- 1Department of Biotechnology, Alagappa University, Karaikudi, India
- 2Department of Bacteriology and Immunology, Medicum, Research Programs Unit, Immunobiology Research Program, University of Helsinki, Helsinki, Finland
- 3Division of Clinical Microbiology, Helsinki University Hospital, HUSLAB, Helsinki, Finland
Background: Alpha-mangostin (α-MG) is a natural xanthone reported to exhibit rapid bactericidal activity against Gram-positive bacteria, and may therefore have potential clinical application in healthcare sectors. This study sought to identify the impact of α-MG on Staphylococcus epidermidis RP62A through integrated advanced omic technologies.
Methods: S. epidermidis was challenged with sub-MIC (0.875 μg/ml) of α-MG at various time points and the differential expression pattern of genes/proteins were analyzed in the absence and presence of α-MG using RNA sequencing and LC-MS/MS experiments. Bioinformatic tools were used to categorize the biological processes, molecular functions and KEGG pathways of differentially expressed genes/proteins. qRT-PCR was employed to validate the results obtained from these analyses.
Results: Transcriptomic and proteomic profiling of α-MG treated cells indicated that genes/proteins affected by α-MG treatment were associated with diverse cellular functions. The greatest reduction in expression was observed in transcription of genes conferring cytoplasmic membrane integrity (yidC2, secA and mscL), cell division (ftsY and divlB), teichoic acid biosynthesis (tagG and dltA), fatty-acid biosynthesis (accB, accC, fabD, fabH, fabI, and fabZ), biofilm formation (icaA) and DNA replication and repair machinery (polA, polC, dnaE, and uvrA). Those with increased expression were involved in oxidative (katA and sodA) and cellular stress response (clpB, clpC, groEL, and asp23). The qRT-PCR analysis substantiated the results obtained from transcriptomic and proteomic profiling studies.
Conclusion: Combining transcriptomic and proteomic methods provided comprehensive information about the antibacterial mode of action of α-MG. The obtained results suggest that α-MG targets S. epidermidis through multifarious mechanisms, and especially prompts that loss of cytoplasmic membrane integrity leads to rapid onset of bactericidal activity.
Introduction
Staphylococcus epidermidis is one of the most important causes of nosocomial and community acquired infections (Gomes et al., 2014). It is often observed in the device related and surgical site infections, where the biofilm formation on implants and tissues further increases the treatment failure (Center for Disease Control Prevention [CDC], 2013; Danzmann et al., 2013; Gomes et al., 2014). In addition, infections associated with biofilms are persisting until the subsequent replacement or removal of implants, which causes distress to patients and lead to superfluous expenditure (Donlan and Costerton, 2002). The available antibiotic therapy can only kill planktonic cells, leaving the bacterial cells to grow within the biofilms continuously even after the termination of antibiotic therapy (Roper et al., 2000; Parra-Ruiz et al., 2012; Reiter et al., 2014). Alarmingly, the ability of biofilm to resist clearance by antibiotics increased the importance of a continuous search for novel antibacterial agents that target both planktonic and biofilm populations. Hence, new antibacterial agents are needed to combat biofilm mediated infections caused by S. epidermidis.
Throughout human history, plants have been the inexhaustible source of novel bioactive compounds with multitude of blockbuster therapeutic drugs, which have been derived directly or indirectly from various plants (Kinghorn et al., 2011; Newman and Cragg, 2012; Veeresham, 2012; Pohlit et al., 2013; Laallam et al., 2015; Satthakarn et al., 2015; Khan et al., 2018). Among the reported plant-derived bioactive compounds, alpha-mangostin (α-MG), a natural xanthone derived from the pericarp of Garcinia mangostana has been reported for various pharmacological properties that includes antibacterial, antifungal, anti-inflammatory, anticancer, and antioxidant activity (Ibrahim et al., 2016). α-MG elicits in vitro rapid bactericidal activity against several Gram-positive pathogens (Nguyen and Marquis, 2011; Koh et al., 2013; Sivaranjani et al., 2017). As reported by Koh et al. (2013) α-MG rapidly disintegrates the cytoplasmic membrane integrity of methicillin resistant Staphylococcus aureus (MRSA), which results in loss of cytoplasmic components. The multi-step resistance selection assay from previous studies suggested that Gram-positive pathogens do not develop resistance against α-MG (Koh et al., 2013; Sivaranjani et al., 2017). Most importantly, data from our previous study confirmed that α-MG effectively inhibits the onset of biofilm formation as well as disrupts the immature and mature biofilms of S. epidermidis RP62A biofilms, though the highest concentration of vancomycin was inefficient in killing the sessile cells of S. epidermidis RP62A (Sivaranjani et al., 2017). Similarly, Nguyen et al. (2014) reported that topical application of α-MG can effectively disrupt the development and structural integrity of Streptococcus mutans biofilm, which facilitates the mechanical clearance of cariogenic biofilms. Besides, several studies have demonstrated efficient methods to synthesize α-MG derivatives that also reflects the importance of α-MG and its derivatives in biological research (Matsumoto et al., 2004; Ha et al., 2009; Xu et al., 2013; Zou et al., 2013; Fei et al., 2014; Koh et al., 2015; Li et al., 2015; Koh et al., 2016). The potential bottleneck to develop α-MG as an effective antibacterial agent is the very limited understanding of the molecular mechanism of action of α-MG. Indeed, several studies have used omics techniques to elucidate the antibacterial mode of action of plant-derived compounds (Reddy et al., 2015; Dos Santos et al., 2016). Though, the rapid antibacterial mode of action of α-MG has been already investigated through in vitro and in silico approaches (Koh et al., 2013), integrated advanced omics technologies will further augment the current knowledge on the mode of action of α-MG. In the present study, we investigated the molecular mechanism of antibacterial activity of α-MG through an integrated transcriptomic and proteomic approach.
