- 1Department of Microbiology, Aichi Gakuin University School of Dentistry, Nagoya, Japan
- 2Department of Removable Prosthodontics, Aichi Gakuin University School of Dentistry, Nagoya, Japan
Cyclic dimeric adenosine 3′,5′-monophosphate (c-di-AMP), a recently identified secondary messenger in bacteria, plays a role in several bacterial processes, including biofilm formation. It is enzymatically produced by diadenylate cyclase and cleaved by c-di-AMP phosphodiesterase. c-di-AMP is believed to be essential for the viability of bacterial cells that produce it. In the current study, the biochemical and biological roles of GdpP (SMU_2140c) and DhhP (SMU_1297), two distinct Streptococcus mutans phosphodiesterases involved in the pathway producing AMP from c-di-AMP, were investigated. Liquid chromatography-tandem mass spectrometry revealed that c-di-AMP was degraded to phosphoadenylyl adenosine (pApA) by truncated recombinant GdpP, and pApA was cleaved by recombinant DhhP to yield AMP. In-frame deletion mutants lacking the dhhP gene (ΔdhhP) and both the gdpP and dhhP genes (ΔgdpPΔdhhP) displayed significantly more biofilm formation than the wild-type and a mutant strain lacking the gdpP gene (ΔgdpP; p < 0.01). Furthermore, biofilm formation was restored to the level of the wild type strain upon complementation with dhhP. Optical and electron microscopy observations revealed that ΔdhhP and ΔgdpPΔdhhP mutants self-aggregated into large cell clumps, correlated with increased biofilm formation, but cell clumps were not observed in cultures of wild-type, ΔgdpP, or strains complemented with gdpP and dhhP. Thus, deletion of dhhP presumably leads to the formation of bacterial cell aggregates and a subsequent increase in biofilm production.
Introduction
Streptococcus mutans is a major etiological agent of dental caries in humans (Hamada and Slade, 1980), a biofilm-associated infectious disease and important health problem worldwide (Thenisch et al., 2006). This microorganism has the capacity to prevail in the complex microbial community of an oral biofilm under the low pH conditions responsible for tooth demineralization, and can adapt to the stressful conditions to which the cariogenic biofilms are exposed (Lemos and Burne, 2008).
Bacterial second messengers, including cyclic dimeric nucleotides, are molecules that control important signaling cascades in bacteria and their hosts during infection (Pesavento and Hengge, 2009). Cyclic dimeric guanosine 3′,5′-monophosphate (c-di-GMP), a signaling molecule that acts as a secondary messenger, is important for control of biofilm formation, adhesion, motility, virulence, and cell morphogenesis (Boyd and O’Toole, 2012). This molecule is synthesized from two molecules of guanosine 3′,5′-triphosphate (GTP) by a Gly-Gly-Asp-Glu-Phe (GGDEF) domain-containing diguanylate cyclase, and hydrolyzed to phosphoguanylyl guanosine by phosphodiesterase enzymes containing a highly conserved Glu-Ala-Leu (EAL) domain (Mills et al., 2011) or a His-Asp-Gly-Tyr-Pro (HD-GYP) domain (Hengge, 2009).
Cyclic dimeric adenosine 3′,5′-monophosphate (c-di-AMP) has recently emerged as a widely conserved secondary messenger (Witte et al., 2008). This molecule is thought to be essential for the viability of bacterial cells that produce it. Interestingly, Gram-positive bacteria mostly synthesize c-di-AMP (Woodward et al., 2010; Corrigan et al., 2011; Kamegaya et al., 2011; Bai et al., 2013; Du et al., 2014). c-di-AMP has been found to regulate many important function in bacteria, including the homeostasis of cell wall peptidoglycan architecture (Luo and Helmann, 2012), size and envelope stress (Corrigan et al., 2011), biofilm formation (Corrigan et al., 2011; Diethmaier et al., 2011; Du et al., 2014; Peng et al., 2016), potassium uptake (Bai et al., 2014), metabolic enzyme function (Sureka et al., 2014), and drug resistance (Banerjee et al., 2010; Corrigan et al., 2011; Griffiths and O’Neill, 2012; Luo and Helmann, 2012; Cho and Kang, 2013). This molecule also reportedly couples DNA integrity with the sporulation progression, cell growth, competence development, and spore resurrection (Oppenheimer-Shaanan et al., 2011). However, these functions of c-di-AMP are not necessarily shared by all Gram-positive bacteria. This molecule also appears to trigger the cytosolic pathway of innate immunity (Woodward et al., 2010; Parvatiyar et al., 2012; Dey et al., 2015).
c-di-AMP is enzymatically produced by diadenylate cyclases and cleaved by c-di-AMP phosphodiesterases. Diadenylate cyclases typically contain a diadenyl cyclase domain that is crucial for the synthesis of c-di-AMP from two molecules of ATP (Corrigan and Grundling, 2013), while phosphodiesterases degrading c-di-AMP are divided into two distinct classes. The first class is characterized by a catalytically active Asp-His-His/Asp-His-His-Ala (DHH/DHHA1) domain, whereas the second class contains a His-Asp (HD) domain in the active center (Huynh et al., 2015). DHH/DHHA1 domain-containing proteins responsible for c-di-AMP degradation are further divided into two subfamilies. The first subfamily comprises homologs of membrane-bound GdpP proteins that contain two N-terminal transmembrane helices, a Per-Arnt-Sim (PAS) domain, a degenerate GGDEF domain, and a catalytic DHH/DHHA1 domain (Rao et al., 2010). PAS domains usually function as sensory domains for detecting light, redox potential, and oxygen (Taylor and Zhulin, 1999). However, the domain appears to function for GdpP, since GdpP without the PAS domain was enzymatically equivalent to that with the domain (Bai et al., 2013). Proteins belonging to the other subfamily are homologs of the soluble DhhP protein from Borrelia burgdorferi that contains only a cytosolic core DHH/DHHA1 domain (Ye et al., 2014). It is interesting that Streptococcus pneumoniae harbors two DHH/DHHA1 domain-containing phosphodiesterases from both subfamilies, named Pde1 and Pde2 (Bai et al., 2013), which are orthologues of GdpP and Dhhp. The enzymatic activity of Pde1 is the same as that of membrane-bound GdpP proteins degrading c-di-AMP to pApA, whereas Pde2 cleaves both c-di-AMP and pApA to yield AMP. A recent study revealed that deletion of the gdpP gene (SMU_2140c) in S. mutans increased both the intracellular c-di-AMP concentration and biofilm production in the presence of sucrose (Peng et al., 2016). Since no ortholog of Pde2 in S. mutans, Dhhp (SMU_1297), has yet been fully investigated, the second putative phosphodiesterase should be characterized. In the current study, the S. mutans genes gdpP and dhhP were expressed in Escherichia coli, and the recombinant proteins were purified and enzymatically characterized. Markerless in-frame deletion mutant strains, including a double mutant strain, were constructed, and phenotypic characterization of these mutant strains was performed to verify the functions of these proteins.
Materials and Methods
Bacterial Strains and Growth Conditions
Streptococcus mutans XC (Koga et al., 1989) and its derivatives (Table 1) were anaerobically grown in Brain Heart Infusion broth (BHI; Becton Dickinson, Franklin Lakes, NJ, United States) at 37°C. Where necessary, erythromycin, kanamycin, or 4-chloro-phenylalanine (p-Cl-Phe; Sigma-Aldrich Japan, Tokyo, Japan) was added to a final concentration of 20 μg ml-1, 700 μg ml-1, or 200 μg ml-1, respectively. E. coli strains DH5α (Thermo Scientific Japan, Tokyo, Japan) and BL21 (Promega, Tokyo, Japan) were used for DNA manipulation and recombinant protein purification, respectively, and were grown aerobically in 2 × YT broth (Becton Dickinson) at 37°C. Where required, chloramphenicol, erythromycin, ampicillin, and kanamycin were added to the media to final concentrations of 20 μg ml-1, 200 μg ml-1, 100 μg ml-1, and 70 μg ml-1, respectively.
