- Chair of Genetics of Prokaryotes, Faculty of Biology and CeBiTec, Bielefeld University, Bielefeld, Germany
Corynebacterium glutamicum is used for the million-ton-scale production of food and feed amino acids such as L-glutamate and L-lysine and has been engineered for production of carotenoids such as lycopene. These fermentation processes are based on sugars present in molasses and starch hydrolysates. Due to competing uses of starch and sugars in human nutrition, this bacterium has been engineered for utilization of alternative feedstocks, for example, pentose sugars present in lignocellulosic and hexosamines such as glucosamine (GlcN) and N-acetyl-D-glucosamine (GlcNAc). This study describes strain engineering and fermentation using N-acetyl-D-muramic acid (MurNAc) as non-food-competing feedstock. To this end, the genes encoding the MurNAc-specific PTS subunits MurP and Crr and the etherase MurQ from Escherichia coli K-12 were expressed in C. glutamicumΔnanR. While MurP and MurQ were required to allow growth of C. glutamicumΔnanR with MurNAc, heterologous Crr was not, but it increased the growth rate in MurNAc minimal medium from 0.15 h-1 to 0.20 h-1. When in addition to murP-murQ-crr the GlcNAc-specific PTS gene nagE from C. glycinophilum was expressed in C. glutamicumΔnanR, the resulting strain could utilize blends of GlcNAc and MurNAc. Fermentative production of the amino acids L-glutamate and L-lysine, the carotenoid lycopene, and the L-lysine derived chemicals 1,5-diaminopentane and L-pipecolic acid either from MurNAc alone or from MurNAc-GlcNAc blends was shown. MurNAc and GlcNAc are the major components of the bacterial cell wall and bacterial biomass is an underutilized side product of large-scale bacterial production of organic acids, amino acids or enzymes. The proof-of-concept for valorization of MurNAc reached here has potential for biorefinery applications to convert non-food-competing feedstocks or side-streams to valuable products such as food and feed additives.
Introduction
Corynebacterium glutamicum is a predominantly aerobic, rod-shaped, Gram-positive soil bacterium which is generally recognized as safe (GRAS). Since the 1960s, C. glutamicum was first used for the production of the flavor enhancer (Kinoshita et al., 1957) under biotin limiting conditions (Shiio et al., 1962). C. glutamicum was developed into an important organism for the biotechnological industry, producing amino acids on a million-ton scale (Wendisch, 2014). C. glutamicum has also been engineered to produce diamines, organic acids, carotenoids, proteins and biopolymers (Wendisch et al., 2016). Recently, metabolic engineering of C. glutamicum to expand its substrate scope allowed to use alternative carbon sources that do not have competing uses in the food industry (Zahoor et al., 2012). Access to the hexosamines GlcN (Uhde et al., 2013) and GlcNAc (Matano et al., 2014) has been reported, but utilization of the hexosamine MurNAc as alternative carbon source by C. glutamicum has not been described (Dominguez et al., 1997; Cramer and Eikmanns, 2007).
GlcN and GlcNAc can be gained by hydrolysis of chitin and chitosan that make up the arthropod exoskeleton and are present in fungal cell walls. Every year, circa 100 billion tons of chitin are produced in Nature and GlcNAc and GlcN can be obtained by acid hydrolysis (Chen et al., 2010; Zhang and Yan, 2017) and are available, e.g., from shrimp shell waste, an abundant side stream of the fishery industry. MurNAc and GlcNAc are the hexosamine constituents of peptidoglycan which makes up about 5% of the cell mass of Gram-negative bacteria and up to 20% of the cell mass of Gram-positive bacteria (Munoz et al., 1967; Reith and Mayer, 2011). The peptidoglycan constituents that can be found in all bacterial habitats have been used as indicators of bacterial biomass content in soils (Domsch, 1982). The Gram-positive C. glutamicum is the main producing organism for the annual production of 5 million tons of amino acids (Wendisch et al., 2016). Under the assumptions that (a) the same amount of cell dry weight is produced, (b) 20% of the cell dry weight is peptidoglycan and (c) about half of peptidoglycan is GlcNAc and MurNAc, about 500.000 tons of MurNAc and GlcNAc would be available from the amino acid fermentation industry. Biotechnological processes with bacterial hosts are used at the million-ton scale to produce secreted compounds such as organic acids, amino acids and enzymes. The spent biomass may be used in waste-to-energy applications either by thermal (e.g., incineration), thermo-chemical (e.g., torrefaction) or by biochemical treatments (e.g., anaerobic digestion). However, it is desirable to make use of the carbon and nitrogen containing hexosamine fraction of peptidoglycan in food and feed fermentation processes. The hexosamines may function both as carbon and nitrogen source for bacterial fermentations.
In chemical hydrolysis, the hexosamine fraction of peptidoglycan is accessible via enzymes of bacterial cell wall recycling. The degradation of the own cell wall by autolytic enzymes as a part of ll recycling is a common pathway in bacteria. When an Escherichia coli lys dap mutant was labeled with [3H]diaminopimelate for two generations and then chased, about 45% of its cell wall peptidoglycan was recycled per generation (Goodell and Schwarz, 1985; Uehara et al., 2006; Uehara and Park, 2008). The Gram-positive Bacillus subtilis can degrade, uptake and metabolize the cell wall component MurNAc in the stationary phase (Borisova et al., 2016). While all bacteria require cell wall peptidoglycan remodeling during growth and cell division, not all can utilize the monomeric components as carbon or nitrogen sources for growth. C. glutamicum may possess a minimal set of autolytic enzymes, however, many orthologs of the peptidoglycan degradation machinery from E. coli are absent (Dahl et al., 2004; Reith and Mayer, 2011). Catabolism of MurNAc in E. coli involves uptake and phosphorylation of MurNAc and GlcNAc via the phosphoenolpyruvate dependent phosphotransferase system (PTS). The MurNAc-specific PTS subunits are MurP and Crr (Figure 1). MurP, a two-domain protein that lacks a PTS-EIIA domain, is phosphorylated by EIIAGlc, a kinase encoded by the crr gene (carbohydrate repression resistance), which interacts with several members of the glucose PTS family (Nuoffer et al., 1988; Tchieu et al., 2001; Dahl et al., 2004). MurNAc-6-phosphate is further catabolized by the etherase MurQ (Figure 1) that cleaves the lactyl ether bond yielding GlcNAc-6-phosphate and D-lactate (Jaeger et al., 2005; Hadi et al., 2008). GlcNAc and GlcN are also taken up via the PTS with the specific subunits NagE (Plumbridge, 2009) and PTSMan (Curtis and Epstein, 1975). NagA deacetylates GlcNAc-6-phosphate to GlcN-6-phosphate which is deaminated by NagB to the glycolytic intermediate fructose-6-phosphate (Figure 1).
