- Department of Microbial Ecology, Netherlands Institute of Ecology (NIOO-KNAW), Wageningen, Netherlands
Organic fertilizers have been shown to stimulate CH4 uptake from agricultural soils. Managing fertilizer application to maximize this effect and to minimize emission of other greenhouse gasses offers possibilities to increase sustainability of agriculture. To tackle this challenge, we incubated an agricultural soil with different organic amendments (compost, sewage sludge, digestate, cover crop residues mixture), either as single application or in a mixture and subjected it to different soil moisture concentrations using different amounts of organic amendments. GHG fluxes and in vitro CH4 oxidation rates were measured repeatedly, while changes in organic matter and abundance of GHG relevant microbial groups (nitrifiers, denitrifiers, methanotrophs, methanogens) were measured at the end of the incubation. Overall the dynamics of the analyzed GHGs differed significantly. While CO2 and N2O differed considerably between the treatments, CH4 fluxes remained stable. In contrast, in vitro CH4 oxidation showed a clear increase for all amendments over time. CO2 fluxes were mostly dependent on the amount of organic residue that was used, while N2O fluxes were affected more by soil moisture. Several combinations of amendments led to reductions of CO2, CH4, and/or N2O emissions compared to un-amended soil. Most optimal GHG balance was obtained by compost amendments, which resulted in a similar overall GHG balance as compared to the un-amended soil. However, compost is not very nutrient rich potentially leading to lower crop yield when applied as single fertilizer. Hence, the combination of compost with one of the more nutrient rich organic amendments (sewage sludge, digestate) provides a trade-off between maintaining crop yield and minimizing GHG emissions. Additionally, we could observe a strong increase in microbial communities involved in GHG consumption in all amendments, with the strongest increase associated with cover crop residue mixtures. Future research should focus on the interrelation of plants, soil, and microbes and their impact on the global warming potential in relation to applied organic amendments.
Introduction
The atmospheric concentrations of the main GHG carbon dioxide (CO2), methane (CH4), and nitrous oxide (N2O) increased dramatically since the industrial revolution by 40, 150, and 20%, respectively (Stocker et al., 2013). Primarily, anthropogenic activities have increased the emission of CO2, CH4, and N2O. An estimated part of ~50% for CH4 and ~60% for N2O originates from agricultural practices (Tian et al., 2016). Intensification of agricultural land used to meet the global food, feed, and bioenergy demand for the growing human population entails increasing reinvestment of climate neutral carbon compounds (residues) into agricultural systems to prevent decline of soil organic matter and subsequent soil quality and fertility. However, agricultural intensification through increased fertilization can lead to the loss of soil CH4 uptake capacity (Bodelier and Steenbergh, 2014) and additionally causes an enhanced emission of N2O by lowering the reduction of N2O to N2. Particularly, CH4 uptake was 3–9 times weaker in agricultural than in unmanaged soils (Maxfield et al., 2008; Levine et al., 2011; Tate, 2015). Two major groups of fertilizers can be distinguished: organic fertilizer (e.g., compost, manure) and mineral forms (e.g., extracted from minerals or produced industrially) which both have been shown to strongly affect GHG emissions (Hallin et al., 2009; Syakila and Kroeze, 2011; Thangarajan et al., 2013; Shaaban et al., 2016). A common problem of mineral fertilizers is the loss of N and P by leaching (Kramer et al., 2006) and the decreased soil pH by repeated addition of N-fertilizer (Cheng et al., 2015) which by itself can give rise to enhanced N2O emissions (Bakken et al., 2012). Organic amendments represent a more sustainable fertilization strategy as they convey more efficient retention of nitrogen and carbon compounds necessary for plant growth. These organic amendments, like composted cattle manure, biochar, or zeolite addition or crop residue addition can also lower the emission of N2O, or increase its reduction to N2 (Thomson et al., 2012; Thangarajan et al., 2013). However, regarding the GHG related, underlying microbiology under influence of fertilizer applications, knowledge is far from complete.
Recent novel insights led to the postulation that representatives of the newly discovered N2O-reducing clade II can possibly turn soils into sinks of N2O (Jones et al., 2014; Domeignoz-Horta et al., 2015). However, attempts to stimulate soil N2O uptake by inoculation with a non-denitrifying nosZ clade II strain lowered the net potential emission but did not turn the soil into a sink of N2O (Domeignoz-Horta et al., 2016b). While the soil sink function of N2O still has to be verified, CH4 uptake can be found in several soils thereby contributing to cooling side of the GHG balance, representing 6% of the total global methane sink (Kirschke et al., 2013; Tian et al., 2016). However, fertilizer effects on the CH4 sink function in agricultural soils have received far less attention as compared to wetlands and well-aerated non-agricultural soils. This is due the very low or negligible methane uptake capacity in these soils as compared to grassland and upland forest soils (Mosier and Delgado, 1997; Veldkamp et al., 2013; Ciais et al., 2014). By converting natural soils into agricultural soils, up to a 7-fold reduction of CH4 consumption was detected (Levine et al., 2011), taking up to 80 years to recover to pre-land use change levels. It has been demonstrated that the decrease in methane uptake in agricultural soils is due to the destruction of the soil physical structure (e.g., plowing, soil compaction), disrupting the methane gradients in the soil, which are proposed to be crucial for high affinity atmospheric methane oxidation. Next to this other agricultural practices (e.g., fertilization) have been demonstrated to have detrimental effects on atmospheric methane uptake (Bender and Conrad, 1992; Boeckx et al., 1997; Hiltbrunner et al., 2012). However, a recently published study (Ho et al., 2015) demonstrated strongly enhanced methane uptake rates after the addition of different organic amendments (e.g., compost, sewage sludge), to different agricultural soils. The observed rates of uptake were even comparable to the ones from well-aerated forest soils. Shackley et al. observed a similar effect upon addition of biochar which improved the GHG balance by reducing N2O and CH4 emissions from soil (Shackley et al., 2016). These findings are further supported by another study which showed that the use of organic fertilizers (in this case biochar and compost) influence microbial processes which resulted in alterations of soil nutrient cycles thereby affecting agricultural properties (Ye et al., 2016). Furthermore, the addition of plant-derived C compounds from external sources such as biochar or composts can increase soil C availability and may result in higher net CO2 removals from the atmosphere (Paustian et al., 2016) thereby lowering the global warming potential (GWP) (Järveoja et al., 2016). Compared to fresh organic residues, mineralization of compost is slower after addition to soil, leading to a several fold greater mean residence time (Ryals et al., 2015). Ho et al. (2015) postulated that a well-balanced mix of different fertilizers could have a positive effect on GHG balance considering the creation of conditions for methanotrophs to take up atmospheric methane while at the same time keeping carbon dioxide and nitrous oxide emissions to a minimum by providing a greater variety of C- and N-compounds to the microorganisms. However, not all organic fertilizers are suitable to serve this purpose, since in single application only a few organic residues showed the capability to increase soil CH4 uptake and keep CO2 and N2O emissions to a minimum (Ho et al., 2017). However, to develop a strategy to reduce GHG emission from agricultural soils without decreasing crop yield requires understanding of the underlying mechanisms of how organic fertilizers influence GHG. This study aims to answer the following research questions: What is the influence of a combination of organic amendments (compost, digestate, sewage sludge, and cover crop residues) on the GWP of agricultural soils? We hypothesize that methane uptake is stimulated while CO2 and N2O emissions are kept to a minimum compared to un-amended soil by application of mixes of organic amendment and mineral fertilizers. We test these hypotheses by performing soil incubations with various combinations of organic and mineral fertilizers and following GHG dynamics as well as soil chemistry and microbial functional gene abundance.
Materials and Methods
Site Description, Soil Sampling, and Residues
The soil was collected in May 2017 at the research station of Wageningen University in Lelystad, the Netherlands (52°32′26.4′′N, 05°33′34.7′′E) representing a clay soil. The field was planted with onions and left fallow after harvest before sampling. Previously, soil physical-chemical properties have been determined (Ho et al., 2015). The upper 10 cm of the soils was collected in May 2017 from 1 × 1 m using a shovel. The soil was air-dried at room temperature before being sieved (2 mm). The residues included in this study comprised materials with a broad C:N ratio ranging from 4.85 to 22.39 (Table 1) and were selected based on their CH4 uptake performance (compost and sewage sludge) (Ho et al., 2015) or their common usage as bio-based additives in agricultural soil. The residues were air-dried at 30°C, the sewage sludge (S), digestate (D), and the cover crop residues (in the following referred to as CC residues) powder mixture were crushed and ground (< 2 mm) (Jaw Crusher Type BB-1/2, Aartselaar, Belgium). Both composts (C1 and C2) were broken down and sieved (< 6 mm), while the CC residues were cut with a scissor to smaller pieces (< 3–5 cm). Both the dried soils and residues were thoroughly mixed and sieved as per treatment prior to setup of the experiment to ensure standardized initial incubation conditions.
Experimental Setup for in situ GHG Flux Measurements
The soil (200 g dry weight) and residues were mixed with a spoon in a pot and put in an incubation bottle (500 mL volume), deionized water was added to 65 or 40% of soil water holding capacity, respectively. The residue addition to the soil corresponded to a rate of either 20-ton ha−1, which is typically used in agricultural practice (Diacono and Montemurro, 2010), or 5-ton ha−1, which is the maximum amount of cover crop biomass incorporated in agricultural fields in spring. Incubation was performed using three replicates for each treatment in a climate chamber at 15°C (mean annual temperature in the Netherlands is 10°C) in the dark for ~1 month (for 28 days). Water loss, measured by weight, was compensated weekly. Periodically (0, 1, 3, 7, 14, 21, 28 d) methane, nitrous oxide and carbon dioxide fluxes were measured under ambient air by closing the bottles tightly with a lid for 3 h and measuring directly after closing, after 1.5 h and after 3 h. At every time point 20 mL of the headspace was withdrawn and stored in exetainers (5.9 mL) vials (Labco Limited, Lampeter, UK). The first 8 ml of sample was used to flush the exetainer, followed by 12 ml sample introduced into the exetainers creating a 2 bar overpressure. Introduction of the sample (1 ml) into the GC was by an autosampler (TriPlus RSH, Thermo Fisher Scientific, Bleiswijk, The Netherlands) connected to a gas chromatograph (GC1300, Thermo Fisher Scientific) equipped with a Methanizer and a Flame Ionization Detector (FID) to detect CH4 and CO2, an electron capture detector (ECD) for detection of N2O and two sets of a pair Rt-Q-Bond capillary columns (L; 15 m and 30 m, ID; 0.53 mm, Restek, Interscience, Breda, The Netherlands). Helium was used as a carrier gas, and oven temperature was set at 80°C. Five different concentrations of CH4 (0.1, 0.2, 0.6, 1.2, 2 ppm), CO2 (100, 200, 600, 1,200, 2,000 ppm), and N2O (0.05, 0.1, 0.3, 0.6, 1.0 ppm) from a gas mixture (2 ppm CH4, 2,000 ppm CO2, 1 ppm N2O) (Linde AG, Velsen-Noord, The Netherlands) were used as a standard. If higher concentrations of CO2 and N2O were measured, additional single gas calibration gases (Linde AG) of the respective gases (CO2: 4,000 and 10,000 ppm; N2O: 10 and 100 ppm) were used. Chromeleon™ Chromatography Data System 7.1 (CDS, Thermo Fisher Scientific) Software was used to analyse the obtained gas chromatograms from the GC and was used to calculate the standard curves. The gas flux rates were determined by linear regression from the three time points. All fluxes with a R2 < 0.70 were discarded.
