- 1Laboratory of Systems and Synthetic Biology, Wageningen University and Research, Wageningen, Netherlands
- 2Enzyme Technology Laboratory, National Center for Genetic Engineering and Biotechnology, Pathumthani, Thailand
- 3International Research Centre in Critical Raw Materials-ICCRAM, University of Burgos, Burgos, Spain
Aspergillus niger is an industrially important source for gluconic acid and glucose oxidase (GOx), a secreted commercially important flavoprotein which catalyses the oxidation of β-D-glucose by molecular oxygen to D-glucolactone and hydrogen peroxide. Expression of goxC, the GOx encoding gene and the concomitant two step conversion of glucose to gluconic acid requires oxygen and the presence of significant amounts of glucose in the medium and is optimally induced at pH 5.5. The molecular mechanisms underlying regulation of goxC expression are, however, still enigmatic. Genetic studies aimed at understanding GOx induction have indicated the involvement of at least seven complementation groups, for none of which the molecular basis has been resolved. In this study, a mapping-by-sequencing forward genetics approach was used to uncover the molecular role of the goxB locus in goxC expression. Using the Illumina and PacBio sequencing platforms a hybrid high quality draft genome assembly of laboratory strain N402 was obtained and used as a reference for mapping of genomic reads obtained from the derivative NW103:goxB mutant strain. The goxB locus encodes a thioredoxin reductase. A deletion of the encoding gene in the N402 parent strain led to a high constitutive expression level of the GOx and the lactonase encoding genes required for the two-step conversion of glucose in gluconic acid and of the catR gene encoding catalase R. This high constitutive level of expression was observed to be irrespective of the carbon source and oxidative stress applied. A model clarifying the role of GoxB in the regulation of the expression of goxC involving hydrogen peroxide as second messenger is presented.
Introduction
Aspergillus niger is a highperformance microbial cell factory and used for cost effective production of food additives, pharmaceuticals and industrial enzymes. Having the abilities of a natural tolerance toward acid media as well as a high secretory capacity, A. niger is exploited as a cell factory to produce citric and gluconic acid (Meyer et al., 2011). Being saprobic, it is additionally equipped with versatile catabolic properties that make it grow on many kinds of, often complex, organic waste materials under various environmental conditions.
A. niger strain N402 (ATCC 64974) is a laboratory work horse strain that has been extensively used as a master strain for the generation of regulatory and structural mutants, with the aim to provide genetic insight in the molecular mechanisms underlying its extraordinary abilities. Additionally, the strain has been frequently used as model cell factory for overproduction of endogenous and heterologous enzymes (Lubertozzi and Keasling, 2009), to improve production of green chemical building blocks such as succinic acid, malic acid (Meyer et al., 2011), and itaconic acid (van der Straat et al., 2014), and as a model system to study the roles and regulation of individual sugar transporters in the catabolism of plant material feedstock components (Sloothaak et al., 2014, 2015, 2016a,b). Laboratory strain N402 is a derivative of the wild type strain N400 (CBS 120.49, NRRL3, ATCC 9029) and was obtained after two successive rounds of low-dosed UV mutagenesis (Bos et al., 1988; Swart et al., 2001). As a result, N402 showed a phenotype different from its origin, notably the development of shorter conidiophores (cspA1), a property that makes this strain much more amendable to genetic manipulations in a laboratory setting than wild type strains.
Organic acid production by Aspergillus species is tightly linked to biotechnological production of commodity chemicals. A. niger strain N400 was originally characterized as an efficient gluconic acid producer (Blom et al., 1952), a multifunctional carbonic acid that is used as a bulk chemical in the food, pharmaceutical, and cement industries (Singh and Kumar, 2007; Yang et al., 2017). Gluconate formation by A. niger occurs extracellularly and therefore does not require the involvement of intracellular metabolic pathways. Nevertheless, accumulated genetic evidence (Swart et al., 1990; Witteveen et al., 1992) suggested that the regulation of GOx expression is a complex trait implicating at least seven complementation groups and that hydrogen peroxide, a product of the first step of GOx mediated biotransformation of glucose to gluconic acid, acts as a possible signaling metabolite for GOx induction (Witteveen et al., 1993). As the underlying molecular mechanisms are still not understood we aimed to apply forward genetics as an approach to identify genes responsible for this trait.
Unraveling a polygenic trait by forward genetics requires the generation of a correspondingly large set of mutants, a well-defined genetic background, and laborious complementation tests to group and genetically map them to a locus. A mapping-by-sequencing method can then be applied to identify the causal genes for further study (Schneeberger, 2014). While for the GOx system the genetic requirements have been met in the past (Swart et al., 1990; Witteveen et al., 1992, 1993) and even though, strain N402 is universally applied in Aspergillus research, its genome information has not yet been published. In this study, a high-quality draft of the N402 genome sequence was obtained by using a hybrid of Illumina and PacBio RS II sequencing techniques. Phenotypic analysis of GOx overproducing mutants indicated a pivotal role of the goxB locus as strains NW102:goxB12 and NW103:goxB21 showed GOx expression virtually independent of the carbon source, at a low pH (Swart et al., 1990) and under conditions of low oxygen availability (Witteveen et al., 1993). Using a mapping-by-sequencing approach the gene linked to the goxB locus, was identified.
The effect of a subsequent deletion in the parental strain of the goxB gene, encoding a thioredoxin reductase, was further evaluated by plate assay and transcriptional analysis using different oxidative stresses and carbon sources. Deletion of the thioredoxin reductase encoding gene led to the goxB phenotype: a high constitutive expression of the genes coding for GOx, lactonase A (EC 3.1.1.17), and catalase R (EC 1.11.1.6). A model clarifying the role of the goxB locus in the regulation of GOx expression, involving hydrogen peroxide as second messenger, is presented.
Materials and Methods
Strains and Growth Conditions
A. niger N402 (cspA1) (Bos et al., 1988) has been used as master strain for the acquisition of gox mutant strains. A. niger NW102 (goxB12, cspA1, pabA1) and NW103 (goxB21, cspA1, pabA1) harbor the goxB mutations. A. niger strain MA169.4 (kusA::DR-amdS-DR, pyrG−) is descendant of N402 and was used as recipient strain in the knock-out transformation experiment (Carvalho et al., 2010) using the pyrG gene from A. oryzae as a selectable marker gene. The pWay-pyrA plasmid was used as a control for transformation. A. niger strains were maintained on complete medium containing 1 g/L casamino acid, 5 g/L yeast extract, 1% glucose, 20 mL/L ASPA + N, 1 mL/L Vishniac solution, and 1 mM MgSO4 at 30°C (Arentshorst et al., 2012).
Isolation of Genomic DNA of A. niger for Whole Genome Sequencing
Mycelial biomass of strains N402 and NW103 was obtained by growth in 100 mL complete medium at 30°C and 200 rpm for 40 h, washed with sterile demi water and dried. The dried mycelium was snap frozen in liquid nitrogen and grinded in liquid nitrogen into a fine powder with a pre-chilled mortar. The powder mycelium was re-suspended in 0.8 mL DNA extraction buffer (0.1 M Tris-HCl, pH 8.0, 1.2 M NaCl, and 5 mM EDTA) and mixed by vortexing. Next, 15 μL of RNase A (10 mg/mL) was added and the solution was incubated at 65°C for 30 min. followed by phenol:chloroform:isoamylacohol (25:24:1) extraction and isopropanol precipitation. Genomic DNA was re-suspended in 50 μL of milliQ water. The concentration was determined using the Qubit® RNA HS Assay Kit (Thermo Fisher Scientific, MA, United States).
Genome Sequencing, Assembly, Annotation, and Bioinformatics
Sequencing
Whole genome sequencing of strain N402 was performed by a commercial vendor (Novogene Bioinformatics Technology Co. Ltd, Beijing, China.) using Illumina HiSeq (300 bp inserts library with 150 bp paired-end sequencing; Illumina, San Diego, CA, United States) and PacBio RS II platform (10 kb inserts library; Pacific Biosciences, Menlo Park, CA, United States).
Assembly
A de novo IDBA_UD (Peng et al., 2012) based assembly pipeline A5 v20160825 (Coil et al., 2015) was used to assemble the HiSeq reads into contigs. Scaffolding on the contigs was performed with the AHA Long Read v1 protocol in the PacBio SMRT Analysis v2.3.0.140936 software suite. The following parameter setting were used: Normal Coverage, Gapfill true, 6 iterations. This was followed by another scaffolding round using SSPACE Longread v1-1 (Boetzer and Pirovano, 2014) with a Maximum link ratio of 0.1. MEDUSA v1.6 (Bosi et al., 2015) for reference scaffolding with A. niger ATCC 1015 strain as reference. Bowtie2 v2.3.0 (Langmead and Salzberg, 2012), samtools v1.3.1 (Li et al., 2009), and Pilon v1.21 (Walker et al., 2014) with the HiSeq reads for automatic assembly improvement. Finally, low coverage (<10) and small size contigs (<500 bp) were removed. HiSeq and PacBio Reads were mapped with bowtie2 v2.3.0 on the scaffolds for manual inspection with Tablet v1.16.09.06 (Milne et al., 2013), breaking the scaffolds at locations with low quality coverage. QUAST v4.4 (Gurevich et al., 2013) was used or assembly quality assessment.
Annotation
For genome annotation, the N402 assembly was imported in SAPP semantic database (Koehorst et al., 2017). STAR v.2.5.2b (Dobin et al., 2013) was used for mapping RNAseq data from Odoni et al. (2017) on the assembly and BRAKER1 (Hoff et al., 2016) for unsupervised RNA-Seq-based gene prediction. Gene predictions were directly stored in the SAPP semantic database (Koehorst et al., 2017). Structural feature description was done using the GBOL ontology (van Dam et al., 2017) Functional genome annotation was done with a standalone version of interproscan v5.24.63.0 (Zdobnov and Apweiler, 2001) in direct interaction with the SAPP database using the pfam31 (Bateman et al., 2004) database. The raw reads and full genome sequence are available from ENA (accession numbers PRJEB21769 and GCA_900248155).
Variant Calling
bowtie2 v2.3.0, samtools v1.3.1, VarScan2 v2.3.9, (Koboldt et al., 2012) and Tablet v1.16.09.06 for manual inspection of SNPs between N402 and NW103.
SignalP4.1 (Petersen et al., 2011) was used to detect the signal peptide for secretion in the putative lactonase amino acid sequences. Clustal Omega (Sievers et al., 2011) was used for multiple sequence alignment of putative lactonases and fungal TrxR strains. The structure of the yeast thioredoxin reductase A chain was visualized using Cn3D (Wang et al., 2000).
