- 1Laboratoire de Biomatériaux et Inflammation en Site Osseux (EA 4691), SFR CAP-Santé, FED 4231, Université de Reims Champagne-Ardenne, Reims, France
- 2Unité de Formation et de Recherche de Pharmacie, Université de Reims Champagne-Ardenne, Reims, France
- 3URCACyt – Plateau technique de cytométrie en flux, Université de Reims Champagne-Ardenne, Reims, France
- 4PICT – Plateforme d’Imagerie Cellulaire et Tissulaire, Université de Reims Champagne-Ardenne, Reims, France
- 5CRITT-MDTS, Charleville-Mézières, France
- 6UFR Odontologie, Reims, France
- 7Pôle Médecine Bucco-Dentaire, Centre Hospitalier Universitaire de Reims, Reims, France
We have recently developed a non-thermal plasma (NTP) equipment intended to sterilize fragile medical devices and maintain the sterile state of items downstream the treatment. With traditional counts on agar plate a six log reduction of Staphylococcus aureus viability was obtained within 120 min of O2, Ar, or N2 NTP treatments. However to determine the best NTP process, we studied the different physiological states of S. aureus by flow cytometry (FC) and confocal laser scanning microscopy (CLSM) focusing on the esterasic activity and membrane integrity of the bacteria. Two fluorochromes, 5-(and-6)-carboxy-2′,7′-dichlorofluorescein diacetate and propidium iodide were used in order to distinguish three sub-populations: metabolically active, permeabilized, and damaged bacteria that can be in the viable but nonculturable state. FC and CLSM highlight that O2 and Ar NTP treatments were the most attractive processes. Indeed, a 5 min of Ar NTP generated a high destruction of the structure of bacteria and a 120 min of O2 NTP treatment led to the higher decrease of the total damaged bacteria population. SEM observations showed that in presence of clusters, bacteria of upper layers are easily altered compared to bacteria in the deeper layers. In conclusion, the plate counting method is not sufficient by itself to determine the best NTP treatment. FC and CLSM represent attractive indicator techniques to select the most efficient gas NTP treatment generating the lowest proportion of viable bacteria and the most debris.
Introduction
A recent interest for non-thermal plasma (NTP) processes has raised in the medical field in order to sterilize fragile devices that do not support conventional techniques such as high pressure saturated steam, ethylene oxide treatment, and ionizing radiation (Moisan et al., 2001; Burts et al., 2009; Hauser et al., 2011; Scholtz et al., 2015).
Non-thermal plasma is an ionized gas consisting of UV-VUV photons, charged particles, reactive species (e.g., reactive oxygen species – ROS, OH., O2, 1O2-; reactive nitrogen species – RNS, NO∙, ONOO∙, …), excited molecules (e.g., excited O2, N2, …) depending on the plasma process and the gas nature (Moisan et al., 2001; Laroussi and Leipold, 2004; Gaunt et al., 2006; Liao et al., 2017). Nowadays there are numerous NTP techniques such as corona discharge, electric barrier discharge, microwave discharge or plasma jet that can inactivate various bacterial strains (Lerouge et al., 2001; Moisan et al., 2001; Boscariol et al., 2008; Sureshkumar et al., 2010; Fröhling et al., 2012; Kvam et al., 2012; Alkawareek et al., 2014; Fröhling and Schlüter, 2015; Scholtz et al., 2015; Impe et al., 2018). These technologies differ from each other in terms of electrical current, discharge reactor designs, working pressures, and operating conditions (nature and flow rate of the gas).
To meet the sterilization standards such as maintaining the sterile state of medical devices after the end of the process, we have developed a new technique able to get NTP inside a sealed bag while maintaining the integrity of bag membranes (Popot and Gelle, 2012). We have recently demonstrated with the plate counting method that our NTP treatment process was highly effective against Bacillus subtilis spores or vegetative bacteria such as Pseudomonas aeruginosa and Staphylococcus aureus (Ben Belgacem et al., 2017). However if the plate counting method is a gold standard to evaluate a sterilization process, this approach does not take into account the different physiological states of bacteria.
Indeed since the 1970s, numerous authors have demonstrated that bacteria can adapt to their environment presenting different states of viability including viable but nonculturable (VBNC) state (Oliver, 2010; Ramamurthy et al., 2014). A cell is usually considered alive when it is metabolically active and capable of reproduction with intact cell membranes (Emerson et al., 2017). VBNC bacteria are alive but they do not divide using conventional culturing technique. However, they can grow and divide again when culture conditions are more favorable for their metabolic recovery as a consequence the bacteria can thereafter express their pathogenicity again (Emerson et al., 2017). Thus VBNC bacteria represent a significant risk in many domains such as medical field (Passerat et al., 2009; Dinu and Bach, 2011; Zandri et al., 2012; Mali et al., 2017; Lee and Bae, 2017).
Recently, different techniques have been developed to study the bacterial different viability states in order to evaluate the efficiency of antimicrobial treatments. These techniques are based on PCR and (RT)-PCR assays (Stanley et al., 2007; Emerson et al., 2017), Fourier transform infrared spectroscopy (FTIR) (Meng et al., 2016) or fluorescence methods (Khan et al., 2010; Sträuber and Müller, 2010; Pasquaroli et al., 2013; Ambriz-Aviña et al., 2014; Li et al., 2017) such as epifluorescence microscopy, flow cytometry (FC) and confocal laser scanning microscopy (CLSM). FC technique represents a fast way to determine the physiological state of bacteria in suspension (Ou et al., 2017). CLSM is a powerful approach for high-resolution imaging of fluorescent bacteria on different focal planes. These fluorescence techniques highlighted some altered characteristics of bacteria such as membrane integrity, pump activity, membrane potential, or metabolic activity: respiratory and enzymatic activities. These methods are highly compatible with a broad range of fluorescent dyes and cell labeling (Léonard et al., 2016).
Staphylococcus aureus is a well-known human pathogen that causes a wide range of clinical infections such as endocarditis, osteoarticular, skin and soft tissue, or medical device-related infections and shock syndrome leading to the death of patients (Burts et al., 2009; Josse et al., 2015; Tong et al., 2015; Kahl et al., 2016; Reddy et al., 2017).
In a previous study, we have demonstrated with culture plating method that S. aureus can be inactivated in 120 min by O2, Ar, or N2 NTP treatments according to the European sterilization norm (Ben Belgacem et al., 2017). The aim of this study, was to control the efficiency of our O2, Ar, and N2 NTP treatments using plate counting, FC, CLSM, and scanning electron microscopy (SEM) observations to investigate more precisely S. aureus viability states.
Materials and Methods
Staphylococcus aureus Strains, Growth Conditions
Staphylococcus aureus CIP 53.154 were provided from Pasteur Institute (Paris, France). Bacteria were stored at -80°C in an appropriate medium supplemented with 50% glycerol. The strain was sequentially subcultured three times in a specific nutrient medium containing glucose, peptone from casein and soybean as well as sodium chloride. The first preculture was carried out at 37°C for 18 h after thawing 1 mL of the cryotube added to 10 mL of nutrient medium. Four milliliters from the previous culture were transferred to a fresh culture medium (50 mL) and incubated for 7 h at 37°C in a rotary shaker at a constant speed of 250 × g. The final bacterial suspension was made by transferring 1 mL from the second culture to a fresh culture medium (50 mL) and incubating for 18 h at 37°C.
Bacteria were centrifuged at 4,200 ×g for 10 min at 4°C and washed twice with distilled water. After the ultimate wash, the bacteria were re-suspended in distilled water to achieve a concentration of approximately 108 colony forming units (CFU)/mL. Twenty microliters of this bacterial suspension were deposited on glass slides and then dried for 10 min before plasma treatment as previously described (Ben Belgacem et al., 2017).
Sterilization by Non-thermal Plasma
The prototype manufactured by Sominex (Bayeux, France) consisted of a stainless steel vacuum chamber 35 L (Popot and Gelle, 2012). A high vacuum state was obtained by using two pumps (Agilent TRISCROLL 300 and Agilent V301). The plasma discharge was generated directly inside a sealed bag (SüdPack®Medica, Germany) by a radio-frequency (RF) polarization plate (Ø 300 mm; RF generator: 13.56 MHz; 300 W) coupled with two magnetic coils (0–14 Gauss; SEF, Labege, France) located at the top and the bottom of the vacuum chamber (Popot and Gelle, 2012; Ben Belgacem et al., 2017). Three mass flow controllers were connected to the gas lines (O2, Ar, and N2) to control the flow rate (0.5 sccm). Contaminated glass slides were placed inside the bag, which was sealed and then set on the RF polarization plate. When the low pressure reached 1.45 × 10-4 mbar, the gases were injected (0.5 sccm) through the first Tyvek®membrane and the excess gas was released through the second Tyvek®membrane into the vacuum chamber. Then, the discharge was induced by the RF polarization (25 W) inside the bag and the plasma was densified by the magnetic field (14 Gauss) for 5, 15, and 120 min. Controlling the pressure difference between the vacuum chamber and the bag, the plasma is kept confined inside the bag.
At the end of the process, the pumping system was stopped and nitrogen was injected into the vacuum chamber until the system returned to atmospheric pressure. To evaluate the antimicrobial efficiency, untreated bacteria were used as controls for each independent experimentations. These controls consisted of non-exposed samples to plasma as well as samples exposed only to low pressure.