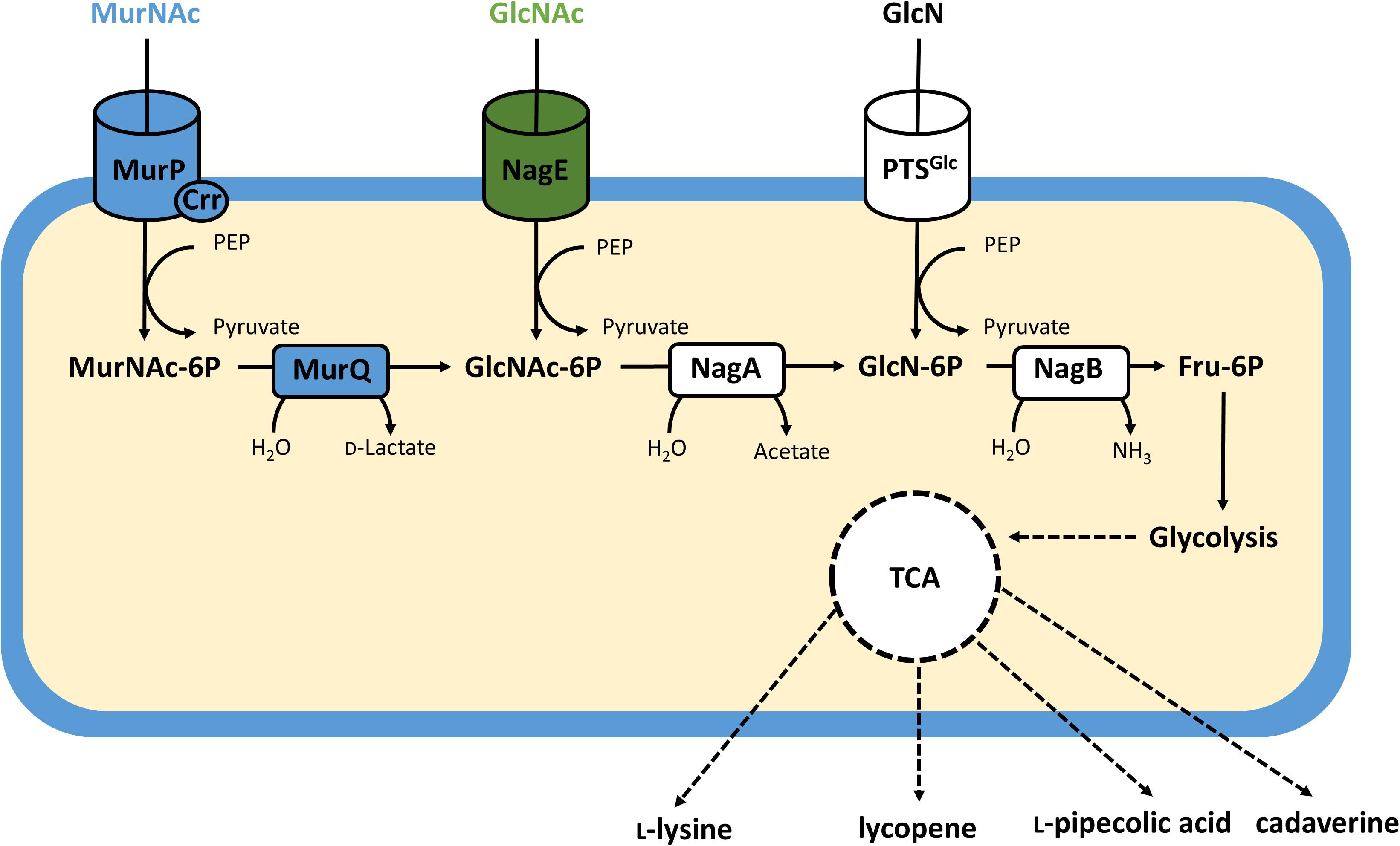
FIGURE 1. Schematic representation of the amino sugar catabolic pathways in recombinant C. glutamicum. Reactions from E. coli are given in blue, those from C. glycinophilum in green and native reactions are given in white with cylinders representing uptake systems and boxes representing enzyme reactions. Bold lines indicate individual reactions, dashed lines indicate multi-reaction conversions. MurP, MurNAc specific EIIBC PTS component; Crr, glucose-family specific PTS EIIA component; NagE, GlcNAc specific EIICBA PTS component; NagA, GlcNAc -6-phosphate deacetylase; NagB, GlcN-6-phosphate deaminase.
Corynebacterium glutamicum is able to take up GlcN (Figure 1) by using its glucose specific PTS PtsG (Arndt and Eikmanns, 2008; Uhde et al., 2013). Efficient growth with GlcN required high levels of the endogenous NagB, e.g., in the absence of the repressor protein NanR (Matano et al., 2016). By contrast, high levels of NagA and NagB were not sufficient to support growth with GlcNAc unless nagE from C. glycinophilum was expressed heterologously (Matano et al., 2014). Recombinant C. glutamicum strains carrying a nanR deletion and expressing nagE from C. glycinophilum produced several value-added products from GlcN or GlcNAc.
Here, C. glutamicum strains were developed for utilization of MurNAc as carbon, nitrogen and energy source and for MurNAc-based production of the food amino acid L-glutamate, the feed amino acid L-lysine, L-lysine-derived chemicals as well as the carotenoid lycopene.
Materials and Methods
Bacterial Strains and Growth Conditions
The strains and plasmids used in this work are listed in Table 1. Pre-cultivation of C. glutamicum strains was carried out at 30°C in baffled shake flasks using BHI supplemented with 45.5 g/L D-sorbitol. E. coli was grown at 37°C in LB (Lysogeny Broth) medium. Kanamycin (50 μg/mL), chloramphenicol (4.5 and 25 μg/mL for C. glutamicum and E. coli, respectively) or tetracycline (5 μg/mL) were added, if necessary. To adjust both cultures to growth conditions in the Biolector® system (m2pLabs, Baesweiler), precultures were washed after 24 h with TN-buffer and transferred to CGXII medium (Eggeling and Bott, 2005) with 100 mM GlcN and antibiotics, if necessary. After 24 h, these were transferred to 1 mL cultures in the Biolector® system (1100 rpm) with CGXII medium containing and, if not otherwise stated, 25 mM MurNAc (BACHEM, Bubendorf, Switzerland) as sole carbon source or a combination of 25 mM MurNAc and 25 mM GlcNAc. To trigger glutamate production, penicillin G (10 μM) was added in the main culture. The initial OD600 was 1 and gene expression from plasmids pVWEx1 and pEC-XT99A was induced by addition of 25 μM IPTG, if not otherwise stated. Correlation factors for light scattering in the Biolector system, OD600 and biomass concentrations were determined.
Construction of Expression Vectors
Escherichia coli DH5α was used for cloning. Codon usage of murP, crr and murQ from E. coli for C. glutamicum was examined using the graphical codon usage analyzer1. The analysis showed, that the codon ATA occurred twice in the sequence of murP. This codon is rarely used in C. glutamicum and was changed to the more frequently used codon of ATC via site directed mutagenesis (SDM). The mutated variation of murP was called murPopt. The genes of murP, crr and murQ were amplified via PCR from genomic DNA of E. coli K-12, while nagE from C. glycinophilum, was amplified from pVWEx1_nagE (Matano et al., 2016). The primers used in this study (see Supplementary Table S1) were obtained from Metabion international AG, Planegg. Using Gibson assembly (Gibson, 2011), the vectors pVWEx1_murP, pVWEx1_murPopt, pVWEx1_murPcrr, pVWEx1_murPoptcrr, pCXE50_murQ and pEC-XT99A_nagE were constructed. The vectors pVWEx1 and pEC-XT99A are IPTG inducible while pCXE50 has a constitutive EFtu promotor. E. coli was transformed by the CaCl2 method while transformation through electroporation was applied for C. glutamicum at 2500 V, 25 μF and 200 Ω.
Carotenoid Extraction
Lycopene was extracted as described before (Heider et al., 2012). In short, 5 wells each containing 1 ml cell suspension were combined and pelleted in safe lock micro reaction tubes by centrifugation at 10,000 g for 15 min and resuspended in 800 μL of a 7:3 methanol/acetone mixture and incubated for 15 min at 60°C and 750 rpm in a thermomixer (Eppendorf). The cell debris was removed by centrifugation and the supernatant used for HPLC analysis. The procedure was repeated to ensure complete extraction until white pellets were obtained.
Quantitation of Fermentation Products
The quantification of MurNAc, GlcNAc, lycopene, L-glutamate, and L-lysine was conducted by HPLC analysis (1200 series HPLC system, Agilent Technologies Sales & Services GmbH & Co. KG, Waldbronn). The supernatant of 1 ml pelleted cell suspension was diluted and analyzed. For quantification of organic acids, the carbo column (300 × 8 mm, 10 μm particle size, 25 Å pore diameter, CS Chromatographie Service GmbH) and a refractive index detector (RID G1362A, 1200 series, Agilent Technologies) was used for quantification of MurNAc and GlcNAc with 5 mM H2SO4 as buffer.
Applying OPA derivatisation, L-lysine and L-glutamate were analyzed using an RP8 column with a sodium acetate (0.25 v/v %) buffer at pH 6 and a 1:50 dilution with an internal L-asparagine standard. Using the RP18 column with a Methanol-Milli-Q-water mixture (9:1), lycopene was quantified (Heider et al., 2012).
