- Department of Veterinary Clinical Sciences, College of Veterinary Medicine, China Agricultural University, Beijing, China
Escherichia coli is a common cause of mastitis in dairy cows. The adaptor protein apoptosis-associated speck-like protein containing a caspase recruitment domain (ASC) synergizes with caspase-1 to regulate inflammasome activation during pathogen infection. Here, the ASC gene was knocked out in bovine mammary epithelial (MAC-T) cells using clustered, regularly interspaced, short palindromic repeat (CRISPR)/CRISPR-associated (Cas)-9 technology. MAC-T cells were pre-incubated with and without Lactobacillus rhamnosus GR-1 and then exposed to E. coli. Western blot analysis demonstrated increased expression of NLRP3 and NLRC4 following E. coli infection, but this increase was attenuated by pre-incubation with L. rhamnosus GR-1, regardless of ASC knockout. Western blot and immunofluorescence analyses revealed that pre-incubation with L. rhamnosus GR-1 decreased E. coli-induced caspase-1 activation at 6 h after E. coli infection, as also observed in ASC-knockout MAC-T cells. The E. coli-induced increase in caspase-4 mRNA expression was inhibited by pre-incubation with L. rhamnosus GR-1. ASC knockout diminished, but did not completely prevent, increased production of IL-1β and IL-18 and cell pyroptosis associated with E. coli infection, whereas pre-incubation with L. rhamnosus GR-1 inhibited this increase. Our data indicate that L. rhamnosus GR-1 suppresses activation of ASC-dependent NLRP3 and NLRC4 inflammasomes and production of downstream IL-lβ and IL-18 during E. coli infection. L. rhamnosus GR-1 also inhibited E. coli-induced cell pyroptosis, in part through attenuation of NLRC4 and non-canonical caspase-4 activation independently of ASC.
Introduction
Escherichia coli is a frequent cause of bovine mastitis and a leading cause of clinical mastitis in bovine (Shaheen et al., 2015). The NLR family member pyrin domain-containing protein 3 (NLRP3) inflammasome is considered a suitable target for new alternatives to antibiotics to treat bovine mastitis (Thacker et al., 2012). Our previous study showed that probiotic Lactobacillus rhamnosus GR-1 ameliorates E. coli-induced inflammatory damage via attenuation of apoptosis-associated speck-like protein containing a caspase recruitment domain (ASC)-independent NLRP3 inflammasome activation in primary bovine mammary epithelial cells (PBMCs) (Wu et al., 2016). Therefore, L. rhamnosus GR-1 represents a potentially promising therapeutic agent targeting inflammasome activity in E. coli-associated bovine mastitis.
Binding of lipopolysaccharide (LPS) from gram-negative bacteria to toll-like receptor (TLR) 4 increases cellular expression of NLRP3 protein through nuclear factor-κB (NF-κB) signaling, leading to rapidly NLRP3 activation (Afonina et al., 2017). Upon activation, NLRP3 nucleates the adaptor protein ASC through interaction with the pyrin domain (PYD). Pro-caspase-1 is subsequently autoproteolytically processed through CARD–CARD (caspase recruitment domain) interactions in the NLRP3/ASC complex scaffold and cleaves precursors of the proinflammatory interleukin (IL)-1 family into their bioactive forms, IL-1β and IL-18. We found that L. rhamnosus GR-1 reduces E. coli-induced caspase-1 activation and production of IL-1β and IL-18. However, in contrast to increases in the expression of NLRP3 and caspase-1, expression of the adaptor protein ASC is decreased in PBMCs infected with E. coli, even in cells pretreated with L. rhamnosus GR-1 (Wu et al., 2016).
In contrast to the multiple stimuli that activate NLRP3, NLRC4 is activated by flagellin and the rod protein EscI of the E. coli type III secretion system (T3SS) apparatus (Miao et al., 2010). NLRC4 contains a CARD motif, through which it directly oligomerizes with caspase-1 independent of ASC; this complex activates caspase-1 without autoproteolysis, triggering pyroptosis, an inflammatory form of cell death (Broz et al., 2010b). However, ASC greatly enhances the efficiency of NLRC4-mediated maturation of IL-1β and IL-18 by inducing caspase-1 autoproteolysis (Lamkanfi and Dixit, 2014). NLRC4-dependent production of IL-1β is induced by pathogenic Salmonella or Pseudomonas but not commensal Lactobacillus plantarum, indicating that the NLRC4 inflammasome specifically discriminates pathogens and probiotic bacteria (Franchi et al., 2012). However, the contributions of the NLRC4 inflammasome to inflammatory responses that control E. coli infections are less clear in relation to L. rhamnosus GR-1.
NLRP3 and NLRC4 inflammasomes play a crucial role in potentiating the host antimicrobial response (Guo et al., 2015). Studies using ASC-deficient cells from ASC-/- mice demonstrated the dual role of ASC in bridging NLRP3 and NLRC4 inflammasomes and caspase-1 via PYD and CARD and regulating the result of inflammasome activation (Broz et al., 2010a; Gueya et al., 2014). ASC-dependent inflammasome activation results in the production of proinflammatory IL-1 family cytokines, whereas ASC-independent inflammasome activation induces cell pyroptosis. Given the significant potential of IL-1 family cytokines to cause detrimental inflammation and pyroptosis to control the spread of intracellular pathogens (Jorgensen et al., 2016; Lannitti et al., 2016), the role of ASC in regulating inflammasome activity during E. coli infection must be examined in detail to determine and how L. rhamnosus GR-1 regulates the immune response to prevent E. coli-associated bovine mastitis.
In the present study, we knocked out the ASC gene in bovine mammary epithelial (MAC-T) cells using the RNA-guided clustered regularly interspaced short palindrome repeats (CRISPR)-CRISPR-associated nuclease 9 (Cas9) system. We hypothesized that during E. coli infection, the activity of NLRP3 and NLRC4 inflammasomes is differentially regulated by L. rhamnosus GR-1, inducing maturation of IL-1β and IL-18 or cell pyroptosis, depending on ASC. We provide evidence that L. rhamnosus GR-1 suppresses E. coli-induced ASC-dependent activation of NLRP3 and NLRC4 inflammasomes and thus decreases production of IL-lβ and IL-18 during E. coli infection. In addition, L. rhamnosus GR-1 suppresses E. coli-induced cell pyroptosis, in part through attenuation of NLRC4 inflammasome and non-canonical caspase-4 activation, independent of ASC.
Materials and Methods
Biosecurity Statement
All bacterial strains were treated in strict accordance with the Regulations on Biological Safety Management of Pathogen Microbiology Laboratory (000014349/2004-00195) from the State Council of the People’s Republic of China. The E. coli CVCC1450 was subjected to all necessary safety procedures to avoid pathogen transmission and infection.
Construction of CRISPR/Cas9 System Expression Vector
Three guide RNAs (ASC-sgRNA1, ASC-sgRNA 2, and ASC-sgRNA 3) were designed to target the exon 1 regions of the bovine ASC gene (Table 1). A pair of oligos for each targeting site was annealed and ligated into the BbsI site of pCRISPR-sg5, which was kindly provided by Professor Sen Wu (China Agricultural University, Beijing, China), to generate pCRISPR-sg5-ASC-sgRNA1, pCRISPR-sg5-ASC-sgRNA2, and pCRISPR-sg5-ASC-sgRNA3 plasmids. All plasmids were confirmed by sequencing (Sangon Biotech, Shanghai, China).
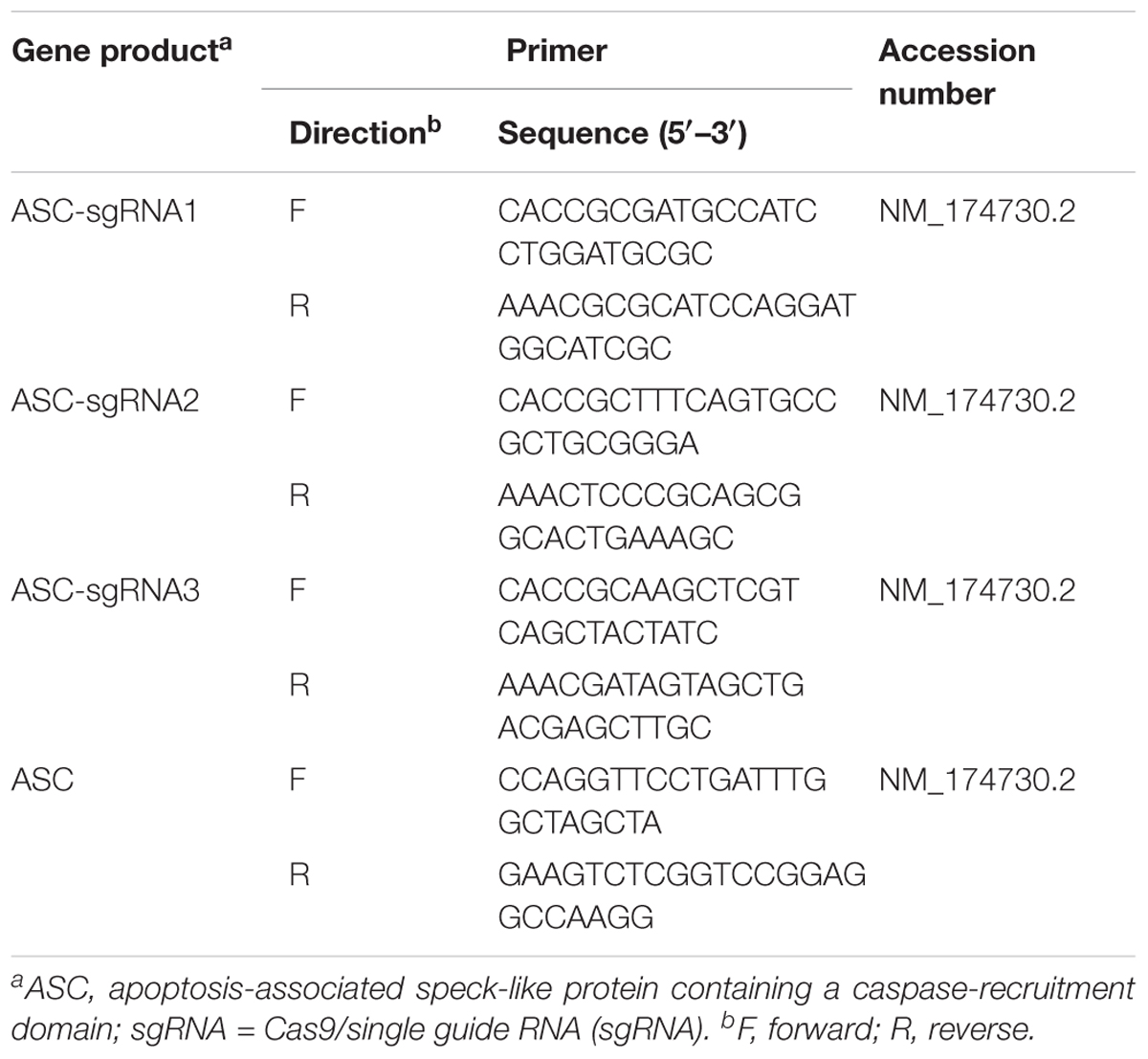
TABLE 1. Sequences of three guide RNAs designed to target the exon 1 region of the bovine ASC gene and primers for PCR amplification.
Cell Culture and Transfection
MAC-T cells transferred with the SV40 T antigen (Huynh et al., 1991) was a gift from Dr. Ying Yu (China Agricultural University). MAC-T cells were cultured in Dulbecco’s Modified Eagle medium/Ham’s F-12 medium (1:1) supplemented with 10% heat-inactivated fetal calf serum, 100 U/mL of penicillin, and 1 g/mL of streptomycin (Invitrogen, Carlsbad, CA, United States) at 37°C in an atmosphere of 5% CO2 and 95% air at 95% relative humidity.
