- 1Department of Municipal Engineering, School of Civil Engineering, Southeast University, Nanjing, China
- 2Department of Civil and Environmental Engineering, Carnegie Mellon University, Pittsburgh, PA, United States
Microbial reductive dechlorination of polychlorinated biphenyls (PCBs) has been observed in many PCB-impacted sediments. However, this biodegradation is relatively site-specific and can be affected by PCB compositions and sediment geochemical conditions. To better understand the influence of a common competing electron acceptor, ferric oxyhydroxide (FeOOH), on dechlorination, two sediments (Hudson River and Grasse River sediments), and two PCB mixtures (PCB 5/12, 64/71, 105/114, and 149/153/170 in Mixture 1 and PCB 5/12, 64/71, 82/97/99, 144/170 in Mixture 2) were used for this microcosm study. The addition of 40 mmole/kg FeOOH completely inhibited PCB dechlorination in the Hudson sediment, but only moderately inhibited PCB dechlorination in the Grasse sediment with a 3-week longer lag time. The inhibitory effect in the Grasse sediment was mainly due to the loss of unflanked para dechlorination activity. Fe(II) analysis showed that dechlorination started prior to the consumption of Fe(III), which indicates PCB reduction and Fe(III) reduction were able to take place concurrently. Dehalococcoides 16S rRNA genes increased with the commencement of dechlorination in the Grasse sediment, but not in the completely inhibited Hudson sediment. Rare ortho dechlorination pathways were identified in FeOOH-amended Grasse sediment microcosms, dominated by transformations of PCB 25(24-3-CB) to PCB 13(3-4-CB) and PCB 28(24-4-CB) to PCB 15(4-4-CB). The addition of carbon sources (acetate or a fatty acid mixture with acetate, propionate, and butyrate) after 27 weeks of incubation reinitiated dechlorination in FeOOH-amended Hudson sediment microcosms. Also, the addition of carbon sources greatly enhanced ortho dechlorination in FeOOH-amended Grasse microcosms, indicating the utilization of acetate and/or the fatty acid mixture for ortho dechlorination-related microorganisms. A dechlorination pathway analysis approach revealed that para-flanked meta dechlorination was primarily preferred followed by ortho-/double-flanked meta dechlorination and single-/double-flanked para dechlorination in the Grasse sediment.
Introduction
Polychlorinated biphenyls (PCBs), containing 209 individual congeners, are listed as persistent organic pollutants (POPs) by the Stockholm Convention (UNEP, 2001). They have 1–10 chlorine atoms substituted at 4 ortho, 4 meta, and 2 para sites on the biphenyl structure. Although the industrial production of PCBs was banned in late 1970s, the global occurrence of PCBs in the environment has raised concerns about potential negative effects on aquatic systems and human health (Brown et al., 1987; Safe, 1989; Quensen et al., 1998; IARC Monographs, 2016; Lyall et al., 2017). Hudson and Grasse Rivers are two historically PCB-impacted rivers in the United States. They received discharges from the capacitor manufacturing plants of the General Electric Company (GE) and an aluminum smelting and fabricating facility of the Aluminum Company of America (ˆAlcoa), respectively (EPA, 1997). Because PCBs are highly hydrophobic and persistent, they are predominantly adsorbed in sediment upon release into the aquatic environment and then enter the food chain via ingestion by benthic organisms (Kjellerup et al., 2008). As sediments are the sinks for PCBs in the environment, the remediation of PCB-contaminated sediments has been a regulatory priority for over three decades (EPA, 1997; Sowers and May, 2013).
Among the remediation methods, microbially mediated anaerobic reductive dechlorination of PCBs is particularly attractive due to its low cost and minimal disruption of the sediment (Bedard and Quensen, 1995; Sowers and May, 2013). Despite the prevalence of PCB dechlorination reported in a variety of natural sediment systems and laboratory microcosms, lack of knowledge of the interactions among sediment geochemical properties and dechlorinating microbial communities has hindered the progress of in situ treatment (Brown et al., 1987; Alder et al., 1993; Wiegel and Wu, 2000; Kjellerup et al., 2008; Xu et al., 2012; Sowers and May, 2013; Praveckova et al., 2016). In general, different sediments proceed to dechlorination following eight classified processes (Bedard and Quensen, 1995; Bedard et al., 2005; Hughes et al., 2010). However, the link among sediment properties, processes and dechlorinating microorganisms is not well understood. To date, only a few PCB dechlorinators within the Chloroflexi phylum have been identified. Bacterium ortho-17 (o-17), isolated from Baltimore Harbor sediment, is the only strain that is able to remove ortho chlorines (Cutter et al., 1998, 2001). Dehalobium chlorocoercia strain DF-1 growing with a Desulfovibrio sp. as co-species catalyzes PCBs with double-flanked chlorines (Wu et al., 2002), Dehalococcoides mccartyi strains 195, CBDB1, CG-1, CG-4, CG-5, and JNA were all found to preferentially target flanked (double-flanked or single-/double-flanked) meta/para chlorines (Fennell et al., 2004; Adrian et al., 2009; Wang and He, 2013; Laroe et al., 2014; Wang et al., 2014; Zhen et al., 2014). None of the identified PCB dechlorinators shows a dechlorination preference on unflanked chlorines. Additionally, among all these PCB dechlorinators, only D. mccartyi strains CBDB1 and JNA were found to match known dechlorination processes H and N, respectively (Adrian et al., 2009; Laroe et al., 2014). Therefore, the observed preferences (patterns) in most PCB dechlorination studies have not been attributed to the identified dechlorinators. Moreover, sediment geochemical conditions have been found to exhibit a significant impact on dechlorination preferences (Cho and Oh, 2005; Yan et al., 2006; Kjellerup et al., 2008). Among the geochemical properties, iron is crucial for sediment biogeochemistry. Fe (III) oxides can serve as alternative electron acceptors and inhibit reductive dechlorination (Morris et al., 1992; Paul et al., 2013), while surface-complexed Fe(II) can induce abiotic dechlorination (Jeong et al., 2011; Chen et al., 2016). Abiotic PCB dechlorination associated with biogenic absorbed Fe(II) was expected in PCB-spiked acetate-amended sediment reactors applied with low-voltage electric fields (Liu et al., 2017). Up to now, little is known about the impact of Fe(III) on PCB dechlorination extent and dechlorination pathway preferences in sediments. Organic carbon is also of great importance in PCB dechlorination. A previous review concluded that the impacts of exogenous organic carbon sources, such as acetate, formate, pyruvate, lactate, glucose, methanol, acetone, and a fatty acid mixture (acetate, propionate, and butyrate) on PCB dechlorination rate, extent, and process were equivocal (Wiegel and Wu, 2000). The addition of carbon sources can benefit PCB dechlorination by providing sufficient carbon and energy sources for PCB dechlorinating microorganisms, or by stimulating the growth of essential co-species of PCB dechlorinators (Wiegel and Wu, 2000). Yet, supplementary carbon can also result in the rapid growth of non-PCB dechlorinating microorganisms, which may ultimately inhibit PCB dechlorinators through competition (Wiegel and Wu, 2000; Zanaroli et al., 2012a). General conclusions are difficult to draw due to the variety of experimental conditions considered. In some cases, researchers add the carbon sources at the initiation of incubation (Pulliam Holoman et al., 1998; Fagervold et al., 2011; Ho and Liu, 2012; Kaya et al., 2017), while others add carbon periodically during incubation (Alder et al., 1993; Zhen et al., 2014; Matturro et al., 2016). No study has considered long-term incubation of PCB spiked sediment microcosms prior to carbon addition. Accordingly, the role of exogenous carbon sources when natural carbon may have been depleted (over long times) has not been evaluated. Also, carbon sources are expected to compensate the “electron donor demand” exhibited by amended Fe(III) (Wei and Finneran, 2011). In our previous study, by developing a data analysis approach focusing on chlorine neighboring conditions, we interpreted different PCB dechlorination extent and preferences in relatively low organic carbon content (1.26%) and low Fe (5310 mg/kg dry wt.) Hudson sediment compared with high organic carbon content (5.73%) and high Fe (18,000 mg/kg dry wt.) Grasse sediment. We reported on rare ortho dechlorination in the Grasse sediment (Xu et al., 2016). These lead to a hypothesis that Fe and carbon content may be the important factors for PCB dechlorination in these sediments.
In the present work, we investigate the effects of exogenous ferric oxyhydroxide (FeOOH) with a concentration relevant to background total Fe on PCB dechlorination in the two sediment systems, again with spiked PCB mixtures that allow us to focus on dechlorination preferences. The specific objectives were to (1) understand changes in dechlorination extent and preferences as well as changes in the populations of putative dechlorinating microorganisms; (2) validate the chlorine per biphenyl (CPB) data analysis approach focusing on chlorine neighboring conditions; (3) further investigate the occurrence of ortho dechlorination under Fe(III)-reducing conditions. The findings of this study improve understanding of dechlorination inhibition caused by Fe(III). Moreover, this is the first attempt to enhance rare ortho dechlorination using simple carbon source amendments in sediment microcosms.
Materials and Methods
Sediment Collection, Storage, and Characterization
Surficial sediments (the top 4 inches of sediments) were collected using a petite ponar dredge sampler from the Hudson River (N: 43°14′55.5506″; W: -073°35′37.4080″, Moreau, NY, United States) in October 2008, and the Grasse River (N:44°57′35.9577″; W: -074°48′59.8695″, Massena, NY, United States) in June 2009 as described elsewhere. The sediments were kept in filled 3-gallon plastic buckets in the dark at 4°C until used. The geochemical properties, including background PCBs, metals (e.g., Fe, Al), acid volatile sulfide, total phosphorous, total residue as percent solids, inorganic anions, and organic carbon content of the two sediments were determined previously (Xu et al., 2016).
Experimental Set-up
Thirteen PCB congeners were used in this study based on criteria previously described (Karcher et al., 2004; Xu et al., 2016). These 13 individual PCB congeners, related to 10 tracker pairs, were classified into two mixtures. PCB Mixture 1 was composed of congeners PCB 5(23-CB)/12(34-CB), 64(236-4-CB)/71(26-34), 105(234-34-CB)/114(2345-4-CB), and 149(236-245-CB)/153(245-245-CB)/170(2345-234-CB). PCB Mixture 2 contained congeners PCB 5/12, 64/71, 82(234-23-CB)/97(245-23-CB)/99(245-24-CB), 144(2346-25-CB)/170. The structures of selected PCB congeners, their theoretical first step dechlorination pathways and the possibly associated dechlorination processes were discussed previously (Xu et al., 2016).
The PCB-spiked dry sediment substrates containing 500 mg/kg of each PCB mixture were prepared as described previously (Xu et al., 2016). Modified reducing anaerobic media (RAMM) using 2 mM L-cysteine⋅HCl as the reducing agent was prepared and 1% (vol/vol) of Wolfe’s vitamin solution was added (Shelton and Tiedje, 1984; Yan et al., 2006). The medium was adjusted to pH 7.0 and autoclaved for 20 min at 121°C under a nitrogen atmosphere. FeOOH stock solution (1.0 M) was synthesized following the method developed by Lovley and Phillips (1986).