Genetic Construction of the goxB/trxR Knock-Out Strains
Using the split-marker approach, the goxB/trxR gene was deleted from the genome of the MA169.4 strain (isogenic of N402), which is defective in the Non-Homologous End-Joining (NHEJ) pathway through a transiently silenced kusA gene (Arentshorst et al., 2015). A schematic representation of the experimental steps required can be found in Supplementary Figure S1. Correct deletion of the gene was confirmed with PCR. Primers used are listed in Supplementary Table S1.
Protoplast Preparation and Transformation
A protoplast-mediated transformation of A. niger was performed by adapting the protocol of Arentshorst et al. (2012). The spore at 1 × 106 spores/mL medium was inoculated in 250 mL complete medium with 10 mM uridine in a 1 L erlenmeyer flask coated with 5% dimethydichlorosilane in heptane. The culture was incubated at 30°C and 100 rpm for 12 h. The mycelium was harvested by filtration through sterile miracloth and washed once with SMC buffer. Novozyme234 was used for protoplast formation (100 mg Novozyme234 per g of mycelium). Mycelium was incubated at 37°C and 80 rpm for 3 h. Protoplasts and mycelium were separated by filtration through the miracloth. The filtrate containing the protoplast was centrifuged at 2,000 g and 10°C for 10 min washed twice with 1 mL STC buffer and re-suspended in 1 mL STC buffer and aliquoted to 100 μL per reaction. After transformation, single A. niger transformant colonies were purified and the transiently silenced kusA gene was restored on MM plates containing fluoroacetamide (FAA) as described (Carvalho et al., 2010).
Glucose Oxidase Agar Plate Assay
Colonies were stained for glucose oxidase (GOx) using a modified method described by Witteveen et al. (1990). The solid assay medium contained 6 g/L, NaNO3, 1.5 g/L KH2PO4, 0.5 g/L KCl, 0.5 g/L MgSO4.7H2O, 1 mL/L Vishniac trace element solution, 12 g/L agar, and supplemented with 2.5 mM o-anisidine and one of the indicated carbon sources including 2 or 50 mM glucose, 50 mM fructose, 50 mM acetate, or 50 mM gluconate. The spores were diluted to 1 × 107 spores/mL and then 5 μL of spore suspension solution was dropped onto the agar plate. The agar plates were incubated at 30°C for 40 h. After incubating, the staining solution containing 20 mM sodium phosphate buffer, pH 7.0, 0.1 mM glucose and 20 μg/mL horseradish peroxidase was added into the plates and then incubated at room temperature for 4 h.
RNA Extraction and Transcriptional Analysis of the GOx System
For carbon source shift experiments, the wild type N402 and the ΔtrxR strains were pre-cultured at 1 × 106 spores/mL at 30°C and 200 rpm, during 18 h, in minimal medium containing 4.5 g/L NaNO3, 1.13 g/L KH2PO4, 0.38 g/L KCl, 0.38 g/L MgSO4.7H2O, 2 g/L casamino acid, 1 g/L yeast extract, 1 mL/L Vishniac trace element solution and supplemented with 100 mM sorbitol. Mycelium was harvested and then rinsed with equal amounts of water and transferred to minimal medium with either 100 mM sorbitol, 2 mM, and 50 mM glucose, 50 mM fructose, 50 mM acetate, or 50 mM gluconate. The initial pH of the medium in all condition was set at 6.0. After 4 h incubation, mycelium transfer samples were taken for RNA isolation, quickly washed, and then dried with a single-use towel, snap-frozen with liquid nitrogen and stored at –80°C until further processing. Two biological replicates per condition were studied in all cases.
Oxidative stress experiment was performed similar to the carbon sources experiment. The wild type N402 and the ΔtrxR strains were pre-cultured in minimal medium supplemented with 50 mM fructose. The water-rinsed mycelium was transferred to minimal medium supplemented with 50 mM fructose with the following oxidizing agents: 75 mM H2O2, 1.8 mM diamide, 0.8 mM menadione, and incubated at 30°C for 3 h. Concentrations of the stress initiating agents and exposure times were taken from (Pócsi et al., 2005).
Transcriptional analysis of A. niger genes: RNA of A. niger mycelium was isolated as described in Sloothaak et al. (2016b). Reverse transcription, quantitative PCRs and calculation were performed following the protocols and instrument described in Mach-Aigner et al. (2012). The previously described histone H4-like transcript (ATCC64974_101030, ATCC 1015 gene ID 207921) was used as reference for normalization of the expression data. Primer sequences are in Supplementary Table S1. Cycling conditions and control reactions were done as described previously (Steiger et al., 2010).
Results
Genome Sequencing of Strain N402
An overview of the bioinformatics workflow is depicted in Figure 1. The most recent common ancestor of the seven GOx complementation groups is strain N402. Since the N402 master strain was first selected for having the cspA1 phenotype, which could only be obtained after 2 consecutive rounds of UV mutagenesis and selection, we surmised that this trait may also be polygenic, and that the N402 master strain could have acquired multiple unlinked mutations which are directly passed on to the NW102 and NW103 goxB strains. As this could hamper molecular identification of specific loci, we first obtained a high-quality A. niger N402 draft genome sequence using a hybrid of Illumina and PacBio RS II sequencing, and subsequently mapped NW103 Illumina reads against the scaffolds, while focusing on linkage group II. An overview of the sequencing and assembly strategy is provided in the method section. As detailed in Table 1, the combination of the Illumina (150 nt paired end reads and ∼200 times coverage) and PacBio RS II sequencing technologies (library insert length 10 kbp and ∼30 times coverage) yielded a high quality 35,5 Mb draft genome. Three of the eight nuclear chromosomes seemed to be complete, as scaffolds spanning their entire length were obtained (Table 1).
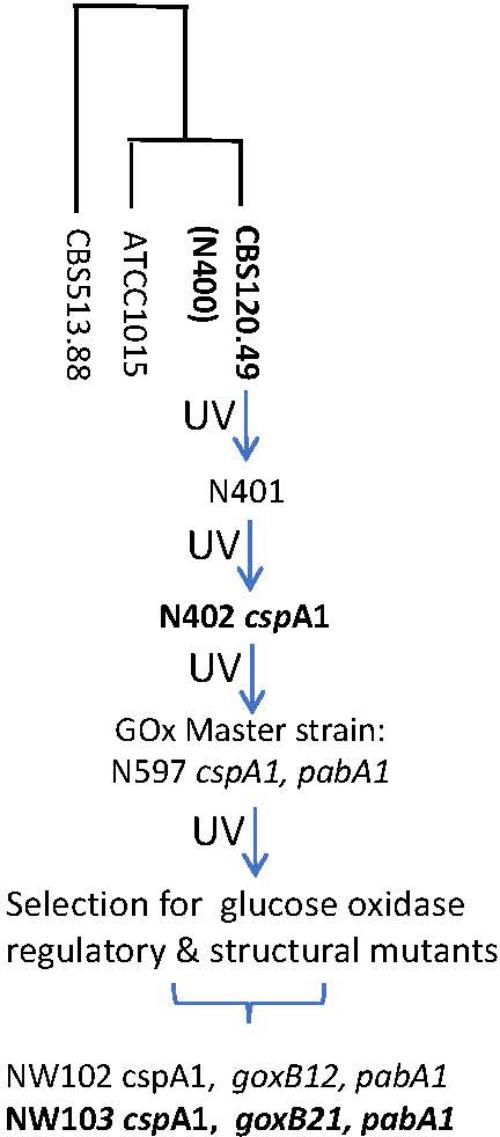
FIGURE 1. Pedigree of glucose oxidase regulatory and structural mutants of A. niger strain N400. For strains in bold genome sequence information was obtained. N400 derived genotypes are in italics; pabA1, p-aminobenzoic acid deficient. The “short conidiophores” cspA1 genotype was obtained after two rounds of UV mutation and selection and therefore most likely represents a complex trait. The construction of the GOx master strains have been described by Bos et al. (1988). The loci of glucose oxidase regulatory and structural mutants have been described by Swart et al. (1990). The genomes of strains CBS513.88 (Pel et al., 2007) and ATCC1015 (Andersen et al., 2011) are used for reference purposes. UV, UV mutagenesis.
A comparison of the N402 genome assembly with the genome assembly of the citric acid producer ATCC 1015 confirmed what already has been suggested based on limited sequence data: strain ATCC 1015 and N402 are nearly identical. Nevertheless, as can be seen in Figure 2 and Supplementary Table S2, there were sufficient differences justifying a RNAseq guided ab initio gene prediction. For this, the Braker1 (Hoff et al., 2016) tool was applied in combination with the AUGUSTUS eukaryotic gene prediction software (Stanke et al., 2008) and a mixed pool of N402 RNAseq data, obtained from various growth conditions (Odoni et al., 2017), as training set. The results of the ab initio predictions are summarized in Table 1. In total, from 11,236 gene models 11,285 transcripts were obtained suggesting that some genes may give rise to alternative spliced isoforms. A comparison of the N402 set of ab initio predicted genes with those of ATCC 1015 using a similar RNAseq guided approach (Reid et al., 2014) showed significant overlap between 10,915 predicted gene models with a complete congruity for 9,657 gene models. 318 of the 321 additionally predicted genes were also present in the ATCC 1015 genome assembly. Vice versa, from the 779 additional genes predicted in ATCC 1015 using the method of Reid et al. (2014), all but one were present in the N402 genome draft.
