- 1Laboratoire de Microbiologie Signaux et Microenvironnement (LMSM EA 4312) – Normandie Université – LMSM, Évreux, France
- 2Structure Fédérative de Recherche Normandie Végétal 4277 (NORVEGE), Mont-Saint-Aignan, France
- 3Seeds Innovation Protection Research and Environment, Achicourt, France
- 4Seeds Innovation Protection Research and Environment, Bretteville du Grand-Caux, France
- 5French Federation of Seed Potato Growers (FN3PT/RD3PT), Paris, France
The biocontrol agent Rhodococcus erythropolis disrupts virulence of plant and human Gram-negative pathogens by catabolizing their N-acyl-homoserine lactones. This quorum-quenching activity requires the expression of the qsd (quorum-sensing signal degradation) operon, which encodes the lactonase QsdA and the fatty acyl-CoA ligase QsdC, involved in the catabolism of lactone ring and acyl chain moieties of signaling molecules, respectively. Here, we demonstrate the regulation of qsd operon expression by a TetR-like family repressor, QsdR. This repression was lifted by adding the pathogen quorum signal or by deleting the qsdR gene, resulting in enhanced lactone degrading activity. Using interactomic approaches and transcriptional fusion strategy, the qsd operon derepression was elucidated: it is operated by the binding of the common part of signaling molecules, the homoserine lactone ring, to the effector-receiving domain of QsdR, preventing a physical binding of QsdR to the qsd promoter region. To our knowledge, this is the first evidence revealing quorum signals as inducers of the suitable quorum-quenching pathway, confirming this TetR-like protein as a lactone sensor. This regulatory mechanism designates the qsd operon as encoding a global disrupting pathway for degrading a wide range of signal substrates, allowing a broad spectrum anti-virulence activity mediated by the rhodococcal biocontrol agent. Understanding the regulation mechanisms of qsd operon expression led also to the development of biosensors useful to monitor in situ the presence of exogenous signals and quorum-quenching activity.
Introduction
Bacteria use cell-to-cell communication systems based on both synthesis and perception of signaling molecules to synchronize their social behavior. These quorum-sensing (QS) systems control diverse functions, which require the concerted actions of numerous cells in order to be productive, such as antibiotic synthesis, motility, symbiosis, biofilm maturation, sporulation, and virulence (Papenfort and Bassler, 2016). Among them, the most-studied system relies on the production of molecules belonging to the family of N-acyl-L-homoserine lactones (AHLs). These play a key role in the pathogenicity of a dozen of clinically relevant pathogens and many agronomically important pathogens (von Bodman et al., 2003; Rutherford and Bassler, 2012). For example, Pseudomonas aeruginosa, one of the leading opportunistic pathogens, uses several QS networks to control the production and release of multiple virulence factors such as LasA and LasB elastases, exotoxin A and alkaline protease involved in acute pulmonary infection (Jimenez et al., 2012). A mutant deficient in the synthesis of AHL signal molecules was nearly avirulent in a murine model of acute pulmonary infection (Pearson et al., 2000). Among the top 10 phytopathogens, Pectobacterium spp. employs QS that can also harbor a high degree of complexity to integrate several AHL signaling networks (Barnard and Salmond, 2007; Mansfield et al., 2012; Valente et al., 2017). Pectobacterial QS ensures the synchronized production of massive amounts of lytic enzymes involved in plant tissues degradation (Barnard and Salmond, 2007; Põllumaa et al., 2012). Many AHL-controlled functions have already been reported in the Pectobacterium atrosepticum species. They comprise up to a quarter of its genome, in particular a huge arsenal of plant cell-wall degrading enzymes as well as their secretion systems (Liu et al., 2008). Therefore, a single mutation of the AHL synthase gene, AHL receptor/transcription factor, or the degradation of signal molecules before their release in the microenvironment is sufficient to remove all symptoms on host plants (Smadja et al., 2004b; Latour et al., 2007; Põllumaa et al., 2012).
Since QS is essential for disease progression in many pathogenic bacteria, disrupting the microbial QS system by a quorum-quenching (QQ) mechanism appears as a promising anti-virulence strategy for human and plant health (Dong et al., 2001, 2007; Njoroge and Sperandio, 2009; LaSarre and Federle, 2013; Helman and Chernin, 2015; Dessaux et al., 2016). Indeed, QQ aims at reducing virulence expression rather than limiting cell growth or eradicating pathogens. It may involve the use of bacteria as well as their products to overcome the risk of microbial invasion, a role and applications exhaustively described in the recent reviews of Grandclément et al. (2016) and Zhang and Li (2016). Furthermore, this QQ strategy is considered more sustainable of the microbial balance (Bais, 2012). If it involves hydrolysis of signals, it also limits the development of resistance because of a less selective pressure on bacterial populations than antibiotics or QS inhibitor molecules (Defoirdt et al., 2010; Mellbye and Schuster, 2011; Garcia-Contreras et al., 2013; Garcia-Contreras, 2016; Guendouze, 2017).
The first reports of QQ activities were carried out in soilborne bacteria belonging to Bacillus or Variovorax genera (Dong et al., 2000; Leadbetter and Greenberg, 2000), then joined by other quencher models associated with plants such as Agrobacterium tumefaciens or Rhodococcus erythropolis (Zhang et al., 1993; Uroz et al., 2003). In the rhodococcal model, enzymes that catabolize AHLs are mainly lactonases that open the homoserine lactone ring (Uroz et al., 2003; Oh et al., 2013) or amidases (also referred as acylases or amidohydrolases) that cleave AHL amide bond and release the homoserine lactone (HSL) and a fatty acid (Lin et al., 2003; Uroz et al., 2005). We screened and isolated the R. erythropolis strain R138, a biocontrol bacterium able to degrade effectively diverse AHL signals (Afriat et al., 2006; Cirou et al., 2007; Oh et al., 2013) and to suppress the disease in hydroponic and field culture conditions (Cirou et al., 2011, 2012). The molecular mechanism of this protection was demonstrated in vivo and mainly involves a new pathway (Barbey et al., 2012, 2013). This pathway requires the expression of the quorum-sensing signal degradation (qsd) operon encoding the phosphotriesterase-like lactonase QsdA (EC 3.1.1.81) for the lactone bond hydrolysis, and the fatty acyl-CoA ligase QsdC (syn. FadD, EC 6.2.1.3) for activation of acyl chains before their oxidation or recycling (Uroz et al., 2008; Latour et al., 2013). The latter was named QsdC because QsdB was previously proposed for a QS-signal degrading amidase (El Sahili et al., 2015). Besides, an AHL amidolytic activity was initially identified by HPLC analysis and chemical trapping of HSL in a R. erythropolis W2 crude cell extract (Uroz et al., 2005). Then, proteomic analysis enabled to identify an amidase (NCBI reference WP_019744590.1) produced by R. erythropolis R138 during growth only in the presence of AHL as sole carbon source (Latour et al., 2013). Interestingly, the rhodococcal biocontrol activity is induced not by the invasion step or the massive presence of the pathogen, but by its QS-based communication; indeed, no qsd operon transcription was observed in the presence of pathogens defective for AHL release (Barbey et al., 2013). This AHL induction phenomenon suggests the existence of a regulatory system for the qsd operon transcription. Sequence analysis of the upstream region of the qsd operon revealed a putative TetR family transcriptional regulator gene, that we named qsdR (Latour et al., 2013), and which belongs to a transcriptional repressor family (Ramos et al., 2005; Cuthbertson and Nodwell, 2013). Then, a substantial biochemical analysis enabled to define the crystal structure of QsdR protein and a genome engineering approach exhibited that the change of only one amino-acid in QsdR leads to enhancement of the QS-signal degradation by R. erythropolis (El Sahili et al., 2015). We describe here the role of QsdR and AHL molecules in the regulation of the qsd operon transcription and propose a model in which QQ activity is triggered by various AHL signals.
Materials and Methods
Bacterial Strains, Growth, and Culture Conditions
The characteristics of bacterial strains and plasmids are presented in Supplementary Table S1. R. erythropolis strains were cultivated in LBP (Barbey et al., 2013) for conjugative transfer of DNA and in 7H9 minimal medium (Difco) supplemented with hexanoate at 6 mM (Sigma-Aldrich) as carbon source for induction assays. Induction experiments were performed by adding at the mid-exponential phase the N-3-oxo-octanoyl-L-homoserine lactone (3 oxo-C8-HSL) (Sigma-Aldrich) molecules at concentrations ranging from 10 nM to 3 mM. Luria-Bertani medium (AES Chemunex, Bruz, France) was used for the cultivation of E. coli strains. Pathogens, P. atrosepticum 6276 and P. aeruginosa PA14, were grown in PGA minimal medium supplemented with 0.4% (w/v) polygalacturonic acid (Sigma-Aldrich, St. Louis, United States) as described elsewhere (Barbey et al., 2013), and King B medium, respectively. When necessary, growth media were supplemented with kanamycin at a concentration of 30 μg/ml for E. coli and 200 μg/ml for R. erythropolis, with apramycin at 50 μg/ml for E. coli and 80 μg/ml for R. erythropolis, with ampicillin at 100 μg/ml for E. coli and solidified with agar (15 g/l). All bacteria were grown on a rotary shaker (180 rpm) at 25°C for R. erythropolis strains and P. atrosepticum 6276 and 37°C for E. coli and P. aeruginosa PA14.
Construction and Complementation of ΔqsdR Mutant in R. erythropolis Strain R138
A markerless qsdR deletion mutant (ΔqsdR) was constructed as previously described (Barbey et al., 2013) using the oligonucleotide pairs qsdR_up_fw/qsdR_up_rv and qsdR_do_fw/qsdR_do_rv (Supplementary Table S2) for the amplification of the qsdR upstream and downstream regions (881 and 878 bp), respectively. The in-frame qsdR deletion mutant containing a truncated version of the qsdR gene (69 bp in length instead of 558 bp for the wild-type gene) was verified by PCR analysis and DNA sequencing.
To generate the complemented strain, the qsdR gene with its promoter was amplified with the Extensor Hi Fidelity polymerase using primers pSET152_qsdR_F and pSET152_qsdR_R (Supplementary Table S2) and cloned as an 855-bp EcoRI-XbaI fragment into the integrative vector pSET152 (Swain et al., 2012). After sequencing of the insert, this plasmid designated pSET152-qsdR was introduced in R. erythropolis R138 cells by biparental mating as previously described (Barbey et al., 2013). The complemented strain was called ΔqsdR-qsdR.
Biocontrol Assays on Potato Tubers
Solanum tuberosum cv. Allians tubers were prepared for inoculation as previously described (Barbey et al., 2013). They were inoculated by injection into the intramedulla (to a depth of 1 cm) with 10 μl of cell suspension containing the following bacterial combinations: [107 CFU of P. atrosepticum CFBP6276 with 108 CFU of R. erythropolis R138/R138 ΔqsdA/E. coli DH5α(pUC19)/DH5α(pUC19-qsdA)] or [108 CFU of P. aeruginosa PA14 with 108 CFU of R. erythropolis R138/R138 ΔqsdA/E. coli DH5α(pUC19)/DH5α(pUC19-qsdA)]. For controls, the suspensions of P. atrosepticum CFBP6276, P. aeruginosa PA14 and/or R. erythropolis R138 were replaced with 0.9% NaCl. The P. atrosepticum and P. aeruginosa inoculated tubers were incubated in a Minitron incubator (Infors, Massy, France) with a relative humidity of 80% at 25 and 30°C, respectively. At 7 days after inoculation, 30 tubers for each condition were sectioned across the middle, photographed, analyzed by measuring maceration diameter and cut for bacterial population analysis as previously described (Barbey et al., 2013). These experiments were conducted three times. The Mann and Whitney test was used to assess differences in maceration symptoms between groups (p-value < 0.01).
Colorimetric Quantification of Quorum Signals
The 3-oxo-C8-HSL molecules used for induction assays were quantified by a colorimetric method typically applied to the analysis of ester molecules (Yang et al., 2006). This method is based on the formation of hydroxamic acids from esters by reaction with hydroxylamine in alkaline solution. The hydroxamic acid forms a highly colored complex with ferric ion, which is spectrophotometrically measurable at 520 nm. Dosage was performed as described by Cirou et al. (2012) with the following modifications. Culture supernatants were collected every 2 h and centrifuged at 5,000 g for 5 min. Stability of 3-oxo-C8-HSL in 7H9 medium was checked by incubating a non-inoculated 7H9 medium under the same conditions as inoculated media. A volume of 200 μl of supernatant or the non-inoculated medium was successively mixed with 250 μl of 2 M hydroxylamine (Sigma-Aldrich)/3.5 M NaOH (Merck) (1:1, v/v) and 250 μl of 10% iron chloride (Sigma-Aldrich) prepared in 4 M HCl / 95% Ethanol (Merck) (1:1, v/v). The absorbance of the mixture was measured at 520 nm against a blank consisting of the same mixture in which culture supernatant was replaced with the non-inoculated 7H9 medium without 3-oxo-C8-HSL.
Quantitative RT-PCR
RNA was extracted by a modified version of the phenol-based extraction procedure described by Crépin et al. (2012a). Cells were first lysed by vortexing twice for 5 min with acid-washed glass beads in the lysis buffer [0.02 M sodium acetate, pH 5.5, 0.5% (w/v) SDS, 1 mM EDTA]. mRNAs were quantified by real-time PCR as described by Bouffartigues et al. (2015) using primers in the Supplementary Table S2. The relative quantification of the mRNAs was obtained by the comparative CT (2-ΔΔCT) method as described (Bouffartigues et al., 2015). This analysis was performed using the recombinase A (recA) gene as endogenous control, as previously described for R. erythropolis gene expression study (Kwasiborski et al., 2015).