Microcosms were established in an anaerobic glove box with a gas atmosphere of N2-H2 (99.1:0.9). Unless stated otherwise, 3 g PCB-spiked dry sediment substrate or dry sediment, 3 g fresh sediment inocula (dry weight basis), with or without 1.2 mmole FeOOH, and freshly prepared RAMM medium were added to a 50 ml serum bottle to achieve a total weight of 30 g. Four Fe(III)-amended PCB spiked experiments, four PCB-spiked experiments, two no PCB control experiments and sterile controls were set up (Table 1). Generally, a total PCB concentration of 50 mg/kg slurry was achieved in each PCB-spiked microcosm and an additional 40 mmole/kg slurry of FeOOH was added in each Fe(III)-amended PCB-spiked microcosm. Individual PCB concentrations of PCB Mixture 1 and PCB Mixture 2 in the sediment microcosms are tabulated in Supplementary Table S1. PCB Mixture 1 had a CPB value of 4.36, with ortho CPB 1.56, meta CPB 1.58, and para CPB 1.23, while PCB Mixture 2 had a CPB value of 4.27 with ortho CPB 1.64, meta CPB 1.48, and para CPB 1.16. The established microcosms were sealed with Teflon-lined gray butyl rubber septa (The West Pharmaceutical Co., PA) and crimped with aluminum crimp caps. After a 24-h rotation on a rotating mixer (40 rpm) in the dark, the first time point sampling was conducted and the rest of microcosms were stored statically at ambient temperature in the dark. Destructive sampling was conducted at 3-week intervals from Week 0 to Week 24, then at Week 30, Week 36, and Week 51 anaerobically. Unless stated otherwise, triplicate microcosms were sampled at each sampling point. The influences of carbon sources were investigated by adding acetate (pH = 7.0, sodium salt) or a fatty acid mixture (acetate/propionate/butyrate 1:1:1, pH = 7.0, sodium salts) after 27 weeks of incubation. The microcosms used for carbon source amendment were those that had been sampled and recapped at Week 21 and Week 24. At Week 27, 7.5 mM of acetate or a fatty acid mixture were added; at Week 30 and Week 36, 15 mM of acetate or a fatty acid mixture were added, respectively. To minimize the change of slurry volume, all the additions were conducted by injecting 200 times concentrated stock carbon source solutions. The sample collections for the triplicate acetate-amended microcosms and the triplicate fatty acid mixture-amended microcosm were non-destructive and performed at Week 30 and Week 36, right before the addition of extra carbon sources. The final sampling was conducted at Week 51. Sterile controls were sampled in duplicate at Week 0, Week 18, Week 36, and Week 51, and no dechlorination was observed.
Headspace Analysis
Prior to microcosm slurry withdrawal, 200 μl of headspace gas was analyzed to determine H2, CH4, and trace O2 following an analytical method mentioned elsewhere (Xu et al., 2016). Considering biological gas production, the amount of each gas species was recalculated with the ideal gas law by simply assuming a constant 1.0 atm partial pressure of nitrogen in the headspace and a room temperature of 20°C.
Ferrous Iron Analysis
The reduction of Fe(III) to Fe(II) in the microcosms was tracked using a ferrozine method described elsewhere (Sorensen, 1982). Briefly, a subsample of 200 μl of sediment slurry was weighed and transferred into a 1.5-ml microcentrifuge tube containing 800 μl of 1.0 M HCl and reacted thoroughly. Thereafter, 10 μl of acid treated sample was added to 4.99 ml ferrozine solution and filtered. Dilutions with more ferrozine solution were performed when necessary. The absorption was measured at 562 nm by a UV-vis spectrophotometer (HACH, Macon, MO, United States).
PCB Extraction, Cleanup, and Analysis
The PCB extraction procedure was described elsewhere (Yan et al., 2006; Xu et al., 2016). Briefly, 2.0 g sediment slurry was collected anaerobically, and 1 μg of PCB 209 in 20 μl of hexane was added as surrogate. After the hexane fully evaporated, the slurry sample was extracted with 10 ml of acetone, followed by 10 ml of 1:1 acetone/hexane (v/v) twice, and 3 ml of hexane, respectively. The pooled extract was phase-separated with 8 ml of organic-free 2% NaCl solution. The obtained hexane layer was collected and concentrated to 2 ml. Tetrabutylammonium (TBA) sulfite was used to remove sulfur, following EPA Method 3660B. The TBA pretreated PCB extract was passed through a glass column (10 mm inner diameter) packed with 4 g Florisil and topped with 1.5 g anhydrous sodium sulfate. The column was pre-washed by 30 ml hexane. The eluate was loaded onto the column, followed by eluting with 30 ml n-hexane and 25 ml n-hexane/CH2Cl2 (4:1, v/v). The combined elutant was concentrated to exact 4.0 ml. The quantification of individual PCBs were performed by Hewlett Packard gas chromatograph (Model 6890) coupled with a micro-electron capture detector (μECD) and a 30-m DB-XLB capillary column (0.18 mm diameter and 0.18 μm film thickness; Agilent Technologies, Palo Alto, CA) (Xu et al., 2012, 2016). Complete dechlorination was confirmed by analyzing biphenyl in selected microcosms (duplicate bottles in each treatment at Week 36) at Energy & Environmental Research Center (EERC), University of North Dakota.
DNA Extraction and Quantitative PCR
The sediment pellet samples from 0.5 ml of slurries were harvested as described previously (Xu et al., 2016). Total genomic DNA was extracted from the sediment pellets using the MoBio PowerSoil DNA Isolation Kit (MoBio, Carlsbad, CA) following the manufacturer’s protocols. Equal volumes of the triplicate DNA extracts at each sampling point were combined and mixed thoroughly for further microbial community analysis.
The 16S rRNA gene copies of total Bacteria, Fe(III)-reducing Family Geobacteraceae, Dehalococcoides, PCB degrading organism strains ortho-17 and D. chlorocoercia strain DF-1 (o-17/DF-1) were estimated using SYBR Green-based quantitative PCR (qPCR). Total Bacteria, Geobacteraceae, Dehalococcoides, and o-17/DF-1 were targeted with primer sets BAC338F/534R (Muyzer et al., 1993), Geo564F/840R(Cummings et al., 2003; Himmelheber et al., 2009), DHC1200F/1271R (He et al., 2003), and modified BAC908F/Dehal1265R (Edwards et al., 1989; Watts et al., 2005; Xu et al., 2012), respectively. qPCR reactions were performed in an ABI 7500 Real-Time PCR System (Applied Biosystems, Carlsbad, CA, United States) as described previously (Xu et al., 2016).
Identification of Dechlorination Preferences
The dechlorination preferences were identified using a modified CPB analysis approach (Xu et al., 2016). Based on the known ortho, meta, and para chlorine positions, the neighboring conditions of each chlorine are considered. Totally, nine chlorine categories are defined, including unflanked ortho (UF ortho), flanked ortho (so called meta-flanked ortho), unflanked meta (UF meta), ortho-flanked meta (OF meta), para-flanked meta (PF meta), double-flanked meta (DF meta) (neighbored with one ortho and one meta chlorine), unflanked para (UF para), single-flanked para (SF para) (so-called meta flanked para), and double-flanked para (DF para).
Results
Headspace Gas
No O2 was detected in any of the microcosms. In the Hudson sediment microcosms amended with FeOOH (H-1-Fe and H-2-Fe), only a trace amount of CH4 (below 0.1% of the headspace gas) was detected in the time course of 51 weeks, indicating a complete inhibition of methanogenesis in these sediment microcosms. In the Grasse sediment microcosms, the addition of FeOOH significantly reduced but did not completely cease the generation of CH4 (see Supplementary Figure S1). The trends of CH4 production in different microcosm groups were generally G-2 > G-1 > G > G-2-Fe > G-1-Fe. The measured CH4 concentrations in FeOOH-amended Grasse microcosms were 30–40% lower than those in microcosms without added FeOOH. This suggests that FeOOH can moderately inhibit methanogenesis in the Grasse sediment. H2 was not detected in any Grasse sediment microcosms during the study period, while in the Hudson sediment microcosms, trace H2 (less than 0.2%) was only found at Week 0.
Fe(III) Reduction
The reduction of Fe(III) was directly confirmed by tracking the concentrations of Fe(II) in the slurries over time. Due to the presence of indigenous Fe, Fe(II) concentrations in FeOOH-amended (H-1-Fe, H-2-Fe, G-1-Fe, and G-2-Fe), non-FeOOH-amended (H-1, H-2, G-2, and G-2), and no PCB control (H, G) microcosms were all measured to better elucidate the influences of supplementary FeOOH and/or PCBs on Fe(III) reduction. The trends of increasing Fe(II) were distinct in the Hudson and the Grasse sediments, but very similar in the same sediment spiked with different PCB Mixtures (see Supplementary Figure S2). In the Grasse sediment microcosms, after approximately 15–18 weeks of incubation, the differences of Fe(II) levels between G-1-Fe (91.3 ± 4.0 mmole/kg slurry at Week 15) and G-1 (51.4 ± 1.2 mmole/kg slurry at Week 15) or between G-2-Fe (105.4 ± 5.1 mmole/kg slurry at Week 18) and G-2 (61.3 ± 1.2 mmole/kg slurry at Week 18) remained at around 40 mmole/kg slurry until the end of 51 weeks. This indicates the complete reduction of supplementary FeOOH was accomplished within a 15–18 weeks’ incubation. Meanwhile, dissolved Fe(II) remained at 1.2–1.5 mmole/kg slurry, indicating the biogenic Fe(II) was mainly in the adsorbed form. In contrast, the Hudson sediment microcosms exhibited a much slower Fe(III) reduction. By the end of 51 weeks, Fe(II) concentrations in H-1-Fe, H-1, H-2-Fe, and H-2 were 47.3 ± 4.1 mmole/kg, 15.2 ± 0.9 mmole/kg, 47.1 ± 0.7 mmole/kg, and 13.2 ± 1.4 mmole/kg, respectively. The difference in corresponding microcosms in the presence and in the absence of supplementary FeOOH was smaller than 40 mmole/kg, suggesting FeOOH in H-1-Fe and H-2-Fe was not completely reduced to Fe(II) within the time course of 51 weeks. Dissolved Fe(II) ranged from 0.4 to 0.6 mmole/kg after 51 weeks of incubation. Additionally, the spiking of PCBs slightly favored Fe(III) reduction (G-1/G-2 with G, H-1/H-2 with H), especially in the first 21 weeks of incubation (Supplementary Figure S2), suggesting a complex microbial interaction in the presence of added alternative electron acceptors.
PCBs in Sediment Microcosms
No dechlorination products were observed in sterile controls (data not shown) or the FeOOH-amended Hudson sediment microcosms within the 51 weeks’ incubation (Supplementary Tables S2, S3). This suggests that dechlorination was microbially catalyzed and the addition of FeOOH led to the complete inhibition of PCB dechlorination in the Hudson sediment. In addition, the negligible levels of generated CO2 and CH4 in H-1-Fe and H-2-Fe suggest low microbial activity in FeOOH-amended Hudson sediment microcosms. By contrast, supplementary FeOOH only moderately inhibited PCB dechlorination in the Grasse sediment microcosms. Figure 1 depicts the total PCB mass over time for each experiment. Generally, the addition of FeOOH resulted in a longer lag time and lower dechlorination extent in the Grasse sediment microcosms. The lag time periods were as long as 6–9 weeks in G-1-Fe and approximately 6 weeks in G-2-Fe, which were around 3 weeks longer than lag times in G-1 and G-2. In addition, as mentioned earlier, it took 15–18 weeks to completely reduce the supplementary FeOOH in the Grasse sediment microcosms. PCB dechlorination occurred at least 9 weeks prior to the completion of FeOOH reduction, suggesting the concurrent Fe(III) reduction and PCB dechlorination. Comparing to the initially spiked PCBs of 50.0 mg/kg slurry, significantly higher residual PCBs (37.5 ± 0.7 and 36.8 ± 0.4 mg/kg slurry in G-1-Fe and G-2-Fe) were observed in FeOOH-amended sediment microcosms than those in non-FeOOH-amended sediment microcosms (32.6 ± 0.7 and 33.6 ± 0.2 mg/kg slurry in G-1 and G-2) after 51 weeks of incubation. Additionally, the reduction trends of total PCB mass were very similar in the Grasse sediment regardless of the PCB mixture spiked. The partial inhibition was also indicated by the changes of CPB values in the presence of supplementary FeOOH. By the end of 51 weeks, the CPB values for G-1-Fe and G-2-Fe declined by 33.8 and 40.2%, whereas the CPB values for the non-FeOOH-amended G-1 and G-2 declined by 52.2 and 49.9%. Seen in Figure 1, the declining trends of total PCB mass and CPB values in FeOOH-amended and non-FeOOH-amended Grasse sediment microcosms had not reached the plateau phase by the end of 51 weeks. This suggests the potential for further dechlorination in the Grasse sediment microcosms after even 1 year of incubation wasn’t changed by supplementary FeOOH.
