- Departamento de Biologia Celular e Molecular e Bioagentes Patogênicos, Faculdade de Medicina de Ribeirão Preto, Universidade de São Paulo, São Paulo, Brazil
Chromobacterium violaceum is an environmental Gram-negative bacterium that causes infections in humans. Treatment of C. violaceum infections is difficult and little is known about the mechanisms of antibiotic resistance in this bacterium. In this work, we identified mutations in the MarR family transcription factor EmrR and in the protein GyrA as key determinants of quinolone resistance in C. violaceum, and we defined EmrR as a repressor of the MFS-type efflux pump EmrCAB. Null deletion of emrR caused increased resistance to nalidixic acid, but not to other quinolones or antibiotics of different classes. Moreover, the ΔemrR mutant showed decreased production of the purple pigment violacein. Importantly, we isolated C. violaceum spontaneous nalidixic acid-resistant mutants with a point mutation in the DNA-binding domain of EmrR (R92H), with antibiotic resistance profile similar to that of the ΔemrR mutant. Other spontaneous mutants with high MIC values for nalidixic acid and increased resistance to fluoroquinolones presented point mutations in the gene gyrA. Using DNA microarray, Northern blot and EMSA assays, we demonstrated that EmrR represses directly a few dozen genes, including the emrCAB operon and other genes related to transport, oxidative stress and virulence. This EmrR repression on emrCAB was relieved by salicylate. Although mutation of the C. violaceum emrCAB operon had no effect in antibiotic susceptibility or violacein production, deletion of emrCAB in an emrR mutant background restored antibiotic susceptibility and violacein production in the ΔemrR mutant. Using a biosensor reporter strain, we demonstrated that the lack of pigment production in ΔemrR correlates with the accumulation of quorum-sensing molecules in the cell supernatant of this mutant strain. Therefore, our data revealed that overexpression of the efflux pump EmrCAB via mutation and/or derepression of EmrR confers quinolone resistance and alters quorum-sensing signaling in C. violaceum, and that point mutation in emrR can contribute to emergence of antibiotic resistance in bacteria.
Introduction
Antibiotic resistance is a global public health problem with high impact on the treatment of bacterial infections, as many multidrug-resistant (MDR) strains have evolved in clinically relevant pathogens (Davies and Davies, 2010; Rossolini et al., 2014). Several mechanisms can mediate intrinsic and acquired resistance, including antibiotic removal by membrane efflux pumps, inactivation of the antibiotic, modification of the antibiotic target, and preventing the entrance of antibiotics into the cell (Cox and Wright, 2013; Blair et al., 2015). In most cases, antibiotic-resistant strains arise by mutations in transcription factors that regulate the genes involved in antibiotic resistance. For instance, a common pathway that leads to overexpression of efflux pumps in MDR bacteria is the occurrence of mutations in transcription factors that regulate the genes encoding these efflux pumps (Fernandez and Hancock, 2012; Blair et al., 2015).
Members of the multiple antibiotic resistance regulator (MarR) family of transcription factors are involved in several biological processes in bacteria, including oxidative stress, virulence, and antibiotic resistance; most of them act as transcription repressors (Deochand and Grove, 2017). The first described member of this family, the marR gene of Escherichia coli, represses the marRAB operon, involved in resistance to multiple antibiotics (George and Levy, 1983; Seoane and Levy, 1995). Other MarR family transcription factors have been described regulating efflux pumps that contribute to antibiotic resistance, such as MexR in Pseudomonas aeruginosa (Chen et al., 2008), MepR in Staphylococcus aureus (Birukou et al., 2013), MarR in Mycobacterium smegmatis (Zhang et al., 2014), and EmrR in E. coli (Lomovskaya and Lewis, 1992). Mutation in emrR, or compounds such as salicylate, disrupt the repression of EmrR on the operon emrRAB, allowing expression of the major facilitator superfamily (MFS) efflux pump EmrAB, which exports hydrophobic compounds such as the antibiotic nalidixic acid (Lomovskaya and Lewis, 1992; Xiong et al., 2000). It has been suggested that EmrAB could form a tripartite pump with the outer membrane protein TolC (Tanabe et al., 2009). Although a similar mechanism has been described controlling the EmrCABsm efflux pump in Stenotrophomonas maltophilia (Huang et al., 2013), it is still unclear whether antibiotic resistance can emerge as a consequence of point mutation in the emrR gene, and other genes regulated by EmrR have not been identified.
Chromobacterium violaceum, a Gram-negative free-living, saprophytic bacterium found in waters and soils of tropical and subtropical regions, is an opportunistic pathogen that causes infections with rapid dissemination and high mortality (Yang and Li, 2011; Batista and da Silva Neto, 2017). It produces the microbicidal purple pigment violacein, whose synthesis is activated by the N-acyl-L-homoserine lactone (AHL)-based quorum-sensing system CviI/CviR (Stauff and Bassler, 2011; Durán et al., 2016). Thus, deletion of the AHL-synthase gene cviI generates a biosensor strain that produce violacein only when exogenous AHL molecules are provided (Morohoshi et al., 2008). Although infection by C. violaceum in the hospital environment is uncommon, cases of nosocomial pneumonia caused by C. violaceum have been reported in patients in intensive care units (Hagiya et al., 2014). Failure in treating C. violaceum infections relates to resistance to some antibiotics, especially β-lactams, but this bacterium is susceptible to quinolones and carbapenems (Aldridge et al., 1988; Yang and Li, 2011; Hagiya et al., 2014; Madi et al., 2015). Recently, it has been suggested that class A β-lactamases (KPC) evolved from the Chromobacterium genus (Gudeta et al., 2016). Investigating the regulatory mechanisms that control antibiotic resistance in C. violaceum is key to understanding how resistance to currently useful antibiotics can emerge under conditions such as those found during the treatment of C. violaceum infections. In this work, we identified the MarR family transcription factor EmrR as a regulator of antibiotic resistance in C. violaceum. We defined the repertoire of genes regulated by EmrR, and characterized both null and spontaneous emrR mutants by antimicrobial susceptibility assays.
Materials and Methods
Bacterial Strains, Growth Conditions, Plasmids, and Oligonucleotides
Bacterial strains and plasmids used in this work are listed in Table 1. Mutant strains of C. violaceum were derived from the wild-type strain ATCC 12472 (Vasconcelos et al., 2003). All bacterial strains of C. violaceum and E. coli were grown at 37°C in Luria-Bertani (LB) medium. Antibiotic susceptibility assays were performed on Mueller-Hinton (MH) medium (Sigma). For plasmid selection in general cloning procedures, LB medium was supplemented with the antibiotics tetracycline (10 μg/ml), kanamycin (50 μg/ml), and ampicillin (100 μg/ml). Oligonucleotide sequences are listed in Supplementary Table S1.
Construction and Complementation of Mutant Strains
The in-frame emrR, emrCAB, emrRCAB, and cviI gene deletions were constructed by a two-step allelic exchange procedure with the vector pNPTS138 as previously described (da Silva Neto et al., 2012). The resulting mutant strains ΔemrR, ΔemrCAB, ΔemrRCAB, and ΔcviI were confirmed by PCR using specific primers (Supplementary Table S1). For complementation of emrR mutants, a DNA fragment containing the full emrR gene was cloned into the vector pMR20 and this construct was introduced into the mutant strains by conjugation (da Silva Neto et al., 2012).
Selection of Spontaneous Nalidixic Acid-Resistant Mutants
Selection was performed by plating 500 μl of an LB-grown overnight culture of C. violaceum ATCC 12472 on LB plates with increased concentrations of nalidixic acid (0.5–64 μg/ml; 1–7 times the MIC). Several spontaneous resistant colonies that appeared on these plates after incubation at 37°C for 24 h were cultivated in LB without antibiotic. After testing these colonies for nalidixic acid susceptibility (see item MIC on LB plates), resistant colonies (4 μg/ml to 512 μg/ml) were selected for sequencing the full emrR and the QRDR region of gyrA, using specific primers (Supplementary Table S1). For Sanger DNA sequencing, the amplicons of emrR and gyrA were obtained by colony PCR and sequenced in both strands using BigDye Terminator V3.1 (Applied Biosystems).
Antibiogram by Disk Diffusion Assays
Disk diffusion assays were performed as recommended by the (Clinical and Laboratory Standards Institute [CLSI], 2013). Briefly, C. violaceum strains, grown on MH plates for 20 h, were resuspended in sterile saline and adjusted to 0.5 MacFarland turbidity standard. These suspensions were seeded onto MH plates using a sterile swab. On the surface of seeded plates were placed disks impregnated with 24 antibiotics (BD BBLTM Sensi-DiscTM Antimicrobial Susceptibility Test Discs) (Supplementary Table S2). The growth inhibition halos were recorded after 24 h incubation at 37°C. Disk diffusion assays were performed at least in biological triplicate.