Preparation of Recombinant GdpP and DhhP Proteins
Truncated gdpP and intact dhhP genes were amplified by PCR using primers listed in Supplementary Table S1 and S. mutans genomic DNA as a template, and cloned into the pCold ProS2 vector (Takara Bio, Otsu, Japan). The N-terminal region (108 amino acids), which were not contained in the recombinant GdpP, was determined as previously described (Bai et al., 2013). The resulting plasmids (Table 1) were verified by DNA sequencing. E. coli BL21 cells harboring either plasmid were grown at 37°C to absorbance at 595 nm (A595) of ∼0.5. The bacterial culture was then cooled to 16°C and isopropyl-β-D-thiogalactoside was added to a final concentration of 0.3 mM. After induction at 16°C for 24 h, cells were sonicated and cell debris was removed by centrifugation at 50,000 × g and 4°C for 1 h. The supernatant was loaded onto a Co-based TALON column (Takara Bio, Otsu, Japan) pre-equilibrated with TBS (Tris-HCl buffer, pH 7.5, supplemented with 300 mM NaCl). After the resin was extensively washed with TBS containing 10 mM imidazole, proteins were eluted using 250 mM imidazole in TBS. Each eluate was dialyzed against 10 mM Tris-HCl (pH 7.4) and stored at -20°C following addition of an equal volume of 80% glycerol. The protein concentration was measured using a Pierce BCA Protein Assay Kit (Thermo Scientific, Tokyo, Japan), and the purity of samples was analyzed by sodium dodecyl sulfate-polyacrylamide gel electrophoresis (SDS-PAGE).
Liquid Chromatography Coupled With Tandem Mass Spectrometry (LC-MS/MS)
Adenosine monophosphate (AMP), pApA, c-di-AMP, and c-di-GMP were detected in reaction mixtures using a Nexera2 LC system (Shimadzu, Kyoto, Japan) connected to a triple quadrupole mass spectrometer (LC-MS 8040; Shimadzu) equipped with an electrospray ionization (ESI) source and operated in positive ion mode for pApA and negative ion mode for AMP, c-di-AMP, and c-di-GMP. Samples were separated using a reversed-phase Mastro SP column (2.1 × 100 mm; Shimadzu). The mobile phases were as follows: (A) 10 mM ammonium acetate/acetonitrile (90:10 v/v) and (B) 50 mM ammonium acetate:acetonitrile (80:20 v/v). A linear gradient from 0 to 60% solvent B over 9 min was applied at a flow rate of 0.5 ml min-1. The A254 value was monitored to quantify AMP, pApA, and c-di-AMP in reaction mixtures. ESI multiple reaction monitoring (MRM) mode was used to identify the compounds. The temperature of the ESI capillary was maintained at 300°C. The drying gas was introduced at a temperature of 300°C and a flow rate of 4 l min-1. The nebulizer gas pressure was 10 psi. Each sample (1 μl) was injected into the LC capillary. All solvents used in these experiments were LC-MS grade from Wako Pure Chemicals (Osaka, Japan) or Sigma-Aldrich Japan.
Enzyme Reactions
To determine kinetic parameters, reaction mixtures (50 μl) contained 50 mM potassium phosphate buffer (pH 5.0 for recombinant GdpP and pH 7.0 for recombinant DhhP), 1 mM MnCl2, 100 μM c-di-AMP, pApA, or c-di-GMP, and 1.2 μg of truncated recombinant GdpP ml-1 or 0.36 μg of recombinant DhhP ml-1. After 10 min, reactions were terminated by addition of 50 μl TE (50 mM Tris-HCl pH 8.0, 10 mM EDTA) -saturated phenol, followed by addition of the same volume of chloroform. After dilution with distilled water, the aqueous phase was analyzed by LC-MS/MS. Reaction mixtures at various concentrations of substrates (0–0.3 mM) were assayed to determine the kinetic properties of the enzymes. The assay mixtures used for divalent metal ion screening contained 1 mM MgCl2, 1 mM ZnCl2, 1 mM CoCl2, 1 mM CaCl2, or different concentrations of MnCl2 (0.1, 1, 5, or 10 mM). To analyze the optimal pH, reaction mixtures consisted of 50 mM potassium phosphate buffer at pH values adjusted to 4.0, 5.0, 6.0, 7.0, 8.0, or 9.0. To investigate the optimal reaction temperature, reaction mixtures were incubated at 20, 30, 37, 40, 50, or 60°C.
Construction of Deletion and Complemented Streptococcus mutans Strains
Markerless mutagenesis was performed to construct three in-frame S. mutans XC deletion mutants, namely, ΔgdpP, ΔdhhP, and ΔgdpPΔdhhP (Table 1 and Supplementary Figures S1, S2, S3). A two-step transformation protocol using an IFDC2 cassette was used as described previously (Xie et al., 2011). This cassette contains an erythromycin resistance gene and a gene encoding mutated phenylalanine tRNA synthetase that incorporates the toxic p-Cl-Phe into proteins during translation. Introduction of the IFDC2 cassette altered the phenotype of the wild-type (WT) strain from p-Cl-Phe resistance and erythromycin sensitivity to p-Cl-Phe sensitivity and erythromycin resistance. First, three DNA fragments (an upstream targeting sequence, the IFDC2 cassette, and downstream targeting sequence) were individually generated by PCR, ligated using an In-fusion HD Cloning kit (Takara Bio, Otsu, Japan), and inserted into the cloning vector pMCL200 (Nakano et al., 1995). S. mutans XC cells were transformed with the respective linearized plasmids as previously described (Yoshida et al., 2005). The sensitivity of transformants (intermediate strains ΔgdpP-Int and ΔdhhP-Int) to p-Cl-Phe was then verified (Supplementary Figures S1, S2, S3). In the second step, two DNA fragments (an upstream targeting sequence and a downstream targeting sequence) were linked using an In-fusion HD Cloning kit and then subcloned into pMCL200. The linearized plasmids were used to transform ΔgdpP-Int and ΔdhhP-Int strains. The transformants were selected and patched onto fresh BHI plates containing 200 μg p-Cl-Phe ml-1, and onto BHI plates containing 20 μg erythromycin ml-1, to confirm loss of the IFDC2 cassette. Deletion of the gdpP and dhhP genes in the mutant strains, designated ΔgdpP and ΔdhhP, respectively, was confirmed by PCR and DNA sequencing. Similarly, a double mutant strain, ΔgdpPΔdhhP was constructed in the ΔgdpP background using the two-step transformation protocol, as described above (Supplementary Figure S3). All strains and plasmids used are listed in Table 1.
To facilitate genetic complementation studies of mutants of S. mutans, shuttle plasmids were constructed using pJY (Yang et al., 2009) with a constitutive promoter (CP25) (Hansen et al., 2001). After PCR-amplified gdpP and dhhP genes with a Shine-Dalgarno sequence (Shine and Dalgarno, 1974) were inserted into the BamHI and NcoI sites of pJY using an In-fusion HD Cloning kit (Takara Bio, Otsu, Japan), the integrity of the cloned sequence was verified by DNA sequencing. The resulting plasmids, pSM2140 and pSM1297 (Table 1), were used to transform S. mutansΔgdpP and ΔdhhP, respectively, to construct ΔgdpP-com and ΔdhhP-com (Table 1).
Biofilm Assay
Biofilm formation assays were performed on polystyrene plates as previously described (Yano et al., 2012) with several minor modifications. Bacterial cultures used for biofilm formation were prepared by inoculating overnight cultures of S. mutans strains into fresh BHI broth at a ratio of 1:20, and anaerobically incubated at 37°C for 24 h. Culture aliquots (5 μl) were inoculated into 95 μl of fresh BHI broth in a 96-well flat-bottomed polystyrene plate. To evaluate the effect of sucrose on biofilm formation, 1.0% sucrose was added to the media. After incubation at 37°C for 24 h, bacterial cultures were gently discarded, and plates were carefully washed three times with phosphate-buffered saline (PBS) to remove non-adhered cells and then stained with 0.2% (w/v) Victoria Blue for 15 min. Bound dye was dissolved using 100 μl of 99% methanol, and biofilm volume was evaluated by measuring the absorbance at 595 nm (A595). When A595 values were >2.0, they were measured again after appropriate dilution. A blank value (medium alone) was subtracted from each value. Data are represented as mean ± standard deviation from 12 replicates in three independent experiments.
Morphological Analyses
To observe planktonic S. mutans cells, cultures were grown for 24 h, inoculated into fresh BHI broth at a ratio of 1:20, and incubated until the A595 value was ∼0.3 (mid-log phase). Aliquots of streptococcal cultures (5 μl) were fixed and stained using a conventional Gram staining method for optical microscopy. Biofilm formation after 12 h was also assessed using scanning electron microscopy (SEM) as previously described (Konno et al., 2015).
Statistical Analysis
Data were analyzed by one-way analysis of variance (ANOVA) followed by Tukey’s post hoc tests at a significance level of p < 0.01.