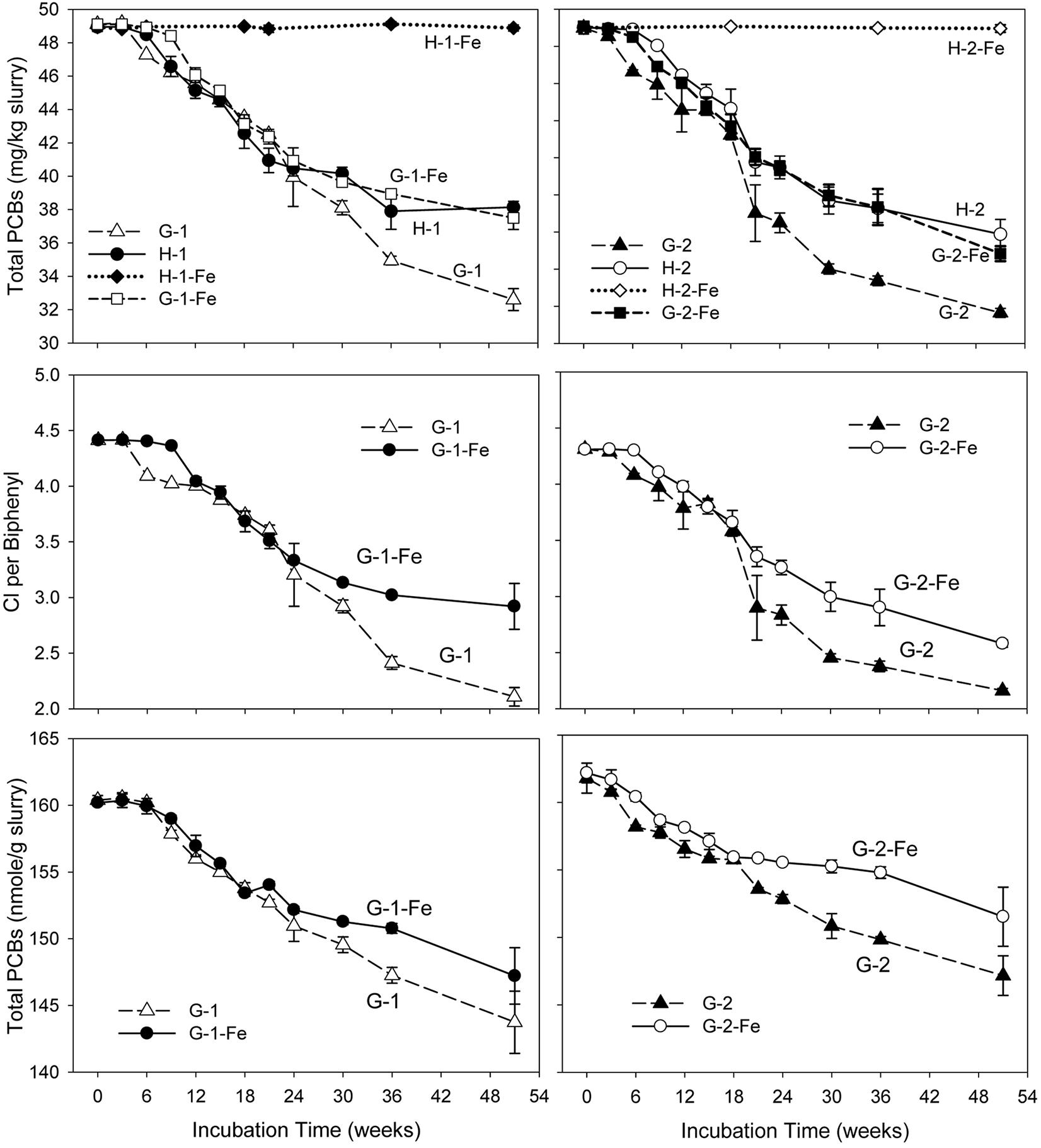
FIGURE 1. Total PCB mass concentrations, CPB values, and molar concentrations over time in sediment microcosms. H-1, Hudson sediment spiked with PCB Mixture 1; H-1-Fe, Hudson sediment spiked with PCB Mixture 1 and FeOOH; G-1, Grasse sediment spiked with PCB Mixture 1; G-1-Fe, Grasse sediment spiked with PCB Mixture 1 and FeOOH; H-2, Hudson sediment spiked PCB Mixture 2; H-2-Fe, Hudson sediment spiked PCB Mixture 2 and FeOOH; G-2, Grasse sediment spiked with PCB Mixture 2; G-2-Fe, Grasse sediment spiked with PCB Mixture 2 and FeOOH. Data plotted are averages of triplicates. Error bars represent standard deviation. Error bars not visible are smaller than the symbol size.
Dechlorination cannot change the number of PCB molecules unless monochlorinated biphenyls are completely dechlorinated to biphenyl molecules. Excluding any experimental loss of PCBs, the decrease of total PCB moles is attributed to complete dechlorination. Illustrated in Figure 1, the reduction of total PCB molar concentrations in G-1-Fe and G-2-Fe was significant (p < 0.001), but less than that in non-FeOOH-amended G-1 and G-2. This was confirmed by biphenyl analysis (data not shown). Analysis of biphenyl suggested that the source of identified biphenyls was not the residual PAHs in sediments, but the spiked PCBs. The decrease of total PCB moles in G-1-Fe and G-2-Fe was mainly attributed to complete dechlorination of the only non-ortho congener spiked in the microcosms-PCB 12 (34-CB) (see Supplementary Tables S4, S5).
Although FeOOH moderately inhibited overall PCB dechlorination in the Grasse sediment microcosms, the influence of FeOOH on the first step dechlorination of spiked PCB congeners was complex. Seen in Figure 2, by the end of 51 weeks, over 96% of parent PCBs had been transformed in all the Grasse sediment microcosms. The less extensive dechlorination observed in G-1-Fe and G-2-Fe was not due to the lack of capability to remove the first chlorine atom from spiked PCB congeners. The changes of typical congeners PCB 12 and PCB 170 in Grasse sediment microcosms are illustrated in Figure 2, and the changes of other congeners are provided in Supplementary Figures S3–S6. Based on the relative amount of parent PCB congeners under methanogenic (G-1 and G-2) and Fe(III)-reducing (G-1-Fe and G-2-Fe) conditions, the parent PCB congeners were classified into three groups (Table 2). The first step of dechlorination for 10 out of 13 parent congeners in this study were either enhanced or not affected with FeOOH addition. Looking into the identical PCB congeners (PCB 5, 12, 64, and 71) spiked in the two PCB Mixtures, PCB Mixture 2 tended to favor more rapid dechlorination of these congeners, as well as the concentration normalized PCB 170 (concentrations were normalized based on PCB 170 concentrations at Week 0) (Figure 2 and Supplementary Figures S3, S4).
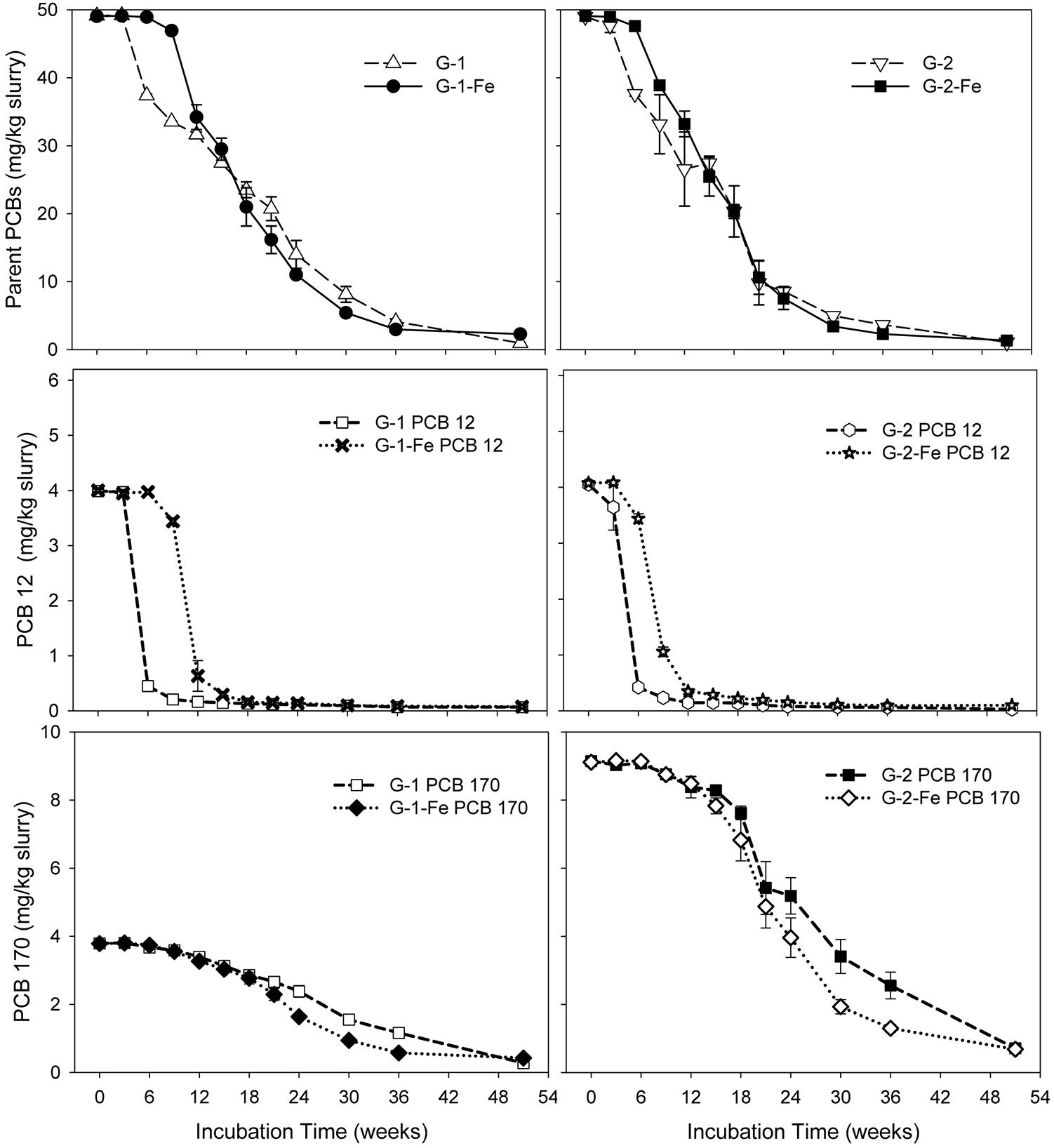
FIGURE 2. Mass concentrations of parent PCBs, PCB 12, and PCB 170 over time in sediment microcosms. Data plotted are averages of triplicates. Error bars represent standard deviation. Error bars not visible are smaller than the symbol size.
Notably, congener specific analysis of FeOOH-amended Grasse sediment microcosms revealed that ortho dechlorination occurred in G-1-Fe (Supplementary Table S4). The two identified pathways were PCB 25(24-3-CB) to PCB 13(3-4-CB) and PCB 28(24-4-CB) to PCB 15(4-4-CB). After 51 weeks of incubation, PCB 13 concentrations were found to be 1.2, 1.9, and 0.7 nmole/g slurry in triplicates, while the concentrations of PCB 15 were 2.3, 1.4, and 0.9 nmole/g slurry in triplicates. The above two ortho dechlorination pathways were also observed in non-FeOOH-amended G-1, suggesting the addition of FeOOH did not inhibit ortho dechlorination activity. Considering the changes of PCB 3(4-CB) in G-1-Fe, the concentrations were 10.8 ± 0.1 (10.8, 10.8, 10.9), 9.0 ± 0.6 (9.5, 8.2, 9.2), and 8.5 ± 4.6 (13.9, 5.4, 6.3) nmole/g at Week 18, Week 36, and Week 51, respectively. In other words, PCB 3 was reduced in two of the triplicates at Week 51, but increased sharply in the third. Moreover, the only spiked non-ortho PCB congener PCB 12(34-CB) was found to be at 0.7 ± 0.0, 0.4 ± 0.0, and 0.3 ± 0.0 nmole/g at Week 18, Week 36, and Week 51, respectively and could not contribute to the apparent increase of PCB 3 observed at Week 51. These results suggest that (1) dechlorination of unflanked para chlorines resumed after a long incubation; (2) the observed leveled PCB 3 in one of the microcosms at Week 51 was not directly linked to the dechlorination of PCB 12. The possible pathways included meta/para dechlorination of PCB 13(3-4-CB) or PCB 15(4-4-CB) and ortho dechlorination of PCB 7(24-CB) and PCB 8(2-4-CB). Regardless of the intermediates, the original parent PCB congeners were the dioxin-like mono-ortho PCB 105(234-34-CB) and PCB 114(2345-4-CB).