Plasmid DNA for cell transfection was prepared using an Omega Endo-free Plasmid Mini Kit II (Omega Bio-Tek Inc., Doraville, GA, United States). MAC-T cells (1 × 106) were electroporated with 1.5 μg of pCRISPR-W9 plasmid, 1.5 μg of pCRISPR-sg5-ASC-sgRNA plasmid, and 1 μg of pCAG-PBase plasmid using the T-020 program of an Amaxa electroporator (Lonza, Allendale, NJ, United States), in which pCRISPR-W9 encoded Cas9 nuclease and pCRISPR-sg5-ASC-sgRNA encoded ASC-sgRNA. After electroporation, 300 cells were plated in a 10-cm dish using growth medium containing 350 μg/ml of selectable marker G418 (Sigma-Aldrich, St. Louis, MO, United States). After 10 days, individual clones were picked, and clonal cell populations were expanded. Before experiments, MAC-T cells were electroporated with pmaxGFPTM (Lonza) encoding green fluorescent protein to determine transfection efficiency using the T-020 and W-001 programs. MAC-T cells were chosen for CRISPR-Cas9 inactivation experiments due to their good transfection efficiency.
Sequencing and Protein Analysis of the Gene Target Site
Genomic DNA samples were extracted using a TIANamp Genomic DNA Kit (Tiangen, Beijing, China) according to the manufacturer’s instructions, and 50 ng of DNA template was used to amplify the 630-bp fragment encompassing the gene inactivation locus in 25 μl of PCR buffer (Takara, Shiga, Japan) using primer pairs listed in Table 1. The resulting PCR products were purified and subsequently sequenced to identify deletions. In addition, clonal cell population whole-cell extracts were analyzed by Western blotting.
Immunocytochemistry
The epithelial origin of MAC-T cells was tested by staining for cytokeratin 18. MAC-T cells (6 × 104 cells/well) were seeded into a 24-well culture plate with glass coverslips. After 24 h, cells were washed three times with phosphate-buffered saline (PBS) and fixed with 4% paraformaldehyde for 15 min on ice. The cells were then permeabilized with 0.2% (v/v) Triton X-100 (Sigma-Aldrich) and blocked with 1% bovine serum albumin. Subsequently, cells were incubated with mouse anti-cytokeratin-18 primary monoclonal antibody at a dilution of 1:200 (Ab668; Abcam, Cambridge, United Kingdom) for 45 min at 4°C, following by incubation with secondary antibody, goat anti-mouse fluorescein isothiocyanate (FITC)-conjugated IgG (F4143; Sigma-Aldrich). Cell nuclei were stained using 4′,6′-diamidino-2-phenylindole (DAPI; Sigma-Aldrich). Coverslips were imaged on an FV1000 confocal laser scanning biological microscope (Olympus, Tokyo, Japan).
Bacterial Strains and Growth Conditions
Lactobacillus rhamnosus GR-1 ATCC 55826 was purchased from the American Type Culture Collection (Manassas, VA, United States) and grown in De Man, Rogosa, and Sharpe (MRS) broth (Oxoid, Hampshire, United Kingdom) for 24 h at 37°C under microaerophilic conditions. After overnight incubation, L. rhamnosus GR-1 was subcultured at a dilution of 1:100 in fresh MRS broth for approximately 8 h until reaching mid-log phase [optical density (OD) at 600 nm (OD600) of 0.5] for all experiments.
Escherichia coli CVCC1450 (serotype O111:K58) was purchased from the China Institute of Veterinary Drug Center (Beijing, China) and grown in Luria–Bertani (LB) broth (Oxoid). After overnight incubation at 37°C with vigorous shaking, bacteria were diluted 1:100 in fresh LB and grown for approximately 3 h until reaching mid-log phase (OD600 of 0.5).
Adhesion Assay
Wild-type (WT) and ASC-/- MAC-T cells (3 × 105 cells/well) were seeded onto a six-well transwell collagen-coated polytetrafluoroethylene (PTFE) filter. Confluent cell monolayers were pretreated with L. rhamnosus GR-1 (3 × 107 CFU) for 3 h, and then were washed three times with PBS and exposed to E. coli (3 × 107 CFU). At 1.5, 3, and 6 h after E. coli challenge, the cell monolayers were washed four times with PBS to remove non-adherent bacteria and treated with 0.05% trypsin for 10 min at 37°C. Cells were harvested by centrifugation for 10 min at 4000 g and lysed using 100 μl of 0.2% Triton X-100 (Sigma-Aldrich) in sterile water. The populations of E. coli and L. rhamnosus GR-1 were determined on LB and MRS agar plates, respectively. The adhesion rate of E. coli was defined as the adhered E. coli population on the cells pretreated with L. rhamnosus GR-1 relative to the adhered E. coli population in the adhesion assay of E. coli infection alone.
Immunofluorescence
Confluent monolayers of WT and ASC-/- MAC-T cells (6 × 104 cells/well) grown on glass coverslips in a 24-well flat-bottom culture plate were treated under four different conditions, as follows: (i) medium alone (CONT); (ii) E. coli alone (6 × 106 CFU) at a multiplicity of infection (MOI) of 100:1 (ECOL); (iii) incubation with L. rhamnosus GR-1 (6 × 106 CFU) at a MOI of 100:1 for 3 h (LRGR); or (iv) pre-incubation with L. rhamnosus GR-1 (6 × 106 CFU) for 3 h prior to addition of E. coli (LRGR + ECOL). At 6 h after E. coli infection, the cells were washed, fixed with 4% paraformaldehyde for 15 min on ice, permeabilized with 0.2% (v/v) Triton X-100 (Sigma-Aldrich), and blocked with 1% bovine serum albumin. Subsequently, the following primary monoclonal antibodies were used: mouse anti-cytokeratin-18 (Ab668, 1:200 dilution; Abcam), rabbit anti-ASC (10500-1-AP, 1:500 dilution; Proteintech Group, Chicago, IL, United States), and mouse anti-caspase-1 (22915-1-AP, 1:500 dilution; Proteintech Group). The cells were incubated with the primary antibody for 45 min at 4°C, followed by incubation with goat anti-rabbit Cy-3 (AP307F, 1:200 dilution; Sigma-Aldrich) or FITC-conjugated IgG (F-0382, 1:40 dilution; Sigma-Aldrich) as the secondary antibody. Cell nuclei were stained with DAPI. The coverslips and slides were visualized and photographed under an FV1000 confocal laser scanning biological microscope (Olympus).
Western Blotting
WT and ASC-/- MAC-T cells (6 × 104 cells/well) were seeded onto a six-well transwell collagen-coated PTFE filter and treated with E. coli or L. rhamnosus GR-1 at a MOI of 100:1, as described above. Cells were also simultaneously treated with lactate at a concentration of 0.6 g/L (equivalent to 7 mM) and E. coli at a MOI of 100:1. At 1.5, 3, and 6 h after E. coli infection, cells were extracted using Radio-Immunoprecipitation Assay buffer (Sigma-Aldrich), as previously described (Wu et al., 2016). The primary antibodies were as follows: rabbit anti-NLRP3 (19771-1-AP, 1:200 dilution; Proteintech Group), rabbit anti-NLRC4 (12421, 1:1,000 dilution; Cell Signaling Technologies Inc., Danvers, MA, United States), mouse anti-caspase-1 (sc56036) (14F468, 1:500 dilution; Santa Cruz Biotechnology, Dallas, TX, United States), rabbit anti-cleaved caspase-4 (Gln81) (GTX86890, 1:250 dilution; GeneTex, Inc., San Antonio, TX, United States), and mouse anti-glyceraldehyde-3-phosphate dehydrogenase (GAPDH, 60004-1-Ig, 1:500 dilution; Proteintech Group). Horseradish peroxidase-conjugated AffiniPure goat anti-mouse IgG (SA00001-1, 1:5,000 dilution; Proteintech Group) or goat anti-rabbit IgG (SA00001-2, 1:5,000 dilution; Proteintech Group) were used as secondary antibodies. The detection of NLRP3 and caspase-1 proteins was performed in the same gel. After NLRP3 and caspase-1 proteins were visualized, blots were then stripped using Restore Western Blot Stripping Buffer (Solarbio, Beijing, China), and re-probed with the desired antibodies for GAPDH. The OD of each band was quantified by densitometric analysis using Quantity One software (Bio-Rad Laboratories, Richmond, CA, United States). Results are presented as the ratio of the NLRP3, NLRC4, caspase p10 subunit or cleaved caspase-4 band intensity to the GAPDH band intensity.
Lactate Dehydrogenase (LDH) Assay
The death of WT or ASC-/- MAC-T cells under the different conditions was assessed using the CytoTox 96 Non-Radioactive Cytotoxicity Assay (Promega, Madison, WI, United States) according to the manufacturer’s instructions. The assay measures the release of LDH into the supernatant, calculated as the percentage of total LDH content as determined from cell lysates (100%). LDH released by uninfected cells was used as a maximum-lysis control. The percentage of LDH released was calculated using the following equation: [(LDH infected–LDH uninfected)/(LDH total lysis–LDH uninfected)] × 100.
Enzyme-Linked Immunosorbent Assay (ELISA)
The concentrations of IL-1β and IL-18 in cell-free supernatants of WT or ASC-/- cells were determined at 1.5, 3, and 6 h after E. coli infection using commercially available ELISA kits specific for bovine IL-1β (DG90995Q) and bovine IL-18 (DG91524Q; Beijing Dongge Biotechnology Co., Beijing, China).
Quantification of Lactate Content
Cell culture supernatants were collected. Lactate content in the supernatants were quantified using the enzymatic kit K-DLATE (Megazyme, Bray, Ireland) that allows the measurement of both D-lactate and L-lactate.
Statistical Analysis
Statistical analysis was performed using the SAS statistical software package, version 9.1 (SAS Institute Inc., Cary, NC, United States). With regard to small sample sizes, normal distribution and homogeneity of variance were assumed using the UNIVARIATE (Shapiro–Wilk test) and HOVTEST procedures. Natural logarithm transformation was performed prior to analysis for IL-1β and IL-18 data to yield a normal distribution. Statistical significance of differences was tested using ANOVA procedures, following Tukey’s honestly significant difference post hoc test. Data of adhesion assay were compared by an unpaired two-tailed Student’s t-test. Data were visualized using GraphPad Prism5 software (GraphPad Software Inc., San Diego, CA, United States). Data from adhesion assay are presented as the mean ± standard deviation (SD) and data from Western blotting, LDH, ELISA and lactate quantification assays are presented as the mean ± standard error of the mean (SEM). Results are representative of three independent experiments, each performed in triplicate. P-values: ∗P < 0.05; ∗∗P < 0.01; ∗∗∗P < 0.001.
Results
CRISPR/Cas9 Mediates Knockout of ASC in MAC-T Cells
To demonstrate the role of ASC in L. rhamnosus GR-1 modulation of inflammasome activation during E. coli infection, we attempted knockout of the ASC gene in MAC-T cells using the CRISPR/Cas9 system. Upon immunocytochemistry analysis, MAC-T cells showed intense positive staining for epithelial cell-specific cytokeratin-18 in the cytoplasmic meshwork of cytokeratin fibrils (Supplementary Figure S1). Compared with program W-001, after electroporation with program T-020, MAC-T cells exhibited higher transfection efficiency (Figure 1A). Thus, the ASC gene knockout experiment was subsequently performed in MAC-T cells using program T-020. Among the three designed sgRNA sequences, the specific 20-nucleotide sgRNA1 sequence targeted the exon 1 regions of the ASC gene and directed Cas9 nuclease to precisely introduce a DNA double-strand break in front of a protospacer adjacent motif (PAM). After cloning and sequencing of the DNA fragment, an 8-bp deletion was observed (Figure 1B). Western blot analysis did not show expression of ASC protein in sgRNA1 sequence-targeted MAC-T cells (Figure 1C). Furthermore, E. coli infection triggered assembly of ASC specks in WT cells, whereas pre-incubation with L. rhamnosus GR-1 attenuated E. coli-induced ASC speck assembly. No ASC specks were observed in ASC-/- cells, regardless of E. coli infection (Figure 1D). These results demonstrated that knockout of the ASC gene in MAC-T cells was successful.