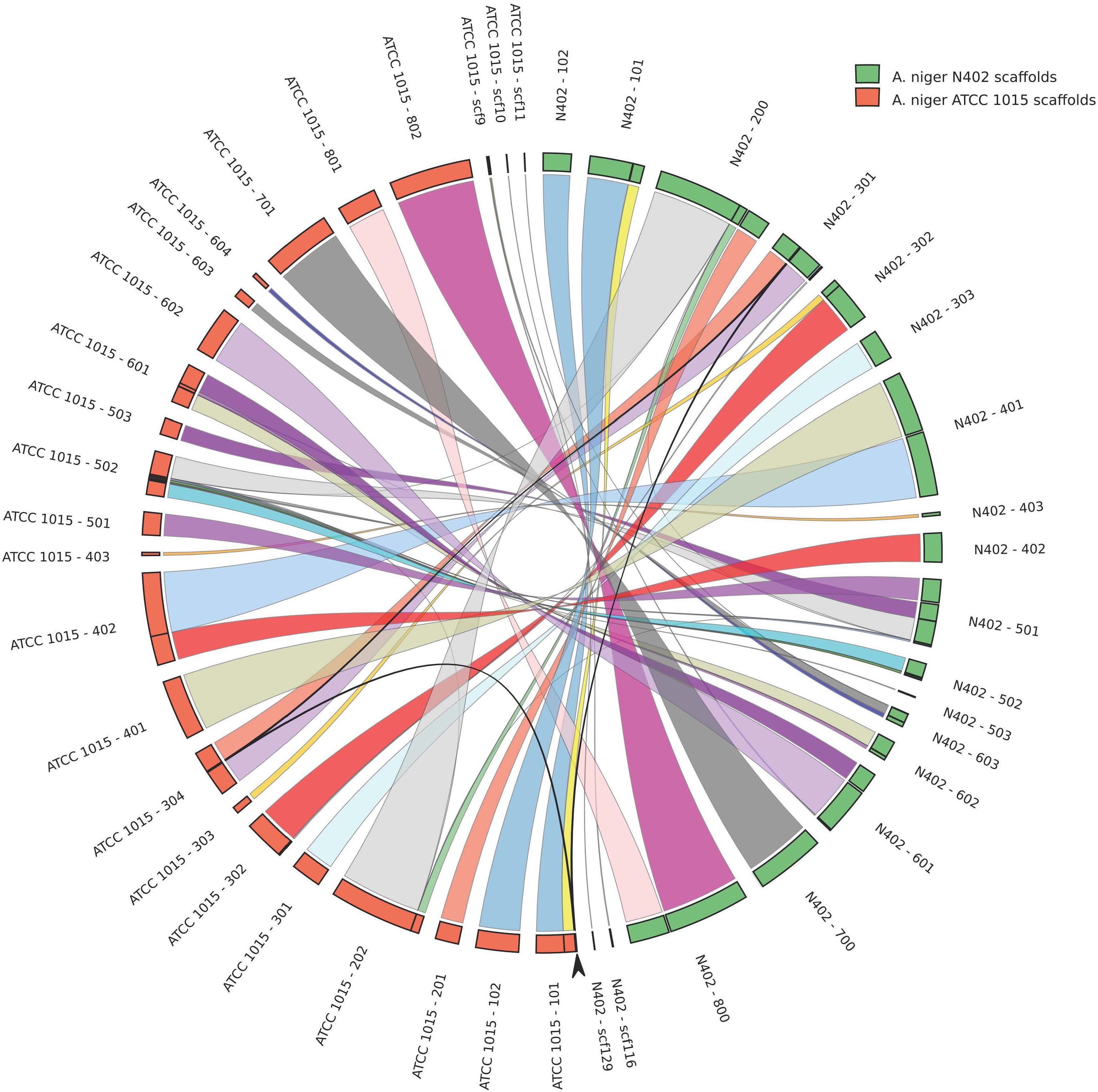
FIGURE 2. High levels of sequence identity and synteny between the proposed genome assemblies of Aspergillus niger strains N402 and ATCC 1015. Genome comparison is plotted in circos format (Krzywinski et al., 2009). Arrow indicates a 50.3 kb region present in chromosome 3 in the N402 and the ATCC1015 genome assembly coding for 21 predicted genes which is repeated on scaffold 101 in the proposed assembly of strain ATCC1015.
Functional Analysis of Chromosome 2
The goxB locus is located on chromosome 2 (Swart et al., 1990). This is also true for the goxC gene encoding GOx and the catR gene coding for a secreted catalase. Pfam domain predictions of N402 protein encoding genes (Supplementary File S3) revealed that N402 genome assembly codes for three putative lactonase (Pfam 10282) encoding genes. The protein products of two of them, which we named lctA (ATCC64974_11650) and lctB (ATCC64974_18030), have a predicted signal peptide for secretion and are located on chromosome 2. Additionally, they share more than 50% sequence similarity with a recently biochemically characterized and secreted aldonolactonase from Penicillium oxalicum, able to catalyze the hydrolysis of D-glucono-1,5-lactone to D-gluconic acid (Peng et al., 2017). Alignment details are provided in Supplementary Figure S2. The lctA encoding gene (ATCC64974_11650) is found immediate upstream of the goxC encoding gene (ATCC64974_11640) and divergently transcribed.
Identification of the goxB Mutation
Paired end Illumina genome sequence reads with a genome coverage of 15 were obtained from the goxB strain NW103. By mapping the NW103:goxB21 sequence reads to the N402 reference genome, potential regions of genomic variation could be observed with multiple chromosomes (see Supplementary Table S3 for details). However, since the two goxB mutants under study are mapped on chromosome II (Swart et al., 1990), protein coding regions of chromosome II were further investigated. One particularly convincing mapped mutation (see Supplementary Table S3 for details) suggested that the goxB locus might be tightly linked to a thioredoxin reductase (TrxR) encoding gene (ATCC64974_16500). TrxR is a flavoprotein with a catalytic selenocysteine residue and part of the two-component thioredoxin system. The thioredoxin system is responsible for maintaining a reducing intracellular environment by controlling the redox potential together with glutathione (GSH) and glutaredoxins. Apart from its function to reduce oxidized Trx, TrxR may also reduce other target proteins (Holmgren and Bjornstedt, 1995; Arnér and Holmgren, 2000).
From translation start to stop, the A. niger N402 trxR locus encompasses 1,243 nt and this includes two intron regions. The coding sequence translates into a 367 amino acids protein sequence. To confirm a tight linkage of goxB locus to the trxR gene, the genome sequences of trxR genes from goxB strains NW102:goxB12 and NW103:goxB21 were subjected to PCR amplification and Sanger sequencing. The results indicated that due to a mutation in NW102:goxB12, the serine residue present at position 214 was altered to proline while, consistent with the genome mapping results, in NW103:goxB21 the amino acid at position 168 was changed from serine to phenylalanine (Figure 3).
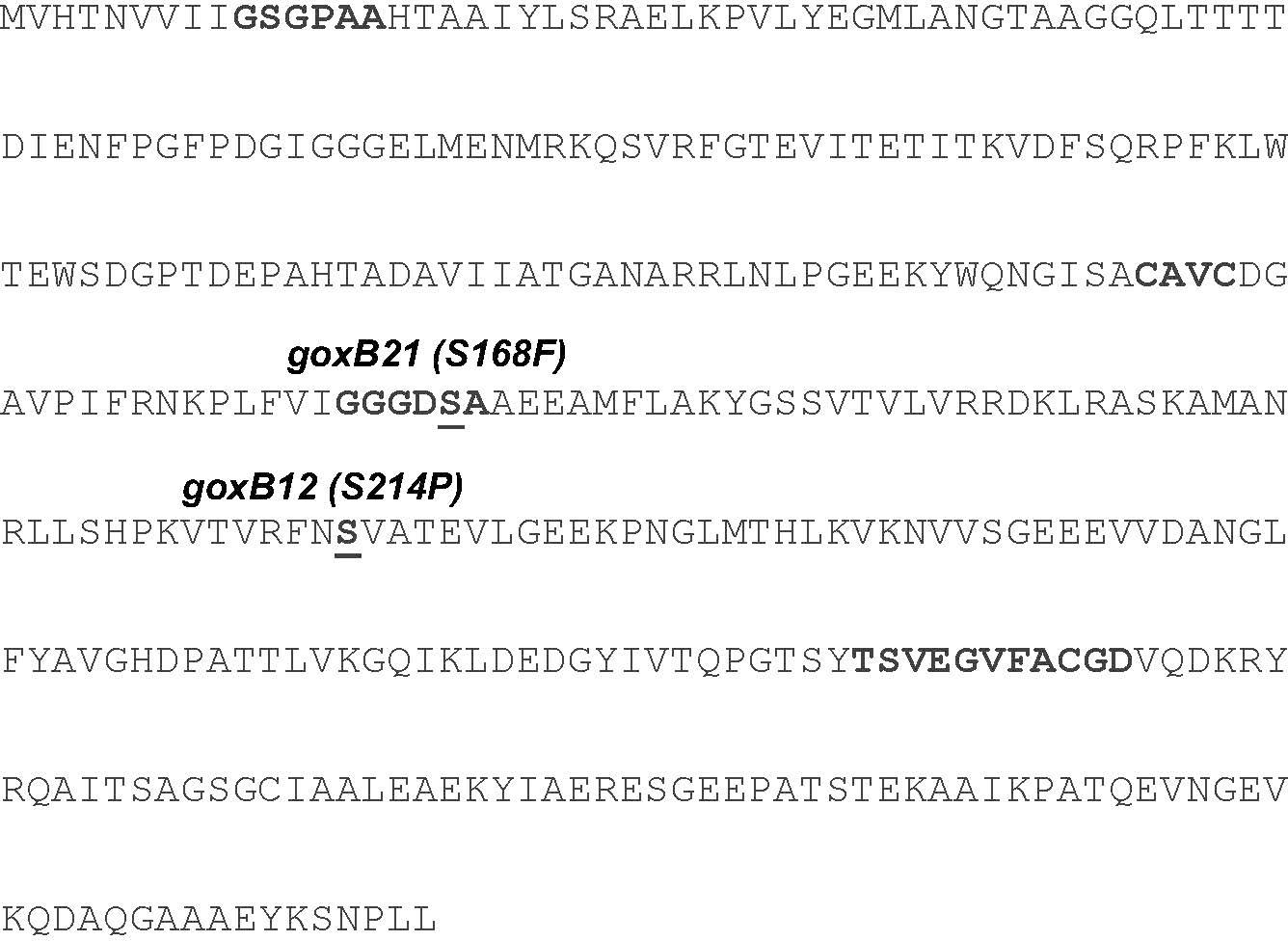
FIGURE 3. Missense mutations observed in the amino acid sequence of A. niger TrxR from two independent goxB strains. Missense mutations are indicated in parentheses. Three essential domains can be distinguished in TrxR: a redox-active cysteine pair (CAVC), NADPH-binding domain (GGGDSA), and the FAD-binding domain (GSGPAA and TSVEGVFACGD). Induced mutations are underlined.