Results
Metabolic Engineering of C. glutamicum for Growth With MurNAc as Carbon Source
Corynebacterium glutamicum, which has been engineered to utilize GlcN and GlcNAc (Matano et al., 2014, 2016), cannot utilize MurNAc since no growth was observed in minimal medium with 25 mM MurNAc and 25 ± 0.1 mM MurNAc remained in the growth medium after 25 h of incubation (Figure 2A). As expected, the C. glutamicum genome lacks genes encoding a MurNAc PTS and MurNAc-6-phosphate etherase for uptake and conversion of MurNAc to GlcNAc-6-phosphate, an endogenous intermediate of C. glutamicum metabolism. As described in Section “Materials and Methods,” the genes for the MurNAc PTS murP from E. coli or codon optimized allele murPopt (Nuoffer et al., 1988; Tchieu et al., 2001; Dahl et al., 2004) were cloned into the IPTG-inducible plasmid pVWEx1 alone or as operon with crr. The gene for the MurNAc-6-phosphate etherase murQ from E. coli (Jaeger et al., 2005) was cloned into the constitutive expression vector pCXE50 (Lee, 2014). Functional expression of murQ from pCXE50_murQ was tested by complementation of the E. coli murQ mutant E. coli JW2421-1. While E. coli JW2421-1(pCXE50_murQ) utilized MurNAc as sole carbon source (ΔOD600 of 3.2 ± 0.1 and μmax of 0.07 ± 0.01 h-1), E. coli JW2421-1ΔmurQ showed no growth (see Supplementary Figure S1). Only murQ was tested by complementation of a E. coli mutant. We neither tested murP nor crr because we expected perturbations due to overexpression since MurP is a membrane protein and Crr serves a regulatory function. C. glutamicum ΔnanR was transformed with the constructed pVWEx1 plasmids and with the pCXE50_murQ. The respective strains were named C. glutamicum ΔnanR PQ, POQ, PCQ, POCQ (Table 1).
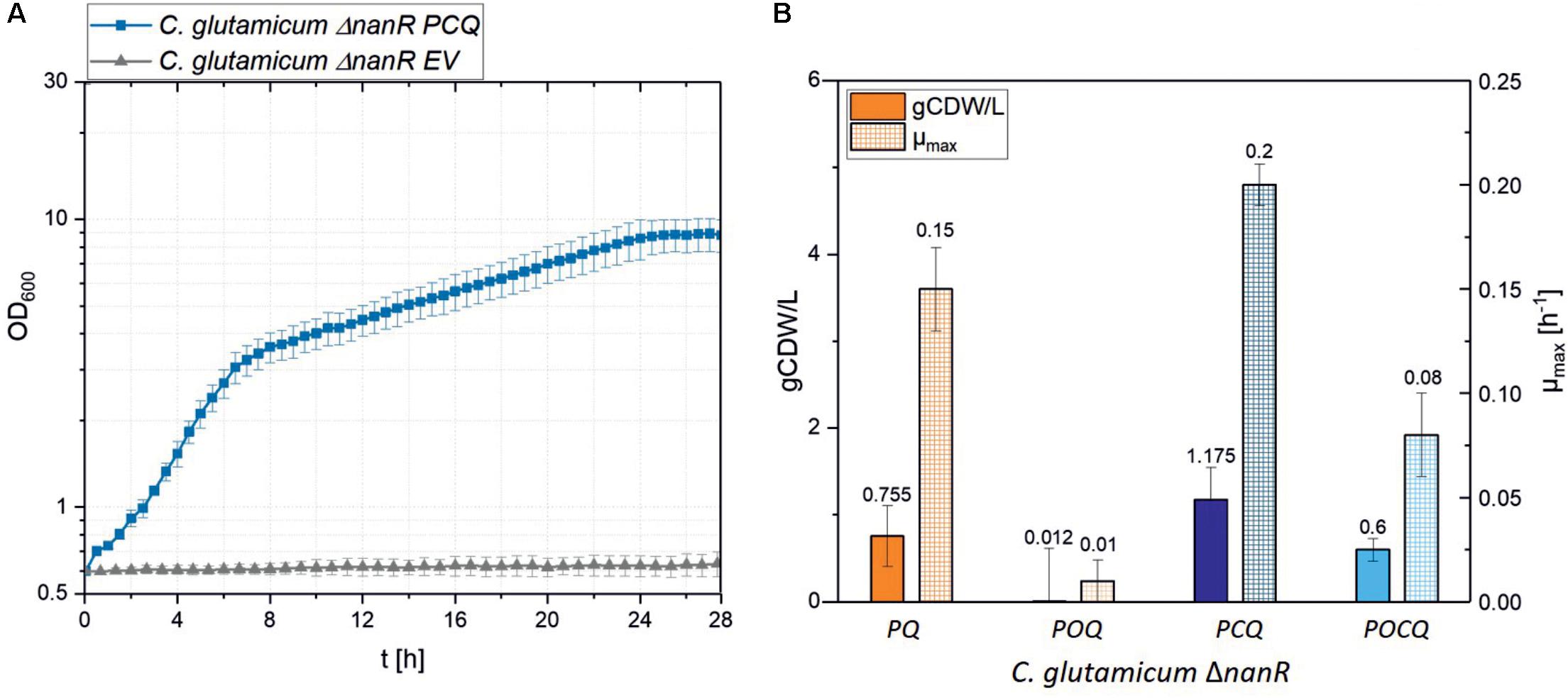
FIGURE 2. (A) Growth parameters of various C. glutamicum strains in MurNAc minimal medium. Growth of the strains C. glutamicum ΔnanR EV (empty vector) and C. glutamicum ΔnanR PCQ was monitored for 25 h at 30°C and 1100 rpm in the Biolector with CGXII medium containing IPTG 25 μM and 20 mM MurNAc. (B) Biomass concentrations (gCDW/L; filled) and maximal growth rates (μmax, checkered) of the strains PQ, POQ, PCQ and POCQ grown for 48 h in the Biolector® system in CGXII medium with MurNAc 20 mM. Values and error bars represent means and standard deviations of triplicates.
Strains expressing crr from E. coli grew faster in minimal medium containing 25 mM MurNAc as sole source of carbon and energy than strains lacking crr (Figure 2B). Strains with native murP grew better than strains expressing codon optimized murP (Figure 2B). IPTG was used at a low concentration (25 μM) to induce heterologous gene expression, since higher concentrations slowed growth (Table 2). This is not unexpected and presumably due to too high expression of transport protein genes as seen previously for dccT (Youn et al., 2008) and dctA (Youn et al., 2009), coding for dicarboxylate transporters. With 25 μM IPTG, strain ΔnanR PCQ expressing native murP, crr and murQ grew in minimal medium containing 25 mM MurNAc to a biomass concentration of 1.2 ± 0.3 gCDW/L and with 50 mM MurNAc to a biomass concentration of 2.0 ± 0.2 gCDW/L (Table 2). Biphasic exponential growth was observed: faster growth between 0 and 6 h and slower growth between 6 and 27 h (Figure 2A). The curves appear linear as the Y axis has been logarithmized (Figure 2). During the transition from the first to the second growth phase the medium contained 3.10 ± 0.12 mM lactate. Thus, lactate released by MurQ from MurNAc-6-phosphate may not have been utilized as fast as GlcNAc-6-phosphate, the other product of the MurQ reaction. In consequence, lactate accumulated in the culture medium in the first exponential growth phase and presumably slowed growth in the second exponential growth phase. Transient accumulation of lactate to growth inhibitory concentrations has been observed during growth of C. glutamicum with various carbon sources (Engels et al., 2008).