Materials and Methods
Bacterial Strain and Chemical
Staphylococcus epidermidis RP62A (ATCC 35984) was routinely grown in Luria-Bertani (LB; HiMedia, India) and was maintained in LB with 30% glycerol at -80°C. α-MG was purchased from Sigma-Aldrich (Catalog No.: M3824) and stock solution of 1 mg/mL was prepared in methanol.
Antibacterial Assays
The minimum inhibitory concentration (MIC), minimum bactericidal concentration (MBC) and time kill kinetics assays were previously determined (Sivaranjani et al., 2017). The MIC and MBC values of α-MG were determined again to precede subsequent assays (Clinical Laboratory Standards Institute [CLSI], 2006). The spot assay was carried out to determine the antibacterial activity of α-MG on mid-log phase cultures. Different concentrations of α-MG [1.25 μg/mL (MIC), 0.875 μg/mL (0.7 MIC), 0.625 μg/mL (0.5 MIC), 0.3125 μg/mL (0.25 MIC)] were added to mid-log phase (∼2.5 × 108 CFU/ml) cultures that were subsequently incubated at 37°C for 10 min. After incubation, 10 μl of serially diluted control (0.1% methanol) and α-MG treated samples were spotted on LB plates. The plates were incubated at 37°C for 18 h.
RNA-Sequencing and Data Analysis
Overnight cultures of S. epidermidis were diluted in 1:100 in LB and were grown to mid-log phase (∼2.5 × 108 CFU/ml) at 37°C. Bacterial cells were treated with 0.7 MIC (0.875 μg/ml) of α-MG or 0.1% methanol (solvent control) for 10 min. After incubation, 2 volumes of RNAprotect Bacteria Reagent (Qiagen, Hilden, Germany) were added to one volume of bacterial cultures. Then, the cells were pelleted by centrifugation, resuspended in 200 μl of TE buffer containing 15 mg/ml of lysozyme and 10 μl of proteinase K and incubated at 37°C for 30 min. After incubation, zirconia beads (50 mg; 0.1 mm) were added to lyse the cells using FastPrep 24 instrument (Qbiogene, Heidelberg, Germany) and the total RNA was extracted using RNeasy Mini kit (Qiagen, Hilden, Germany). To remove genomic DNA, on-column DNA digestion was done using RNase-Free DNase set (Qiagen, Hilden, Germany). The RNA integrity was analyzed using LabChip GXII Touch HT (PerkinElmer, United States). The ribosomal RNA (rRNA) was depleted using Ribo-Zero rRNA Removal kit for Gram-positive bacteria (Illumina, San Diego, CA, United States). Sequencing library was prepared as described before with modifications (Macosko et al., 2015). The libraries were sequenced in Biomedicum Functional Genomics Unit (Helsinki, Finland). Reverse transcription of mRNA was performed using specially designed primers. Similarly to the method described in Macosko et al. (2015), all the primers shared a common sequence that served as a PCR handle. Additionally, the primers contained known 12 bp barcode (unique for each sample) and different random unique molecular identifiers (UMIs) (Macosko et al., 2015). The poly (T) regions of the oligos were replaced by random hexamers to enable the capturing of the bacterial mRNA particles. The reverse transcription, PCR amplification of the cDNA and tagmentation were performed as described by Macosko et al. (2015). Paired-end sequencing was performed on NextSeq500 sequencer (Illumina) using the sequencing primers (Macosko et al., 2015). Sequencing in 75 cycles produced 20 bp Read 1 (bases 1–12 sample barcode, bases 13–20 UMI) and 55 bp Read 2 (sequence derived from the bacterial mRNA). The raw sequencing reads were analyzed using the Dropseq Core Computational Protocol (Macosko et al., 2015). The obtained sequencing reads were aligned against the strain S. epidermidis RP62A (ATCC 35984) genome using the STAR aligner (Dobin et al., 2013). The edgeR, a Bioconductor package was used to obtain the list of differentially expressed genes (Robinson et al., 2010). The RNA sequence data has been deposited to NCBI Gene Expression Omnibus (Accession No.: GSE113302).
Quantitative Proteomics
The mid-exponential growth phase (∼2.5 × 108 CFU/ml) cultures were treated with 0.7 MIC (0.875 μg/ml) of α-MG or 0.1% methanol (solvent control) for 10 and 30 min. After incubation, α-MG treated and untreated cells were harvested by centrifugation at 3500 × g, washed with sterile PBS and resuspended in 1 ml of lysis buffer (0.1% RapiGest, 8 M urea, 100 mM ammonium bicarbonate). The cell suspension was sonicated for 10 min (Branson Sonifier 450; 30 s running, 30 s pause, pulsed mode 30%) and stored at -70°C. Prior to trypsin digestion of proteins, the protein samples were reduced with Tris (2-carboxyethyl) phosphine and alkylated with iodoacetamide. Subsequently, tryptic peptide digests were purified using C18 reversed-phase columns (Varjosalo et al., 2013) and the mass spectrometric analysis was performed in Orbitrap Elite ETD mass spectrometer (Thermo Scientific), using Xcalibur version 2.7.1 coupled with a Thermo Scientific nLCII nanoflow HPLC system. MS peak extraction and subsequent protein identification was analyzed using Proteome Discoverer software (Thermo Scientific). Calibrated peak files were searched against the S. epidermidis RP62A proteins (Uniprot) by a SEQUEST search engine. Error tolerance on the fragment and precursor ions were +0.8 Da and +15 p.p.m, respectively. For peptide identification, a stringent cut-off value (0.5% false discovery rate, FDR) was used.