Deletion of trxR Induces the goxB Phenotype
The forward genetics approach pinpointed to either a dysfunctional or nonfunctional thioredoxin reductase as cause of the goxB phenotype. To understand the molecular consequences of a trxR deletion, the trxR gene was replaced by the selection marker pyrG from A. oryzae. Two independent ΔtrxR strains labeled ΔtrxR-1A and ΔtrxR- 3A were selected for further study. First, a GOx plate assay was used to monitor GOx activity of the wild type, the two goxB mutants and the two ΔtrxR strains under normal GOx inducing and non-inducing conditions. The wild type control, the two mutants and the two ΔtrxR strains were grown on agar plates containing the following previously tested carbon sources: glucose, fructose, acetate, and gluconate. Compared to the wild type strain the knock-out strains showed a slightly reduced l growth and a normal morphology. Colonies were stained for GOx activity 40 h after incubation (Figure 4). Demonstrating the normal GOx activity patterns under these conditions (Swart et al., 1990) the wild type strain N402 colored noticeably only when grown on a 50 mM glucose plate but no visual GOx staining could be observed with 2 mM glucose as carbon source. Likewise, no GOx activity was found when N402 was grown with other carbon sources, while the two ΔtrxR strains successfully reproduced the goxB phenotype: a high level of GOx activity at high and at low glucose concentration as well as under GOx non-inducing conditions, using either fructose, acetate or gluconate as carbon source. From studies with S. cerevisiae, it is known that lack of the thioredoxin system blocks sulfate assimilation (Draculic et al., 2000) suggesting that the (slight) reduction in growth observed for the ΔtrxR strains could be overcome by adding an organic sulfur source. Supplementing solid assay medium with 5 mM methionine indeed had a positive effect on growth of the ΔtrxR strains but there was no effect on the goxB strains indicating that indeed both goxB mutants have a dysfunctional rather than a nonfunctional thioredoxin reductase (Supplementary Figure S5).
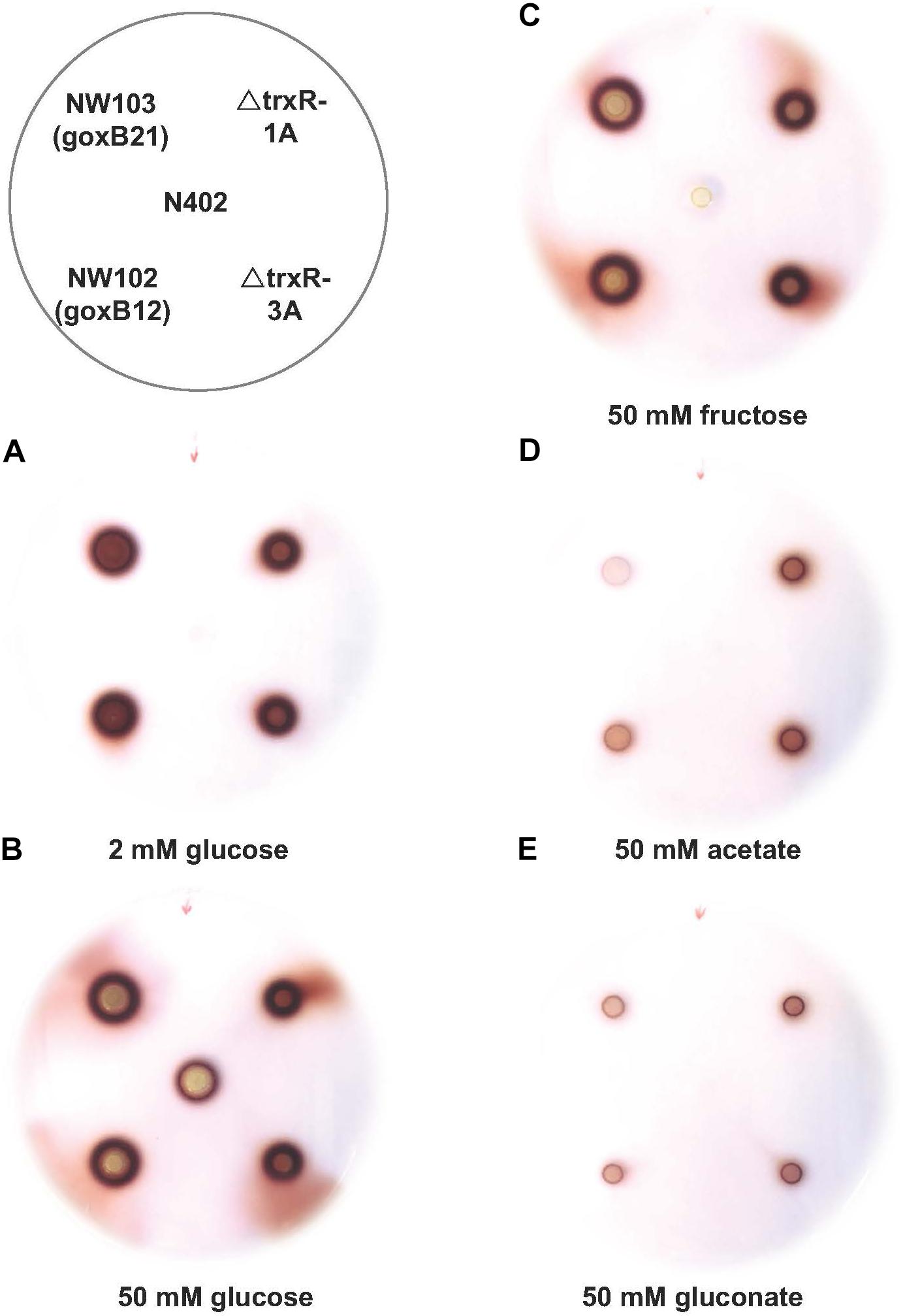
FIGURE 4. Phenotypic characterisation of trxR gene knock-out strains. Glucose oxidase plate assay of A. niger strain N402, NW102:goxB12, NW103:goxB21, ΔtrxR-1A, and ΔtrxR-3A on various carbon sources: (A) 2 mM glucose, (B) 50 mM glucose, (C) 50 mM fructose, (D) 50 mM acetate, and (E) 50 mM gluconate.
Transcriptional Response of goxC, lctA, and catR in Oxidative Stress Conditions
In the GOx mediated bioconversion of glucose to D-glucono-1,5-lactone, hydrogen peroxide is formed in equimolar amounts. Witteveen et al. (1993) observed that in the absence of glucose, addition of hydrogen peroxide to the medium resulted in the induction of GOx, lactonase, and catalase activities in the wild-type strain. Hydrogen peroxide is not only a non-radical reactive oxygen species (ROS), but can also function as second messenger through reversible H2O2-induced oxidative activation of thiol proteins, and one role of the thioredoxin system is to reverse H2O2-induced activation (reviewed by Sies et al., 2017). To determine the effects of the absence of this antioxidant system on the expression of goxC, lctA, and catR, the wild type and knock-out strains were monitored by RT-qPCR in the presence of three different oxidative stress-inducing agents, used to generate ROS: H2O2, diamide and menadione. Hydrogen peroxide increases the intracellular peroxide (O22−) level and menadione generates superoxide anion (O−), while diamide is a thio-oxidizing agent resulting in fast oxidation of GSH to glutathione disulphide (GSSG), that leads to a GSH/GSSG redox imbalance. To monitor the physiological response of A. niger to the oxidative stress applied, two ROS responsive marker genes were included in the RT-qPCR analysis; gstA coding for glutathione-S-transferase A and sodA, coding for superoxide dismutase A. In A. nidulans, sodA gene expression is responding solely to menadione treatment (Pócsi et al., 2005). Cells were pre-grown with fructose as carbon source. Then, either H2O2 (Figure 5), diamide or menadione (Supplementary Figure S3), were added to the medium, and cultures were exposed for 3 h to each of the oxidative agents. Figure 5 displays the effect of a trxR knock-out on the expression of the genes in the normal culture conditions. In absence of oxidative stress; in the two ΔtrxR strains significantly higher expression levels were obtained for all.
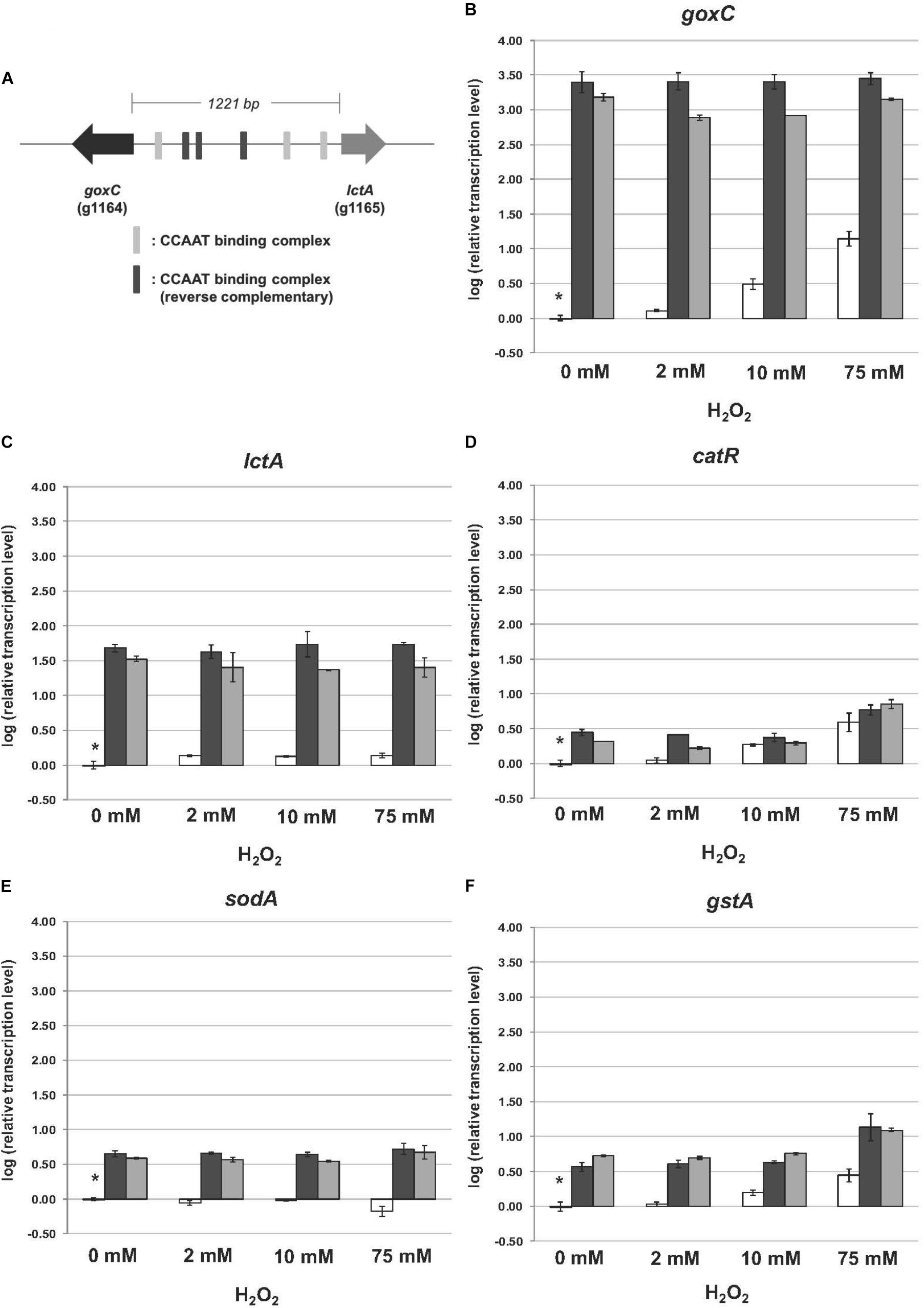
FIGURE 5. Expression of the glucose oxidase, lactonase, catalase, superoxide dismutase, and glutathione-S-transferase encoding genes upon hydrogen peroxide addition. Samples were taken 3 h after mycelium transfer to minimal medium with different concentrations of hydrogen peroxide (0–75 mM). (A) Genomic organization of goxC and lctA on chromosome II. The six putative CCAAT boxes are indicated in gray. (B–F) Expression analysis performed by RT-qPCR with the primers specific for goxC (B), lctA (C), catR (D), sodA (E), and gstA (F) gene. White bars represent expression levels obtained in strain N402, black bars in ΔtrxR-1A and gray bars in ΔtrxR-3A. Normalization of the expression data was done using the histone 4-like gene transcript. Results were calculated as relative transcript ratio in logarithmic scale (log10) with means of two biological replicates. Asterisks represents transcript level of the reference sample.