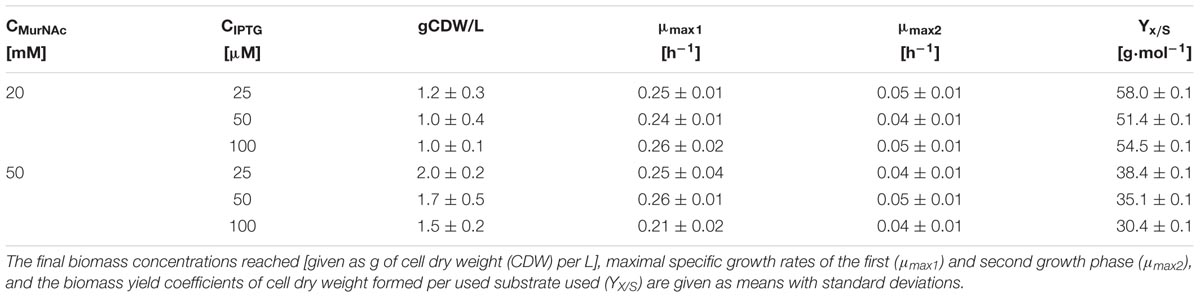
TABLE 2. Growth characteristics of C. glutamicum ΔnanR PCQ on different MurNAc and IPTG concentrations.
As PTS systems typically support growth on their cognate substrates with high affinity, the dependence of the growth rate on the initial MurNAc concentration in the growth medium was determined using strain ΔnanR PCQ. Different concentrations of MurNAc (1, 2.5, 5, 10, and 20 mM) were used and the maximal growth rates were plotted against the MurNAc concentration to derive the maximal growth rate of 0.22 h-1 and the Monod constant of 0.9 ± 0.1 mM as shown in Figure 3. A sub-millimolar Monod constant is typical for PTS mediated uptake.
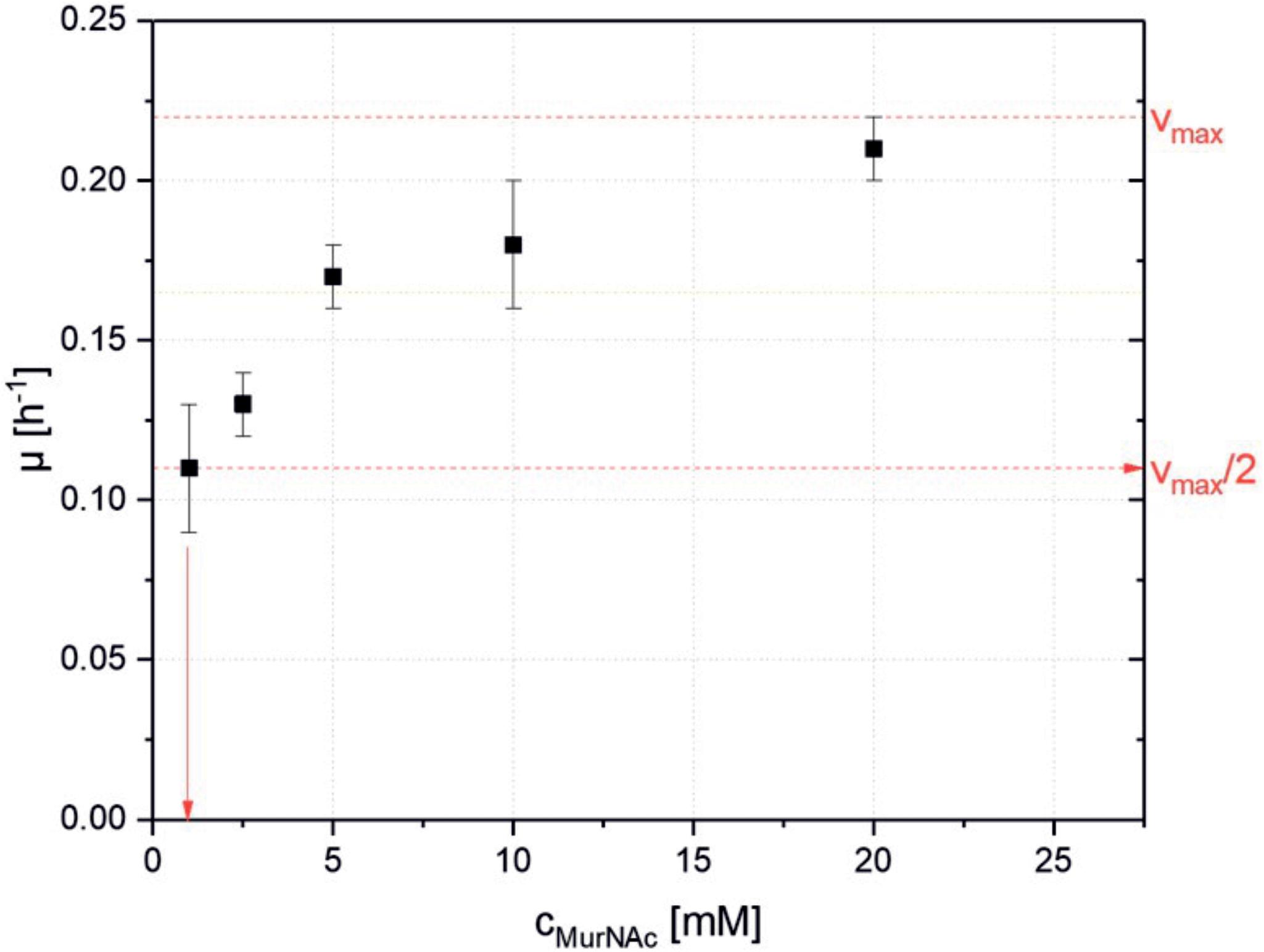
FIGURE 3. Specific maximal growth rates of C. glutamicum ΔnanR PCQ as function of the used MurNAc concentrations. Values and error bars represent means and standard deviations of triplicates.
Comparative Analysis of Growth With MurNAc and/or GlcNAc
Growth of recombinant C. glutamicum with MurNAc and/or GlcNAc as sole carbon sources was compared (Figure 4 and Table 3). With 25 mM MurNAc C. glutamicumΔnanR PCQnE grew to a biomass concentration of 3.0 ± 0.1 gCDW/L, while the maximal biomass concentration was only 2.4 ± 0.1 gCDW/L with GlcNAc. The higher biomass concentration observed with MurNAc in comparison to GlcNAc indicated that lactate released from MurNAc by MurQ contributed to biomass formation. However, the biomass yield was higher with GlcNAc (0.44 ± 0.01 g⋅g-1) than with MurNAc (0.39 ± 0.02 g⋅g-1). GlcNAc catabolism was faster than MurNAc catabolism as the maximal growth rates and the specific substrate uptake rates were lower with MurNAc (0.22 ± 0.10 h-1 and 1.80 ± 0.10 mmol⋅g-1⋅h-1) than with GlcNAc (0.30 ± 0.01 h-1 and 3.00 ± 0.10 mmol⋅g-1⋅h-1) as shown in Table 3.