Bioinformatics Tools
Gene ontology (GO) annotation and Kyoto Encyclopedia of Genes and Genomes (KEGG) pathway assignations were carried out to determine the function of differentially regulated genes, using STRING (version 10.5) with high confidence score of 0.7 (Carvalhais et al., 2014). Categories with a p-value ≤ 0.05 were set as statistically significant for gene enrichment. Differentially expressed hypothetical proteins were analyzed for putative functions using protein BLAST to search Pfam (protein families) domains in a Pfam database (version 31.0) and subcellular localization was predicted using PSORTb (Yu et al., 2010). The differentially expressed genes were also analyzed in the CELLO2GO1 to retrieve the sub-cellular localization and functional GO annotation against Gram-positive bacteria with an E-value 0.001 (Yu et al., 2014).
Experimental Validation Using Quantitative Real-Time-PCR (qRT-PCR)
Twenty-one differentially expressed genes were selected for validation using qRT-PCR. The gene specific primers were designed using Primer3 software (version 0.4.0). The details of primers are listed in Supplementary Table 1. Total RNA was isolated as described above and cDNA was synthesized using Superscript III kit (Invitrogen Inc., United States) as per the manufacturer’s instructions. The qRT-PCR reaction was performed in 7500 Sequence Detection System (Applied Biosystems Inc., Foster, CA, United States). The mRNA expression patterns of selected genes were normalized against constitutively expressed two internal controls (16SrRNA and rplU) and quantified using 2-ΔΔCt method. Three independent experiments were carried out in duplicate. Statistical significance was analyzed using unpaired Student t-test and considered significant at P < 0.05.
Results
Determination of α-MG Concentration for Transcriptomic and Proteomic Profiling Studies
The MIC and MBC of α-MG was found to be 1.25 and 5 μg/mL, respectively, which substantiates the results obtained from the previous study (Sivaranjani et al., 2017). Besides, Sivaranjani et al. (2017) have already reported the rapid bactericidal property of α-MG against S. epidermidis cells, where it displayed 6 log (4× MIC; 5 μg/mL) and 4 log (2× MIC; 2.5 μg/mL) reduction of viable count within 5 min of exposure time (Sivaranjani et al., 2017). Since transcriptional profiling is typically performed by the addition of antibacterial agents to mid-log phase cultures, spot assay was carried out to choose the concentration and duration of α-MG treatment. Based on the results, 0.7 MIC (0.875 μg/mL) of α-MG was chosen for 10 min and 30 min of antibacterial challenge with S. epidermidis (Supplementary Figure 1).
Global Transcriptome and Proteome Response to α-MG Treatment
Transcriptome Analysis
To uncover the antibacterial mode of action of α-MG, RNA sequencing was performed from α-MG treated (0.7 MIC, 10 min) and untreated S. epidermidis RP62A cells. A total of 2,411 genes were identified under both the conditions, which correspond to 91.84% of S. epidermidis RP62A (2,625 genes in GenBank). Of 2,411 genes, the edgeR analysis revealed differential expression of 98 genes (4.06%) with ≥1.5-fold difference in log FC (log fold change) with P < 0.001, wherein 48 (1.9%) genes were upregulated while the remaining 50 (2.07%) genes were downregulated in response to α-MG treatment. The details of differentially regulated genes, their role and the log FC are listed in Table 1. Besides, 33.6% (33/98) of differentially regulated genes were encoding uncharacterized proteins. Hence, identities of protein families and sub-cellular localization of hypothetical proteins were predicted accordingly using Pfam and PSORTb (Supplementary Table 2). In addition, 30.3% (10/33), 27.2% (9/33), 3% (1/33), and 39.3% (13/33) of the hypothetical proteins were predicted to be localized in the cytoplasmic membrane-associated, cytoplasic, extracellular and location-unknown, respectively.