Identification of Attacked Chlorines
The changes of ortho CPB (OCPB), meta CPB (MCPB), and para CPB (PCPB) for FeOOH-amended Grasse sediment microcosms are plotted in Figure 3. Illustrated in Figures 3A,B, OCPB values remained high in both G-1-Fe and G-2-Fe. In G-1-Fe, OCPB values slightly increased from 1.57 ± 0.00 to 1.66 ± 0.00 from Week 0 to Week 30. Thereafter, CPB values of 1.66 ± 0.01 and 1.65 ± 0.04 appeared at Week 36 and Week 51, respectively. In G-2-Fe, OCPB values kept increasing from 1.64 ± 0.00 to 1.74 ± 0.02 in the 51 weeks of incubation. The increase of OCPB was caused by the reduction of total molar concentration due to complete dechlorination of non-ortho PCB congener (PCB12, 34-CB) as decreasing the denominator in the OCPB calculation. A stable or reduced number of ortho chlorines was observed at Week 51 in G-1-Fe (Figure 3A), where ortho dechlorination occurred and was comparable with the reduction of total PCB molar concentration, resulting in similar decreases in the numerator and denominator in OCPB. In both G-1-Fe and G-2-Fe, unflanked ortho chlorines increased, whereas flanked ortho chlorines decreased over time. Considering the limited ortho dechlorination in G-1-Fe but not in G-2-Fe, the increase of unflanked ortho chlorines was due to the removal of ortho- or double-flanked meta chlorines, which resulted in fewer flanked chlorines on the ortho positioned chlorines but no overall change in the total number of ortho chlorines. By the end of 51 weeks, the remaining flanked ortho chlorines were as low as 0.07 ± 0.01 and 0.06 ± 0.00 in G-1-Fe and G-2-Fe, respectively, suggesting almost all ortho chlorines were unflanked at the end of the study. These findings were consistent with those observed in G-1 and G-2 (Xu et al., 2016), indicating the effect of FeOOH on the shifts of OCPBs was very limited.
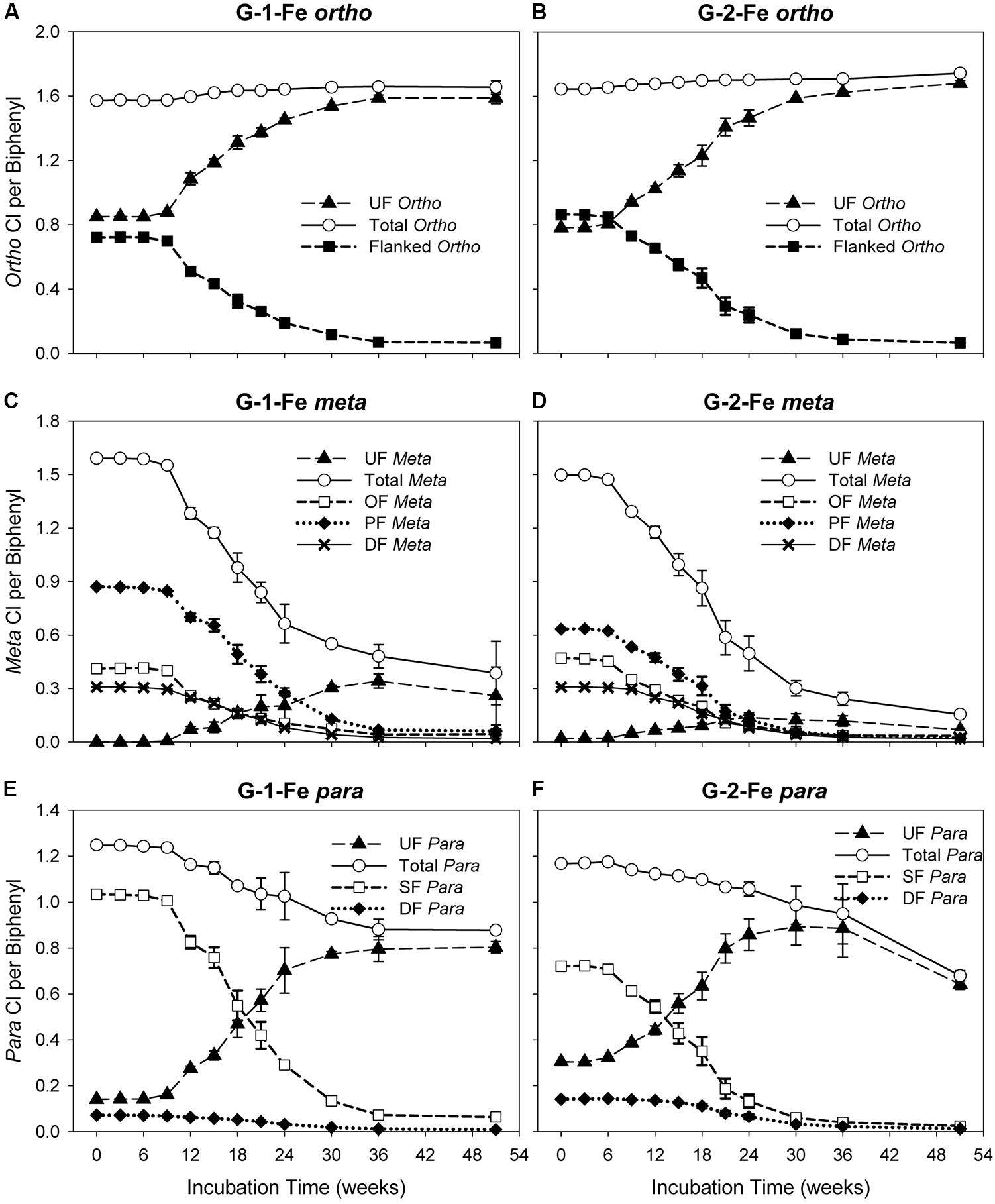
FIGURE 3. Changes of ortho, meta, para chlorines over time in G-1-Fe and G-2-Fe. (A) ortho in G-1-Fe; (B) ortho in G-2-Fe; (C) meta in G-1-Fe; (D) meta in G-2-Fe; (E) para in G-1-Fe; (F) para in G-2-Fe. UF, unflanked; SF, single-flanked; DF, double-flanked; OF, ortho-flanked; PF, para-flanked. All data points averaged triplicate microcosms. Error bars represent standard deviation. Error bars not visible are smaller than the symbol size.
Meta dechlorination was preferred but PCB composition dependent. The initial MCPB values for both mixtures were similar (see in Figures 3C,D). After 51 weeks of incubation, MCPB values declined from 1.59 ± 0.00 to 0.39 ± 0.18 in G-1-Fe and from 1.50 ± 0.00 to 0.16 ± 0.01 in G-2-Fe. Approximately 80–90% of meta chlorines were removed and the declining trends suggest a further meta chlorination potential. Compared with G-1 (0.16 ± 0.05 at Week 51) and G-2 (0.17 ± 0.01 at Week 51), the inhibitory effect of supplementary FeOOH was relatively limited. The meta dechlorination rates in the rapid phase of G-1-Fe (9–24 weeks) and G-2-Fe (6–30 weeks) were 0.056 ± 0.002 Cl/week and 0.051 ± 0.002 Cl/week, respectively, which exceeded the corresponding rapid phase meta dechlorination rates in G-1 (0.043 ± 0.004 Cl/week) and G-2 (0.043 ± 0.002 Cl/week). Seen in Figures 3C,D, unflanked meta chlorines increased in the first 30–36 weeks, then decreased slightly (solid triangle). Due to the lack of ortho removal before 36 weeks, the accumulated unflanked meta chlorines could be attributed to the dechlorination of single-/double-flanked para chlorines, where ortho chlorine was absent for the target phenyl ring. The maximum unflanked meta chlorines per biphenyl for G-1-Fe (0.34 ± 0.04 at Week 36) were much higher than those for G-1 (0.18 ± 0.01 at Week 30), indicating a partially inhibitory effect for the unflanked meta dechlorination. But in G-2-Fe and G-2, the maximum unflanked meta chlorines per biphenyl were similar (0.14 ± 0.03 for G-2-Fe; 0.13 ± 0.01 for G-2). Thereafter, the slightly decreased unflanked meta chlorines were the result of dechlorination of unflanked meta chlorines. Similarly, the declining of ortho-flanked meta chlorines (open square) was caused by removal of meta chlorines. By comparing the reduction rates of ortho-flanked meta chlorines and meta-flanked ortho chlorines in the rapid phase (9–24 weeks in G-1-Fe and 6–24 weeks in G-2-Fe), meta-flanked ortho chlorines (0.035 ± 0.002 Cl/week for G-1-Fe, 0.035 ± 0.001 Cl/week for G-2-Fe) decreased faster than ortho-flanked meta chlorines (0.021 ± 0.003 Cl/week for G-1-Fe; 0.021 ± 0.001 Cl/week for G-2-Fe), suggesting meta dechlorination attacked both ortho-flanked and double-flanked meta chlorines. Moreover, the shifts of the sub meta chlorine groups illustrated that overall meta dechlorination was mainly due to the reduction of para-flanked meta chlorines (solid diamond) rather than ortho-flanked meta or double-flanked meta. Also, this confirmed the meta dechlorination preference when meta chlorines were para-flanked. In general, meta dechlorination was prevalent in FeOOH-amended Grasse sediment microcosms, and para-flanked, ortho-flanked, and double-flanked meta, as well as relatively limited unflanked meta, were all targeted.
Compared to meta chlorination, para dechlorination was less extensive in FeOOH-amended Grasse sediment microcosms; approximately 30–40% of para chlorines were removed (Figures 3C–F). In G-1-Fe, from Week 9 to Week 36, averaged para dechlorination rate was 0.013 ± 0.001 Cl/week, while in G-2-Fe, from Week 6 to Week 36, the rate was 0.007 ± 0.000 Cl/week. From Week 36 to Week 51, PCBP values for G-1-Fe remained at approximately 0.88, whereas the values for G-2-Fe decreased from 0.88 ± 0.13 to 0.68 ± 0.02. Meanwhile, the unflanked para per biphenyl values remained at around 0.80 for G-1-Fe, and declined from 0.80 ± 0.02 to 0.64 ± 0.02 for G-2-Fe. Compared with non-FeOOH-amended G-1 and G-2, 75 and 80% of para chlorines were removed and the remaining unflanked para chlorines per biphenyl were only 0.32 ± 0.04 and 0.18 ± 0.01 after 51 weeks of incubation. These results suggest that (1) the remaining para chlorines were predominantly unflanked after a long time of incubation in G-1-Fe and G-2-Fe; (2) the inhibitory effect of FeOOH on PCB dechlorination was solely due to the loss of para dechlorinating activities targeting unflanked para. The unflanked para dechlorination resumed in G-2-Fe after 36 weeks of incubation but not in G-1-Fe, suggesting that PCB composition affected dechlorination preferences. Seen in Figures 3E,F, the reduction rates of single-flanked para chlorines (open square) (0.047 ± 0.001 Cl/week from Week 9 to Week 24 for G-1-Fe, 0.033 ± 0.001 Cl/week from Week 6 to Week 24 for G-2-Fe) were three to four times faster than the overall para removal rates shown above. Along with the reduction of single-flanked para chlorines, unflanked para (solid triangle) increased rapidly at rates 0.035 ± 0.001 Cl/week, 0.031 ± 0.002 Cl/week in the same time periods for G-1-Fe and G-2-Fe, respectively. This suggests para dechlorination preferred flanked para chlorines, but meta removal was more prevalent for PCBs with a single meta and para chlorine on the same phenyl ring. In addition, double-flanked para chlorines, although not dominant in either mixture decreased over time, where para and meta dechlorination both occurred.
