Hodge, A., and Fitter, A. H. (2010). Substantial nitrogen acquisition by arbuscular mycorrhizal fungi from organic material has implications for N cycling. Proc. Natl. Acad. Sci. U.S.A. 107, 13754–13759. doi: 10.1073/pnas.1005874107
Huedo-Medina, T. B., Sanchez-Meca, J., Marin-Martinez, F., and Botella, J. (2006). Assessing heterogeneity in meta-analysis: Q statistic or I2 index? Psychol. Methods 11, 193–206. doi: 10.1037/1082-989X.11.2.193
Huque, M. (1988). Experiences with meta-analysis in NDA submissions. Proc. Biopharm. Section Am. Statist. Assoc. 2, 28–33.
Jablonski, L. M., Wang, X., and Curtis, P. S. (2002). Plant reproduction under elevated CO2 conditions: a meta-analysis of reports on 79 crop and wild species. New Phytol. 156, 9–26. doi: 10.1046/j.1469-8137.2002.00494.x
Jakobsen, I., Smith, S. E., Smith, F. A., Watts-Williams, S. J., Clausen, S. S., and Gronlund, M. (2016). Plant growth responses to elevated atmospheric CO2 are increased by phosphorus sufficiency but not by arbuscular mycorrhizas. J. Exp. Bot. 67, 6173–6186. doi: 10.1093/jxb/erw383
Johnson, N. C., Rowland, D. L., Corkidi, L., Egerton-Warburton, L. M., and Allen, E. B. (2003). Nitrogen enrichment alters mycorrhizal allocation at five mesic to semiarid grasslands. Ecology 84, 1895–1908. doi: 10.1890/0012-9658(2003)084[1895:NEAMAA]2.0.CO;2
Klironomos, J. N., Allen, M. F., Rillig, M. C., Piotrowski, J., Makvandi-Nejad, S., Wolfe, B. E., et al. (2005). Abrupt Rise in Atmospheric CO2 Overestimates Community Response in a Model Plant-Soil System. London: Nature Publishing Group.
Klironomos, J. N., Ursic, M., Rillig, M., and Allen, M. F. (1998). Interspecific differences in the response of arbuscular mycorrhizal fungi to Artemisia tridentata grown under elevated atmospheric CO2. New Phytol. 138, 599–605. doi: 10.1046/j.1469-8137.1998.00141.x
Kohler, J., Caravaca, F., del Mar Alguacil, M., and Roldán, A. (2009). Elevated CO2 increases the effect of an arbuscular mycorrhizal fungus and a plant-growth-promoting rhizobacterium on structural stability of a semiarid agricultural soil under drought conditions. Soil Biol. Biochem. 41, 1710–1716. doi: 10.1016/j.soilbio.2009.05.014
Kohler, J., Knapp, B. A., Waldhuber, S., Caravaca, F., Roldán, A., and Insam, H. (2010). Effects of elevated CO2, water stress, and inoculation with Glomus intraradices or Pseudomonas mendocina on lettuce dry matter and rhizosphere microbial and functional diversity under growth chamber conditions. J. Soils Sediments 10, 1585–1597. doi: 10.1007/s11368-010-0259-6
Kostiainen, K., SaranpÄÄ, P, Lundqvist, S.-O., Kubiske, M. E., and Vapaavuori, E. (2014). Wood properties of Populus and Betula in long-term exposure to elevated CO2 and O3. Plant Cell Environ. 37, 1452–1463. doi: 10.1111/pce.12261
Kuehny, J. S., Peet, M. M., Nelson, P. V., and Willits, D. H. (1991). Nutrient dilution by starch in CO2-enriched chrysanthemum. J. Exp. Bot. 42, 711–716. doi: 10.1093/jxb/42.6.711
Langley, J. A., Dijkstra, P., Drake, B. G., and Hungate, B. A. (2003). Ectomycorrhizal colonization, biomass, and production in a regenerating scrub oak forest in response to elevated CO2. Ecosystems 6, 424–430. doi: 10.1007/PL00021509
Langley, J. A., and Megonigal, J. P. (2010). Ecosystem response to elevated CO2 levels limited by nitrogen-induced plant species shift. Nature 466, 96–99. doi: 10.1038/nature09176