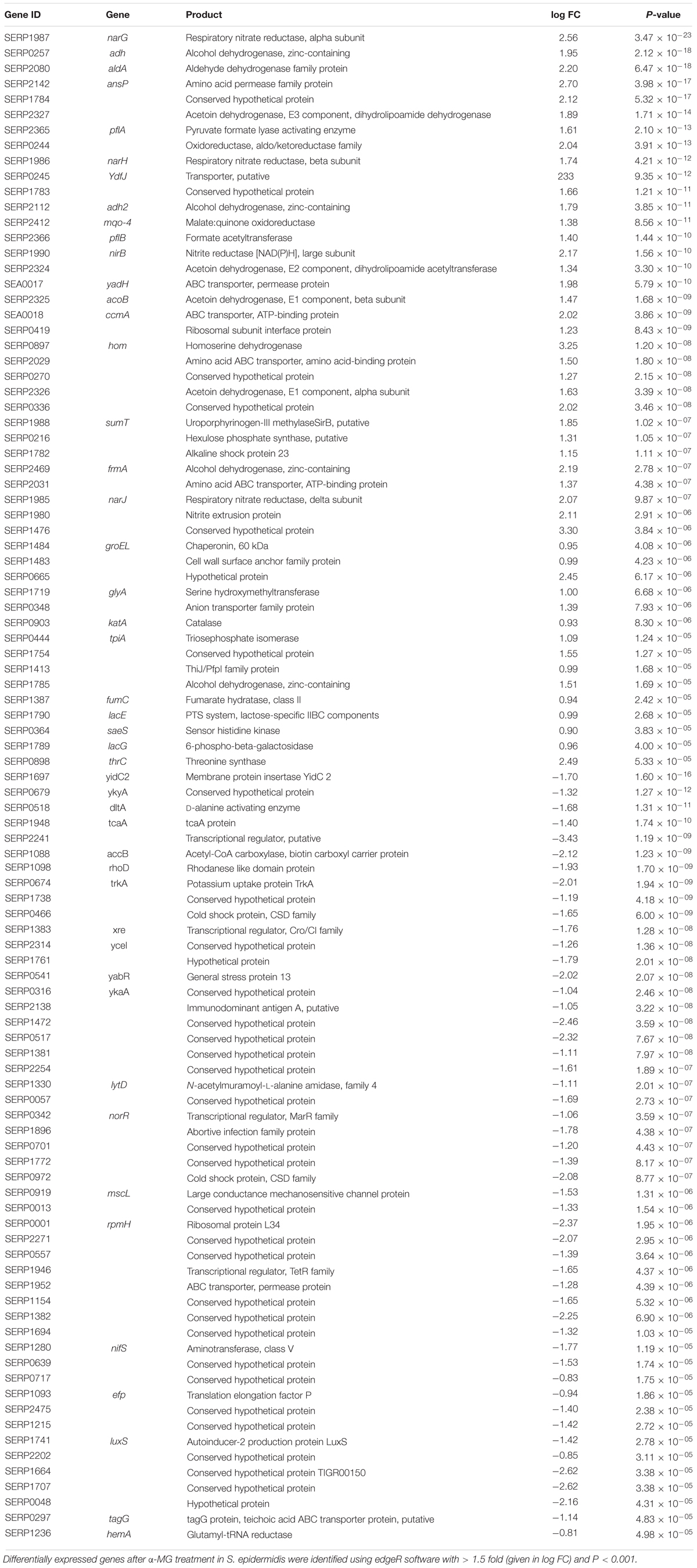
Table 1. List of differentially expressed genes of S. epidermidis treated with α-MG identified using RNA sequencing.
The functional categorization for upregulated and downregulated genes was analyzed separately using STRING10.5. Subsequently, GO (biological process, molecular function and cellular component) analysis for differentially regulated genes were annotated manually and the interacting network maps are shown in Figures 1A, 2A. According to GO annotation, the upregulated genes were scattered among various biological processes which included oxidation-reduction, metabolic, phosphorylation, nucleobase-containing small molecule metabolic, tricarboxylic acid (TCA) cycle and aerobic respiration processes (Figure 1B). The predominant molecular functions of upregulated genes were catalytic activity, oxidoreductase activity and other molecular functions (Figure 1C). Further, in the KEGG pathway analysis, the upregulated genes were shown to be involved in glycolysis/gluconeogenesis, TCA cycle, carbon metabolism, nitrogen metabolism, pyruvate metabolism, aminoacid biosynthesis, ABC transporters, RNA and fatty-acid degradation pathways (Figure 3A). Meanwhile, GO annotation of downregulated genes were involved in various biological processes viz., protoporphyrinogen IX biosynthetic, heme-biosynthetic, cofactor biosynthetic, sulfur compound biosynthetic and malonyl-CoA biosynthetic processes (Figure 2B). In addition, the KEGG pathway analysis indicated that the downregulated genes are involved in several metabolic pathways that include biosynthesis of secondary metabolites, fatty-acid biosynthesis, DNA replication and mismatch repair (Figure 3B). Acetyl-CoA carboxylase complex (GO.0009317) was the only significant cellular component encoded by the downregulated genes. Furthermore, the CELLO2GO, used for functional GO annotation and sub-cellular localization for proteins encoded by differentially expressed genes (Supplementary Figure 2), substantiated the results obtained from STRING10.5 analysis. It has been reported to predict sub-cellular localization of proteins with 99.4% accuracy among Gram-positive bacteria (Yu et al., 2014). Among the proteins encoded by the 98 differentially expressed genes, 66.7, 18.9, 13.5, and 0.9% were predicted to be cytoplasmic, cytoplasmic membrane- associated, extracellular and cell wall-associated proteins, respectively.