In silico Studies and Molecular Docking
The R. erythropolis R138 genome sequence is available from the NCBI database with the accession no. NZ_CM002793.1. The qsd cluster is composed of qsdR (gene ID H351_RS26320), qsdA (gene ID H351_RS26315), and qsdC (gene ID H351_RS26310). The qsdR gene is located upstream of a FadR encoding gene (gene ID H351_RS05745). The clone manager software (professional edition version 9.00) was used to identify palindrome motifs. Regulator binding sites were predicted using the web-based software bprom in the Softberry package (Solovyev and Salamov, 2011). The crystallized structure of the R. erythropolis R138 QsdR (receptor) was obtained from the Protein Data Bank (PDB) with the accession number 4ZA6. The structure was downloaded in a.pdb format and was cleaned by removing co-crystallized ligands and water by using Discovery Studio 4.5, and finally save in.pdb format. The potential ligands were drawn by using Discovery Studio 4.5 and save in.pdb format. The docking was carried out with AutoDock Vina (Trott and Olson, 2010) by using AutoDock Tools 1.5.6 for preparing the receptor and each ligand tested (Morris et al., 2009). Potential ligand–protein interactions were investigated in silico by molecular docking. H-atoms of the receptor were added for correct ionization and tautomeric states of amino acid residues with AutoDock Tools 1.5.6 (Morris et al., 2009; Trott and Olson, 2010). Docking simulations were made using the Lamarckian genetic algorithm (LGA). Kollman united atom type charges and solvation parameters were added with AutoDock Tools 1.5.6 (Morris et al., 2009). Initial position, orientation and torsions of the ligands were set randomly. The energy scoring grid box was centered in the middle of the receptor and was set to 126, 126, and 126 Å (x, y, and z) centered at X = -22.709; Y = -9.312 and Z = 10.331 with 0.753 Å spacing. AutoDock Vina generated the docking results (ΔG) in a.pdbqt file in kcal/mol, subsequently transformed in kJ/mol.
Mapping of Transcription Start Sites
5′ Rapid Amplification of cDNA ends (RACE) was performed to define the transcription start site (TSS) of the operon qsd and of the qsdR gene using primers listed in the Supplementary Table S2 and the 5′ RACE System, Version 2.0 (Invitrogen) according to the manufacturer’s instructions. PCR products were cloned into pGEM-T Easy vector according to the manufacturer’s instructions (Promega) and sequenced to identify the 5′ end of the qsdA and qsdR mRNA (Beckman Coulter Genomics, Villepinte, France).
Electrophoretic Mobility Shift Assay (EMSA)
The qsdR ORF was amplified with Extensor Hi Fidelity polymerase using primers pET19-qsdR F and pET19-qsdR R (Supplementary Table S2) and cloned as a 570-bp EcoRI-BamHI fragment into the expression vector pET19. The E. coli BL21 (DE3) cells were transformed with the resulting pET19-qsdR vector and were grown at 20°C in LB medium containing ampicillin (100 mg/l). When absorbance at 580 nm reached 0.6, isopropyl-1-thio-β-D-galactoside (IPTG) was added at a final concentration of 0.15 mM and the cells were harvested 12 h later by centrifugation. QsdR recombinant protein was purified using Ni NTA agarose as described by the manufacturer (Qiagen). Complementation experiments with the ΔqsdR strain were performed to verify that the His tag does not interfere with the QsdR function. The qsdR qsdA intergenic DNA fragment used in EMSA was amplified using the qsdR-qsdA-EMSA F and qsdR-qsdA-EMSA R primers (Supplementary Table S2) with the genomic DNA of R. erythropolis R138 as template. EMSA was performed with 0.3 to 3 μg of purified His tagged QsdR, and 0.4 ng DNA fragment using LightShift® Chemiluminescent EMSA Kit as described by the manufacturer (Thermo Scientific). For negative control, qsdA-qsdA-EMSA F and qsdA-qsdA-EMSA R primers were used to amplify a biotin labeled DNA internal fragment of qsdA between the nucleotide positions 431 and 607 from the start codon. The effect of HSL or AHLs on the ability of QsdR to bind the qsdR qsdA intergenic DNA fragment were tested by adding a ligand/QsdR molecules ratio increasing from 0.1 to 100 into the 20 μl EMSA binding reaction. In control samples, ligand solution was replaced by ethyl acetate to check the lack of solvent effect on protein/DNA binding.
Mass Spectrometry Analysis
Protein spots to be identified were manually excised from Coomassie stained SDS-PAGE. Excised gel fractions were washed in 100 mM ammonium bicarbonate (Sigma-Aldrich)/acetonitrile (Merck) (1:1, v/v) until completely destained. After drying with acetonitrile, gel fragments were rehydrated in 20 μl of trypsin solution (Trypsin Gold, Mass Spectrometry grade, Promega) at 13 ng/μl in 10 mM ammonium bicarbonate solution containing 10% acetonitrile (v/v). Then, digestion was performed at 37°C for 16 h. Peptide extraction was carried out twice for 1 h with 20 μl 50% acetonitrile/5% formic acid (Sigma-Aldrich). In-gel trypsin digestion products were analyzed by a Matrix Assisted Laser Desorption Ionization Time-of-Flight mass spectrometer (MALDI-TOF/TOF LIFT, AutoFlex III, Bruker Daltonics) in positive/reflector mode controlled by FlexControl software Version 3.3, as previously described (Barbey et al., 2012).
Construction and Analysis of Transcriptional Fusion Strains by Confocal Laser Scanning Microscopy (CLSM)
The pEPR1 promoter probe vector (Knoppová et al., 2007) was modified to construct three derivative vectors. The pEPR1-mCherry vector was built by introducing the mCherry cassette to constitutively tag rhodococcal cells. The mCherry cassette was amplified with the primers mCherry_F and mCherry_R (Supplementary Table S2) from the pPSV35-mCherry plasmid DNA and cloned into pEPR1 after NheI digestion. The pEPR1-Pqsd::gfp-mCherry was made by amplifying the qsdA promoter region using primers Pqsd_F and Pqsd_R (Supplementary Table S2). The resulting PCR product was cloned into the pEPR1-mCherry vector after digestion by NsiI and BamHI. For the construction of the pEPR1-qsdR-Pqsd::gfp-mCherry, the DNA fragment containing the qsdR gene and the qsd promoter was introduced into the pEPR1-mCherry vector after PCR amplification using the primers qsdR-Pqsd_F and qsdR-Pqsd_R (Supplementary Table S2) and digestion by NsiI and BamHI. All PCR, ligation and restriction reactions were carried out with Phusion Hi Fidelity polymerase (Biolabs NEB), T4 DNA ligase (Biolabs NEB) and NEB endonucleases in accordance with the supplier’s recommendations, respectively. Each resulting vector was introduced into the R. erythropolis R138 cells by electroporation as previously described (Barbey et al., 2013). Bacterial strains transformed with plasmids harboring reporter genes were cultivated in the same medium and under the same conditions as those used for qsd expression assays by qRT-PCR. For induction experiments, different concentrations of HSL or AHLs (1 μM to 1 mM) were added at mid-exponential phase. Analysis of induced and non-induced cultures by confocal microscopy was performed by fixing bacteria with ethanol on glass slides. Slides were examined by inverted CLSM (LSM 710, Carl Zeiss MicroImaging, Le Pecq, France). To excite the GFP or mCherry in bacterial cells, a 488 or 594 nm laser with 509 or 610 nm emission filters was used, respectively. Confocal images were acquired with Zen 2009H software (Carl Zeiss MicroImaging) using the same gains and offset parameters for all images. Three bacterial smears from three independent culture conditions were analyzed for each condition.
Results
The qsd Operon Is the Source of the Anti-virulence Activity of R. erythropolis Against AHL-Producing Gram-Negative Pathogens
Rhodococcus erythropolis strain R138 was isolated from the S. tuberosum potato rhizosphere in which it degrades effectively AHLs (Cirou et al., 2011; Barbey et al., 2013). Involvement of qsd operon in the anti-virulence activity of R. erythropolis against the human pathogen P. aeruginosa PA14 and the potato pathogen P. atrosepticum CFBP6276 was studied using a plant infection model sensitive to both pathogens (Rahme et al., 1997; Smadja et al., 2004a). This potato tuber assay makes it easy to quantify the bacterial virulence by measuring the extent of cellular damage (i.e., maceration) (Crépin et al., 2012b). P. aeruginosa is well known to use two AHL-based QS systems regulating the production of many virulence factors: the las and rhl systems produce and respond to the N-3-oxo-dodecanoyl-L-homoserine lactone (3-oxo-C12-HSL) and N-butanoyl-L-homoserine lactone (C4-HSL), respectively (Jimenez et al., 2012; Mund et al., 2017). In contrast, P. atrosepticum produces and responds mainly to the N-(3-oxo-octanoyl)-L-homoserine lactone (3-oxo-C8-HSL) in order to coordinate its attack and overwhelm the host plant defenses (Latour et al., 2007; Crépin et al., 2012c). To investigate the role of the qsd operon in biocontrol, anti-virulence activity of R. erythropolis R138 was compared with a R. erythropolis mutant strain defective in the production of the lactonase QsdA. The key role of QsdA in protection was then validated by its heterologous expression in Escherichia coli, a bacterium unable to degrade AHLs (Figure 1). First, the qsdA gene was introduced into E. coli DH5α, and the heterologous expression of QsdA was verified by SDS PAGE analysis and MALDI-TOF sequencing. For tubers infected with P. aeruginosa, drastic differences in tissue maceration were observed between tubers co-inoculated with the quorum quencher strains (R. erythropolis R138 and E. coli pUC19-qsdA) and those co-inoculated with their derivative strains (R. erythropolis R138 ΔqsdA and E. coli pUC19) 7 days after inoculation. The same results were obtained for tubers infected with P. atrosepticum (Figure 1). Under all assay conditions, pathogen and antagonist populations grew in mixed populations, with P. aeruginosa PA14 and P. atrosepticum CFBP6276 populations reaching 108 and 109 CFU/g of fresh tuber after 7 days, respectively, confirming that R. erythropolis R138 did not express antibiotic activities against these bacteria as already observed (Barbey et al., 2013; Kwasiborski et al., 2015).
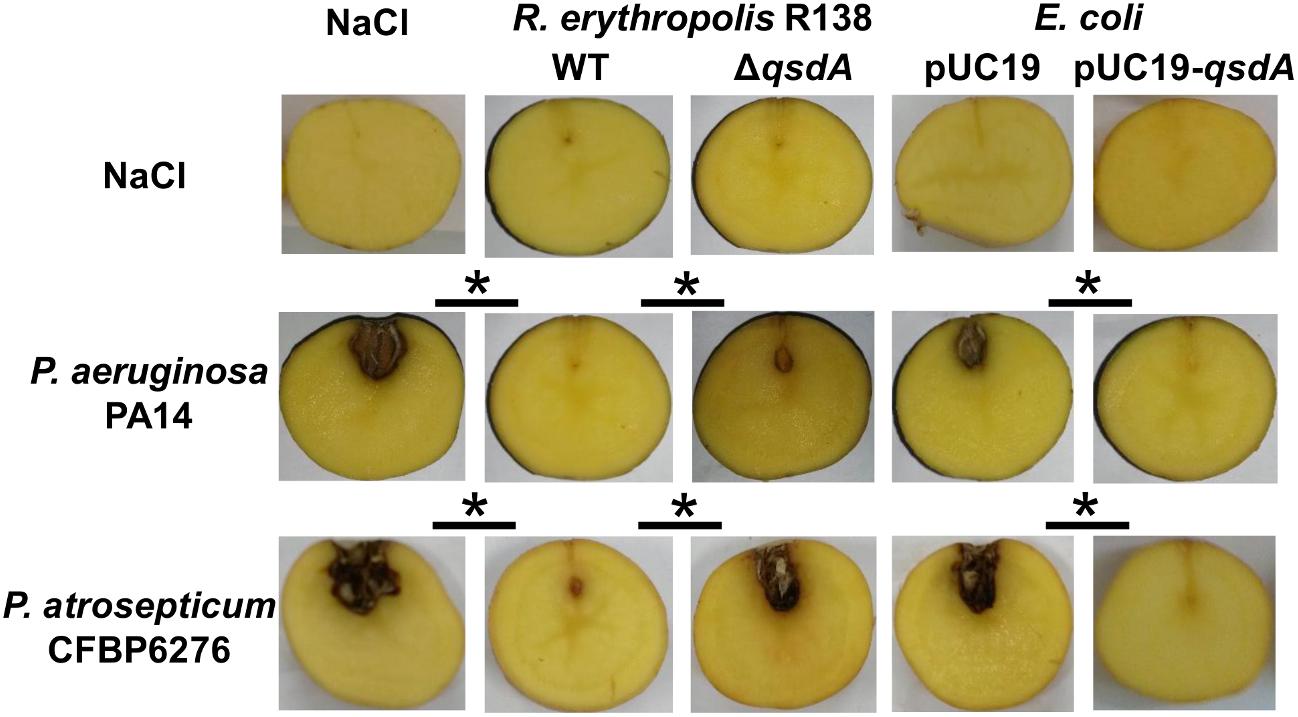
FIGURE 1. Anti-virulence activity of Rhodococcus erythropolis and QsdA lactonase expressing E. coli strains against AHL-producing Gram-negative pathogens. Quorum quenchers and their corresponding control strains (R. erythropolis ΔqsdA mutant strain defective in QsdA lactonase production and E. coli pUC19) were compared for biocontrol activity against a 3-oxo-C12-HSL and C4-HSL producer, the human pathogen P. aeruginosa PA14, as well as a 3-oxo-C8-HSL producer, the potato pathogen Pectobacterium atrosepticum CFBP6276. Seven days after inoculation in potato tubers, tissue necrosis or soft-rot due to P. aeruginosa and P. atrosepticum, respectively, were analyzed and compared between tuber lots (black bars). For the controls, one or both strains were replaced in the inoculum with a 0.9% NaCl solution. Significant differences (Mann and Whitney test; p-value < 0.01) in maceration symptoms between infected tubers inoculated with control and quorum quencher strains are indicated with an asterisk.
qsd Operon Transcription Is Induced by QS Signals
To investigate the induction mechanism of the rhodococcal QQ activity, R. erythropolis was grown in a minimal medium containing hexanoate as the sole carbon source. This aliphatic compound was chosen because it is not able to form a lactone ring and its assimilation does not induce the production of lactonase QsdA (Barbey et al., 2012). The 3-oxo-C8-HSL was used as a model inducer for its prevalence in numerous plant-associated bacteria (Cha et al., 1998; D’Angelo-Picard et al., 2005) including the P. atrosepticum species (Latour et al., 2007; Crépin et al., 2012c). The 3-oxo-C8-HSL was added to the R. erythropolis R138 culture at mid-exponential growth phase. Bacterial cells were then harvested every 2 h for the qsd operon expression analysis by qRT-PCR and 3-oxo-C8-HSL degradation kinetics. Expression levels were compared with expression levels obtained from the same culture conditions without the addition of the AHL molecules. Exposure of R. erythropolis to 3-oxo-C8-HSL induced a significant increase in qsdA and qsdC expression levels. For both genes, the transcription levels increased significantly from 5 h, reached a maximum between 7 and 9 h after induction and then decreased (Figure 2).