Results
Identification and Purification of Putative c-di-AMP Phosphodiesterases GdpP and DhhP
A homology search revealed that the amino acid sequences of GdpP (SMU_2140c) and DhhP (SMU_1297) from S. mutans are 56 and 68% identical with Pde1 and Pde2 from S. pneumoniae, respectively, and several functional domains are shared between the respective homologs (Figure 1A). To evaluate the enzymatic functions of GdpP and DhhP in S. mutans, we attempted to purify the recombinant proteins following heterologous expression in E. coli but failed to obtain intact GdpP. Thus, genes encoding a truncated GdpP variant lacking transmembrane helices and a part of PAS domain (spanning amino acids 1–108), and an intact DhhP were cloned, and recombinant proteins were purified from E. coli lysates (Figure 1). The N-terminal hydrophobic transmembrane domains are dispensable for c-di-AMP phosphodiesterase activity (Corrigan et al., 2011; Rao et al., 2011; Bai et al., 2013). In addition, the PAS domain also did not affect the enzymatic function of GdpP (Bai et al., 2013). Each protein was generated as a fusion construct with a 23 kDa ProS2 tag that rendered the recombinant protein soluble and stable. The recombinant GdpP and DhhP were designated rGdpP109-657-ProS2 and rDhhP-ProS2, respectively. The molecular masses of the denatured fusion proteins were consistent with their predicted molecular masses (i.e., 84 kDa and 57 kDa for rGdpP109-657-ProS2 and rDhhP-ProS2, respectively; Figure 1B).
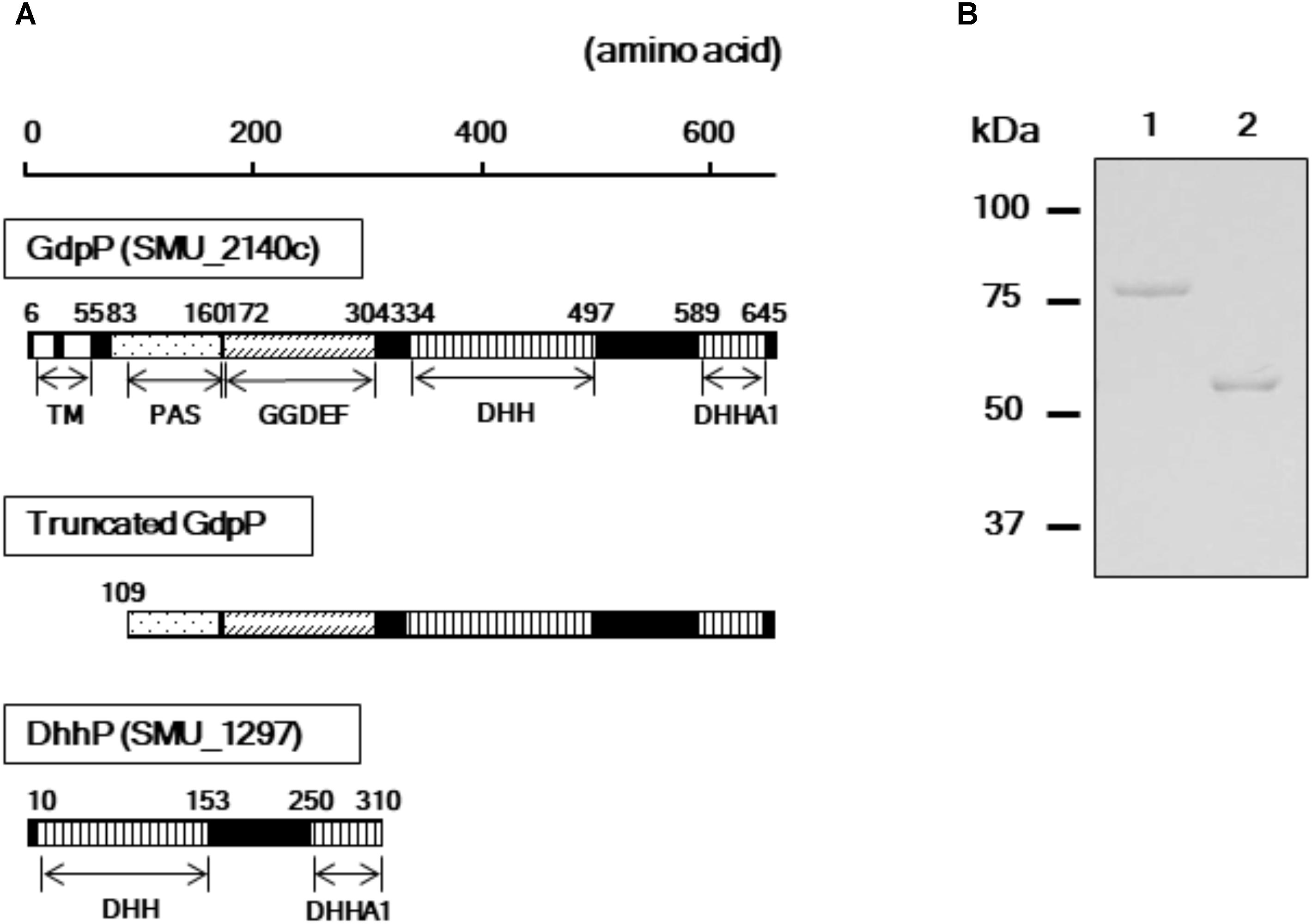
FIGURE 1. Purification of rGdpP109-657-ProS2 and rDhhP-ProS2. (A) Protein domain architecture. Numbers indicate amino acid positions in the full-length protein sequences. The N-terminus of the intact GdpP (657 amino acids) contains two transmembrane helices (TMs), a PAS domain, a GGDEF domain, a DHH domain, and a DHHA1 domain. rGdpP109-657-ProS2 generated in this study does not contain a TM. Both rGdpP109-657-ProS2 and rDhhP-ProS2 were generated with N-terminal 23 kDa ProS2 domains to increase their stability. (B) Sodium dodecyl sulfate-polyacrylamide gel electrophoresis analysis. Lane 1, rGdpP109-657-ProS2; Lane 2, rDhhP-ProS2. The positions of the molecular mass markers are shown on the left.
Enzymatic Characterization of rGdpP109-657-ProS2 and rDhhP-ProS2 From S. mutans as c-di-AMP Phosphodiesterases
LC analysis of A254 values revealed that incubation of rGdpP109-657-ProS2 with c-di-AMP resulted in the production of pApA (Figure 2), and this was further confirmed by MRM mode LC-MS/MS, in which transitions from m/z 677.4 (1+, precursor ion) to m/z 136.1 (product ion) and m/z 97.0 (product ion) were observed. However, pApA was not degraded by rGdpP109-657-ProS2, but incubation of rDhhP-ProS2 with pApA resulted in the production of AMP, and this was also confirmed by LC-MS/MS, in which the transition of m/z 346.05 (1-, precursor ion) to m/z 79.1 (product ion) was observed. Unlike Pde2 from S. pneumoniae, c-di-AMP was not cleaved by rDhhP-ProS2 (Figure 2), even when a large amount of rDhhP-ProS2 (100-fold more than usual) was incubated with c-di-AMP for a long incubation time (12-fold longer than usual). This suggests that direct production of AMP from c-di-AMP by DhhP is negligible in S. mutans. Neither rGdpP109-657-ProS2 nor rDhhP-ProS2 cleaved c-di-GMP (data not shown), indicating that c-di-GMP is not a substrate for either of GdpP and DhhP.