MIC Assays
MICs of nalidixic acid, kanamycin, streptomycin, tetracycline, doxycycline, erythromycin, chloramphenicol, and cefotaxime for each C. violaceum strain were determined by a broth macrodilution method according to CLSI guidelines (Clinical and Laboratory Standards Institute [CLSI], 2013). The MIC values were determined as the lowest concentration of the antibiotic that inhibited visible bacterial growth after incubation of the cultures in MH broth for 24 h at 37°C under shaking.
MIC on LB Plates
The strains were grown on LB plates for 24 h, and streaked on LB plates with increasing concentrations of nalidixic acid (0.5, 1.0, and 2.0 μg/ml, for emrRCAB mutants). The susceptibility profiles of the spontaneous nalidixic acid-resistant mutants were determined using this method (1 μg/ml to 512 μg/ml of nalidixic acid). After 24 h incubation at 37°C, the bacterial growth was recorded.
Detection of Extracellular Quorum-Sensing Molecules
The presence of N-acyl-L-homoserine lactones (AHLs) in the culture supernatant of C. violaceum strains was evaluated by the production of violacein in the biosensor strain ΔcviI, using an agar plate assay, as previously described (Morohoshi et al., 2008). Briefly, filtered supernatants (80 μl) of overnight cultures were added inside wells sunken on LB agar plates soaked with the ΔcviI strain. After 48 h incubation at 25°C, the appearance of a violacein halo was recorded on the plates. Biofilm formation was assayed on polypropylene tubes with the strains cultured in LB medium for 16 h under static conditions, using the crystal violet method (Azeredo et al., 2017).
RNA Isolation
Chromobacterium violaceum strains were grown at 37°C in LB until mid-log phase (OD600 of 0.8–1.0). After cell harvesting by centrifugation, the total RNA was extracted with TRIzol reagent (Ambion), and purified with the illustra RNAspin Mini RNA isolation kit (GE Healthcare), which includes a DNase treatment step. To test emrCAB-inducing conditions by Northern blot, the wild-type strain ATCC 12472 was grown at 37°C in LB until OD600 of 0.8. Then, the culture was split into four aliquots and either left untreated or treated for 10 min with salicylate (0.1, 1, and 10 mM), nalidixic acid (0.1, 0.2, and 0.5 mM), or ethidium bromide (0.1, 0.2, and 0.5 mM), and the cells were used for RNA extraction. RNA concentration was determined with a NanoDrop spectrophotometer (Thermo Scientific) and RNA integrity was checked by using formaldehyde-denaturing agarose gels.
DNA Microarray Analysis
A detailed description of the custom-designed oligonucleotide microarray slides (Agilent Technologies) was published previously (Previato-Mello et al., 2017). All procedures for cRNA labeling, hybridization, and washing of the slides as well as data acquisition, extraction, and normalization were performed exactly as described (Previato-Mello et al., 2017), and following manufacturer’s instructions (Agilent Technologies). Data sets included three independent biological experiments with RNA extracted from C. violaceum ATCC 12472 and ΔemrR strains grown in LB at 37°C until mid-log phase as stated above. Differentially expressed genes were those that had their expression levels altered at least two-fold (ΔemrR/WT).
Accession Number(s)
Microarray raw data have been deposited in the Gene Expression Omnibus (GEO) database1 with accession number GSE112521.
Northern Blot Analysis
Samples of total RNA (7 μg) extracted as stated above were used for Northern blot assays as previously described (da Silva Neto et al., 2012; Previato-Mello et al., 2017). Specific probes for each indicated gene were amplified by PCR (primers listed in Supplementary Table S1), and labeled with [α-32P]dCTP (PerkinElmer) by using an Exo-Klenow enzyme DECAprime II kit (Ambion). After membrane hybridization in ULTRAhyb buffer (Ambion) and washing, the signal was detected by autoradiography.
Expression and Purification of EmrR
The coding region of the C. violaceum emrR gene (CV_0769) was amplified by PCR using specific primers (Supplementary Table S1), and cloned into the vector pET15b as a 501-pb NdeI/BamHI DNA fragment. The recombinant His-EmrR protein was produced in E. coli BL21(DE3) after induction with 1 mM IPTG, and purified by NTA-resin affinity chromatography (Qiagen) as previously described (da Silva Neto et al., 2012).
Western Blot
Proteins from total extracts of C. violaceum strains were separated on 15% SDS-PAGE gels, and transferred onto a nitrocellulose membrane (Amershan Protran). Membranes were blocked and incubated with a 1:1,000 dilution of anti-EmrR mouse polyclonal antiserum. After membrane incubation with a secondary anti-mouse IgG conjugated to peroxidase, detection was performed using the LumiGLO western blotting protein detector kit as recommended by the manufacturer (KPL). The polyclonal anti-EmrR antibody was developed in mice according to an experimental protocol approved by the Local Ethical Animal Committee (CEUA) of FMRP-USP (protocol number 147/2014).
Electrophoretic Mobility Shift Assay (EMSA)
DNA probes corresponding to promoter regions of indicated genes were amplified by PCR from the ATCC 12472 genome, using specific primers (Supplementary Table S1). These DNA fragments were end labeled with [γ-32P]ATP (PerkinElmer) by using T4 polynucleotide kinase (Thermo Scientific) and purified with the Wizard® SV gel and PCR clean-up system (Promega). The DNA binding reactions containing the DNA probes and different amounts of DTT-reduced His-EmrR were performed in an interaction buffer, as previously described (da Silva Neto et al., 2012; Previato-Mello et al., 2017).
Results
Null Deletion of emrR Confers Resistance to Nalidixic Acid
In the genome of C. violaceum ATCC 12472 there are at least 15 genes encoding MarR family transcription factors (Vasconcelos et al., 2003), but only ohrR has been characterized (da Silva Neto et al., 2012; Previato-Mello et al., 2017). Analysis of sequence alignment and genomic position indicated that CV_0769 and the nearby genes CV_0768, CV_0767, and CV_0766 resemble the emrR, emrCAB cluster (Figure 1A) described in other bacteria, which encode the MarR family transcription factor EmrR and the MFS-type efflux pump EmrCAB (Lomovskaya and Lewis, 1992; Huang et al., 2013). To define the role of EmrR in antibiotic resistance, we constructed and characterized an emrR null mutant strain by antimicrobial susceptibility assays. Antibiogram tests with 24 antibiotics (Supplementary Figure S1) and determination of MIC with eight antibiotics (Supplementary Table S3) revealed that the ΔemrR mutant showed increased resistance specifically to nalidixic acid, a quinolone (a 13-mm decrease in the halo and a fourfold increase in the MIC). After complementation of the ΔemrR mutant (Figure 1B), this increased resistance to nalidixic acid was reverted, as determined by disk diffusion (Figure 2A), and MIC (Table 2). A phenotype of decreased violacein production, verified for the ΔemrR mutant in LB liquid cultures, was also complemented (Figure 2B). These results indicate that EmrR controls antibiotic resistance and pigment production in C. violaceum.
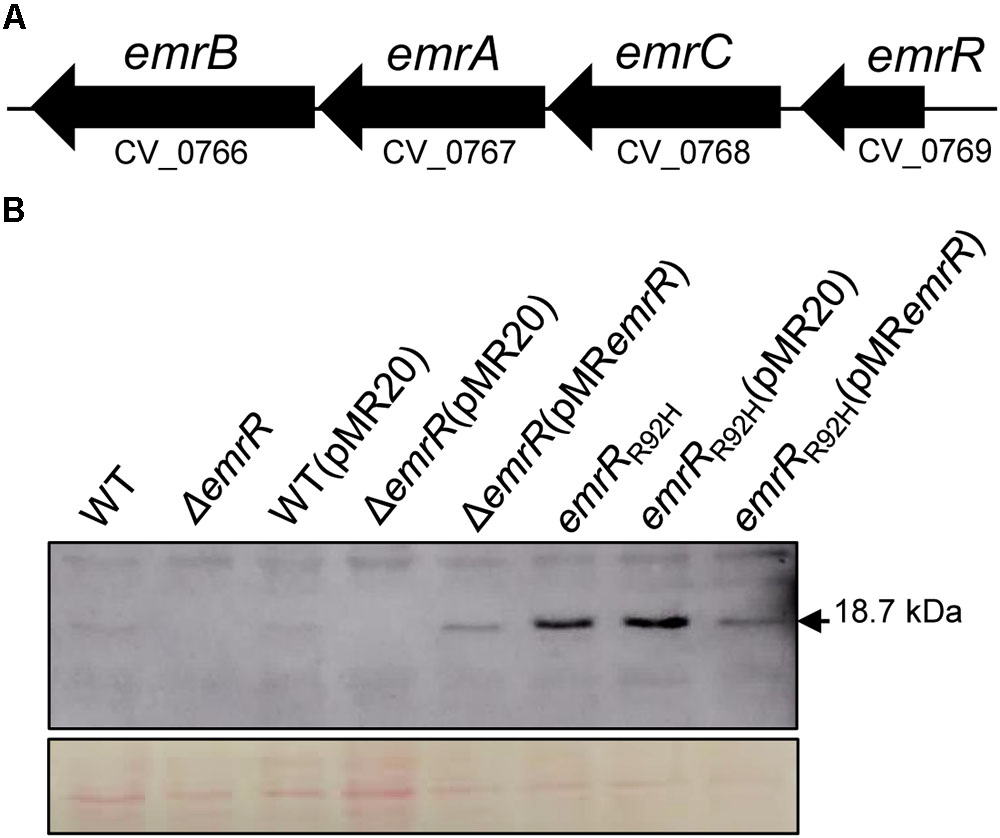
FIGURE 1. Genomic analysis of the emrR/emrCAB cluster and molecular characterization of emrR mutant strains. (A) Scheme showing that the gene emrR is located near to the operon emrCAB on the chromosome of C. violaceum. (B) Western blot confirmed that deletion of the emrR gene abolished production of the EmrR protein, while point mutation in emrR resulted in an EmrR variant unable to autorepress its own production. Levels of EmrR in the indicated C. violaceum strains detected with anti-EmrR antiserum. Equal protein loading determined by membrane staining with Ponceau S (bottom panel).