Quantification of Putative PCB Dechlorinating Bacteria and Fe(III)-Reducing Bacteria
The copy numbers of 16S rRNA genes of putative PCB dechlorinating bacteria Dehalococcoides over time are plotted in Figure 4. Initially, Dehalococcoides 16S rRNA genes in the Grasse sediment were one order of magnitude higher than those in the Hudson sediment. In H-1-Fe and H-2-Fe, no increase of Dehalococcoides 16S rRNA genes was observed over the course of incubation and the concentrations remained below 1.5 × 105 copies/ml, which was the minimum Dehalococcoides 16S rRNA gene number found in H-1 and H-2 showing dechlorination activities. This suggests that the complete inhibition of PCB dechlorination in the FeOOH-amended Hudson sediment microcosms was very likely due to the lack of active Dehalococcoides. By contrast, G-1-Fe and G-2-Fe showed clear increasing trends of Dehalococcoides 16S rRNA genes over time. The Dehalococcoides 16S rRNA genes increased from 2.4 × 106± 1.2 × 105 to 9.2 × 107 ± 3.1 × 106 copies/ml in G-1-Fe and from 1.8 × 106 ± 2.5 × 105 to 1.1 × 108 ± 3.1 × 106 copies/ml in G-2-Fe. The supplementary FeOOH led to 5–10 times more Dehalococcoides 16S rRNA genes than observed in G-1 and G-2. The high Dehalococcoides 16S rRNA genes found at Week 51 indicate a potential of continuing dechlorination in G-1-Fe and G-2-Fe. The relative abundance of Dehalococcoides in G-1-Fe and G-2-Fe expressed as the averaged percentage of Dehalococcoides 16S rRNA genes in total Bacteria 16S rRNA genes is shown in Figure 5. The relative percentage of Dehalococcoides 16S rRNA genes increased from 0.2 to 2.5% in G-1-Fe and from 0.2 to 2.6% in G-2-Fe, while in G-1 and G-2, the percentage increased to around 1.0%. The addition of FeOOH enhanced not only the Dehalococcoides 16S rRNA gene numbers but also the relative abundance of Dehalococcoides in the microbial community. The o-17/DF-1 16S rRNA genes were much lower than those of Dehalococcoides and the relative abundance of o-17/DF-1 did not show any increase in G-1-Fe and G-2-Fe (data not shown). Thus, the ortho dechlorination activity observed in the Grasse sediment microcosms was likely associated with other unknown ortho dechlorinating organisms. Geobacteraceae 16S rRNA genes were from 2.5 × 105± 4.8 × 104 to 2.0 × 106 ± 5.3 × 103 copies/ml in H-1-Fe and H-2-Fe, while significantly higher Geobacteraceae 16S rRNA genes (5.5 × 105± 1.3 × 104 to 1.1 × 107 ± 4.3 × 105 copies/ml) were found in control group H (p < 0.01). Additionally, Geobacteraceae 16S rRNA genes in H-1-Fe/H-2-Fe had no significant differences with those in H-1/H-2 (p > 0.05). These indicate that there was no Geobacteraceae enrichment by the addition of FeOOH. The low Geobacteraceae level could explain the weak Fe(III) reduction observed in H-1-Fe and H-2-Fe. Meanwhile, Geobacteraceae 16S rRNA genes detected in the Grasse sediment microcosms remained one to three orders of magnitude higher than those in the Hudson sediment microcosms (data not shown). Notably, the addition of FeOOH did not further stimulate the growth of Geobacteraceae.
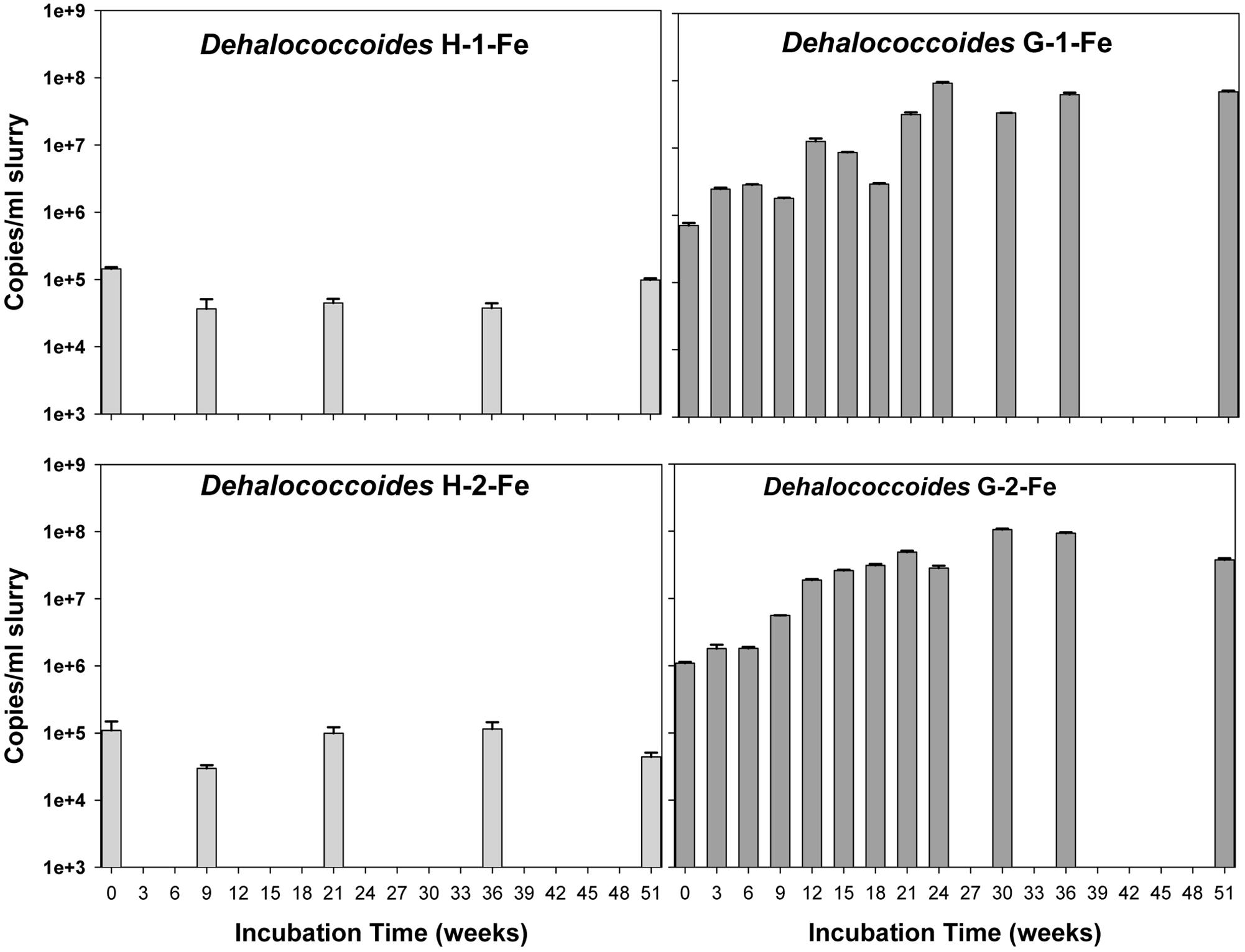
FIGURE 4. Quantitative assessment of Dehalococcoides 16S rRNA genes in H-1-Fe, G-1-Fe, and H-2-Fe, G-2-Fe. All data points averaged duplicate tests. Error bars represent standard deviation.
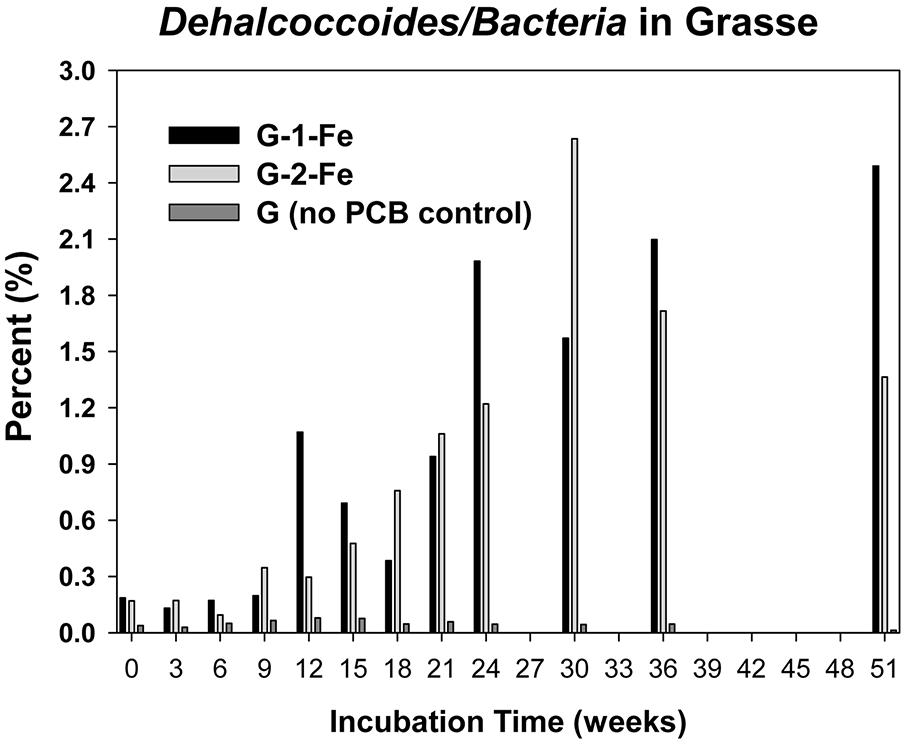
FIGURE 5. Relative abundance of Dechalococcoides 16S rRNA genes in total Bacteria 16S rRNA genes of G-1-Fe, G-2-Fe, and G.
Supplementary Carbon Sources and Ortho Dechlorination Enhancement
In the Hudson sediment microcosms amended with FeOOH, supplementary acetate or the fatty acid mixture led to extensive methanogenesis after the second addition at Week 36 (Supplementary Table S6). Along with the generation of CH4, enhanced reduction of Fe(III) was observed in carbon-amended microcosms at Week 36 and Week 51 for H-1-Fe and at Week 51 only for the fatty acid mixture amended H-2-Fe (Supplementary Figure S7). Notably, PCB dechlorination was observed after the addition of carbon sources. Table 3 shows the mass concentrations of total parent PCB concentrations at Week 36 and Week 51. By the end of 51 weeks, total parent PCBs were reduced by 3–20% on the average of triplicate microcosms with two carbon sources additions (incubating with carbon sources from Week 27 to Week 51). The fatty acid mixture and sole acetate additions both reinitiated PCB dechlorination in H-1-Fe and H-2-Fe. The dominant pathways included PCB 5(23-CB) to PCB 1(2-CB), PCB 12(34-CB) to PCB 2(3-CB) and PCB 3(4-CB), and PCB 64(236-4-CB) to PCB 32(26-4-CB). Flanked meta and flanked para dechlorination took place first. However, 16S rRNA genes of Dehalococcoides and o-17/DF-1 did not increase apparently in H-1-Fe and H-2-Fe when PCB dechlorination occurred after the supplementation of carbon sources, indicating the existence of other PCB dechlorinating organisms not closely related to Dehalococcoides or o-17/DF-1 (data not shown). Additionally, it was possible that biogenic Fe(II) also induced abiotic dechlorination. In contrast, the additions of carbon sources did not facilitate further dechlorination in H-1 or H-2 (Supplementary Table S7).
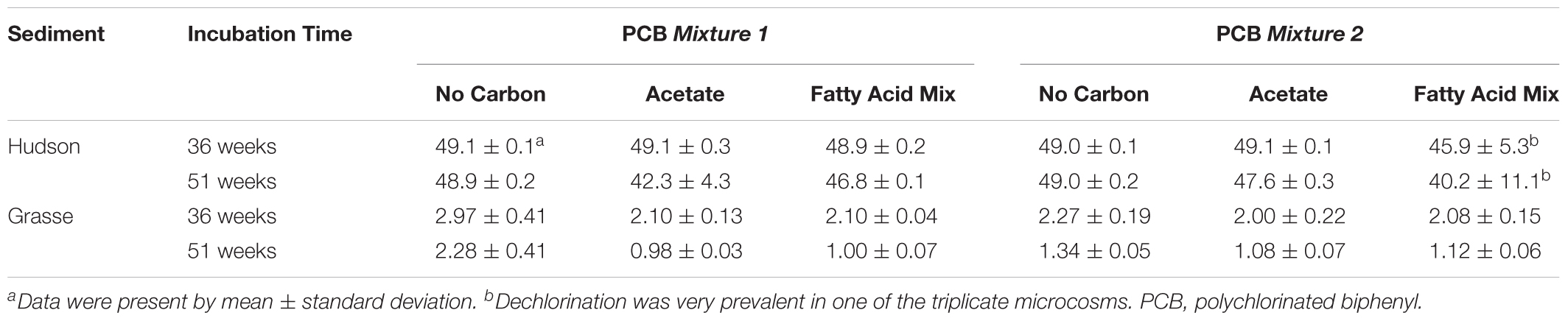
TABLE 3. Total parent PCB concentrations (mg/kg slurry) of the FeOOH-amended sediment microcosms with/without additional carbon sources.