Leakey, A. D. B., Ainsworth, E. A., Bernacchi, C. J., Rogers, A., Long, S. P., and Ort, D. R. (2009). Elevated CO2 effects on plant carbon, nitrogen, and water relations: six important lessons from FACE. J. Exp. Bot. 60, 2859–2876. doi: 10.1093/jxb/erp096
Lee, S.-H., Kim, S.-Y., Ding, W., and Kang, H. (2015). Impact of elevated CO2 and N addition on bacteria, fungi, and archaea in a marsh ecosystem with various types of plants. App. Microbiol. Biotechnol. 99, 5295–5305. doi: 10.1007/s00253-015-6385-8
Leifheit, E. F., Veresoglou, S. D., Lehmann, A., Morris, E. K., and Rillig, M. C. (2014). Multiple factors influence the role of arbuscular mycorrhizal fungi in soil aggregation—a meta-analysis. Plant Soil 374, 523–537. doi: 10.1007/s11104-013-1899-2
Liang, J., Qi, X., Souza, L., and Luo, Y. (2016). Processes regulating progressive nitrogen limitation under elevated carbon dioxide: a meta-analysis. Biogeosciences 13, 2689–2699. doi: 10.5194/bg-13-2689-2016
Lipson, D. A., Kuske, C. R., Gallegos-Graves, L. V., and Oechel, W. C. (2014). Elevated atmospheric CO2 stimulates soil fungal diversity through increased fine root production in a semiarid shrubland ecosystem. Global Change Biol. 20, 2555–2565. doi: 10.1111/gcb.12609
Loladze, I., and Elser, J. J. (2011). The origins of the Redfield nitrogen-to-phosphorus ratio are in a homoeostatic protein-to-rRNA ratio. Ecol. Lett. 14, 244–250. doi: 10.1111/j.1461-0248.2010.01577.x
Long, S. P., and Drake, B. G. (1991). Effect of the long-term elevation of CO2 concentration in the field on the quantum yield of photosynthesis of the C3 Sedge, Scirpus olneyi. Plant Physiol. 96, 221–226. doi: 10.1104/pp.96.1.221
Luo, Y., Su, B., Currie, W. S., Dukes, J. S., Finzi, A., Hartwig, U., et al. (2004). Progressive nitrogen limitation of ecosystem responses to rising atmospheric carbon dioxide. BioScience 54, 731–739. doi: 10.1641/0006-3568(2004)054[0731:PNLOER]2.0.CO;2
Makino, A., and Mae, T. (1999). Photosynthesis and plant growth at elevated levels of CO2. Plant Cell Physiol. 40, 999–1006. doi: 10.1093/oxfordjournals.pcp.a029493
McDonald, E. P., Erickson, J. E., and Kruger, E. L. (2002). Can decreased transpiration limit plant nitrogen acquisition in elevated CO2? Funct. Plant Biol. 29, 1115–1120. doi: 10.1071/FP02007
McGrath, J. M., and Lobell, D. B. (2013). Reduction of transpiration and altered nutrient allocation contribute to nutrient decline of crops grown in elevated CO2 concentrations. Plant Cell Environ. 36, 697–705. doi: 10.1111/pce.12007
Mosse, B., and Phillips, J. M. (1971). The influence of phosphate and other nutrients on the development of vesicular-arbuscular mycorrhiza in culture. Microbiology 69, 157–166. doi: 10.1099/00221287-69-2-157
Nehls, U., and Hampp, R. (2000). Carbon allocation in ectomycorrhizas. Physiol. Mol. Plant Pathol. 57, 95–100. doi: 10.1006/pmpp.2000.0285
Orwin, K. H., Kirschbaum, M. U., St John, M. G., and Dickie, I. A. (2011). Organic nutrient uptake by mycorrhizal fungi enhances ecosystem carbon storage: a model-based assessment. Ecol. Lett. 14, 493–502. doi: 10.1111/j.1461-0248.2011.01611.x
Pang, J., Zhu, J.-G., Xie, Z.-B., Liu, G., Zhang, Y.-L., Chen, G.-P., et al. (2006). A new explanation of the N concentration decrease in tissues of rice (Oryza sativa L.) exposed to elevated atmospheric pCO2. Environ. Exp. Bot. 57, 98–105. doi: 10.1016/j.envexpbot.2005.04.004