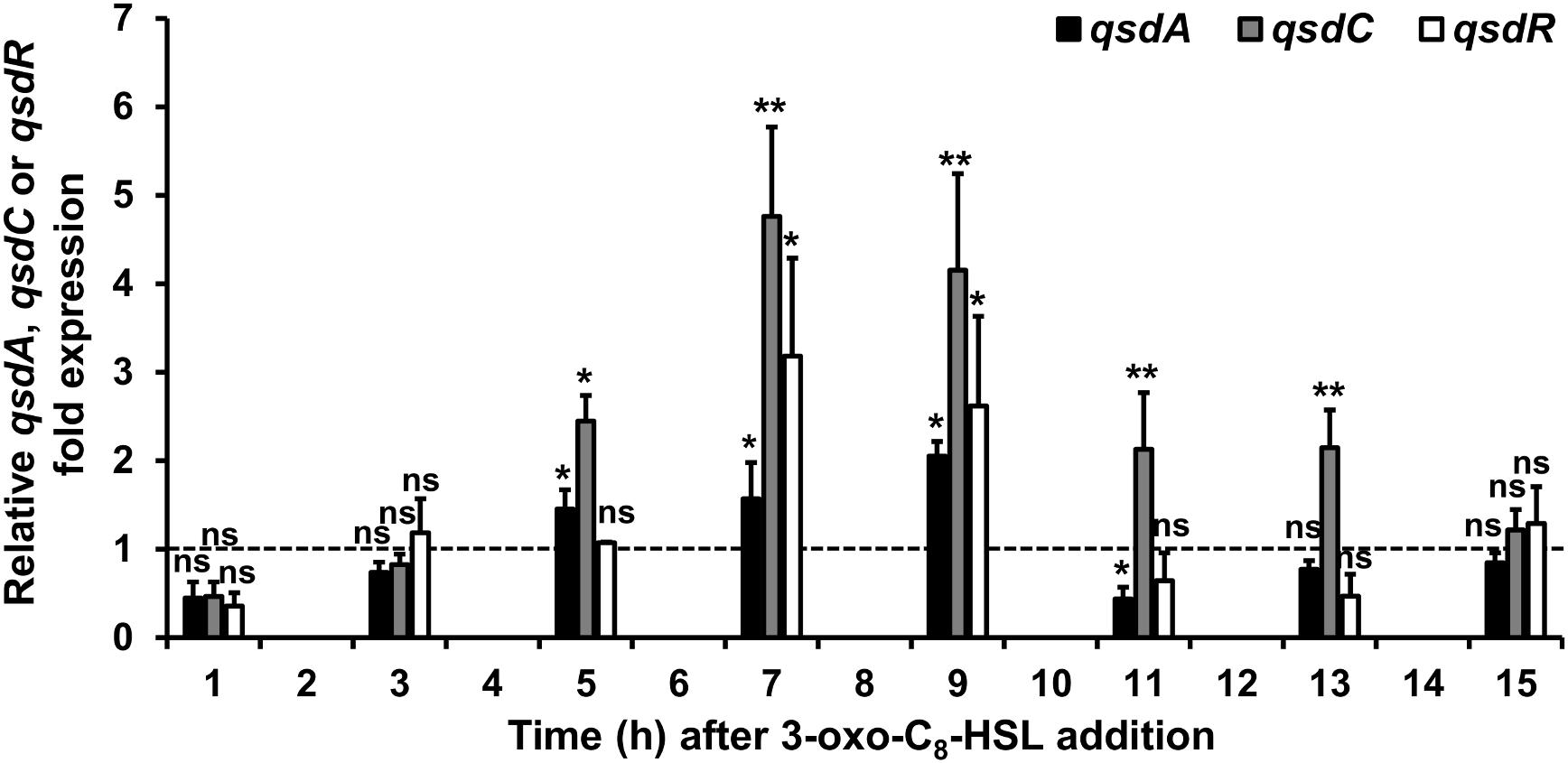
FIGURE 2. Induction of the qsd operon expression by 3-oxo-C8-HSL. qRT-PCR analysis of the qsdA, qsdC, and qsdR transcription was conducted in the R. erythropolis R138 wild-type strain grown in 7H9 medium in which 3-oxo-C8-HSL was added at mid-exponential phase. Expression levels of qsdA, qsdC, and qsdR are relative to those obtained in the same medium without the inducer. The dotted line indicates the same mRNA expression between the induced condition and without the inducers. Data shown are mean values obtained from three independent experiments. Statistical analysis was performed by the DataAssistTM software (v3.01) used for calculating relative quantitation of gene expression, based on the comparative CT (2-ΔΔCT) method. ∗p-value < 0.05; ∗∗p-value < 0.01; ns means no significant difference.
Identification of a qsdR Gene Upstream of the qsd Operon: Influence of This Gene on qsd Expression and QS Signal Degradation
The complete genome sequence of the biocontrol strain R. erythropolis R138 was recently achieved. It consists of a circular chromosome (about 6.7 Mbp) and two plasmids (Kwasiborski et al., 2015). Sequence analysis of the upstream region from the R138 chromosomal qsd operon revealed the presence of a gene encoding a putative TetR family transcriptional regulator, QsdR (Supplementary Figure S1). Due to its key position, this gene was already suspected to play a key role in the regulation of the qsd operon during initial investigations (Latour et al., 2013; El Sahili et al., 2015). qsdR is located less than 200 bp from the divergently oriented neighboring qsdA gene, as is the case for the most genes encoding TetR regulators and their target genes (Ahn et al., 2012; Cuthbertson and Nodwell, 2013). Interestingly, after 3-oxo-C8-HSL addition in cultures, the profile of the amounts of qsdR transcript showed a pattern of mRNA accumulation similar to that of the qsdA and qsdC genes (Figure 2). The 5′ end of the mRNA transcribed from the qsd operon or the qsdR gene was determined by 5′RACE. Individual TSSs were identified for both qsdA and qsdR RNAs. The qsdA and qsdR transcription began at a T located 43 and 104 bp upstream of their respective start codons, respectively. In the qsdR-qsdA intergenic region, two palindrome motifs with free energy ΔG of -22.3 kJ for IR1 and -49.1 kJ for IR2 were detected and might correspond to putative operator sequences for a potential QsdR binding. Interestingly, the qsd cluster bordered by qsdR and qsdC genes is preceded by a FadR encoding gene located 102 bp downstream of the stop codon of qsdR. Besides, a putative binding site for FadR transcriptional factor was predicted upstream of the -35 box of qsdR promoter (Supplementary Figure S1). This sequence shares 75% identity with the consensus sequence recognized by FadR of E. coli (Bacik et al., 2015).
To verify the hypothesis that the qsdR gene plays a role in regulating the qsd operon, a qsdR deletion mutant was constructed as described in the Section “Materials and Methods” and the qsdR, qsdA, and qsdC gene expression was analyzed using qRT-PCR and compared with that obtained in the non-induced wild-type strain (Figure 3). As expected, no qsdR expression was detected in the ΔqsdR deletion mutant. Furthermore, qsdA and qsdC expression levels were significantly higher than those observed in the wild-type strain, with around 80-fold change after 10 h of incubation and 150-fold change after 19 h of incubation (Figure 3). The qsdA and qsdC expression levels were restored to wild-type levels in the ΔqsdR mutant complemented with the qsdR gene on the integrative vector pSET152, corresponding to the ΔqsdR-qsdR strain.
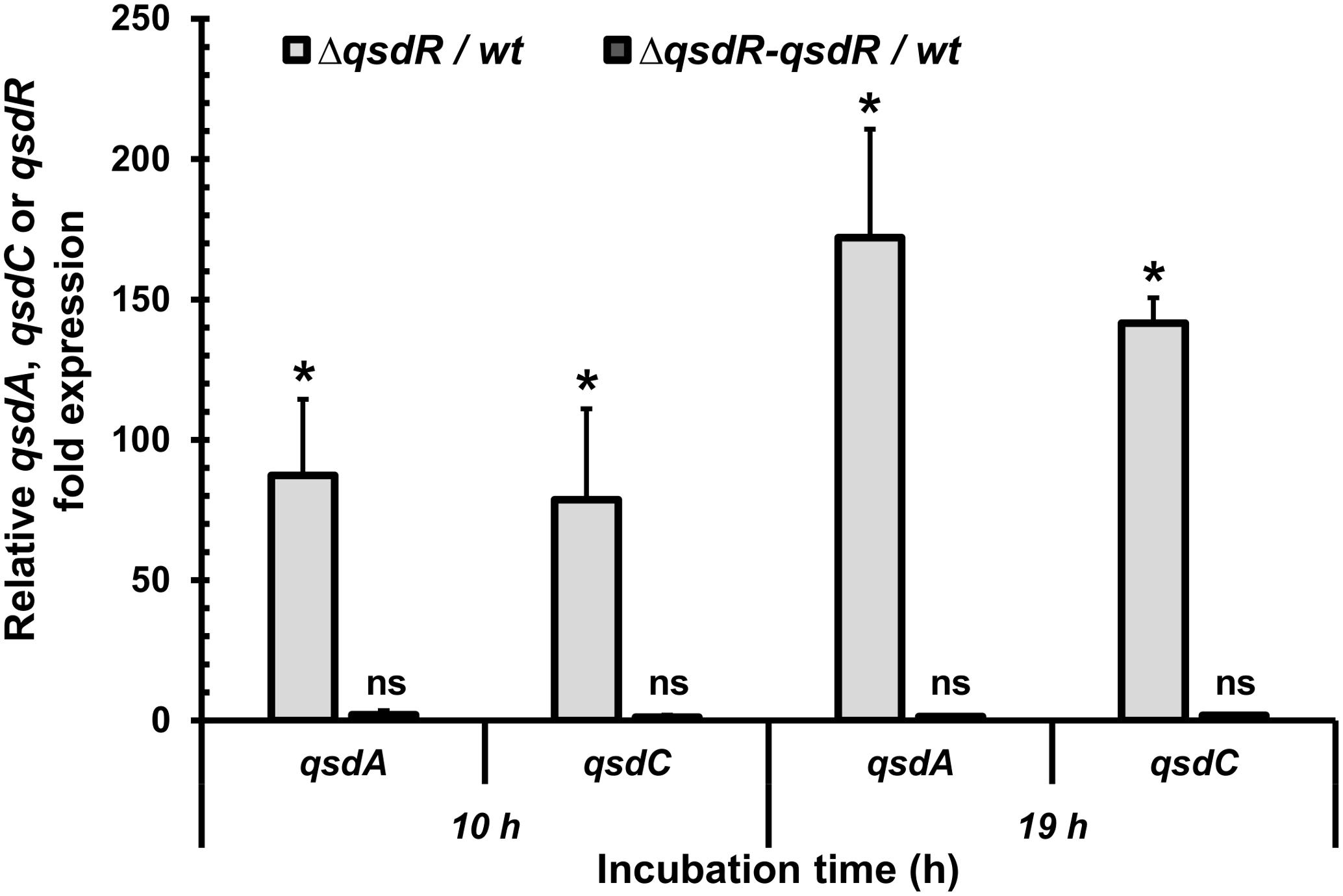
FIGURE 3. Increased qsdA and qsdC mRNA levels in a ΔqsdR mutant strain. qRT-PCR analysis shows the qsdA and qsdC mRNA levels in the ΔqsdR mutant and in the complementing strain (ΔqsdR-qsdR) grown in 7H9 medium at the mid-exponential (10 h) and stationary (19 h) phases. These mRNA levels are relative to the wild type strain. Data shown are mean values obtained from three independent experiments. Statistical analysis was performed by the DataAssistTM software (v3.01) used for calculating relative quantitation of gene expression, based on the comparative CT (2-ΔΔCT) method. ∗p-value < 0.05; ns means no significant difference.
In order to analyze the QS signal degrading activity of R. erythropolis in the ΔqsdR mutant, 3-oxo-C8-HSL molecules were added at mid-exponential phase and quantified at different time points along the growth curve by a colorimetric method based on the formation of hydroxamic acids from esters by reaction with hydroxylamine in alkaline solution. In the presence of the wild-type strain, the 3-oxo-C8-HSL amount in the medium diminished continuously until reaching 60 and 40% of its initial quantity 9 and 15 h after its addition, respectively (Figure 4). In contrast, the 3-oxo-C8-HSL degradation by the ΔqsdR mutant was faster than with the wild-type strain. The decrease of 3-oxo-C8-HSL reached 50% with the ΔqsdR mutant 3 h after the addition of the molecule, whereas with the parental strain it was only of 14%. For the complemented strain, the 3-oxo-C8-HSL degradation kinetic followed closely the degradation profile of the wild-type strain (Figure 4).