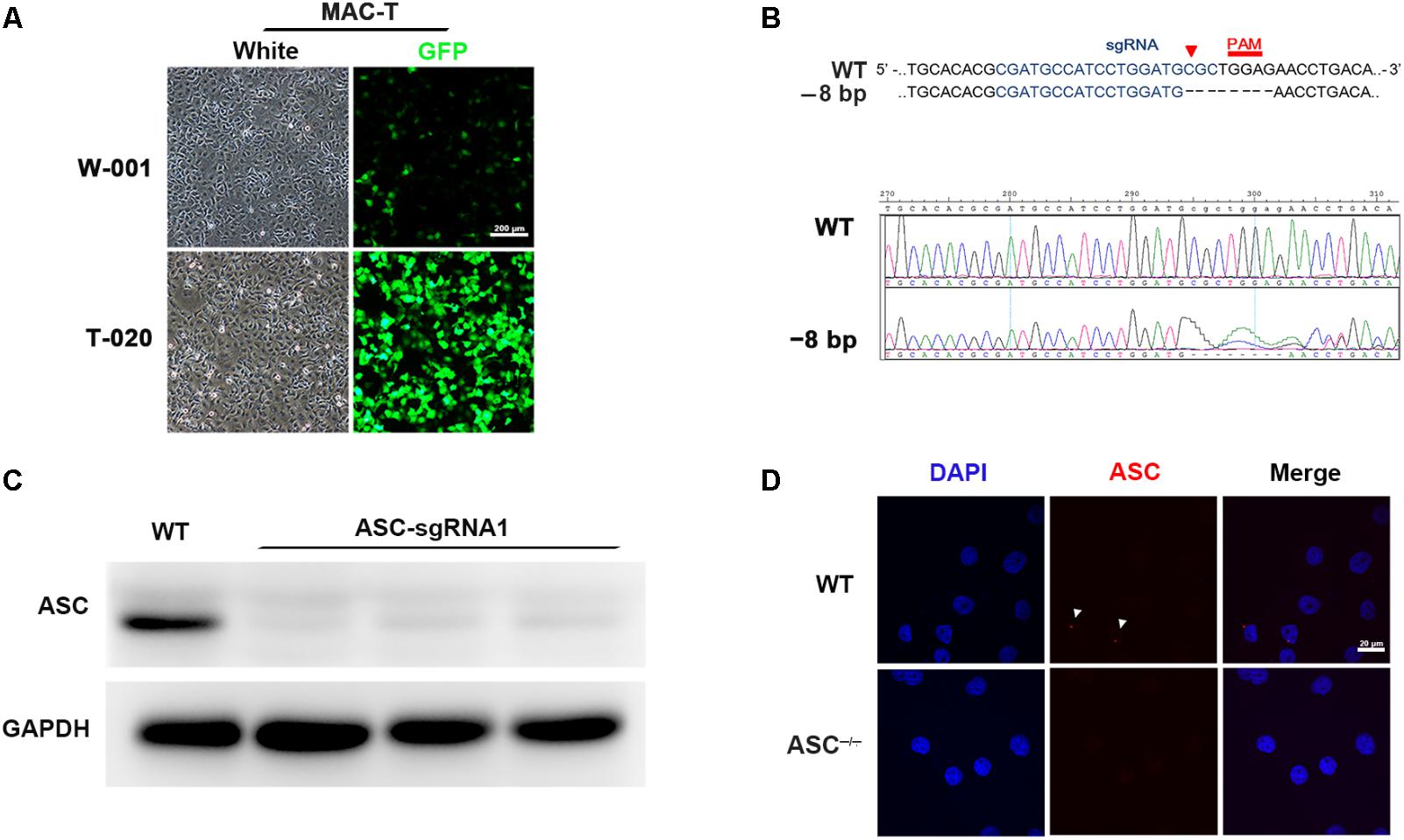
FIGURE 1. CRISPR/Cas9 system-mediated knockout of the ASC gene in MAC-T cells. (A) To determine transfection efficiency, MAC-T cells were electroporated with pmaxGFP encoding green fluorescent protein using the programs W-001 and T-020, respectively. (B) Schematic illustrating Cas9 inactivation of the bovine ASC locus. The 20-bp guide RNA target sequence is shown in blue, and the protospacer-adjacent motif (PAM) is shown in red. An 8-bp deletion was detected (Upper). Representative Sanger sequencing results of target regions of ASC (frameshift indels; Lower). (C) Analysis of ASC protein in WT and ASC-/- cells transfected with Cas-9 and ASC guide RNA expression vector by Western blotting. (D) Immunofluorescence staining of ASC (red) in WT and ASC-/- cells at 6 h after Escherichia coli challenge. DAPI was used for nuclear staining (blue). Representative confocal immunofluorescence images show staining of ASC. Scale bar, 20 μm. Data are representative of three independent experiments.
Pre-incubation With L. rhamnosus GR-1 Reduces the Adhesion of E. coli to MAC-T Cell Monolayers
Escherichia coli or L. rhamnosus GR-1 exhibited similar adhesion capacity in WT and ASC-/- MAC-T cells. The number of adherent E. coli was about 1.29 × 104 ± 0.67 × 102 CFU (means ± standard deviation) at 1.5 h after E. coli infection, and increased to 1.95 × 104 ± 1.17 × 103 CFU at 6 h. At 3 h after E. coli challenge, the number of adherent E. coli in ASC-/- cells was lower (P = 0.007) than in WT MAC-T cells (Figure 2A). L. rhamnosus GR-1 had a lower adhesion capacity and the number of adherent L. rhamnosus GR-1 was about 8.75 × 103 ± 1.56 × 103 CFU, regardless of infection time. Pre-incubation with L. rhamnosus GR-1 resulted in a reduction in the E. coli adhesion rate to 57% of that observed in MAC-T cells infected with E. coli alone (Figure 2B). No differences were observed in the number of E. coli recovered from the supernatant fraction among two groups (Figure 2C).

FIGURE 2. Pre-incubation with Lactobacillus rhamnosus GR-1 reduced the adhesion of E. coli to MAC-T cell monolayers. An adhesion assay was performed. Cells and supernatants were collected. The number of adherent E. coli and L. rhamnosus GR-1 was determined (A). The adhesion rate of E. coli was defined as the adhered E. coli population on the cells pretreated with L. rhamnosus GR-1 relative to the adhered E. coli population in the adhesion assay of E. coli infection alone (B). The number of E. coli recovered was determined in adhesion assay supernatants (C). Data are presented as the mean ± SD of three independent experiments. Asterisks indicate the significances among different treatments in the same cell type. Pound signs indicate the significance among WT and ASC-/- MAC-T cells infected with E. coli alone at 3 h. ∗P < 0.05, ∗∗P < 0.01, ##P < 0.01.
Pre-incubation With L. rhamnosus GR-1 Attenuates E. coli-Induced NLRP3 Expression and Increases Lactate Content in the Supernatants
Compared with untreated control WT cells, Western blot analysis showed an increase in NLRP3 protein expression at 1.5, 3, and 6 h after E. coli challenge in cells only infected with E. coli, but not in cells incubated with L. rhamnosus GR-1 alone (P = 0.046, P < 0.001, and P < 0.001, respectively; Figures 3A–C). In contrast, WT cells pre-incubated with L. rhamnosus GR-1 had a lower expression of NLRP3 protein than did WT cells only infected with E. coli at 3 and 6 h (P < 0.001 for both).
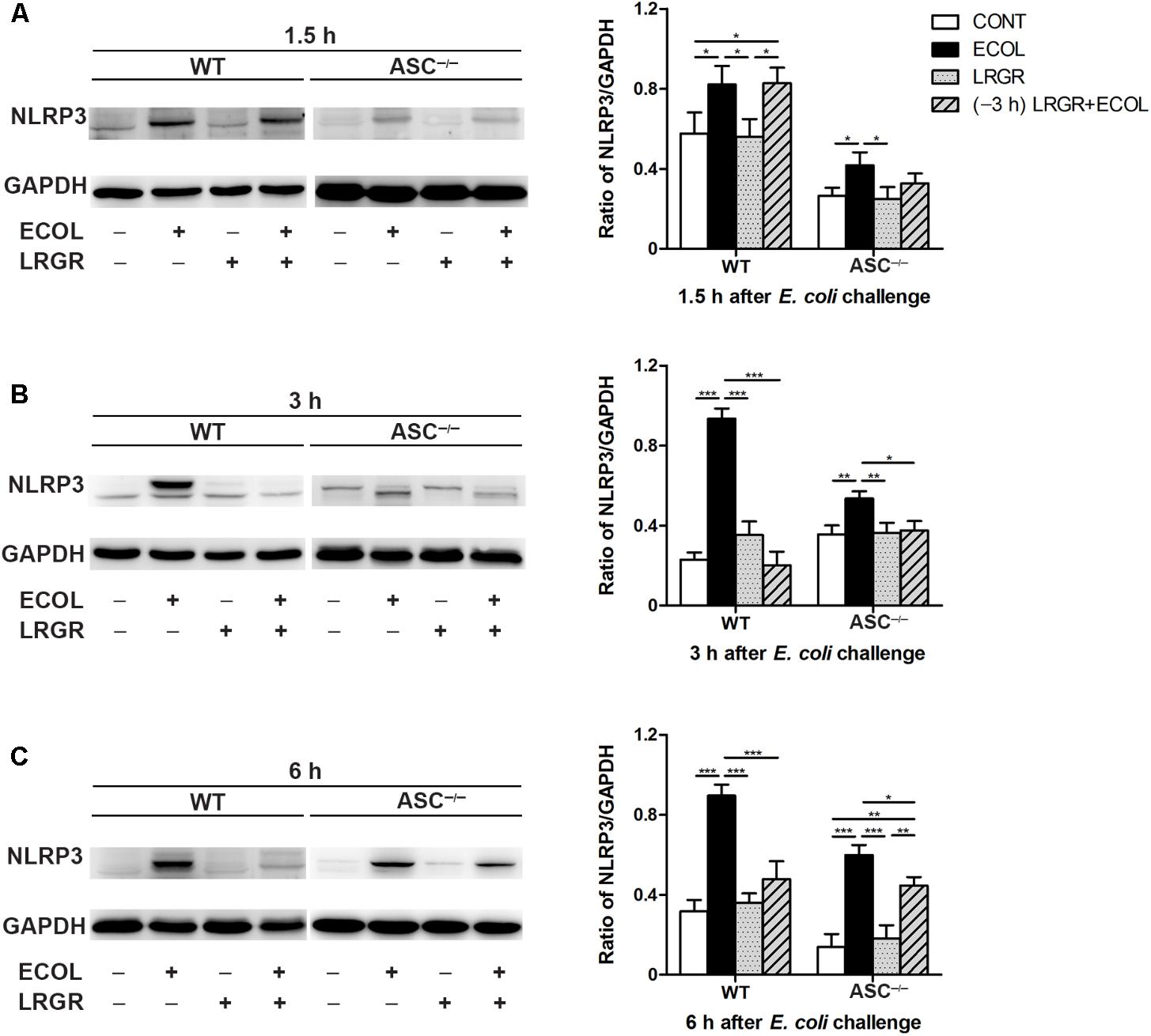
FIGURE 3. Pre-incubation with L. rhamnosus GR-1 attenuated E. coli-induced NLRP3 expression. Western blot detection of NLRP3 in WT and ASC-/- cells collected from the indicated cell cultures at 1.5 h (A), 3 h (B), and 6 h (C) after E. coli challenge. Representative panels showing expression of NLRP3 protein (Left). Results are presented as the ratio of NLRP3 band intensity to that of GAPDH (Right). Data are presented as the mean ± SEM of three independent experiments. ∗P < 0.05, ∗∗P < 0.01, ∗∗∗P < 0.001.
Compared with WT cells, ASC-/- MAC-T cells exhibited a similar differential response to E. coli challenge and L. rhamnosus GR-1 incubation. Compared with untreated control ASC-/- cells, at 1.5, 3, and 6 h after E. coli challenge, NLRP3 protein expression was elevated in ASC-/- cells only infected with E. coli (P = 0.036, P = 0.005, and P < 0.001, respectively; Figures 3A–C) but not in ASC-/- cells pre-incubated with L. rhamnosus GR-1.