The supplementation of carbon sources into FeOOH-amended Grasse sediment microcosms did not significantly enhance overall dechlorination by re-initiating unflanked para dechlorination activity. However, it did favor the reduction of parent PCBs (Table 3). The reduction was more apparent for the highly chlorinated parent PCB congeners, indicating a relatively high carbon source-dependence for these congeners. Surprisingly, rare ortho dechlorination, targeting PCB congeners with exclusive unflanked ortho chlorines, was apparently enhanced by the addition of acetate or the fatty acid mixture in both G-1 and G-1-Fe (congener-specific concentrations provided in Supplementary Tables S8, S9). Ortho dechlorination products, which were only detected at Week 51 in G-1 and G-1-Fe without additional carbon sources, appeared 15 weeks ahead with carbon source addition. The enhancement was relatively weak in G-1 (see Supplementary Material). By contrast, in the triplicate acetate/fatty acid mixture amended G-1-Fe microcosms, PCB 13 and PCB 15 concentrations increased sharply from Week 36 to Week 51 (PCB 13 and PCB 15 were 15.0 ± 1.2, 7.0 ± 1.2 for acetate-amended G-1-Fe, 12.7 ± 5.0, 10.1 ± 5.0 for fatty acid mixture-amended G-1-Fe at Week 51) and no significant difference of the concentrations of ortho dechlorination products was observed by adding two carbon sources (Supplementary Table S9). Moreover, a rough mass balance could be obtained by the transformation of PCB 25 to PCB 13 and PCB 28 to PCB 15 between Week 36 and Week 51, where PCB 105, PCB 114, and their first and second generation dechlorination products were very low (see text in Supplementary Material and Supplementary Table S9). By the end of the incubation, in the G-1-Fe microcosms with carbon sources, ortho dechlorination products PCB 13 and PCB 15 accounted for 10–15% of the total PCB molar, which were over five times higher than those in the regular G-1-Fe microcosms without extra carbon sources. These findings suggest the similar enhancement ability of acetate or the fatty acid mixture and further confirmed the existence of ortho dechlorination pathways in Grasse River sediment. However, the 16S rRNA gene numbers of Dehalococcoides and o-17/DF-1 in the microcosms with carbon sources did not change significantly comparing with the microcosms without carbon source addition (data not shown). In addition, Fe(II) analysis in the FeOOH-amended Grasse sediment microcosms with supplementary carbon sources showed that no further Fe(III) reduction was induced. This further confirmed that Fe(III) reduction had completed prior to the addition of carbon sources. The supplementation of carbon sources stimulated prevalent methane production. The fatty acid mixture induced more methane production than did the addition of acetate alone (Supplementary Figure S1 and Supplementary Table S6).
Discussion
Impact of Indigenous Sediment Properties and PCB Composition Under Fe(III)-Reducing Condition
Many studies suggested that the dechlorination of PCBs was controlled by their sediment geochemical properties and/or microbial species (Wiegel and Wu, 2000; Yan et al., 2006; Park et al., 2011; Payne et al., 2012). This is the first observation of complete dechlorination inhibition in Fe(III)-amended Hudson sediment. A previous study had reported that 50 mM FeOOH reduced dechlorination by 12% in enriched cultures of sediment collected from a different location in the Hudson River (Morris et al., 1992). Due to the lack of sediment property characterization in that study, the difference is difficult to explain. The relatively low background Fe concentration (approximately 19 mmole/kg slurry), low Geobacteraceae and slow Fe(III) reduction observed in our Hudson sediment suggest poor acclimation to high Fe concentration, resulting in complete inhibition of PCB dechlorination and methanogenesis in FeOOH-amended Hudson sediment microcosms. In the Fe(III)-amended Grasse sediment microcosms, there was a 20–30% reduction of overall dechlorination in a time course of 51 weeks compared to the non-FeOOH-amended Grasse sediment microcosms, mainly due to less para dechlorination. The high background Fe content (approximately 64 mmole/kg slurry), abundant Geobacteraceae and fast Fe(III) reduction suggest good capability for reducing competing electron acceptor and easy resumption of PCB dechlorination. Additionally, our previous study showed that para dechlorination in the high Fe content Grasse sediment (G-1, G-2) was slower than that in the low Fe content Hudson sediment (H-1, H-2) in the rapid phase (Xu et al., 2016). These results suggest that indigenous Fe content could profoundly influence dechlorination preferences. Also, FeOOH further slowed down para dechlorination, but facilitated meta dechlorination in the rapid phase of G-1-Fe and G-2-Fe, indicating that meta/para dechlorination activities might be competing with each other and Fe(III) was likely a controlling factor. Moreover, PCB composition, even with four identical PCB congeners, the same total concentrations, and similar chlorine contents, was found to significantly affect PCB dechlorination in FeOOH-amended Grasse sediment microcosms. PCB Mixture 2 favored the dechlorination of PCB 5, 12, 64, 71, and 170. This trend was found in non-FeOOH-amended G-1 and G-2, suggesting the addition of FeOOH did not alter the composition related dechlorination preferences. These findings might be explained by co-metabolism or halopriming effect in a mixture (Krumins et al., 2009; Fagervold et al., 2011; Xu et al., 2016). FeOOH, especially freshly precipitated FeOOH, has high specific surface area and thus can absorb significant amount of pollutants in sediment and soil (Herbich, 2000; Okkenhaug et al., 2013). In this study, freshly prepared FeOOH was introduced directly into the sediment microcosms and the microcosms were incubated statically. Therefore, it was likely that PCBs adsorbed on the sediment particles aggregated on FeOOH. Along with the reduction of Fe(III), there may have been more bioavailable PCBs in the niches, where prevalent Fe(III)-reducing bacteria as well as related PCB dechlorinators were expected. In addition, biogenic Fe(II), mostly as adsorbed Fe(II), was likely to contribute to PCB dechlorination (Watson et al., 2002; Gong et al., 2016; Liu et al., 2017). This hypothesis was partially supported by the observation that more hydrophobic PCB congeners (highly chlorinated PCB 144, 149, 153, 170, and coplanar PCB 105, 114) exhibited more rapid dechlorination when FeOOH was added. A previous study demonstrated enhanced PCB dechlorination following Processes M and Q with the addition of FeSO4 in Hudson River sediment and proposed the beneficial effect caused by precipitation of toxic sulfide by Fe2+ (Zwiernik et al., 1998). However, the dechlorination induced by FeS could not be excluded (Watson et al., 2002). FeCl2 without sulfate was also found to favor PCB dechlorination (Zwiernik et al., 1998). This further suggested that Fe(II) might be a reductant for chemical dechlorination. Generally, the mineral reactivity of dechlorination of chlorinated solvents was disorder FeS > FeS > FeS2 > sorbed Fe2+ (He et al., 2015). This indicates that the influences of Fe(II)-containing minerals on PCB dechlorination might be complicated.
Putative PCB Dechlorinators and Fe(III) Reducers
Although exhibiting less extensive dechlorination, FeOOH-amended Grasse sediment microcosms showed an increase of Dehalococcoides 16S rRNA genes when compared with non-FeOOH-amended Grasse sediment microcosms. This indicates complex interactions between Fe(III)-reduction and Dehalococcoides growth. The possible mechanistic explanation is that some Fe(III) reducers within the Genus Geobacter of the Family Geobacteraceae, like Geobacter sulfurreducens, are involved in H2 generation in cooperation with hydrogen-oxidizing microorganisms or produce H2 via fermentation. H2 is believed to be the preferred electron donor for Dehalococcoides (Cord-Ruwisch et al., 1998; He et al., 2003, 2005; Wei and Finneran, 2011; Zanaroli et al., 2012b; Karatas et al., 2014). Enrichment of Dehalococcoides has been reported during dechlorination of chlorinated solvents under Fe(III)-reducing conditions (Wei and Finneran, 2011). Moreover, two known Fe(III)-reducing bacteria, Geobacter thiogenes strain K and Geobacter lovleyi strain SZ were capable of utilizing organohalides as their terminal electron acceptors for energy conservation and growth (Sung et al., 2006), and more recently, indirect evidence linking PCB dechlorination with Geobacteraceae was reported, although D. mccartyi was still considered as the main dechlorinators (Praveckova et al., 2016). These reports suggest complex dechlorination mechanisms in the presence of Fe(III) in addition to the potential for competition for electron donors. Geobacteraceae were not stimulated in Fe(III)-amended Grasse sediments, suggesting that electron acceptor Fe(III) was no longer the limiting factor in the Fe rich sediment. Similar findings were reported in Fe rich sediment from South China (Liu et al., 2017).
Carbon Source and Ortho Dechlorination Enhancement
A previous study demonstrated that repeated addition of fatty acids (acetate, propionate, butyrate, and hexanoic acid) stimulated PCB dechlorination in carbon-limiting Hudson sediment, but not in high carbon content New Bedford Harbor and Silver Lake sediments.(Alder et al., 1993) In the present study, the influences of auxiliary carbon sources on PCB dechlorination were also sediment dependent. For the relatively low carbon and low Fe Hudson sediment, the addition of acetate or the fatty acid mixture did not enhance the overall dechlorination in H-1 and H-2 (under methanogenic conditions). This might be due to the experimental dechlorination limit observed as a flat plateau phase after 30 weeks. On the other hand, the addition of carbon sources led to the resumption of PCB dechlorination in FeOOH-amended microcosms (H-1-Fe and H-2-Fe, no methanogenesis activity before carbon sources addition), suggesting the effective compensation of the extra “electron donor demand” derived from Fe(III) (Wei and Finneran, 2011). For the relatively higher carbon content and high Fe Grasse sediment, the addition of acetate or the fatty acid mixture did not enhance the overall dechlorination rate and extent in all the sediment microcosms (G-1, G-2, G-1-Fe, and G-2-Fe), where methanogenesis was remained. However, auxiliary carbon sources apparently shortened the lag time for ortho dechlorination and greatly favored ortho removal in G-1-Fe. These results indicate that the impact of carbon source was not only controlled by the sediment background carbon content, but also by the other geochemical properties, such as Fe(III).
This work provides the first observation of ortho dechlorination enhancement by auxiliary carbon sources (acetate or a fatty acid mixture). The isolated ortho dechlorinators o-17 is known as an acetate-utilizing bacterium (Cutter et al., 2001; May et al., 2006; Fagervold et al., 2011). It is expected that the ortho dechlorinating bacteria in this study are also utilizing acetate as their electron donor and carbon source. As there was no significant difference for the enhancement of ortho removal in acetate-amended and the fatty acid mixture-amended microcosms, acetate was not likely the obligate carbon source in this Grasse sediment. Most previous studies observed ortho dechlorination capable of dechlorinating PCBs with two or more ortho chlorines (Vandort and Bedard, 1991; Pulliam Holoman et al., 1998; Cutter et al., 2001; May et al., 2006; Fagervold et al., 2011; Kjellerup et al., 2014). O-17, isolated from Baltimore Harbor marine sediment preferentially dechlorinates meta-flanked ortho chlorine when the meta chlorine is only ortho-flanked and the target PCB congener contains two to three ortho chlorines, such as PCB 65(2356-CB) and PCB 90(235-24-CB) (May et al., 2006; Fagervold et al., 2007, 2011). Although PCB 65 was ultimately dechlorinated to PCB 14(35-CB) with PCB 23(235-CB) as an intermediate, the ortho dechlorination activity was not sustained when only PCB 23 was spiked, indicating o-17 lacks ortho dechlorination activity for mono-ortho substituted PCBs (May et al., 2006). Another marine sediment from Hunters Point California, in which a fatty acid mixture of acetate, propionate and butyrate was added, exhibited ortho removal targeting PCB 116(23456-CB) (meta chlorines are double-flanked) and the only dechlorination product was PCB 14(35-CB), indicating a stepwise reduction of two ortho chlorines.(Kjellerup et al., 2014) Ortho dechlorination observed in two freshwater sediments (Silver Lake and Woods Pond) without auxiliary carbon source, attacked unflanked ortho chlorine on PCB 30(246-CB) (Williams, 1994). Our previous study also revealed unflanked ortho dechlorination activity on PCB 28(24-4-CB) and PCB 25(24-3-CB) in Grasse River sediment without auxiliary carbon source (Xu et al., 2016). In the present study, the single unflanked ortho chlorine removal activity was maintained under Fe(III)-reducing conditions despite no change in known ortho dechlorinating organism populations. This further supports the existence of distinct ortho dechlorinating organisms in Grasse River sediment. Also, an easy approach to selectively enhance ortho dechlorination was provided. Most recently, a study in Grasse River sediment microcosms focusing on Aroclor 1254 detoxification used dioxin-like PCB 105 as a test congener, in which PCB 28 and PCB 25 were also the dominant dechlorination products after 180 days (Kaya et al., 2017). Therefore, the ortho dechlorination preference with the potential for complete dechlorination of mono-ortho PCB congeners, including some dioxin-like congeners, might be crucial for further potential risk reduction in Grasse sediment.