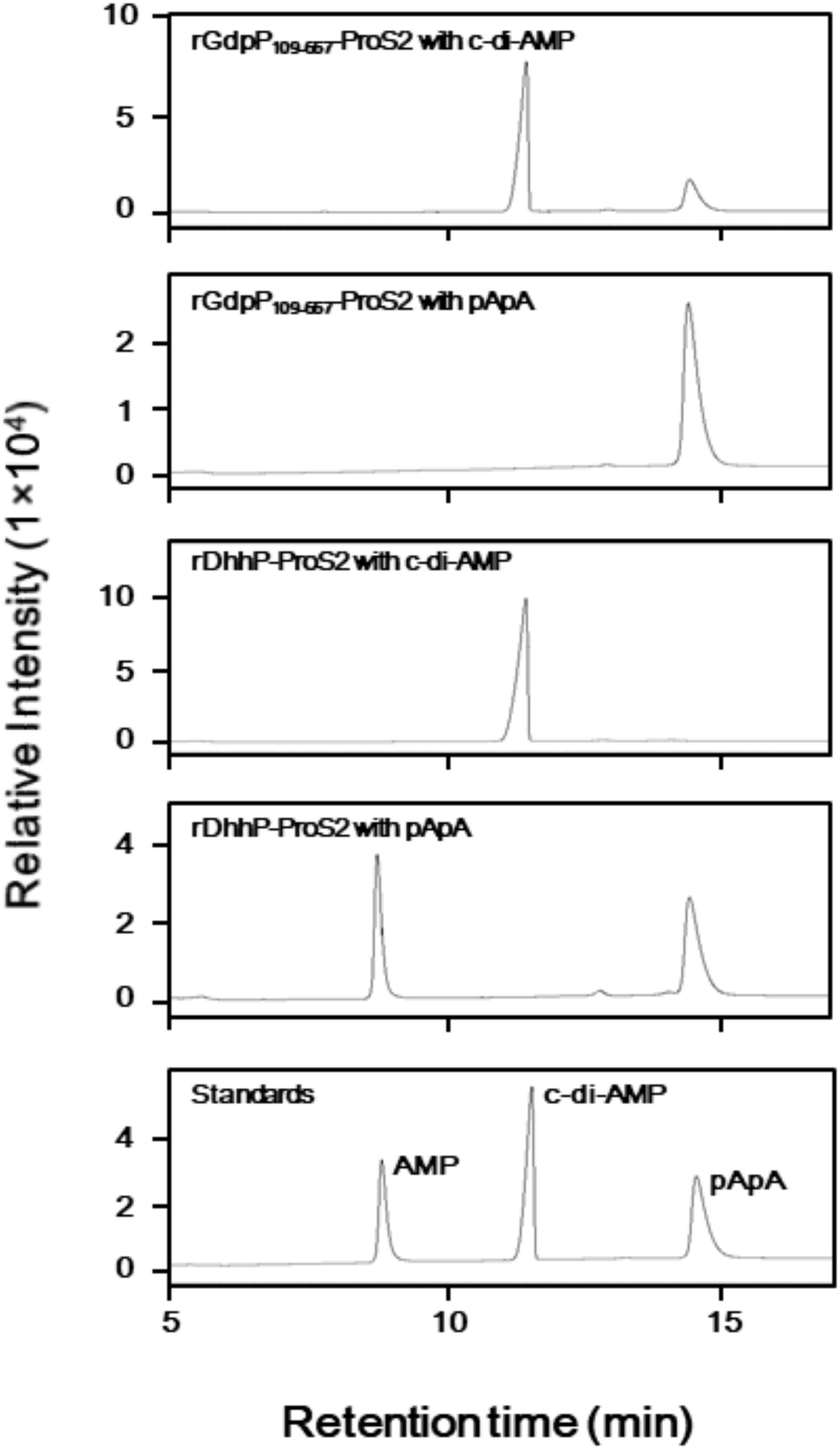
FIGURE 2. Phosphodiesterase activity of rGdpP109-657-ProS2 and rDhhP-ProS2 by liquid chromatography (LC) analysis. Reaction mixtures (50 μl) contained 50 mM potassium phosphate buffer (pH 5.0 for GdpP, pH 7.0 for rDhhP-ProS2), 1 mM MnCl2, 100 μM substrate (c-di-AMP or pApA), and 1.2 μg ml-1 rGdpP109-657-ProS2 or 0.36 μg ml-1 rDhhP-ProS2. Reactions were incubated for 15 min and terminated by addition of TE-saturated phenol and chloroform, and an aliquot (1 μl) of the aqueous phase was injected onto the LC column. Each sample was separated by LC and monitored at 254 nm. Purified AMP, c-di-AMP, and pApA were used as standards.
Reaction conditions for rGdpP109-657-ProS2 and rDhhP-ProS2 from S. mutans were optimized by quantifying pApA and AMP produced from c-di-AMP and pApA, respectively, in different pH, temperatures, and divalent ions (Figure 3). Maximum activity of rGdpP109-657-ProS2 was observed at pH 5.0 and 37°C, and Co2+ was the most effective of the divalent metal ions tested. Mn2+ also stimulated the enzyme with an optimal concentration of 1 mM, equivalent to ∼60% of the activity with Co2+. Interestingly, the enzymatic activity of rGdpP109-657-ProS2 in the presence of Zn2+ or Ca2+ was lower than that of the control, suggesting that these ions inhibit catalytic activity. rDhhP-ProS2 exhibited highest activity under slightly different conditions, with an optimal pH of 7.0 and an optimal temperature of 37°C. Of the divalent metal ions tested, Mn2+ was the most effective for stimulating enzymatic activity, and rDhhP-ProS2 was also stimulated by Mg2+, Co2+, and Ca2+, and inhibited by Zn2+, but not by Ca2+.
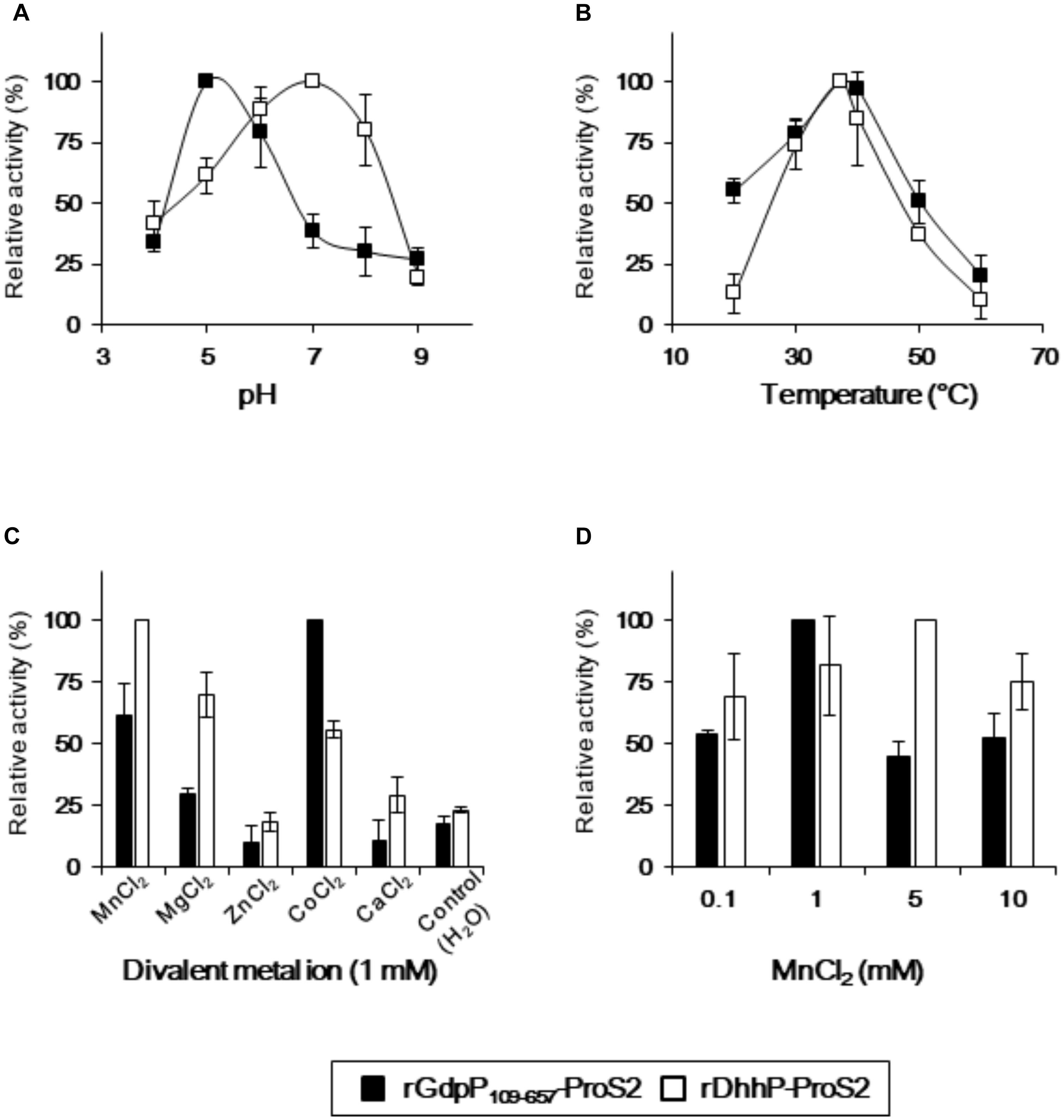
FIGURE 3. Optimization of reaction conditions for rGdpP109-657-ProS2 and rDhhP-ProS2. The relative activity was measured at different (A) pH values, (B) temperatures, (C) divalent metal ion concentrations, and (D) MnCl2 concentrations. Enzyme activity is presented relative to the maximum activity for each variable. Data are presented as mean ± standard deviation from three independent experiments.