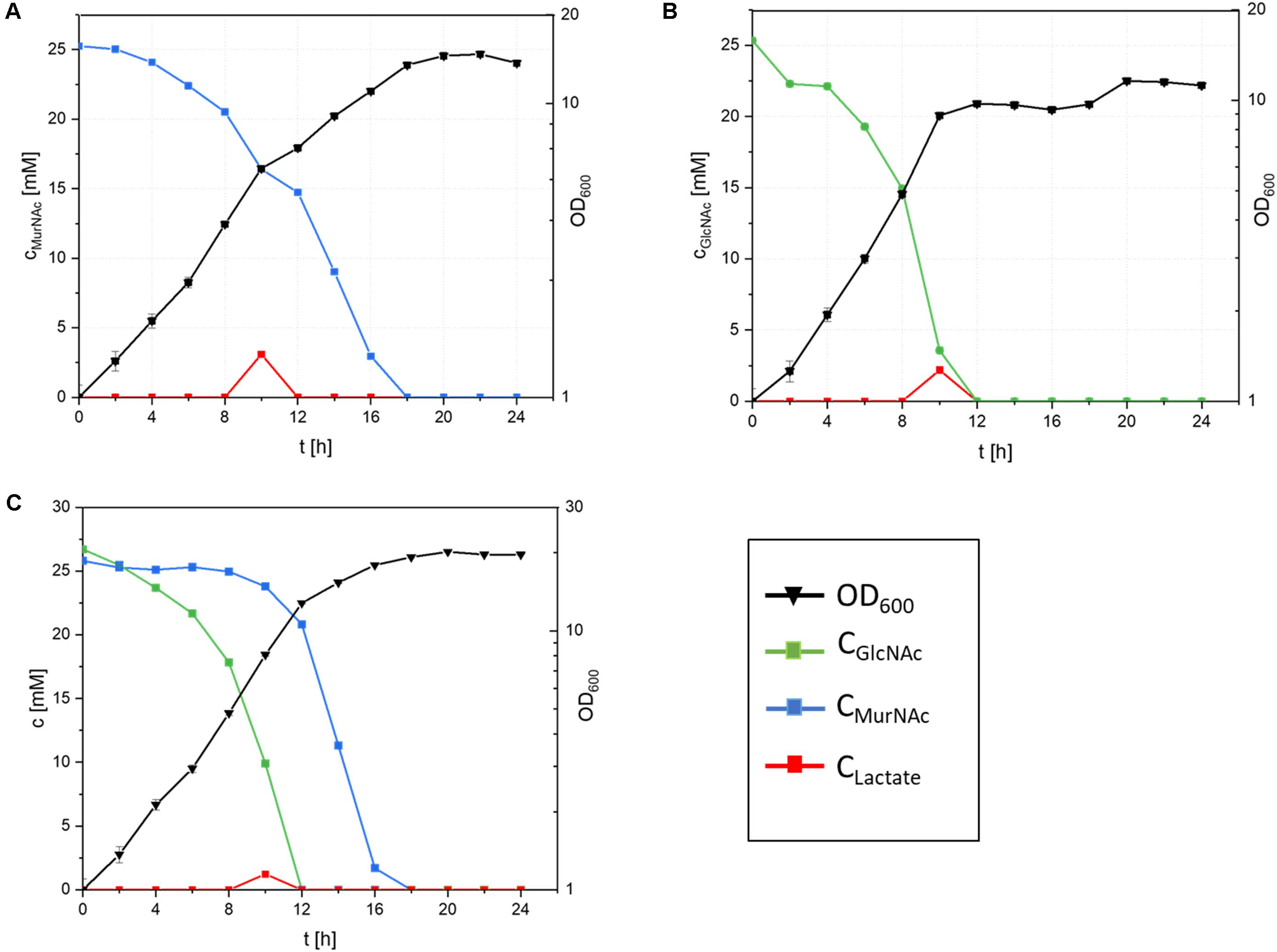
FIGURE 4. Cultivation of C. glutamicum ΔnanR PCQnE in minimal medium with 25 mM MurNAc (A), 25 mM GlcNAc (B) or a mixture of 25 mM MurNAc and 25 mM GlcNAc (C). Incubation was done with 25 μM IPTG at 30°C and 130 rpm. OD600 (filled triangles), the concentrations of GlcNAc (blue squares), MurNAc (green squares) and lactate (red columns) are given as means and standard deviations of triplicates.
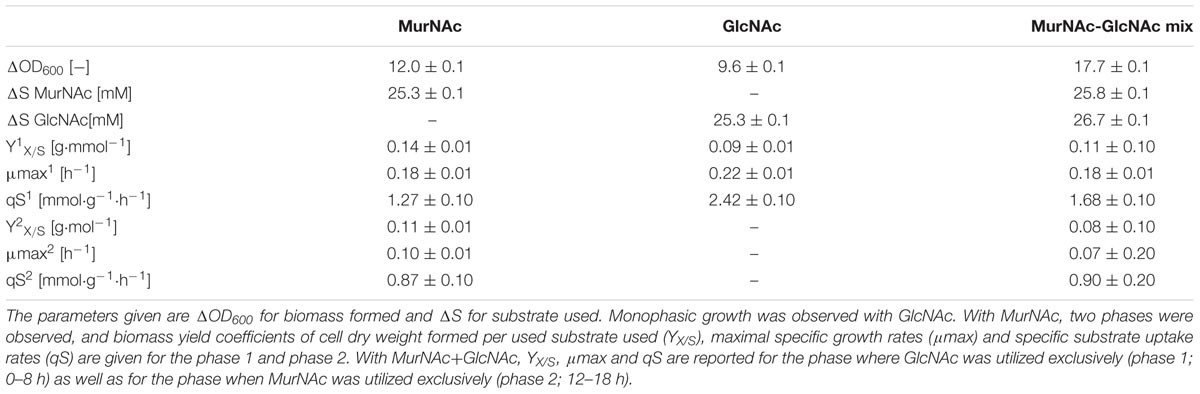
TABLE 3. Cultivation parameters of C. glutamicum ΔnanR PCQnE growing on MurNAc (25 mM), GlcNAc (25 mM) or a MurNAc-GlcNAc-mixture (both 25 mM).
Unlike E. coli and B. subtilis, it is typical for C. glutamicum to simultaneously co-utilize carbon substrates present in blends (Blombach and Seibold, 2010). Therefore, C. glutamicum strain ΔnanR PCQnE was constructed by transforming strain ΔnanR PCQnE with plasmid pEC-XT99A-nagE for expression of the gene for the GlcNAc-specific PTS uptake system to establish whether MurNAc and GlcNAc are co-utilized or utilized sequentially. C. glutamicum strain ΔnanR PCQnE were grown with 25 mM MurNAc and/or 25 mM GlcNAc (Figure 4). With the blend of MurNAc and GlcNAc C. glutamicum ΔnanR PCQnE grew to a biomass concentration of 3.8 ± 0.1 gCDW/L, while a biomass concentration of only 2.1 ± 0.1 g/L was reached in the absence of nagE. Determination of the residual substrate concentrations revealed sequential utilization of GlcNAc before MurNAc (Figure 4C). Thus, unlike many growth substrates MurNAc and GlcNAc were not co-utilized.
MurNAc-Based Production of Food and Feed Additives and Derived Chemicals
MurNAc was expected not only to support growth of recombinant C. glutamicum strains, but also production of value-added compounds. Therefore, MurNAc was tested as sole carbon source or in blends with GlcNAc for production of the amino acids L-lysine and L-glutamate, the diamine 1,5-diaminopentane, the cyclic non-proteinogenic amino acid L-pipecolic acid, and the carotenoid lycopene.
The lycopene accumulating strain C. glutamicum ΔcrtYEb ΔnanR (Matano et al., 2014) was transformed with the plasmids pVWEx1_murP_crr, pEC-XT99A_nagE and pCXE50_murQ as described above and the resulting strains were named ΔcrtYEb ΔnanR PCQ and ΔcrtYEb ΔnanR PCQnE. Cells of both strains accumulated lycopene when grown in MurNAc containing minimal medium. Strain ΔcrtYEb ΔnanR PCQ showed a lycopene content of 0.04 mg ± 0.01 (g CDW)-1 in MurNAc minimal medium. Growth of C. glutamicum ΔcrtYEb ΔnanR PCQnE with a MurNAc/GlcNAc blend led to a lycopene content of 0.10 ± 0.01 mg (g CDW)-1.
The L-lysine producing strains C. glutamicum DM1729ΔnanR PCQ and DM1729ΔnanR PCQnE were constructed based on DM1729ΔnanR as described above for lycopene accumulating strains. DM1729ΔnanR PCQ produced 7 ± 1 mM L-lysine (YP/S 0.27 ± 0.05 mmol mmol-1) and DM1729ΔnanR PCQnE produced 11 ± 1 mM L-lysine (YP/S 0.21 ± 0.10 mmol mmol-1) in minimal medium with either 25 mM MurNAc or a combination of 25 mM MurNAc and 25 mM GlcNAc., whereas 7.6 ± 0.3 mM L-lysine (YP/S 0.30 ± 0.01 mmol mmol-1) have been produced from DM1729PCQnE with 25 mM GlcNAc (Table 4).