Conclusion
The amendment with FeOOH greatly affected PCB dechlorination and the influences were largely controlled by the indigenous differences in sediment. Generally, the low carbon content and low Fe Hudson sediment showed complete inhibition for PCB dechlorination after adding FeOOH, while the relatively high carbon content and high Fe Grasse sediment showed moderate inhibition in the presence of FeOOH. The dechlorination preferences analysis revealed that para-flanked meta dechlorination was primarily preferred followed by ortho-/double-flanked meta dechlorination and single-/double-flanked para dechlorination in Grasse sediment and the partially inhibitory effect was caused by the loss of unflanked para removal activity. Despite the longer lag time observed in the FeOOH-amended Grasse sediment microcosms, Fe(III) reduction and PCB reduction were found to take place concurrently. Rare ortho dechlorination pathways were confirmed in FeOOH-amended Grasse sediment microcosms. Auxiliary carbon sources (acetate, or a fatty acid mixture with acetate, propionate and butyrate) could reinitiate dechlorination in FeOOH-amended Hudson sediment microcosms, as well as support the favored ortho dechlorination in FeOOH-amended Grasse microcosms, indicating the utilization of acetate and/or fatty acids for ortho dechlorination related microorganisms in Grasse sediment.
Author Contributions
YX has conducted the experiments, analyzed data, and prepared the manuscript. KG has written the manuscript. JV has conceived and led the project and finalized the manuscript. All authors approved the current version of the manuscript.
Funding
The work was funded by U.S. Department of Defense, Strategic Environmental Research and Development Program (SERDP) through the Environmental Restoration Program (Grant No. ER-1495), the National Natural Science Foundation of China (Grant Nos. 41671468 and 41301546), the Natural Science Foundation of Jiangsu Province (Grant No. BK20171356), State Key Laboratory of Pollution Control and Resource Reuse (Grant No. PCRRF16018), the Priority Academic Program Development of Jiangsu Higher Education Institutions and the Fundamental Research Funds for the Central Universities.
Conflict of Interest Statement
The authors declare that the research was conducted in the absence of any commercial or financial relationships that could be construed as a potential conflict of interest.
Acknowledgments
The authors are grateful to collaborators at The Aluminum Company of America (ALCOA) for their kind support in provision of sediments.
Supplementary Material
The Supplementary Material for this article can be found online at: https://www.frontiersin.org/articles/10.3389/fmicb.2018.01574/full#supplementary-material
References
Adrian, L., Dudkova, V., Demnerova, K., and Bedard, D. L. (2009). Dehalococcoides sp strain CBDB1 extensively dechlorinates the commercial polychlorinated biphenyl mixture Aroclor 1260. Appl. Environ. Microbiol. 75, 4516–4524. doi: 10.1128/aem.00102-09
Alder, A. C., Haggblom, M. M., Oppenhelmer, S. R., and Young, L. Y. (1993). Reductive dechlorination of polychlorinated-biphenyls in anaerobic sediments. Environ. Sci. Technol. 27, 530–538. doi: 10.1021/es00040a012
Bedard, D. L., and Quensen, J. F. (1995). “Microbial reductive dechlorination of polychlorinated biphenyls,” in Microbial Transformation and Degradation of Toxic Organic Chemicals, eds L. Y. Young and G. E. Cerniglia (New York, NY: Wiley-Liss, Inc.), 127–216.
Bedard, D. L., Pohl, E. A., Bailey, J. J., and Murphy, A. (2005). Characterization of the PCB substrate range of microbial dechlorination process LP. Environ. Sci. Technol. 39, 6831–6838. doi: 10.1021/es050255i
Brown, J. F., Bedard, D. L., Brennan, M. J., Carnahan, J. C., Feng, H., and Wagner, R. E. (1987). Polychlorinated biphenyl dechlorination in aquatic sediments. Science 236, 709–712. doi: 10.1126/science.236.4802.709
Chen, M. J., Tong, H., Liu, C. S., Chen, D. D., Li, F. B., and Qiao, J. T. (2016). A humic substance analogue AQDS stimulates Geobacter sp abundance and enhances pentachlorophenol transformation in a paddy soil. Chemosphere 160, 141–148. doi: 10.1016/j.chemosphere.2016.06.061
Cho, Y. C., and Oh, K. H. (2005). Effects of sulfate concentration on the anaerobic dechlorination of polychlorinated biphenyls in estuarine sediments. J. Microbiol. 43, 166–171.
Cord-Ruwisch, R., Lovley, D. R., and Schink, B. (1998). Growth of Geobacter sulfurreducens with acetate in syntrophic cooperation with hydrogen-oxidizing anaerobic partners. Appl. Environ. Microbiol. 64, 2232–2236.
Cummings, D. E., Snoeyenbos-West, O. L., Newby, D. T., Niggemyer, A. M., Lovley, D. R., Achenbach, L. A., et al. (2003). Diversity of geobacteraceae species inhabiting metal-polluted freshwater lake sediments ascertained by 16S rDNA analyses. Microb. Ecol. 46, 257–269. doi: 10.1007/s00248-002-0005-8
Cutter, L., Sowers, K. R., and May, H. D. (1998). Microbial dechlorination of 2,3,5,6-tetrachlorobiphenyl under anaerobic conditions in the absence of soil or sediment. Appl. Environ. Microbiol. 64, 2966–2969.
Cutter, L. A., Watts, J. E. M., Sowers, K. R., and May, H. D. (2001). Identification of a microorganism that links its growth to the reductive dechlorination of 2,3,5,6-chlorobiphenyl. Envrion. Microbiol. 3, 699–709. doi: 10.1046/j.1462-2920.2001.00246.x
Edwards, U., Rogall, T., Blocker, H., Emde, M., and Bottger, E. C. (1989). Isolation and direct complete nucleotide determination of entire genes. Characterization of a gene coding for 16S ribosomal RNA. Nucleic Acids Res. 17, 7843–7853. doi: 10.1093/nar/17.19.7843
EPA (1997). Phase II Reassessment: Data Evaluation and Interpretation Report for the Hudson River PCB Superfund Site. Washington, DC: EPA.
Fagervold, S. K., May, H. D., and Sowers, K. R. (2007). Microbial reductive dechlorination of Aroclor 1260 in Baltimore Harbor sediment microcosms is catalyzed by three phylotypes within the phylum Chloroflexi. Appl. Environ. Microbiol. 73, 3009–3018. doi: 10.1128/aem.02958-06
Fagervold, S. K., Watts, J. E. M., May, H. D., and Sowers, K. R. (2011). Effects of bioaugmentation on indigenous PCB dechlorinating activity in sediment microcosms. Water Res. 45, 3899–3907. doi: 10.1016/j.watres.2011.04.048
Fennell, D. E., Nijenhuis, I., Wilson, S. F., Zinder, S. H., and Haggblom, M. M. (2004). Dehalococcoides ethenogenes strain 195 reductively dechlorinates diverse chlorinated aromatic pollutants. Environ. Sci. Technol. 38, 2075–2081. doi: 10.1021/es034989b
Gong, Y. Y., Tang, J. C., and Zhao, D. Y. (2016). Application of iron sulfide particles for groundwater and soil remediation: a review. Water Res. 89, 309–320. doi: 10.1016/j.watres.2015.11.063
He, J., Sung, Y., Krajmalnik-Brown, R., Ritalahti, K. M., and Loffler, F. E. (2005). Isolation and characterization of Dehalococcoides sp strain FL2, a trichloroethene (TCE)- and 1,2-dichloroethene-respiring anaerobe. Environ. Microbiol. 7, 1442–1450. doi: 10.1111/j.1462-2920.2005.00830.x
He, J. Z., Ritalahti, K. M., Aiello, M. R., and Loffler, F. E. (2003). Complete detoxification of vinyl chloride by an anaerobic enrichment culture and identification of the reductively dechlorinating population as a Dehalococcoides species. Appl. Environ. Microbiol. 69, 996–1003. doi: 10.1128/aem.69.2.996-1003.2003
He, Y. T., Wilson, J. T., Su, C., and Wilkin, R. T. (2015). Review of abiotic degradation of chlorinated solvents by reactive iron minerals in aquifers. Ground Water Monit. Remediat. 35, 57–75. doi: 10.1111/gwmr.12111
Herbich, J. B. (2000). Handbook of Dredging Engineering, 2nd Edn. New York City, NY: McGraw-Hill Professional.
Himmelheber, D. W., Thomas, S. H., Loffler, F. E., Taillefert, M., and Hughes, J. B. (2009). Microbial colonization of an in situ sediment cap and correlation to stratified redox zones. Environ. Sci. Technol. 43, 66–74. doi: 10.1021/es801834e
Ho, C. H., and Liu, S. M. (2012). Impact of coplanar PCBs on microbial communities in anaerobic estuarine sediments. J. Environ. Sci. Health B 45, 437–448. doi: 10.1080/03601231003800172
Hughes, A. S., Vanbriesen, J. M., and Small, M. J. (2010). Identification of structural properties associated with polychlorinated biphenyl dechlorination processes. Environ. Sci. Technol. 44, 2842–2848. doi: 10.1021/es902109w
IARC Monographs (2016). “Polychlorinated biphenyls and polybrominated biphenyls,” in IARC Monographs on the Evaluation of Carcinogenic Risks to Humans (Lyon: International Agency for Research on Cancer).
Jeong, H. Y., Anantharaman, K., Han, Y. S., and Hayes, K. F. (2011). Abiotic reductive dechlorination of cis-dichloroethylene by Fe species formed during iron- or sulfate-reduction. Environ. Sci. Technol. 45, 5186–5194. doi: 10.1021/es104387w
Karatas, S., Hasar, H., Taskan, E., Ozkaya, B., and Sahinkaya, E. (2014). Bio-reduction of tetrachloroethen using a H-2-based membrane biofilm reactor and community fingerprinting. Water Res. 58, 21–28. doi: 10.1016/j.watres.2014.03.053
Karcher, S. C., Small, M. J., and Vanbriesen, J. M. (2004). Statistical method to evaluate the occurrence of PCB transformations in river sediments with application to Hudson River data. Environ. Sci. Technol. 38, 6760–6766. doi: 10.1021/es0494149
Kaya, D., Imamoglu, I., Sanin, F. D., Payne, R. B., and Sowers, K. R. (2017). Potential risk reduction of Aroclor 1254 by microbial dechlorination in anaerobic Grasse River sediment microcosms. J. Hazard. Mater. 321, 879–887. doi: 10.1016/j.jhazmat.2016.10.009
Kjellerup, B. V., Naff, C., Edwards, S. J., Ghosh, U., Baker, J. E., and Sowers, K. R. (2014). Effects of activated carbon on reductive dechlorination of PCBs by organohalide respiring bacteria indigenous to sediments. Water Res. 52, 1–10. doi: 10.1016/j.watres.2013.12.030
Kjellerup, B. V., Sun, X. L., Ghosh, U., May, H. D., and Sowers, K. R. (2008). Site-specific microbial communities in three PCB-impacted sediments are associated with different in situ dechlorinating activities. Envrion. Microbiol. 10, 1296–1309. doi: 10.1111/j.1462-2920.2007.01543.x
Krumins, V., Park, J. W., Son, E. K., Rodenburg, L. A., Kerkhof, L. J., Haggblom, M. M., et al. (2009). PCB dechlorination enhancement in Anacostia River sediment microcosms. Water Res. 43, 4549–4558. doi: 10.1016/j.watres.2009.08.003
Laroe, S. L., Fricker, A. D., and Bedard, D. L. (2014). Dehalococcoides mccartyi strain JNA in pure culture extensively dechlorinates Aroclor 1260 according to polychlorinated biphenyl (PCB) dechlorination Process N. Environ. Sci. Technol. 48, 9187–9196. doi: 10.1021/es500872t
Liu, X. P., Wan, H., Xue, Y. Z., Feng, C. H., and Wei, C. H. (2017). Addition of iron oxides in sediments enhances 2,3,4,5-tetrachlorobiphenyl (PCB 61) dechlorination by low-voltage electric fields. RSC Adv. 7, 26019–26027. doi: 10.1039/c7ra02849k
Lovley, D. R., and Phillips, E. J. P. (1986). Organic-matter mineralization with reduction of ferric iron in anaerobic sediments. Appl. Environ. Microbiol. 51, 683–689.