Peters, J. L., Sutton, A. J., Jones, D. R., Abrams, K. R., and Rushton, L. (2007). Performance of the trim and fill method in the presence of publication bias and between-study heterogeneity. Statist. Med. 26, 4544–4562. doi: 10.1002/sim.2889
Phillips, R. P., Bernhardt, E. S., and Schlesinger, W. H. (2009). Elevated CO2 increases root exudation from loblolly pine (Pinus taeda) seedlings as an N-mediated response. Tree Physiol. 29, 1513–1523. doi: 10.1093/treephys/tpp083
Phillips, R. P., Brzostek, E., and Midgley, M. G. (2013). The mycorrhizal-associated nutrient economy: a new framework for predicting carbon–nutrient couplings in temperate forests. New Phytol. 199, 41–51. doi: 10.1111/nph.12221
Poorter, H., and Pérez-Soba, M. (2001). The growth response of plants to elevated CO2 under non-optimal environmental conditions. Oecologia 129, 1–20. doi: 10.1007/s004420100736
Porcel, R., Aroca, R., Azcon, R., and Ruiz-Lozano, J. M. (2016). Regulation of cation transporter genes by the arbuscular mycorrhizal symbiosis in rice plants subjected to salinity suggests improved salt tolerance due to reduced Na+ root-to-shoot distribution. Mycorrhiza 26, 673–684. doi: 10.1007/s00572-016-0704-5
Prasad, P. V. V., Staggenborg, S. A., and Ristic, Z. (2008). “Impacts of drought and/or heat stress on physiological, developmental, growth, and yield processes of crop plants,” in Response of Crops to Limited Water: Understanding and Modeling Water Stress Effects on Plant Growth Processes, eds L. R. Ahuja, V. R. Reddy, S. A. Saseendran, and Q. Yu. (Madison, WI: American Society of Agronomy, Crop Science Society of America, Soil Science Society of America), 301–355.
Quinn Thomas, R., Canham, C. D., Weathers, K. C., and Goodale, C. L. (2009). Increased tree carbon storage in response to nitrogen deposition in the US. Nat. Geosci. 3, 13–17. doi: 10.1038/ngeo721
Read, D. J., and Perez-Moreno, J. (2003). Mycorrhizas and nutrient cycling in ecosystems – a journey towards relevance? New Phytol. 157, 475–492. doi: 10.1046/j.1469-8137.2003.00704.x
Reed, S. C., Yang, X., and Thornton, P. E. (2015). Incorporating phosphorus cycling into global modeling efforts: a worthwhile, tractable endeavor. New Phytol. 208, 324–329. doi: 10.1111/nph.13521
Reich, P. B. (2009). Elevated CO2 reduces losses of plant diversity caused by nitrogen deposition. Science 326, 1399–1402. doi: 10.1126/science.1178820
Rillig, M. C., Aguilar-Trigueros, C. A., Bergmann, J., Verbruggen, E., Veresoglou, S. D., and Lehmann, A. (2015). Plant root and mycorrhizal fungal traits for understanding soil aggregation. New Phytol. 205, 1385–1388. doi: 10.1111/nph.13045
Rillig, M. C., Field, C. B., and Allen, M. F. (1999). Fungal root colonization responses in natural grasslands after long-term exposure to elevated atmospheric CO2. Global Change Biol. 5, 577–585. doi: 10.1046/j.1365-2486.1999.00251.x
Rogers, H. H., Prior, S. A., Runion, G. B., and Mitchell, R. J. (1995). Root to shoot ratio of crops as influenced by CO2. Plant Soil 187, 229–248. doi: 10.1007/BF00017090
Rosenberg, M., Adams, D., and Gurevitch, J. (2000). MetaWin: Statistical Software for Meta-Analysis. Sunderland, MA: Sinauer Associates.