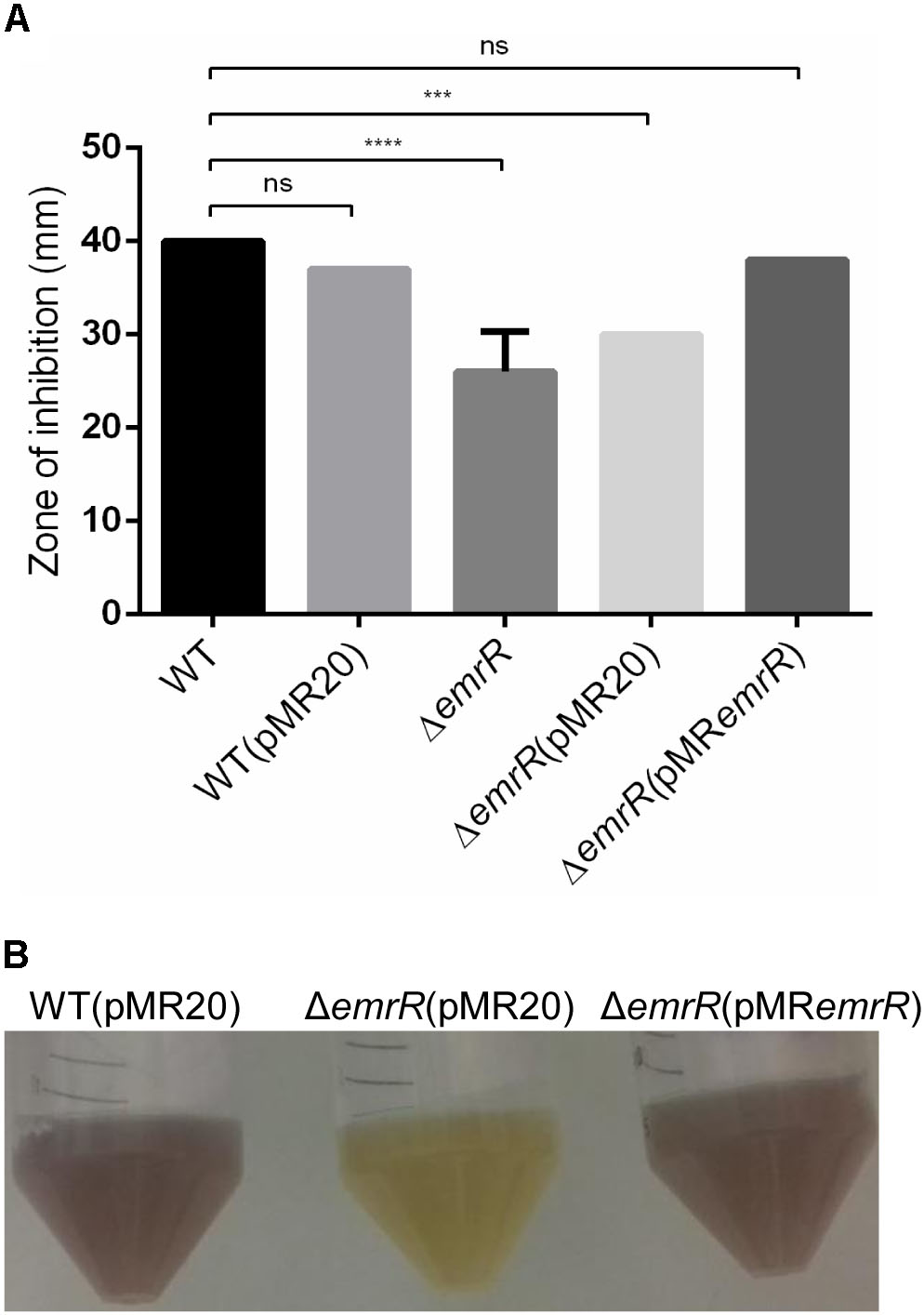
FIGURE 2. Null deletion of emrR confers increased resistance to nalidixic acid and decreased production of violacein. (A) Disk diffusion assays for nalidixic acid performed on MH plates in biological triplicate with the indicated C. violaceum strains. The error bars indicate standard deviations. (B) Decreased production of violacein in the null mutant emrR. The indicated strains were grown in LB broth at 37°C for 24 h. P-values were determined by one-way ANOVA Tukey’s multiple comparisons test: ∗∗∗∗P < 0.0001; ∗∗∗P = 0.0007.
Point Mutations in Both EmrR and GyrA Confer Nalidixic Acid Resistance
To evaluate whether the emergence of nalidixic acid-resistant mutants can occur by point mutation in emrR, we isolated spontaneous mutants from C. violaceum wild-type cultivated in LB with increasing concentrations of nalidixic acid (0.5–64 μg/ml), and determined the MIC of these mutants (Table 3). DNA sequencing revealed that three isolates with MIC of 4 μg/ml had two types of mutation in emrR (substitution and deletion), while for the 25 remaining isolates sequenced the mutations occurred in gyrA, a hotspot gene for mutations that confer quinolone resistance (Giles et al., 2004). We selected two spontaneous mutants, emrRR92H and gyrAT85I, for further characterization (Figure 3). In emrRR92H, histidine replaced arginine at position 92 of EmrR. As this substitution arose in the second (position 91–106) of a four-element fingerprint signature for the MarR family predicted in EmrR (Figure 3A) and the levels of the protein EmrR were increased in the emrRR92H strain probably by loss of self-repression of the regulator (Figure 1B), we suggest that this point mutation affects the DNA binding activity of EmrR. In fact, emrRR92H displayed the same antibiotic resistance profile observed for the null mutant ΔemrR, namely increased resistance to nalidixic acid, but not to other quinolones (Figures 3A,B). Although all point mutations in gyrA mapped within the quinolone resistance determining region (QRDR) of GyrA (position 67–106), the substitution of threonine for isoleucine at position 85 of GyrA had particular impact on antibiotic resistance, as the gyrAT85I mutant showed the highest level of resistance to nalidixic acid, and presented cross resistance to other quinolones, such as ciprofloxacin, norfloxacin, and levofloxacin (Table 3 and Figure 3C). These results indicate that point mutations in EmrR and GyrA are related to the emergence of quinolone resistance in C. violaceum.
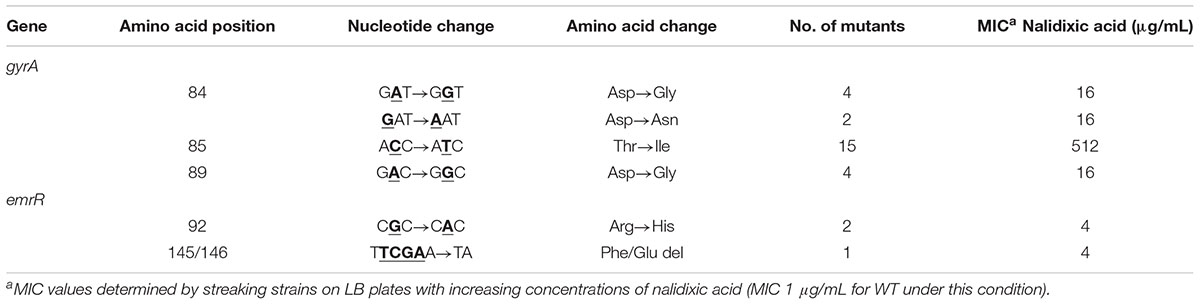
TABLE 3. Point mutations and amino acid changes in emrR and gyrA genes associated with increased resistance to nalidixic acid in C. violaceum.