Regarding the A. niger response to the presence of increasing concentrations of hydrogen peroxide, we observed different transcriptional responses between the five studied genes in the N402 strain. In the wild type strain, the mRNA levels of goxC, catR, and the ROS responsive marker gene gstA increased along with the increased H2O2 concentration present in the culture media. The lctA gene was also induced in the presence of hydrogen peroxide, but transcriptional levels were not increasing along with the increased H2O2 concentration present in the culture media. In contrast, the ROS responsive marker gene sodA, was not upregulated upon hydrogen peroxide addition, and even showed a certain degree of downregulation in the presence of the high concentration (75 mM) of the oxidizing agent in the N402 strain. Regarding the two ΔtrxR strains, the expression level of goxC and lctA showed to be much higher than in the N402 strain, in any of the culture conditions, but no further difference in mRNA accumulation levels were observed between oxidative stress and non-inducing conditions. Deletion of the trxR gene also influenced the regulation of catR and gstA, In the absence of exogenous hydrogen peroxide, catR and gstA showed, compared to the wild-type, higher transcriptional levels in the ΔtrxR strains. The absence of trxR also influenced the regulation of catR and gstA, which also showed higher transcriptional levels in the mutant strains. However, in the ΔtrxR strains their expression was still further increased in the presence of 75 mM of hydrogen peroxide.
The presence of diamide (1.8 mM) and menadione (0.8 mM) in the media also had an effect in the transcriptional response of the studied genes in the N402 strain. All of them were transcriptionally activated in the presence of both oxidative agents (Supplementary Figure S3). In response to diamide and menadione the goxC gene showed a similar response to that observed in the presence of 75 mM hydrogen peroxide, while lctA showed higher expression levels with diamide and menadione. The gstA gene had a similar transcriptional response in the presence of 75 mM H2O2 and diamide, but as previously reported (Pócsi et al., 2005), higher transcriptional levels were achieved in the menadione condition. The mRNA accumulation levels of catR showed some variation between the three oxidative conditions, and showed a higher transcriptional response in the menadione condition too. The sodA gene showed an upregulation in expression in response to menadione, and in contrast to the response in A. nidulans, also responded to diamide. Regarding the response of the ΔtrxR strains to the oxidative stress produced by diamide and menadione, goxC, and lctA continued to be clearly upregulated when compared to their expression in the reference strain. This was also the case for catR and gstA in the presence of diamide. However, the expression of these two latter genes in the menadione condition was at the same level in the N402 and ΔtrxR transformants.
Transcriptional Analysis of goxC, lctA, and catR in the Presence of Different Carbon Sources
Mycelium transfer experiments were used to study carbon source induced transcriptional changes of goxC, lctA, and catR. Mycelium was pre-grown in sorbitol and transferred to cultures containing minimal medium supplemented with either a low (2 mM) or high (50 mM) concentration of glucose, fructose (50 mM), acetate (50 mM), gluconate (50 mM), and sorbitol (100 mM). Samples for RT-qPCR analysis were taken 4 h after mycelium transfer.
In the N402 strain, the transcript levels of the goxC gene were significantly increased upon a carbon source shift to 50 mM acetate, and in 4 h only slightly induced when the carbon source was shifted to 50 mM glucose. Upon a shift to 50 mM fructose and 2 mM glucose, goxC transcript levels remained similar to those observed in the reference condition (Figure 6). In addition, goxC transcript levels showed to be slightly downregulated in the gluconate condition. No significant changes in expression level were observed for catR and lctA.
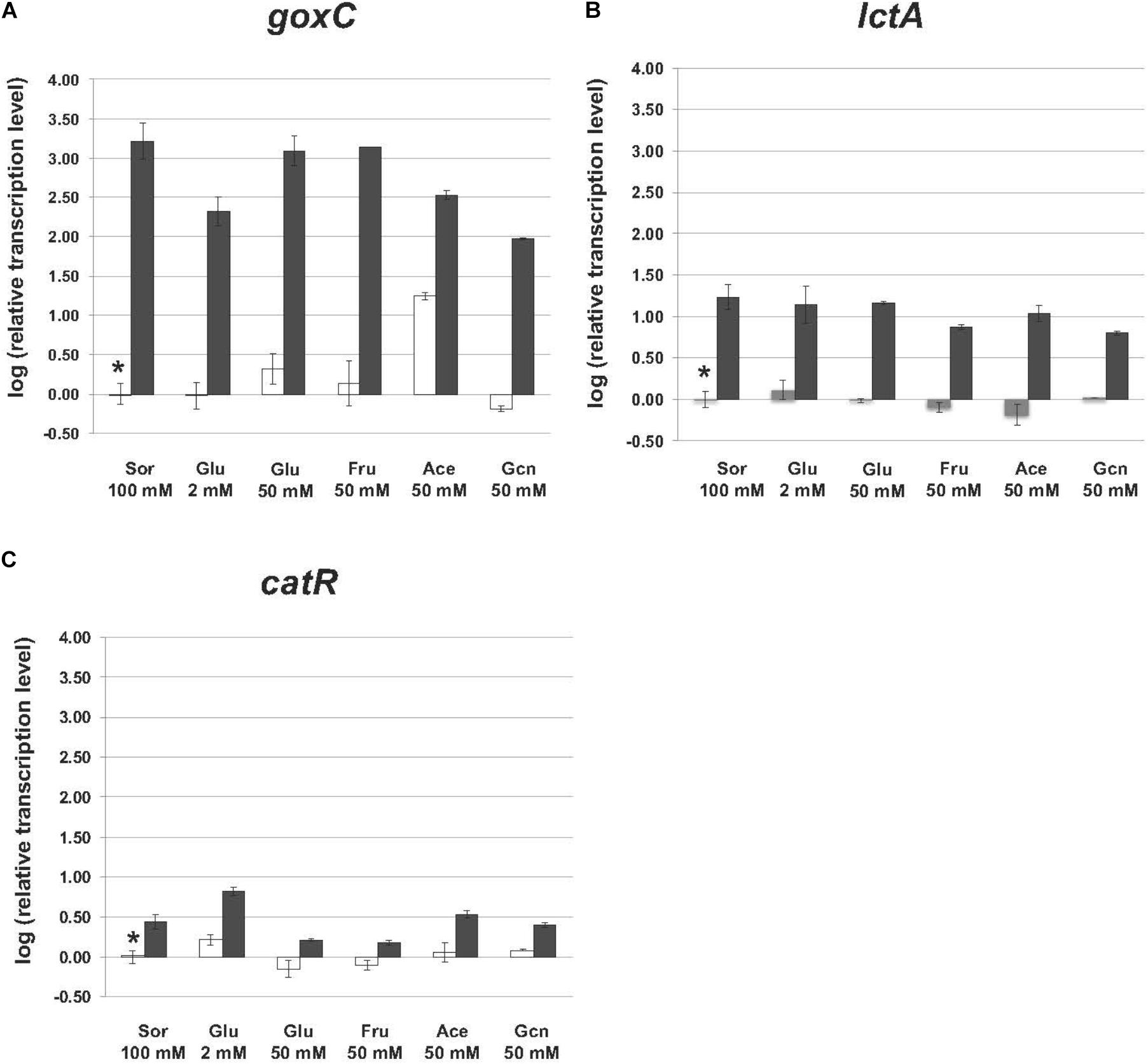
FIGURE 6. Expression of the glucose oxidase and lactonase, encoding genes of A. niger strain N402 and ΔtrxR-1A in various carbon sources. Samples were taken 4 h after mycelium transfer to the medium with different carbon sources including glucose (Glu), fructose (Fru), acetate (Ace) gluconate (Gcn), and sorbitol (Sor). Expression analysis was performed by RT-qPCR with the primers specific for goxC (A), lctA (B), and catR (C) gene. White bars indicate expression levels obtained in strain N402, black bars in ΔtrxR-1A and gray bars in ΔtrxR-3A. Normalization of the expression data was done using the histone 4-like gene transcript. Results were calculated as relative transcript ratio in logarithmic scale (log10) with means of two biological replicates. Asterisks represents transcript level of the reference sample.
In agreement with the result observed in the GOx plate activity assays, in the ΔtrxR strain, goxC, and lctA transcriptional levels remained highly upregulated in all carbon conditions studied. This was also the case for catR, which showed higher mRNA levels in the mutant strains.
Discussion
The power of forward genetics is that it starts with a phenotype of interest. In an unbiased genetic screen Swart et al. (1990) identified nine independent loci on at least four different chromosomes, all interfering with the normal pattern of GOx expression. While GOx functions outside the cell, and is therefore uncoupled from the internal metabolism, seven GOx complementation groups have in common that they lead to unregulated GOx activities suggesting that GOx expression is complex, involving multiple factors and apparently tightly regulated. As the molecular basis of none of these factors was known, a mapping by sequencing approach was employed. In principle, next-generation sequencing provides a powerful way to efficiently identify artificially induced mutations in genomes. However, a statistically relevant identification of possible nucleotide mutations requires a high-quality genome draft of the immediate parent strain, and given the inherent base-calling error rates of high-throughput sequence mapping data (Quail et al., 2012), defined loci to efficiently search for such mutations. While the genetic criteria have been met, a high-quality genome draft of the immediate parent was not available and was therefore obtained using current standard procedures; a hybrid assembly strategy, mixing long, and short reads of sufficiently high coverage.