Counts on Agar Plate
For each gas plasma treatment and each time period, seven independent experimentations were performed. Glass slides were submitted to mechanical agitation [1 min of vortex, 1 min in a ultrasonic bath (VWR TH USC 300 THD, 45 KHz), and 1 min of vortex] in 15 mL distilled water to detach bacteria and obtain homogenous suspensions. Both bacterial dilution and seeding on nutrient agar plates (trypto-casein soy agar, Biokar, France) were performed with EasySpiral Pro® (Interscience, France). After 24 h of incubation at 37°C, the colonies on agar plates were counted with the Scan®1200 (Interscience, France). A second count was made after 48 h to verify if new colonies could be visualized.
Fluorescent Dye Preparation
Live and dead heat-treated (15 min at 80°C) bacteria used as controls as well as bacterial samples were stained with 5-(and-6)-carboxy-2′,7′-dichlorofluorescein diacetate (DCFDA) and propidium iodide (PI). The stock solution at 9.4 mM of carboxy-DCFDA (C369, ThermoFisher Scientific) was prepared by dissolving the dye in acetone and stored at -20°C in the dark. The PI (Sigma-Aldrich Co., United States) was dissolved in milliQ water in order to have a 0.7 mM stock solution that was kept at -20°C in the dark.
Flow Cytometric Analysis
Staining Procedure
Live and dead heat-treated bacteria (15 min at 80°C) were used as controls to optimize the staining procedure. As described above, glass slides were immersed in 15 mL of distilled water, sonicated and vortexed to obtain bacterial suspensions. One milliliter of each suspension was introduced in a Trucount tube (Becton Dickinson, United States) containing 50,950 beads to accurately collect the same volume at each FC acquisition and thereby allow the calculation of the bacterial concentration per mL (GuoHui et al., 2013).
The bacterial suspension was incubated with 19 μM of DCFDA for 15 min at 37°C to allow deacetylation of DCFDA by esterases into a fluorescent derivative, the dichlorofluorescein (DCF). Ten min after DCFDA, PI (0.75 μM) was added to the mix to allow labeling of permeabilized membranes of bacteria. Stained samples were kept in the dark for no more than 30 min until flow cytometric analysis was performed.
Flow Cytometric Measurement
Analyses were performed with a LSR FortessaTM (Becton Dickinson, United States) flow cytometer. Excitations of DCFDA and PI were done at wavelengths of 488 and 561 nm, respectively, while fluorescence emissions were detected using band pass filters of 530/30 and 610/20 nm, respectively. No compensation settings were applied. Trucount beads were excited at wavelength of 640 nm and emission was detected using band pass filter of 670/14 nm. All recorded signals were logarithmically amplified. Gates created in the dot-plot FSC(H) vs. SSC(H) were preset to identify bacterial and beads populations. The bacterial population was gated using the side scatter (SSC) and forward scatter (FSC) parameters to dissociate it from the background signal and debris. The fluorescent dot plots were gated from this bacterial population. Data acquisition was set to 1,000 beads at a low flow rate (12 μL/min) corresponding to 19.6 μL of the sample. Seven independent assays were performed for each condition. The software FlowJo® (FlowJo, Oregon, United States) was used to analyze FC data.
Confocal Laser Scanning Microscopy
Staining Procedure
Mix DCFDA (470 μM final concentration) and PI (35 μM final concentration) were prepared extemporaneously in 40 μL of distilled water. This stained solution was deposited on controls or NTP treatments glasses slides, covered with a cover slip, and sealed with a clear varnish to prevent drying of the bacterial sample during visualization with the CLSM.
Images Acquisition
Immunofluorescence-labeled bacteria preparations were studied using a Zeiss LSM 710® NLO confocal laser scanning microscope (Carl ZEISS SAS, Marly le Roi, France) with the 20× objective (ON 0.8) and numerical zoom of 2. DCFDA and PI were simultaneously excited by 488 nm line of argon laser and diode laser 561 nm. Emitted signals were, respectively, collected with 505–544 nm and 600–700 nm bandpass filters. Two images from 10 mm2 sample areas were acquired for each condition. A minimum of triplicate experiments were done for each condition.
Images Analysis and Quantification
Quantification of images was performed using a home-made macro on ImageJ software (National Institute of Health, United States). The macro steps included intensity threshold and count of metabolically active and permeabilized bacteria using “particle analysis” with shape parameter on 505–544 nm and 600–700 nm images, respectively. The number of bacteria was then divided by the image area size to obtain a density of events. Then, the damaged number of events was calculated by counting bacteria on logic AND image between the two acquired images.
Scanning Electron Microscopy
Contaminated glass slides before or after the different NTP treatments were fixed with 2.5% glutaraldehyde in phosphate buffer solution (PBS) for 1 h at room temperature, and rinsed twice with PBS for 10 min. The samples were than dehydrated in graded series of ethanol/water solutions at 50, 70, 90, and 100% (twice) and covered with hexamethyldisilazane solution (Sigma-Aldrich, St. Louis, MO, United States) (Braux et al., 2011). The samples were dessicated overnight at room temperature then coated with platinum on a Jeol Ultrafine Coat JUC 5000 and observed on a FEG Scanning Electron Microscope Zeiss Ultra Plus (Ibisa Platform of Electron Microscopy, Tours, France). Two independent assays were performed for each condition.
Statistical Analysis
Statistical analysis was performed by the Wilcoxon’s t test (Prism 5, GraphPad Software, San Diego, CA, United States). A value of ∗p < 0.05, ∗∗p < 0.01, ∗∗∗p < 0.001 was considered to be statistically significant.
Results
Efficiency of Non-thermal Gas Plasma Against S. aureus Determined by the Plate Counting Method
Plate counts, a gold standard method highlighting only the bacteria capable to grow on agar plate, was used to quantify the biocidal efficiency of different NTP treatments. The bactericidal effect on S. aureus was measured by comparing the number of CFUs in the plasma treated sample with the control (1.07 × 107 CFU/mL) (Figure 1).
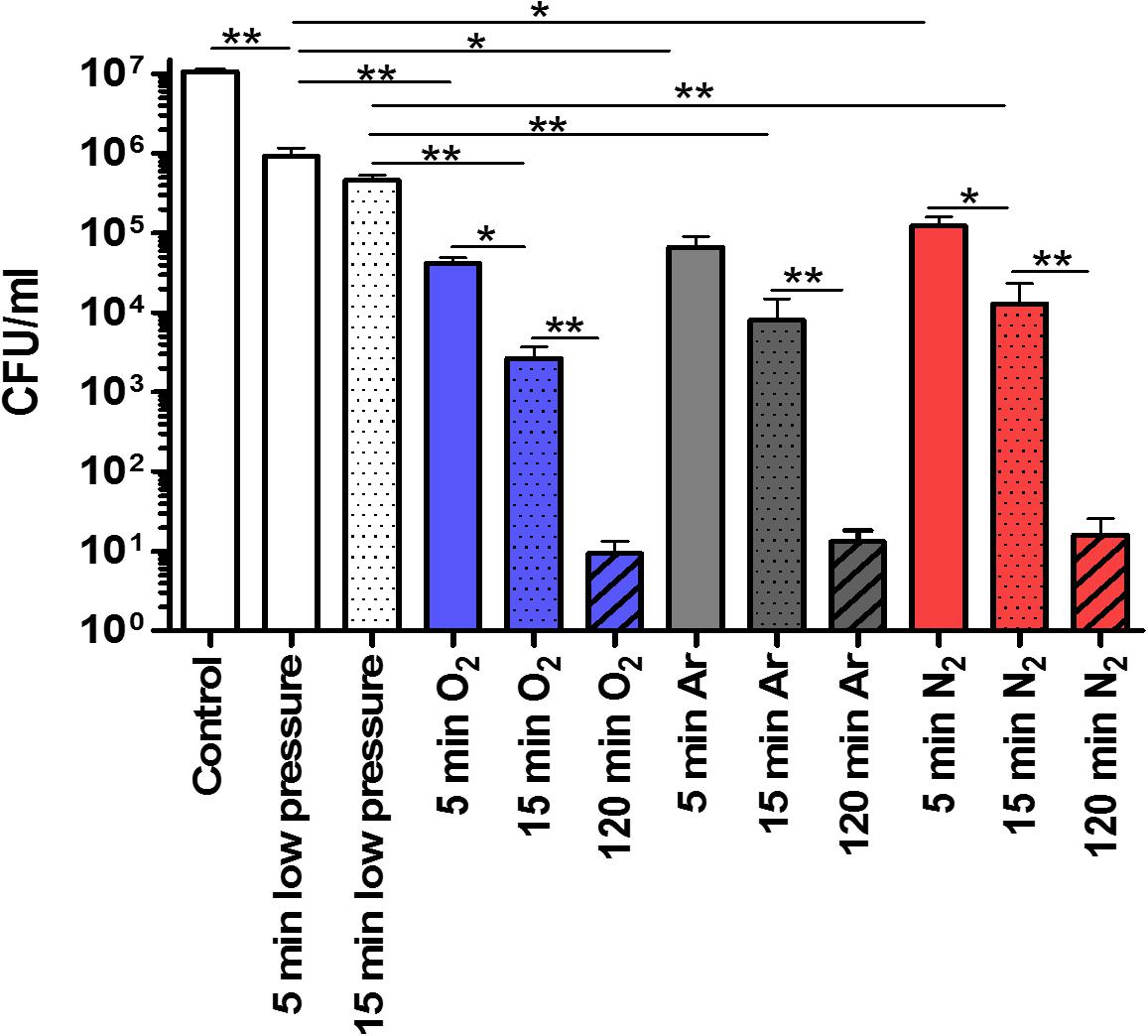
FIGURE 1. Inactivation of Staphylococcus aureus with N2, O2, Ar NTP (25 W; 14 G; and 0.5 sccm) determined by counting on agar plate. Error bars represent the standard error of the mean from seven experiments in triplicate. Statistical analysis was performed by the Wilcoxon test ∗p < 0.05; ∗∗p < 0.01.