Lyall, K., Croen, L. A., Sjodin, A., Yoshida, C. K., Zerbo, O., Kharrazi, M., et al. (2017). Polychlorinated biphenyl and organochlorine pesticide concentrations in maternal mid-pregnancy serum samples: association with autism spectrum disorder and intellectual disability. Environ. Health Perspect. 125, 474–480. doi: 10.1289/ehp277
Matturro, B., Ubaldi, C., and Rossetti, S. (2016). Microbiome dynamics of a polychlorobiphenyl (PCB) historically contaminated marine sediment under conditions promoting reductive dechlorination. Front. Microbiol. 7:1502. doi: 10.3389/fmicb.2016.01502
May, H. D., Cutter, L. A., Miller, G. S., Milliken, C. E., Watts, J. E. M., and Sowers, K. R. (2006). Stimulatory and inhibitory effects of organohalides on the dehalogenating activities of PCB-dechlorinating bacterium o-17. Environ. Sci. Technol. 40, 5704–5709. doi: 10.1021/es052521y
Morris, P. J., Mohn, W. W., Quensen, J. F., Tiedje, J. M., and Boyd, S. A. (1992). Establishment of a polychlorinated biphenyl-degrading enrichment culture with predominantly meta dechlorination. Appl. Environ. Microbiol. 58, 3088–3094.
Muyzer, G., Dewaal, E. C., and Uitterlinden, A. G. (1993). Profiling of complex microbial populations by denaturing gradient gel electrophoresis analysis of polymerase chain reaction-amplified genes coding for 16S rRNA. Appl. Environ. Microbiol. 59, 695–700.
Okkenhaug, G., Amstatter, K., Bue, H. L., Cornelissen, G., Breedveld, G. D., Henriksen, T., et al. (2013). Antimony (Sb) contaminated shooting range soil: sb mobility and immobilization by soil amendments. Environ. Sci. Technol. 47, 6431–6439. doi: 10.1021/es302448k
Park, J. W., Krumins, V., Kjellerup, B. V., Fennell, D. E., Rodenburg, L. A., Sowers, K. R., et al. (2011). The effect of co-substrate activation on indigenous and bioaugmented PCB dechlorinating bacterial communities in sediment microcosms. Appl. Microbiol. Biotechnol. 89, 2005–2017. doi: 10.1007/s00253-010-2958-8
Paul, L., Herrmann, S., Koch, C. B., Philips, J., and Smolders, E. (2013). Inhibition of microbial trichloroethylene dechorination by Fe (III) reduction depends on Fe mineralogy: a batch study using the bioaugmentation culture KB-1. Water Res. 47, 2543–2554. doi: 10.1016/j.watres.2013.02.029
Payne, R. B., May, H. D., and Sowers, K. R. (2012). Enhanced reductive dechlorination of polychlorinated biphenyl impacted sediment by bioaugmentation with a dehalorespiring bacterium. Environ. Sci. Technol. 45, 8772–8779. doi: 10.1021/es201553c
Praveckova, M., Brennerova, M. V., Holliger, C., De Alencastro, F., and Rossi, P. (2016). Indirect evidence link PCB dehalogenation with Geobacteraceae in anaerobic sediment-free microcosms. Front. Microbiol. 7:933. doi: 10.3389/fmicb.2016.00933
Pulliam Holoman, T. R., Elberson, M. A., Cutter, L. A., May, H. D., and Sowers, K. R. (1998). Characterization of a defined 2,3,5,6-tetrachlorobiphenyl-ortho-dechlorinating microbial community by comparative sequence analysis of genes coding for 16S rRNA. Appl. Environ. Microbiol. 64, 3359–3367.
Quensen, J. F., Mousa, M. A., Boyd, S. A., Sanderson, J. T., Froese, K. L., and Giesy, J. P. (1998). Reduction of aryl hydrocarbon receptor-mediated activity of polychlorinated biphenyl mixtures due to anaerobic microbial dechlorination. Environ. Toxicol. Chem. 17, 806–813. doi: 10.1002/etc.5620170507
Safe, S. (1989). Polychlorinated-biphenyls (PCBs) - mutagenicity and carcinogenicity. Mutat. Res. 220, 31–47. doi: 10.1016/0165-1110(89)90007-9
Shelton, D. R., and Tiedje, J. M. (1984). General-method for determining anaerobic biodegradation potential. Appl. Environ. Microbiol. 47, 850–857.
Sorensen, J. (1982). Reduction of ferric iron in anaerobic, marine sediment and interaction with reduction of nitrate and sulfate. Appl. Environ. Microbiol. 43, 319–324.
Sowers, K. R., and May, H. D. (2013). In situ treatment of PCBs by anaerobic microbial dechlorination in aquatic sediment: are we there yet? Curr. Opin. Biotechnol. 24, 482–488. doi: 10.1016/j.copbio.2012.10.004
Sung, Y., Fletcher, K. F., Ritalaliti, K. M., Apkarian, R. P., Ramos-Hernandez, N., Sanford, R. A., et al. (2006). Geobacter lovleyi sp nov strain SZ, a novel metal-reducing and tetrachloroethene-dechlorinating bacterium. Appl. Environ. Microbiol. 72, 2775–2782. doi: 10.1128/aem.72.4.2775-2782.2006
Vandort, H. M., and Bedard, D. L. (1991). Reductive ortho-dechlorination and meta-dechlorination of a polychlorinated biphenyl congener by anaerobic microorganisms. Appl. Environ. Microbiol. 57, 1576–1578.
Wang, S. Q., Chng, K. R., Wilm, A., Zhao, S. Y., Yang, K. L., Nagarajan, N., et al. (2014). Genomic characterization of three unique Dehalococcoides that respire on persistent polychlorinated biphenyls. Proc. Natl. Acad. Sci. U.S.A. 111, 12103–12108. doi: 10.1073/pnas.1404845111
Wang, S. Q., and He, J. Z. (2013). Phylogenetically distinct bacteria involve extensive dechlorination of Aroclor 1260 in sediment-free cultures. PLoS One 8:e59178. doi: 10.1371/journal.pone.0059178
Watson, J. H. P., Ellwood, D. C., Pavoni, B., Lazzari, L., and Sperni, L. (2002). Degradation and Removal of Sediment PCBs Using Microbially Generated Iron Sulfide. Columbus, OH: Battelle Press.
Watts, J. E. M., Fagervold, S. K., May, H. D., and Sowers, K. R. (2005). A PCR-based specific assay reveals a population of bacteria within the Chloroflexi associated with the reductive dehalogenation of polychlorinated biphenyls. Microbiology 151(Pt 6), 2039–2046. doi: 10.1099/mic.0.27819-0
Wei, N., and Finneran, K. T. (2011). Influence of ferric iron on complete dechlorination of trichloroethylene (TCE) to ethene: Fe(III) reduction does not always inhibit complete dechlorination. Environ. Sci. Technol. 45, 7422–7430. doi: 10.1021/es201501a
Wiegel, J., and Wu, Q. Z. (2000). Microbial reductive dehalogenation of polychlorinated biphenyls. FEMS Microbiol. Ecol. 32, 1–15. doi: 10.1111/j.1574-6941.2000.tb00693.x
Williams, W. A. (1994). Microbial reductive dechlorination of trichlorobiphenyls in anaerobic sediment slurries. Environ. Sci. Technol. 28, 630–635. doi: 10.1021/es00053a015
Wu, Q. Z., Watts, J. E. M., Sowers, K. R., and May, H. D. (2002). Identification of a bacterium that specifically catalyzes the reductive dechlorination of polychlorinated biphenyls with doubly flanked chlorines. Appl. Environ. Microbiol. 68, 807–812. doi: 10.1128/AEM.68.2.807-812.2002
Xu, Y., Gregory, K. B., and VanBriesen, J. M. (2016). Microbial-catalyzed reductive dechlorination of polychlorinated biphenyls in Hudson and Grasse River sediment microcosms: determination of dechlorination preferences and identification of rare ortho removal pathways. Environ. Sci. Technol. 50, 12767–12778. doi: 10.1021/acs.est.6b03892
Xu, Y., Yu, Y., Gregory, K. B., and VanBriesen, J. M. (2012). Comprehensive assessment of bacterial communities and analysis of PCB congeners in PCB-contaminated sediment with depth. J. Environ. Eng. 138, 1167–1178. doi: 10.1061/(asce)ee.1943-7870.0000595
Yan, T., LaPara, T. M., and Novak, P. J. (2006). The impact of sediment characteristics on polychlorinated biphenyl- dechlorinating cultures: implications for bioaugmentation. Bioremediat. J. 10, 143–151. doi: 10.1080/10889860601021381
Zanaroli, G., Balloi, A., Negroni, A., Borruso, L., Daffonchio, D., and Fava, F. (2012a). A Chloroflexi bacterium dechlorinates polychlorinated biphenyls in marine sediments under in situ-like biogeochemical conditions. J. Hazard. Mater. 209, 449–457. doi: 10.1016/j.jhazmat.2012.01.042
Zanaroli, G., Negroni, A., Vignola, M., Nuzzo, A., Shu, H. Y., and Fava, F. (2012b). Enhancement of microbial reductive dechlorination of polychlorinated biphenyls (PCBs) in a marine sediment by nanoscale zerovalent iron (NZVI) particles. J. Chem. Technol. Biotechnol. 87, 1246–1253. doi: 10.1002/jctb.3835
Zhen, H. J., Du, S. Y., Rodenburg, L. A., Mainelis, G., and Fennell, D. E. (2014). Reductive dechlorination of 1,2,3,7,8-pentachlorodibenzo-p-dioxin and Aroclor 1260, 1254 and 1242 by a mixed culture containing Dehalococcoides mccartyi strain 195. Water Res. 52, 51–62. doi: 10.1016/j.watres.2013.12.038
Keywords: polychlorinated biphenyls, sediment, reductive dechlorination, electron acceptor, ferric oxyhydroxide, Dehalococcoides
Citation: Xu Y, Gregory KB and VanBriesen JM (2018) Effects of Ferric Oxyhydroxide on Anaerobic Microbial Dechlorination of Polychlorinated Biphenyls in Hudson and Grasse River Sediment Microcosms: Dechlorination Extent, Preferences, Ortho Removal, and Its Enhancement. Front. Microbiol. 9:1574. doi: 10.3389/fmicb.2018.01574
Received: 30 March 2018; Accepted: 25 June 2018;
Published: 20 July 2018.
Edited by:
Shanquan Wang, Sun Yat-sen University, ChinaReviewed by:
Maoyong Song, Research Center for Eco-Environmental Sciences (CAS), ChinaNa Liu, Jilin University, China
Copyright © 2018 Xu, Gregory and VanBriesen. This is an open-access article distributed under the terms of the Creative Commons Attribution License (CC BY). The use, distribution or reproduction in other forums is permitted, provided the original author(s) and the copyright owner(s) are credited and that the original publication in this journal is cited, in accordance with accepted academic practice. No use, distribution or reproduction is permitted which does not comply with these terms.
*Correspondence: Yan Xu, eHV4dWNhbG1tQHNldS5lZHUuY24= Jeanne M. VanBriesen, amVhbm5lQGNtdS5lZHU=