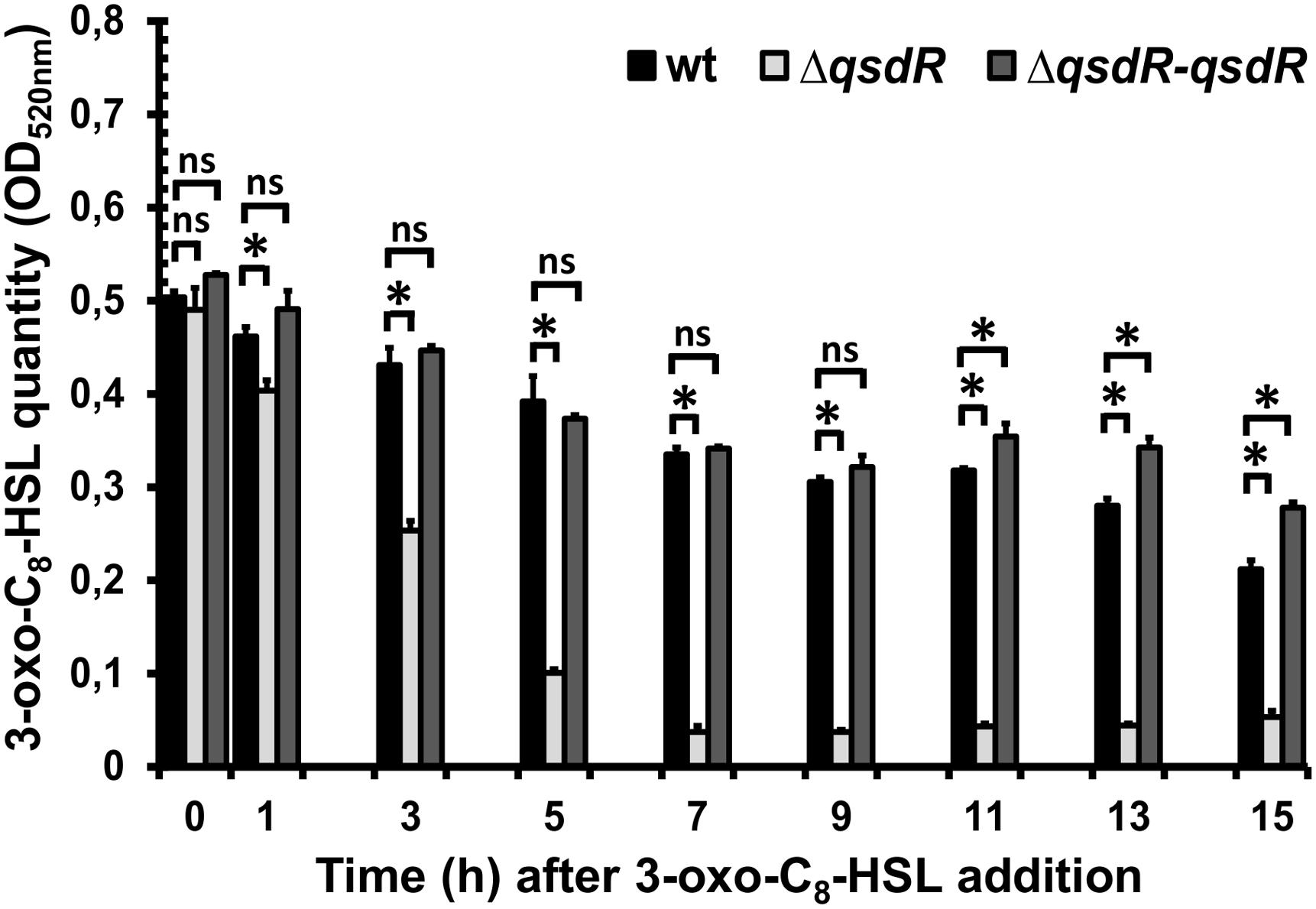
FIGURE 4. Degradation kinetics of 3-oxo-C8-HSL by the R. erythropolis R138 wild-type, ΔqsdR or the complementing strains. Dosage was performed by a colorimetric method in the culture supernatant after centrifugation. Data are the mean ± standard deviation of three independent experiments. Statistical analyses were performed using non-parametric Mann–Whitney tests (two tailed). For each incubation time, ∗ indicates a significant difference in the 3-oxo-C8-HSL quantity in the presence of the ΔqsdR mutant or the complementing strains (p-value < 0.05) relative to that observed in the presence of the wild-type strain. ns mean no significant difference.
Binding of QsdR to the Promoter Regions of the qsd Operon Is Prevented by the Binding of the Lactone Ring of QS Signals to the QsdR Effector Domain
The QsdR structure of R. erythropolis R138 (RCSB PDB accession number 4ZA6) was elucidated after crystallization and X-Ray diffraction (El Sahili et al., 2015). QsdR encodes a monomer (186 residues) capable of an asymmetric dimerization (Figure 5A). Each monomer presents both a DNA-binding domain (N-domain) and an effector binding domain (C-domain), two traits shared by TetR-like family members (Figure 5B). To determine if QsdR could bind to the DNA intergenic region qsdR-qsdA, the purified His-tagged QsdR protein was obtained by cloning qsdR ORF into the expression vector pET19 and by purifying the recombinant protein by affinity chromatography. Gel retardation assays were performed with a 5′ end labeled 170 bp PCR product that contained the qsdR and qsdA promoter regions. Retardation was readily detected when an increased amount of QsdR was added (Figure 6A). Addition of 2- and 10-fold excess of unlabeled promoter containing fragment resulted in a reduction in the proportion of the labeled fragment that was retarded. This competitive binding of unlabeled fragment demonstrated the specificity of the interaction. No retardation was observed with a 5′ end labeled PCR product corresponding to an internal fragment of the qsdA gene.
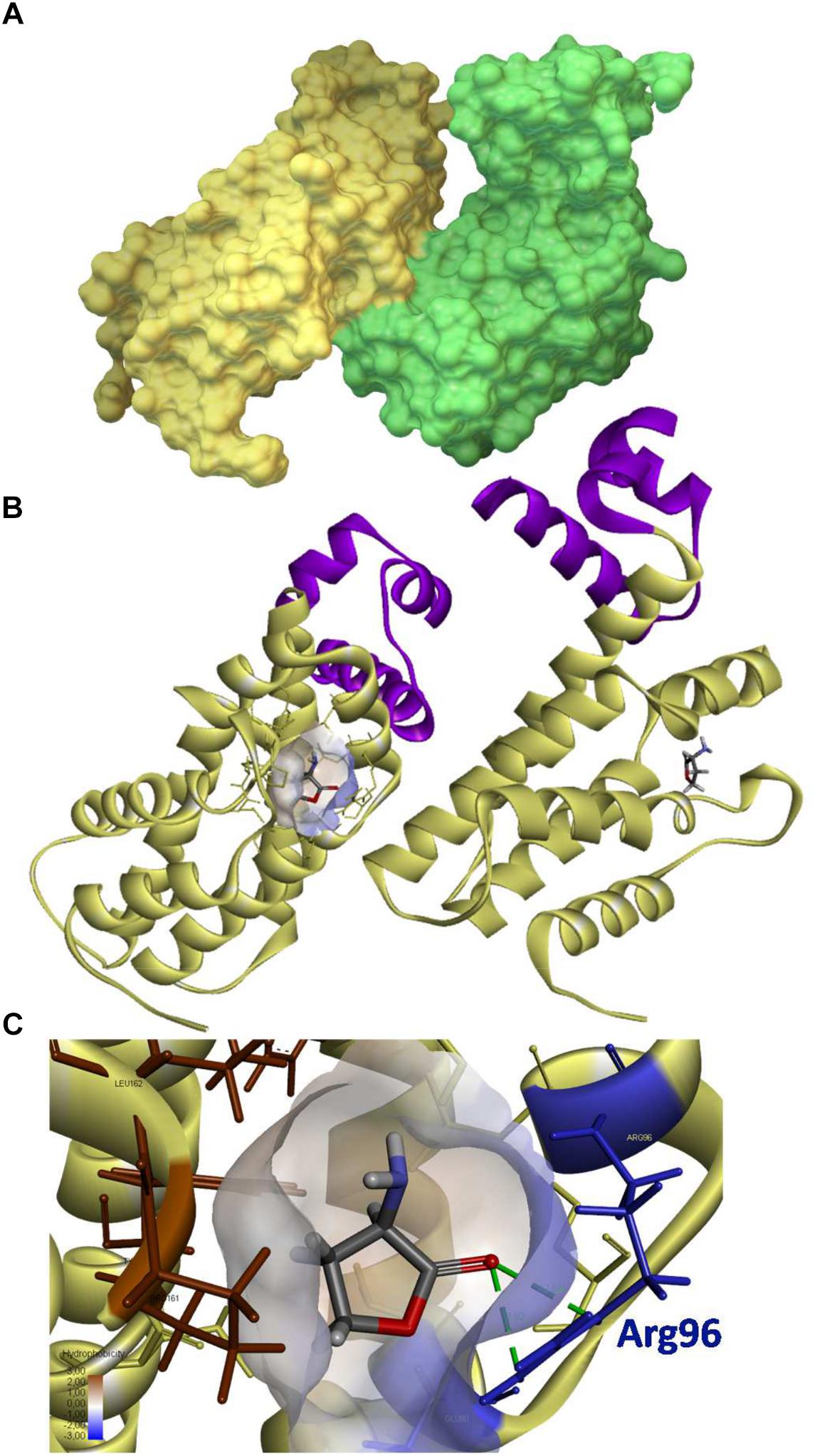
FIGURE 5. 3D structure of QsdR and its potential ligand. The QsdR structure of the R138 strain (RCSB PDB accession number 4ZA6) was elucidated after crystallization and X-Ray diffraction (El Sahili et al., 2015). (A) A solvent-accessible surface area representation shows the functional structure of QsdR consisting of two monomers (yellow and green) that interact asymmetrically. (B) A secondary structure representation shows that each monomer presents both a DNA-binding domain (N-domain) colored in purple and an effector binding domain (C-domain) indicated in yellow, this last one harboring at the core a narrow half apolar/half polar cavity (drawn only on one of the monomers). (C) A close-up of the core cavity and HSL ligand are presented. Molecular docking methodology predicts a binding of the homoserine lactone (HSL), which is also the cyclic moiety of the QS signal, in each of the effector pockets (B,C). The lactone ester bond of the homoserine lactone is oriented toward the hydrophilic part of the pocket (colored in translucent blue) while the rest of the ligand molecule is oriented toward the hydrophobic part (colored in translucent brown). The hydrogen bonds between the oxygen atom of lactone carbonyl and the nitrogen atoms of Arg96 residue are indicated in green dashed line.
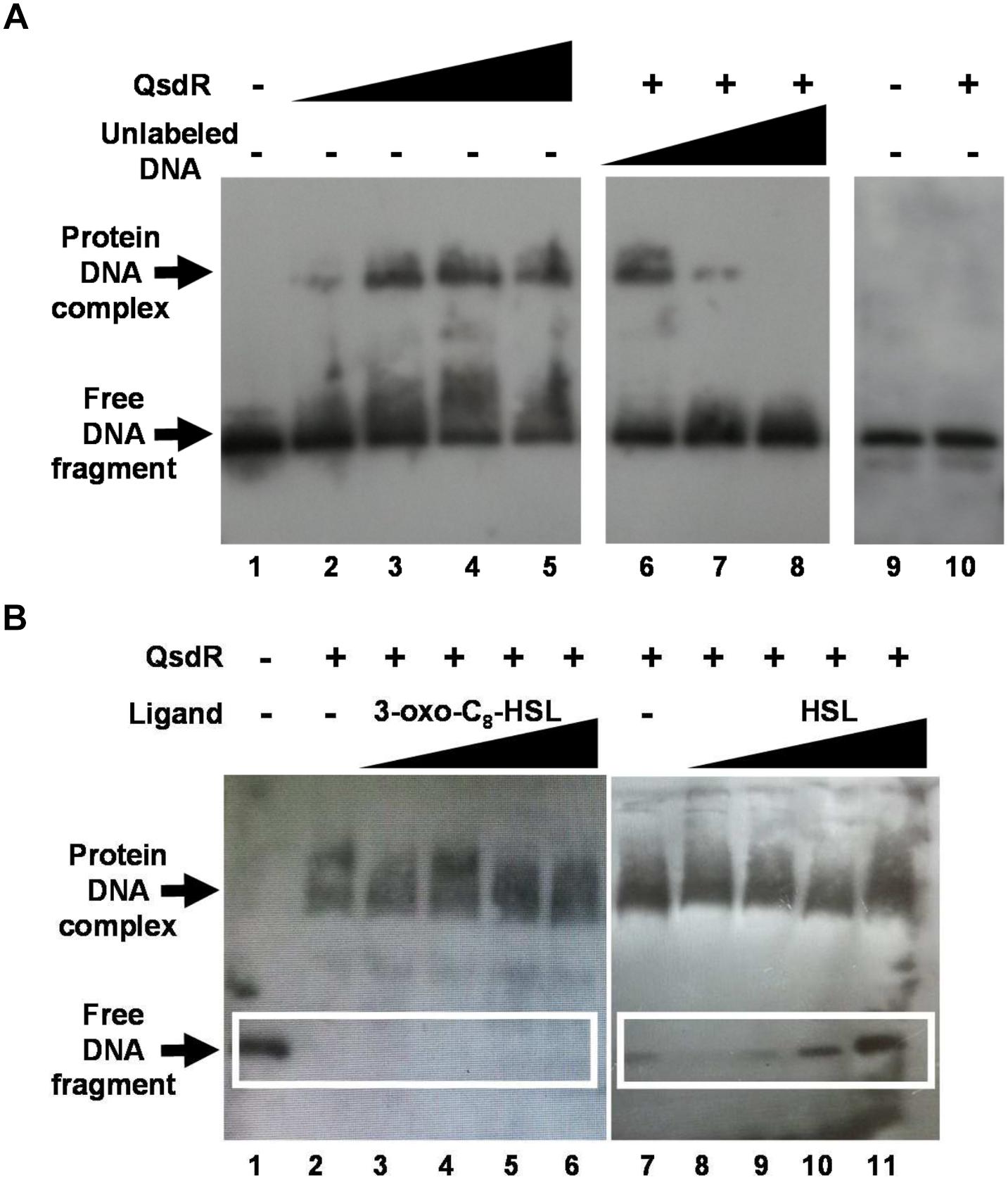
FIGURE 6. Detection of a QsdR–DNA complex by EMSA using biotin labeled qsdR qsdA intergenic DNA fragment. (A) Binding of QsdR to 0.4 ng of the qsdR qsdA intergenic DNA fragment with increasing amounts of His-tagged QsdR. Lane 1: biotin labeled fragment in the absence of protein (-); lanes 2–5: 0.3, 1, 2, and 3 μg, respectively, of the purified His-tagged QsdR protein; lanes 6–8: 0.4, 0.8, and 4 ng, respectively, of the unlabeled competitor qsdR qsdA intergenic DNA fragment with 2 μg of the purified QsdR protein (+); lanes 9–10: EMSA performed with 0.4 ng of a biotin labeled DNA internal fragment of qsdA gene, used as negative control, in the absence (-) or in the presence (+) of 2 μg of the purified QsdR protein. (B) Effect of ligands on the QsdR binding activity. EMSA was performed under the same conditions as described in panel (A) and without (lanes 1, 2, and 7) or with increasing amounts of 3-oxo-C8-HSL (lanes 3–6) or HSL (lanes 8–11) molecules by adding a ligand/QsdR molecules ratio increasing from 0.1 to 100 to the reaction binding. The data shown are representative pictures of three independent experiments. Symbols + and - mean the presence or absence of the corresponding molecule, respectively.