Measuring Methane Oxidation and Organic Matter
To determine near atmospheric soil methane emission or uptake under influence of the different amendments after 7, 14, 21, and 28 d, the bottles were closed for 6 days and ~10 ppm CH4 was added to the headspace. CH4 decrease was measured every day in duplicates from each bottle using an Ultra GC gas chromatograph (Interscience, Breda, The Netherlands) equipped with a Flame Ionization Detector (FID) and a Rt-Q-Bond (L; 30 m, ID; 0.32 mm, Restek, Interscience) capillary column. Helium was used as a carrier gas, and oven temperature was set at 80°C. Chromeleon™ Chromatography Data System 7.1 (CDS, Thermo Fisher Scientific) Software was used to analyse the obtained gas chromatograms from the GC.
Sample Storage and Soil Organic Matter Measurements
After finishing the incubation ~10 g of soil samples were stored at −20°C for later DNA extractions. Another ~50 g of soil was dried at 30°C and stored for soil nutrient determination. To measure the soil organic matter content after incubation, 10–15 g of soil was dried in a porcelain cup at 105°C for 1 day. Afterwards, the dried sample was burned in an oven at 430°C for another day, both times the sample was weighed. To calculate the organic matter content per g 100 g−1 dry soil the following formula was used: 100* (g dry soil—g ashed soil)/g dry soil.
DNA Extraction and qPCR Assays
DNA was extracted using the DNeasy PowerSoil Kit (Qiagen, Venlo, The Netherlands) according to manufacturer's instruction. We performed qPCR assays targeting amoA for ammonium oxidizing Achaea (AOA) and bacteria (AOB), nifH (N-fixers), nosZ clade I/II (denitrifiers), mcrA (methanogens), pmoA (methane oxidizers), 16S rRNA gene for Archaea and Bacteria as well as the 18S rRNA gene of fungi. Each assay was performed in duplicate for each DNA extract with primers, primer concentration, and PCR profiles as shown in Supplementary Table 1. Briefly, each qPCR (total volume 20 μl) for all assays consisted of 10 μl 2 × SensiFAST SYBR (BIOLINE, Alphen aan den Rijn, The Netherlands), 1 μl of forward and reverse primers each (10 pmol μl−1; Sigma-Alderich, Zwijndrecht, The Netherlands), 1 μl bovine serum albumin (5 μg μl−1; Invitrogen, Breda, The Netherlands), 4.5 μl DNase- and RNase-free water and 2.5 μl diluted template DNA. The qPCR for the EUBAC(bacterial 16S rRNA gene) assay (total volume 15 μl) consisted of 7.5 μl 2 × SensiFAST SYBR (BIOLINE), 0.75 μl of forward and reverse primers each (5 pmol μl−1; Sigma-Alderich), 1.5 μl bovine serum albumin (5 μg μl−1; Invitrogen), 1.5 μl DNase- and RNase-free water, and 3 μl diluted template DNA. Standard curves were obtained using serial 10-fold dilutions of a known amount of plasmid DNA from different pure cultures representing the target gene fragment (108–101 gene copies) containing the respective gene fragment. The qPCR was performed with an iCycler IQ5 (Applied Biosystem, Carlsbad, CA, USA). Negative controls were always run with water instead of template DNA. PCR reactions were done with 1:20 and 1:60 diluted DNA extracts. Amplification efficiencies for all assays were between 79 and 98% with R2 values between 0.969 and 0.995. Amplicon specificity was inferred from the melt curve.
Statistical Analyses of Collected Data
All statistical analyses were done using R version 3.0.1 (R Development Core Team, 2013). The mean total GHG fluxes, the GWP, the organic matter loss and abundance of the different functional marker genes were tested for normality by Kolmogorov–Smirnov test and for homogeneity of variance by Levene's test. If necessary, normal distribution was achieved by log-transformation of the data. Treatment effects and differences between means were assessed using one-way ANOVA followed by Tukey's post-hoc test. All levels of significance were defined at P < 0.05.
Results
GHG Flux Measurements
The fluxes of the three major GHG (CH4, CO2, N2O) from the soils amended with the organic amendments were measured continuously through the experiment at different level of SM and different applied concentrations of organic amendments. An overview about values of the different GHG as well as the calculated GWP100yr for the different samples is shown in Table 2.
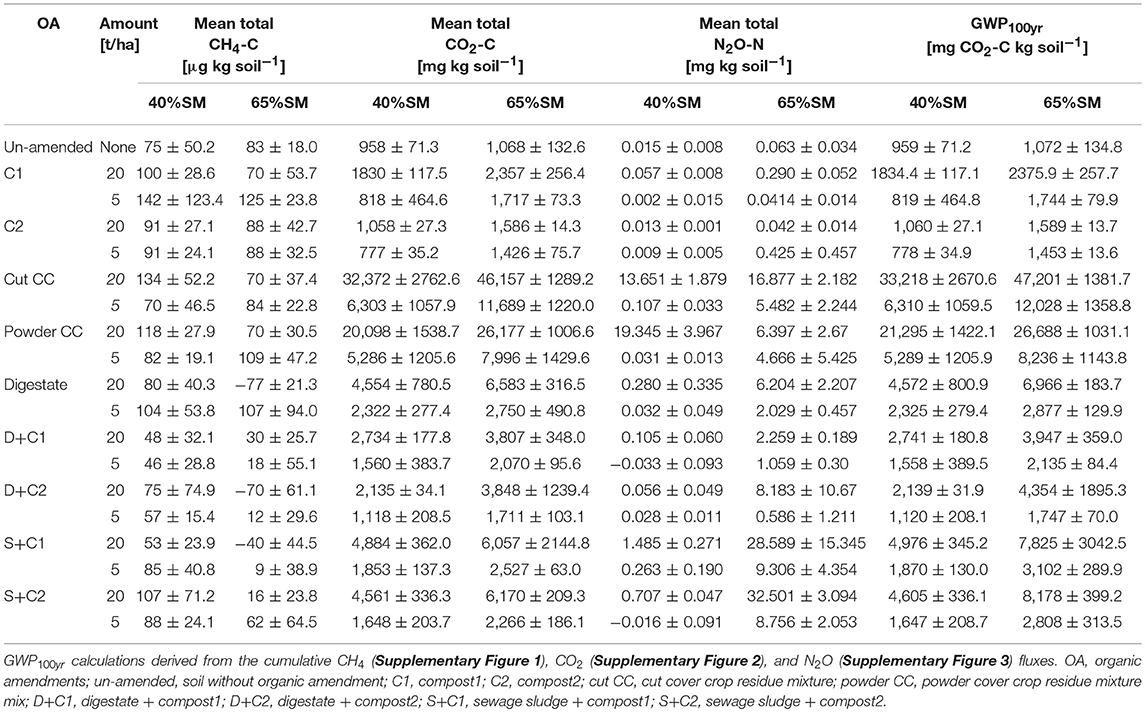
Table 2. Overview of mean total CH4. CO2, N2O, and calculated GWP100yr values of the different organic amendments, amounts, and soil moisture concentration that were used.
CH4
The CH4 flux measurements under 65% SM (Supplementary Figures 1A,B) showed variation over time considering uptake or emission of CH4. Both amounts of organic amendments applied (5 and 20 t/ha) led to similar fluxes during the incubation without fluctuation. However, total CH4 fluxes (Figures 1A,B) varied between treatments, mostly releasing CH4 over time irrespective of the amount of organic amendment used. Only three amendments (digestate, D + C2, S + C1 at 20 t/ha) led to increased methane uptake. Under 40% SM, minor fluctuations in CH4 fluxes over time were detected with both organic amendment amounts (Supplementary Figures 1C,D). Calculated mean cumulative CH4 fluxes (Figures 1C,D) demonstrated that all samples emitted CH4 during the incubation.
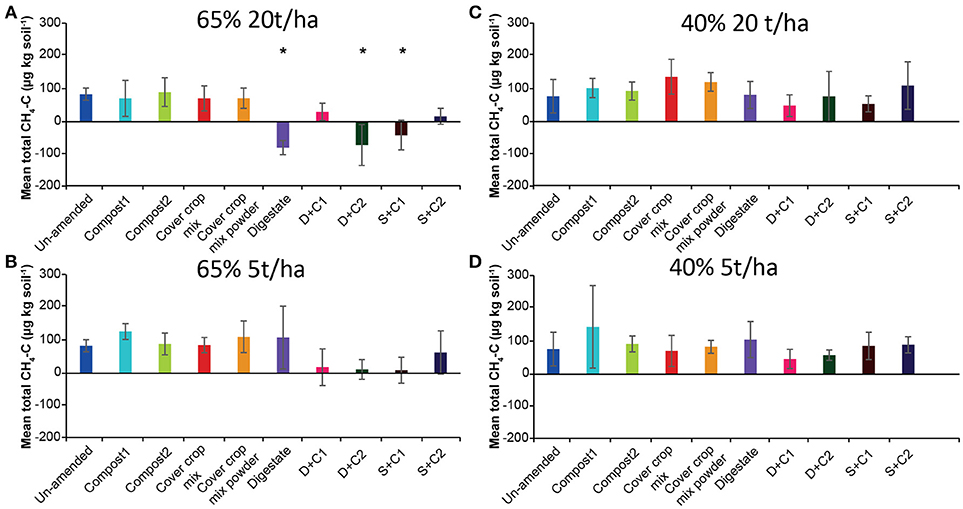
Figure 1. Mean total CH4 emitted or consumed over the period of 28 d in un-amended clay soil and after amendments with compost1, compost2, CC residues mixtures, digestate, digestate+compost1, digestate+compost2, sewage sludge+compost1, and sewage sludge+compost2 (mean ± SD; n = 3) at (A) high amount (20 t/ha) and high water content (65%), (B) low amount (5 t/ha) and high water content, (C) high amount and low water content (40%), and (D) low amount and low water content, derived from the cumulative CH4 (Supplementary Figure 1) fluxes. Asterisk (*) indicate significant differences in the mean total CH4 fluxes between the soils with organic amendments and the un-amended soil within the four separate superordinate treatments (ANOVA: P < 0.05).