The lactate content (D-lactate plus L-lactate) in the supernatants was quantified. Compared with untreated control cells, the lactate content was increased in the supernatants from both WT and ASC-/- cells incubated with L. rhamnosus GR-1 alone or pre-incubated with L. rhamnosus GR-1, but not the cells only infected with E. coli at 1.5, 3, and 6 h after infection, regardless of ASC knockout (P < 0.05; Supplementary Figure S2A). Western blot analysis showed that compared with untreated control cells, at 6 h after E. coli challenge, NLRP3 protein expression was elevated in WT or ASC-/- cells infected with E. coli, but not in cells only treated with lactate alone (P < 0.05; Supplementary Figure S2B). Lactate addition did not attenuate E. coli-induced increase in NLRP3 protein expression in either WT or ASC-/- cells.
Effect of L. rhamnosus GR-1 on NLRC4 Activation During E. coli Infection
Compared with untreated control cells, expression of NLRC4 protein was elevated at 3 h after E. coli infection in WT cells only infected with E. coli (P = 0.027; Figure 4B) but not in WT cells incubated with L. rhamnosus GR-1 alone or pre-incubated with L. rhamnosus GR-1. Compared with WT cells only infected with E. coli, expression of NLRC4 protein at 3 h was decreased in WT cells incubated with L. rhamnosus GR-1 alone and pre-incubated with L. rhamnosus GR-1 (P = 0.019 and P = 0.001, respectively). No differences were observed at 3 h in ASC-/- cells, regardless of treatment.
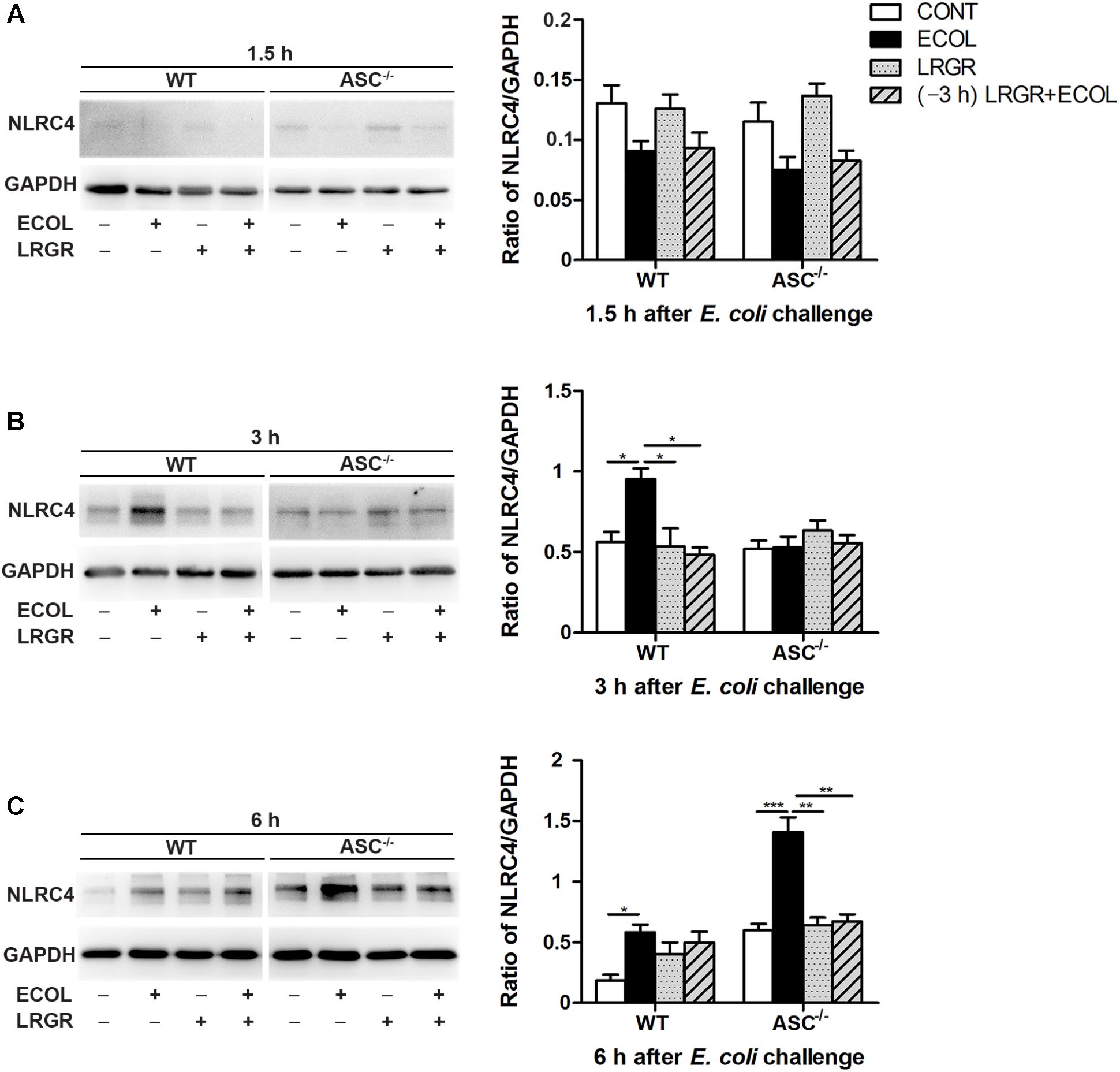
FIGURE 4. Western blot detection of NLRC4 protein. Representative panels showing expression of NLRP4 protein in WT and ASC-/- cells collected from the indicated cultures at 1.5 h (A), 3 h (B), and 6 h (C) after E. coli challenge (Left). Results are presented as the ratio of NLRC4 band intensity to that of GAPDH (Right). Data are presented as the mean ± SEM of three independent experiments. ∗P < 0.05, ∗∗P < 0.01, ∗∗∗P < 0.001.
Compared with untreated control cells, E. coli challenge led to increased expression of NLRC4 protein at 6 h after E. coli challenge in WT cells (P = 0.019) and ASC-/- cells (P < 0.001; Figure 4C). Expression of NLRC4 protein was lower in ASC-/- cells incubated with L. rhamnosus GR-1 alone and pre-incubated with L. rhamnosus GR-1 (P = 0.001 for both) than in ASC-/- cells only infected with E. coli. No changes were observed at 1.5 h after E. coli infection in WT cells or ASC-/- cells, regardless of treatment (Figure 4A).
Pre-incubation With L. rhamnosus GR-1 Attenuates E. coli-Induced Caspase-1 Maturation
Immunofluorescence staining showed that compared with untreated control cells, E. coli challenge triggered assembly of ASC specks in WT cells at 6 h after E. coli challenge, and this was attenuated in WT cells pre-incubated with L. rhamnosus GR-1 (Figure 5A). No ASC specks were observed in ASC-/- cells, regardless of treatment. Compared with untreated control cells, bright foci indicative of increased caspase-1 expression was observed in WT cells only infected with E. coli at 6 h after challenge; this increase was attenuated by incubation with L. rhamnosus GR-1 (Figure 5B). Compared with WT cells, ASC deletion attenuated, but did not abolish, caspase-1 staining in response to E. coli infection and pre-incubation with L. rhamnosus GR-1. A punctate staining pattern for caspase-1 was observed in ASC-/- cells only infected with E. coli, and pre-incubation with L. rhamnosus GR-1 inhibited the E. coli-induced punctate caspase-1 staining pattern. Compared with untreated control cells, incubation with L. rhamnosus GR-1 only did not result in increase in caspase-1 activation either in WT or ASC-/- cells (Figure 5B).
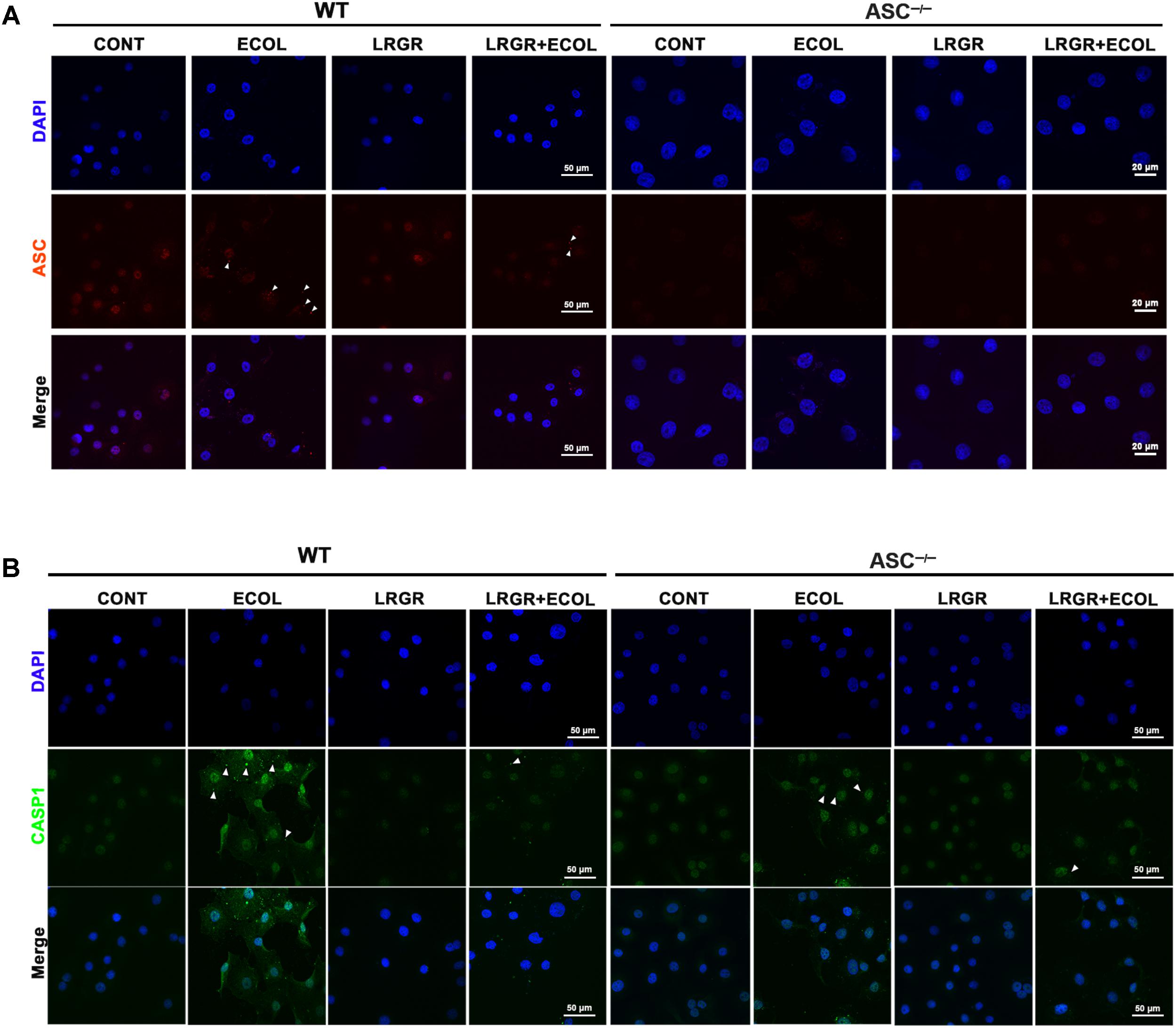
FIGURE 5. Immunofluorescence staining for ASC and caspase-1. Immunofluorescence staining for ASC (A) and caspase-1 (B) in WT and ASC-/- cells collected from the indicated cell cultures at 6 h after E. coli challenge. Cells were immunostained for ASC (red) and caspase-1 (green). DAPI (blue) was used to localize nuclei. Arrows mark specks. Scale bars, immunofluorescence staining for ASC in WT cells and for caspase-1 in WT and ASC-/- cells: 50 μm, and immunofluorescence staining for ASC in ASC-/- cells: 20 μm. Data are representative of three independent experiments.
Compared with untreated control cells, increased maturation of procaspase-1 into its catalytic 10-kDa subunit was observed at 6 h after E. coli challenge in WT and ASC-/- cells only infected with E. coli (P < 0.001 for both; Figure 6C). However, maturation of caspase-1 declined in WT and ASC-/- cells pre-incubated with L. rhamnosus GR-1 (P = 0.014 and P = 0.006, respectively) or incubated with L. rhamnosus GR-1 alone (P < 0.001 for both), compared with WT cells infected with E. coli only. No differences were observed in caspase-1 maturation at 1.5 and 3 h after E. coli infection in WT cells or ASC-/- cells, regardless of treatment (Figures 6A,B).