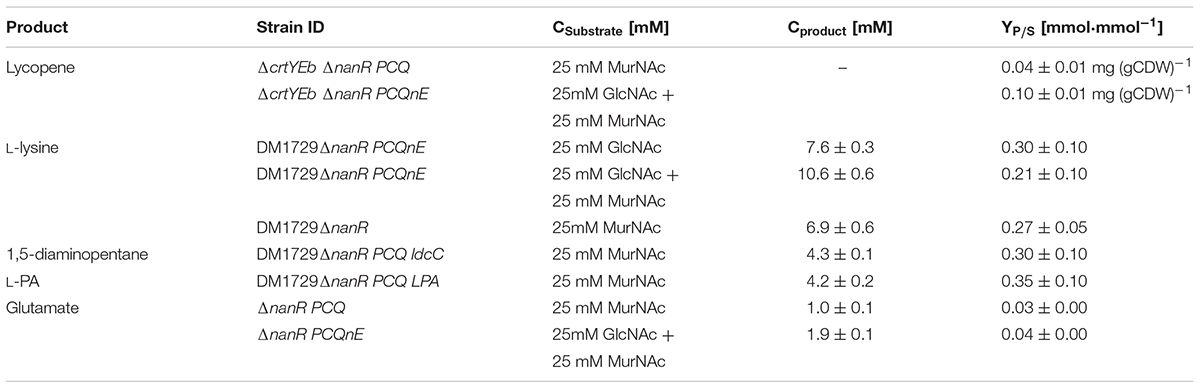
TABLE 4. Parameters describing concentration in mM and production yield (YP/S) of L-lysine,L-PA and 1,5-diaminopentane after 72 h with either 25 mM GlcNAc, either 25 mM MurNAc or 25mM GlcNAc of indicated strains and glutamate production after 48 h from either 25 mM MurNAc either a mixture of GlcNAc and MurNAc, each 25 mM.
To test if the L-lysine derived compounds 1,5-diaminopentane and L-pipecolic acid can also be produced from MurNAc, strain DM1729ΔnanR PCQ was transformed with either pEC-XT99A-ldcC or pEC-XT99A-lysDH-proC. 1,5-Diaminopentane can be generated from L-lysine by lysine decarboxylase LdcC and L-pipecolic acid can be generated from L-lysine in a three-step pathway by L-lysine-6-dehydrogenase (encoded by lysDH from S. pomeroyi), spontaneous ring formation and by pyrroline 5-carboxylate reductase (encoded by endogenous proC) (Pérez-García et al., 2016, 2017). Although the strains showed poor growth, C. glutamicum ΔnanR DM1729PCQ ldcC was able to produce 4.3 ± 0.1 mM of 1,5-diaminopentane (YP/S 0.30 ± 0.10 mmol mmol-1) and C. glutamicum ΔnanR DM1729PCQ LPA produced 4.0 ± 0.2 mM of L-pipecolic acid (YP/S 0.35 ± 0.10 mmol mmol-1) from MurNAc as sole carbon source as shown in Table 4.
L-Glutamate production was accomplished by C. glutamicum ΔnanR PCQ and C. glutamicum ΔnanR PCQnE using penicillin G as trigger. C. glutamicum ΔnanR PCQ accumulated 1 ± 0 mM of L-glutamate from 25 mM MurNAc after 48 h, whereas C. glutamicum ΔnanR PCQnE produced 2 ± 0 mM of L-glutamate under these conditions.
Discussion
In this work, production of food and feed additives by C. glutamicum from MurNAc, an alternative carbon source without competing use in human and animal nutrition, has been established. The food amino acid L-glutamate, the feed amino acid L-lysine and the feed additive lycopene were produced from MurNAc, GlcNAc and blends of both hexosamines.
Metabolic engineering of C. glutamicum for access to MurNAc relied on E. coli genes. Its MurNAc PTS system was active in C. glutamicum and strains expressing crr in addition were able to grow faster and yield more biomass than the strains without heterologous Crr (Figure 2). Crr is a glucose-family specific EIIA component, but it can interact with the PTS-EIIBC components of several members of the glucose PTS family (Barabote and Saier, 2005). C. glutamicum has two complete PTSGlc systems (Barabote and Saier, 2005). The finding that MurNAc could be utilized without heterologous Crr indicated that a PTS component of C. glutamicum made up for its absence. Moreover, the low Monod constant found for growth of the recombinant with MurNAc (Figure 3) indicated that the MurNAc PTS catalyzed high-affinity MurNAc uptake in C. glutamicum, although the MurNAc PTS seems to have a lower affinity for its substrate than, e.g., the heterologous expressed GlcNAc specific PTS system NagE from Corynebacterium glycinophilum which showed a KM value 3.8 ± 0.6 μM (Ferenci, 1996; Matano et al., 2014). The KM for the etherase MurQ in E. coli found in literature had a similar range of value (1.2 mM) (Hadi et al., 2008) with the KM value found experimentally in this study for the MurNAc PTS (0.9 ± 0.1 mM) system, making the two enzymatic steps of up taking, phosphorylation and esterification balanced.
Growth of recombinant C. glutamicum with MurNAc was biphasic and lactate accumulated during the interphase (Figure 4A). MurNAc differs from GlcNAc only by one additional lactoyl group that is hydrolysed to lactate by etherase MurQ. Although C. glutamicum can utilize lactate as sole carbon source (Eikmanns, 2005), lactate accumulated. Utilization of D-lactate requires dld encoding quinone-dependent D-lactate dehydrogenase (Stansen et al., 2005; Kato et al., 2010). Utilization of L-lactate requires quinone-dependent L-lactate dehydrogenase which is encoded in the LldR repressed operon cg3227-lldD (Engels et al., 2008; Georgi et al., 2008). L-Lactate is secreted by C. glutamicum under certain conditions, e.g., during growth with glucose when oxygen is limiting, but is quickly re-utilized once cg3227-lldD is derepressed (Eggeling and Bott, 2005; Stansen et al., 2005; Georgi et al., 2008).
Corynebacterium glutamicum co-utilizes glucose simultaneously with many different carbon sources including those that required introduction of heterologous pathways, for example, xylose (requiring xylose isomerase gene from E. coli (Kawaguchi et al., 2006), arabinose (requiring the araBAD operon from E. coli (Kawaguchi et al., 2008; Schneider et al., 2011), cellobiose (requiring β-glucosidase) or glycerol (requiring E. coli glycerol kinase and glycerol-3-phosphate dehydrogenase), (Rittmann et al., 2008; Sasaki et al., 2008; Zahoor et al., 2012; Meiswinkel et al., 2013; Wendisch et al., 2016). Only rarely, glucose repression has been observed, for example, during sequential utilization of glucose before ethanol (due to catabolite repression of the alcohol dehydrogenase gene adhA) (Gerstmeir et al., 2004) or before glutamate (due to catabolite repression of the operon gluABCD encoding the glutamate uptake system) (Wendisch et al., 2000; Blombach and Seibold, 2010). The preferential utilization of GlcNAc before MurNAc by C. glutamicum ΔnanR PCQnE may be explained by an offset between fast uptake and hydrolysis of MurNAc yielding GlcNac-6-P and D-lactate followed by fast utilization of GlcNAc-6-P, but accumulation of D-lactate. It is conceivable that overexpression of dld would accelerate D-lactate catabolism precluding transient D-lactate accumulation during growth with MurNAc.
A proof-of-concept for MurNAc-based fermentative production of food and feed additives was reached. The engineered strain DM1729ΔnanR PCQ and DM1729ΔnanR PCQnE showed comparable L-lysine production as observed previously for GlcN and GlcNAc (Matano et al., 2014). Similarly, lycopene production from MurNAc by C. glutamicumΔcrtYEbΔnanR PCQ was comparable to that observed by similar strains with 100 mM glucose [30 ± 10 μg⋅g (CDW)-1] and 100 mM GlcNAc [29.6 ± 4.5 μg⋅g (CDW)-1] (Heider et al., 2012; Matano et al., 2014). To establish viable production processes with MurNac as sole or combined carbon source, more work to increase titres, yields and volumetric productivities is needed. Conceptually, however, this work laid the foundation for recycling the cell wall fraction of bacterial biomass from large-scale production processes as substrate for fermentative production of food and feed additives.
Conclusion
Corynebacterium glutamicum was successfully metabolically engineered for utilization of the amino sugar MurNAc as alternative carbon source for growth and production of relevant value-added compounds, specifically L-lysine, L-glutamate and lycopene, from this carbon source lacking competing uses in human and animal nutrition.
Author Contributions
VW conceived the study. VW and ES planned the experiments. ES and LB performed and analyzed the experiments. ES and LB drafted the manuscript. VW finalized the manuscript. All the authors agreed to the final version of the manuscript.