CO2
Measured CO2 fluxes under 65% SM (Supplementary Figures 2A,B) showed the same trends, irrespective of the amounts of organic amendment applied. Highest CO2 fluxes were observed for cut and powdered cover crop residues, respectively, followed by digestate and the sewage sludge + compost 2 combination. Independent of the amount applied, cut as well as powdered CC residues continuously released CO2 over the complete incubation. Both types of compost led to the lowest CO2 fluxes among the organic amendments used and were comparable or lower than the CO2 fluxes of the un-amended soil. The mean cumulative CO2 fluxes (Figures 2A,B) reflect the dynamics of the CO2 fluxes over time and treatments (Supplementary Figures 2A,B). Highest CO2 emissions were observed for cut CC residue material, followed by powdered CC residue, digestate, and the sewage sludge amendments. This was true for both tested amounts. Highest CO2 fluxes under 40% SM were always observed for cut CC residue material followed by powdered CC residues, digestate and the two sewage sludge treatments (Supplementary Figures 2C,D). While high amounts of CC residues showed emission of CO2 over the whole incubation period, no emissions were detected after 21 d with low amounts. Similarly, cumulative CO2 fluxes (Figures 2C,D) were always lower with lower amounts of organic amendments, the extent of which differed between the type of organic amendment. While both cover crop residue treatments were 4- to 5-fold higher, all other organic amendments were only 1.4- to 2.7-fold higher when 20t/ha was applied.
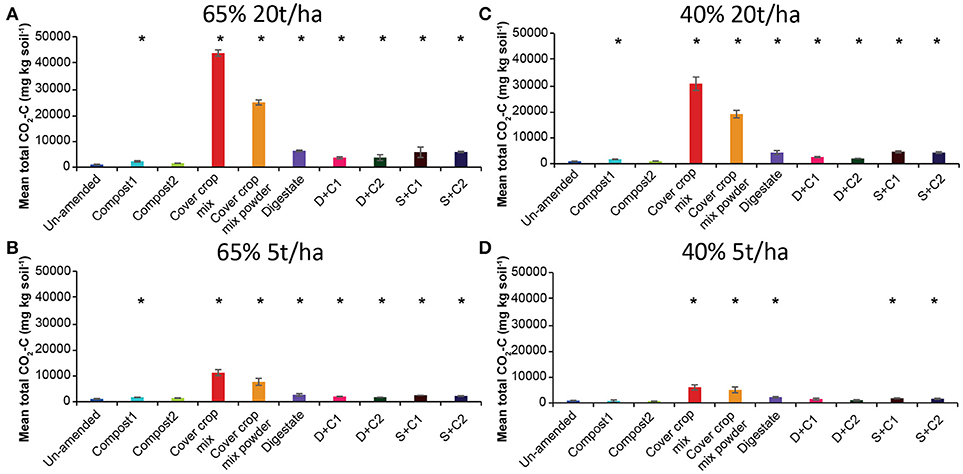
Figure 2. Mean total CO2 emitted over the period of 28 d in un-amended clay soil and after amendments with compost1, compost2, CC residues mixtures, digestate, digestate+compost1, digestate+compost2, sewage sludge+compost1, and sewage sludge+compost2 (mean ± SD; n = 3) at (A) high amount (20 t/ha) and high water content (65%), (B) low amount (5 t/ha) and high water content, (C) high amount and low water content (40%), and (D) low amount and low water content, derived from the cumulative CO2 (Supplementary Figure 2) fluxes. Asterisk (*) indicate significant differences in the mean total CO2 fluxes between the soils with organic amendments and the un-amended soil within the four separate superordinate treatments (ANOVA: P < 0.05).
Lower SM always lead to lower CO2 fluxes when same amounts organic amendments were applied.
N2O
Both sewage sludge combinations showed the highest N2O flux rates at 65% SM, regardless of the applied amounts of organic amendments, followed by digestate and cut CC residue material (Supplementary Figures 3A,B). Both composts, as well as the un-amended soil, showed almost no N2O fluxes. In general, 20 t/ha led to higher overall measurable N2O fluxes. These findings are also underlined by the cumulative N2O fluxes (Figure 3). The N2O fluxes of both sewage sludge combination, digestate, digestate + compost 1, and both CC residue mixtures were 2- to 4-fold lower with 5 t/ha. The digestate + compost 2 amendment showed a 13-fold reduction, while the un-amended and both single compost applications did not lead to any N2O emission at all. After 14 d of incubation both combinations of digestate with compost at an application rate of 5 t/ha resulted in lower N2O emissions.
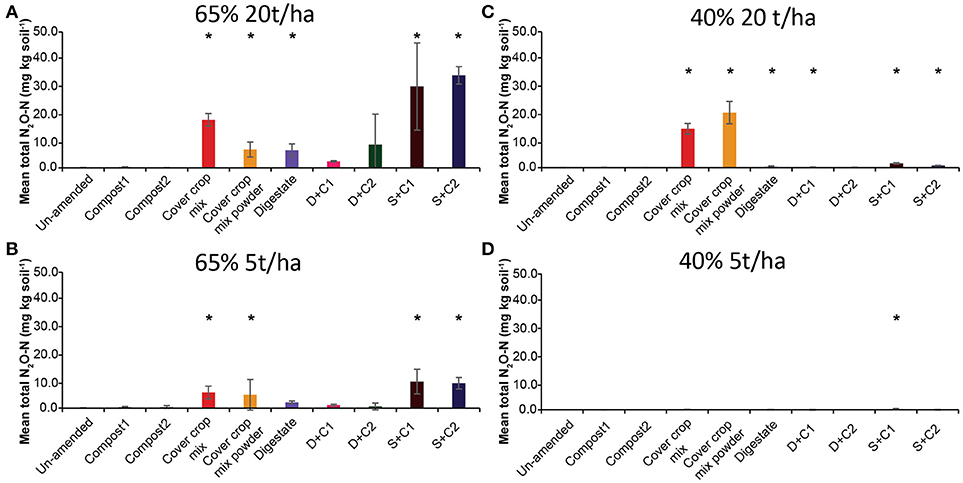
Figure 3. Mean total N2O emitted over the period of 28 d in un-amended clay soil and after amendments with compost1, compost2, CC residues mixtures, digestate, digestate+compost1, digestate+compost2, sewage sludge+compost1, and sewage sludge+compost2 (mean ± SD; n = 3) at (A) high amount (20 t/ha) and high water content (65%), (B) low amount (5 t/ha) and high water content, (C) high amount and low water content (40%), and (D) low amount and low water content, derived from the cumulative N2O (Supplementary Figure 3) fluxes. Asterisk (*) indicate significant differences in the mean total N2O fluxes between the soils with organic amendments and the un-amended soil within the four separate superordinate treatments (ANOVA: P < 0.05).
Only low N2O emissions were detected at 40% SM (Supplementary Figures 3C,D). All organic amendments applied at a rate of 5 t/ha showed no N2O emissions during the complete incubation period while at 20 t/ha only small amounts of N2O were released in the first 14 d of incubation. After 14 d both CC residue amendments (cut and powdered) showed a rapid increase in N2O emissions, which peaked at day 21. After 28 d the cut CC residues still released N2O from the soil, while the powdered CC residue enabled soil N2O uptake from this point onward.
GWP Analyses
We derived the GWP in mg CO2 equivalent per kg soil by combining the cumulative CH4, CO2, and N2O flux (Supplementary Figures 1–3). In these calculations, the GWP value for CH4 and N2O are considered to be 28 and 265, respectively over a hundred- year time frame, while the GWP value for CO2 is considered to be 1 (IPCC, 2014).
The GWP values showed similar trends as the cumulative CO2 fluxes, irrespective of the SM and amount of organic amendment (Figure 4). Notably, compost1 and 2 treatments led to lower GWP as compared to un-amended soil with low amounts applied under 40% SM (Figure 4).
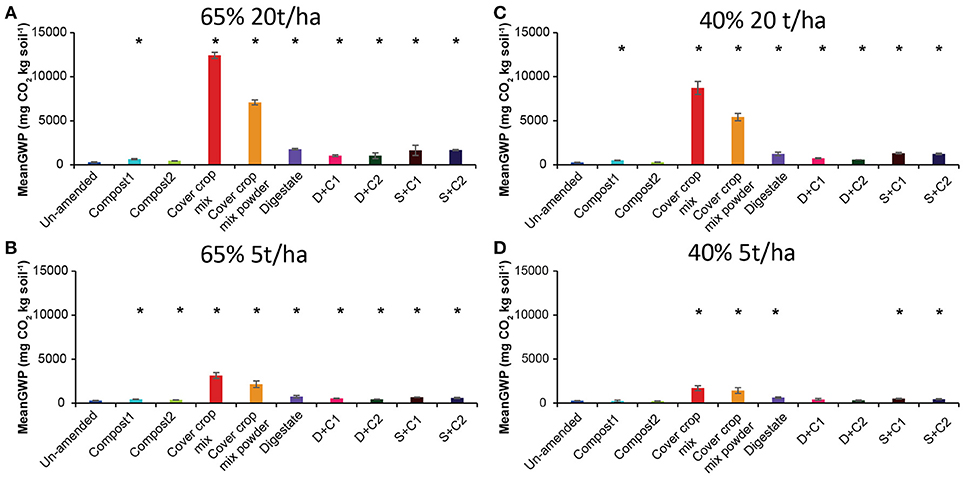
Figure 4. Mean global warming potential (GWP) over the period of 28 d in un-amended clay soil and after amendments with compost1, compost2, CC residues mixtures, digestate, digestate+compost1, digestate+compost2, sewage sludge+compost1, and sewage sludge+compost2 (mean ± SD; n = 3) at (A) high amount (20 t/ha) and high water content (65%), (B) low amount (5 t/ha) and high water content, (C) high amount and low water content (40%), and (D) low amount and low water content, derived from the cumulative CH4 (Supplementary Figure 1), CO2 (Supplementary Figure 2), and N2O (Supplementary Figure 3) fluxes. Asterisk (*) indicate significant differences in the GWP between the soils with organic amendments and the un-amended soil within the four separate superordinate treatments (ANOVA: P < 0.05).