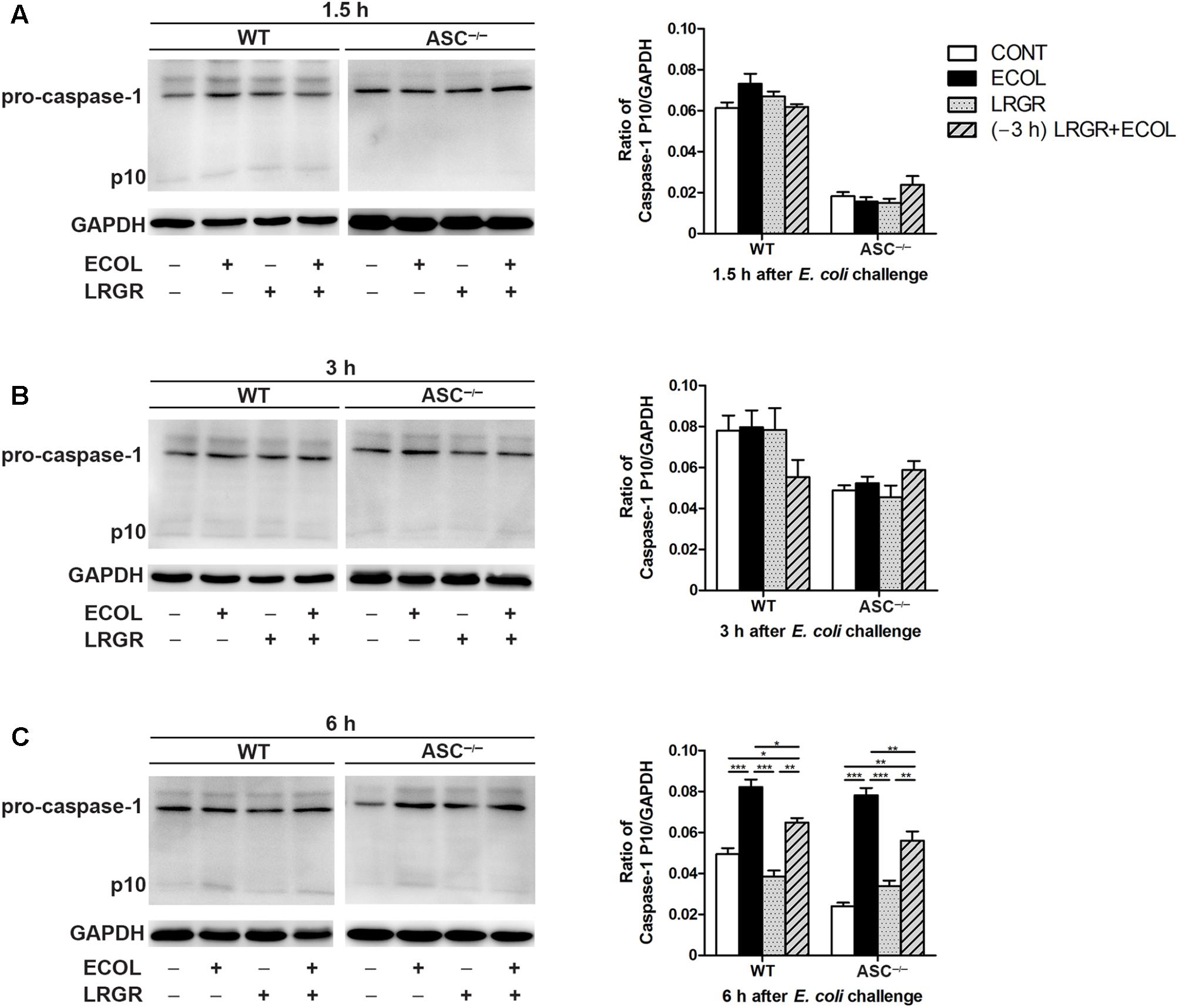
FIGURE 6. Pre-incubation with L. rhamnosus GR-1 attenuated E. coli-induced activation of caspase-1. Western blot detection of caspase-1 in WT and ASC-/- cells collected from the indicated cell cultures at 1.5 h (A), 3 h (B), and 6 h (C) after E. coli challenge. Representative panels showing expression of caspase-1 (Left). Results are presented as the ratio of caspase-1 p10 subunit band intensity to that of GAPDH (Right). The detection of NLRP3 and caspase-1 proteins was performed in the same gel. Caspase-1 blots shared the same GAPDH blots with NLRP3 protein. Data are presented as the mean ± SEM of three independent experiments. ∗P < 0.05, ∗∗P < 0.01, ∗∗∗P < 0.001.
Pre-incubation With L. rhamnosus GR-1 Attenuates E. coli-Induced Caspase-4 Activation
At 3 h after E. coli infection, expression of cleaved caspase-4 (26 kDa) in WT and ASC-/- cells pre-incubated with L. rhamnosus GR-1 was lower than in untreated control cells and cells only infected with E. coli (Figure 7B). Infection with E. coli resulted in increased expression of cleaved caspase-4 in WT and ASC-/- cells (P = 0.001 and P = 0.004, respectively; Figure 7C) at 6 h. However, expression of caspase-4 decreased in WT and ASC-/- cells pre-incubated with L. rhamnosus GR-1, compared with cells only infected with E. coli (P < 0.001 and P = 0.001, respectively). No differences were observed in the expression of cleaved caspase-4 at 1.5 h after E. coli infection in WT or ASC-/- cells, regardless of treatment (Figure 7A).
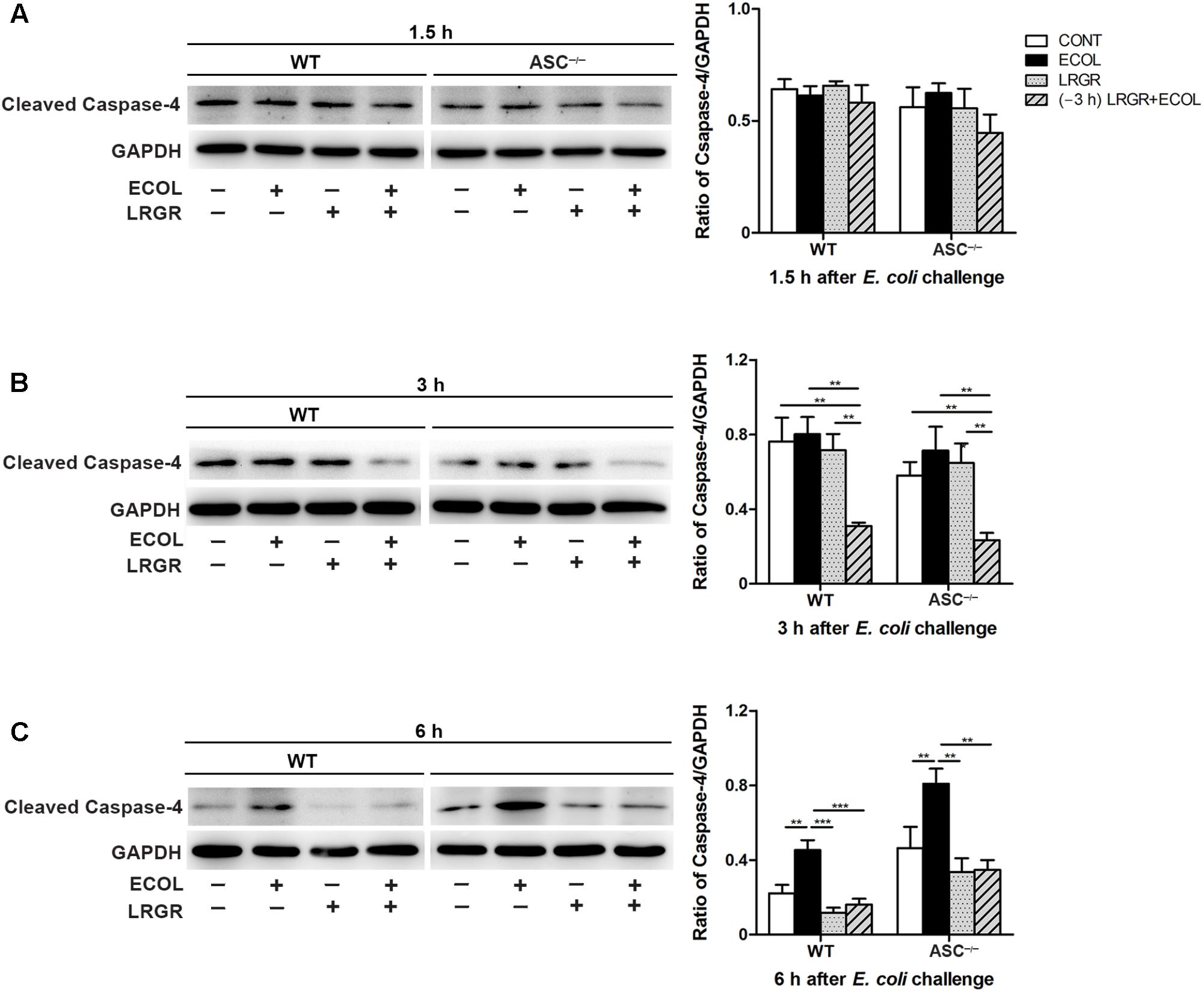
FIGURE 7. Pre-incubation with L. rhamnosus GR-1 attenuated E. coli-induced activation of caspase-4. Western blot detection of cleaved caspase-4 in WT and ASC-/- cells collected from the indicated cell cultures at 1.5 h (A), 3 h (B), and 6 h (C) after E. coli challenge. Representative panels showing expression of cleaved caspase-4 (Left). Results are presented as the ratio of cleaved caspase-4 band intensity to that of GAPDH (Right). Data are presented as the mean ± SEM of three independent experiments. ∗∗P < 0.01, ∗∗∗P < 0.001.
Pre-incubation With L. rhamnosus GR-1 Attenuates E. coli-Induced Production of IL-1β and IL-18 and Cell Pyroptosis
Compared with untreated control cells, IL-1β production was increased at 3 and 6 h after E. coli infection in WT cells only infected with E. coli (P < 0.001 for both; Figure 8A). IL-1β production was lower at 3 and 6 h both in WT cells incubated with L. rhamnosus GR-1 alone (P = 0.002 and P = 0.004, respectively) and cells pre-incubated with L. rhamnosus GR-1 (P = 0.013 and P = 0.011, respectively) than in WT cells only infected with E. coli. Compared with WT cells, ASC deletion led to a decrease in production of IL-1β in ASC-/- cells in response to different treatments. Challenge with E. coli resulted in elevated production of IL-1β at 1.5, 3, and 6 h after E. coli infection in ASC-/- cells only infected with E. coli (P = 0.009, P = 0.021, and P = 0.001, respectively; Figure 8A) compared with untreated control cells; however, the E. coli-induced increase in IL-1β production was attenuated at 3 and 6 h by incubation with L. rhamnosus GR-1 alone (P = 0.014 and P = 0.007, respectively) and pre-incubation with L. rhamnosus GR-1 (P = 0.009 and P = 0.003, respectively).