Kinetic parameters of rGdpP109-657-ProS2 and rDhhP-ProS2 enzymes were determined using the initial rate method, with end products (separated by LC) quantified using A254 values (Table 2).

TABLE 2. Kinetic properties for the reactions catalyzed by rGdpP109-657-ProS2 and rDhhP-ProS2 from S. mutans.
Deletion of dhhP in S. mutans Results in Suppression of Growth
To examine the biological roles of GdpP and DhhP, three markerless in-frame mutant strains, in which the potentially confounding polar effect of the promoter of the antibiotic resistance gene on the downstream genes was eliminated, were constructed (Supplementary Figures S1, S2, S3). In addition, strains complemented with gdpP and dhhP were also constructed (Table 1). Since c-di-AMP homeostasis plays an important role in cell growth in some bacteria (Mehne et al., 2013; Ye et al., 2014), the growth of S. mutans and its derivatives was examined by measuring A595 values (Figure 4A). The growth of the ΔgdpP strain was similar to that of the WT strain, while the growth of ΔdhhP and ΔgdpPΔdhhP strains was slower than that of the WT. Furthermore, the slow growth of the ΔdhhP strain was restored by complementation with dhhP (Figure 4A).
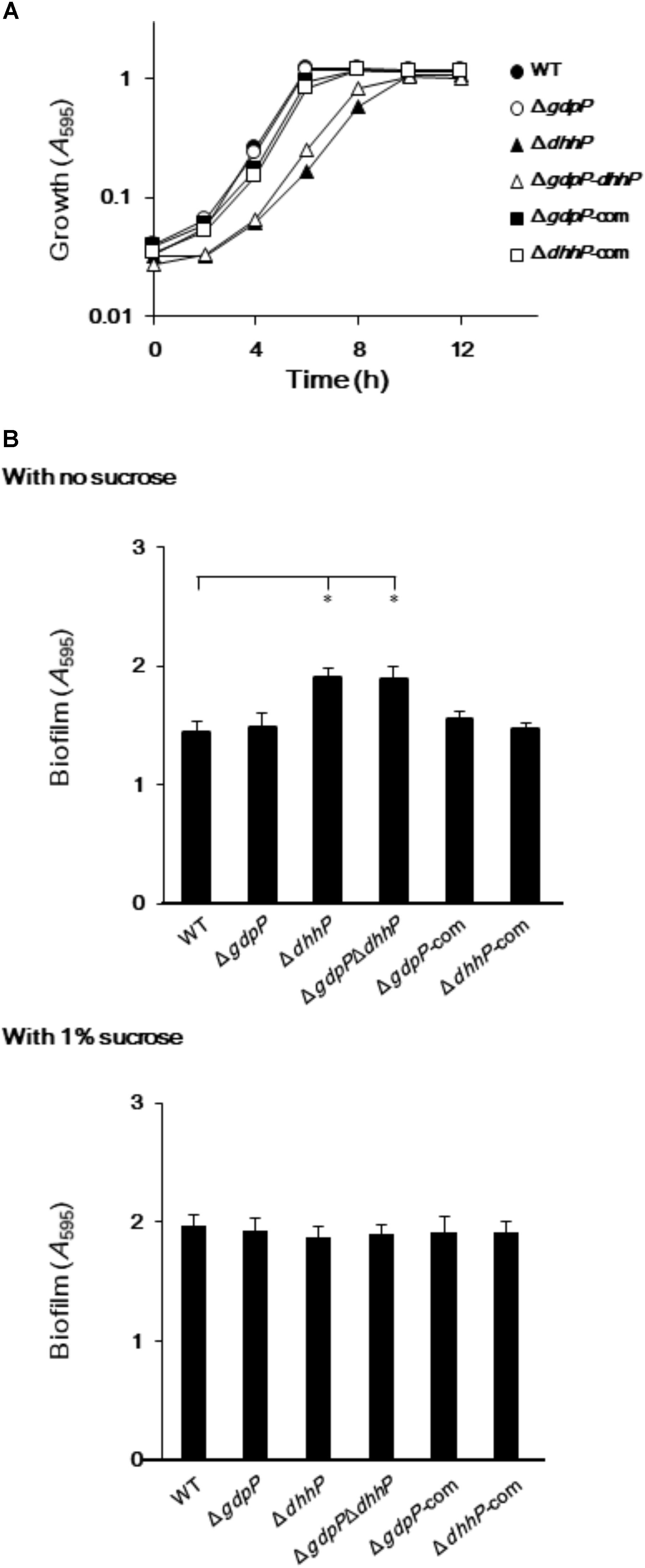
FIGURE 4. Growth and biofilm formation of Streptococcus mutans XC, ΔgdpP, ΔdhhP, ΔgdpPΔdhhP, ΔgdpP-com, and ΔdhhP-com strains. (A) A595 values of bacterial cell cultures, monitored every 2 h. (B) Sucrose-independent and -dependent biofilm formation in 96-well polystyrene plates after 24 h. Data are expressed as the mean ± standard deviation from 12 replicates from three independent experiments. Data were analyzed by one-way analysis of variance (ANOVA) followed by Tukey’s post hoc tests. ∗p < 0.01.
Deletion of dhhP in S. mutans Results in Increased Biofilm Production
When S. mutans WT and derivative strains were incubated for 24 h in the absence of sucrose, biofilm formation was significantly more pronounced in strains ΔdhhP and ΔgdpPΔdhhP than in the other strains (Figure 4B). By contrast, the amount of biofilm formed by the ΔgdpP mutant in the absence of sucrose was not significantly different from that of the WT strain when incubated for 24 h (Figure 4B). Importantly, the amount of biofilm produced by the ΔdhhP strain was restored to WT levels upon complementation with dhhP (Figure 4B). These findings revealed that the function of DhpP plays an important role in stimulating biofilm formation. Biofilm formation was not significantly different among strains in the presence of 1.0% sucrose (Figure 4B).
Optical and electron microscopy observations showed that cells of both ΔdhhP and ΔgdpPΔdhhP strains clumped together to form large bacterial aggregates of ∼0.1 mm in diameter under both biofilm and planktonic conditions, whereas cell clumps were not observed in cultures of WT, ΔgdpP, ΔgdpP-com, or ΔdhhP-com cells (Figure 5), revealing that lack of DhhP resulted in the formation of S. mutans aggregates.