As expected in a TetR-like regulator, a potential binding site appears in the core of each effector domains (Cuthbertson and Nodwell, 2013). This cavity is narrow with both polar and apolar traits, suggesting the binding of small amphiphilic molecules such as small lactone molecules to the QsdR protein (Figure 5B). Conversely, the affinity of QsdR to lactones bearing long aliphatic chain should be limited. In order to identify potential ligand(s) of QsdR, an in silico analysis was carried out by molecular docking using the functional structure of QsdR and those of some suspected ligands. Molecules tested included both complete known signaling molecules and the AHL γ-butyrolactone ring, i.e., homoserine lactone (HSL) molecules. Among those molecules, only the HSL showed a docking in the effector binding site of each QsdR monomers (Figures 5B,C). The calculated affinity value (-17.6 kJ/mol) was sufficient to support a strong interaction with HSL and was identical for each QsdR binding pockets. QsdR–HSL interaction should be favored by (i) the close correspondence between the size of the ligand and that of the binding site, (ii) the presence of two hydrogen bonds (2.92 and 2.93 Å length) between the lactone carbonyl oxygen and the Arg96 residue, and (iii) the amphiphilic character of the effector cavity. The latter should involve the Glu90 and Arg96 polar residues and the Phe106, Leu158, Tyr159, Pro161, and Leu162 apolar residues (Figure 5C).
Based on the results of molecular docking, HSL and 3-oxo-C8-HSL interaction was investigated by gel retardation assays to evaluate their ability to affect the DNA binding activity of QsdR. Increasing amounts of HSL or 3-oxo-C8-HSL were used in a 10-fold dilution series in binding reactions of the labeled fragment with a constant concentration of QsdR. Addition of increased amounts of HSL induced a proportional increase in free DNA, indicating a reduction of DNA-QsdR complex formation. By contrast, 3-oxo-C8-HSL failed to inhibit the DNA binding activity of QsdR (Figure 6B). These results are fully consistent with molecular docking studies.
A Transcriptional Fusion Approach Allows Proposal of a Model for the Regulation of the qsd Operon
In order to go further into the validation of the interaction between QsdR and QS signals, we constructed a vector pEPR1-qsdR-Pqsd::gfp-mCherry that mimics the regulation system of the qsd operon. Firstly, a mCherry cassette under constitutive promoter was introduced into the pEPR1 vector to tag bacteria constitutively in red fluorescence (Figure 7A). Secondly, the promoter probe vector pEPR1-mCherry was modified by introducing the qsd promoter upstream of the gfp ORF to make a Pqsd::gfp transcriptional fusion and investigate qsd promoter activity. Confocal microscopy analysis of R138 pEPR1-Pqsd::gfp-mCherry cultures without inducer (AHL) molecules revealed the presence of yellow-greenish tagged bacteria, resulting from the expression of a mixture of red and green fluorescent proteins (Figure 7B). This demonstrates a constitutive expression of gfp under the control of qsd promoter. Thirdly, the introduction of the qsdR gene into the pEPR1-Pqsd::gfp-mCherry vector led to the observation by confocal microscopy of mCherry producing R138 pEPR1-qsdR-Pqsd::gfp-mCherry bacteria in the absence of inducer molecules (Figure 7C). The absence of gfp expressing bacteria demonstrates the role of QsdR as a repressor of the qsd promoter activity. Addition of 3-oxo-C8-HSL molecules in R138 pEPR1-qsdR-Pqsd::gfp-mCherry cultures induced the appearance of yellow tagged bacteria 2 h after the onset of the experiment (Figure 7C). Analysis by confocal microscopy of R138 pEPR1-qsdR-Pqsd::gfp-mCherry cultivated with different inducer concentrations (from 10 nM to 1 mM) led to the determination of a threshold 3-oxo-C8-HSL concentration for qsd promoter induction. This was estimated to 1 μM. Interestingly, identical results were obtained when 3-oxo-C12-HSL or C4-HSL were used as inducer (Figure 7), or when identical concentrations of HSL were used in place of the signaling molecules (Figure 7). These results confirmed the key role of the lactone ring structure in this regulatory mechanism.
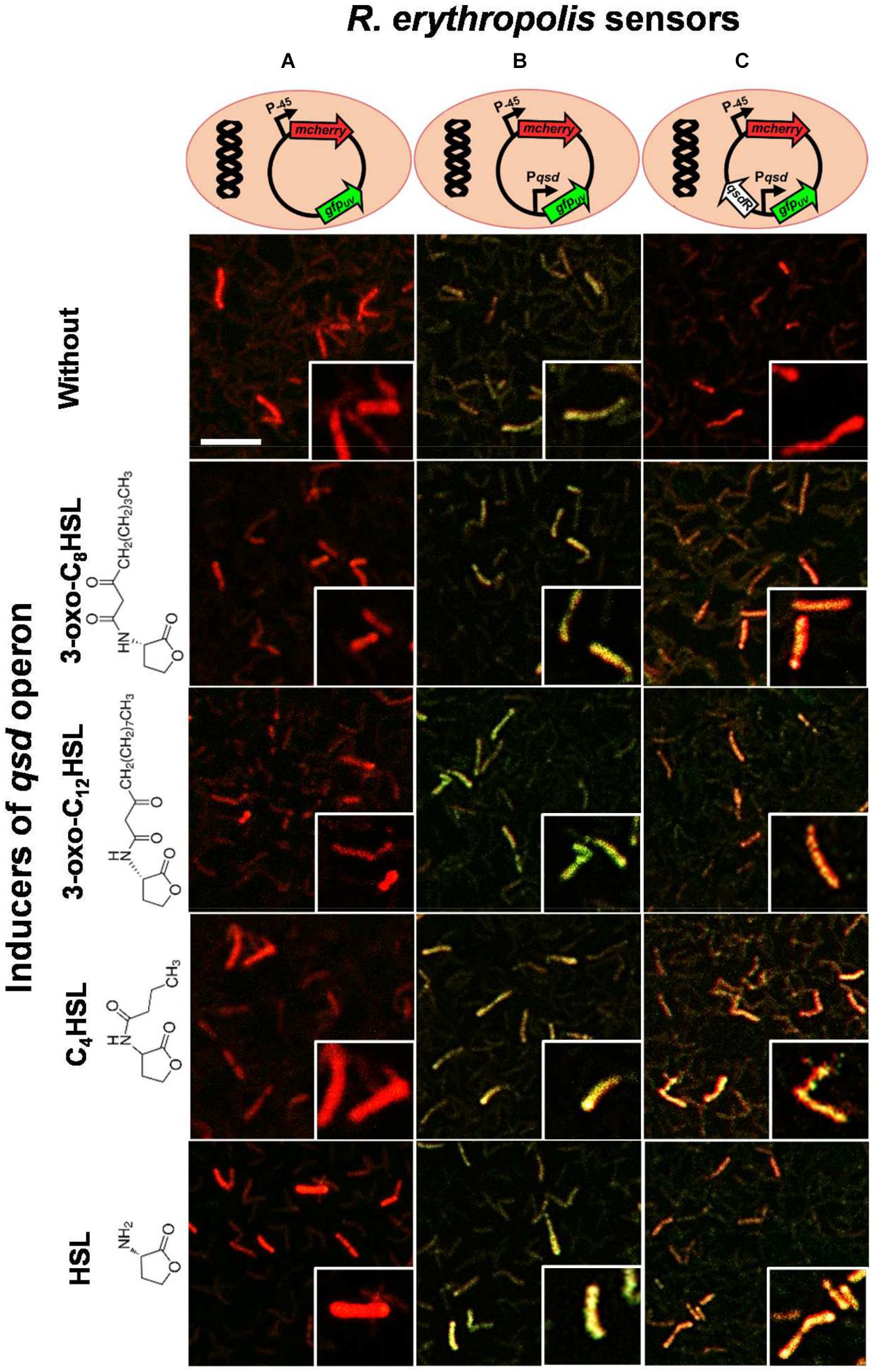
FIGURE 7. Confocal microscopy analysis of the transcriptional activity of the qsd operon. Three dual-colored R. erythropolis R138 sensors were constructed using the pEPR1 vector containing a green fluorescent protein gene as reporter gene and a red fluorescent protein mcherry gene as a cell tag. These strains carry (A) the gfp gene without promoter (as a control), (B) the gfp gene under control of the qsd promoter, and (C) the gfp gene under control of the qsd promoter and QsdR regulator (mimicking the regulation system of qsd operon expression). Rhodococcal cells were cultured in hexanoate minimal medium and harvested every 2 h after the addition of one micromolar of 3-oxo-C8-HSL, 3-oxo-C12-HSL, C4-HSL, or HSL. Cultures in hexanoate without inducer were used as control. Map of each designed vector are schematized at the top of the figure and the structure of inducers with the common γ-lactone ring is drawn on the left of the figure. Fluorescence images were combined in the green and red channels (see section “Materials and Methods”), the co-expression of the two fluorescent proteins causing a yellow-greenish to amber fluorescence depending on the intensity of gfp expression. A close-up of some cells is proposed in a lower right window. Scale bar represents 10 μm.
Discussion
The existence of communication mechanisms within microbial populations should imply the concomitant existence of signal sensing and disrupting systems in competing environmental populations belonging to common ecological niches (Reimmann et al., 2002; D’Angelo-Picard et al., 2005). Knowledge of such systems should permit better understanding of the ecological roles dedicated to QQ and their various potential applications (Njoroge and Sperandio, 2009; Grandclément et al., 2016; Zhang and Li, 2016). However, due to the lack of screening systems, QQ remains as a mechanism under-explored among the promising Actinobacteria as pointed out by Polkade et al. (2016). Members of the R. erythropolis species are characterized by their remarkable metabolic versatility with specific enzymatic abilities to degrade recalcitrant and xenobiotic compounds (van der Geize and Dijkhuizen, 2004; de Carvalho and da Fonseca, 2005; Larkin et al., 2005; Martínková et al., 2009). This metabolic capacity is notably illustrated in strain R138 by the qsd pathway involved in its biocontrol activity against soft-rot phytopathogens (Barbey et al., 2013). This pathway strongly implicates the lactonase QsdA (Uroz et al., 2008; Barbey et al., 2012). Even though the 3-oxo-C8-HSL appears as one of the QsdA’s best known substrate, a wide range of AHLs including C4-HSL and 3-oxo-C12-HSL may be hydrolyzed by this intracellular lactonase enzyme (Afriat et al., 2006; Uroz et al., 2008; Fetzner, 2015). This broad substrate specificity is confirmed in vivo by the rhodococcal control of potato soft-rot, and by the rhodococcal suppression of tuber necrosis due to P. aeruginosa PA14 without bacterial population diminution (Figure 1). Since the virulence of the PA14 strain was weakly affected in the presence of the R. erythropolis mutant strain defective in the production of the lactonase QsdA, the biocontrol activity against P. aeruginosa may be explained by the degradation of 3-oxo-C12-HSL and C4-HSL signaling molecules. These AHL signals are known to regulate the production of Pseudomonas virulence factors altering both plant and animal host cells, like phospholipase C and exotoxin A (Rahme et al., 2000; Reimmann et al., 2002).
Rhodococcal Quorum-Quenching Activity Is Regulated by a TetR-Like Repressor
The QQ QsdA enzyme is encoded by the qsd operon, the expression of which has been shown in planta to be induced by AHL when a quorum pathogen density is reached (Barbey et al., 2013). This induction by AHLs was confirmed in the present work by analyzing the qsd operon expression by qRT-PCR and by the transcriptional fusion approaches. The latter led to the establishment of a threshold concentration of 1 μM for induction by AHLs. This concentration was observed for all studied AHLs. This value might have ecological significance since it corresponds to previous measurements recorded in soft-rotting tuber colonized by the P. atrosepticum CFBP6276 strain (2.5 μM) (Barbey et al., 2013) or P. atrosepticum SCRI1043 strain (290 μM) (Liu et al., 2008).