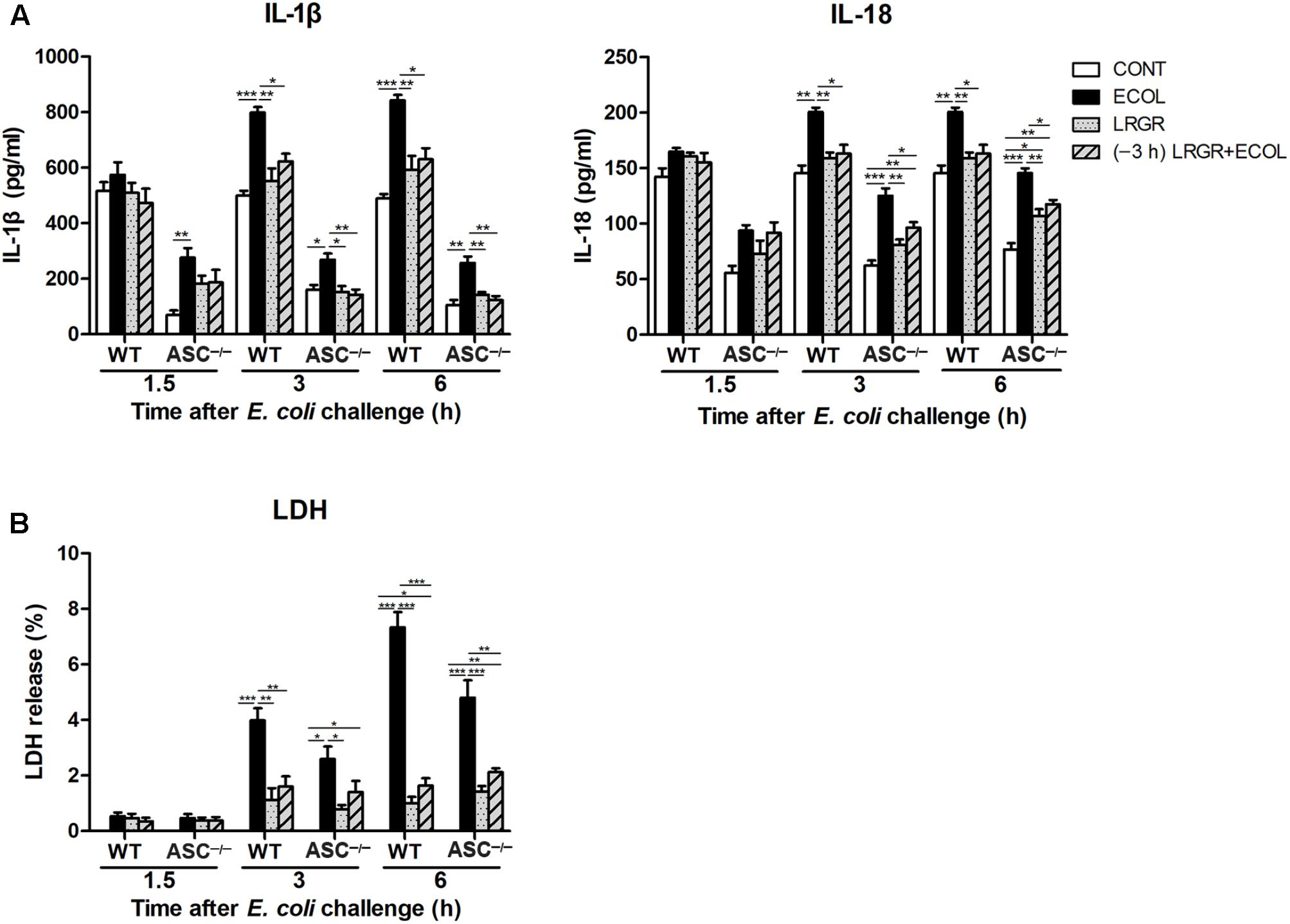
FIGURE 8. Pre-incubation with L. rhamnosus GR-1 attenuated E. coli-induced production of IL-1β and IL-18 and cell pyroptosis. Secretion of IL-1β and IL-18 (A) into the indicated cell culture supernatants at 1.5, 3, and 6 h after E. coli challenge as determined by ELISA. Cell pyroptosis was determined by measurement of LDH release (B). Data are presented as the mean ± SEM of three independent experiments. ∗P < 0.05, ∗∗P < 0.01, ∗∗∗P < 0.001.
IL-18 exhibited similar differential production as IL-1β. Compared with untreated control cells, production of IL-18 was increased at 3 and 6 h after E. coli challenge both in WT (P = 0.001 for both) and ASC-/- (P < 0.001 for both) cells only infected with E. coli (Figure 8A). However, incubation with L. rhamnosus GR-1 alone and pre-incubation with L. rhamnosus GR-1 attenuated the E. coli-induced elevation in the concentration of IL-18 at 3 and 6 h after E. coli challenge both in WT and ASC-/- cells. Compared with untreated control ASC-/- cells, the concentration of IL-18 was elevated at 3 and 6 h in ASC-/- cells pre-incubated with L. rhamnosus GR-1 (P = 0.008 and P = 0.002, respectively).
Cell pyroptosis was quantified by monitoring the release of LDH into the supernatants after E. coli challenge. Compared with untreated control cells, the percentage of pyroptotic cells at 3 and 6 h was increased in WT cells only infected with E. coli (P < 0.001 for both; Figure 8B) but not in WT cells incubated with L. rhamnosus GR-1 alone and pre-incubated with L. rhamnosus GR-1. Compared with WT cells, ASC deletion let to a similarly differential but attenuated cell pyroptosis. Compared with untreated control cells, the percentage of pyroptotic cells was increased at 3 and 6 h in ASC-/- cells only infected with E. coli (P = 0.020 and P < 0.001, respectively), whereas incubation with L. rhamnosus GR-1 alone and pre-incubation with L. rhamnosus GR-1 ameliorated the E. coli-induced increase in pyroptotic cell death at 6 h (P < 0.001 and P = 0.002, respectively). No changes were observed in WT and ASC-/- cells, regardless of treatment.
Discussion
Bacterial adhesion to host epithelial cells is an essential step in the initiation of infection. Lactobacillus can reduce pathogen adhesion to epithelial cells and exert direct antimicrobial activity due to accumulation of antimicrobial substances (Gudina et al., 2015). We found that L. rhamnosus GR-1 did not directly kill E. coli, but did decrease the level of E. coli adhesion to 57% of that observed in MAC-T cells infected with E. coli alone. We previously revealed that live and ultraviolet-irradiated L. rhamnosus GR-1 rather than culture supernatant of L. rhamnosus GR-1 and medium acidified with lactate lead to a decrease in the E. coli adhesion rate in bovine mammary epithelial cells (Wu et al., 2016). The reduced E. coli adhesion level mediated by L. rhamnosus GR-1 may be attributed to steric hindrance due to competition for attachment sites (Ardita et al., 2014; Tytgat et al., 2016).
NLRP3 is activated by a wide variety of stimuli, including pore-forming toxins, extracellular adenosine triphosphate, RNA-DNA hybrid molecules, and pathogens (Jo et al., 2016). We found that E. coli infection also increased the expression of NLRP3 protein from 1.5 to 6 h, but L. rhamnosus GR-1 pretreatment inhibited this increase. NLRP3 must be primed before activation. Escherichia coli LPS binds to TLR4 to induce expression of NLRP3 protein via NF-κB signaling. Bacterial mRNA from viable E. coli cells that have been phagocytosed enters the cytosolic compartment, resulting in assembly of the NLRP3 inflammasome (Sander et al., 2011). Intake of L. plantarum CECT 7315/7316 downregulates expression of Nlrp3 in the ileum of rats (Vilahur et al., 2015). However, the NLRP3 inflammasome is also activated by L. rhamnosus GG and LC705 originating from dairy sources (Miettinen et al., 2012).
In a mouse immune hepatitis model, lactate treatment was shown to attenuate hepatic and pancreatic injury by negatively regulating TLR4-mediated activation of the NLRP3 inflammasome and production of IL-1β through arrestin β2 and G-protein-coupled receptor 81 (Hoque et al., 2014). In the present study, there was a higher lactate content in the supernatants of cells incubated with L. rhamnosus GR-1. However, additional lactate treatment did not attenuate the E. coli-induced increase in expression of NLRP3. This indicates that the elevated lactate content in the supernatant is a secondary effect of L. rhamnosus GR-1 treatment and cannot account for attenuation of E. coli-induced activation of NLRP3. Previously, we have shown that L. rhamnosus GR-1 attenuates E. coli-induced TLR4 expression in bovine mammary epithelial cells (Wu et al., 2016). This may contribute to attenuating the priming step and subsequent activation of NLRP3 through TLR4-mediated NF-κB signaling (Bauernfeind et al., 2009). The mitogen-activated protein kinase (MAPK) subfamilies, c-Jun N-terminal kinase (JNK) and extracellular regulated protein kinase (ERK) are essential for NLRP3 inflammasome activation and addition of JNK1/2 inhibitor SP600125 or upstream MAPK/ERK kinase inhibitor PD98059 of ERK inhibits the LPS-induced increase in NLRP3 protein expression (Liao et al., 2013). Culture medium of L. rhamnosus GR-1 inhibits LPS-induced JNK activation in macrophages or monocytic THP-1 cells (Kim et al., 2006). Histamine derived from Lactobacillus reuteri 6475 inhibits activation of ERK in THP-1 cells (Thomas et al., 2012). It is possible that L. rhamnosus GR-1 attenuates E. coli-induced NLRP3 activation through reducing the adhesion of E. coli to MAC-T cells and subsequently negatively regulating the functional synergy between NF-κB and JNK/ERK MAPK pathways mediated by some uncertain soluble factors. Further studies are required to determine active components derived from L. rhamnosus GR-1 and elucidate possible mechanisms underlying the antagonistic effects of L. rhamnosus GR-1 on NLRP3 activation during E. coli infection.
NLRP3 contains only a PYD, which engages the PYD of ASC, leaving the CARD of ASC to interact with the CARD-containing region of pro-caspase-1. Caspase-1 is thought to be activated by a proximity-induced dimerization and autoproteolytic process in the NLRP3/ASC complex platform (Shi, 2004). Active caspase-1 cleaves pro-IL-lβ and pro-IL-18 into mature IL-lβ and IL-18, which are essential for coordination of immune responses to pathogen infection through allograft neutrophil sequestration, mononuclear phagocyte recruitment, and T-cell activation (Samuel Weigt et al., 2017). In the present study, L. rhamnosus GR-1 attenuated E. coli-induced caspase-1 autoproteolysis and elevated production of mature IL-lβ and IL-18 at 6 h after challenge. Another study also showed that the NLRP3 inflammasome pathway plays a critical role in the host immune response to pathogen infection (Dikshit et al., 2018). However, inappropriate activation of the NLRP3 inflammasome is linked not only to local inflammation but also several autoimmune inflammatory disorders in humans (Seo et al., 2015). Indeed, activation of the NLRP3 inflammasome amplifies inflammation and promotes pathogen infection via a process involving triggering of T helper 2-biased adaptive immune responses (Gurung et al., 2015) or secretion of secondary danger-associated molecular pattern molecules (Bui et al., 2016). Our data suggest that L. rhamnosus GR-1 prevents E. coli-induced inflammation by suppressing activation of ASC-dependent NLRP3 inflammasomes.
In mice, non-canonical caspase-11 was identified as a key regulator of NLRP3 inflammasome-associated caspase-1 activation in response to E. coli infection. Caspase-11 is activated via NLRP3-independent mechanisms, but it is essential for NLRP3-dependent and ASC-dependent caspase-1 processing and IL-1β maturation in response to E. coli infection (Kayagaki et al., 2011). In addition, binding of LPS to human caspase-4 or murine caspase-11 via the CARD directly induces cell pyroptosis, independently of NLRP3 and ASC (Shi et al., 2014). A recent study revealed that outer-membrane-vesicle-mediated cytoplasmic delivery of extracellular E. coli LPS activates murine caspase-11 to induce pyroptosis and IL-1β maturation (Vanaja et al., 2016). Bovine caspase-4 is a homolog of human caspase-4 and mouse caspase-11 and plays a role in the processing of IL-1β and IL-18 precursors (Koenig et al., 2001; Martinon and Tschopp, 2004). In the present study, caspase-4 was activated by E. coli at 6 h; however, L. rhamnosus GR-1 pretreatment attenuated this activation.