When samples were subjected only to the low pressure, a significant decrease in S. aureus viability was observed after 5 min (9.24 × 105 CFU/mL, p < 0.01). However, after 15 min of low pressure no statistical significant decrease was observed as compared to 5 min with a recovery of 4.62 × 105 CFU/mL.
Looking at the effects of 5 min of O2, Ar, or N2 NTP treatments, we observed each time a significantly higher reduction of S. aureus viability than in presence of only low pressure treatment (Figure 1, O2, p < 0.01; Ar, p < 0.05; N2, p < 0.05). Furthermore, the effectiveness of all NTP treatments increased as a function of the time. After 15 min of NTP treatment, O2 treatment tended to be more effective (2.67 × 103 CFU/mL) as compared to Ar (8 × 103 CFU/mL) and N2 (1.29 × 104 CFU/mL) NTP treatments. However, after 120 min of NTP treatments, the bactericidal effect (6 log reduction) was obtained with all the three gases (O2: 9.38 × 100 CFU/mL; Ar: 1.32 × 101 CFU/mL; N2: 1.6 × 101 CFU/mL).
Efficacy of Non-thermal Gas Plasma Against S. aureus Determined by FC
To assess the different physiological states of the S. aureus population after short NTP exposures, the samples were analyzed by FC. Figure 2 shows an example of the bacterial population detected in the gate. This is a heterogeneous control population (1.9 × 107 events/mL = 100%) composed of bacteria with different sizes and granularities.
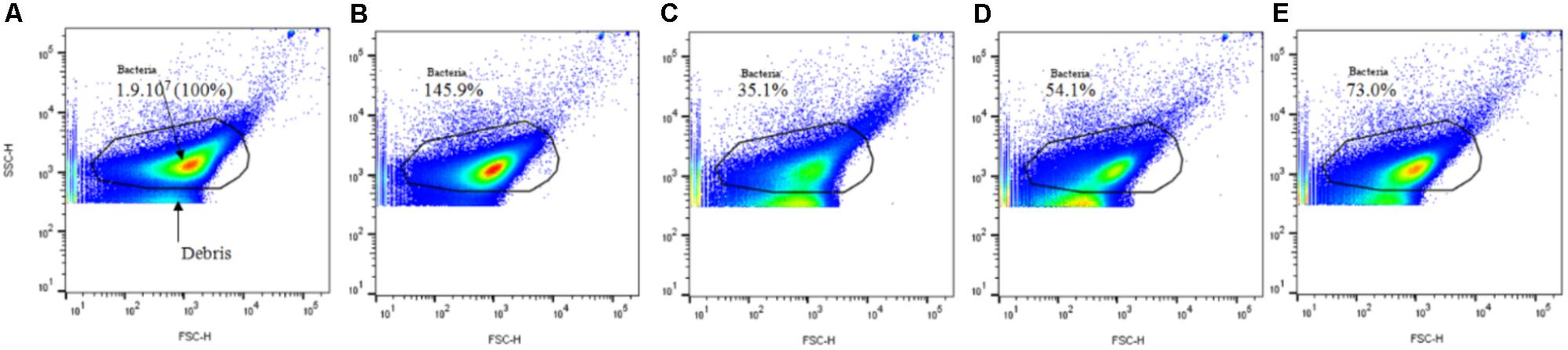
FIGURE 2. Selection of the Staphylococcus aureus population by FC based on FSC and SSC parameters. Effect of 15 min of NTP treatments. (A) Control: number of events/mL; (B) 15 min low pressure; (C) 15 min O2; (D) 15 min Ar; (E) 15 min N2. (B–E) Expressed as a percentage of the control population.
After 15 min of low pressure, this population seemed to increase (145.9% of the bacterial population control) (Figures 2A,B). After 15 min of NTP treatments, with each gas, the bacterial population density decreased (O2: 35.1%; Ar: 54.1%; N2: 73%) (Figures 2C–E).
From the previous gate, bacteria population presenting esterasic activities and/or permeabilized membrane was analyzed after 15 min of NTP treatments. As seen in Figure 3, bacteria populations were divided in four distinct sub-populations based on their differential PI and DCFDA staining characteristics. These sub-populations consisted of: metabolically active bacteria (upper left quadrant; DCFDA+ and PI-); damaged bacteria with esterasic activity and permeabilized membrane (upper right quadrant; both DCFDA+ and PI+); permeabilized bacteria without esterasic activity (lower right quadrant; DCFDA- and PI+); and unlabeled population corresponding to lysed or degraded bacteria (lower left quadrant; both DCFDA- and PI-). The whole three labeled sub-populations (active, damaged, and permeabilized bacteria; 1.78.107 events/mL) represented the labeled bacteria control.
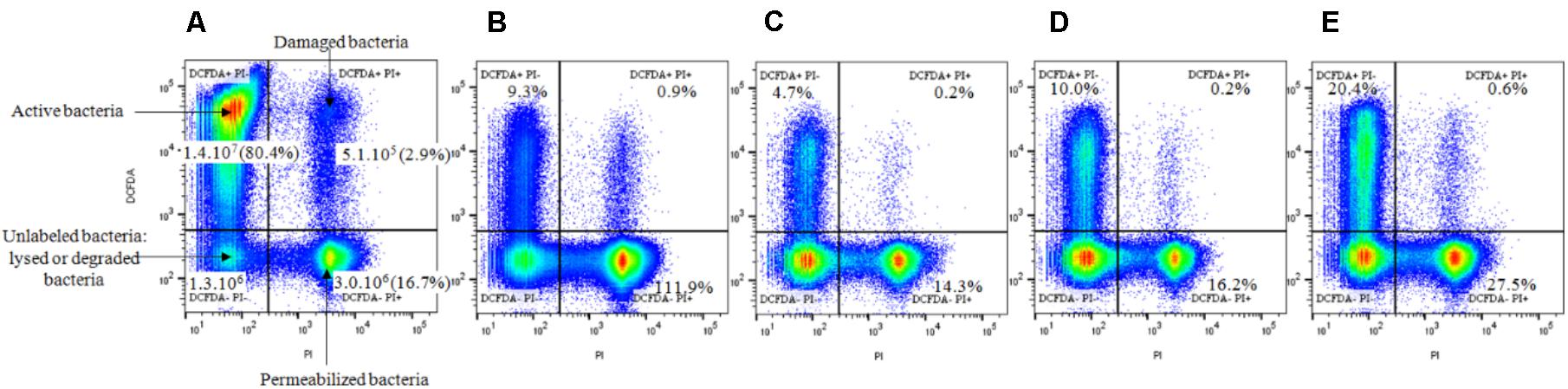
FIGURE 3. Influence of the gas nature (N2, O2, and Ar) after 15 min of NTP treatments on S. aureus physiological states, determined by FC. The fluorescent dot plots were gated from the bacterial population previously determined and were obtained in response to double-staining with DCFDA and PI. (A) Control: total labeled events (1.93 × 107 events/mL = 100%); (B) 15 min low pressure; (C) 15 min O2; (D) 15 min Ar; (E) 15 min N2. (B–E) Expressed as a percentage of the total labeled events of the control.
Active bacteria (1.4 × 107 events/mL) represented the major labeled sub-population (80.4% of the labeled bacteria control), whereas the other sub-populations were smaller: 5.1 × 105 events/mL (2.9%) for damaged bacteria, 3.0 × 106 events/mL (16.7%) for permeabilized bacteria and 1.3 × 106 events/mL for unlabeled population (Figure 3A).
Low pressure treatment of 15 min increased the permeabilized bacteria population to 111.9% of the labeled bacteria control, essentially by decreasing the active bacteria population (from 80.4 to 9.3%) (Figure 3B). After 15 min of NTP treatments, a decrease of the active bacteria population (O2: 4.7%; Ar: 10%; N2: 20.4%) was observed for the benefit of the permeabilized bacterial population (O2: 14.3%; Ar: 16.2%; N2: 27.5%) and unlabeled bacteria or debris (Figures 3C–E).
Based on the total gated population, Table 1 and Supplementary Figure S1 show the distribution of the three sub-populations (active, damaged, and permeabilized) after 5 and 15 min of treatments (n = 7). The labeled population in the control (1.93 × 107 events/mL) was composed of 72.36% active, 3.21% damaged, and 24.44% permeabilized bacteria. Looking more in details, the different treatments (low pressure and NTP), systematically induced a reduction of the whole labeled populations and modified their repartitions.