Previous observations added to in silico DNA analysis confirms the existence of a regulator for the qsd operon expression named QsdR (Latour et al., 2013; El Sahili et al., 2015). QsdR belongs to the TetR transcriptional regulator family, which represents a large and important family of one-component signal transduction systems (Cuthbertson and Nodwell, 2013). The disruption of qsdR resulted in high level expression of the qsd operon, preventing the repression of its expression. This lifting of repression mechanism was phenotypically observed with the enhancement of the AHL degrading activity in the ΔqsdR strain, compared to the wild-type strain. Furthermore, EMSA experiments demonstrated a physical binding of QsdR to the qsd promoter region. All of these results support the hypothesis that QsdR acts as a negative regulator for the qsd operon expression, as reported for most TetR-like regulators (Ramos et al., 2005; Cuthbertson and Nodwell, 2013). A simplified model of the qsd operon repression is illustrated in Figure 8A: the absence of QsdA and QsdC products results from repression of transcription by QsdR binding to the qsd promoter region. TetR family regulators are known to regulate the expression of efflux pumps and transporters involved in antibiotic resistance and tolerance to toxic chemicals (Ramos et al., 2005; Reichheld et al., 2009). A large majority of them also control other functions: carbon, nitrogen and co-factor metabolism as well as cell division and cell-to-cell signaling (Ramos et al., 2005; Cuthbertson and Nodwell, 2013). Interestingly, another TetR-like regulator implicated in lactone metabolism was described in R. erythropolis: this is the LplR repressor involved in the regulation of the L-pantoyl lactone dehydrogenase encoding gene expression essential for the vitamin B5 synthesis (Si et al., 2012). Besides, a TetR based regulation of a lactonase encoding gene expression was reported in A. tumefaciens: the transcriptional repressor BclR regulates the expression of blcABC operon which codes for the BclC lactonase (Khan and Farrand, 2009; Lang and Faure, 2014). However, bclC expression was demonstrated to be induced by a byproduct of γ-butyrolactone metabolism, succinate semialdehyde, but not by AHL signals or its byproducts.
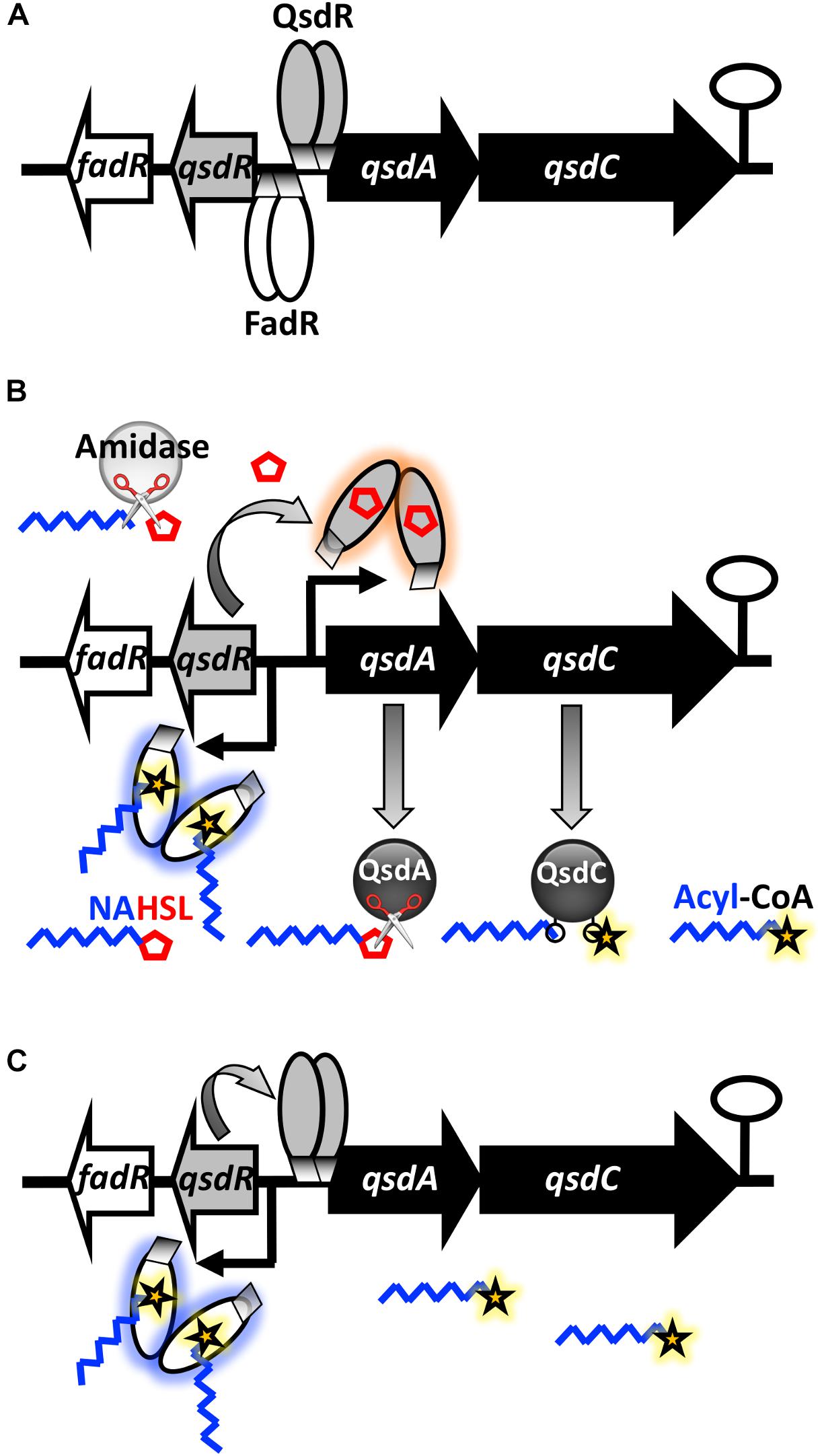
FIGURE 8. Proposed model for the regulation of the qsd operon expression by quorum-sensing signals in R. erythropolis. (A) In the absence of an inducer, the TetR-like QsdR regulator forms a dimer that binds to the promoter region and directly represses qsdA and qsdC expression, switching off the pathway. (B) When N-acyl-L-homoserine lactones (AHLs) are present in cellular environment, some of them are cleaved by the previously characterized extra-operonic gene encoded AHL amidase (Latour et al., 2013) which hydrolyses the amide bond of these molecules (symbolized by scissors). The γ-butyrolactone ring moiety (i.e., homoserine lactone) binds to QsdR, thereby changing the conformation, such that QsdR cannot bind to the promoter region, inducing derepression of the qsd cluster gene expression. This leads to the production of QsdA and QsdC enzymes, responsible for the lactone ester bond hydrolysis and activation (coenzyme A ligation) of the aliphatic part of signaling molecules, respectively. (C) When AHL molecules are fully hydrolyzed, the disappearance of HSL rings restores the binding between QsdR and the DNA promoter sequence leading to the lock of qsd operon expression by the QsdR repressor. The concomitant increasing in the intracellular amount of acyls-CoA molecules facilitates this phenomenon, since these molecules bind to the effector domain of the FadR regulator, causing a conformational change in the dimeric protein and the production of the QsdR repressor. This step is proposed according to in silico analysis of the genome of R. erythropolis strain R138 and the recent and well-documented bibliography related to the functioning of FadR regulator (Bacik et al., 2015; My et al., 2015). (Black curved arrows and hairpin represents the initiation of gene transcription and a putative transcription terminator, respectively.)
Quorum-Sensing Signals Indirectly Activate Quorum-Quenching Activity
According to qRT-PCR and transcriptional fusion assays, addition of the QS signal induced an increase in qsd operon expression and in qsd promoter activity, respectively, demonstrating a role of these inducers in derepression of gene expression. In the well-characterized TetR family regulators, this derepression is usually operated by the direct binding of small-molecule ligands to the signal-receiving domain of TetR, that causes a conformational change preventing its specific binding at the promoters of target genes (Reichheld et al., 2009; Cuthbertson and Nodwell, 2013). Generally, ligands involved in metabolism regulation correspond to the substrate intended to be degraded or a catabolic intermediate of the target gene product (Cuthbertson and Nodwell, 2013). A docking approach predicted the binding of the AHL γ-butyrolactone ring (i.e., HSL) molecules to the core of each QsdR effector domain. These results were experimentally directly supported by a decrease in DNA-protein complex formation only in the presence of HSL in EMSA experiments and indirectly by confocal microscopy GFP fluorescence analysis (Figure 7). Thus, the induction of the qsd operon expression by QS signals seems to be operated by HSL, especially since a same threshold induction value (1 μM) has been recorded for HSL and AHLs (Figure 7). However, we cannot exclude the existence in the environment of other ligands whose structure should be close to that of HSL, nor the involvement of the qsd pathway in the degradation of other QS signals such as the 2,3-disubstituted γ-butyrolactones used by some Streptomyces (Takano, 2006) and Rhodococcus (Latour et al., 2013; Ceniceros et al., 2017a,b) species. In this case, the QQ pathway of R. erythropolis would be also involved in the turn-over of its QS signals and therefore the regulation of its own communication.
The HSL-QsdR binding requires the implication of an extra-operonic gene encoded AHL amidase to release the lactone ring from the signaling molecules. This hydrolysis should generate two byproducts, a fatty acid and one molecule of free HSL, the latter serving as an inducer of their own catabolism (Figure 8B). This AHL cleavage hypothesis is supported by (i) the AHL amide bond cleavage activity previously detected in R. erythropolis (Uroz et al., 2005), (ii) the fact that the deletion of the qsdA gene did not completely abolish the biocontrol activity of the strain R138 (Figure 1) implying the complementary activity of another AHLase, and above all (iii) the characterization of an AHL amidase (NCBI reference WP_019744590.1) in the R138 strain proteome obtained after bacterial growth with 3-oxo-C8-HSL as a sole C source (Latour et al., 2013). In this case, the question of the substrates diversity of this amidase arises, since it will determine the range of AHLs that can be degraded. Most AHL amidases display a preference for long-chain AHLs with or without a substituent at C3 of the acyl chain (Fetzner, 2015). However, AiiC produced by Anabaena sp. PCC7120 (Romero et al., 2010) is involved in the hydrolysis of a wide range of AHLs including C4-HSL, the signal of the rhl system of P. aeruginosa.
The regulation of the qsd operon expression probably involves autoregulation systems. The expression of QsdR should be influenced by fatty acid metabolism due to the presence of both a FadR encoding gene located immediately downstream of qsdR and a FadR binding site predicted upstream of the -35 box of the qsdR promoter (Supplementary Figure S1). FadR is a fatty acid degradation transcriptional regulator controlling fatty acid metabolism in response to intracellular concentrations of acyl-CoA esters (DiRusso et al., 1992; My et al., 2015). In the E. coli model, FadR repression of fad genes expression is due to its binding to the promoter sites. This repression is lifted by interaction between FadR and long-chain acyls-CoA that have accumulated inside the cell (Bacik et al., 2015; My et al., 2015). In Gram-positive bacteria, FadR also regulates several operons required for fatty acid degradation and recognizes fatty acyls-CoA as effectors (Matsuoka et al., 2007). The existence and location of such regulator was first reported in the R. erythropolis species by Uroz et al. (2008) and echoes the presence of the QsdC (syn. FadD) fatty acyls-CoA ligase encoded by the qsd operon. Indeed, in E. coli, acyl-CoA esters resulting from FadD activity on fatty acids bind to FadR, repressing the expression of catabolic genes (Pech-Canul et al., 2011). This suggests a control of qsdR expression by FadR and consequently a ligand role for the acyl part of QS signals after ligation with coenzyme A (Figures 8A,B). In this hypothesis, the qsd operon expression might be locked in response to the hydrolysis of all γ-lactone rings coupled with the presence of a large amount of acyl-CoA esters resulting from QsdA and QsdC activities on signaling molecules (Figure 8C). This hypothesis might explain the decrease in the qsdR expression following the 3-oxo-C8-HSL molecules consumption by Rhodococcus (Figure 2). Significant work remains to be done to understand the possible role of FadR in this regulatory mechanism.
Conclusion
This work provides evidence for AHL-based regulation of QQ enzymes in Rhodococcus which appear to cause a bacterial communication interference. This system seems well adapted to the detection and the degradation of a wide range substrates, since it appears based on detection of the common structure (the HSL ring) of different AHLs. Since signal specificity of AHLs is determined by the length and the substitution of the acyl chain (Brader et al., 2005; Welch et al., 2005; Crépin et al., 2012a), such a regulatory mechanism appears to have a global metabolic action toward the AHL family rather than targeting the communication of a particular population. This asset may confer a selective advantage to rhodococci to successfully compete for resource uptakes and colonization in the same ecological niche as many AHL-producing bacteria (e.g., biofilm and rhizosphere) (Cha et al., 1998; D’Angelo-Picard et al., 2005). This hypothesis is also supported by the fact that 3-oxo substituted-HSL molecules have been demonstrated to be bactericidal in Gram-positive bacteria (Kaufmann et al., 2005). Future studies should elucidate and integrate the role of amidase in the QsdR based regulation system.
Author Contributions
CB, AC, J-FB, and XL conceived and designed the experiments. CB, AC, and OM performed the experiments. CB, AC, and XL analyzed the data and wrote the paper. AM, YK-G, MG, AB-C, VG, and MF participated to technical and scientific discussion.