Interestingly, there was a lower number of adherent E. coli in ASC-/- MAC-T cells at 3 h after E. coli challenge compared with WT MAC-T cells. It must be noted that ASC deficiency reduced, but did not abolish, caspase-1 processing, IL-1β and IL-18 maturation, and cell pyroptosis during E. coli infection. Decreased E. coli adhesion may delay the expression of NLRC4 receptor and the downstream activation of caspase-1, maturation of proinflammatory cytokines and cell pyroptosis in ASC-/- MAC-T cells. Caspase-1 activation by E. coli requires NLRP3 and ASC, but caspase-11 processing and cell pyroptosis do not (Kayagaki et al., 2011). A previous study reported weaker oligomerization of both ASC and caspase-1 in macrophages infected with E. coli compared with the canonical NLRP3 inflammasome activator nigericin, but with comparable production of IL-1β (Rathinam et al., 2012). Caspase-11 interacts with caspase-1 in infected cells, forming a heterodimeric complex (Kayagaki et al., 2011). These data suggest that caspase-4/-11 amplify caspase-1 activation independently of ASC by enabling caspase-1 autoprocessing through heterodimerization. Indeed, in the present study, caspase-4 activation was enhanced in ASC-/- cells compared with WT cells, which could have compensated for the loss of caspase-1 activation due to ASC-dependent NLRP3 inflammasome activation. Our findings indicate that E. coli infection activates caspase-4, subsequently resulting in cell pyroptosis and maturation of IL-1β and IL-18 via an NLRP3 inflammasome-dependent and ASC-independent pathway. Lactobacillus rhamnosus GR-1 suppresses ASC-dependent NLRP3 inflammasome activation and ASC-independent caspase-1 processing by inhibiting caspase-4 activation, thereby attenuating cell pyroptosis and cytokine production and thus preventing establishment of E. coli infection.
In contrast to NLRP3 activation in response to diverse stimuli, upon E. coli infection, NLRC4 responds to bacterial rod protein of the T3SS apparatus and flagellin (Zhao et al., 2011). We found that L. rhamnosus GR-1 inhibited E. coli-induced NLRC4 expression, as was also observed in ASC-/- cells. NLRC4 contains a CARD motif through which it directly interacts with caspase-1 to induce pyroptosis, independently of ASC. This NLRC4-dependent/ASC-independent cell death pathway proceeds in the absence of caspase-1 autoproteolysis. Interestingly, we observed weaker staining for caspase-1 in ASC-/- cells. Caspase-1 autoproteolysis is often used as an indicator of caspase-1 activation. However, it was also reported that uncleaved caspase-1 is enzymatically active in ASC-/- cells and can induce pyroptosis. In contrast to the formation of a single large ASC/caspase-1 focus for efficient IL-lβ and IL-18 processing, pro-caspase-1 could be recruited to NLRC4, with which it forms a smaller complex that induces pyroptosis (Broz et al., 2010b). Although NLRC4 contains a CARD, ASC amplifies NLRC4 inflammasome activity because ASC is essential for NLRC4-induced caspase-1 autoprocessing and maturation of IL-lβ and IL-18 (Brubaker et al., 2015). Our data suggest that L. rhamnosus GR-1 inhibits E. coli-induced cell pyroptosis via suppression of ASC-independent NLRC4 inflammasome activation. During E. coli infection in the present study, L. rhamnosus GR-1 decreased the secretion of IL-lβ and IL-18, in part due to suppression of ASC-dependent NLRC4 inflammasome activation.
This MAC-T cell model of E. coli and L. rhamnosus GR-1 co-incubation presents an in vitro framework for assessing bovine mammary immune response to pathogen infection, and evaluating the efficiency of Lactobacillus-based intervention in preventing bovine mastitis. The results require further confirmation in other cell lines and in vivo studies. However, several concerns need to be addressed before the clinical application of Lactobacillus in bovine mastitis. Oral ingestion of probiotics promotes mucosal immune response to pathogen infection in the gut. The mechanism underlying how the immunomodulatory effect extends to the mammary glands remains unclear. The means of probiotic supplementation (e.g., mixed into the feed, oral capsules or intra-mammary infection) and dose effect also needs to be studied in more details. The molecular mechanism underlying regulation of inflammasome activity by Lactobacillus requires further investigation. Our findings identify NLRP3 and NLRC4 inflammasomes as potential targets for bovine mastitis therapy and could strengthen the development for other inflammasome-targeted therapies in E. coli-associated mastitis.
Conclusion
In conclusion, our findings suggest that L. rhamnosus GR-1 ameliorates E. coli-induced inflammatory damage by attenuating both ASC-dependent and ASC-independent inflammasome activation in MAC-T cells (Figure 9). L. rhamnosus GR-1 inhibits activation of ASC-dependent NLRP3 and NLRC4 inflammasome activation and production of the downstream proinflammatory cytokines IL-lβ and IL-18 during E. coli infection. In addition, L. rhamnosus GR-1 suppresses E. coli-induced cell pyroptosis, in part through attenuation of NLRC4 inflammasome activation, independently of ASC. Furthermore, L. rhamnosus GR-1 inhibits non-canonical caspase-4 activation, which subsequently synergizes with NLRP3-/ASC-dependent caspase-1 activation to potentially inhibit ASC-independent caspase-1 activation, thus suppressing cell pyroptosis and IL-lβ and IL-18 production.
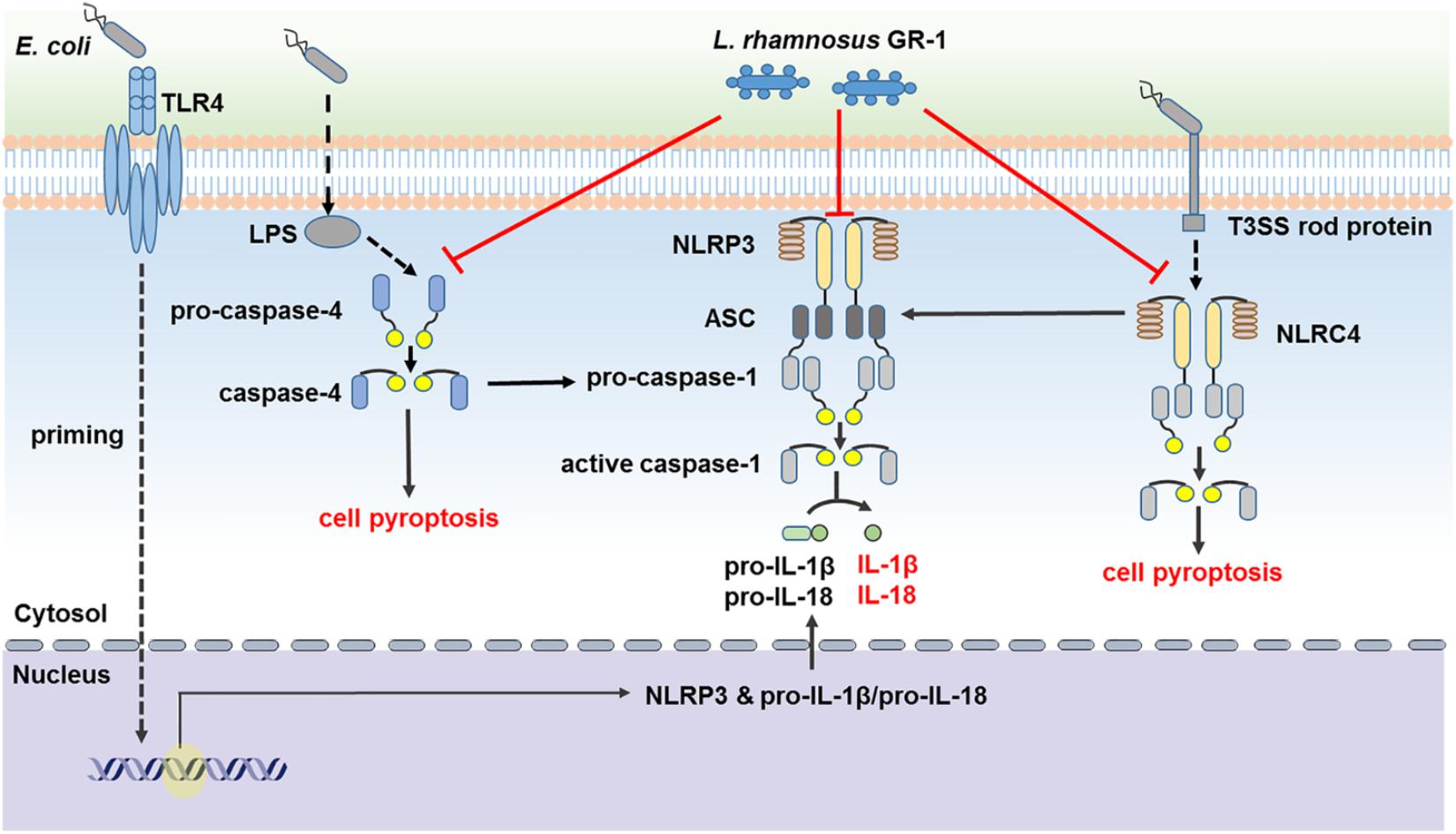
FIGURE 9. Lactobacillus rhamnosus GR-1 attenuated activation of ASC-dependent and ASC-independent inflammasomes during E. coli infection. L. rhamnosus GR-1 ameliorates E. coli-induced inflammatory damage via attenuation of both ASC-dependent and ASC-independent inflammasome activation in MAC-T cells. L. rhamnosus GR-1 inhibits activation of ASC-dependent NLRP3 and NLRC4 inflammasomes and production of the downstream proinflammatory cytokines IL-lβ and IL-18 during E. coli infection. L. rhamnosus GR-1 suppresses E. coli-induced cell pyroptosis, in part through attenuation of NLRC4 inflammasome activation, independently of ASC. In addition, L. rhamnosus GR-1 inhibits non-canonical caspase-4 activation, which subsequently synergizes with the NLRP3 inflammasome to attenuate caspase-1 activation and potentially inhibit caspase-1-independent cell pyroptosis and IL-lβ and IL-18 production. Full lines represent the results of the present study, and dashed lines represent the conclusions drawn in other studies.
Author Contributions
QW, Y-HZ, and J-FW conceived and designed the experiments, analyzed the data, and wrote the manuscript. QW, JX, XL, CD, and M-JW performed the experiments.
Funding
This work was supported by the National Key R&D Program of China (Project No. 2017YFD0502200), the National Natural Science Foundation of China (Project Nos. 31472242 and 31672613), and J-FW received funding from the program for the Beijing Dairy Industry Innovation Team.
Conflict of Interest Statement
The authors declare that the research was conducted in the absence of any commercial or financial relationships that could be construed as a potential conflict of interest.
Acknowledgments
We would like to thank Professor Sen Wu for the pCRISPR-sg5 plasmid and Dr. Ying Yu for providing MAC-T cells.
Supplementary Material
The Supplementary Material for this article can be found online at: https://www.frontiersin.org/articles/10.3389/fmicb.2018.01661/full#supplementary-material
FIGURE S1 | Immunodetection of cytokeratin-18 in MAC-T cells. Representative confocal immunocytochemistry images showing typical morphology of MAC-T cells. MAC-T cells were immunostained for cytokeratin-18 (red). DAPI (blue) was used to localize nuclei. Scale bar, 20 μm. Data are representative of three independent experiments.
FIGURE S2 | The lactate content in the cell supernatants and the effect of lactate on NLRP3 expression during E. coli infection. Lactate content in the supernatants was determined (A). Cells were also simultaneously treated with lactate (LACT) at a concentration of 0.6 g/L (equivalent to 7 mM) and E. coli at a MOI of 100:1. Western blot detection of NLRP3 in WT and ASC-/- cells collected from the indicated cell cultures at 6 h after E. coli challenge (B). Representative panels showing expression of NLRP3 protein (Left). NLRP3 band intensity was determined using Quantity One software. Results are presented as the ratio of NLRP3 band intensity to that of GAPDH (Right). Data are presented as the mean ± SEM of three independent experiments. ∗P < 0.05, ∗∗P < 0.01, ∗∗∗P < 0.001.