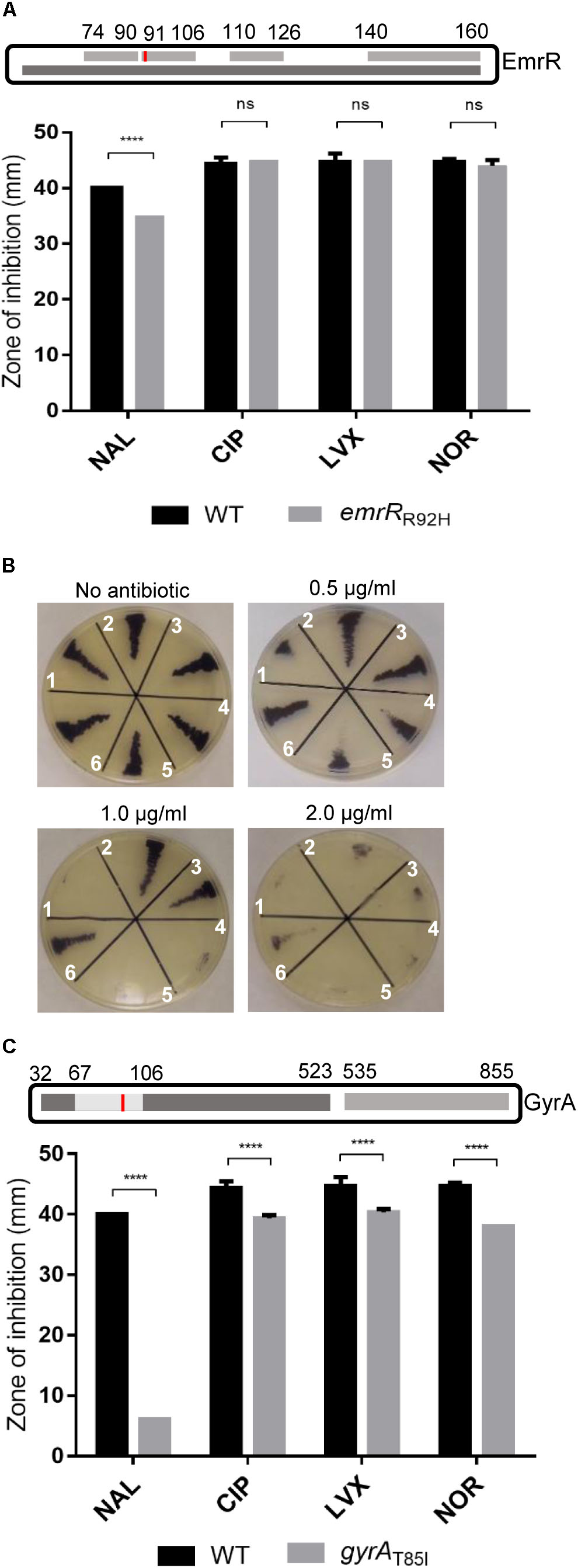
FIGURE 3. Point mutations in EmrR and GyrA confer distinct levels of quinolone resistance in C. violaceum. (A) Profile of quinolone resistance in the spontaneous mutant emrRR92H indicated specific resistance to nalidixic acid. (Upper panel) Scheme showing the MarR domain of the EmrR protein (dark gray, entry IPR000835 from the InterPro database) with a predicted four-element fingerprint signature for the MarR family (light gray, entry PR00598 from the PRINTS database). Substitution of arginine for histidine at position 92 found in the mutant emrRR92H is indicated (red bar). (Bottom panel) Disk diffusion assay performed on MH plates in triplicate with the indicated strains to verify the susceptibility to nalidixic acid (NAL), ciprofloxacin (CIP), norfloxacin (NOR), and levofloxacin (LVX). (B) MIC assay on LB plate with increasing concentrations of nalidixic acid in emrR mutant strains. (1) C. violaceum ATCC 12472; (2) ΔemrR; (3) emrRR92H; (4) WT(pMR20); (5) ΔemrR(pMRemrR); (6) ΔemrR(pMR20). (C) Point mutation in gyrA conferred resistance to multiple quinolones. (Upper panel) Schema locating the mutation in threonine 85 (red bar) of the spontaneous mutant gyrAT85I within the QRDR region (amino acids 67–106) of GyrA. Domains of GyrA are indicated (DNA topoisomerase N and C-terminal domains indicated in dark and light gray). (Bottom panel) Disk diffusion assay for gyrAT85I mutant with NAL, CIP, NOR, and LVX as stated in A. EmrR and GyrA proteins in the scheme are not in scale. P-values were determined by two-way ANOVA Sidak’s multiple comparisons test: ∗∗∗∗P < 0.0001.
The EmrR Regulon Comprises Mostly EmrR-Repressed Genes, Including Other Genes Related to Transport Besides emrCAB
To identify the full repertoire of genes regulated by EmrR, we compared, by DNA microarray analysis, the transcriptome of the wild-type and ΔemrR mutant strains grown in LB medium, at log phase. From this analysis, 22 genes were upregulated and 14 genes were downregulated in the ΔemrR mutant compared to wild-type strain (Supplementary Table S4), indicating that EmrR acts mostly as a transcription repressor. The EmrR-repressed genes discussed in the text are shown in Figure 4A, and most of them were validated by Northern blot (Figure 4B) and EMSA (see the next item). Among the genes upregulated in the ΔemrR mutant, CV_0766 (emrB), CV_0767 (emrA), and CV_0768 (emrC) showed more than ten-fold increase in expression in the mutant. These genes compose the operon emrCAB, which encodes the putative MFS-type efflux pump EmrCAB (Figure 1A). However, other genes encoding putative transport proteins were upregulated (pcaK, CV_1769, crcB, CV_3014), including three MFS-type transporters. Interestingly, some genes from a pathogenicity island required for C. violaceum virulence (cipA, cipB) (Miki et al., 2010), and genes related to the response of C. violaceum to oxidative stress (garA, gstA) (Previato-Mello et al., 2017) were also upregulated in the ΔemrR mutant (Supplementary Table S4 and Figure 4). These data suggest that EmrR acts as a repressor of several putative transporters, including the EmrCAB efflux pump, and also regulates genes involved in other processes (virulence and oxidative stress).
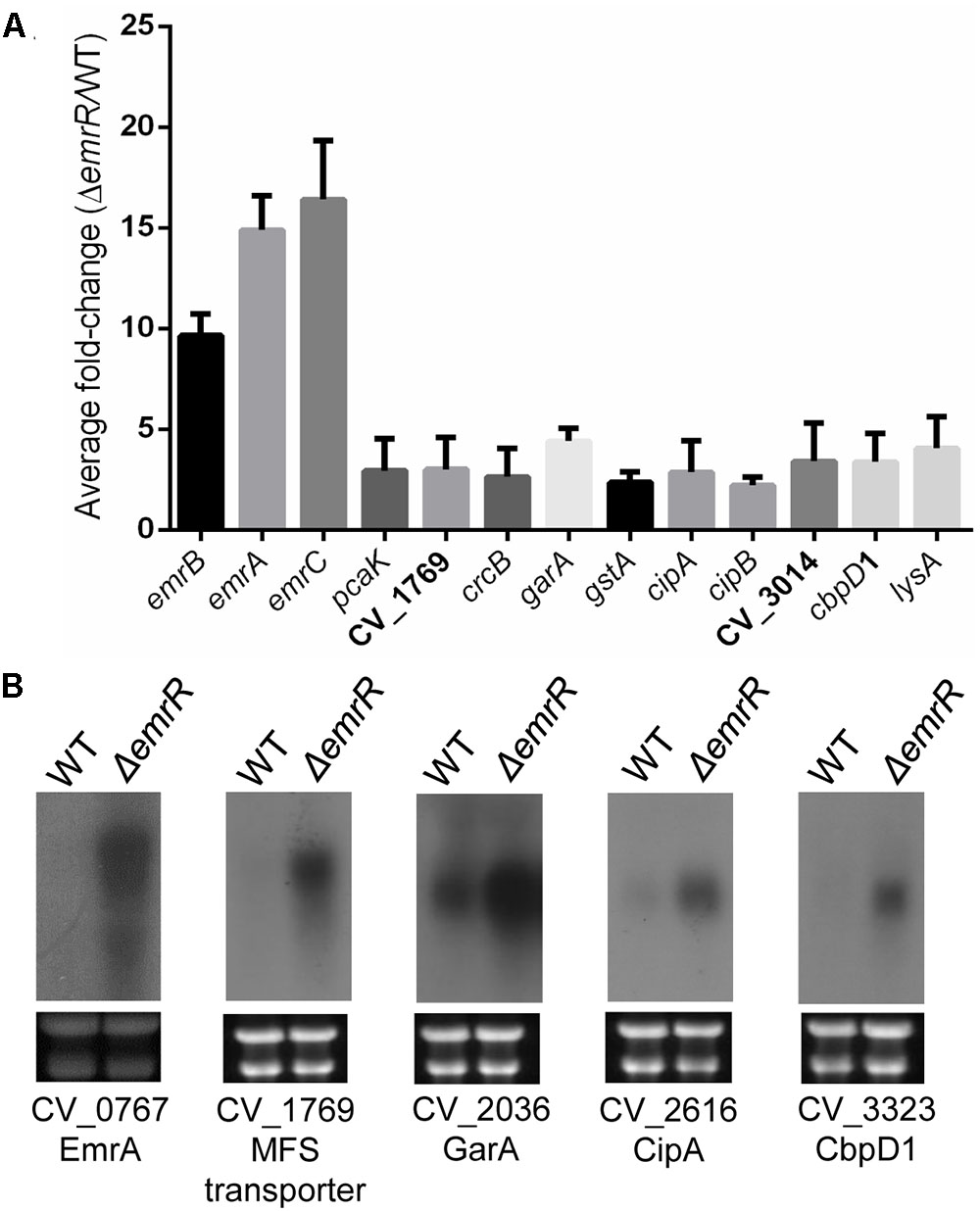
FIGURE 4. The EmrR regulon includes mainly EmrR-repressed genes. (A) Data from DNA microarray analysis for selected EmrR-repressed genes are shown as average ratios with standard deviations from three biological replicates comparing transcriptome from C. violaceum ATCC 12472 with ΔemrR mutant. For a complete list of the genes with altered expression levels, see Supplementary Table S4. (B) Northern blot assays validated several genes as members of the EmrR regulon. Total RNA extracted from C. violaceum ATCC 12472 and ΔemrR mutant were probed for the indicated genes. Levels of rRNA indicated equal RNA loading (bottom panels).