Comparison of ATCC 64974 (N402) and ATTC 1015 Whole Genome Assemblies
The newly obtained genome assembly from the N402 strain was compared with the nearest published genome sequence of A. niger strain ATCC 1015 (Andersen et al., 2011). Strain N402 is a descendent of the wild type strain N400 and frequently used as a laboratory strain as it carries the cspA1 mutation making it more suitable for laboratory manipulations. Overall, there is very high level of sequence identity (Supplementary Table S2). Having said that, we also observed a 50 kb duplication in the ATCC 1015 assembly between chromosome 1 and 3 not present in the N402 assembly (Figure 2), single and multiple, consecutive nucleotide differences, and multiple small gaps and insertions (Supplementary Table S2). Note that for the two strains different strategies were used for genome sequencing and assembly and therefore many of these differences may be due to technical issues.
Although, not the aim of this study, with such a high level of sequence identity, it is tempting to speculate on the possible genomic locations of loci that may contribute to the cspA1 phenotype. In that respect, the most promising difference between the ATCC 1015 wild type strain and the N402 cspA1 strain is an ∼9 kb deletion in N402 scaffold 301, at nucleotide position 1.037.003. The deletion in the N402 assembly is responsible for deleting the 3-prime half of gene ATCC64974_3000 (incomplete N402 version) encoding the C-terminal half of a 1641 amino acid protein (Pubmed protein_id EHA24670) harboring three kinesin associated Pfam domains (pfam00225; pfam12473; pfam16183). Kinesin family proteins have important roles in intracellular transport and in cell division. In aspergilli, the encoded gene is ubiquitous present and appears to be a single copy gene. Additionally, the 9 kb deletion in strain N402, deletes a gene coding for a hypothetical protein (Aspni3p7_00651; corresponding protein id CAK42436.1) that seems to be unique for A. niger. It should be noted that two rounds of low dosed UV mutagenesis and selection were required to obtain the cspA1 phenotype. While, based on our N400 Illumina genome sequence data, the 9 kb sequence gap is non-existent in the N400 parental strain, and the kinesin like protein appears to be a promising candidate, other less obvious mutations may play an equally important role.
A Model for GOx Regulation
Regarding goxB, previous studies indicated that A. niger strains carrying mutations in this locus (NW102:goxB12 and NW103:goxB21) resulted in constitutive expression, modulated to some extent by the carbon source, of three enzyme activities: GOx, lactonase and catalase (Witteveen et al., 1990, 1993). In a forward genetic screen the goxB21 locus in NW103 could be linked to a thioredoxin reductase coding gene (trxR). Missense mutations in this gene, which could compromise the TrxR enzyme function, were found in both goxB strains.
The thioredoxin system of A. nidulans, composed thioredoxin (TrxA) and thioredoxin reductase (TrxR) has been biochemically fully characterized (Thön et al., 2007). The role of TrxA is to reduce other proteins by cysteine thiol-disulfide exchange. The oxidized form of TrxA is subsequently reduced by TrxR using NADPH as electron donor and its own redox-active cysteine pair, and FAD as cofactor. A. niger TrxR shows 85% sequence identity with A. nidulans TrxR and 65% identity with the two yeast thioredoxin reductases. In NW103:goxB21, the mapped mutation changed the polar serine residue 168 into an aromatic and much larger phenylalanine residue.
A multiple alignment of A. niger N402 TrxR with a selection of other low molecular weight fungal thioredoxin reductases (Supplementary Figure S4) indicated that Ser168 is strongly conserved and part of the NADPH-binding domain (GGGDSA) which suggests a direct interference with NADPH binding. Although, the goxB12 and goxB21 mutations have a similar phenotype for GOx expression the impact of the Ser214Pro mutation is less clear. There is a lower level of sequence conservation for this particular region and the particular serine can also be threonine. We further investigated the consequences of this mutation at structural level by evaluating the equivalent position in the available yeast structure (Oliveira et al., 2010) (Supplementary Figure S4) which suggested that changing serine 214 into a more rigid proline may have serious structural consequences.
TrxA and TrxR comprise a thioredoxin system. While the goxB/trxR mutations in A. niger NW102 and NW103 did not show an severe growth phenotype (Supplementary Figure S5), a trxA deletion in A. nidulans showed a decreased growth, an inability to form reproductive structures and a high sensitivity toward added hydrogen peroxide (Thön et al., 2007). To exclude that the goxB mutations represented a dysfunctional rather than a nonfunctional TrxR, two independent trxR knock-out strains were obtained and used (ΔtrxR-1A and ΔtrxR-3A) to study the knock-out phenotype. Once the deletion of trxR gene was confirmed in the ΔtrxR-1A and ΔtrxR- 3A strains, both of which showed no strong growth phenotype, a GOx activity plate assay confirmed that the ΔtrxR strains could successfully reproduce the goxB phenotype. By comparing on agar plates, growth of the goxB mutant strains with the ΔtrxR strains, both mutants appear to express a dysfunctional rather than a nonfunctional TrxR. Upon supplementation with 5 mM methionine, growth of the knock-out strain was stimulated and almost similar to the wild type strain N402 while we did not observe such a growth defect for the mutants. Upon deletion of TrxA in A. nidulans (Thön et al., 2007) could restore the growth defect by adding glutathione. We also tested glutathione and somewhat surprising there was no restoration of growth, however in all strains a delay of spore formation was observed (Supplementary Figure S5). Although, TrxR and TrxA are the two parts of the thioredoxin system, a direct comparison between a ΔtrxA in A. nidulans (Thön et al., 2007) and ΔtrxR in A. niger is difficult.
In order to further understand the pivotal role of TrxR in GOx regulation, a series of transcriptional analyses of goxC, lctA, and catR, relevant for the biotransformation of glucose to gluconic acid and the fungus oxidative stress response, were performed. In the absence of oxidative stress, these genes showed a basal expression level in the N402 strain. However, all of them, were upregulated in the ΔtrxR mutants, indicating a prominent role of the thioredoxin/thioredoxin reductase redox system in their transcriptional regulation. To study the role of the carbon source in regulation of expression of the GOx system carbon source shift experiments were used. In contrast to the immediate effect elicited by hydrogen peroxide addition, on the short term the expression level of the goxC gene was not significantly induced upon a substrate shift from sorbitol to glucose and most other carbon sources in the N402 strain. A shift to 50 mM acetate as carbon source, however, resulted in a 10-fold upregulation of the goxC gene expression. In yeast, acetate has been described as an agent able to produce oxidative stress involving the Yap1p transcriptional regulator (Semchyshyn et al., 2011).
The absence of the trxR gene affected strongly the expression of the goxC gene, in all carbon conditions tested. The gene was highly upregulated in the presence of sorbitol, both glucose concentrations, fructose, and gluconate, with differences in mRNA accumulation between N402 and ΔtrxR strains of around 2–3 orders of magnitude. The results obtained confirmed that the goxB mutation and knock-out (ΔtrxR) results in a constitutive expression of GOx, lactonase and catalase, although this may be modulated to some extent depending on the carbon source.
The presence of six CCAAT sequences present in the 1,221 bp intergenic region between the goxC and lctA gene (Figure 5A) and the direct involvement of H2O2, the second product of the conversion of glucose to D-glucolactone as signaling molecule for induction of the GOx system, supports a model of regulation of the GOx system involving positive and a negative feedback loops. Signal amplification is achieved via a positive feedback loop in which H2O2 targets the CCAAT-binding factor (AnCF) (Thön et al., 2010) and/or YAP-like basic leucine zipper (bZIP) transcription factor (NapA) (Marinho et al., 2014). In either case, H2O2 induced oxidative modification of thiol groups in these proteins leads to activation of goxC expression possibly through derepression (AnCF) and/or activation (NapA) (Thön et al., 2010). Simultaneously, in a negative feedback loop, ROS activation of the thioredoxin system leads to reactivation of AnCF activity and inactivation of NapA activity (Thön et al., 2010). In the presence of a sufficient supply of oxygen and glucose, increased GOx activity will lead to an increased turnover of glucose in gluconic acid and H2O2. Outside the cell, the effect of this positive feedback loop is counterbalanced by H2O2 induced expression of the catR gene. The catR encodes an extracellular catalase and a single CCAAT box is found 633 bp upstream of the catR start-site. Independent from this mechanism, the thioredoxin system is involved in the redox regulation of the AnCF/NapA controlled transcriptional activity, thereby making it a monostable system (Figure 7).
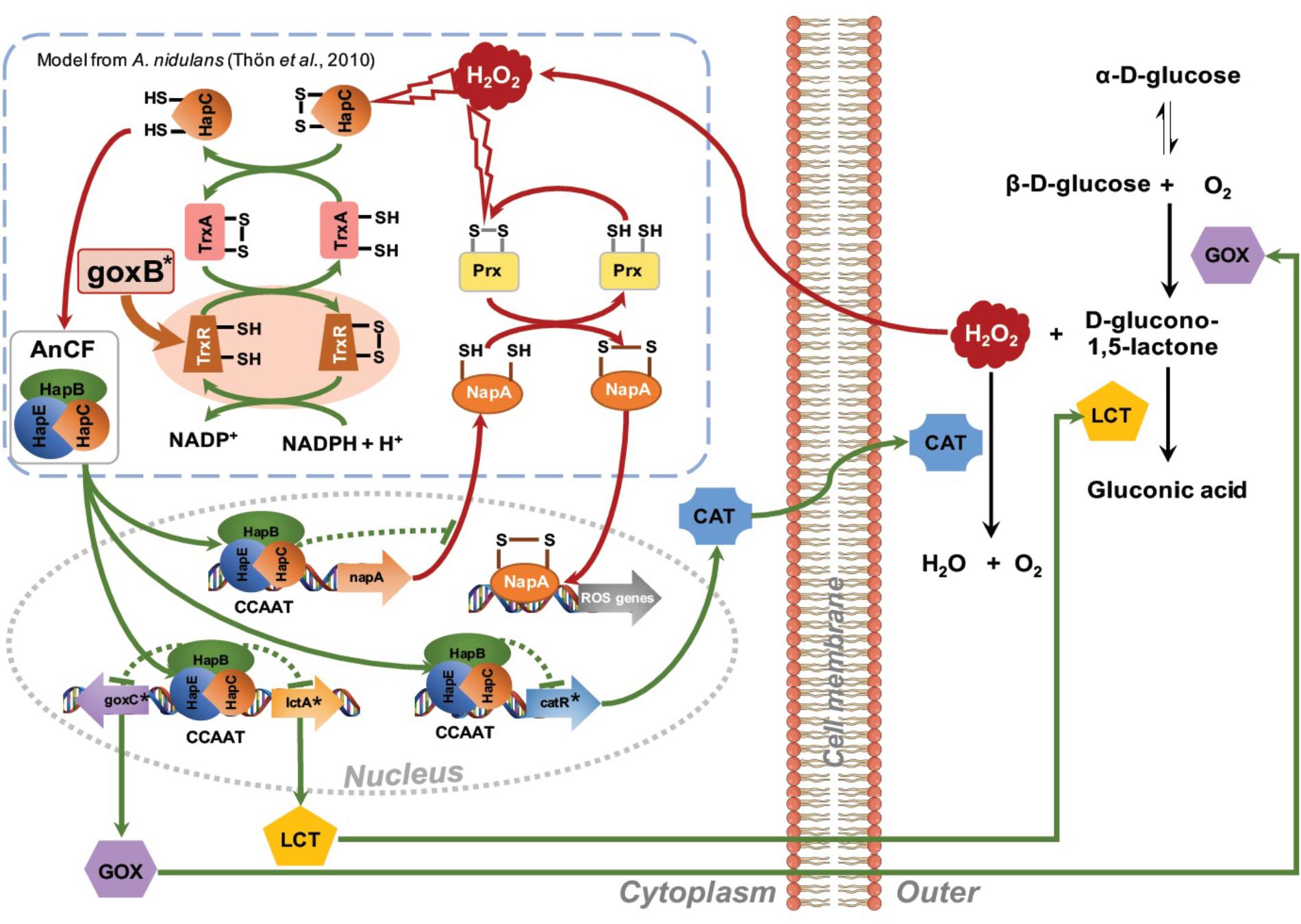
FIGURE 7. Proposed network for regulation of the glucose oxidase system. Amplification of the H2O2 signal is achieved via a positive feedback loop. H2O2 induced oxidative modification of thiol groups in ROS sensitive regulatory proteins such as AnCF complex (Thön et al., 2010) or NapA (Marinho et al., 2014) leads to activation of goxC expression. Outside the cell an increase GOx activity may lead to a further increase in turnover of glucose in gluconic acid and H2O2. The increased extracellular H2O2 concentration is counterbalanced by H2O2 induced expression of the catR gene, leading to an extracellular catalase activity. Simultaneously, in a negative feedback loop, ROS activation of the thioredoxin system facilitates a return to the native state. GOx specific genes are indicated with an asterisk.