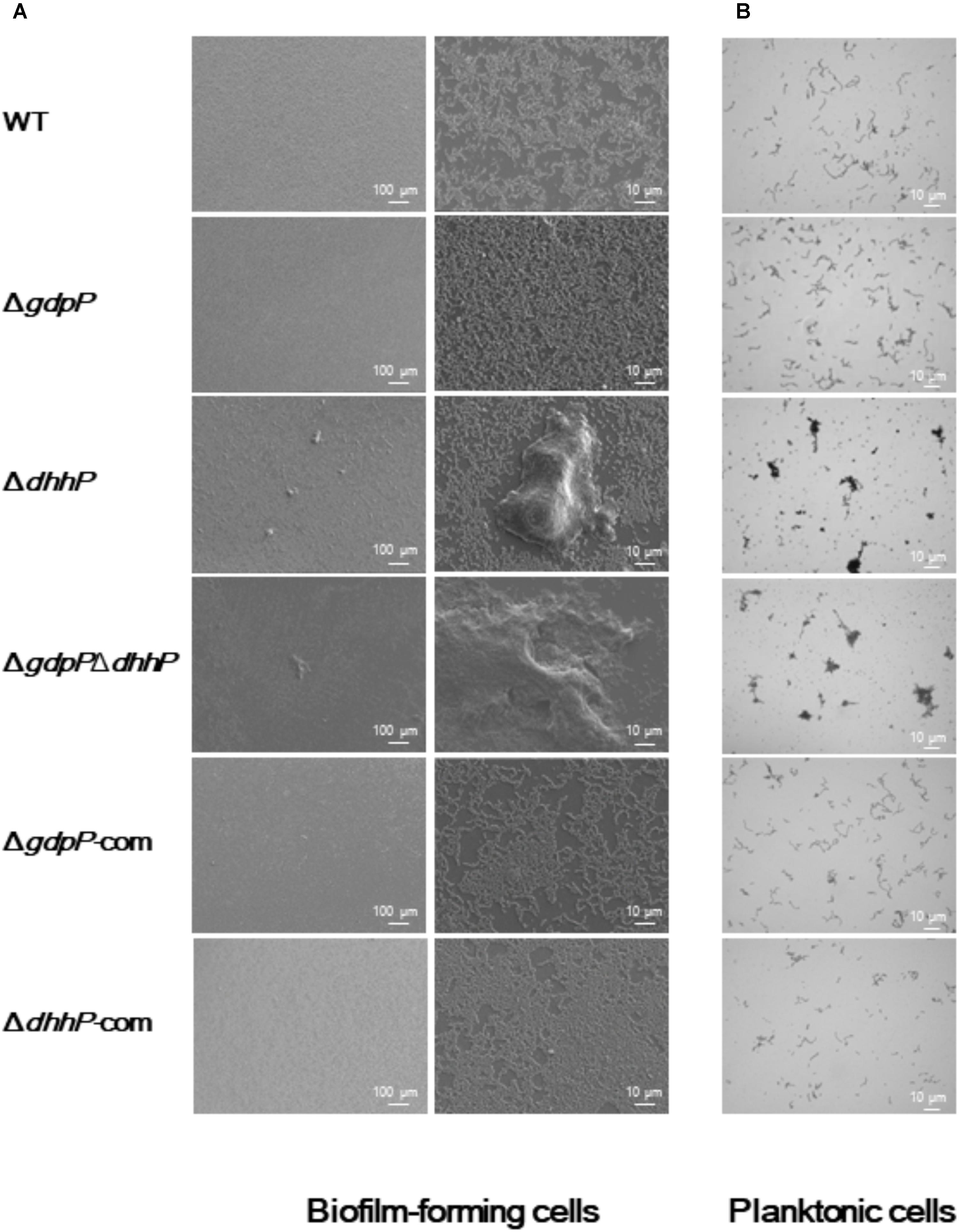
FIGURE 5. Morphology of S. mutans XC, ΔgdpP, ΔdhhP, ΔgdpPΔdhhP, ΔgdpP-com, and ΔdhhP-com strains. (A) Scanning electron microscopy images of biofilms formed after 12 h on a cover glass. Each image is representative of 3–5 independent experiments. (B) Optical microscopy analysis of S. mutans strains grown in liquid media. Cells grown to log phase were observed after conventional Gram staining. Each image is representative of three independent experiments.
Discussion
The secondary messenger c-di-AMP was recently identified in bacteria and appears to be required for cell viability (Song et al., 2005; Woodward et al., 2010; Corrigan et al., 2011; Luo and Helmann, 2012; Mehne et al., 2013). c-di-AMP is synthesized by most of Gram-positive bacteria (Woodward et al., 2010; Corrigan et al., 2011; Kamegaya et al., 2011; Bai et al., 2013; Du et al., 2014), whereas the molecule is also present in some Gram-negative pathogens (Barker et al., 2013) and Spirochaetae (Ye et al., 2014). By contrast, the enzymes associated with c-di-GMP and its functions for the secondary messenger have been investigated in a diverse range of bacterial species (Jenal et al., 2017).
GdpP and DhhP in S. mutans are orthologs of Pde1 and Pde2 in S. pneumoniae, respectively, despite sharing relatively low sequence identity. Homology analyses revealed that streptococcal species, including S. sobrinus, S. gordonii, S. salivarius, S. pyogenes, S. agalactiae, S. oralis, S. suis, and S. anginosus, contain one ortholog of GdpP and one ortholog of DhhP. Thus, the two distinct c-di-AMP phosphodiesterases characterized in this study appear to be present in most streptococci.
The rGdpP109-657-ProS2 appears to share the same substrate specificity as the Pde1 enzyme from S. pneumoniae (Bai et al., 2013). By contrast, the substrate specificity of rDhhP-ProS2 was different from that of Pde2 from S. pneumoniae, which produced AMP not from c-di-AMP but from pApA (Figure 2), whereas Pde2 from S. pneumoniae produced AMP both from c-di-AMP and pApA. Notably, the enzymatic activity of S. pneumoniae Pde2 to produce AMP from c-di-AMP was much lower (approximately 1/2000) than that of the same enzyme to produce AMP from pApA (Bai et al., 2013). Based on these previous findings and our current results (Figure 2), we conclude that DhhP has no detectable capacity to degrade c-di-AMP to produce AMP, and mainly cleaves pApA to AMP in S. mutans. It is probable that in S. mutans, c-di-AMP is degraded to the pApA intermediate by GdpP, and this is subsequently degraded to AMP by DhhP.
The optimal pH for the enzymatic activities of rGdpP109-657-ProS2 and rDhhP-ProS2 (pH 5.0 and 7.0, respectively) was lower than that of the orthologs listed above (mostly pH 8.0 or 8.5). This lower optimal pH for rGdpP109-657-ProS2 and rDhhP-ProS2 might be related to the ability of S. mutans to survive in more acidic environments (Hamilton and Buckley, 1991). DHH family proteins generally require a divalent cation for function (Yamagata et al., 2002). Indeed, Mn2+ is indispensable for the enzymatic activity of many phosphodiesterases that act on c-di-AMP, including Pde2 from S. pneumoniae (Bai et al., 2013), CnpB from Mycobacterium tuberculosis (Yang et al., 2014), DhhP from B. burgdorferi (Ye et al., 2014), and GdpP from B. subtilis (Rao et al., 2011). These findings, together with our current results (Figure 3C), suggest that Mn2+ might be the physiological metal ion of GdpP and DhhP proteins. Interestingly, the enzymatic activity of these enzymes was inhibited by Zn2+ and Ca2+, consistent with previous reports of inhibition of phosphodiesterase activity by Zn2+ and Ca2+ in several bacteria (Rao et al., 2011; Ye et al., 2014; Tang et al., 2015).
Biofilm formation by ΔgdpP was not significantly different from that of the WT strain (Figure 4), unlike other streptococcal species; deletion of gdpP resulted in increased biofilm production by S. suis (Du et al., 2014) and S. pneumoniae (Bai et al., 2013). Our data from three independently constructed mutant strains (ΔdhhP, ΔgdpPΔdhhP, and ΔdhhP-com) clearly demonstrated that deletion of the dhhP gene led to increased biofilm formation, probably due to aggregation of S. mutans (Figures 4B, 5). Bacterial aggregates of ΔdhhP and ΔgdpPΔdhhP strains could act as scaffolds on which thick biofilms are formed. However, the exact mechanism by which deletion of the dhhP gene is associated with the formation of cell clumps remains to be elucidated. Given the products of the rDhhP-ProS2 enzymatic reaction (Figure 2), exhaustion of AMP in bacterial cells and/or accumulation of pApA might affect biofilm formation. Otherwise, it would be possible that AMP has an inhibitory effect on biofilm formation. Deletion of gdpP, which leads to exhaustion of AMP in S. mutans cells, did not result in aggregation of the bacteria (Figure 5), suggesting that the concentration of AMP in cells might not be associated with formation of biofilms. Further studies are necessary to precisely elucidate the relationship between increased biofilm formation and deletion of dhhP since the intercellular level of c-di-AMP affects the expression of ∼700 genes in B. subtilis (Gundlach et al., 2016). Peng et al. (2016) reported that biofilm formation by a gdpP-deficient mutant of S. mutans UA159 was greater than the WT strain in the presence of sucrose. Contrary to this previous report, we showed that the amount of biofilm formed by S. mutans XC and its derivatives in the presence of 1.0% sucrose, which was higher than that in the absence of sucrose, was not significantly different among the strains tested (Figure 4B). S. mutans glucosyltransferases, including GTF-I and GTF-SI, synthesize water-insoluble glucans from sucrose (Kuramitsu, 1993), thereby forming extraordinarily strong and thick biofilms (Bowen and Koo, 2011). Therefore, it might be difficult to precisely evaluate how gdpP and dhhP deletion affects biofilm formation because large amounts of insoluble glucans produced in the presence of sucrose could mask the relatively weak effect of gdpP and dhhP deletion on biofilm formation.
Growth as a biofilm is usually triggered to assist survival in inhospitable conditions, and deletion of the dhhP gene may constitute a stressful situation for bacteria. Interestingly, the DhhP protein identified as a phosphodiesterase in the current study was previously reported to be essential for the superoxide stress response (Zhang and Biswas, 2009). Together with the existing literature, our present findings indicate that DhhP protein and related molecules such as c-di-AMP, pApA, and AMP might play an important role in the response of S. mutans to stress conditions.