EmrR Acts as a Direct Transcription Repressor in C. violaceum
Most MarR family transcription factors act as repressors by direct binding in the promoter region of their target genes (Perera and Grove, 2010). Thus, we employed EMSA to determine whether EmrR acts as a repressor by direct interaction with promoters of the EmrR-repressed genes. After purification of EmrR as a Histag recombinant protein with high degree of purity and homogeneity (Supplementary Figure S2), the labeled DNA fragments for promoter region of nine selected genes were incubated with increasing concentrations of EmrR. For all probes, the shift was first observed at 100 nM EmrR, whereas for a non-specific probe, the shift occurred only at 500 nM EmrR (Figure 5). Competition assays using specific and non-specific unlabeled DNA confirmed the specificity of EmrR binding (Figure 5, right panels). These data confirm that EmrR directly repressed these genes, including the emrR gene itself, as well as the operon emrCAB.
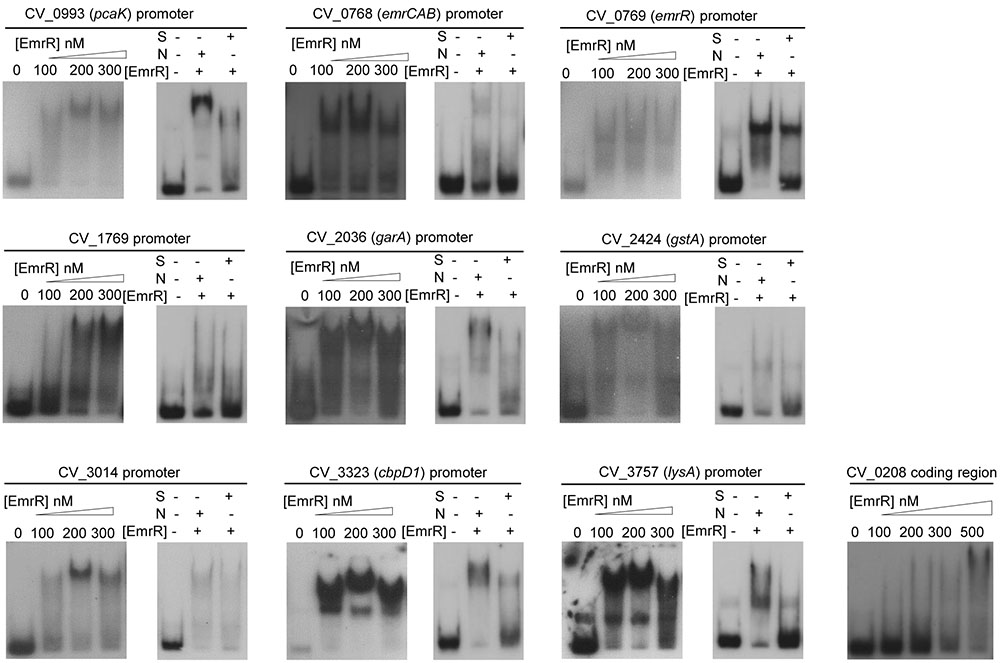
FIGURE 5. EmrR acts as a direct transcription repressor. EMSA assays with labeled probes containing the promoter regions of the indicated EmrR target genes, incubated with different concentrations of purified EmrR protein (100, 200, and 300 nM EmrR). For all cases, competition assays were performed (right panels) using 200 nM EmrR in the presence of 30-fold excess of unlabeled fragments of the same region (S) or the coding region of CV_0208 (N) as competitors. Differences in some competition assays suggest that EmrR has distinct affinities for its binding sites. A control assay performed with the coding region of CV_0208 indicated that at 500 nM, EmrR bound in a nonspecific manner.
Nalidixic Acid Resistance and Decreased Violacein Production in emrR Mutants Are Due to Derepression of the Efflux Pump EmrCAB
DNA microarray analysis and Northern blot assay using the wild-type and ΔemrR strains indicated that EmrR repressed the operon emrCAB (Figure 4). To further reinforce the regulation of emrCAB by EmrR, the Northern blot assays were performed with other strains and conditions (Figure 6). When the strains were grown in LB medium, the operon emrCAB was highly expressed in both the ΔemrR and emrRR92H mutant strains, whereas in the wild-type and complemented strains its expression was fully repressed, suggesting that ΔemrR and emrRR92H produce the EmrCAB efflux pump at high levels (Figure 6A). To test if emrCAB expression can increase in wild-type C. violaceum in response to other compounds, salicylate, ethidium bromide, and nalidixic acid were tested with Northern blot assays. Only salicylate induced the operon emrCAB in a dose-dependent manner (Figure 6B). To confirm whether the phenotypes of increased nalidixic acid resistance and decreased violacein production in the ΔemrR mutant were because this strain overexpresses emrCAB, we constructed and characterized ΔemrCAB and ΔemrRCAB mutant strains (Figure 7). Deletion of emrCAB in the wild-type strain had no effect on susceptibility to several antibiotics (Supplementary Figure S1), including nalidixic acid (Table 2, Supplementary Figures S1, and Figure 7B), nor altered violacein production (Figure 7C) in relation to the wild-type strain, suggesting that the absence of the EmrCAB efflux pump can be replaced by other efflux pumps. On the other hand, deletion of emrCAB in the ΔemrR background (strain ΔemrRCAB) restored nalidixic acid susceptibility (Table 2 and Figures 7A,B) and violacein production (Figure 7C) altered in the ΔemrR mutant, confirming that EmrR controls these phenotypes via EmrCAB. As violacein production is activated by AHL-mediated signaling in C. violaceum (Morohoshi et al., 2008; Stauff and Bassler, 2011), we thought that the absence of violacein in ΔemrR could be related to the secretion of AHL mediated by EmrCAB. Indeed, using the biosensor strain ΔcviI, which does not produce AHLs nor violacein, but does produce violacein in response to exogenous AHL, we verified that ΔemrR accumulated extracellular AHLs (Figures 7D,E) and presented decreased biofilm formation (Figure 7F), suggesting that overexpression of EmrCAB decreases the intracellular accumulation of AHLs and of nalidixic acid.
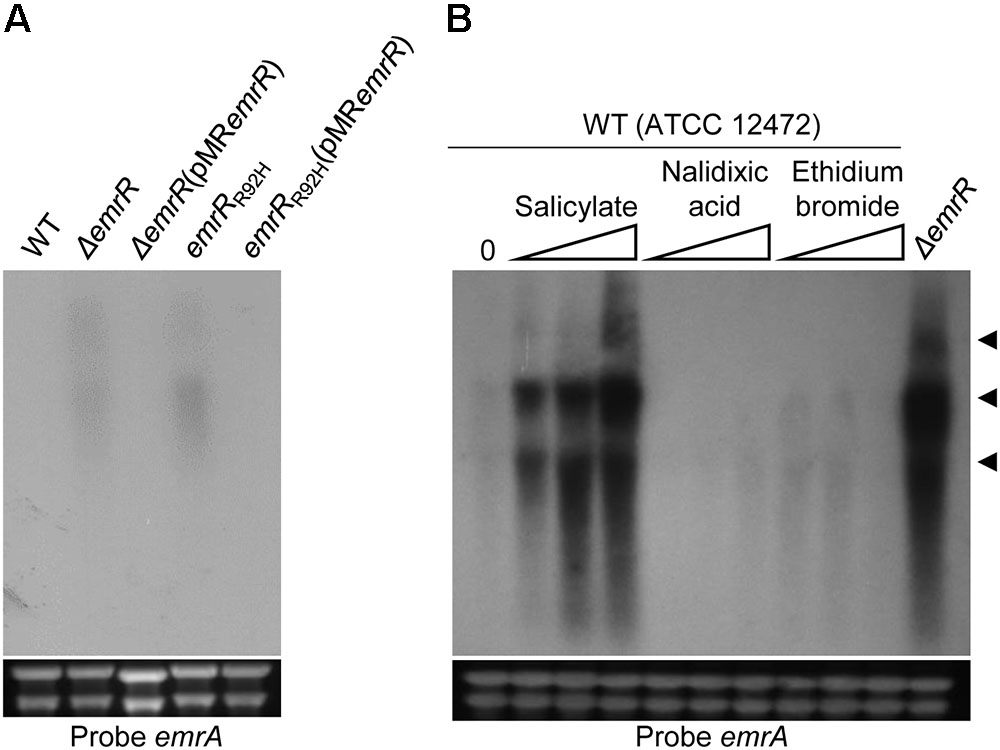
FIGURE 6. Nalidixic acid resistance in emrR mutants correlated to overexpression of the efflux pump EmrCAB. (A) Northern blot analysis using total RNA from the indicated strains grown in LB medium revealed that null or point mutation in emrR causes overexpression of emrCAB. (B) Salicylate caused derepression of emrCAB. Northern blot assay was performed using total RNA extracted from the wild-type strain ATCC 12472 before (0) or after exposure for 10 min to salicylate (0.1, 1.0, and 10 mM), nalidixic acid, and ethidium bromide (0.1, 0.2, and 0.5 mM), or RNA extracted from ΔemrR grown in LB medium. Levels of rRNA were used as a loading control (bottom panels). RNA samples were hybridized with a probe for emrA. Head arrows indicate putative isoform transcripts of the emrCAB operon. Distinct band intensity between (A,B) reflects differences in time exposure.