Simonin, M., Nunan, N., Bloor, J. M. G., Pouteau, V., and Niboyet, A. (2017). Short-term responses and resistance of soil microbial community structure to elevated CO2 and N addition in grassland mesocosms. FEMS Microbiol. Lett. 1:364. doi: 10.1093/femsle/fnx077
Smith, S. E., and Smith, F. A. (2012). Fresh perspectives on the roles of arbuscular mycorrhizal fungi in plant nutrition and growth. Mycologia 104, 1–13. doi: 10.3852/11-229
Soudzilovskaia, N. A., van der Heijden, M. G., Cornelissen, J. H., Makarov, M. I., Onipchenko, V. G., Maslov, M. N., et al. (2015). Quantitative assessment of the differential impacts of arbuscular and ectomycorrhiza on soil carbon cycling. New Phytol. 208, 280–293. doi: 10.1111/nph.13447
Staddon, P. L., Jakobsen, I., and Blum, H. (2004). Nitrogen input mediates the effect of free-air CO2 enrichment on mycorrhizal fungal abundance. Global Change Biol. 10, 1678–1688. doi: 10.1111/j.1365-2486.2004.00853.x
Sterne, J. A., Gavaghan, D., and Egger, M. (2000). Publication and related bias in meta-analysis: power of statistical tests and prevalence in the literature. J. Clin. Epidemiol. 53, 1119–1129. doi: 10.1016/S0895-4356(00)00242-0
Sterner, R. W., Elser, J. J., and Vitousek, P. (2002). Ecological Stoichiometry: The Biology of Elements from Molecules to the Biosphere. Princeton, NJ: Princeton University Press.
Sulman, B. N., Phillips, R. P., Oishi, A. C., Shevliakova, E., and Pacala, S. W. (2014). Microbe-driven turnover offsets mineral-mediated storage of soil carbon under elevated CO2. Nat. Clim. Change 4, 1099–1022. doi: 10.1038/nclimate2436
Tang, J., Chen, J., and Chen, X. (2006). Response of 12 weedy species to elevated CO2 in low-phosphorus-availability soil. Ecol. Res. 21, 664–670. doi: 10.1007/s11284-006-0161-2
Terrer, C., Vicca, S., Hungate, B. A., Phillips, R. P., and Prentice, I. C. (2016). Mycorrhizal association as a primary control of the CO2 fertilization effect. Science 353, 72–74. doi: 10.1126/science.aaf4610
Treseder, K. K. (2004). A meta-analysis of mycorrhizal responses to nitrogen, phosphorus, and atmospheric CO2 in field studies. New Phytol. 164, 347–355. doi: 10.1111/j.1469-8137.2004.01159.x
Treseder, K. K., and Allen, M. F. (2000). Mycorrhizal fungi have a potential role in soil carbon storage under elevated CO2 and nitrogen deposition. New Phytol. 147, 189–200. doi: 10.1046/j.1469-8137.2000.00690.x
Vargas, R., Baldocchi, D. D., Querejeta, J. I., Curtis, P. S., Hasselquist, N. J., Janssens, I. A., et al. (2010). Ecosystem CO2 fluxes of arbuscular and ectomycorrhizal dominated vegetation types are differentially influenced by precipitation and temperature. New Phytol. 185, 226–236. doi: 10.1111/j.1469-8137.2009.03040.x
Veresoglou, S. D., Menexes, G., and Rillig, M. C. (2012). Do arbuscular mycorrhizal fungi affect the allometric partition of host plant biomass to shoots and roots? A meta-analysis of studies from 1990 to 2010. Mycorrhiza 22, 227–235. doi: 10.1007/s00572-011-0398-7