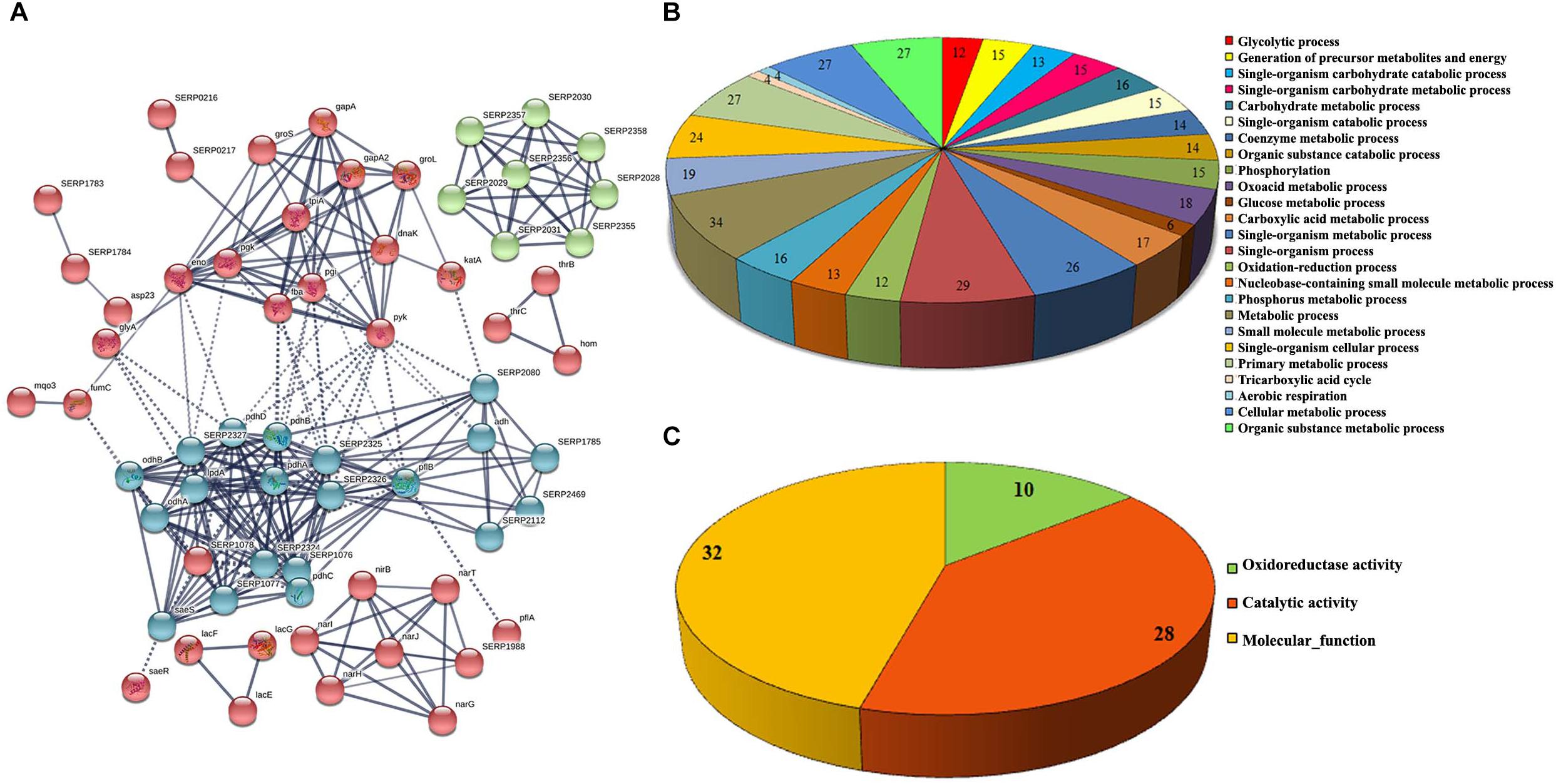
Figure 1. Interaction analysis of upregulated genes identified by RNA-sequencing. (A) Interactome map predicted using STRING10.5. The network nodes represent the proteins encoded by the genes. Edges represent protein-protein associations with high confidence score. The associations are meant to be specific and meaningful with associated proteins jointly contributing to a shared function. This does not necessarily mean that the proteins are physically binding each other, (B) significant biological process and (C) molecular function of upregulated genes.
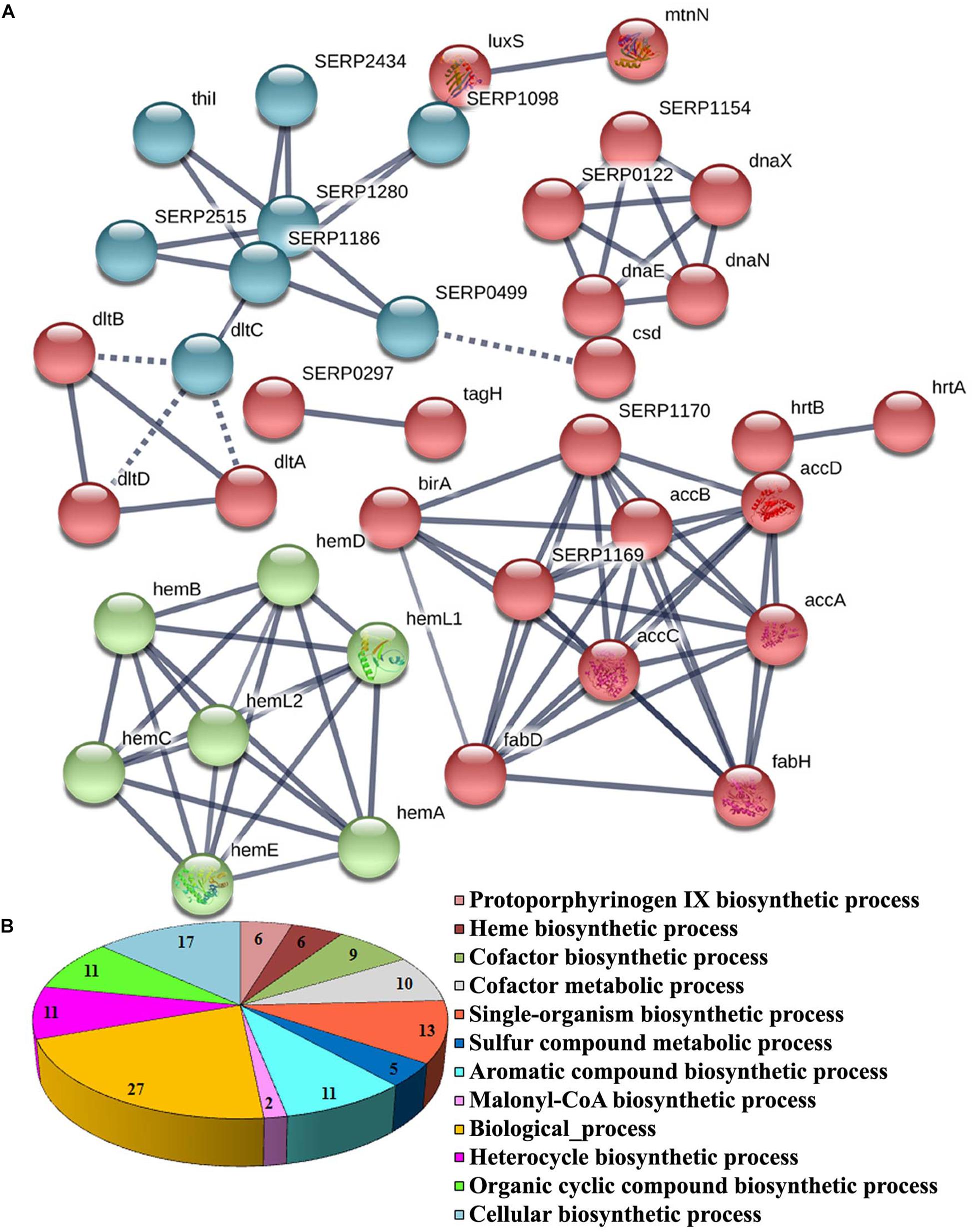