Funding
This research was supported by grants from French institutions (Ministère de l’Enseignement Supérieur, de la Recherche et de l’Innovation, Régions Bretagne, Normandie et Pays de la Loire, and Evreux Portes de Normandie Agglomération), the network VASI, the Pôle de Compétitivité Valorial, and FEDER (European Union). Works are related to COST 631 action-Understanding and Modeling Plant-Soil Interactions in the Rhizosphere Environment (UMPIRE).
Conflict of Interest Statement
AB-C and VG were employed by an Agricultural Technical Institute, the French Federation of Seed Potato Growers.
The remaining authors declare that the research was conducted in the absence of any commercial or financial relationships that could be construed as a potential conflict of interest.
Acknowledgments
We thank Prof. C. Hulen and Dr. E. Bouffartigues-Maillot for critical discussion. We are also grateful to Dr. William Mohn (Department of Microbiology and Immunology, University of British Columbia, Vancouver, BC, Canada) for the gift of the plasmid pSET152, Dr. Jan Nešvera (Laboratory of Molecular Genetics of Bacteria, Institute of Microbiology, Academy of Sciences of the Czech Republic) for the gift of the plasmid pEPR1, and Prof. C. Thomas (School of Biosciences, University of Birmingham, United Kingdom) for the gift of the plasmid pAKE604.
Supplementary Material
The Supplementary Material for this article can be found online at: https://www.frontiersin.org/articles/10.3389/fmicb.2018.02800/full#supplementary-material
References
Afriat, L., Roodveldt, C., Manco, G., and Tawfik, D. S. (2006). The latent promiscuity of newly identified microbial lactonases is linked to a recently diverged phosphotriesterase. Biochemistry 45, 13677–13686. doi: 10.1021/bi061268r
Ahn, S. K., Cuthbertson, L., and Nodwell, J. R. (2012). Genome context as a predictive tool for identifying regulatory targets of the TetR family transcriptional regulators. PLoS One 7:e50562. doi: 10.1371/journal.pone.0050562
Bacik, J. P., Yeager, C. M., Twary, S. N., and Martí-Arbona, R. (2015). Modulation of FadR binding capacity for acyl-CoA fatty acids through structure-guided mutagenesis. Protein J. 34, 359–366. doi: 10.1007/s10930-015-9630-1
Bais, H. P. (2012). Shoot the messages not the messengers. Plant Soil 358, 7–10. doi: 10.1007/s11104-011-1114-2
Barbey, C., Crépin, A., Bergeau, D., Ouchiha, A., Mijouin, L., Taupin, L., et al. (2013). In Planta biocontrol of Pectobacterium atrosepticum by Rhodococcus erythropolis involves silencing of pathogen communication by the rhodococcal gamma-lactone catabolic pathway. PLoS One 8:e66642. doi: 10.1371/journal.pone.0066642
Barbey, C., Crépin, A., Cirou, A., Budin-Verneuil, A., Orange, N., Feuilloley, M., et al. (2012). Catabolic pathway of gamma-caprolactone in the biocontrol agent Rhodococcus erythropolis. J. Proteome Res. 11, 206–216. doi: 10.1021/pr200936q
Barnard, A. M. L., and Salmond, G. P. C. (2007). Quorum sensing in Erwinia species. Anal. Bioanal. Chem. 387, 415–423. doi: 10.1007/s00216-006-0701-1
Bouffartigues, E., Moscoso, J. A., Duchesne, R., Rosay, T., Fito-Boncompte, L., Gicquel, G., et al. (2015). The absence of the Pseudomonas aeruginosa OprF protein leads to increased biofilm formation through variation in c-di-GMP level. Front. Microbiol. 6:630. doi: 10.3389/fmicb.2015.00630
Brader, G., Sjöblom, S., Hyytiäinen, H., Sims-Huopaniemi, K., and Palva, E. T. (2005). Altering substrate chain length specificity of an acylhomoserine lactone synthase in bacterial communication. J. Biol. Chem. 280, 10403–10409. doi: 10.1074/jbc.M408603200
Ceniceros, A., Dijkhuizen, L., and Petrusma, M. (2017a). Molecular characterization of a Rhodococcus jostii RHA1 γ-butyrolactone(-like) signalling molecule and its main biosynthesis gene gblA. Sci. Rep. 7:17743. doi: 10.1038/s41598-017-17853-6
Ceniceros, A., Dijkhuizen, L., Petrusma, M., and Medema, M. H. (2017b). Genome-based exploration of the specialized metabolic capacities of the genus Rhodococcus. BMC Genomics 18:593. doi: 10.1186/s12864-017-3966-1
Cha, C., Gao, P., Chen, Y. C., Shaw, P. D., and Farrand, S. K. (1998). Production of acyl-homoserine lactone quorum-sensing signals by gram-negative plant-associated bacteria. Mol. Plant Microbe. Interact. 11, 1119–1129. doi: 10.1094/MPMI.1998.11.11.1119
Cirou, A., Diallo, S., Kurt, C., Latour, X., and Faure, D. (2007). Growth promotion of quorum-quenching bacteria in the rhizosphere of Solanum tuberosum. Environ. Microbiol. 9, 1511–1522. doi: 10.1111/j.1462-2920.2007.01270.x
Cirou, A., Mondy, S., An, S., Charrier, A., Sarrazin, A., Thoison, O., et al. (2012). Efficient biostimulation of the native and introduced quorum-quenching Rhodococcus erythropolis is revealed by a combination of analytical chemistry, microbiology and pyrosequencing. Appl. Environ. Microbiol. 78, 481–492. doi: 10.1128/AEM.06159-11
Cirou, A., Raffoux, A., Diallo, S., Latour, X., Dessaux, Y., and Faure, D. (2011). Gamma-caprolactone stimulates growth of quorum-quenching Rhodococcus populations in a large-scale hydroponic system for culturing Solanum tuberosum. Res. Microbiol. 162, 945–950. doi: 10.1016/j.resmic.2011.01.010
Crépin, A., Barbey, C., Beury-Cirou, A., Hélias, V., Taupin, L., Reverchon, S., et al. (2012a). Quorum sensing signaling molecules produced by reference and emerging soft-rot bacteria (Dickeya and Pectobacterium spp.). PLoS One 7:e35176. doi: 10.1371/journal.pone.0035176
Crépin, A., Barbey, C., Cirou, A., Tannières, M., Orange, N., Feuilloley, M., et al. (2012b). Biological control of pathogen communication in the rhizosphere: a novel approach applied to potato soft rot due to Pectobacterium atrosepticum. Plant Soil 358, 27–37. doi: 10.3390/s120303484
Crépin, A., Beury-Cirou, A., Barbey, C., Farmer, C., Hélias, V., Burini, J. F., et al. (2012c). N-acyl homoserine lactones in diverse Pectobacterium and Dickeya plant pathogens: diversity, abundance, and involvement in virulence. Sensors 12, 3484–3497. doi: 10.3390/s120303484
Cuthbertson, L., and Nodwell, J. R. (2013). The TetR family of regulators. Microbiol. Mol. Biol. Rev. 77, 440–475. doi: 10.1128/MMBR.00018-13
D’Angelo-Picard, C., Faure, D., Penot, I., and Dessaux, Y. (2005). Diversity of N-acyl homoserine lactone-producing and -degrading bacteria in soil and tobacco rhizosphere. Environ. Microbiol. 7, 1796–1808. doi: 10.1111/j.1462-2920.2005.00886.x
de Carvalho, C. C., and da Fonseca, M. M. (2005). The remarkable Rhodococcus erythropolis. Appl. Microbiol. Biotechnol. 67, 715–726. doi: 10.1007/s00253-005-1932-3
Defoirdt, T., Boon, N., and Bossier, P. (2010). Can bacteria evolve resistance to quorum sensing disruption? PLoS Pathog. 6:e1000989. doi: 10.1371/journal.ppat.1000989
Dessaux, Y., Grandclément, C., and Faure, D. (2016). Engineering the Rhizosphere. Trends Plant Sci. 21, 266–278. doi: 10.1016/j.tplants.2016.01.002
DiRusso, C., Heimert, T. L., and Metzger, A. K. (1992). Characterization of FadR, a global transcriptional regulator of fatty acid metabolism in Escherichia coli. Interaction with the fadB promoter is prevented by long chain fatty acid coenzyme A. J. Biol. Chem. 267, 8685–8691.
Dong, Y. H., Wang, L. H., Xu, J. L., Zhang, H. B., Zhang, X. F., and Zhang, L. H. (2001). Quenching quorum-sensing-dependent bacterial infection by an N-acyl homoserine lactonase. Nature 411, 813–817. doi: 10.1038/35081101
Dong, Y. H., Wang, L. H., and Zhang, L. H. (2007). Quorum-quenching microbial infections: mechanisms and implications. Philos. Trans. R. Soc. Lond. B Biol. Sci. 362, 1201–1211. doi: 10.1098/rstb.2007.2045
Dong, Y. H., Xu, J. L., Li, X. Z., and Zhang, L. H. (2000). AiiA, an enzyme that inactivates the acylhomoserine lactone quorum-sensing signal and attenuates the virulence of Erwinia carotovora. Proc. Natl. Acad. Sci. U.S.A. 97, 3526–3531. doi: 10.1073/pnas.97.7.3526
El Sahili, A., Kwasiborski, A., Mothe, N., Velours, C., Legrand, P., Moréra, S., et al. (2015). Natural guided genome engineering reveals transcriptional regulators controlling quorum-sensing signal degradation. PLoS One 10:e0141718. doi: 10.1371/journal.pone.0141718
El-Sayed, A. K., Hothersall, J., and Thomas, C. M. (2001). Quorum-sensing-dependent regulation of biosynthesis of the polyketide antibiotic mupirocin in Pseudomonas fluorescens NCIMB 10586. Microbiology 147, 2127–2139. doi: 10.1099/00221287-147-8-2127
Fetzner, S. (2015). Quorum quenching enzymes. J. Biotechnol. 201, 2–14. doi: 10.1016/j.jbiotec.2014.09.001
Garcia-Contreras, R. (2016). Is quorum sensing interference a viable alternative to treat Pseudomonas aeruginosa infections? Front. Microbiol. 7:1454. doi: 10.3389/fmicb.2016.01454
Garcia-Contreras, R., Maeda, T., and Wood, T. K. (2013). Resistance to quorum-quenching compounds. Appl. Environ. Microbiol. 79, 6840–6846. doi: 10.1128/AEM.02378-13
Grandclément, C., Tannières, M., Moréra, S., Dessaux, Y., and Faure, D. (2016). Quorum quenching: role in nature and applied developments. FEMS Microbiol. Rev. 40, 86–116. doi: 10.1093/femsre/fuv038
Guendouze, A. (2017). Effect of quorum quenching lactonase in clinical isolates of Pseudomonas aeruginosa and comparison with quorum sensing inhibitors. Front. Microbiol. 14:227. doi: 10.3389/fmicb.2017.00227
Helman, Y., and Chernin, L. (2015). Silencing the mob: disrupting quorum sensing as a means to fight plant disease. Mol. Plant Pathol. 16, 316–329. doi: 10.1111/mpp.12180
Jimenez, P. N., Koch, G., Thompson, J. A., Xavier, K. B., Cool, R. H., and Quax, W. J. (2012). The multiple signaling systems regulating virulence in Pseudomonas aeruginosa. Microbiol. Mol. Biol. Rev. 76, 46–65. doi: 10.1128/MMBR.05007-11
Kaufmann, G. F., Sartorio, R., Lee, S. H., Rogers, C. J., Meijler, M. M., Moss, J. A., et al. (2005). Revisiting quorum sensing: discovery of additional chemical and biological functions for 3-oxo-N-acylhomoserine lactones. Proc. Natl. Acad. Sci. U.S.A. 102, 309–314. doi: 10.1073/pnas.0408639102
Khan, S. R., and Farrand, S. K. (2009). The BlcC (AttM) lactonase of Agrobacterium tumefaciens does not quench the quorum-sensing system that regulates Ti plasmid conjugative transfer. J. Bacteriol. 191, 1320–1329. doi: 10.1128/JB.01304-08
Knoppová, M., Phensaijai, M., Veseý, M., Zemanová, M., Nešvera, J., and Pátek, M. (2007). Plasmid vectors for testing in vivo promoter activities in Corynebacterium glutamicum and Rhodococcus erythropolis. Curr. Microbiol. 55, 234–239. doi: 10.1007/s00284-007-0106-1
Kwasiborski, A., Mondy, S., Chong, T. M., Barbey, C., Chan, K. G., Beury-Cirou, A., et al. (2015). Transcriptome of the quorum-sensing signal-degrading Rhodococcus erythropolis responds differentially to virulent and avirulent Pectobacterium atrosepticum. Heredity 114, 476–484. doi: 10.1038/hdy.2014.121
Lang, J., and Faure, D. (2014). Functions and regulation of quorum-sensing in Agrobacterium tumefaciens. Front. Plant Sci. 5:14. doi: 10.3389/fpls.2014.00014
Larkin, M. J., Kulakov, L. A., and Allen, C. C. (2005). Biodegradation and Rhodococcus-masters of catabolic versatility. Curr. Opin. Biotechnol. 16, 282–290. doi: 10.1016/j.copbio.2005.04.007
LaSarre, B., and Federle, M. J. (2013). Exploiting quorum sensing to confuse bacterial pathogens. Microbiol. Mol. Biol. Rev. 77, 73–111. doi: 10.1128/MMBR.00046-12
Latour, X., Barbey, C., Chane, A., Groboillot, A., and Burini, J. F. (2013). Rhodococcus erythropolis and its γ-lactone catabolic pathway: an unusual biocontrol system that disrupts pathogen quorum sensing communication. Agronomy 3, 816–838. doi: 10.3390/agronomy3040816