CH4 Fluxes After Addition of 10 ppm CH4
CH4 fluxes after the addition of 10 ppm CH4 at multiple times, did not differ significantly between the four major treatments irrespective of SM and organic amendment rate applied (Supplementary Figure 4). The fluxes in most cases vary between 0 and −0.003 μmol m−2 min−1, which can be referred to as CH4 uptake. At the last sampling point the amendment with compost2 at 40% SM and 5 t/ha increased to an uptake of −0.008 μmol m−2 min−1, which was the highest uptake measured. However, most organic amendments improve their CH4 uptake over time.
Organic Matter
When low amounts of organic amendment are applied at 65% SM, the organic matter loss is constant through all treatments ranging from −0.4 to −0.6% loss of the original OM content which was around 2.5–3% (Figure 5). At high concentration of organic amendments the loss of OM is lower being around −0.4% with exception of the cut CC residue amendment, resulting in 1.4% loss in organic matter. In general incubations at 40% SM lost more organic matter than their counterpart at 65% SM (Figure 5). The lowest losses were observed for digestate, compost1, and D+C1 with a loss of ~-0.55%. These organic amendments are followed by compost2, D+C2, S+C1, and S+C2 with a loss of −0.8 to −1.0% organic matter content. The highest loss could be observed for cut and powdered CC residue mixture with −1.2 and −1.4%, respectively.
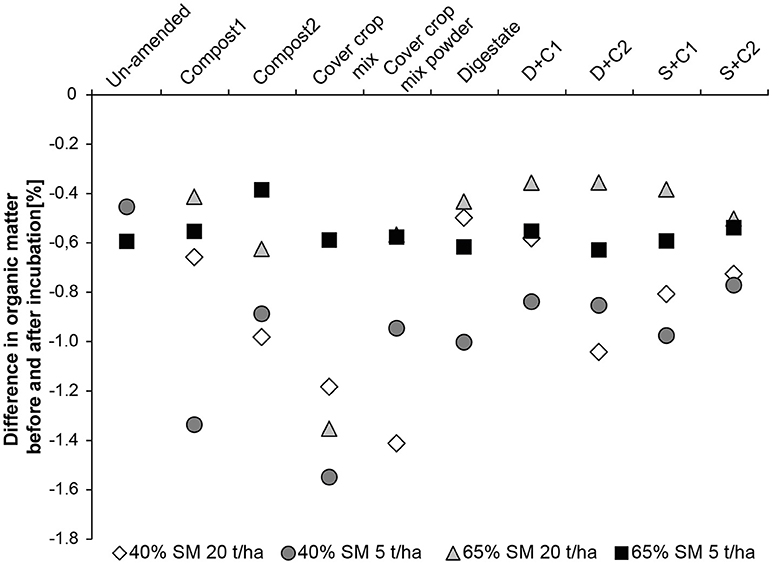
Figure 5. Loss in organic matter content during the incubation period of 28 d in un-amended clay soil and during amendments with compost1, compost2, CC residues mixtures, digestate, digestate+compost1, digestate+compost2, sewage sludge+compost1, and sewage sludge+compost2 (mean ± SD; n = 3) at () high amount (20 t/ha) and high water content (65%), (■) low amount (5 t/ha) and high water content, (♢) high amount and low water content (40%), and (
) low amount and low water content.
Abundance Analyses of Microbial Groups
To assess changes in the abundance of the microbial communities, the ratio was calculated between gene copy numbers of the analyzed genes in the initial soil and at the end of the incubation. The individual gene copy numbers of all samples analyzed can be found in Supplementary Tables 2, 3.
The overall bacterial abundance stayed either stable or increased over time (Figure 6A), with high amounts of CC residues leading to the highest stimulation in abundance (4- to 7-fold). All other organic amendments at high application rate led to at least to a doubling of bacterial numbers, while numbers in the un-amended remained constant. When applying low amounts of organic amendments, microbial abundances did not change in any of the treatments.
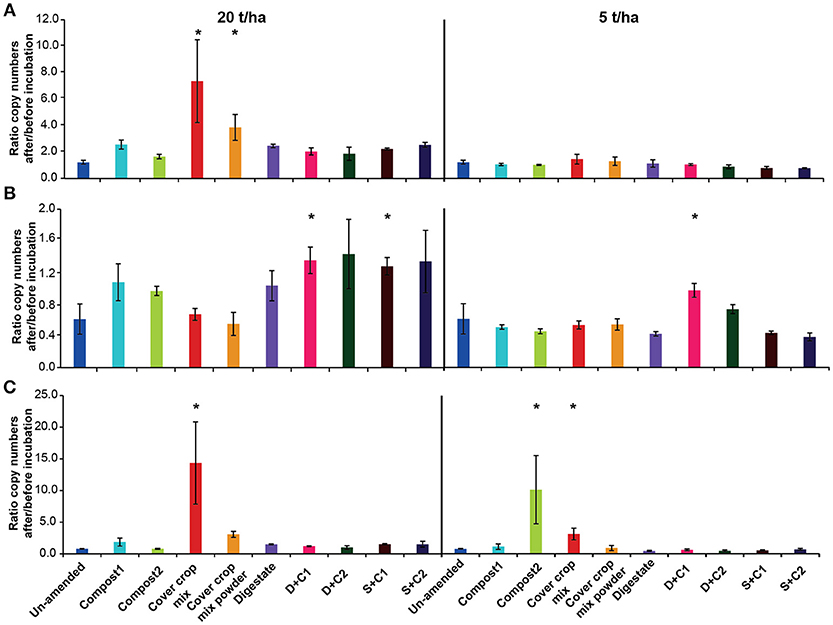
Figure 6. Ratio of the copy numbers of (A) bacterial 16S rRNA gene, (B) archaeal 16S rRNA gene, and (C) fungal 18S rRNA gene after and before an incubation of un-amended clay soil and during amendments with compost1, compost2, CC residues mixtures, digestate, digestate+compost1, digestate+compost2, sewage sludge+compost1 and sewage sludge+compost2 (mean ± SD; n = 3) for 28 d. Asterisk (*) indicate significant differences in the ratio of the individual genes in the soils with organic amendments and the un-amended soil within the four separate superordinate treatments (ANOVA: P < 0.05).
In contrast to the bacterial abundance, archaea communities either remained stable or decreased over the time (Figure 6B). Typically, all digestate combinations, both composts and sewage sludge combinations at high application rate did not lead to change in archaeal abundance, while it decreased in all other treatments.
Overall, fungal abundance was rather constant during the incubation (Figure 6C). However, the cut CC residue mixture led to a 15- and 5-fold increase in fungal abundance at high and low organic amendment application rate, respectively while the 20 t/ha powdered CC residue treatment increased around 3-fold. Compost 2 at low application led a 10-fold in increase. All other treatments at high application rate did not lead to change in fungal abundance.
For most of the functional marker genes there was no change in the un-amended soil, except for a decrease of AOAs and a doubling of nosZ clade II (Figure 7).
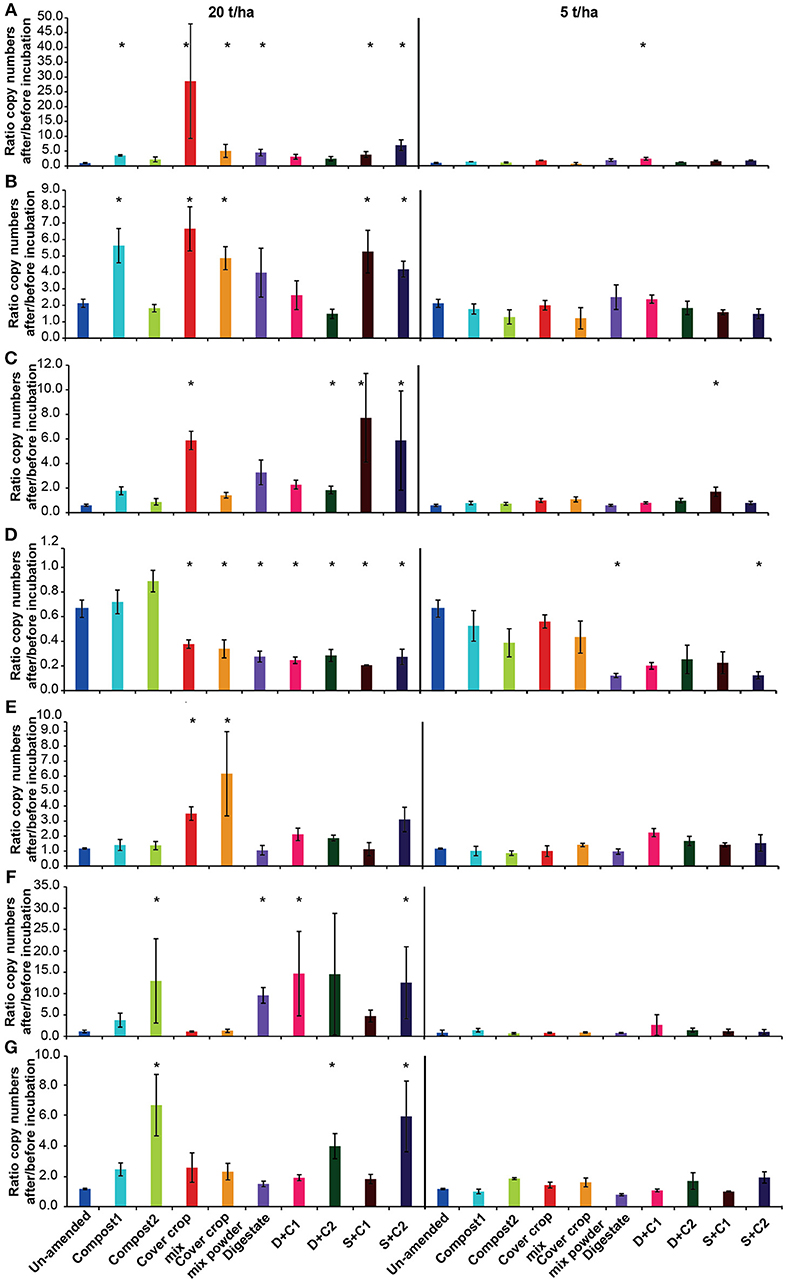
Figure 7. Ratio of the copy numbers of the functional marker genes (A) nosZ clade I, (B) nosZ clade II, (C) bacterial amoA, (D) archaeal amoA, (E) nifH, (F) mcrA, and (G) pmoA after and before an incubation of un-amended clay soil and during amendments with compost1, compost2, CC residues mixtures, digestate, digestate+compost1, digestate+compost2, sewage sludge+compost1, and sewage sludge+compost2 (mean ± SD; n = 3) for 28 d. Asterisk (*) indicate significant differences in the ratio of the individual genes in the soils with organic amendments and the un-amended soil within the four separate superordinate treatments (ANOVA: P < 0.05).