Figure 2. Significant GO annotation of downregulated genes identified by RNA-sequencing. (A) Interactome map predicted using STRING10.5. The network nodes represent the proteins encoded by the genes. Edges represent protein–protein associations with high confidence score. The associations are meant to be specific and meaningful with associated proteins jointly contributing to a shared function. This does not necessarily mean that the proteins are physically binding each other and (B) biological process of downregulated genes.
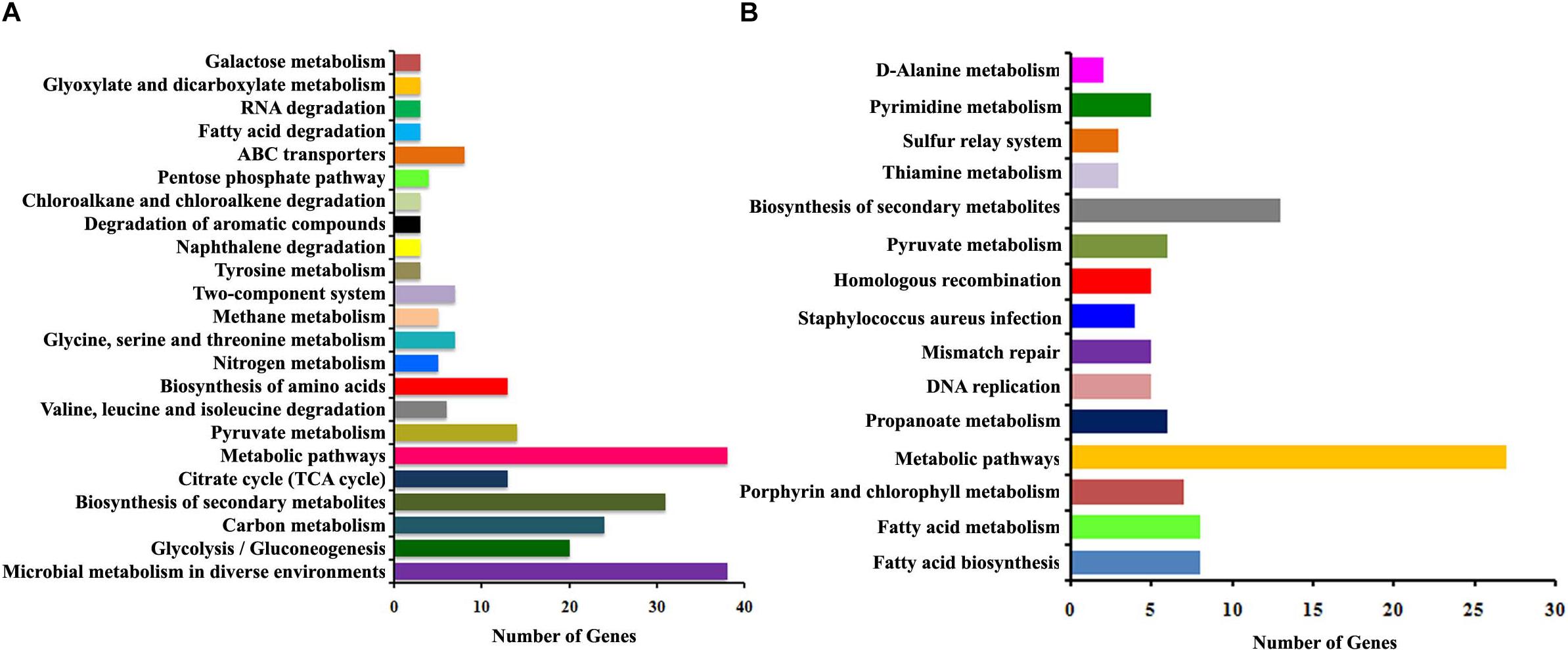
Figure 3. The histogram summarizing the enriched KEGG pathways of differentially expressed genes identified using RNA-sequencing. (A) Upregulated pathways and (B) downregulated pathways upon α-MG treatment.