Walker, R. F., Johnson, D. W., Geisinger, D. R., and Ball, J. T. (1998). Growth and ectomycorrhizal colonization of ponderosa pine seedlings supplied different levels of atmospheric CO2 and soil N and P. For. Ecol. Manag. 109, 9–20. doi: 10.1016/S0378-1127(98)00262-X
Wang, X., Qu, L., Mao, Q., Watanabe, M., Hoshika, Y., Koyama, A., et al. (2015). Ectomycorrhizal colonization and growth of the hybrid larch F1 under elevated CO2 and O3. Environ. Pollut. 197, 116–126. doi: 10.1016/j.envpol.2014.11.031
Wang, Y.-P., and Houlton, B. Z. (2009). Nitrogen constraints on terrestrial carbon uptake: implications for the global carbon-climate feedback. Geophys. Res. Lett. 36:L24403. doi: 10.1029/2009GL041009
Wang, Y. P., Law, R. M., and Pak, B. (2010). A global model of carbon, nitrogen and phosphorus cycles for the terrestrial biosphere. Biogeosciences 7, 2261–2282. doi: 10.5194/bg-7-2261-2010
Wilson, D. R., Lima, M. T., and Durham, S. R. (2005). Sublingual Immunotherapy For Allergic Rhinitis : Systematic Review and Meta-Analysis. Oxford: Blackwell.
Yang, Y., Song, Y., Scheller, H. V., Ghosh, A., Ban, Y., Chen, H., et al. (2015). Community structure of arbuscular mycorrhizal fungi associated with Robinia pseudoacacia in uncontaminated and heavy metal contaminated soils. Soil Biol. Biochem. 86, 146–158. doi: 10.1016/j.soilbio.2015.03.018
Zaehle, S., Medlyn, B. E., Kauwe, M. G. D., Walker, A. P., Dietze, M. C., Hickler, T., et al. (2014). Evaluation of 11 terrestrial carbon–nitrogen cycle models against observations from two temperate free-air CO2 enrichment studies. New Phytol. 202, 803–822. doi: 10.1111/nph.12697
Zhang, Q., Wang, Y. P., Matear, R. J., Pitman, A. J., and Dai, Y. J. (2014). Nitrogen and phosphorous limitations significantly reduce future allowable CO2 emissions. Geophys. Res. Lett. 41, 632–637. doi: 10.1002/2013GL058352
Keywords: mycorrhizal fungi-plant symbiont, eCO2 fertilization effect, experimental duration, fertilization, global climate change
Citation: Dong Y, Wang Z, Sun H, Yang W and Xu H (2018) The Response Patterns of Arbuscular Mycorrhizal and Ectomycorrhizal Symbionts Under Elevated CO2: A Meta-Analysis. Front. Microbiol. 9:1248. doi: 10.3389/fmicb.2018.01248
Received: 13 December 2017; Accepted: 23 May 2018;
Published: 11 June 2018.
Edited by:
David Berry, Universität Wien, AustriaReviewed by:
Frédérique Reverchon, Instituto de Ecología (INECOL), MexicoGerald Moser, Justus Liebig Universität Gießen, Germany
Copyright © 2018 Dong, Wang, Sun, Yang and Xu. This is an open-access article distributed under the terms of the Creative Commons Attribution License (CC BY). The use, distribution or reproduction in other forums is permitted, provided the original author(s) and the copyright owner are credited and that the original publication in this journal is cited, in accordance with accepted academic practice. No use, distribution or reproduction is permitted which does not comply with these terms.
*Correspondence: Hui Xu, eHVodWlAaWFlLmFjLmNu