Both nosZ clades showed an increase in abundance, in all organic amendment-treatments, irrespective of the application rate (Figures 7A,B). While the two clades with low amendments increased mainly between 1.2- and 2.5-fold, a 2- to 7-fold increase was observed with 20 t/ha. The highest increase occurred in the incubation with cut CC residue material with 28-fold in nosZ clade I. In general, the nosZ clade II was 10- to 100-fold more abundant than nosZ clade I (Supplementary Table 2).
At low application rates organic amendments had no effect on the bacterial amoA abundance (Figure 7C). At high concentrations, the cut CC residue, both sewage sludge combinations and all treatments with digestate lead to an increase in bacterial amoA of 2- to 8-fold (Figure 7C).
In contrast to the abundance of the bacterial amoA, archaeal amoA abundance decreased in all organic amendment-treatments (Figure 7D). The strongest decrease was observed for the digestate and sewage sludge combinations with both composts, which decreased 3- to 4-fold in both applied concentrations. In all compost, CC residue and digestate amendments AOA gene copy numbers were 2- to 10-fold higher than for AOBs. This is contrast with the sewage sludge treatments, which at low amendment led to higher numbers of AOA, whereas AOBs showed a 2- to 4-fold higher abundance at high organic amendment (Supplementary Table 2).
The abundance of N-fixers in the cut and powdered CC residue mixture increased in the application with 20 t/ha by 3- and 6-fold, respectively (Figure 7E). The only other treatment with a positive effect on the abundance of nifH was the sewage sludge + compost 2 amendment, which showed an increase of ~3-fold.
The methanogenic abundance did not changed for both cover crop treatments, but increased 3-fold for compost1, 5-fold for sewage sludge+compost1 and between 10- and 14-fold for the remaining organic amendments at high rates of application while at low rates mcrA gene abundance stayed stable (Figure 7F).
Gene copy number of methanotrophs (pmoA) increased for all samples with 20 t/ha, except in the digestate amendment, in which no differences to un-amended soil were reported (Figure 7G). The compost2 amendment and the combination with compost2 showed the strongest effect on the copy numbers with a 4- to 6-fold increase. Low organic amendment application rates only showed minor positive effects on the abundance of methanotrophs.
The abundance of the two CC residue amendments at low SM and high organic amendment application behaved very similar for all analyzed genes (Supplementary Table 4). The abundance of the archaeal 16S rRNA gene and archaeal amoA dropped by 2-fold, while it stayed stable for nifH, mcrA, and pmoA. A 5-fold increase was observed for the fungal 18S rRNA gene and nosZ clade I for the cut CC residues, while the powder led to a 3- and 2-fold increase, respectively. nosZ clade II numbers increase for both CC residue materials around 3-fold. While the cut CC residue material resulted in a 2-fold increase for the bacterial 16S rRNA gene and the bacterial amoA, the powdered CC residue material did not show a change for these two genes.
Discussion
In this study, we investigated the influence of combinations of organic amendments on the GHG balance and the CH4 uptake as well as on dynamics of different soil microbial groups that are involved in producing or reducing GHGs in agricultural soil. Several combinations of amendments led to reductions of CO2, CH4, and/or N2O emissions compared to un-amended soil. Most optimal GHG balance was obtained by compost amendments, which resulted in similar overall GHG balance as compared to the un-amended soil. Additionally, we could observe a strong increase in microbial communities involved in GHG consumption in all amendments, with the strongest increase associated with cover crop residue mixtures.
GHG Dynamics and GWP in Relation to Different Organic Amendments and Manipulation of Soil Moisture
CH4
We did not observe significant uptake of CH4 in any of our samples except for digestate (D), D+C2, S+C1 at high SM and high application rate, which led to CH4 uptake over the complete incubation period (Figure 1). However, the in vitro methane uptake capacity at near atmospheric (i.e., 10 ppm) methane concentrations increased in all samples over time. As proposed by Ho et al. (2015), it seems that the methanotrophic community needs elevated methane to gear up the enzyme machinery. A similar result was found in rice soils where high methane concentration spikes were necessary to induce atmospheric methane uptake (Cai et al., 2016). Especially the 5 t/ha compost2 treatment under 40% SM showed a very strong improvement in CH4 uptake at the end of the incubation. Potentially, the release of rare earth metals (e.g., La, Ce, Nd), which are stored in the compost (La ~2.2 μg g−1; Ce ~3.8 μg g−1; Nd ~2.2 μg g−1; El-Ramady, 2011) stimulated the CH4 uptake (Keltjens et al., 2014). Recent studies found that for some methanotrophs rare earth metals are essential as cofactors in the active center of an alternative methanol dehydrogenase (Keltjens et al., 2014; Pol et al., 2014; Shiller et al., 2017). Furthermore, it was shown that the La-dependent methanol dehydrogenase can also be more efficient hydrolytic catalysts because they are stronger Lewis acids (electrophilic electron acceptor) than the Ca dependent one (Lim and Franklin, 2004). This La-dependent methanol dehydrogenase which can also be found in the newly isolated atmospheric CH4 oxidizer belonging to the USCα cluster (Pratscher et al., 2018). However, all studies with rare earth metals and their effect on CH4 oxidation were performed in liquid cultures. Another possible explanation for the increase in CH4 oxidation rates at the end of the incubation in compost2 incubations, could be its relatively low C- and N-content in comparison to the other organic amendments. This could lead to higher amounts of essential substrates (O2) or lower amounts of inhibiting compounds (e.g., NH) for methane oxidation (Conrad and Rothfuss, 1991; Bender and Conrad, 1992; Malyan et al., 2016). In contrast, the higher amount of C- and N-compounds in the other organic amendments could result in a reduced or delayed start of CH4 oxidation. Furthermore, it is known that compost could lead to an increase in the soil's cation binding capacity (Epstein et al., 1976), leading to lowering of the availability of ammonium ions, potentially inhibiting the particulate methane monooxygenase (Singh and Seneviratne, 2017).
CO2
The first addition of water induced a direct emission of CO2 from the soil in samples with organic amendments. The extend of these CO2 emissions is strongly dependent on the amendment used. The lowest CO2 emissions were obtained with both compost amendments, showing similar values as the un-amended soil, where the fungal based compost emitted less CO2 than the compost from green cut materials (Table 2). The reason for this could be a low total C concentration together with not easily degradable C-compounds (Ryals et al., 2015). Based on this it seems that the different material of the compost can contribute better or worse to reducing GHG, which would need further analyses.
The decrease respiration in organic matter added through the experiment did not correlate with most of the CO2 fluxes. Only the CO2 fluxes under moist conditions (R2 = 0.633) and high amount of organic amendment (R2 = 0.783) correlated with the decrease of organic matter. This is in accordance with our previous study (Ho et al., 2017), demonstrating that C:N alone is not a good predictor of amendment effects on GHG fluxes. In this study the organic amendment with the highest C:N ratio was the fungal based compost which showed the lowest measurable CO2 fluxes of all organic amendments. However, the highest measured CO2 fluxes were emitted by both CC residue mixtures which indeed have the second highest C:N ratio. We observed a correlation between the total C concentration measured in the organic amendments and the CO2 fluxes. The quality and composition of the amendments, seem to be more important for influencing the CO2 fluxes. For example the sewage sludge+compost2 amendment has the same total C-content as compost1, but emitted 4-fold higher CO2 fluxes. In accordance with this, digestate has a lower total C concentration compared to CC residue material, but emitted 15-fold less CO2. One explanation is that the digestate is not as easily degradable as the plant material for the microorganisms, since its origin is already anaerobically digestaed manure. It was already shown that CO2 respiration from digestate is highly dependent on the initial source from which the digestate is produced, which led to a broad range of CO2 respiration rates (Alburquerque et al., 2012). According to our results, this statement can be extended to a variety of organic amendments.
Surprisingly, we saw a second peak of increased CO2 emission after 21 d in almost all treatments. This may be explained by the fact after 14 d substrates which are more difficult to degrade are reduced to a more accessible form of shorter chain molecules. Succession in microbial community composition may be involved which can take place in just a short period of time (13–15 d) following amendment with organic residues as shown by Ho et al. (2017). Additionally, changes in soil parameters (e.g., O2 availability, N availability) may cause a second phase of CO2 respiration due to alleviation of initial limitations.
N2O
Surprisingly, the highest N2O fluxes were not observed from the N richest organic amendment (digestate), but from the combinations of sewage sludge with compost, followed by the CC residue mixtures (Table 2). Hence, the N2O emission is not only depending on the N-content of the organic amendments, but also in which form the N-source is provided to the microorganisms. These observations are similar to our findings of the relatively weak correlation between C-content and CH4/CO2 fluxes. Additionally, we could not find any correlation of C/N or C-content to N2O fluxes (data not shown). Contrary to a recent study we also did not observe a linear relation between N fertilization and N2O emission (Shcherbak et al., 2014) in a study where all soil and environmental parameters were kept stable.
Only in case of the high organic amendment application we observed a second N2O flux peak after 21 d of incubation. In these incubations, the existing input of fresh N through the organic amendments was probably already processed and either turned into gaseous N, microbial N, or remains in refractory form. The microbial biomass or refractory N may release ammonium by mineralization, but this may take more time explaining the temporal pattern observed. Another explanation maybe that the soil parameters changed and stimulated the production of N2O again (e.g., through more anoxic zones). The results of the abundance analyses from these samples (Supplementary Table 3) revealed a strong increase of fungi in these samples, which could be causing the observed N2O production in our incubations. Fungi are known for possessing denitrification genes to produce N2O, but as yet have not been demonstrated to harbor N2O-reductase gene (Takaya, 2002; Shoun et al., 2012). It was also shown that denitrifying fungi already prefer drier conditions than denitrifying Bacteria (Chen et al., 2015). Additionally, since a SM of 40% normally does not favor denitrification processes (Skiba et al., 2002; Bateman and Baggs, 2005), changes in soil structure or chemistry (e.g., pH, O2 availability, aggregate composition) could have occurred leading to “hotspots” of N2O production as proposed to be responsible for local, temporary high denitrification activity (Groffman et al., 2009).