The proposed model can explain the goxB phenotype and, the requirements of a sufficient amounts of oxygen and a high glucose concentration for induction of the GOx system. It should be noted, however, that this model is highly speculative and lacks many details on the exact nature of the element(s) involved in the regulation of goxC in A. niger. Along with goxC, ROS defense systems appear to be upregulated in the two goxB knockout strains (Figure 5). Currently it is, unclear whether this due to insufficient amount of reduced TrxA or results from a direct involvement of the compromised thioredoxin system in AnCF /NapA controlled transcriptional activity.
Author Contributions
TL and PS conceived and designed the work. TL performed the experiments. TL, BN, and PJ analyzed the data. TL, BN, JT-R, and PS contributed to the interpretation of the data. TL and PJ wrote the manuscript. TL, JT-R, and PS critically revised the manuscript for intellectual content. All authors have read and agreed to the submission of the manuscript.
Funding
TL was financially supported by a Royal Thai Government Scholarship and National Science and Technology Development Agency (NSTDA), Thailand.
Conflict of Interest Statement
The authors declare that the research was conducted in the absence of any commercial or financial relationships that could be construed as a potential conflict of interest.
Acknowledgments
We would like to thank Mark Arentshorst for providing us the A. niger MA169.4 strain. We also thank Tom Schonewille, and Dorett I. Odoni for their contribution to this work.
Supplementary Material
The Supplementary Material for this article can be found online at: https://www.frontiersin.org/articles/10.3389/fmicb.2018.02269/full#supplementary-material
References
Andersen, M. R., Salazar, M. P., Schaap, P. J., Van De Vondervoort, P. J. I., Culley, D., Thykaer, J., et al. (2011). Comparative genomics of citric-acid-producing Aspergillus niger ATCC 1015 versus enzyme-producing CBS 513.88. Genome Res. 21, 885–897. doi: 10.1101/gr.112169.110
Arentshorst, M., Lagendijk, E. L., and Ram, A. F. (2015). A new vector for efficient gene targeting to the pyrG locus in Aspergillus niger. Fungal Biol. Biotechnol. 2:2. doi: 10.1186/s40694-015-0012-4
Arentshorst, M., Ram, A. F. J., and Meyer, V. (2012). “Using non-homologous end-joining-deficient strains for functional gene analyses in filamentous fungi,” in Plant Fungal Pathogens: Methods and Protocols, eds M. D. Bolton and B. P. H. J. Thomma (Totowa, NJ: Humana Press), 133–150.
Arnér, E. S. J., and Holmgren, A. (2000). Physiological functions of thioredoxin and thioredoxin reductase. Eur. J. Biochem. 267, 6102–6109. doi: 10.1046/j.1432-1327.2000.01701.x
Bateman, A., Coin, L., Durbin, R., Finn, R. D., Hollich, V., Griffiths-Jones, S., et al. (2004). The Pfam protein families database. Nucleic Acids Res. 32, D138–D141. doi: 10.1093/nar/gkh121
Blom, R. H., Pfeifer, V. F., Moyer, A. J., Traufrer, D. H., Conway, H. F., Crocker, C. K., et al. (1952). Sodium gluconate production. fermentation with Aspergillus niger. Ind. Eng. Chem. 44, 435–440. doi: 10.1021/ie50506a061
Boetzer, M., and Pirovano, W. (2014). SSPACE-longread: scaffolding bacterial draft genomes using long read sequence information. BMC Bioinformatics 15:211. doi: 10.1186/1471-2105-15-211
Bos, C. J., Debets, A. J. M., Swart, K., Huybers, A., Kobus, G., and Slakhorst, S. M. (1988). Genetic analysis and the construction of master strains for assignment of genes to six linkage groups in Aspergillus niger. Curr. Genet. 14, 437–443. doi: 10.1007/BF00521266
Bosi, E., Donati, B., Galardini, M., Brunetti, S., Sagot, M.-F., Lió, P., et al. (2015). MeDuSa: a multi-draft based scaffolder. Bioinformatics 31, 2443–2451. doi: 10.1093/bioinformatics/btv171
Carvalho, N. D., Arentshorst, M., Jin Kwon, M., Meyer, V., and Ram, A. F. J. (2010). Expanding the ku70 toolbox for filamentous fungi: establishment of complementation vectors and recipient strains for advanced gene analyses. Appl. Microbiol. Biotechnol. 87, 1463–1473. doi: 10.1007/s00253-010-2588-1
Coil, D., Jospin, G., and Darling, A. E. (2015). A5-miseq: an updated pipeline to assemble microbial genomes from illumina MiSeq data. Bioinformatics 31, 587–589. doi: 10.1093/bioinformatics/btu661
Dobin, A., Davis, C. A., Schlesinger, F., Drenkow, J., Zaleski, C., Jha, S., et al. (2013). STAR: ultrafast universal RNA-seq aligner. Bioinformatics 29, 15–21. doi: 10.1093/bioinformatics/bts635
Draculic, T., Dawes, I. W., and Grant, C. M. (2000). A single glutaredoxin or thioredoxin gene is essential for viability in the yeast Saccharomyces cerevisiae. Mol. Microbiol. 36, 1167–1174. doi: 10.1046/j.1365-2958.2000.01948.x
Gurevich, A., Saveliev, V., Vyahhi, N., and Tesler, G. (2013). QUAST: quality assessment tool for genome assemblies. Bioinformatics 29, 1072–1075. doi: 10.1093/bioinformatics/btt086
Hoff, K. J., Lange, S., Lomsadze, A., Borodovsky, M., and Stanke, M. (2016). BRAKER1: unsupervised RNA-seq-based genome annotation with geneMark-ET and AUGUSTUS. Bioinformatics 32, 767–769. doi: 10.1093/bioinformatics/btv661
Holmgren, A., and Bjornstedt, M. (1995). “Thioredoxin and thioredoxin reductase,” in Methods in Enzymology, ed. L. Packer (Cambridge, MA: Academic Press), 199–208. doi: 10.1016/0076-6879(95)52023-6
Koboldt, D. C., Zhang, Q., Larson, D. E., Shen, D., Mclellan, M. D., Lin, L., et al. (2012). VarScan 2: somatic mutation and copy number alteration discovery in cancer by exome sequencing. Genome Res. 22, 568–576. doi: 10.1101/gr.129684.111
Koehorst, J. J., Dam, J. C. J., Saccenti, E., Martins Dos Santos, V. A. P., Suarez-Diez, M., and Schaap, P. J. (2017). SAPP: functional genome annotation and analysis through a semantic framework using FAIR principles. Bioinformatics 34, 1401–1403. doi: 10.1093/bioinformatics/btx767
Krzywinski, M. I., Schein, J. E., Birol, I., Connors, J., Gascoyne, R., Horsman, D., et al. (2009). Circos: an information aesthetic for comparative genomics. Genome Res. 19, 1639–1645. doi: 10.1101/gr.092759.109
Langmead, B., and Salzberg, S. L. (2012). Fast gapped-read alignment with Bowtie 2. Nat. Methods 9, 357–359. doi: 10.1038/nmeth.1923
Li, H., Handsaker, B., Wysoker, A., Fennell, T., Ruan, J., Homer, N., et al. (2009). The sequence alignment/map format and SAMtools. Bioinformatics 25,2078–2079. doi: 10.1093/bioinformatics/btp352
Lubertozzi, D., and Keasling, J. D. (2009). Developing Aspergillus as a host for heterologous expression. Biotechnol. Adv. 27, 53–75. doi: 10.1016/j.biotechadv.2008.09.001
Mach-Aigner, A. R., Omony, J., Jovanovic, B., Van Boxtel, A. J. B., and De Graaff, L. H. (2012). d-Xylose concentration-dependent hydrolase expression profiles and the function of CreA and XlnR in Aspergillus niger. Appl. Environ. Microbiol. 78, 3145–3155. doi: 10.1128/AEM.07772-11
Marinho, H. S., Real, C., Cyrne, L., Soares, H., and Antunes, F. (2014). Hydrogen peroxide sensing, signaling and regulation of transcription factors. Redox Biol. 2, 535–562. doi: 10.1016/j.redox.2014.02.006
Meyer, V., Wu, B., and Ram, A. F. J. (2011). Aspergillus as a multi-purpose cell factory: current status and perspectives. Biotechnol. Lett. 33, 469–476. doi: 10.1007/s10529-010-0473-8
Milne, I., Stephen, G., Bayer, M., Cock, P. J. A., Pritchard, L., Cardle, L., et al. (2013). Using tablet for visual exploration of second-generation sequencing data. Brief. Bioinform. 14, 193–202. doi: 10.1093/bib/bbs012
Odoni, D. I., Van Gaal, M. P., Schonewille, T., Tamayo-Ramos, J. A., Martins Dos Santos, V. A. P., Suarez-Diez, M., et al. (2017). Aspergillus niger secretes citrate to increase iron bioavailability. Front. Microbiol. 8:1424. doi: 10.3389/fmicb.2017.01424
Oliveira, M. A., Discola, K. F., Alves, S. V., Medrano, F. J., Guimarães, B. G., and Netto, L. E. S. (2010). Insights into the specificity of thioredoxin reductase-thioredoxin interactions. A structural and functional investigation of the yeast thioredoxin system. Biochemistry 49, 3317–3326. doi: 10.1021/bi901962p
Pel, H. J., De Winde, J. H., Archer, D. B., Dyer, P. S., Hofmann, G., Schaap, P. J., et al. (2007). Genome sequencing and analysis of the versatile cell factory Aspergillus niger CBS 513.88. Nat. Biotechnol. 25, 221–231. doi: 10.1038/nbt1282