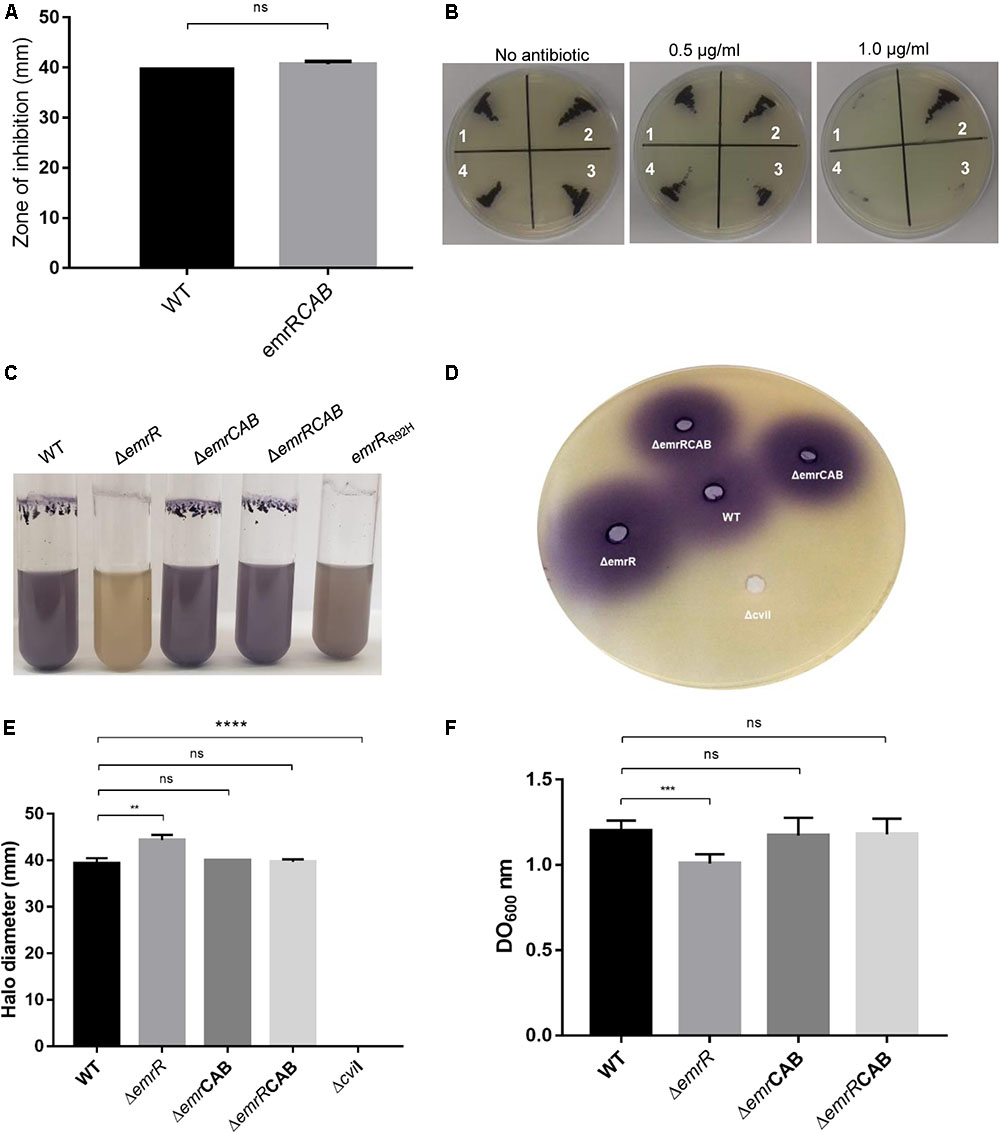
FIGURE 7. The phenotypes of increased antibiotic resistance and decreased violacein production in ΔemrR are relieved by deletion of emrCAB in this mutant strain. (A) Disk diffusion assays for nalidixic acid performed on MH plates in triplicate with the indicated C. violaceum strains. The error bars indicate standard deviations. (B) MIC assay on LB plate with increasing concentrations of nalidixic acid: (1) C. violaceum ATCC 12472; (2) ΔemrR; (3) ΔemrCAB; (4) ΔemrRCAB. (C) Production of violacein in the C. violaceum strains. The indicated strains were grown in LB broth at 37°C for 24 h. The emrRR92H mutant produces a little more violacein than the ΔemrR mutant does, but both strains have decreased violacein production. (D,E) Quantification of violacein production in the biosensor strain ΔcviI (poured into the agar) stimulated by supernatants of the indicated strains revealed that ΔemrR accumulates extracellular AHLs. Supernatant of a ΔcviI culture was used as negative control. (F) Quantification of biofilm production by the indicated strains grown under static conditions. P-values were determined by t-test Student: ∗∗P = 0.0061; ∗∗∗P < 0.001; ∗∗∗∗P < 0.0001.
Discussion
In this work, we characterized the involvement of the MarR family transcription factor EmrR in antibiotic resistance and violacein production in C. violaceum. The mutant strain ΔemrR showed increased resistance to the antibiotic nalidixic acid and decreased production of violacein. Because these phenotypes were relieved after simultaneous deletion of emrR and emrCAB, we propose that EmrR acts in these cases via the MFS-type efflux pump EmrCAB. Moreover, we isolated spontaneous mutant strains in increased nalidixic acid concentration that had point mutations in emrR and displayed phenotypic characteristics similar to the null mutant ΔemrR. This suggests that point mutation in emrR can contribute to emergence of antibiotic resistance. Null deletion or point mutation in emrR, or molecules that attenuate EmrR repression, such as salicylate, all caused overexpression of the EmrCAB efflux pump, indicating that EmrR confers resistance to nalidixic acid and controls violacein production by acting as a repressor of the emrCAB operon. Thus, at high levels, EmrCAB could export nalidixic acid, and AHL molecules involved in activating violacein biosynthesis. Finally, transcriptome analysis and validation by biochemical assays demonstrated that EmrR repressed other putative transporters besides the emrCAB operon, by directly binding to the promoter regions of these genes.
Overexpression of efflux pumps as consequence of mutation in transcription factors is associated with antibiotic resistance in many bacteria (Fernandez and Hancock, 2012; Blair et al., 2015). Although the emergence of antibiotic-resistant strains due to point mutations in genes encoding repressors of the MarR family has been reported (for instance MexR and MepR) (Higgins et al., 2003; Birukou et al., 2013), this is the first description of this mechanism occurring in EmrR, at least under laboratory selection conditions. Indeed, most of the spontaneous nalidixic acid-resistant mutants presented point mutation in the QRDR region of GyrA, a mechanism well-documented in the literature (Redgrave et al., 2014). Our results also suggest that the arginine 92 is critical for the DNA binding activity of EmrR, as emrRR92H presented a phenotype similar to ΔemrR. Considering that we sequenced only emrR and gyrA genes, we can not exclude the occurrence of mutation in other topoisomerase genes (parC, gyrB) as contributing for quinolone resistance in C. violaceum.
Our data indicated that the emrR mutant strain showed increased resistance only to nalidixic acid, among the 24 antibiotics tested. This limited effect, also found for emrR mutants of E. coli and S. maltophilia, has been attributed to the fact that the EmrR-regulated efflux pump is specific for hydrophobic compounds (Lomovskaya and Lewis, 1992; Lomovskaya et al., 1995; Huang et al., 2013). Interestingly, our findings indicated that EmrCAB seems to have a role in exporting long-chain AHL molecules, which are synthesized by CviI and are required to activate violacein biosynthesis in C. violaceum (Morohoshi et al., 2008; Stauff and Bassler, 2011). In fact, RND-type efflux pumps have been associated with the secretion of quorum sensing molecules in other bacteria (Yang et al., 2006; Chan et al., 2007; Fernandez and Hancock, 2012). In C. violaceum, mutation of the emrCAB operon in the wild-type background had no effect on antibiotic susceptibility (including nalidixic acid), suggesting that other efflux pumps could be more active in absence of emrCAB (perhaps other putative transporters found as members of the EmrR regulon), and that the EmrCAB efflux pump should be relevant only in conditions that increase its expression. In fact, in S. maltophilia, mutation of emrCABsm only contributed to antibiotic susceptibility when combined with mutation in tolC, a condition that inactivated several TolC-dependent efflux pumps (Huang et al., 2013). Thus, high expression of the EmrCAB efflux pump (as in emrR mutants), but not its absence, allows a clear identification of its role in antibiotic resistance.