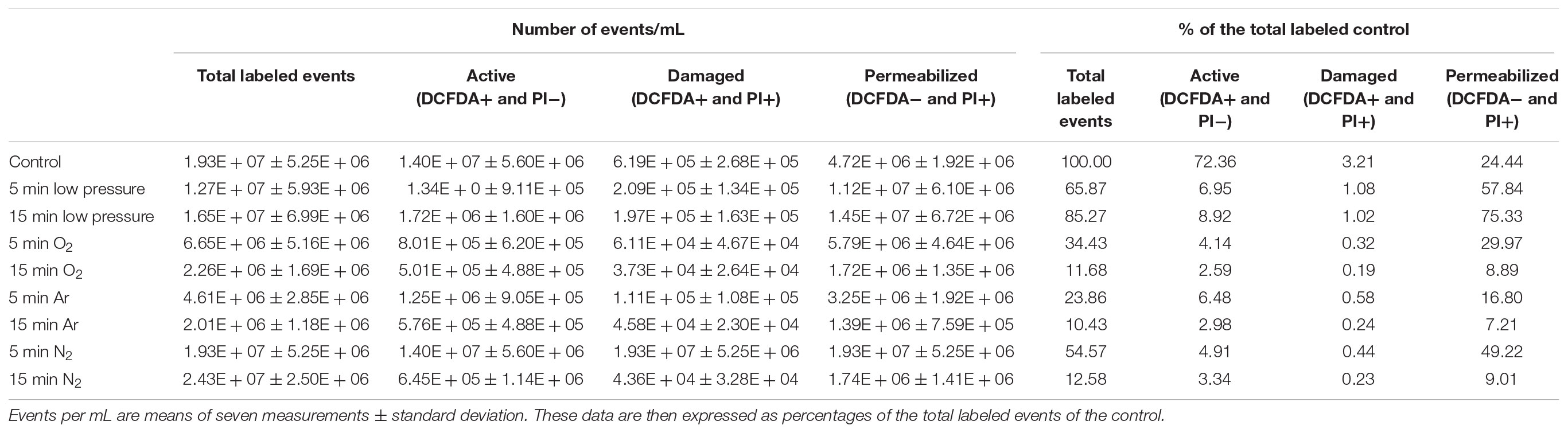
TABLE 1. Analyse by FC of Staphylococcus aureus sub-populations [total labeled, metabolically active sub-population (DCFDA+ and PI-), damaged sub-population (DCFDA+ and PI+), and permeabilized sub-population (DCFDA- and PI+)] labeled with PI and DCFDA fluorochromes after 5 and 15 min of O2, Ar, and N2 NTP treatments.
Low pressure treatments decreased active bacteria population to 8.92% after a 15 min of treatment and in the meantime increased the permeabilized bacteria population to 75.33%. NTP treatments induced a time-dependent decrease of the active and permeabilized bacteria populations, whatever the gas used. After 5 min of treatments, Ar plasma led to the highest decrease of the total labeled population (23.86%) compared to O2 (34.43%) and N2 (54.57%). After 15 min of NTP treatments, this decline was enhanced (Ar: 10.43%; O2: 11.68%; N2: 12.58%). The 15 min of O2 NTP treatment was the most efficient in regard to the bacteria populations with esterasic activity (2.78%), compared to the others NTP treatments (Ar: 3.22%; N2: 3.57%).
Beyond 15 min of NTP treatments, the remaining labeled bacteria populations were too low and may have been drowned in the background noise. Therefore, the FC analysis was not suitable.
Efficiency of NTP Plasma Against S. aureus Determined by CLSM
Confocal laser scanning microscopy approach is complementary to the FC as it allows to evaluate low density of bacteria such as active (DCFDA+ and PI-; green event), damaged (DCFDA+ and PI+; yellow event), and permeabilized (DCFDA- and PI+; red event) sub-populations (Figure 4, Table 2, and Supplementary Figure S2). The control population was composed of 64.85% active bacteria and only 12.38% permeabilized or 22.72% damaged bacteria (Figure 4A and Table 2).
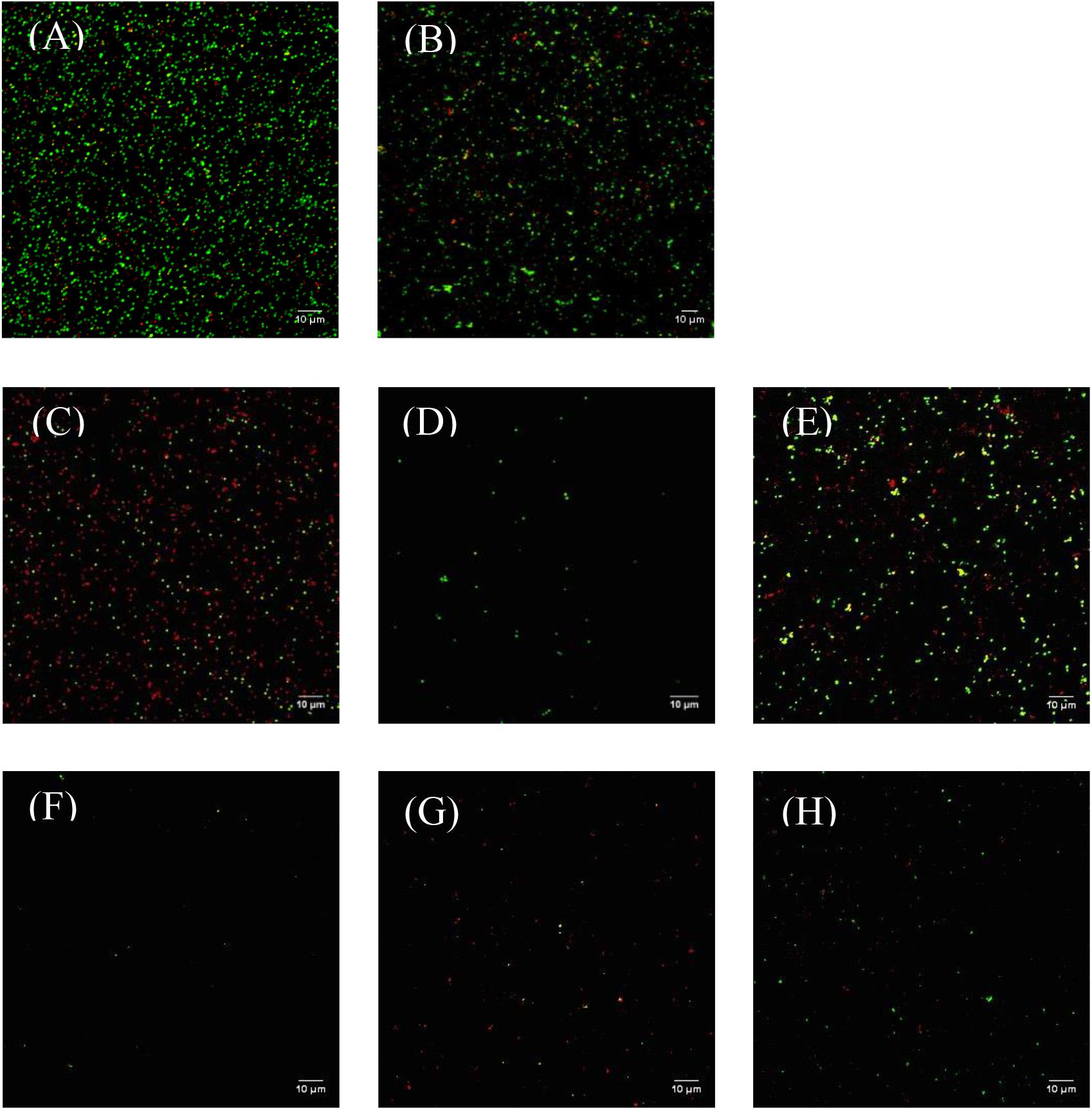
FIGURE 4. Representative CLSM images of Staphylococcus aureus labeled with PI and DCFDA fluorochromes after 5 or 120 min of O2, Ar, or N2 NTP treatments. DCFDA+ and PI– bacteria (green). DCFDA+ and PI+ bacteria (yellow). DCFDA– and PI+ bacteria (red). (A) Control; (B) 5 min low pressure; (C) 5 min O2; (D) 5 min Ar; (E) 5 min N2; (F) 120 min O2; (G) 120 min Ar; (H) 120 min N2.
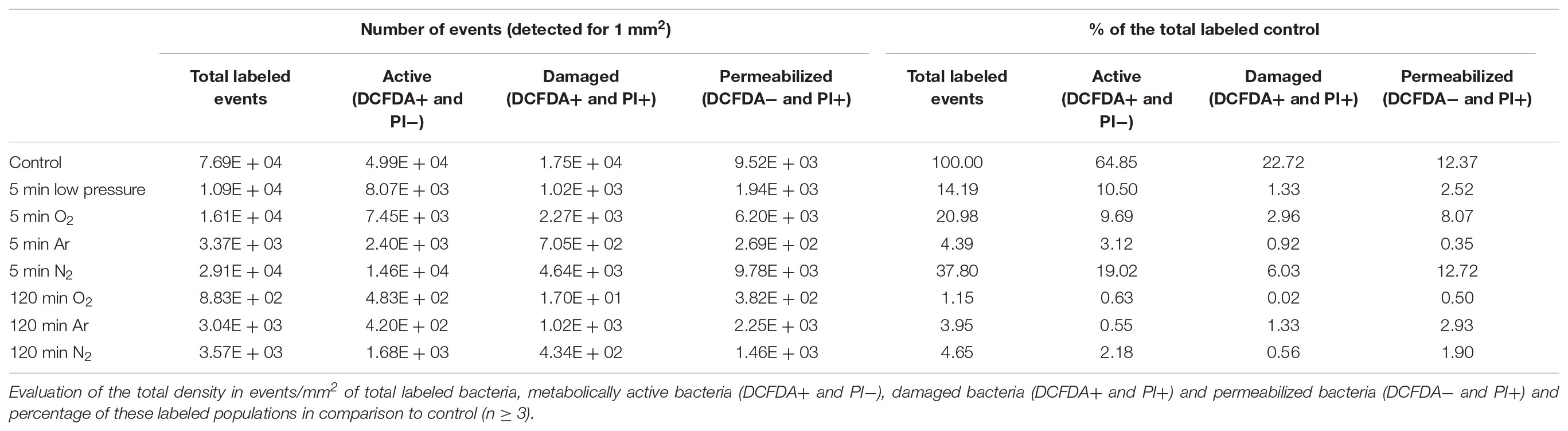
TABLE 2. Density of Staphylococcus aureus population determined by CLSM with DCFDA and PI after low pressure for 5 min, O2, Ar, and N2 NTP treatments for 5 and 120 min (events/mm2).