Conclusion
Herein, two S. mutans c-di-AMP phosphodiesterases, GdpP, and DhhP, were identified and characterized. rGdpP109-657-ProS2 degrades c-di-AMP to produce pApA, which is subsequently degraded by rDhhP-ProS2 to yield AMP. Deletion of dhhP in S. mutans resulted in the formation of large cell clumps, leading to increased biofilm formation.
Author Contributions
YY conceived of and designed the experiments. HK and YY acquired the data. HK, YY, and KN analyzed the data. HK, YY, KN, JT, and YH interpreted the data.
Funding
This study was supported in part by JSPS KAKENHI Grant Number 26462804 (YY) from the Ministry of Education, Culture, Sports, Science and Technology of Japan.
Conflict of Interest Statement
The authors declare that the research was conducted in the absence of any commercial or financial relationships that could be construed as a potential conflict of interest.
Acknowledgments
The authors thank Dr. Y. Sato (Tokyo Dental College, Tokyo, Japan) for providing the IFDC2 cassette with the approval of the developer, Dr. J. Merritt (University of Oklahoma, Oklahoma City, OK, United States). We also thank Dr. Y. Hayakawa (Aichi Institute of Technology, Toyota, Japan) and Dr. K. Ishige (Yamasa Shoyu, Choshi, Japan) for providing c-di-GMP.
Supplementary Material
The Supplementary Material for this article can be found online at: https://www.frontiersin.org/articles/10.3389/fmicb.2018.02347/full#supplementary-material
References
Bai, Y., Yang, J., Eisele, L. E., Underwood, A. J., Koestler, B. J., Waters, C. M., et al. (2013). Two DHH subfamily 1 proteins in Streptococcus pneumoniae possess cyclic di-AMP phosphodiesterase activity and affect bacterial growth and virulence. J. Bacteriol. 195, 5123–5132. doi: 10.1128/JB.00769-13
Bai, Y., Yang, J., Zarrella, T. M., Zhang, Y., Metzger, D. W., and Bai, G. (2014). Cyclic di-AMP impairs potassium uptake mediated by a cyclic di-AMP binding protein in Streptococcus pneumoniae. J. Bacteriol. 196, 614–623. doi: 10.1128/JB.01041-13
Banerjee, R., Gretes, M., Harlem, C., Basuino, L., and Chambers, H. F. (2010). A mecA-negative strain of methicillin-resistant Staphylococcus aureus with high-level β-lactam resistance contains mutations in three genes. Antimicrob. Agents Chemother. 54, 4900–4902. doi: 10.1128/AAC.00594-10
Barker, J. R., Koestler, B. J., Carpenter, V. K., Burdette, D. L., Waters, C. M., Vance, R. E., et al. (2013). STING-dependent recognition of cyclic di-AMP mediates type I interferon responses during Chlamydia trachomatis infection. mBio 4:e00018-13. doi: 10.1128/mBio.00018-13
Bowen, W. H., and Koo, H. (2011). Biology of Streptococcus mutans-derived glucosyltransferases: role in extracellular matrix formation of cariogenic biofilms. Caries Res. 45, 69–86. doi: 10.1159/000324598
Boyd, C. D., and O’Toole, G. A. (2012). Second messenger regulation of biofilm formation: breakthroughs in understanding c-di-GMP effector systems. Annu. Rev. Cell Dev. Biol. 28, 439–462. doi: 10.1146/annurev-cellbio-101011-155705
Cho, K. H., and Kang, S. O. (2013). Streptococcus pyogenes c-di-AMP phosphodiesterase, GdpP, influences SpeB processing and virulence. PLoS One 8:e69425. doi: 10.1371/journal.pone.0069425
Corrigan, R. M., Abbott, J. C., Burhenne, H., Kaever, V., and Grundling, A. (2011). c-di-AMP is a new second messenger in Staphylococcus aureus with a role in controlling cell size and envelope stress. PLoS Pathog. 7:e1002217. doi: 10.1371/journal.ppat.1002217
Corrigan, R. M., and Grundling, A. (2013). Cyclic di-AMP: another second messenger enters the fray. Nat. Rev. Microbiol. 11, 513–524. doi: 10.1038/nrmicro3069
Dey, B., Dey, R. J., Cheung, L. S., Pokkali, S., Guo, H., Lee, J. H., et al. (2015). A bacterial cyclic dinucleotide activates the cytosolic surveillance pathway and mediates innate resistance to tuberculosis. Nat. Med. 21, 401–406. doi: 10.1038/nm.3813
Diethmaier, C., Pietack, N., Gunka, K., Wrede, C., Lehnik-Habrink, M., Herzberg, C., et al. (2011). A novel factor controlling bistability in Bacillus subtilis: the YmdB protein affects flagellin expression and biofilm formation. J. Bacteriol. 193, 5997–6007. doi: 10.1128/JB.05360-11
Du, B., Ji, W., An, H., Shi, Y., Huang, Q., Cheng, Y., et al. (2014). Functional analysis of c-di-AMP phosphodiesterase, GdpP, in Streptococcus suis serotype 2. Microbiol. Res. 169, 749–758. doi: 10.1016/j.micres.2014.01.002
Griffiths, J. M., and O’Neill, A. J. (2012). Loss of function of the gdpP protein leads to joint beta-lactam/glycopeptide tolerance in Staphylococcus aureus. Antimicrob. Agents Chemother. 56, 579–581. doi: 10.1128/AAC.05148-11
Gundlach, J., Rath, H., Herzberg, C., Mader, U., and Stulke, J. (2016). Second messenger signaling in Bacillus subtilis: accumulation of cyclic di-AMP inhibits biofilm formation. Front. Microbiol. 7:804. doi: 10.3389/fmicb.2016.00804
Hamada, S., and Slade, H. D. (1980). Biology, immunology, and cariogenicity of Streptococcus mutans. Microbiol. Rev. 44, 331–384.
Hamilton, I. R., and Buckley, N. D. (1991). Adaptation by Streptococcus mutans to acid tolerance. Oral Microbiol. Immunol. 6, 65–71. doi: 10.1111/j.1399-302X.1991.tb00453.x
Hansen, M. C., Palmer, R. J. Jr., Udsen, C., White, D. C., and Molin, S. (2001). Assessment of GFP fluorescence in cells of Streptococcus gordonii under conditions of low pH and low oxygen concentration. Microbiology 147(Pt 5), 1383–1391. doi: 10.1099/00221287-147-5-1383
Hengge, R. (2009). Principles of c-di-GMP signalling in bacteria. Nat. Rev. Microbiol. 7, 263–273. doi: 10.1038/nrmicro2109
Huynh, T. N., Luo, S., Pensinger, D., Sauer, J. D., Tong, L., and Woodward, J. J. (2015). An HD-domain phosphodiesterase mediates cooperative hydrolysis of c-di-AMP to affect bacterial growth and virulence. Proc. Natl. Acad. Sci. U.S.A. 112, E747–E756. doi: 10.1073/pnas.1416485112
Jenal, U., Reinders, A., and Lori, C. (2017). Cyclic di-GMP: second messenger extraordinaire. Nat. Rev. Microbiol. 15, 271–284. doi: 10.1038/nrmicro.2016.190
Kamegaya, T., Kuroda, K., and Hayakawa, Y. (2011). Identification of a Streptococcus pyogenes SF370 gene involved in production of c-di-AMP. Nagoya J. Med. Sci. 73, 49–57.
Koga, T., Asakawa, H., Okahashi, N., and Takahashi, I. (1989). Effect of subculturing on expression of a cell-surface protein antigen by Streptococcus mutans. J. Gen. Microbiol. 135, 3199–3207.
Konno, H., Yoshida, Y., Nagano, K., Hasegawa, Y., Nakamura, Y., Tanaka, Y., et al. (2015). Quantification of bacterial biofilms on different thermoplastic denture base resins using immunoblotting with enhanced chemiluminescence. Aichi Gakuin Dent. Sci. 28, 11–18.