Similarly to what we observed in C. violaceum, in both E. coli and S. maltophilia EmrR represses the operon emrR(C)AB (Xiong et al., 2000; Huang et al., 2013). However, the natural ligand of EmrR remains to be determined, or even whether the same ligand works in emrR of all species. Indeed, derepression of emrCAB by salicylate occurs in both C. violaceum and E. coli (Lomovskaya et al., 1995), but was not observed in S. maltophilia (Huang et al., 2013).
The scope of action of EmrR seems broader than just regulating antibiotic resistance, because our global analysis of its regulon in C. violaceum revealed that it repressed genes involved in processes distinct from transport (the emrCAB operon and other putative transporters). One of these processes can be response to oxidative stress. Indeed, we verify that an antioxidant enzyme involved in C. violaceum resistance to peroxide (Previato-Mello et al., 2017) was regulated by EmrR. Accordingly, in both E. coli and C. violaceum EmrR was found to be induced under oxidant conditions (Sakamoto et al., 2015; Lima et al., 2016). Thus, it will be interesting to investigate whether EmrR can act as a redox sensing regulator, as is the case for other MarR family transcription factors related to antibiotic resistance (MgrA, MexR, OspR) (Chen et al., 2011).
The mechanism of point mutation for emergence of intrinsic resistance appears be more relevant for C. violaceum than those involving transfer of mobile genetic elements, considering that C. violaceum does not seem to harbor plasmids (Vasconcelos et al., 2003) and that several clinical isolates of this bacterium were found to be resistant to antibiotics (Aldridge et al., 1988; Yang and Li, 2011; Hagiya et al., 2014; Madi et al., 2015). In fact, two recent reports described the emergence of antibiotic resistance in C. violaceum by point mutations in transcription factors from the TetR family that regulate RND-type efflux pumps (Banerjee et al., 2017; Evans et al., 2018). Our data demonstrating that mutation in emrR is a potential mechanism that can favor the emergence of resistant strains improves the understanding on the regulatory mechanisms of antibiotic resistance in C. violaceum, contributing to formulating better treatment strategies against this pathogen.
Author Contributions
KB, MP-M, BB, and JB performed the experimental work. KB prepared the figures and tables. JSN contributed in study conception and data interpretation. JSN, KB, and BB wrote the manuscript. All authors participated in data interpretation and contributed to manuscript revision.
Funding
This research was supported by grants from the São Paulo Research Foundation (FAPESP; grants 2012/20435-9 and 2018/01388-6) and Fundação de Apoio ao Ensino, Pesquisa e Assistência do Hospital das Clínicas da FMRP-USP (FAEPA). During the course of this work, KB (grant 2015/12605-0) and MP-M (grant 2013/25745-9) were supported by FAPESP fellowships, while BB and JB were supported by fellowships from the Coordenação de Aperfeiçoamento de Pessoal de Nível Superior (CAPES).
Conflict of Interest Statement
The authors declare that the research was conducted in the absence of any commercial or financial relationships that could be construed as a potential conflict of interest.
Acknowledgments
We thank Geraldo A. Passos and his lab member Amanda F. Assis for assistance with the microarray scanning and data extraction.
Supplementary Material
The Supplementary Material for this article can be found online at: https://www.frontiersin.org/articles/10.3389/fmicb.2018.02756/full#supplementary-material
Footnote
References
Aldridge, K. E., Valainis, G. T., and Sanders, C. V. (1988). Comparison of the in vitro activity of ciprofloxacin and 24 other antimicrobial agents against clinical strains of Chromobacterium violaceum. Diagn. Microbiol. Infect. Dis. 10, 31–39. doi: 10.1016/0732-8893(88)90124-1
Azeredo, J., Azevedo, N. F., Briandet, R., Cerca, N., Coenye, T., Costa, A. R., et al. (2017). Critical review on biofilm methods. Crit. Rev. Microbiol. 43, 313–351. doi: 10.1080/1040841X.2016.1208146
Banerjee, D., Parmar, D., Bhattacharya, N., Ghanate, A. D., Panchagnula, V., and Raghunathan, A. (2017). A scalable metabolite supplementation strategy against antibiotic resistant pathogen Chromobacterium violaceum induced by NAD(+)/NADH(+) imbalance. BMC Syst. Biol. 11:51. doi: 10.1186/s12918-017-0427-z
Batista, J. H., and da Silva Neto, J. F. (2017). Chromobacterium violaceum pathogenicity: updates and insights from genome sequencing of novel Chromobacterium species. Front. Microbiol. 8:2213. doi: 10.3389/fmicb.2017.02213
Birukou, I., Tonthat, N. K., Seo, S. M., Schindler, B. D., Kaatz, G. W., and Brennan, R. G. (2013). The molecular mechanisms of allosteric mutations impairing MepR repressor function in multidrug-resistant strains of Staphylococcus aureus. mBio 4:e00528-13. doi: 10.1128/mBio.00528-13
Blair, J. M., Webber, M. A., Baylay, A. J., Ogbolu, D. O., and Piddock, L. J. (2015). Molecular mechanisms of antibiotic resistance. Nat. Rev. Microbiol. 13, 42–51. doi: 10.1038/nrmicro3380
Chan, Y. Y., Bian, H. S., Tan, T. M. C., Mattmann, M. E., Geske, G. D., Igarashi, J., et al. (2007). Control of quorum sensing by a Burkholderia pseudomallei multidrug efflux pump. J. Bacteriol. 189, 4320–4324. doi: 10.1128/JB.00003-07
Chen, H., Hu, J., Chen, P. R., Lan, L., Li, Z., Hicks, L. M., et al. (2008). The Pseudomonas aeruginosa multidrug efflux regulator MexR uses an oxidation-sensing mechanism. Proc. Natl. Acad. Sci. U.S.A. 105, 13586–13591. doi: 10.1073/pnas.0803391105
Chen, P. R., Brugarolas, P., and He, C. (2011). Redox signaling in human pathogens. Antioxid. Redox Signal. 14, 1107–1118. doi: 10.1089/ars.2010.3374
Clinical and Laboratory Standards Institute [CLSI]. (2013). Performance Standards for Antimicrobial Susceptibility Testing. Wayne, PA: Clinical and Laboratory Standards Institute.
Cox, G., and Wright, G. D. (2013). Intrinsic antibiotic resistance: mechanisms, origins, challenges and solutions. Int. J. Med. Microbiol. 303, 287–292. doi: 10.1016/j.ijmm.2013.02.009
da Silva Neto, J. F., Negretto, C. C., and Netto, L. E. (2012). Analysis of the organic hydroperoxide response of Chromobacterium violaceum reveals that OhrR is a Cys-based redox sensor regulated by thioredoxin. PLoS One 7:e0047090. doi: 10.1371/journal.pone.0047090
Davies, J., and Davies, D. (2010). Origins and evolution of antibiotic resistance. Microbiol. Mol. Biol. Rev. 74, 417–433. doi: 10.1128/MMBR.00016-10
Deochand, D. K., and Grove, A. (2017). MarR family transcription factors: dynamic variations on a common scaffold. Crit. Rev. Biochem. Mol. Biol. 52, 595–613. doi: 10.1080/10409238.2017.1344612
Durán, N., Justo, G. Z., Durán, M., Brocchi, M., Cordi, L., Tasic, L., et al. (2016). Advances in Chromobacterium violaceum and properties of violacein-Its main secondary metabolite: a review. Biotechnol. Adv. 34, 1030–1045. doi: 10.1016/j.biotechadv.2016.06.003
Evans, K. C., Benomar, S., Camuy-Velez, L. A., Nasseri, E. B., Wang, X., Neuenswander, B., et al. (2018). Quorum-sensing control of antibiotic resistance stabilizes cooperation in Chromobacterium violaceum. ISME J. 12, 1263–1272. doi: 10.1038/s41396-018-0047-7
Fernandez, L., and Hancock, R. E. (2012). Adaptive and mutational resistance: role of porins and efflux pumps in drug resistance. Clin. Microbiol. Rev. 25, 661–681. doi: 10.1128/CMR.00043-12
George, A. M., and Levy, S. B. (1983). Amplifiable resistance to tetracycline, chloramphenicol, and other antibiotics in Escherichia coli: involvement of a non-plasmid-determined efflux of tetracycline. J. Bacteriol. 155, 531–540.