After 5 min of treatments (low pressure and NTP), a decrease of the whole labeled populations was observed and it appeared clearly that the Ar NTP treatment generated the highest decrease in bacterial density (>95%, Figure 4D and Table 2). Furthermore, this total decrease was not linked to an increase of permeabilized or damaged bacteria populations, suggesting that the bacteria were completely destroyed, making impossible the labeling with PI.
To obtain a bacterial viability reduction of 6 log, the plasma process had to be extended to 120 min according to the plate counting method (Figure 1). In these conditions, applying solely low pressure that decreased up to 10-5 mbar was not relevant as it was only reaching 1.45 × 10-4 mbar during the NTP treatments. Whatever 120 min of NTP treatments, a reduction of almost 95% of the total labeled population could be observed (Figures 4F–H and Table 2). However, the O2 NTP treatment was the most efficient (0.63% active bacteria; 0.50% permeabilized bacteria; and 0.02% damaged bacteria). Like in the FC analysis, the N2 NTP treatment was the least efficient (2.18% active bacteria, 0.56% damaged bacteria, and 1.90% permeabilized bacteria).
Plasma Effect on S. aureus Morphology Determined by SEM
The observation by SEM confirmed that S. aureus had a round shape (Figure 5A) and that 120 min of low pressure exposure at 10-5 mbar did not lead to major bacteria alterations (Figure 5B). After 5 min exposure if a large proportion of bacteria were undamaged with the N2 NTP treatment (Figure 5E), O2 and Ar NTP treatments led to some cell alterations such as rupture of membranes and loss of intracellular material (Figures 5C,D). After 15 min exposure, NTP treatments were more effective (Figures 5F–H). However if they were more debris, the bacteria located in the deepest layers of the deposit seemed to be protected, keeping their round shape (Figures 5G,H). After 120 min whatever NTP treatments, there were mainly bacterial debris and only very few bacteria could still be visualized (Figures 5I–K).
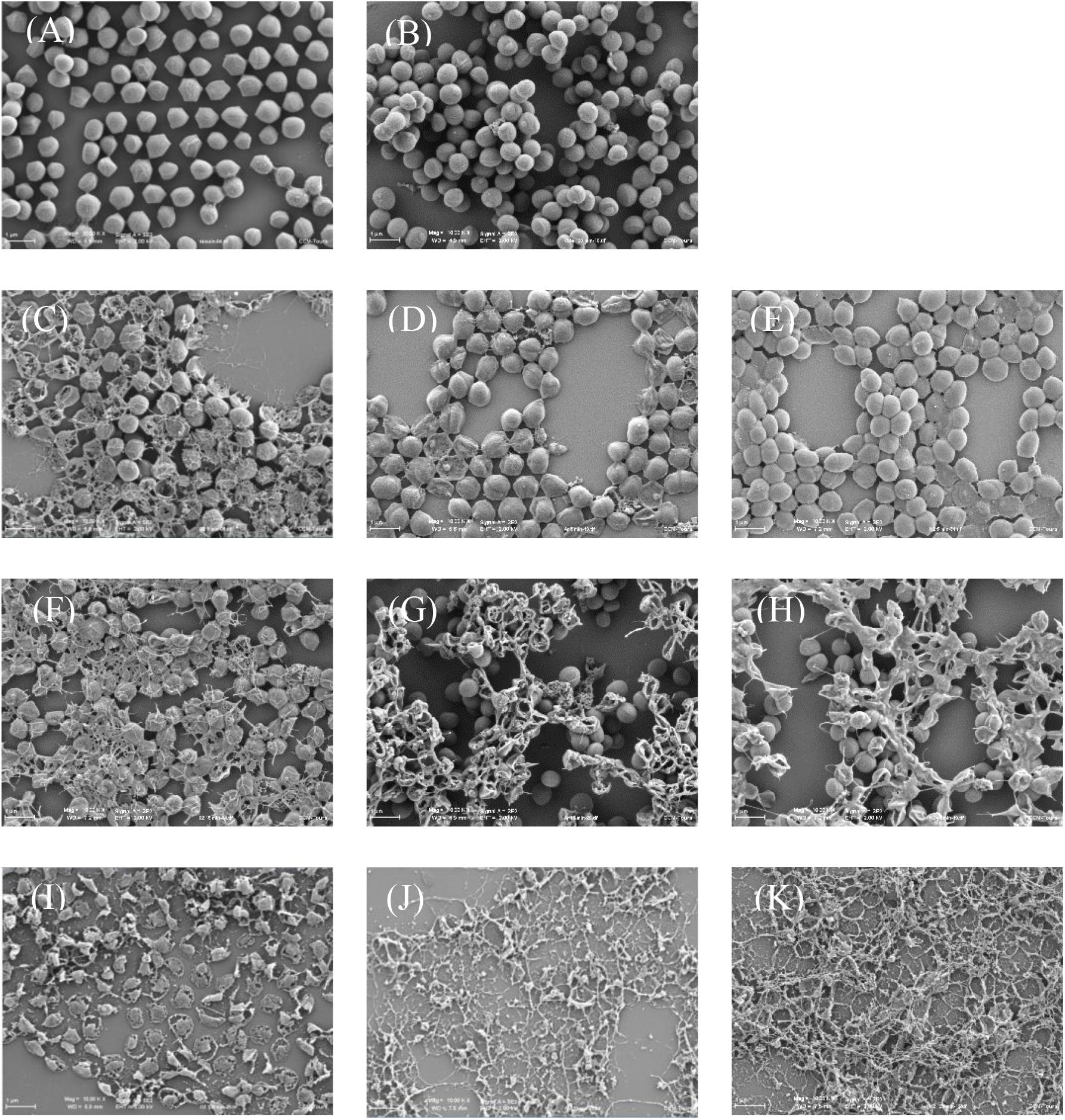
FIGURE 5. Representative SEM images of Staphylococcus aureus before and after plasma treatments (G × 10,000). (A) Control; (B) 120 min low pressure; (C) 5 min O2; (D) 5 min Ar; (E) 5 min N2; (F) 120 min O2; (G) 15 min Ar; (H) 15 min N2; (I) 15 min O2; (J) 120 min Ar; and (K) 120 min N2.
Discussion
The aim of this study was to control the efficiency of our O2, N2, and Ar NTP process by two fluorescent techniques (FC and CLSM). These techniques allow to highlight more precisely S. aureus viability states compared to standard culture plating method.
Recently, we have demonstrated through the plate counting method that O2, Ar, or N2 NTP process could inactivate S. aureus within 120 min, which is line with the European norm of sterilization (Ben Belgacem et al., 2017). This technique highlights only bacteria dividing and not the damaged bacteria, such as VBNC, that are capable to divide and express their pathogenicity when conditions are more favorable thereafter. In literature, S. aureus can enter into VBNC or dormancy states when subjected to environmental stresses such as short NTP exposure (Abramzon et al., 2006; Vandervoort et al., 2008; Joaquin et al., 2009). VBNC are considered as a threat to public health due to their non-detectability by conventional methods in the medical field (Oliver, 2010; Zandri et al., 2012; Pasquaroli et al., 2013; Gonçalves and de Carvalho, 2016).
Flow cytometry (a quantitative method) and CLSM (a semi-quantitative method) analyses allowed to characterize the physiological states of S. aureus (active, permeabilized, and damaged bacteria) after NTP treatments. Two probes were used: PI staining bacterial DNA when the cell membranes are permeabilized and DCFDA revealing the esterasic activity of bacteria.
Our process requires working at low pressure before inducing a plasma in a sealed bag. Therefore we have determined the effect of low pressure on S. aureus. Although it did not allow to meet the sterility standards by itself according to culture plating method (Ben Belgacem et al., 2017), some bacterial alterations such as a decrease of esterasic activity and an increase of membranes permeabilization have been observed. Indeed, it can be suggested that bacteria have been weakened by low pressure unlike B. subtilis spores in the same process conditions (Ben Belgacem et al., 2017).