Funding
ES and VW acknowledge the financial support from ZiM project (Grant No. KF2969003SB2). We acknowledge the support for the article processing charge from the Deutsche Forschungsgemeinschaft and the Open Access Publication Fund of Bielefeld University.
Conflict of Interest Statement
The authors declare that the research was conducted in the absence of any commercial or financial relationships that could be construed as a potential conflict of interest.
Supplementary Material
The Supplementary Material for this article can be found online at: https://www.frontiersin.org/articles/10.3389/fmicb.2018.02046/full#supplementary-material
Abbreviations
μmax, maximal specific growth rate; ΔS, variation of substrate concentration; gCDWL-1, gram of Cell Dry Weight per liter; qS, specific substrate uptake rate; YP/S, yield coefficient of cell product per used substrate; YP/X, yield coefficient of product per cell dry weight; YX/S, yield coefficient of cell dry weight per used substrate.
Footnotes
References
Arndt, A., and Eikmanns, B. J. (2008). “Regulation of carbon metabolism in Corynebacterium glutamicum,” in Corynebacteria: Genomics and Molecular Biology, ed. A. Burkovski (Wymondham: Caister Academic Press).
Baba, T., Ara, T., Hasegawa, M., Takai, Y., Okumura, Y., Baba, M., et al. (2006). Construction of Escherichia coli K-12 in-frame, single-gene knockout mutants: the Keio collection. Mol. Syst. Biol. 2, 1–11.
Barabote, R. D., and Saier, M. H. Jr. (2005). Comparative genomic analyses of the bacterial phosphotransferase system. Microbiol. Mol. Biol. Rev. 69, 608–634.
Blombach, B., and Seibold, G. M. (2010). Carbohydrate metabolism in Corynebacterium glutamicum and applications for the metabolic engineering of l-lysine production strains. Appl. Microbiol. Biotechnol. 86, 1313–1322. doi: 10.1007/s00253-010-2537-z
Borisova, M., Gaupp, R., Duckworth, A., Schneider, A., Dalügge, D., Mühleck, M., et al. (2016). Peptidoglycan recycling in gram-positive bacteria is crucial for survival in stationary phase. mBio 7:e00923-16. doi: 10.1128/mBio.00923-16
Chen, J.-K., Shen, C.-R., and Liu, C.-L. (2010). N-Acetylglucosamine: production and applications. Mar. Drugs 8, 2493–2516. doi: 10.3390/md8092493
Cramer, A., and Eikmanns, B. J. (2007). RamA, the transcriptional regulator of acetate metabolism in Corynebacterium glutamicum, is subject to negative autoregulation. J. Mol. Microbiol. Biotechnol. 12, 51–59.
Curtis, S. J., and Epstein, W. (1975). Phosphorylation of d-glucose in Escherichia coli mutants defective in glucosephosphotransferase, mannosephosphotransferase, and glucokinase. J. Bacteriol. 122, 1189–1199.
Dahl, U., Jaeger, T., Nguyen, B. T., Sattler, J. M., and Mayer, C. (2004). Identification of a phosphotransferase system of Escherichia coli required for growth on N-Acetylmuramic Acid. J. Bacteriol. 186, 2385–2392.
Dominguez, H., Cocaign-Bousquet, M., and Lindley, N. D. (1997). Simultaneous consumption of glucose and fructose from sugar mixtures during batch growth of Corynebacterium glutamicum. Appl. Microbiol. Biotechnol. 47, 600–603.
Domsch, K. H. (1982). Soil Biochemistry: Books in Soils and the Environment Series, Vol. 5, eds E. A. Paul and J. N. Ladd. New York, NY: Marcel Dekker Inc., 96–97.
Eggeling, L., and Bott, M. (2005). Handbook of Corynebacterium glutamicum. Boca Raton, FL: CRC Press.
Eikmanns, B. J. (2005). “Central metabolism: Tricarboxylic acid cycle and anaplerotic reactions,” in Handbook on Corynebacterium glutamicum, eds L. Eggeling and M. Bott (Boca Raton, FL: CRC Press), 241–276.
Engels, V., Lindner, S. N., and Wendisch, V. F. (2008). The global repressor SugR controls expression of genes of glycolysis and of the L-lactate dehydrogenase LdhA in Corynebacterium glutamicum. J. Bacteriol. 190, 8033–8044. doi: 10.1128/JB.00705-08
Ferenci, T. (1996). Adaptation to life at micromolar nutrient levels: the regulation of Escherichia coli glucose transport by endoinduction and cAMP. FEMS Microbiol. Rev. 18, 301–317.
Georgi, T., Engels, V., and Wendisch, V. F. (2008). Regulation of L-lactate utilization by the FadR-type regulator LldR of Corynebacterium glutamicum. J. Bacteriol. 190, 963–971.
Gerstmeir, R., Cramer, A., Dangel, P., Schaffer, S., and Eikmanns, B. J. (2004). RamB, a novel transcriptional regulator of genes involved in acetate metabolism of Corynebacterium glutamicum. J. Bacteriol. 186, 2798–2809.
Gibson, D. G. (2011). Enzymatic assembly of overlapping DNA fragments. Methods Enzymol. 498, 349–361. doi: 10.1016/B978-0-12-385120-8.00015-2
Goodell, E. W., and Schwarz, U. (1985). Release of cell wall peptides into culture medium by exponentially growing Escherichia coli. J. Bacteriol. 162, 391–397.
Hadi, T., Dahl, U., Mayer, C., and Tanner, M. E. (2008). Mechanistic studies on N-acetylmuramic acid 6-phosphate hydrolase (MurQ): an etherase involved in peptidoglycan recycling. Biochemistry 47, 11547–11558. doi: 10.1021/bi8014532
Hanahan, D. (1983). Studies on transformation of Escherichia coli with plasmids. J. Mol. Biol 166, 557–580.
Heider, S. A., Peters-Wendisch, P., and Wendisch, V. F. (2012). Carotenoid biosynthesis and overproduction in Corynebacterium glutamicum. BMC Microbiol. 12:198. doi: 10.1186/1471-2180-12-198
Jaeger, T., Arsic, M., and Mayer, C. (2005). Scission of the lactyl ether bond of N-acetylmuramic acid by Escherichia coli “etherase”. J. Biol. Chem. 280, 30100–30106.
Kato, O., Youn, J.-W., Stansen, K. C., Matsui, D., Oikawa, T., and Wendisch, V. F. (2010). Quinone-dependent D-lactate dehydrogenase Dld (Cg1027) is essential for growth of Corynebacterium glutamicum on D-lactate. BMC Microbiol. 10:321. doi: 10.1186/1471-2180-10-321
Kawaguchi, H., Sasaki, M., Vertes, A. A., Inui, M., and Yukawa, H. (2008). Engineering of an L-arabinose metabolic pathway in Corynebacterium glutamicum. Appl. Microbiol. Biotechnol. 77, 1053–1062.
Kawaguchi, H., Vertes, A. A., Okino, S., Inui, M., and Yukawa, H. (2006). Engineering of a xylose metabolic pathway in Corynebacterium glutamicum. Appl. Environ. Microbiol. 72, 3418–3428.
Kinoshita, S., Udaka, S., and Shimono, M. (1957). Studies on the amino acid fermentation. Production of l-glutamic acid by various microorganisms. J. Gen. Appl. Microbiol. 3, 193–205.
Kirchner, O., and Tauch, A. (2003). Tools for genetic engineering in the amino acid-producing bacterium Corynebacterium glutamicum. J. Biotechnol. 104, 287–299.
Lee, J. (2014). Development and characterization of expression vectors for Corynebacterium glutamicum. J. Microbiol. Biotechnol. 24, 70–79.