Peng, S., Cao, Q., Qin, Y., Li, X., Liu, G., and Qu, Y. (2017). An aldonolactonase AltA from Penicillium oxalicum mitigates the inhibition of β-glucosidase during lignocellulose biodegradation. Appl. Microbiol. Biotechnol. 101, 3627–3636. doi: 10.1007/s00253-017-8134-7
Peng, Y., Leung, H. C. M., Yiu, S. M., and Chin, F. Y. L. (2012). IDBA-UD: a de novo assembler for single-cell and metagenomic sequencing data with highly uneven depth. Bioinformatics 28, 1420–1428. doi: 10.1093/bioinformatics/bts174
Petersen, T. N., Brunak, S., Von Heijne, G., and Nielsen, H. (2011). SignalP 4.0: discriminating signal peptides from transmembrane regions. Nat. Methods 8, 785. doi: 10.1038/nmeth.1701
Pócsi, I., Miskei, M., Karányi, Z., Emri, T., Ayoubi, P., Pusztahelyi, T., et al. (2005). Comparison of gene expression signatures of diamide, H2O2 and menadione exposed Aspergillus nidulans cultures – linking genome-wide transcriptional changes to cellular physiology. BMC Genomics 6:182. doi: 10.1186/1471-2164-6-182
Quail, M. A., Smith, M., Coupland, P., Otto, T. D., Harris, S. R., Connor, T. R., et al. (2012). A tale of three next generation sequencing platforms: comparison of ion torrent, pacific biosciences and illumina MiSeq sequencers. BMC Genomics 13:341. doi: 10.1186/1471-2164-13-341
Reid, I., O’toole, N., Zabaneh, O., Nourzadeh, R., Dahdouli, M., Abdellateef, M., et al. (2014). SnowyOwl: accurate prediction of fungal genes by using RNA-Seq and homology information to select among ab initio models. BMC Bioinformatics 15:229. doi: 10.1186/1471-2105-15-229
Schneeberger, K. (2014). Using next-generation sequencing to isolate mutant genes from forward genetic screens. Nat. Rev. Genet. 15, 662–676. doi: 10.1038/nrg3745
Semchyshyn, H. M., Abrat, O. B., Miedzobrodzki, J., Inoue, Y., and Lushchak, V. I. (2011). Acetate but not propionate induces oxidative stress in bakers’ yeast Saccharomyces cerevisiae. Redox Rep. 16, 15–23. doi: 10.1179/174329211X12968219310954
Sies, H., Berndt, C., and Jones, D. P. (2017). Oxidative Stress. Annu. Rev. Biochem. 86, 715–748. doi: 10.1146/annurev-biochem-061516-045037
Sievers, F., Wilm, A., Dineen, D., Gibson, T. J., Karplus, K., Li, W., et al. (2011). Fast, scalable generation of high-quality protein multiple sequence alignments using clustal omega. Mol. Syst. Biol. 7, 539–539. doi: 10.1038/msb.2011.75
Singh, O. V., and Kumar, R. (2007). Biotechnological production of gluconic acid: future implications. Appl. Microbiol. Biotechnol. 75, 713–722. doi: 10.1007/s00253-007-0851-x
Sloothaak, J., Odoni, D. I., De Graaff, L. H., Martins Dos Santos, V. A. P., Schaap, P. J., and Tamayo-Ramos, J. A. (2015). Aspergillus niger membrane-associated proteome analysis for the identification of glucose transporters. Biotechnol. Biofuels 8:150. doi: 10.1186/s13068-015-0317-9
Sloothaak, J., Odoni, D. I., Martins Dos Santos, V. A. P., Schaap, P. J., and Tamayo-Ramos, J. A. (2016a). Identification of a novel L-rhamnose uptake transporter in the filamentous fungus Aspergillus niger. PLoS Genetics 12:e1006468. doi: 10.1371/journal.pgen.1006468
Sloothaak, J., Tamayo-Ramos, J. A., Odoni, D. I., Laothanachareon, T., Derntl, C., Mach-Aigner, A. R., et al. (2016b). Identification and functional characterization of novel xylose transporters from the cell factories Aspergillus niger and Trichoderma reesei. Biotechnol. Biofuels 9:148. doi: 10.1186/s13068-016-0564-4
Sloothaak, J., Schilders, M., Schaap, P. J., and De Graaff, L. H. (2014). Overexpression of the Aspergillus niger GatA transporter leads to preferential use of D-galacturonic acid over D-xylose. AMB Express 4:66. doi: 10.1186/s13568-014-0066-3
Stanke, M., Diekhans, M., Baertsch, R., and Haussler, D. (2008). Using native and syntenically mapped cDNA alignments to improve de novo gene finding. Bioinformatics 24, 637–644. doi: 10.1093/bioinformatics/btn013
Steiger, M. G., Mach, R. L., and Mach-Aigner, A. R. (2010). An accurate normalization strategy for RT-qPCR in Hypocrea jecorina (Trichoderma reesei). J. Biotechnol. 145, 30–37. doi: 10.1016/j.jbiotec.2009.10.012
Swart, K., Debets, A. J. M., Bos, C. J., Slakhorst, M., Holub, E. F., and Hoekstra, R. F. (2001). Genetic analysis in the asexual fungus Aspergillus niger. Acta Biol. Hung. 52, 335–343. doi: 10.1556/ABiol.52.2001.2-3.18
Swart, K., Van De Vondervoort, P. J. I., Witteveen, C. F. B., and Visser, J. (1990). Genetic localization of a series of genes affecting glucose oxidase levels in Aspergillus niger. Curr. Genet. 18, 435–439. doi: 10.1007/BF00309913
Thön, M., Al Abdallah, Q., Hortschansky, P., Scharf, D. H., Eisendle, M., Haas, H., et al. (2010). The CCAAT-binding complex coordinates the oxidative stress response in eukaryotes. Nucleic Acids Res. 38, 1098–1113. doi: 10.1093/nar/gkp1091
Thön, M., Al-Abdallah, Q., Hortschansky, P., and Brakhage, A. A. (2007). The thioredoxin system of the filamentous fungus Aspergillus nidulans: impact on development and oxidative stress response. J. Biol. Chem. 282, 27259–27269. doi: 10.1074/jbc.M704298200
van Dam, J. C. J., Koehorst, J. J. J., Vik, J. O., Schaap, P. J., and Suarez-Diez, M. (2017). Interoperable genome annotation with GBOL, an extendable infrastructure for functional data mining. bioRxiv [Preprint]. doi: 10.1101/184747
van der Straat, L., Vernooij, M., Lammers, M., Van Den Berg, W., Schonewille, T., Cordewener, J., et al. (2014). Expression of the Aspergillus terreus itaconic acid biosynthesis cluster in Aspergillus niger. Microb. Cell Fact. 13:11. doi: 10.1186/1475-2859-13-11
Walker, B. J., Abeel, T., Shea, T., Priest, M., Abouelliel, A., Sakthikumar, S., et al. (2014). Pilon: an integrated tool for comprehensive microbial variant detection and genome assembly improvement. PLoS One 9:e112963. doi: 10.1371/journal.pone.0112963
Wang, Y., Geer, L. Y., Chappey, C., Kans, J. A., and Bryant, S. H. (2000). Cn3D: sequence and structure views for Entrez. Trends Biochem. Sci. 25, 300–302. doi: 10.1016/S0968-0004(00)01561-9
Witteveen, C. F. B., Van De Vondervoort, P., Swart, K., and Visser, J. (1990). Glucose oxidase overproducing and negative mutants of Aspergillus niger. Appl. Microbiol. Biotechnol. 33, 683–686. doi: 10.1007/BF00604938
Witteveen, C. F. B., Van De Vondervoort, P. J. I., Van Den Broeck, H. C., Van Engelenburg, F. A. C., De Graaff, L. H., Hillebrand, M. H. B. C., et al. (1993). Induction of glucose oxidase, catalase, and lactonase in Aspergillus niger. Curr. Genet. 24, 408–416. doi: 10.1007/BF00351849
Witteveen, C. F. B., Veenhuis, M., and Visser, J. (1992). Localization of glucose oxidase and catalase activities in Aspergillus niger. Appl. Environ. Microbiol. 58, 1190–1194.
Yang, L., Lübeck, M., and Lübeck, P. S. (2017). Aspergillus as a versatile cell factory for organic acid production. Fungal Biol. Rev. 31, 33–49. doi: 10.1016/j.fbr.2016.11.001
Keywords: forward genetics, genome sequencing, Aspergillus niger, N402, goxB, glucose oxidase
Citation: Laothanachareon T, Tamayo-Ramos JA, Nijsse B and Schaap PJ (2018) Forward Genetics by Genome Sequencing Uncovers the Central Role of the Aspergillus niger goxB Locus in Hydrogen Peroxide Induced Glucose Oxidase Expression. Front. Microbiol. 9:2269. doi: 10.3389/fmicb.2018.02269
Received: 07 February 2018; Accepted: 05 September 2018;
Published: 24 September 2018.
Edited by:
Martin G. Klotz, Washington State University Tri-Cities, United StatesReviewed by:
István Pócsi, University of Debrecen, HungaryHubertus Haas, Innsbruck Medical University, Austria
Ekaterina Shelest, German Center for Integrative Biodiversity Research, Germany
Copyright © 2018 Laothanachareon, Tamayo-Ramos, Nijsse and Schaap. This is an open-access article distributed under the terms of the Creative Commons Attribution License (CC BY). The use, distribution or reproduction in other forums is permitted, provided the original author(s) and the copyright owner(s) are credited and that the original publication in this journal is cited, in accordance with accepted academic practice. No use, distribution or reproduction is permitted which does not comply with these terms.
*Correspondence: Peter J. Schaap, cGV0ZXIuc2NoYWFwQHd1ci5ubA==