Giles, J. A., Falconio, J., Yuenger, J. D., Zenilman, J. M., Dan, M., and Bash, M. C. (2004). Quinolone resistance-determining region mutations and por type of Neisseria gonorrhoeae isolates: resistance surveillance and typing by molecular methodologies. J. Infect. Dis. 189, 2085–2093. doi: 10.1086/386312
Gudeta, D. D., Bortolaia, V., Jayol, A., Poirel, L., Nordmann, P., and Guardabassi, L. (2016). Chromobacterium spp. harbour Ambler class A beta-lactamases showing high identity with KPC. J. Antimicrob. Chemother. 71, 1493–1496. doi: 10.1093/jac/dkw020
Hagiya, H., Murase, T., Suzuki, M., Shibayama, K., Kokumai, Y., Watanabe, N., et al. (2014). Chromobacterium violaceum nosocomial pneumonia in two Japanese patients at an intensive care unit. J. Infect. Chemother. 20, 139–142. doi: 10.1016/j.jiac.2013.10.001
Hanahan, D. (1983). Studies on transformation of Escherichia coli with plasmids. J. Mol. Biol. 166, 557–580. doi: 10.1016/S0022-2836(83)80284-8
Higgins, P. G., Fluit, A. C., Milatovic, D., Verhoef, J., and Schmitz, F. J. (2003). Mutations in GyrA. ParC, MexR and NfxB in clinical isolates of Pseudomonas aeruginosa. Int. J. Antimicrob. Agents 21, 409–413. doi: 10.1016/S0924-8579(03)00009-8
Huang, Y. W., Hu, R. M., Chu, F. Y., Lin, H. R., and Yang, T. C. (2013). Characterization of a major facilitator superfamily (MFS) tripartite efflux pump EmrCABsm from Stenotrophomonas maltophilia. J. Antimicrob. Chemother. 68, 2498–2505. doi: 10.1093/jac/dkt250
Lima, D. C., Duarte, F. T., Medeiros, V. K., Carvalho, P. C., Nogueira, F. C., Araujo, G. D., et al. (2016). GeLC-MS-based proteomics of Chromobacterium violaceum: comparison of proteome changes elicited by hydrogen peroxide. Sci. Rep. 6:28174. doi: 10.1038/srep28174
Lomovskaya, O., and Lewis, K. (1992). Emr, an Escherichia coli locus for multidrug resistance. Proc. Natl. Acad. Sci. U.S.A. 89, 8938–8942. doi: 10.1073/pnas.89.19.8938
Lomovskaya, O., Lewis, K., and Matin, A. (1995). EmrR is a negative regulator of the Escherichia coli multidrug resistance pump EmrAB. J. Bacteriol. 177, 2328–2334. doi: 10.1128/jb.177.9.2328-2334.1995
Madi, D. R., Vidyalakshmi, K., Ramapuram, J., and Shetty, A. K. (2015). Successful treatment of Chromobacterium violaceum sepsis in a south Indian adult. Am. J. Trop. Med. Hyg. 93, 1066–1067. doi: 10.4269/ajtmh.15-0226
Miki, T., Iguchi, M., Akiba, K., Hosono, M., Sobue, T., Danbara, H., et al. (2010). Chromobacterium pathogenicity island 1 type III secretion system is a major virulence determinant for Chromobacterium violaceum-induced cell death in hepatocytes. Mol. Microbiol. 77, 855–872. doi: 10.1111/j.1365-2958.2010.07248.x
Morohoshi, T., Kato, M., Fukamachi, K., Kato, N., and Ikeda, T. (2008). N-acylhomoserine lactone regulates violacein production in Chromobacterium violaceum type strain ATCC 12472. FEMS Microbiol. Lett. 279, 124–130. doi: 10.1111/j.1574-6968.2007.01016.x
Perera, I. C., and Grove, A. (2010). Molecular mechanisms of ligand-mediated attenuation of DNA binding by MarR family transcriptional regulators. J. Mol. Cell. Biol. 2, 243–254. doi: 10.1093/jmcb/mjq021
Previato-Mello, M., Meireles, D. A., Netto, L. E. S., da Silva, and Neto, J. F. (2017). Global transcriptional response to organic hydroperoxide and the role of OhrR in the control of virulence traits in Chromobacterium violaceum. Infect. Immun. 85:e00017-17. doi: 10.1128/IAI.00017-17
Redgrave, L. S., Sutton, S. B., Webber, M. A., and Piddock, L. J. (2014). Fluoroquinolone resistance: mechanisms, impact on bacteria, and role in evolutionary success. Trends Microbiol. 22, 438–445. doi: 10.1016/j.tim.2014.04.007
Roberts, R. C., Toochinda, C., Avedissian, M., Baldini, R. L., Gomes, S. L., and Shapiro, L. (1996). Identification of a Caulobacter crescentus operon encoding hrcA, involved in negatively regulating heat-inducible transcription, and the chaperone gene grpE. J. Bacteriol. 178, 1829–1841. doi: 10.1128/jb.178.7.1829-1841.1996
Rossolini, G. M., Arena, F., Pecile, P., and Pollini, S. (2014). Update on the antibiotic resistance crisis. Curr. Opin. Pharmacol. 18, 56–60. doi: 10.1016/j.coph.2014.09.006
Sakamoto, A., Terui, Y., Yoshida, T., Yamamoto, T., Suzuki, H., Yamamoto, K., et al. (2015). Three members of polyamine modulon under oxidative stress conditions: two transcription factors (SoxR and EmrR) and a glutathione synthetic enzyme (GshA). PLoS One 10:e0124883. doi: 10.1371/journal.pone.0124883
Seoane, A. S., and Levy, S. B. (1995). Characterization of MarR, the repressor of the multiple antibiotic resistance (mar) operon in Escherichia coli. J. Bacteriol. 177, 3414–3419. doi: 10.1128/jb.177.12.3414-3419.1995
Simon, R., Priefer, U., and Pühler, A. (1983). A broad host range mobilization system for in vivo genetic engineering: transposon mutagenesis in Gram-negative bacteria. Nat. Biotechnol. 1, 784–791. doi: 10.1038/nbt1183-784
Stauff, D. L., and Bassler, B. L. (2011). Quorum sensing in Chromobacterium violaceum: DNA recognition and gene regulation by the CviR receptor. J. Bacteriol. 193, 3871–3878. doi: 10.1128/JB.05125-11
Tanabe, M., Szakonyi, G., Brown, K. A., Henderson, P. J., Nield, J., and Byrne, B. (2009). The multidrug resistance efflux complex, EmrAB from Escherichia coli forms a dimer in vitro. Biochem. Biophys. Res. Commun. 380, 338–342. doi: 10.1016/j.bbrc.2009.01.081
Vasconcelos, A. T. R., Almeida, D. F., Hungria, M., Guimarães, C. T., Antônio, R. V., Almeida, F. C., et al. (2003). The complete genome sequence of Chromobacterium violaceum reveals remarkable and exploitable bacterial adaptability. Proc. Natl. Acad. Sci. U.S.A. 100, 11660–11665. doi: 10.1073/pnas
Xiong, A., Gottman, A., Park, C., Baetens, M., Pandza, S., and Matin, A. (2000). The EmrR protein represses the Escherichia coli emrRAB multidrug resistance operon by directly binding to its promoter region. Antimicrob. Agents Chemother. 44, 2905–2907. doi: 10.1128/AAC.44.10.2905-2907.2000
Yang, C. H., and Li, Y. H. (2011). Chromobacterium violaceum infection: a clinical review of an important but neglected infection. J. Chin. Med. Assoc. 74, 435–441. doi: 10.1016/j.jcma.2011.08.013
Yang, S., Lopez, C. R., and Zechiedrich, E. L. (2006). Quorum sensing and multidrug transporters in Escherichia coli. Proc. Natl. Acad. Sci. U.S.A. 103, 2386–2391. doi: 10.1073/pnas.0502890102
Keywords: Chromobacterium violaceum, MarR transcription factors, antibiotic resistance, quinolone resistance, drug efflux pumps, quorum sensing, violacein
Citation: Barroso KCM, Previato-Mello M, Batista BB, Batista JH and da Silva Neto JF (2018) EmrR-Dependent Upregulation of the Efflux Pump EmrCAB Contributes to Antibiotic Resistance in Chromobacterium violaceum. Front. Microbiol. 9:2756. doi: 10.3389/fmicb.2018.02756
Received: 03 August 2018; Accepted: 29 October 2018;
Published: 15 November 2018.
Edited by:
Kunihiko Nishino, Osaka University, JapanReviewed by:
Maria Blanca Sanchez, Instituto IMDEA Agua, SpainMichael Göttfert, Technische Universität Dresden, Germany
Copyright © 2018 Barroso, Previato-Mello, Batista, Batista and da Silva Neto. This is an open-access article distributed under the terms of the Creative Commons Attribution License (CC BY). The use, distribution or reproduction in other forums is permitted, provided the original author(s) and the copyright owner(s) are credited and that the original publication in this journal is cited, in accordance with accepted academic practice. No use, distribution or reproduction is permitted which does not comply with these terms.
*Correspondence: José F. da Silva Neto, amZzbmV0b0B1c3AuYnI=