The water content has a more pronounced influence on the N2O emission than on the CH4 and CO2 fluxes. At low SM almost no N2O emission was detected. Since high SM reduces O2 availability and gas diffusivity and therefore will favor denitrification (Skiba et al., 2002), it can be assumed that in our incubation denitrification processes are the main source of N2O production. It was already observed in other studies that an increasing SM led to an increase of N2O production by denitrification (peak above 65% water-filled pore space), since the optimal SM concentration for nitrification peaks at around 55–65% water-filled pore space (Bateman and Baggs, 2005; Vargas et al., 2014; Sanz-Cobena et al., 2016). Contrary to this, the high amount of CC residue mixtures showed a strong increase in N2O emission at a low SM (Figure 4) just after 15 d. Even more surprising was the uptake of N2O after 28 d for the powdered CC residue mixture. This can either be caused by the high concentrations of N2O stimulating N2O reducers, or by a change in the soil characteristics (e.g., pH, O2 availability). Growth of fungi, which occurred in the CC residue bottles after some days of incubation, could also increase production of N2O activating the N2O-reducing community in the soil. It was shown recently that through application of plant residues, hotspots of N2O emission can occur, by enhanced water absorption from the plant residues which will lead to reduced O2 concentrations in the surrounding (Kravchenko et al., 2017). Combined with mineralized N and fungal growth this could explain the N2O peak caused by CC residues. To our knowledge this is the first time that such a behavior of N2O emission/consumption was observed after applying crop residues to the soil. More studies that confirm these results need to be conducted in the future.
Abundance of Microorganisms in Relation to GHG Fluxes and Organic Amendment Application
Microbial dynamics following application of organic amendments clearly offers scope for modulating functional groups involved in consumption of GHGs. In this light, the CC residues materials showed the best results, by increasing the abundance of the denitrifiers (nosZ), methanotrophs (pmoA), and nitrogen fixers (nifH) genes, while only moderately increasing the nitrifiers (AOB) and methanogens (mcrA). This could be either through the introduction of microbes already present in the organic amendments or stimulation of growth from indigenous microorganisms harboring these genes. Here, the effect is highly related to the amount of organic amendment applied to the soil. Small amounts of organic amendments have only a minor effect on the different microbial groups, which is also in accordance with the distinct lower GHG flux measurements from these incubations. On the opposite site, organic amendments cannot only increase the gene copy numbers, but can also lead to a decrease of microbial groups (AOA) in comparison to an un-amended soil.
The overall bacteria and fungi abundance correlate quite well with the CO2 respiration rates (R2 = 0.942/R2 = 0.858, respectively). The strong increase, especially in the CC residue application in bacterial and fungal abundance, could mainly occur due to the high application rate of the CC residue in our experiment. Normally, around 4- to 6-fold lower amounts of CC residues are plowed under in the field after the winter (Marinari et al., 2015; Coombs et al., 2017). However, we observe also an increase in the fungal abundance at the low amount of applied CC residues, which is comparable to recent studies (Maul et al., 2014).
The differences in abundance of the different groups are highly influenced by the different organic amendments that are used. For example, the application with the fungi based compost has a great effect (7-fold increase) on the abundance of the methanotrophs, compared to the green cut compost material which (like the other organic amendments) had only a doubling effect on the abundance of methanotrophs. Like mentioned before, a stimulation of rare-earth metal-dependent methanotrophs, which harbor the XOXF dependent methanol dehydrogenase gene, in these samples could be a possible explanation (Gu and Semrau, 2017; Krause et al., 2017). However, in a previous study (Ho et al., 2015) USCα pmoA sequences, which are known to poses the XOXF enzyme and is capable of atmospheric CH4 oxidation, was not detected in soil samples from the same location. This would rather support the hypotheses that the increase in pmoA copies is due to the introduction of methanotrophs by the organic amendment.
In contrast to the methanotrophic community, we observe more distinct differences of the effect of organic amendments on the methanogenic abundance. Especially organic amendments (compost and digestate) that undergo a treatment in which anoxic habitats are formed to provide a perfect environment for methanogens (Hellmann et al., 1997; Alburquerque et al., 2012). Especially, CC residue amendment increased the ratio of methanotrophs to methanogens, which can harbor a positive effect on the ratio of CH4 consumption to CH4 production (Conrad, 2007).
In our soil the newly found nosZ clade II (Jones et al., 2013) is 10- to 100-fold more abundant than nosZ clade I. While clade I is mainly associated with soil type (clay), nutrient status, total organic carbon, organic matter or C:N ratio, it is unclear what the drivers for the abundance of clade II in soils are (Highton et al., 2016; Hallin et al., 2017). Our soil is a clay soil, which would be expected to show a higher correlation to nosZ clade I bacteria, but instead we see a clear preference of N2O-reducers with a nosZ clade II gene. We think that the differentiation between the two clades cannot be broken down to just one or two single soil characteristics. More knowledge about the ecology of nosZ clade II bacteria, which seem to be the major drivers for soil N2O sink capacity (Jones et al., 2014; Domeignoz-Horta et al., 2016a), is necessary. This knowledge may be used to design strategies to enrich agricultural soils either directly with nosZ clade II microorganisms or using amendments that are rich in these denitrifiers. In our study almost all organic amendments had a stimulating effect on the two nosZ clades. The rise in N2O production may have stimulated the N2O-reducers during the incubation (Hallin et al., 2017).
The archaeal 16s rRNA gene and archaeal amoA are the only two genes that are decreasing during the incubation. For archaea and especially the AOA inside the archaea kingdom it was already shown that they are more affected by rewetting stress compared to bacteria and AOB (Conrad et al., 2014; Thion and Prosser, 2014). The decrease in the archaeal amoA seem to be higher with the addition of either CC residues, digestate or sewage sludge to the soil (Figure 7). Potentially, the high N-content in these organic amendments, along with the high water level is known to favor denitrification processes (Skiba et al., 2002). Furthermore, it is believed that the addition of fertilizer normally lead to an increase in the AOB/AOA ratio (Wertz et al., 2012; Hartmann et al., 2013; Kastl et al., 2015), since it was shown that AOB grow faster after the addition of fertilizer, this may also true for our study. Even though a recent study showed that this effect is not occurring in every occasion by showing that AOA and AOB had changed in the same way during an incubation (Orellana et al., 2018).
It is not surprising that the treatments with CC residues harbored the highest abundance of N-fixing bacteria, since 1/3 of the CC residues mixtures we added were legumes (Sprent et al., 2017). N-fixers cannot directly be linked to a GHG production or consumption, but can have an indirect effect on N2O production by converting N2 to NH4 which then can be consumed by nitrifiers in the soil (Galloway et al., 1995).
Conclusion
In our study we analyzed different organic amendments and their influence on the GWP as well as functional microbial groups which are involved in GHG transformations in an agricultural soil. Our results indicate that compost amendments perform best with respect to the soil GWP calculated from the three major GHGs (CH4, CO2, N2O) and have a similar GWP as the un-amended soil (Table 2). Combinations of sewage sludge and digestate with both composts have also moderate effects on the soil GWP and will provide higher nutrients supply for plants. Although CC residues had the least favorable GWP, it still harbors a great long-term benefit to reduce GHG emissions from agricultural soils in manipulating the microbial communities. The CC residue amendment increased microbial groups that are involved in the reduction of GHGs (N2O-reducers, methanotrophs) or keeping the producing microbial community stable (methanogens, nitrifiers) compared to other organic amendments and the un-amended soil. This could provide a better GWP in the long-term. The next step would be to study the effect of plants on the GWP and have a deeper investigation of the associated microbial communities that are involved in GHG consumption and perform a longer running long-term incubation experiment to verify the short-term results. Further well-aerated agricultural soils need to be investigated in their potential as a sink for CH4, especially in combination with organic fertilizers and the potential of rare earth metals in these organic amendments. Understanding the underlying mechanisms of how organic fertilizers influence and possibly decrease GHG would allow us to develop a strategy to reduce GHG emission from agricultural soils without affecting the plant yield.
Author Contributions
KB designed the study, performed the laboratory experiment, performed all lab work (flux measurements, nucleic-acid extractions, qPCR analysis, analytical analyses), performed statistical analysis, evaluated the data, and wrote the manuscript. SD helped with the set-up of the laboratory experiment, evaluated the data, and wrote the manuscript. GK helped with collecting the organic amendments and wrote the manuscript. PB designed the study, evaluated the data, and wrote the manuscript.
Conflict of Interest Statement
The authors declare that the research was conducted in the absence of any commercial or financial relationships that could be construed as a potential conflict of interest.
Acknowledgments
We are grateful to Iris Chardon, Agata Pijl, and Hans Zweers for excellent technical assistance. We thank Andre Huisman (Attero, The Netherlands) and Jeroen van Lanen (Van Iersel Compost, The Netherlands) for providing us with the compost, Phillipe Packbier (Joordens company, The Netherlands) for providing us with the CC residues, Rob Verheijn (Vallei Veluwe, The Netherlands) for providing us with the sewage sludge, Rommie van der Weide and Sjaak van Brugge (ACRRES, The Netherlands) for providing us with digestate. KB is financially supported by the grant from the German DFG BR 5535/1-1. The publication number for the Netherlands Institute of Ecology is 6640. Experimental data are available at the DataverseNL server: https://dataverse.nl/dataset.xhtml?persistentId=hdl%3A10411%2FWVULDO&version=DRAFT.