Matano, C., Kolkenbrock, S., Hamer, S. N., Sgobba, E., Moerschbacher, B. M., and Wendisch, V. F. (2016). Corynebacterium glutamicum possesses beta-N-acetylglucosaminidase. BMC Microbiol. 16:177. doi: 10.1186/s12866-016-0795-3
Matano, C., Uhde, A., Youn, J. W., Maeda, T., Clermont, L., Marin, K., et al. (2014). Engineering of Corynebacterium glutamicum for growth and L-lysine and lycopene production from N-acetyl-glucosamine. Appl. Microbiol. Biotechnol 98, 5633–5643. doi: 10.1007/s00253-014-5676-9
Meiswinkel, T. M., Gopinath, V., Lindner, S. N., Nampoothiri, K. M., and Wendisch, V. F. (2013). Accelerated pentose utilization by Corynebacterium glutamicum for accelerated production of lysine, glutamate, ornithine and putrescine. Microb Biotechnol 6, 131–140. doi: 10.1111/1751-7915.12001
Munoz, E., Ghuysen, J.-M., and Heymann, H. (1967). Cell Walls of Streptococcus pyogenes, Type 14. C Polysaccharide-Peptidoglycan and G Polysaccharide-Peptidoglycan Complexes. Biochemistry 6, 3659–3670.
Nuoffer, C., Zanolari, B., and Erni, B. (1988). Glucose permease of Escherichia coli. The effect of cysteine to serine mutations on the function, stability, and regulation of transport and phosphorylation. J Biol Chem 263, 6647–6655.
Pérez-García, F., Max Risse, J., Friehs, K., and Wendisch, V. F. (2017). Fermentative production of L-pipecolic acid from glucose and alternative carbon sources. Biotechnol J 12, 1600646. doi: 10.1002/biot.201600646
Pérez-García, F., Peters-Wendisch, P., and Wendisch, V. F. (2016). Engineering Corynebacterium glutamicum for fast production of L-lysine and L-pipecolic acid. Appl. Microbiol. Biotechnol. 100, 8075–8090. doi: 10.1007/s00253-016-7682-6
Peters-Wendisch, P. G., Schiel, B., Wendisch, V. F., Katsoulidis, E., Mockel, B., Sahm, H., et al. (2001). Pyruvate carboxylase is a major bottleneck for glutamate and lysine production by Corynebacterium glutamicum. J. Mol. Microbiol. Biotechnol 3, 295–300.
Plumbridge, J. (2009). An Alternative Route for Recycling of N-Acetylglucosamine from Peptidoglycan Involves the N-Acetylglucosamine Phosphotransferase System in Escherichia coli. J. Bacteriol. 191, 5641–5647. doi: 10.1128/JB.00448-09
Reith, J., and Mayer, C. (2011). Peptidoglycan turnover and recycling in Gram-positive bacteria. Appl. Microbiol. Biotechnol. 92, 1–11. doi: 10.1007/s00253-011-3486-x
Rittmann, D., Lindner, S. N., and Wendisch, V. F. (2008). Engineering of a glycerol utilization pathway for amino acid production by Corynebacterium glutamicum. Appl. Environ. Microbiol. 74, 6216–6222. doi: 10.1128/AEM.00963-08
Sasaki, M., Jojima, T., Inui, M., and Yukawa, H. (2008). Simultaneous utilization of D-cellobiose, D-glucose, and D-xylose by recombinant Corynebacterium glutamicum under oxygen-deprived conditions. Appl. Microbiol. Biotechnol. 81, 691–699. doi: 10.1007/s00253-008-1703-z
Schneider, J., Niermann, K., and Wendisch, V. F. (2011). Production of the amino acids L-glutamate, L-lysine, L-ornithine and L-arginine from arabinose by recombinant Corynebacterium glutamicum. J. Biotechnol. 154, 191–198. doi: 10.1016/j.jbiotec.2010.07.009
Shiio, I., Otsuka, S. I., and Takahashi, M. (1962). Effect of biotin on the bacterial formation of glutamic acid. I. Glutamate formation and cellular premeability of amino acids. J Biochem 51, 56–62.
Stansen, C., Uy, D., Delaunay, S., Eggeling, L., Goergen, J. L., and Wendisch, V. F. (2005). Characterization of a Corynebacterium glutamicum lactate utilization operon induced during temperature-triggered glutamate production. Appl. Environ. Microbiol. 71, 5920–5928.
Tchieu, J. H., Norris, V., Edwards, J. S., and Saier, M. H. Jr. (2001). The complete phosphotransferase system in Escherichia coli. J. Mol. Microbiol. Biotechnol. 3, 329–346.
Uehara, T., and Park, J. T. (2008). Growth of Escherichia coli: Significance of Peptidoglycan Degradation during Elongation and Septation. J. Bacteriol. 190, 3914–3922. doi: 10.1128/JB.00207-08
Uehara, T., Suefuji, K., Jaeger, T., Mayer, C., and Park, J. T. (2006). MurQ Etherase is required by Escherichia coli in order to metabolize anhydro-N-acetylmuramic acid obtained either from the environment or from its own cell wall. J. Bacteriol. 188, 1660–1662.
Uhde, A., Youn, J. W., Maeda, T., Clermont, L., Matano, C., Kramer, R., et al. (2013). Glucosamine as carbon source for amino acid-producing Corynebacterium glutamicum. Appl. Microbiol. Biotechnol 97, 1679–1687.
Wendisch, V. F. (2014). Microbial production of amino acids and derived chemicals: synthetic biology approaches to strain development. Curr. Opin. Biotechnol 30, 51–58. doi: 10.1016/j.copbio.2014.05.004
Wendisch, V. F., Brito, L. F., Gil Lopez, M., Hennig, G., Pfeifenschneider, J., Sgobba, E., et al. (2016). The flexible feedstock concept in Industrial Biotechnology: Metabolic engineering of Escherichia coli, Corynebacterium glutamicum, Pseudomonas, Bacillus and yeast strains for access to alternative carbon sources. J. Biotechnol. 234, 139–157. doi: 10.1016/j.jbiotec.2016.07.022
Wendisch, V. F., De Graaf, A. A., Sahm, H., and Eikmanns, B. J. (2000). Quantitative determination of metabolic fluxes during coutilization of two carbon sources: comparative analyses with Corynebacterium glutamicum during growth on acetate and/or glucose. J. Bacteriol. 182, 3088–3096.
Youn, J. W., Jolkver, E., Kramer, R., Marin, K., and Wendisch, V. F. (2008). Identification and characterization of the dicarboxylate uptake system DccT in Corynebacterium glutamicum. J. Bacteriol. 190, 6458–6466. doi: 10.1128/JB.00780-08
Youn, J. W., Jolkver, E., Kramer, R., Marin, K., and Wendisch, V. F. (2009). Characterization of the dicarboxylate transporter DctA in Corynebacterium glutamicum. J. Bacteriol. 191, 5480–5488. doi: 10.1128/JB.00640-09
Zahoor, A., Lindner, S. N., and Wendisch, V. F. (2012). Metabolic engineering of Corynebacterium glutamicum aimed at alternative carbon sources and new products. Computational and Structural Biotechnology Journal 3, e201210004. doi: 10.5936/csbj.201210004
Keywords: L-lysine, diamino pentane, lycopene, L-glutamate, biorefinery, food additives, peptidoglycan, N-acetyl-muraminic acid
Citation: Sgobba E, Blöbaum L and Wendisch VF (2018) Production of Food and Feed Additives From Non-food-competing Feedstocks: Valorizing N-acetylmuramic Acid for Amino Acid and Carotenoid Fermentation With Corynebacterium glutamicum. Front. Microbiol. 9:2046. doi: 10.3389/fmicb.2018.02046
Received: 06 June 2018; Accepted: 13 August 2018;
Published: 24 September 2018.
Edited by:
Laurent Dufossé, Université de La Réunion, FranceReviewed by:
Stefan Junne, Technische Universität Berlin, GermanyChristian U. Riedel, Universität Ulm, Germany
Copyright © 2018 Sgobba, Blöbaum and Wendisch. This is an open-access article distributed under the terms of the Creative Commons Attribution License (CC BY). The use, distribution or reproduction in other forums is permitted, provided the original author(s) and the copyright owner(s) are credited and that the original publication in this journal is cited, in accordance with accepted academic practice. No use, distribution or reproduction is permitted which does not comply with these terms.
*Correspondence: Volker F. Wendisch, dm9sa2VyLndlbmRpc2NoQHVuaS1iaWVsZWZlbGQuZGU=
†These authors have contributed equally to this work