Concerning the O2, N2, and Ar NTP treatments, a time-dependent decrease of cultivable bacteria population was observed, up to 6 log reduction after 120 min of treatment. Similarly both fluorescent methods revealed a time-dependent decrease of the total labeled bacteria. Nonetheless, these techniques highlighted some differences between the three bacteria sub-populations repartition depending on the NTP treatments. They suggested that a short time of Ar NTP treatment was more efficient in the alteration of bacteria and the production of the lysed bacteria or debris. After 15 min and whatever the gas used, the efficiency of the treatments was enhanced, as underlined with FC or plate counting method. However, no significant difference in the sub-populations repartition was observed by FC whatever the NTP treatments.
Although the FC provides a quick analysis of different physiological states there are some limitations for its use, e.g., the low remaining numbers of events that can be labeled may be drowned in the background noise. Consequently, CLSM has been used to highlight the different physiological states at the end of process. CLSM revealed that a 120 min of O2 or Ar NTP treatments led to the smaller proportion of active bacteria compared to N2 treatment. Nevertheless O2 plasma treatment can be considered as the most interesting while reducing significantly the number of damaged S. aureus that could have been potential VBNC bacteria.
Scanning electron microscopy method was used to observe the S. aureus surface after the different NTP treatments. Within the 5 min of O2 or Ar NTP treatments, remarkable morphological changes have been observed, which correlates with CLSM results. When S. aureus multilayers are present on the glass slides, only the upper layers of the bacterial deposit were damaged after 15 min of NTP treatments, while bacteria located in the deepest layers seemed morphologically intact. This bacterial organization close to a biofilm structuration is well known for its strong resistance to NTP treatments (Moreau et al., 2005; Kamgang et al., 2007; Joshi et al., 2010; Ermolaeva et al., 2011; Guo et al., 2015; Puligundla and Mok, 2017). After 120 min of NTP treatments, whatever the gas used, a 6 log reduction was obtained with the plate counting method, and only debris could be visualized by SEM. However, a few labeled bacteria were observed by CLSM even if O2 NTP treatment seemed to be the most efficient. Figure 6 summarizes the techniques used to monitor and determine the efficiency of our NTP treatments up to 120 min.
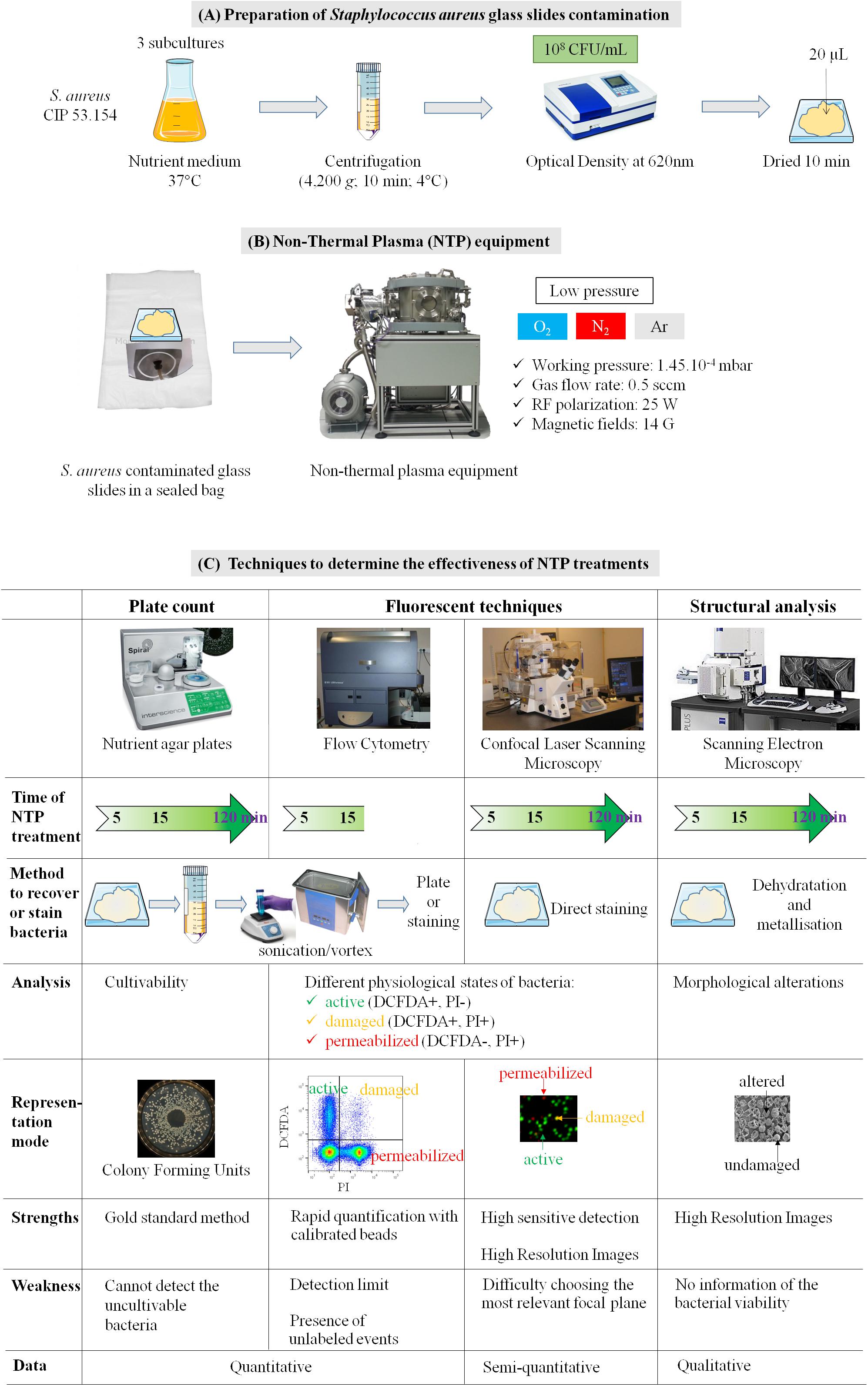
FIGURE 6. General flow chart of (A) preparation of contaminated glass slides; (B) NTP equipment; (C) techniques selected to determine the efficiency of NTP treatments in function to the duration of treatments.
The inactivation mechanism of NTP is still unclear. However, three mechanisms are usually proposed: UV radiations of genetic material, photodesorption of cell by UV radiations and etching by ions and chemical reactive species (Moisan et al., 2001, 2002; De Geyter and Morent, 2012; Fröhling and Schlüter, 2015).
In our low pressure NTP process, the reactive species of Ar, O2, and N2 and UV photons are generated to inactivate bacteria and presumably VUV photons too (Halfmann et al., 2007; Rauscher et al., 2010; Guo et al., 2015). However, some authors highlighted the main role of chemical active species in the inactivation compared to UV and VUV photons (Rauscher et al., 2010; Guo et al., 2015).
We have demonstrated previously that a short time of Ar NTP treatment was the most efficient to inactivate S. aureus. To explain this early efficiency between the plasmas, we could supposed that Ar+ could lead to an etching process causing perforations of bacteria membranes as suggested by Opretzka et al. (2007). When Ar–He plasma was applied, they observed perforations of Bacillus atrophaeus spore coat allowing reactive components from plasma to penetrate in the cell to act directly. Moreover, they suggested that it was a crucial point to alter multi-layers stacks of bacteria or bacteria embedded in a matrix.
After a longer time of exposure, O2 NTP treatment was the most efficient. O2 plasma is well-known to generate ROS, UV photons and probably metastable state O2∗ (Guo et al., 2015). Among to these reactive components, the ROS are involved in etching process and then to oxidative stress leading to the bacteria death.
Moreover, different authors have shown the interest of O2 and Ar mixture NTP treatment to inactive resistant microbes as spores or to remove bacterial protein residues (Raballand et al., 2008; Rossi et al., 2009; von Keudell et al., 2010). Etching and desorption mechanisms could implied in the permeabilization of bacteria membranes and specially of the characteristic peptidoglycan of Gram-positive bacteria. As a consequence, it can be suggested that if this permeabilization did not lead to the S. aureus death, VBNC could be generated.
Conclusion
Our NTP process is a promising alternative to conventional technologies for innovative medical devices sterilization. However, to evaluate its efficiency, several precautions must be taken. Indeed, the plate counting method was not sufficient by itself to ensure that no bacteria can recover and grow. Hence, fluorescent methods by determining different physiological states have showed their interest to evaluate the efficiency of the NTP process. CLSM technique showed that a few active bacteria are still present downstream the NTP treatment. To improve the treatment efficiency, it would be interesting to test an Ar–O2 mixture NTP at different concentrations or alternate use of both Ar and O2 plasmas. Additionally to optimize our sterilization technique, we will identify the generated particles from our NTP process and then will investigate their bactericidal mechanisms.
Author Contributions
MB and M-PG contributed to the prototype conception. GC, EC, CD, ZBB, and M-PG contributed to the bacterial studies. GC, EC, and M-PG contributed to the conception of the work. GC and EC performed the fluorescent experiment. GC, SA, and CT analyzed the cytometric and confocal data. GC, SA, CT, SG, and M-PG wrote the manuscript. All authors read and approved the final manuscript.
Funding
This study was supported by the national agency of Research of France (Project TecSanPlas’Ster ANR-12-TECS-0007), French Defence Procurement Agency and the Champagne-Ardenne Region.
Conflict of Interest Statement
The authors declare that the research was conducted in the absence of any commercial or financial relationships that could be construed as a potential conflict of interest.