Proteome Analysis
Proteomic profiling was employed to gain additional insights on the different cellular responses upon α-MG treatment. A total of 589 proteins were identified from all conditions, which corresponds to 23.51% of the predicted proteome (2505 proteins in UniProtKB database) of S. epidermidis RP62A. Prior to further analyses, Venn diagram (Supplementary Figure 3) was constructed to summarize the differences and similarities between proteins expressed in each conditions. It demonstrated that 492 proteins were expressed under all the conditions. Changes in proteome level were studied by comparing control cells with α-MG treated (0.7 MIC) cells at two different time points (10 and 30 min). Over all, differential expression (≥1.5-fold up- or down-regulation, p ≤ 0.05) of 89 proteins in response to α-MG treatment was observed: 34 proteins at 10 min (2 upregulated and 32 downregulated) and 70 proteins at 30 min (38 upregulated and 32 downregulated) (Table 2). However, only fifteen differentially expressed proteins (1 up- and 14 down-regulated) were found at both the time points. Of 89 proteins, 24 proteins were uncharacterized protein. The details of protein families and sub-cellular localization of uncharacterized proteins are listed in Supplementary Table 3. It was observed that most of the hypothetical proteins were localized in cytoplasmic membrane and cytoplasm (Supplementary Table 3). According to GO annotation, the upregulated and downregulated proteins are involved in various biological process and molecular function (Figure 4). The protein-protein interaction maps of upregulated and downregulated proteins are shown in Supplementary Figure 4. The KEGG pathway analysis predicted that the most strongly upregulated proteins are ribosomal proteins, and the most strongly downregulated proteins are involved in various metabolic pathways that include nucleotide excision repair, ABC transporters and phosphotransferase system (Figure 5). Besides, the GO annotation analysis of differentially regulated proteins using CELLO2GO is depicted in the Supplementary Figure 5. Among 89 differentially regulated proteins, 71, 15, and 11% were predicted to be cytoplasmic, membrane-associated, and extracellular proteins, respectively.
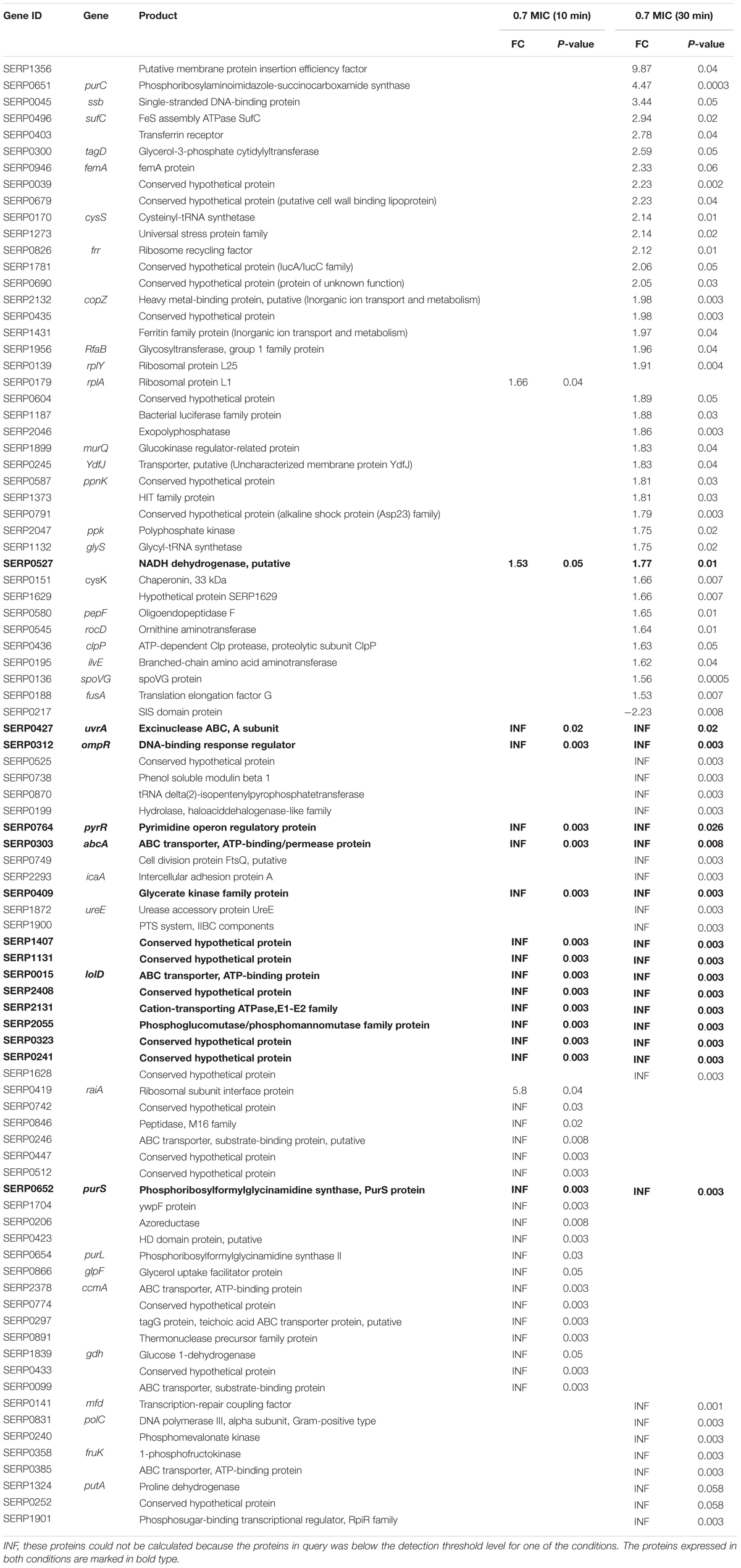
Table 2. List of differentially expressed proteins of S. epidermidis treated with α-MG identified using LC-MS/MS.