References
Afonina, I. S., Zhong, Z., Karin, M., and Beyaert, R. (2017). Limiting inflammation-the negative regulation of NF-kappaB and the NLRP3 inflammasome. Nat. Immunol. 18, 861–869. doi: 10.1038/ni.3772
Ardita, C. S., Mercante, J. W., Kwon, Y. M., Luo, L., Crawford, M. E., Powell, D. N., et al. (2014). Epithelial adhesion mediated by pilin SpaC is required for Lactobacillus rhamnosus GG-induced cellular responses. Appl. Environ. Microbiol. 80, 5068–5077. doi: 10.1128/AEM.01039-14
Bauernfeind, F. G., Horvath, G., Stutz, A., Alnemri, E. S., MacDonald, K., Speert, D., et al. (2009). NF-kB activating pattern recognition and cytokine receptors license NLRP3 inflammasome activation by regulating NLRP3 expression. J. Immunol. 183, 787–791. doi: 10.4049/jimmunol.0901363
Broz, P., Newton, K., Lamkanfi, M., Mariathasan, S., Dixit, V. M., and Monack, D. M. (2010a). Redundant roles for inflammasome receptors NLRP3 and NLRC4 in host defense against Salmonella. J. Exp. Med. 207, 1745–1755. doi: 10.1084/jem.20100257
Broz, P., von Moltke, J., Jones, J. W., Vance, R. E., and Monack, D. M. (2010b). Differential requirement for caspase-1 autoproteolysis in pathogen-induced cell death and cytokine processing. Cell Host Microbe 8, 471–483. doi: 10.1016/j.chom.2010.11.007
Brubaker, S. W., Bonham, K. S., Zanoni, I., and Kagan, J. C. (2015). Innate immune pattern recognition: a cell biological perspective. Annu. Rev. Immunol. 33, 257–290. doi: 10.1146/annurev-immunol-032414-112240
Bui, F. Q., Johnson, L., Roberts, J., Hung, S. C., Lee, J., Atanasova, K. R., et al. (2016). Fusobacterium nucleatum infection of gingival epithelial cells leads to NLRP3 inflammasome-dependent secretion of IL-1beta and the danger signals ASC and HMGB1. Cell Microbiol. 18, 970–981. doi: 10.1111/cmi.12560
Dikshit, N., Kale, S. D., Khameneh, H. J., Balamuralidhar, V., Tang, C. Y., Kumar, P., et al. (2018). NLRP3 inflammasome pathway has a critical role in the host immunity against clinically relevant Acinetobacter baumannii pulmonary infection. Mucosal Immunol. 11, 257–272. doi: 10.1038/mi.2017.50
Franchi, L., Kamada, N., Nakamura, Y., Burberry, A., Kuffa, P., Suzuki, S., et al. (2012). NLRC4-driven production of IL-1beta discriminates between pathogenic and commensal bacteria and promotes host intestinal defense. Nat. Immunol. 13, 449–456. doi: 10.1038/ni.2263
Gudina, E. J., Fernandes, E. C., Teixeira, J. A., and Rodrigues, L. R. (2015). Antimicrobial and anti-adhesive activities of cell-bound biosurfactant from Lactobacillus agilis CCUG31450. RSC Adv. 5, 90960–90968. doi: 10.1039/C5RA11659G
Gueya, B., Bodnara, M., Maniéa, S. N., Tardivele, A., and Petrilli, V. (2014). Caspase-1 autoproteolysis is differentially required for NLRP1b and NLRP3 inflammasome function. Proc. Natl. Acad. Sci. U.S.A. 111, 17254–17259. doi: 10.1073/pnas.1415756111
Guo, H., Callaway, J. B., and Ting, J. P. (2015). Inflammasomes: mechanism of action, role in disease, and therapeutics. Nat. Med. 21, 677–687. doi: 10.1038/nm.3893
Gurung, P., Karki, R., Vogel, P., Watanabe, M., Bix, M., Lamkanfi, M., et al. (2015). An NLRP3 inflammasome-triggered Th2-biased adaptive immune response promotes leishmaniasis. J. Clin. Invest. 125, 1329–1338. doi: 10.1172/JCI79526
Hoque, R., Farooq, A., Ghani, A., Gorelick, F., and Mehal, W. Z. (2014). Lactate reduces liver and pancreatic injury in Toll-like receptor- and inflammasome-mediated inflammation via GPR81-mediated suppression of innate immunity. Gastroenterology 146, 1763–1774. doi: 10.1053/j.gastro.2014.03.014
Huynh, H. T., Robitaille, G., and Turner, J. D. (1991). Establishment of bovine mammary epithelial cells (MAC-T): an in vitro model for bovine lactation. Exp. Cell Res. 197, 191–199. doi: 10.1016/0014-4827(91)90422-Q
Jo, E. K., Kim, J. K., Shin, D. M., and Sasakawa, C. (2016). Molecular mechanisms regulating NLRP3 inflammasome activation. Cell. Mol. Immunol. 13, 148–159. doi: 10.1038/cmi.2015.95
Jorgensen, I., Lopez, J. P., Laufer, S. A., and Miao, E. A. (2016). IL-1beta, IL-18, and eicosanoids promote neutrophil recruitment to pore-induced intracellular traps following pyroptosis. Eur. J. Immunol. 46, 2761–2766. doi: 10.1002/eji.201646647
Kayagaki, N., Warming, S., Lamkanfi, M., Vande Walle, L., Louie, S., Dong, J., et al. (2011). Non-canonical inflammasome activation targets caspase-11. Nature 479, 117–121. doi: 10.1038/nature10558
Kim, S. O., Sheikh, H. I., Ha, S., Martins, A., and Reid, G. (2006). G-CSF-mediated inhibition of JNK is a key mechanism for Lactobacillus rhamnosus-induced suppression of TNF production in macrophages. Cell. Microbiol. 8, 1958–1971. doi: 10.1111/j.1462-5822.2006.00763.x
Koenig, U., Eckhart, L., and Tschachler, E. (2001). Evidence that caspase-13 is not a human but a bovine gene. Biochem. Biophys. Res. Commun. 285, 1150–1154. doi: 10.1006/bbrc.2001.5315
Lamkanfi, M., and Dixit, V. M. (2014). Mechanisms and functions of inflammasomes. Cell 157, 1013–1022. doi: 10.1016/j.cell.2014.04.007
Lannitti, R. G., Napolioni, V., Oikonomou, V., De Luca, A., Galosi, C., Pariano, M., et al. (2016). IL-1 receptor antagonist ameliorates inflammasome-dependent inflammation in murine and human cystic fibrosis. Nat. Commun. 7:10791. doi: 10.1038/ncomms10791
Liao, P. C., Chao, L. K., Chou, J. C., Dong, W. C., Lin, C. N., Lin, C. Y., et al. (2013). Lipopolysaccharide/adenosine triphosphate-mediated signal transduction in the regulation of NLRP3 protein expression and caspase-1-mediated interleukin-1β secretion. Inflamm. Res. 62, 89–96. doi: 10.1007/s00011-012-0555-2
Martinon, F., and Tschopp, J. (2004). Inflammatory caspases: linking an intracellular innate immune system to autoinflammatory diseases. Cell 117, 561–574. doi: 10.1016/j.cell.2004.05.004
Miao, E. A., Mao, D. P., Yudkovsky, N., Bonneau, R., Lorang, C. G., Warren, S. E., et al. (2010). Innate immune detection of the type III secretion apparatus through the NLRC4 inflammasome. Proc. Natl. Acad. Sci. U.S.A. 107, 3076–3080. doi: 10.1073/pnas.0913087107
Miettinen, M., Pietila, T. E., Kekkonen, R. A., Kankainen, M., Latvala, S., Pirhonen, J., et al. (2012). Nonpathogenic Lactobacillus rhamnosus activates the inflammasome and antiviral responses in human macrophages. Gut Microbes 3, 510–522. doi: 10.4161/gmic.21736
Rathinam, V. A., Vanaja, S. K., Waggoner, L., Sokolovska, A., Becker, C., Stuart, L. M., et al. (2012). TRIF licenses caspase-11-dependent NLRP3 inflammasome activation by gram-negative bacteria. Cell 150, 606–619. doi: 10.1016/j.cell.2012.07.007
Samuel Weigt, S., Palchevskiy, V., and Belperio, J. A. (2017). Inflammasomes and IL-1 biology in the pathogenesis of allograft dysfunction. J. Clin. Invest. 127, 2022–2029. doi: 10.1172/JCI93537
Sander, L. E., Davis, M. J., Boekschoten, M. V., Amsen, D., Dascher, C. C., Ryffel, B., et al. (2011). Detection of prokaryotic mRNA signifies microbial viability and promotes immunity. Nature 474, 385–389. doi: 10.1038/nature10072
Seo, S. U., Kamada, N., Munoz-Planillo, R., Kim, Y. G., Kim, D., Koizumi, Y., et al. (2015). Distinct commensals induce interleukin-1beta via NLRP3 inflammasome in inflammatory monocytes to promote intestinal inflammation in response to injury. Immunity 42, 744–755. doi: 10.1016/j.immuni.2015.03.004
Shaheen, M., Tantary, H. A., and Nabi, S. U. (2015). A treatise on bovine mastitis: disease and disease economics, etiological basis, risk factors, impact on human health, therapeutic management, prevention and control strategy. Adv. Dairy Res. 4:150.
Shi, J., Zhao, Y., Wang, Y., Gao, W., Ding, J., Li, P., et al. (2014). Inflammatory caspases are innate immune receptors for intracellular LPS. Nature 514, 187–192. doi: 10.1038/nature13683
Shi, Y. (2004). Caspase activation: revisiting the induced proximity model. Cell 117, 855–858. doi: 10.1016/j.cell.2004.06.007
Thacker, J. D., Balin, B. J., Appelt, D. M., Sassi-Gaha, S., Purohit, M., Rest, R. F., et al. (2012). NLRP3 inflammasome is a target for development of broad-spectrum anti-infective drugs. Antimicrob. Agents Chemother. 56, 1921–1930. doi: 10.1128/AAC.06372-11
Thomas, C. M., Hong, T., Peter van Pijkeren, J., Hemarajata, P., Trinh, D. V., Hu, W., et al. (2012). Histamine derived from probiotic Lactobacillus reuteri suppresses TNF via modulation of PKA and ERK signaling. PLoS One 7:e31951. doi: 10.1371/journal.pone.0031951
Tytgat, H. L. P., Douillard, F. P., Reunanen, J., Rasinkangas, P., Hendrickx, A. P., Laine, P. K., et al. (2016). Lactobacillus rhamnosus GG outcompetes Enterococcus faecium via mucus-binding pili: evidence for a novel and heterospecific probiotic mechanism. Appl. Environ. Microbiol. 82, 5756–5762. doi: 10.1128/AEM.01243-16
Vanaja, S. K., Russo, A. J., Behl, B., Banerjee, I., Yankova, M., Deshmukh, S. D., et al. (2016). Bacterial outer membrane vesicles mediate cytosolic localization of LPS and caspase-11 activation. Cell 165, 1106–1119. doi: 10.1016/j.cell.2016.04.015
Vilahur, G., Lopez-Bernal, S., Camino, S., Mendieta, G., Padro, T., and Badimon, L. (2015). Lactobacillus plantarum CECT 7315/7316 intake modulates the acute and chronic innate inflammatory response. Eur. J. Nutr. 54, 1161–1171. doi: 10.1007/s00394-014-0794-9
Wu, Q., Liu, M. C., Yang, J., Wang, J. F., and Zhu, Y. H. (2016). Lactobacillus rhamnosus GR-1 ameliorates Escherichia coli-induced inflammation and cell damage via attenuation of ASC-independent NLRP3 inflammasome activation. Appl. Environ. Microbiol. 82, 1173–1182. doi: 10.1128/AEM.03044-15
Keywords: bovine mammary epithelial cell, Lactobacillus rhamnosus, Escherichia coli, inflammasome, ASC
Citation: Wu Q, Zhu Y-H, Xu J, Liu X, Duan C, Wang M-J and Wang J-F (2018) Lactobacillus rhamnosus GR-1 Ameliorates Escherichia coli-Induced Activation of NLRP3 and NLRC4 Inflammasomes With Differential Requirement for ASC. Front. Microbiol. 9:1661. doi: 10.3389/fmicb.2018.01661
Received: 27 February 2018; Accepted: 04 July 2018;
Published: 24 July 2018.
Edited by:
Sanna Sillankorva, University of Minho, PortugalReviewed by:
Atte Von Wright, University of Eastern Finland, FinlandRebecca Leigh Schmidt, Upper Iowa University, United States
Copyright © 2018 Wu, Zhu, Xu, Liu, Duan, Wang and Wang. This is an open-access article distributed under the terms of the Creative Commons Attribution License (CC BY). The use, distribution or reproduction in other forums is permitted, provided the original author(s) and the copyright owner(s) are credited and that the original publication in this journal is cited, in accordance with accepted academic practice. No use, distribution or reproduction is permitted which does not comply with these terms.
*Correspondence: Jiu-Feng Wang, aml1ZmVuZ193YW5nQGhvdG1haWwuY29t
†These authors have contributed equally to this work.