Kuramitsu, H. K. (1993). Virulence factors of mutans streptococci: role of molecular genetics. Crit. Rev. Oral Biol. Med. 4, 159–176. doi: 10.1177/10454411930040020201
Lemos, J. A., and Burne, R. A. (2008). A model of efficiency: stress tolerance by Streptococcus mutans. Microbiology 154(Pt 11), 3247–3255. doi: 10.1099/mic.0.2008/023770-0
Luo, Y., and Helmann, J. D. (2012). Analysis of the role of Bacillus subtilis σ(M) in beta-lactam resistance reveals an essential role for c-di-AMP in peptidoglycan homeostasis. Mol. Microbiol. 83, 623–639. doi: 10.1111/j.1365-2958.2011.07953.x
Mehne, F. M., Gunka, K., Eilers, H., Herzberg, C., Kaever, V., and Stulke, J. (2013). Cyclic di-AMP homeostasis in Bacillus subtilis: both lack and high level accumulation of the nucleotide are detrimental for cell growth. J. Biol. Chem. 288, 2004–2017. doi: 10.1074/jbc.M112.395491
Mills, E., Pultz, I. S., Kulasekara, H. D., and Miller, S. I. (2011). The bacterial second messenger c-di-GMP: mechanisms of signalling. Cell Microbiol. 13, 1122–1129. doi: 10.1111/j.1462-5822.2011.01619.x
Nakano, Y., Yoshida, Y., Yamashita, Y., and Koga, T. (1995). Construction of a series of pACYC-derived plasmid vectors. Gene 162, 157–158. doi: 10.1016/0378-1119(95)00320-6
Oppenheimer-Shaanan, Y., Wexselblatt, E., Katzhendler, J., Yavin, E., and Ben-Yehuda, S. (2011). c-di-AMP reports DNA integrity during sporulation in Bacillus subtilis. EMBO Rep. 12, 594–601. doi: 10.1038/embor.2011.77
Parvatiyar, K., Zhang, Z., Teles, R. M., Ouyang, S., Jiang, Y., Iyer, S. S., et al. (2012). The helicase DDX41 recognizes the bacterial secondary messengers cyclic di-GMP and cyclic di-AMP to activate a type I interferon immune response. Nat. Immunol. 13, 1155–1161. doi: 10.1038/ni.2460
Peng, X., Zhang, Y., Bai, G., Zhou, X., and Wu, H. (2016). Cyclic di-AMP mediates biofilm formation. Mol. Microbiol. 99, 945–959. doi: 10.1111/mmi.13277
Pesavento, C., and Hengge, R. (2009). Bacterial nucleotide-based second messengers. Curr. Opin. Microbiol. 12, 170–176. doi: 10.1016/j.mib.2009.01.007
Rao, F., Ji, Q., Soehano, I., and Liang, Z. X. (2011). Unusual heme-binding PAS domain from YybT family proteins. J. Bacteriol. 193, 1543–1551. doi: 10.1128/JB.01364-10
Rao, F., See, R. Y., Zhang, D., Toh, D. C., Ji, Q., and Liang, Z. X. (2010). YybT is a signaling protein that contains a cyclic dinucleotide phosphodiesterase domain and a GGDEF domain with ATPase activity. J. Biol. Chem. 285, 473–482. doi: 10.1074/jbc.M109.040238
Shine, J., and Dalgarno, L. (1974). The 3′-terminal sequence of Escherichia coli 16S ribosomal RNA: complementarity to nonsense triplets and ribosome binding sites. Proc. Natl. Acad. Sci. U.S.A. 71, 1342–1346. doi: 10.1073/pnas.71.4.1342
Song, J. H., Ko, K. S., Lee, J. Y., Baek, J. Y., Oh, W. S., Yoon, H. S., et al. (2005). Identification of essential genes in Streptococcus pneumoniae by allelic replacement mutagenesis. Mol. Cells 19, 365–374.
Sureka, K., Choi, P. H., Precit, M., Delince, M., Pensinger, D. A., Huynh, T. N., et al. (2014). The cyclic dinucleotide c-di-AMP is an allosteric regulator of metabolic enzyme function. Cell 158, 1389–1401. doi: 10.1016/j.cell.2014.07.046
Tang, Q., Luo, Y., Zheng, C., Yin, K., Ali, M. K., Li, X., et al. (2015). Functional Analysis of a c-di-AMP-specific Phosphodiesterase MsPDE from Mycobacterium smegmatis. Int. J. Biol. Sci. 11, 813–824. doi: 10.7150/ijbs.11797
Taylor, B. L., and Zhulin, I. B. (1999). PAS domains: internal sensors of oxygen, redox potential, and light. Microbiol. Mol. Biol. Rev. 63, 479–506.
Thenisch, N. L., Bachmann, L. M., Imfeld, T., Leisebach Minder, T., and Steurer, J. (2006). Are mutans streptococci detected in preschool children a reliable predictive factor for dental caries risk? A systematic review. Caries Res. 40, 366–374. doi: 10.1159/000094280
Witte, G., Hartung, S., Buttner, K., and Hopfner, K. P. (2008). Structural biochemistry of a bacterial checkpoint protein reveals diadenylate cyclase activity regulated by DNA recombination intermediates. Mol. Cell 30, 167–178. doi: 10.1016/j.molcel.2008.02.020
Woodward, J. J., Iavarone, A. T., and Portnoy, D. A. (2010). c-di-AMP secreted by intracellular Listeria monocytogenes activates a host type I interferon response. Science 328, 1703–1705. doi: 10.1126/science.1189801
Xie, Z., Okinaga, T., Qi, F., Zhang, Z., and Merritt, J. (2011). Cloning-independent and counterselectable markerless mutagenesis system in Streptococcus mutans. Appl. Environ. Microbiol. 77, 8025–8033. doi: 10.1128/AEM.06362-11
Yamagata, A., Kakuta, Y., Masui, R., and Fukuyama, K. (2002). The crystal structure of exonuclease RecJ bound to Mn2+ ion suggests how its characteristic motifs are involved in exonuclease activity. Proc. Natl. Acad. Sci. U.S.A. 99, 5908–5912. doi: 10.1073/pnas.092547099
Yang, J., Bai, Y., Zhang, Y., Gabrielle, V. D., Jin, L., and Bai, G. (2014). Deletion of the cyclic di-AMP phosphodiesterase gene (cnpB) in Mycobacterium tuberculosis leads to reduced virulence in a mouse model of infection. Mol. Microbiol. 93, 65–79. doi: 10.1111/mmi.12641
Yang, J., Ritchey, M., Yoshida, Y., Bush, C. A., and Cisar, J. O. (2009). Comparative structural and molecular characterization of ribitol-5-phosphate-containing Streptococcus oralis coaggregation receptor polysaccharides. J. Bacteriol. 191, 1891–1900. doi: 10.1128/JB.01532-08
Yano, A., Kikuchi, S., Takahashi, T., Kohama, K., and Yoshida, Y. (2012). Inhibitory effects of the phenolic fraction from the pomace of Vitis coignetiae on biofilm formation by Streptococcus mutans. Arch. Oral Biol. 57, 711–719. doi: 10.1016/j.archoralbio.2012.01.001
Ye, M., Zhang, J. J., Fang, X., Lawlis, G. B., Troxell, B., Zhou, Y., et al. (2014). DhhP, a cyclic di-AMP phosphodiesterase of Borrelia burgdorferi, is essential for cell growth and virulence. Infect. Immun. 82, 1840–1849. doi: 10.1128/IAI.00030-14
Yoshida, Y., Ganguly, S., Bush, C. A., and Cisar, J. O. (2005). Carbohydrate engineering of the recognition motifs in streptococcal co-aggregation receptor polysaccharides. Mol. Microbiol. 58, 244–256. doi: 10.1111/j.1365-2958.2005.04820.x
Keywords: cyclic di-AMP, phosphodiesterase, Streptococcus mutans, biofilm, pApA
Citation: Konno H, Yoshida Y, Nagano K, Takebe J and Hasegawa Y (2018) Biological and Biochemical Roles of Two Distinct Cyclic Dimeric Adenosine 3′,5′-Monophosphate- Associated Phosphodiesterases in Streptococcus mutans. Front. Microbiol. 9:2347. doi: 10.3389/fmicb.2018.02347
Received: 06 July 2018; Accepted: 12 September 2018;
Published: 27 September 2018.
Edited by:
Jörg Stülke, Georg-August-Universität Göttingen, GermanyReviewed by:
Juan Carlos Alonso, Centro Nacional de Biotecnología (CNB), SpainGerd M. Seibold, Universität Ulm, Germany
Copyright © 2018 Konno, Yoshida, Nagano, Takebe and Hasegawa. This is an open-access article distributed under the terms of the Creative Commons Attribution License (CC BY). The use, distribution or reproduction in other forums is permitted, provided the original author(s) and the copyright owner(s) are credited and that the original publication in this journal is cited, in accordance with accepted academic practice. No use, distribution or reproduction is permitted which does not comply with these terms.
*Correspondence: Yasuo Yoshida, eWFzdW95QGRwYy5hZ3UuYWMuanA=
†These authors have contributed equally to this work