Latour, X., Diallo, S., Chevalier, S., Morin, D., Smadja, B., Burini, J. F., et al. (2007). Thermoregulation of N-acyl homoserine lactone-based quorum sensing in the soft rot bacterium Pectobacterium atrosepticum. Appl. Environ. Microbiol. 73, 4078–4081. doi: 10.1128/AEM.02681-06
Leadbetter, J. R., and Greenberg, E. P. (2000). Metabolism of acyl-homoserine lactone quorum-sensing signals by Variovorax paradoxus. J. Bacteriol. 182, 6921–6926. doi: 10.1128/JB.182.24.6921-6926.2000
Lin, Y. H., Xu, J. L., Hu, J., Wang, L. H., Ong, S. L., Leadbetter, J. R., et al. (2003). Acyl-homoserine lactone acylase from Ralstonia strain XJ12B represents a novel and potent class of quorum-quenching enzymes. Mol. Microbiol. 47, 849–860. doi: 10.1046/j.1365-2958.2003.03351.x
Liu, H., Coulthurst, S. J., Pritchard, L., Hedley, P. E., Ravensdale, M., Humphris, S., et al. (2008). Quorum sensing coordinates brute force and stealth modes of infection in the plant pathogen Pectobacterium atrosepticum. PLoS Pathog. 20:e1000093. doi: 10.1371/journal.ppat.1000093
Mansfield, J., Genin, S., Magori, S., Citovsky, V., Sriariyanum, M., Ronald, P., et al. (2012). Top 10 plant pathogenic bacteria in molecular plant pathology. Mol. Plant Pathol. 13, 614–629. doi: 10.1111/j.1364-3703.2012.00804.x
Martínková, L., Uhnáková, B., Pátek, M., Nešvera, J., and Kren, V. (2009). Biodegradation potential of the genus Rhodococcus. Environ. Int. 35, 162–177. doi: 10.1016/j.envint.2008.07.018
Matsuoka, H., Hirooka, K., and Fujita, Y. (2007). Organization and function of the YsiA regulon of Bacillus subtilis involved in fatty acid degradation. J. Biol. Chem. 282, 5180–5194. doi: 10.1074/jbc.M606831200
Mellbye, B., and Schuster, M. (2011). The sociomicrobiology of antivirulence drug resistance: a proof of concept. mBio 2:e00131-11. doi: 10.1128/mBio.00131-11
Morris, G. M., Huey, R., Lindstrom, W., Sanner, M. F., Belew, R. K., Goodsell, D. S., et al. (2009). AutoDock4 and AutoDockTools4: automated docking with selective receptor flexibility. J. Comput. Chem. 30, 2785–2791. doi: 10.1002/jcc.21256
Mund, A., Diggle, S. P., and Harrison, F. (2017). The fitness of Pseudomonas aeruginosa quorum sensing signal cheats is influenced by the diffusivity of the environment. mBio 8:e00353-17. doi: 10.1128/mBio.00353-17
My, L., Ghandour Achkar, N., Viala, J. P., and Bouveret, E. (2015). Reassessment of the genetic regulation of fatty acid synthesis in Escherichia coli: global positive control by the functional dual regulator FadR. J. Bacteriol. 197, 1862–1872. doi: 10.1128/JB.00064-15
Njoroge, J., and Sperandio, V. (2009). Jamming bacterial communication: new approaches for the treatment of infectious diseases. EMBO Mol. Med. 1, 201–210. doi: 10.1002/emmm.200900032
Oh, H. S., Kim, S. R., Cheong, W. S., Lee, C. H., and Lee, J. K. (2013). Biofouling inhibition in MBR by Rhodococcus sp. BH4 isolated from real MBR plant. Appl. Microbiol. Biotechnol. 97, 10223–10231. doi: 10.1007/s00253-013-4933-7
Papenfort, K., and Bassler, B. L. (2016). Quorum sensing signal-response systems in gram-negative bacteria. Nat. Rev. Microbiol. 14, 576–588. doi: 10.1038/nrmicro.2016.89
Pearson, J. P., Feldman, M., Iglewski, B. H., and Prince, A. (2000). Pseudomonas aeruginosa cell-to-cell signaling is required for virulence in a model of acute pulmonary infection. Infect. Immun. 68, 4331–4334. doi: 10.1128/IAI.68.7.4331-4334.2000
Pech-Canul, A., Nogales, J., Miranda-Molina, A., Álvarez, L., Geiger, O., Soto, M. J., et al. (2011). FadD is required for utilization of endogenous fatty acids released from membrane lipids. J. Bacteriol. 193, 6295–6304. doi: 10.1128/JB.05450-11
Polkade, A. V., Mantri, S. S., Patwekar, U. J., and Jangid, K. (2016). Quorum-sensing: an under-explored phenomenon in the phylum Actinobacteria. Front. Microbiol. 7:131. doi: 10.3389/fmicb.2016.00131
Põllumaa, L., Alamäe, T., and Mäe, A. (2012). Quorum sensing and expression of virulence in Pectobacteria. Sensors 12, 3327–3349. doi: 10.3390/s120303327
Rahme, L. G., Ausubel, F. M., Cao, H., Drenkard, E., Goumnerov, B. C., Lau, G. W., et al. (2000). Plants and animals share functionally common bacterial virulence factors. Proc. Natl. Acad. Sci. U.S.A. 97, 8815–8821. doi: 10.1073/pnas.97.16.8815
Rahme, L. G., Tan, M. W., Le, L., Wong, S. M., Tompkins, R. G., Calderwood, S. B., et al. (1997). Use of model plant hosts to identify Pseudomonas aeruginosa virulence factors. Proc. Natl. Acad. Sci. U.S.A. 94, 13245–13250. doi: 10.1073/pnas.94.24.13245
Ramos, J. L., Martinez-Bueno, M., Molina-Henares, A. J., Teran, W., Watanabe, K., Zhang, X., et al. (2005). The TetR family of transcriptional repressors. Microbiol. Mol. Biol. Rev. 69, 326–356. doi: 10.1128/MMBR.69.2.326-356.2005
Reichheld, S. E., Yu, Z., and Davidson, A. R. (2009). The induction of folding cooperativity by ligand binding drives the allosteric response of tetracycline repressor. Proc. Natl. Acad. Sci. U.S.A. 106, 22263–22268. doi: 10.1073/pnas.0911566106
Reimmann, C., Ginet, N., Michel, L., Keel, C., Michaix, P., Krishnapillai, V., et al. (2002). Genetically programmed autoinducer destruction reduces virulence gene expression and swarming motility in Pseudomonas aeruginosa PAO1. Microbiology 148, 923–932. doi: 10.1099/00221287-148-4-923
Romero, M., Avendano-Herrera, R., Magarinos, B., Cámara, M., and Otero, A. (2010). Acylhomoserine lactone production and degradation by the fish pathogen Tenacibaculum maritimum, a member of the Cytophaga-Flavobacterium-Bacteroides (CFB) group. FEMS Microbiol. Lett. 304, 131–139. doi: 10.1111/j.1574-6968.2009.01889.x
Rutherford, S. T., and Bassler, B. L. (2012). Bacterial quorum sensing: its role in virulence and possibilities for its control. Cold Spring Harb. Perspect. Med. 2:a012427. doi: 10.1101/cshperspect.a012427
Si, D., Urano, N., Shimizu, S., and Kataoka, M. (2012). LplR, a repressor belonging to the TetR family, regulates expression of the L-pantoyl lactone dehydrogenase gene in Rhodococcus erythropolis. Appl. Environ. Microbiol. 78, 7923–7930. doi: 10.1128/AEM.01583-12
Simon, R., Priefer, U., and Pühler, A. (1983). A broad host range mobilization system for in vivo genetic engineering: transposon mutagenesis in Gram-negative bacteria. Nat. Biotechnol. 1, 784–791. doi: 10.1038/nbt1183-784
Smadja, B., Latour, X., Faure, D., Chevalier, S., Dessaux, Y., and Orange, N. (2004a). Involvement of N-acylhomoserine lactones throughout plant infection by Erwinia carotovora subsp. atroseptica (Pectobacterium atrosepticum). Mol. Plant Microbe. Interact. 17, 1269–1278. doi: 10.1094/MPMI.2004.17.11.1269
Smadja, B., Latour, X., Trigui, S., Burini, J. F., Chevalier, S., and Orange, N. (2004b). Thermodependence of growth and enzymatic activities implicated in pathogenicity of two Erwinia carotovora subspecies (Pectobacterium spp.). Can. J. Microbiol. 50, 19–27. doi: 10.1139/w03-099
Solovyev, V., and Salamov, A. (2011). “Automatic annotation of microbial genomes and metagenomic sequences,” in Metagenomics and Its Applications in Agriculture, Biomedicine and Environmental Studies, ed. R. W. Li (Hauppauge, NY: Nova Science Publishers), 61–78.
Swain, K., Casabon, I., Eltis, L. D., and Mohn, W. W. (2012). Two transporters essential for reassimilation of novel cholate metabolites by Rhodococcus jostii RHA1. J. Bacteriol. 194, 6720–6727. doi: 10.1128/JB.01167-12
Takano, E. (2006). Gamma-butyrolactones: Streptomyces signalling molecules regulating antibiotic production and differentiation. Curr. Opin. Microbiol. 9, 287–294. doi: 10.1016/j.mib.2006.04.003
Trott, O., and Olson, A. J. (2010). AutoDock vina: improving the speed and accuracy of docking with a new scoring function, efficient optimization and multithreading. J. comput. Chem. 31, 455–461. doi: 10.1002/jcc.21334
Uroz, S., Chhabra, S. R., Càmara, M., Williams, P., Oger, P., and Dessaux, Y. (2005). N-acylhomoserine lactone quorum-sensing molecules are modified and degraded by Rhodococcus erythropolis W2 by both amidolytic and novel oxidoreductase activities. Microbiology 151, 3313–3322. doi: 10.1099/mic.0.27961-0
Uroz, S., D’Angelo-Picard, C., Carlier, A., Elasri, M., Sicot, C., Petit, A., et al. (2003). Novel bacteria degrading N-acylhomoserine lactones and their use as quenchers of quorum-sensing-regulated functions of plant-pathogenic bacteria. Microbiology 149, 1981–1989. doi: 10.1099/mic.0.26375-0
Uroz, S., Oger, P. M., Chapelle, E., Adeline, M. T., Faure, D., and Dessaux, Y. (2008). A Rhodococcus qsdA-encoded enzyme defines a novel class of large-spectrum quorum-quenching lactonases. Appl. Environ. Microbiol. 74, 1357–1366. doi: 10.1128/AEM.02014-07
Valente, R. S., Nadal-Jimenez, P., Carvalho, A. F. P., Viera, F. J. D., and Xavier, K. B. (2017). Signal integration in quorum sensing enables cross-species induction of virulence in Pectobacterium wasabiae. mBio 8:e00398-17. doi: 10.1128/mBio.00398-17
van der Geize, R., and Dijkhuizen, L. (2004). Harnessing the catabolic diversity of rhodococci for environmental and biotechnological applications. Curr. Opin. Microbiol. 7, 255–261. doi: 10.1016/j.mib.2004.04.001
von Bodman, S. B., Bauer, W. D., and Coplin, D. L. (2003). Quorum sensing in plant-pathogenic bacteria. Annu. Rev. Phytopathol. 41, 455–482. doi: 10.1146/annurev.phyto.41.052002.095652
Welch, M., Dutton, J. M., Glansdorp, F. G., Thomas, G. L., Smith, D. S., Coulthurst, S. J., et al. (2005). Structure-activity relationships of Erwinia carotovora quorum sensing signaling molecules. Bioorg. Med. Chem. Lett. 15, 4235–4238. doi: 10.1016/j.bmcl.2005.06.066
Yang, Y. H., Lee, T. H., Kim, J. H., Kim, E. J., Joo, H. S., Lee, C. S., et al. (2006). High-throughput detection method of quorum-sensing molecules by colorimetry and its applications. Anal. Biochem. 356, 297–299. doi: 10.1016/j.ab.2006.05.030
Zhang, L., Murphy, P. J., Kerr, A., and Tate, M. E. (1993). Agrobacterium conjugation and gene regulation by N-acyl-L-homoserine lactones. Nature 362, 446–448. doi: 10.1038/362446a0
Keywords: biocontrol, Actinobacterium, Rhodococcus, TetR-like regulator, metabolism
Citation: Barbey C, Chane A, Burini J-F, Maillot O, Merieau A, Gallique M, Beury-Cirou A, Konto-Ghiorghi Y, Feuilloley M, Gobert V and Latour X (2018) A Rhodococcal Transcriptional Regulatory Mechanism Detects the Common Lactone Ring of AHL Quorum-Sensing Signals and Triggers the Quorum-Quenching Response. Front. Microbiol. 9:2800. doi: 10.3389/fmicb.2018.02800
Received: 06 September 2018; Accepted: 31 October 2018;
Published: 19 November 2018.
Edited by:
Essaid Ait Barka, Université de Reims Champagne-Ardenne, FranceReviewed by:
Pol Huedo, Institute of Biotechnology and Biomedicine (IBB), SpainBrett Mellbye, Oregon State University, United States
Copyright © 2018 Barbey, Chane, Burini, Maillot, Merieau, Gallique, Beury-Cirou, Konto-Ghiorghi, Feuilloley, Gobert and Latour. This is an open-access article distributed under the terms of the Creative Commons Attribution License (CC BY). The use, distribution or reproduction in other forums is permitted, provided the original author(s) and the copyright owner(s) are credited and that the original publication in this journal is cited, in accordance with accepted academic practice. No use, distribution or reproduction is permitted which does not comply with these terms.
*Correspondence: Xavier Latour, eGF2aWVyLmxhdG91ckB1bml2LXJvdWVuLmZy
†These authors have contributed equally to this work
‡This article is dedicated to Jean-François Burini, Associate-Professor of the Normandy University and member of the Laboratory of Microbiology Signals and Microenvironment with a special expertise in regulatory mechanisms of bacteria. Jean-François Burini passed away on December 25, 2014 at the age of 56