Supplementary Material
The Supplementary Material for this article can be found online at: https://www.frontiersin.org/articles/10.3389/fmicb.2018.03035/full#supplementary-material
References
Alburquerque, J. A., de la Fuente, C., and Bernal, M. P. (2012). Chemical properties of anaerobic digestates affecting C and N dynamics in amended soils. Agric. Ecosyst. Environ. 160, 15–22. doi: 10.1016/j.agee.2011.03.007
Bakken, L. R., Bergaust, L., Liu, B., and Frostegård, Å. (2012). Regulation of denitrification at the cellular level: a clue to the understanding of N2O emissions from soils. Phil. Trans. R. Soc. B 367, 1226–1234. doi: 10.1098/rstb.2011.0321
Bateman, E., and Baggs, E. (2005). Contributions of nitrification and denitrification to N 2 O emissions from soils at different water-filled pore space. Biol. Fertil. Soils 41, 379–388. doi: 10.1007/s00374-005-0858-3
Bender, M., and Conrad, R. (1992). Kinetics of CH4 oxidation in oxic soils exposed to ambient air or high CH4 mixing ratios. FEMS Microbiol. Lett. 101, 261–269. doi: 10.1111/j.1574-6941.1992.tb01663.x
Bodelier, P. L., and Steenbergh, A. K. (2014). Interactions between methane and the nitrogen cycle in light of climate change. Curr. Opin. Environ. Sustain. 9, 26–36. doi: 10.1016/j.cosust.2014.07.004
Boeckx, P., Van Cleemput, O., and Villaralvo, I. (1997). Methane oxidation in soils with different textures and land use. Nutr. Cycling Agroecosyst. 49, 91–95. doi: 10.1023/A:1009706324386
Cai, Y., Zheng, Y., Bodelier, P. L., Conrad, R., and Jia, Z. (2016). Conventional methanotrophs are responsible for atmospheric methane oxidation in paddy soils. Nat. Commun. 7:11728. doi: 10.1038/ncomms11728
Chen, H., Mothapo, N. V., and Shi, W. (2015). Soil moisture and pH control relative contributions of fungi and bacteria to N 2 O production. Microb. Ecol. 69, 180–191. doi: 10.1007/s00248-014-0488-0
Cheng, Y., Wang, J., Zhang, J.-B., Müller, C., and Wang, S.-Q. (2015). Mechanistic insights into the effects of N fertilizer application on N2O-emission pathways in acidic soil of a tea plantation. Plant Soil 389, 45–57. doi: 10.1007/s11104-014-2343-y
Ciais, P., Sabine, C., Bala, G., Bopp, L., Brovkin, V., Canadell, J., et al. (2014). Carbon and Other Biogeochemical Cycles. Climate Change 2013: The Physical Science Basis. Contribution of Working Group I to the Fifth Assessment Report of the Intergovernmental Panel on Climate Change, Cambridge University Press, 465–570.
Conrad, R. (2007). Microbial ecology of methanogens and methanotrophs. Adv. Agron. 96, 1–63. doi: 10.1016/S0065-2113(07)96005-8
Conrad, R., Ji, Y., Noll, M., Klose, M., Claus, P., and Enrich-Prast, A. (2014). Response of the methanogenic microbial communities in Amazonian oxbow lake sediments to desiccation stress. Environ. Microbiol. 16, 1682–1694. doi: 10.1111/1462-2920.12267
Conrad, R., and Rothfuss, F. (1991). Methane oxidation in the soil surface layer of a flooded rice field and the effect of ammonium. Biol. Fertil. Soils 12, 28–32. doi: 10.1007/BF00369384
Coombs, C., Lauzon, J. D., Deen, B., and Van Eerd, L. L. (2017). Legume cover crop management on nitrogen dynamics and yield in grain corn systems. Field Crops Res. 201, 75–85. doi: 10.1016/j.fcr.2016.11.001
Diacono, M., and Montemurro, F. (2010). Long-term effects of organic amendments on soil fertility. a review. Agron. Sustain. Dev. 30, 401–422. doi: 10.1051/agro/2009040
Domeignoz-Horta, L., Spor, A., Bru, D., Bizouard, F., Leonard, J., and Philippot, L. (2015). The diversity of the N2O reducers matters for the N2O: N2 denitrification end-product ratio across an annual and a perennial cropping system. Front. Microbiol. 6:971. doi: 10.3389/fmicb.2015.00971
Domeignoz-Horta, L. A., Peyrard, C., Bru, D., Breuil, M.-C., Bizouard, F., Justes, E., et al. (2016a). Effects of Agricultural Practices and Soil Properties on Soil N2O-Reducing Bacteria and in situ N2O Emissions. Écologie des Bactéries N2O Réductrices Dans les Sols Agricoles, 57.
Domeignoz-Horta, L. A., Putz, M., Spor, A., Bru, D., Breuil, M.-C., Hallin, S., et al. (2016b). Non-denitrifying nitrous oxide-reducing bacteria-an effective N2O sink in soil. Soil Biol. Biochem. 103, 376–379. doi: 10.1016/j.soilbio.2016.09.010
El-Ramady, H. R. H. (2011). A Contribution on the Bio-actions of Rare Earth Elements in the Soil/Plant Environment. Dissertationen aus dem Julius Kühn-Institut.
Epstein, E., Taylor, J., and Chancy, R. (1976). Effects of sewage sludge and sludge compost applied to soil on some soil physical and chemical properties 1. J. Environ. Q. 5, 422–426. doi: 10.2134/jeq1976.00472425000500040021x
Galloway, J. N., Schlesinger, W. H., Levy, H., Michaels, A., and Schnoor, J. L. (1995). Nitrogen fixation: anthropogenic enhancement-environmental response. Glob. Biogeochem. Cycles 9, 235–252. doi: 10.1029/95GB00158
Groffman, P. M., Butterbach-Bahl, K., Fulweiler, R. W., Gold, A. J., Morse, J. L., Stander, E. K., et al. (2009). Challenges to incorporating spatially and temporally explicit phenomena (hotspots and hot moments) in denitrification models. Biogeochemistry 93, 49–77. doi: 10.1007/s10533-008-9277-5
Gu, W., and Semrau, J. D. (2017). Copper and cerium-regulated gene expression in Methylosinus trichosporium OB3b. Appl. Microbiol. Biotechnol. 101, 8499–8516. doi: 10.1007/s00253-017-8572-2
Hallin, S., Jones, C. M., Schloter, M., and Philippot, L. (2009). Relationship between N-cycling communities and ecosystem functioning in a 50-year-old fertilization experiment. ISME J. 3, 597–605. doi: 10.1038/ismej.2008.128
Hallin, S., Philippot, L., Löffler, F. E., Sanford, R. A., and Jones, C. M. (2017). Genomics and ecology of novel N2O-reducing microorganisms. Trends Microbiol. 26, 43–55 doi: 10.1016/j.tim.2017.07.003
Hartmann, A. A., Barnard, R. L., Marhan, S., and Niklaus, P. A. (2013). Effects of drought and N-fertilization on N cycling in two grassland soils. Oecologia 171, 705–717. doi: 10.1007/s00442-012-2578-3
Hellmann, B., Zelles, L., Palojarvi, A., and Bai, Q. (1997). Emission of climate-relevant trace gases and succession of microbial communities during open-windrow composting. Appl. Environ. Microbiol. 63, 1011–1018.
Highton, M. P., Roosa, S., Crawshaw, J., Schallenberg, M., and Morales, S. E. (2016). Physical factors correlate to microbial community structure and nitrogen cycling gene abundance in a nitrate fed eutrophic lagoon. Front. Microbiol. 7:1691. doi: 10.3389/fmicb.2016.01691
Hiltbrunner, D., Zimmermann, S., Karbin, S., Hagedorn, F., and Niklaus, P. A. (2012). Increasing soil methane sink along a 120-year afforestation chronosequence is driven by soil moisture. Glob. Change Biol. 18, 3664–3671. doi: 10.1111/j.1365-2486.2012.02798.x
Ho, A., Ijaz, U. Z., Janssens, T. K., Ruijs, R., Kim, S. Y., de Boer, W., et al. (2017). Effects of bio-based residue amendments on greenhouse gas emission from agricultural soil are stronger than effects of soil type with different microbial community composition. Gcb Bioenergy 9, 1707–1720. doi: 10.1111/gcbb.12457
Ho, A., Reim, A., Kim, S. Y., Meima-Franke, M., Termorshuizen, A., de Boer, W., et al. (2015). Unexpected stimulation of soil methane uptake as emergent property of agricultural soils following bio-based residue application. Glob. Change Biol. 21, 3864–3879. doi: 10.1111/gcb.12974
IPCC (2014). “Climate change 2014: synthesis report,” in Contribution of Working Groups I, II and III to the Fifth Assessment Report of the Intergovernmental Panel on Climate Change, eds R. K. Pachauri and L. A. Meyer (Geneva: IPCC), 151.