Acknowledgments
We thank Sylvie Guillaume (EA 4691 BIOS) for their technical assistance. We also thank Sonia Georgeault (Laboratoire de Biologie Cellulaire et Microscopie Electronique, Tours, France) for performing SEM analysis.
Supplementary Material
The Supplementary Material for this article can be found online at: https://www.frontiersin.org/articles/10.3389/fmicb.2018.02171/full#supplementary-material
References
Abramzon, N., Joaquin, J. C., Bray, J., and Brelles-Mariño, G. (2006). Biofilm destruction by RF high-pressure cold plasma jet. IEEE Trans. Plasma Sci. 34, 1304–1309. doi: 10.1109/TPS.2006.877515
Alkawareek, M. Y., Gorman, S. P., Graham, W. G., and Gilmore, B. F. (2014). Potential cellular targets and antibacterial efficacy of atmospheric pressure non-thermal plasma. Int. J. Antimicrob. Agents 43, 154–160. doi: 10.1016/j.ijantimicag.2013.08.022
Ambriz-Aviña, V., Contreras-Garduño, J. A., and Pedraza-Reyes, M. (2014). Applications of flow cytometry to characterize bacterial physiological responses. Biomed. Res. Int. 2014:461941. doi: 10.1155/2014/461941
Ben Belgacem, Z. B., Carré, G., Charpentier, E., Le-Bras, F., Maho, T., Robert, E., et al. (2017). Innovative non-thermal plasma disinfection process inside sealed bags: assessment of bactericidal and sporicidal effectiveness in regard to current sterilization norms. PLoS One 12:e0180183. doi: 10.1371/journal.pone.0180183
Boscariol, M. R., Moreira, A. J., Mansano, R. D., Kikuchi, I. S., and Pinto, T. J. A. (2008). Sterilization by pure oxygen plasma and by oxygen–hydrogen peroxide plasma: an efficacy study. Int. J. Pharm. 353, 170–175. doi: 10.1016/j.ijpharm.2007.11.059
Braux, J., Velard, F., Guillaume, C., Bouthors, S., Jallot, E., Nedelec, J.-M., et al. (2011). A new insight into the dissociating effect of strontium on bone resorption and formation. Acta Biomater. 7, 2593–2603. doi: 10.1016/j.actbio.2011.02.013
Burts, M. L., Alexeff, I., Meek, E. T., and McCullers, J. A. (2009). Use of atmospheric non-thermal plasma as a disinfectant for objects contaminated with methicillin-resistant Staphylococcus aureus. Am. J. Infect. Control 37, 729–733. doi: 10.1016/j.ajic.2009.03.010
De Geyter, N., and Morent, R. (2012). Nonthermal plasma sterilization of living and nonliving surfaces. Annu. Rev. Biomed. Eng. 14, 255–274. doi: 10.1146/annurev-bioeng-071811-150110
Dinu, L.-D., and Bach, S. (2011). Induction of viable but nonculturable Escherichia coli O157:H7 in the phyllosphere of lettuce: a food safety risk factor. Appl. Environ. Microbiol. 77, 8295–8302. doi: 10.1128/AEM.05020-11
Emerson, J. B., Adams, R. I., Román, C. M. B., Brooks, B., Coil, D. A., Dahlhausen, K., et al. (2017). Schrödinger’s microbes: tools for distinguishing the living from the dead in microbial ecosystems. Microbiome 5:86. doi: 10.1186/s40168-017-0285-3
Ermolaeva, S. A., Varfolomeev, A. F., Chernukha, M. Y., Yurov, D. S., Vasiliev, M. M., Kaminskaya, A. A., et al. (2011). Bactericidal effects of non-thermal argon plasma in vitro, in biofilms and in the animal model of infected wounds. J. Med. Microbiol. 60, 75–83. doi: 10.1099/jmm.0.020263-0
Fröhling, A., Baier, M., Ehlbeck, J., Knorr, D., and Schlüter, O. (2012). Atmospheric pressure plasma treatment of Listeria innocua and Escherichia coli at polysaccharide surfaces: inactivation kinetics and flow cytometric characterization. Innovative Food Sci. Emerg. Technol. 13, 142–150. doi: 10.1016/j.ifset.2011.11.002
Fröhling, A., and Schlüter, O. K. (2015). Flow cytometric evaluation of physico-chemical impact on gram-positive and Gram-negative bacteria. Front. Microbiol. 6:939. doi: 10.3389/fmicb.2015.00939
Gaunt, L. F., Beggs, C. B., and Georghiou, G. E. (2006). Bactericidal action of the reactive species produced by gas-discharge nonthermal plasma at atmospheric pressure: a review. IEEE Trans. Plasma Sci. 34, 1257–1269. doi: 10.1109/TPS.2006.878381
Gonçalves, F. D. A., and de Carvalho, C. C. C. R. (2016). Phenotypic modifications in Staphylococcus aureus cells exposed to high concentrations of Vancomycin and Teicoplanin. Front. Microbiol. 7:13. doi: 10.3389/fmicb.2016.00013
Guo, J., Huang, K., and Wang, J. (2015). Bactericidal effect of various non-thermal plasma agents and the influence of experimental conditions in microbial inactivation: a review. Food Control 50, 482–490. doi: 10.1016/j.foodcont.2014.09.037
GuoHui, B., Chunhui, Y., Miao, H., Hong, W., Jiaxin, L., Ye, C., et al. (2013). Three different bacterial detection systems for platelet concentrates under inter-laboratory conditions. Transfus. Apher. Sci. 49, 600–607. doi: 10.1016/j.transci.2013.07.029
Halfmann, H., Denis, B., Bibinov, N., Wunderlich, J., and Awakowicz, P. (2007). Identification of the most efficient VUV/UV radiation for plasma based inactivation of Bacillus atrophaeus spores. J. Phys. D Appl. Phys. 40:5907. doi: 10.1088/0022-3727/40/19/019
Hauser, J., Esenwein, S.-A., Awakowicz, P., Steinau, H.-U., Köller, M., and Halfmann, H. (2011). Sterilization of heat-sensitive silicone implant material by low-pressure gas plasma. Biomed. Instrum. Technol. 45, 75–79. doi: 10.2345/0899-8205-45.1.75
Impe, J., Smet, C., Tiwari, B., Greiner, R., Ojha, S., Stulić, V., et al. (2018). State of the art of nonthermal and thermal processing for inactivation of micro-organisms. J. Appl. Microbiol. 125, 16–35. doi: 10.1111/jam.13751
Joaquin, J. C., Kwan, C., Abramzon, N., Vandervoort, K., and Brelles-Mariño, G. (2009). Is gas-discharge plasma a new solution to the old problem of biofilm inactivation? Microbiol. Read. Engl. 155, 724–732. doi: 10.1099/mic.0.021501-0
Joshi, S. G., Paff, M., Friedman, G., Fridman, G., Fridman, A., and Brooks, A. D. (2010). Control of methicillin-resistant Staphylococcus aureus in planktonic form and biofilms: a biocidal efficacy study of nonthermal dielectric-barrier discharge plasma. Am. J. Infect. Control 38, 293–301. doi: 10.1016/j.ajic.2009.11.002
Josse, J., Velard, F., and Gangloff, S. C. (2015). Staphylococcus aureus vs. osteoblast: relationship and consequences in osteomyelitis. Front. Cell. Infect. Microbiol. 5:85. doi: 10.3389/fcimb.2015.00085
Kahl, B. C., Becker, K., and Löffler, B. (2016). Clinical significance and pathogenesis of staphylococcal small colony variants in persistent infections. Clin. Microbiol. Rev. 29, 401–427. doi: 10.1128/CMR.00069-15
Kamgang, J. O., Briandet, R., Herry, J. M., Brisset, J. L., and Naïtali, M. (2007). Destruction of planktonic, adherent and biofilm cells of Staphylococcus epidermidis using a gliding discharge in humid air. J. Appl. Microbiol. 103, 621–628. doi: 10.1111/j.1365-2672.2007.03286.x
Khan, M. M. T., Pyle, B. H., and Camper, A. K. (2010). Specific and rapid enumeration of viable but nonculturable and viable-culturable gram-negative bacteria by using flow cytometry. Appl. Environ. Microbiol. 76, 5088–5096. doi: 10.1128/AEM.02932-09
Kvam, E., Davis, B., Mondello, F., and Garner, A. L. (2012). Non-thermal atmospheric plasma rapidly disinfects multidrug-resistant microbes by inducing cell surface damage. Antimicrob. Agents Chemother. 56, 2028–2036. doi: 10.1128/AAC.05642-11
Laroussi, M., and Leipold, F. (2004). Evaluation of the roles of reactive species, heat, and UV radiation in the inactivation of bacterial cells by air plasmas at atmospheric pressure. Int. J. Mass Spectrom. 233, 81–86. doi: 10.1016/j.ijms.2003.11.016
Lee, S., and Bae, S. (2017). Molecular viability testing of viable but non-culturable bacteria induced by antibiotic exposure. Microb. Biotechnol. doi: 10.1111/1751-7915.13039 [Epub ahead of print].