Järveoja, J., Peichl, M., Maddison, M., Teemusk, A., and Mander, Ü. (2016). Full carbon and greenhouse gas balances of fertilized and nonfertilized reed canary grass cultivations on an abandoned peat extraction area in a dry year. Gcb Bioenergy 8, 952–968. doi: 10.1111/gcbb.12308
Jones, C. M., Graf, D. R., Bru, D., Philippot, L., and Hallin, S. (2013). The unaccounted yet abundant nitrous oxide-reducing microbial community: a potential nitrous oxide sink. ISME J. 7, 417–426. doi: 10.1038/ismej.2012.125
Jones, C. M., Spor, A., Brennan, F. P., Breuil, M.-C., Bru, D., Lemanceau, P., et al. (2014). Recently identified microbial guild mediates soil N 2 O sink capacity. Nat. Climate Change 4, 801–805. doi: 10.1038/nclimate2301
Kastl, E.-M., Schloter-Hai, B., Buegger, F., and Schloter, M. (2015). Impact of fertilization on the abundance of nitrifiers and denitrifiers at the root–soil interface of plants with different uptake strategies for nitrogen. Biol. Fertil. Soils 51, 57–64. doi: 10.1007/s00374-014-0948-1
Keltjens, J. T., Pol, A., Reimann, J., and den Camp, H. J. O. (2014). PQQ-dependent methanol dehydrogenases: rare-earth elements make a difference. Appl. Microbiol. Biotechnol. 98, 6163–6183. doi: 10.1007/s00253-014-5766-8
Kirschke, S., Bousquet, P., Ciais, P., Saunois, M., Canadell, J. G., Dlugokencky, E. J., et al. (2013). Three decades of global methane sources and sinks. Nat. Geosci. 6, 813–823. doi: 10.1038/ngeo1955
Kramer, S. B., Reganold, J. P., Glover, J. D., Bohannan, B. J., and Mooney, H. A. (2006). Reduced nitrate leaching and enhanced denitrifier activity and efficiency in organically fertilized soils. Proc. Natl. Acad. Sci. U.S.A. 103, 4522–4527. doi: 10.1073/pnas.0600359103
Krause, S. M., Johnson, T., Karunaratne, Y. S., Fu, Y., Beck, D. A., Chistoserdova, L., et al. (2017). Lanthanide-dependent cross-feeding of methane-derived carbon is linked by microbial community interactions. Proc. Natl. Acad. Sci. U.S.A. 114, 358–363. doi: 10.1073/pnas.1619871114
Kravchenko, A., Toosi, E., Guber, A., Ostrom, N., Yu, J., Azeem, K., et al. (2017). Hotspots of soil N2O emission enhanced through water absorption by plant residue. Nat. Geosci. 10, 496–500. doi: 10.1038/ngeo2963
Levine, U. Y., Teal, T. K., Robertson, G. P., and Schmidt, T. M. (2011). Agriculture's impact on microbial diversity and associated fluxes of carbon dioxide and methane. ISME J. 5, 1683–1691. doi: 10.1038/ismej.2011.40
Lim, S., and Franklin, S. (2004). Lanthanide-binding peptides and the enzymes that might have been. Cell. Mol. Life Sci. 61, 2184–2188. doi: 10.1007/s00018-004-4156-2
Malyan, S. K., Bhatia, A., Kumar, A., Gupta, D. K., Singh, R., Kumar, S. S., et al. (2016). Methane production, oxidation and mitigation: a mechanistic understanding and comprehensive evaluation of influencing factors. Sci. Total Environ. 572, 874–896. doi: 10.1016/j.scitotenv.2016.07.182
Marinari, S., Mancinelli, R., Brunetti, P., and Campiglia, E. (2015). Soil quality, microbial functions and tomato yield under cover crop mulching in the Mediterranean environment. Soil Tillage Res. 145, 20–28. doi: 10.1016/j.still.2014.08.002
Maul, J. E., Buyer, J. S., Lehman, R. M., Culman, S., Blackwood, C. B., Roberts, D. P., et al. (2014). Microbial community structure and abundance in the rhizosphere and bulk soil of a tomato cropping system that includes cover crops. Appl. Soil Ecol. 77, 42–50. doi: 10.1016/j.apsoil.2014.01.002
Maxfield, P., Hornibrook, E., and Evershed, R. (2008). Acute impact of agriculture on high-affinity methanotrophic bacterial populations. Environ. Microbiol. 10, 1917–1924. doi: 10.1111/j.1462-2920.2008.01587.x
Mosier, A., and Delgado, J. (1997). Methane and nitrous oxide fluxes in grasslands in western Puerto Rico. Chemosphere 35, 2059–2082. doi: 10.1016/S0045-6535(97)00231-2
Orellana, L. H., Chee-Sanford, J. C., Sanford, R. A., Löffler, F. E., and Konstantinidis, K. T. (2018). Year-round shotgun metagenomes reveal stable microbial communities in agricultural soils and novel ammonia oxidizers responding to fertilization. Appl. Environ. Microbiol. 84, e01646–e01617. doi: 10.1128/AEM.01646-17
Paustian, K., Lehmann, J., Ogle, S., Reay, D., Robertson, G. P., and Smith, P. (2016). Climate-smart soils. Nature 532, 49–57. doi: 10.1038/nature17174
Pol, A., Barends, T. R., Dietl, A., Khadem, A. F., Eygensteyn, J., Jetten, M. S., et al. (2014). Rare earth metals are essential for methanotrophic life in volcanic mudpots. Environ. Microbiol. 16, 255–264. doi: 10.1111/1462-2920.12249
Pratscher, J., Vollmers, J., Wiegand, S., Dumont, M. G., and Kaster, A. K. (2018). Unravelling the identity, metabolic potential and global biogeography of the atmospheric methane-oxidizing upland soil cluster α. Environ. Microbiol. 20, 1016–1029. doi: 10.1111/1462-2920.14036
R Development Core Team (2013). R: A Language and Environment for Statistical Computing. Vienna: R Foundation for Statistical Computing. Available online at: http://www.R-project.org/.
Ryals, R., Hartman, M. D., Parton, W. J., DeLonge, M. S., and Silver, W. L. (2015). Long-term climate change mitigation potential with organic matter management on grasslands. Ecol. Appl. 25, 531–545. doi: 10.1890/13-2126.1
Sanz-Cobena, A., Abalos, D., Meijide, A., Sanchez-Martin, L., and Vallejo, A. (2016). Soil moisture determines the effectiveness of two urease inhibitors to decrease N2O emission. Mitigation Adapt. Strateg. Glob. Change 21, 1131–1144. doi: 10.1007/s11027-014-9548-5
Shaaban, M., Peng, Q.-A., Lin, S., Wu, Y., Khalid, M. S., Wu, L., et al. (2016). Dolomite application enhances CH 4 uptake in an acidic soil. Catena 140, 9–14. doi: 10.1016/j.catena.2016.01.014
Shackley, S., Ruysschaert, G., Zwart, K., and Glaser, B. (2016). Biochar in European Soils and Agriculture: Science and Practice. London: Routledge. doi: 10.4324/9781315884462
Shcherbak, I., Millar, N., and Robertson, G. P. (2014). Global metaanalysis of the nonlinear response of soil nitrous oxide (N2O) emissions to fertilizer nitrogen. Proc. Natl. Acad. Sci. U.S.A. 111, 9199–9204. doi: 10.1073/pnas.1322434111
Shiller, A., Chan, E., Joung, D., Redmond, M., and Kessler, J. (2017). Light rare earth element depletion during deepwater horizon blowout methanotrophy. Sci. Rep. 7:10389. doi: 10.1038/s41598-017-11060-z
Shoun, H., Fushinobu, S., Jiang, L., Kim, S.-W., and Wakagi, T. (2012). Fungal denitrification and nitric oxide reductase cytochrome P450nor. Philos. Trans. R. Soc. B 367, 1186–1194. doi: 10.1098/rstb.2011.0335
Singh, J. S., and Seneviratne, G. (2017). Agro-Environmental Sustainability: Volume 2: Managing Environmental Pollution. Basel: Springer International Publishing.
Skiba, U., Dijk, S., and Ball, B. (2002). The influence of tillage on NO and N2O fluxes under spring and winter barley. Soil Use Manage. 18, 340–345. doi: 10.1111/j.1475-2743.2002.tb00250.x
Sprent, J. I., Ardley, J., and James, E. K. (2017). Biogeography of nodulated legumes and their nitrogen-fixing symbionts. N. Phytol. 215, 40–56. doi: 10.1111/nph.14474
Stocker, T. F., Qin, D., Plattner, G.-K., Tignor, M., Allen, S. K., Boschung, J., et al. (2013). IPCC, 2013: Climate Change 2013: The Physical Science Basis. Contribution of Working Group I to The Fifth Assessment Report of the Intergovernmental Panel on Climate Change. Cambridge University Press.
Syakila, A., and Kroeze, C. (2011). The global nitrous oxide budget revisited. Greenhouse Gas Meas. Manage. 1, 17–26. doi: 10.3763/ghgmm.2010.0007
Takaya, N. (2002). Dissimilatory nitrate reduction metabolisms and their control in fungi. J. Biosci. Bioeng. 94, 506–510. doi: 10.1016/S1389-1723(02)80187-6
Tate, K. R. (2015). Soil methane oxidation and land-use change–from process to mitigation. Soil Biol. Biochem. 80, 260–272. doi: 10.1016/j.soilbio.2014.10.010
Thangarajan, R., Bolan, N. S., Tian, G., Naidu, R., and Kunhikrishnan, A. (2013). Role of organic amendment application on greenhouse gas emission from soil. Sci. Total Environ. 465, 72–96. doi: 10.1016/j.scitotenv.2013.01.031
Thion, C., and Prosser, J. I. (2014). Differential response of nonadapted ammonia-oxidising archaea and bacteria to drying–rewetting stress. FEMS Microbiol. Ecol. 90, 380–389. doi: 10.1111/1574-6941.12395
Thomson, A. J., Giannopoulos, G., Pretty, J., Baggs, E. M., and Richardson, D. J. (2012). Biological sources and sinks of nitrous oxide and strategies to mitigate emissions. Philos. Trans. R. Soc. Lond. B Biol. Sci. 367, 1157–1168. doi: 10.1098/rstb.2011.0415
Tian, H., Lu, C., Ciais, P., Michalak, A. M., Canadell, J. G., Saikawa, E., et al. (2016). The terrestrial biosphere as a net source of greenhouse gases to the atmosphere. Nature 531, 225–228. doi: 10.1038/nature16946
Vargas, V. P., Cantarella, H., Martins, A. A., Soares, J. R., do Carmo, J. B., and de Andrade, C. A. (2014). Sugarcane crop residue increases N2O and CO2 emissions under high soil moisture conditions. Sugar Tech. 16, 174–179. doi: 10.1007/s12355-013-0271-4
Veldkamp, E., Koehler, B., and Corre, M. (2013). Indications of nitrogen-limited methane uptake in tropical forest soils. Biogeosciences 10, 5367–5379. doi: 10.5194/bg-10-5367-2013
Wertz, S., Leigh, A. K., and Grayston, S. J. (2012). Effects of long-term fertilization of forest soils on potential nitrification and on the abundance and community structure of ammonia oxidizers and nitrite oxidizers. FEMS Microbiol. Ecol. 79, 142–154. doi: 10.1111/j.1574-6941.2011.01204.x
Keywords: nitrous oxide, carbon dioxide, methane oxidation, agricultural soil, organic amendment, flux measurements, qPCR
Citation: Brenzinger K, Drost SM, Korthals G and Bodelier PLE (2018) Organic Residue Amendments to Modulate Greenhouse Gas Emissions From Agricultural Soils. Front. Microbiol. 9:3035. doi: 10.3389/fmicb.2018.03035
Received: 10 July 2018; Accepted: 23 November 2018;
Published: 07 December 2018.
Edited by:
Pil Joo Kim, Gyeongsang National University, South KoreaReviewed by:
Tapan Kumar Adhya, KIIT University, IndiaJeanette M. Norton, Utah State University, United States
Yahai Lu, Peking University, China
Copyright © 2018 Brenzinger, Drost, Korthals and Bodelier. This is an open-access article distributed under the terms of the Creative Commons Attribution License (CC BY). The use, distribution or reproduction in other forums is permitted, provided the original author(s) and the copyright owner(s) are credited and that the original publication in this journal is cited, in accordance with accepted academic practice. No use, distribution or reproduction is permitted which does not comply with these terms.
*Correspondence: Kristof Brenzinger, k.brenzinger@nioo.knaw.nl