Léonard, L., Bouarab Chibane, L., Ouled Bouhedda, B., Degraeve, P., and Oulahal, N. (2016). Recent advances on multi-parameter flow cytometry to characterize antimicrobial treatments. Front. Microbiol. 7:1225. doi: 10.3389/fmicb.2016.01225
Lerouge, S., Wertheimer, M. R., and Yahia, L. ’H. (2001). Plasma sterilization: a review of parameters, mechanisms, and limitations. Plasmas Polym. 6, 175–188. doi: 10.1023/A:1013196629791
Li, J., Ding, T., Liao, X., Chen, S., Ye, X., and Liu, D. (2017). Synergetic effects of ultrasound and slightly acidic electrolyzed water against Staphylococcus aureus evaluated by flow cytometry and electron microscopy. Ultrason. Sonochem. 38, 711–719. doi: 10.1016/j.ultsonch.2016.08.029
Liao, X., Liu, D., Xiang, Q., Ahn, J., Chen, S., Ye, X., et al. (2017). Inactivation mechanisms of non-thermal plasma on microbes: a review. Food Control 75, 83–91. doi: 10.1016/j.foodcont.2016.12.021
Mali, S., Mitchell, M., Havis, S., Bodunrin, A., Rangel, J., Olson, G., et al. (2017). A proteomic signature of dormancy in the actinobacterium micrococcus luteus. J. Bacteriol. 199:e00206-17. doi: 10.1128/JB.00206-17
Meng, J., Gong, Y., Qian, P., Yu, J.-Y., Zhang, X.-J., and Lu, R.-R. (2016). Combined effects of ultra-high hydrostatic pressure and mild heat on the inactivation of Bacillus subtilis. LWT Food Sci. Technol. 68, 59–66. doi: 10.1016/j.lwt.2015.12.010
Moisan, M., Barbeau, J., Crevier, M. C., Pelletier, J., Philip, N., and Saoudi, B. (2002). Plasma sterilization. Methods and mechanisms. Pure Appl. Chem. 74, 349–358. doi: 10.1351/pac200274030349
Moisan, M., Barbeau, J., Moreau, S., Pelletier, J., Tabrizian, M., and Yahia, L. (2001). Low-temperature sterilization using gas plasmas: a review of the experiments and an analysis of the inactivation mechanisms. Int. J. Pharm. 226, 1–21. doi: 10.1016/S0378-5173(01)00752-9
Moreau, M., Feuilloley, M. G. J., Orange, N., and Brisset, J.-L. (2005). Lethal effect of the gliding arc discharges on Erwinia spp. J. Appl. Microbiol. 98, 1039–1046. doi: 10.1111/j.1365-2672.2004.02535.x
Oliver, J. D. (2010). Recent findings on the viable but nonculturable state in pathogenic bacteria. FEMS Microbiol. Rev. 34, 415–425. doi: 10.1111/j.1574-6976.2009.00200.x
Opretzka, J., Benedikt, J., Awakowicz, P., Wunderlich, J., and Von Keudell, A. (2007). The role of chemical sputtering during plasma sterilization of Bacillus atrophaeus. J. Phys. D Appl. Phys. 40, 2826–2830. doi: 10.1088/0022-3727/40/9/024
Ou, F., McGoverin, C., Swift, S., and Vanholsbeeck, F. (2017). Absolute bacterial cell enumeration using flow cytometry. J. Appl. Microbiol. 123, 464–477. doi: 10.1111/jam.13508
Pasquaroli, S., Zandri, G., Vignaroli, C., Vuotto, C., Donelli, G., and Biavasco, F. (2013). Antibiotic pressure can induce the viable but non-culturable state in Staphylococcus aureus growing in biofilms. J. Antimicrob. Chemother. 68, 1812–1817. doi: 10.1093/jac/dkt086
Passerat, J., Got, P., Dukan, S., and Monfort, P. (2009). Respective roles of culturable and viable-but-nonculturable cells in the heterogeneity of Salmonella entericaserovar typhimurium invasiveness. Appl. Environ. Microbiol. 75,5179–5185. doi: 10.1128/AEM.00334-09
Popot, J.-M., and Gelle, M.-P. (2012). Device for Cold Plasma Sterilization of an Object, Such as a Medical Device, Particularly an Implant, and Method Using This Device. Available at: https://patentscope.wipo.int/search/en/detail.jsf?docId=WO2012038669 [accessed April 9, 2018].
Puligundla, P., and Mok, C. (2017). Potential applications of nonthermal plasmas against biofilm-associated micro-organisms in vitro. J. Appl. Microbiol. 122, 1134–1148. doi: 10.1111/jam.13404
Raballand, V., Benedikt, J., Wunderlich, J., and Von Keudell, A. (2008). Inactivation of Bacillus atrophaeus and of Aspergillus niger using beams of argon ions, of oxygen molecules and of oxygen atoms. J. Phys. Appl. Phys. 41:115207. doi: 10.1088/0022-3727/41/11/115207
Ramamurthy, T., Ghosh, A., Pazhani, G. P., and Shinoda, S. (2014). Current perspectives on viable but non-culturable (VBNC) pathogenic bacteria. Front. Public Health 2:103. doi: 10.3389/fpubh.2014.00103
Rauscher, H., Kylián, O., Benedikt, J., von Keudell, A., and Rossi, F. (2010). Elimination of biological contaminations from surfaces by plasma discharges: chemical sputtering. Chemphyschem 11, 1382–1389. doi: 10.1002/cphc.200900757
Reddy, P. N., Srirama, K., and Dirisala, V. R. (2017). An update on clinical burden, diagnostic tools, and therapeutic options of Staphylococcus aureus. Infect. Dis. 10:1179916117703999. doi: 10.1177/1179916117703999
Rossi, F., Kylian, O., Rauscher, H., Hasiwa, M., and Gilliland, D. (2009). Low pressure plasma discharges for the sterilization and decontamination of surfaces. New J. Phys. 11:115017. doi: 10.1088/1367-2630/11/11/115017
Scholtz, V., Pazlarova, J., Souskova, H., Khun, J., and Julak, J. (2015). Nonthermal plasma—a tool for decontamination and disinfection. Biotechnol. Adv. 33, 1108–1119. doi: 10.1016/j.biotechadv.2015.01.002
Stanley, E. C., Mole, R. J., Smith, R. J., Glenn, S. M., Barer, M. R., McGowan, M., et al. (2007). Development of a new, combined rapid method using phage and PCR for detection and identification of viable Mycobacterium paratuberculosis bacteria within 48 hours. Appl. Environ. Microbiol. 73, 1851–1857. doi: 10.1128/AEM.01722-06
Sträuber, H., and Müller, S. (2010). Viability states of bacteria–specific mechanisms of selected probes. Cytom. Part J. Int. Soc. Anal. Cytol. 77, 623–634. doi: 10.1002/cyto.a.20920
Sureshkumar, A., Sankar, R., Mandal, M., and Neogi, S. (2010). Effective bacterial inactivation using low temperature radio frequency plasma. Int. J. Pharm. 396, 17–22. doi: 10.1016/j.ijpharm.2010.05.045
Tong, S. Y. C., Davis, J. S., Eichenberger, E., Holland, T. L., and Fowler, V. G. (2015). Staphylococcus aureus infections: epidemiology, pathophysiology, clinical manifestations, and management. Clin. Microbiol. Rev. 28, 603–661. doi: 10.1128/CMR.00134-14
Vandervoort, K. G., Abramzon, N., and Brelles-Mariño, G. (2008). Plasma interactions with bacterial biofilms as visualized through atomic force microscopy. IEEE Trans. Plasma Sci. 36, 1296–1297. doi: 10.1109/TPS.2008.917953
von Keudell, A., Awakowicz, P., Benedikt, J., Raballand, V., Yanguas-Gil, A., Opretzka, J., et al. (2010). Inactivation of bacteria and biomolecules by low-pressure plasma discharges. Plasma Process. Polym. 7, 327–352. doi: 10.1002/ppap.200900121
Zandri, G., Pasquaroli, S., Vignaroli, C., Talevi, S., Manso, E., Donelli, G., et al. (2012). Detection of viable but non-culturable staphylococci in biofilms from central venous catheters negative on standard microbiological assays. Clin. Microbiol. Infect. 18, 259–261. doi: 10.1111/j.1469-0691.2012.03893.x
Keywords: non-thermal plasma, Staphylococcus aureus, fluorescence, flow cytometry, confocal microscopy
Citation: Carré G, Charpentier E, Audonnet S, Terryn C, Boudifa M, Doliwa C, Belgacem ZB, Gangloff SC and Gelle M-P (2018) Contribution of Fluorescence Techniques in Determining the Efficiency of the Non-thermal Plasma Treatment. Front. Microbiol. 9:2171. doi: 10.3389/fmicb.2018.02171
Received: 01 June 2018; Accepted: 23 August 2018;
Published: 10 September 2018.
Edited by:
Fabian Cieplik, Universitätsklinikum Regensburg, GermanyCopyright © 2018 Carré, Charpentier, Audonnet, Terryn, Boudifa, Doliwa, Belgacem, Gangloff and Gelle. This is an open-access article distributed under the terms of the Creative Commons Attribution License (CC BY). The use, distribution or reproduction in other forums is permitted, provided the original author(s) and the copyright owner(s) are credited and that the original publication in this journal is cited, in accordance with accepted academic practice. No use, distribution or reproduction is permitted which does not comply with these terms.
*Correspondence: Marie-Paule Gelle, bWFyaWUtcGF1bGUuZ2VsbGVAdW5pdi1yZWltcy5mcg== Gaëlle Carré, Y2FycmVnYWVsbGUxQGdtYWlsLmNvbQ==