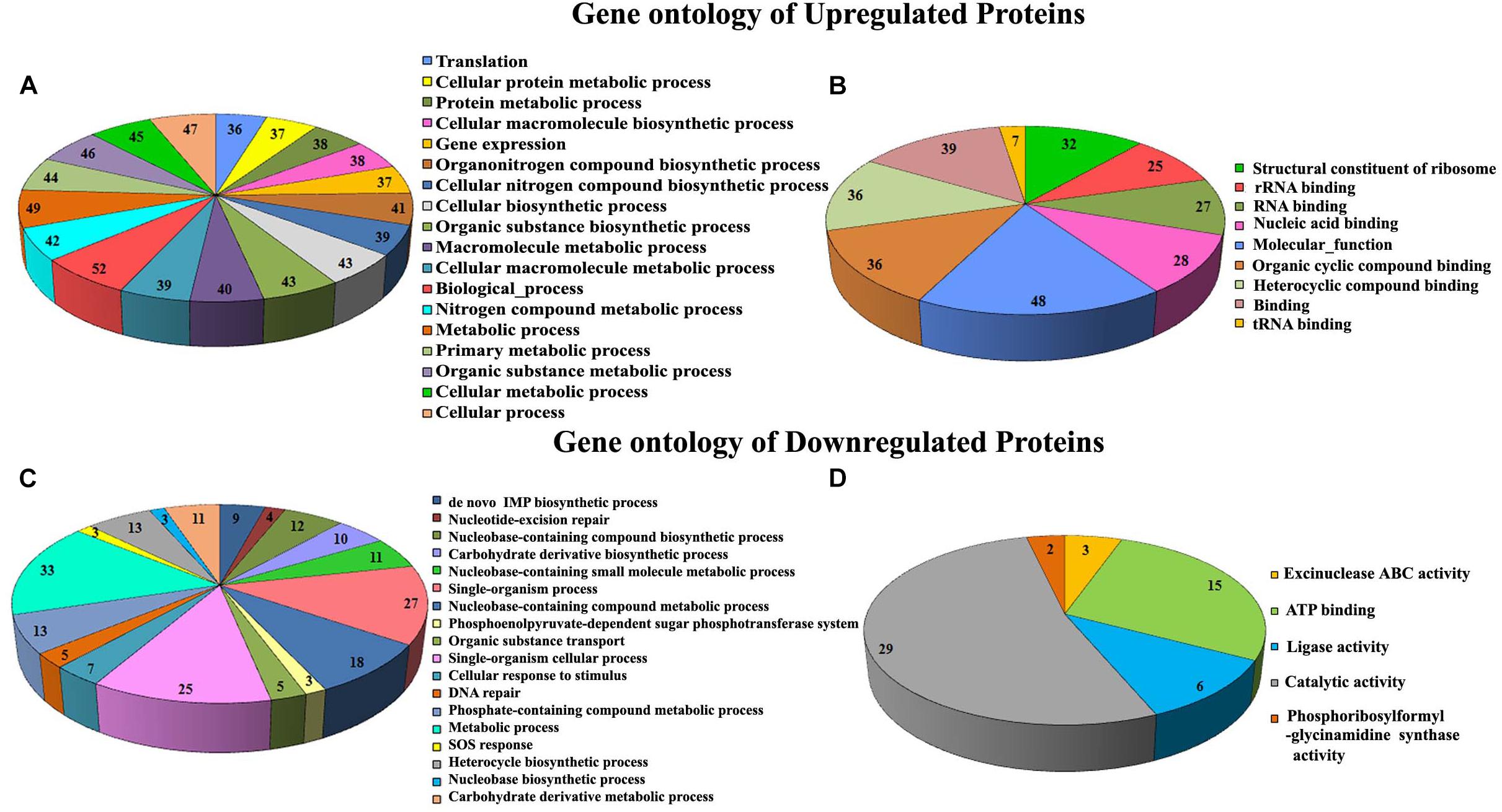
Figure 4. GO annotation of differentially expressed proteins of S. epidermidis upon α-MG treatment identified using LC-MS/MS analysis. Biological process (A,C) and molecular function (B,D).
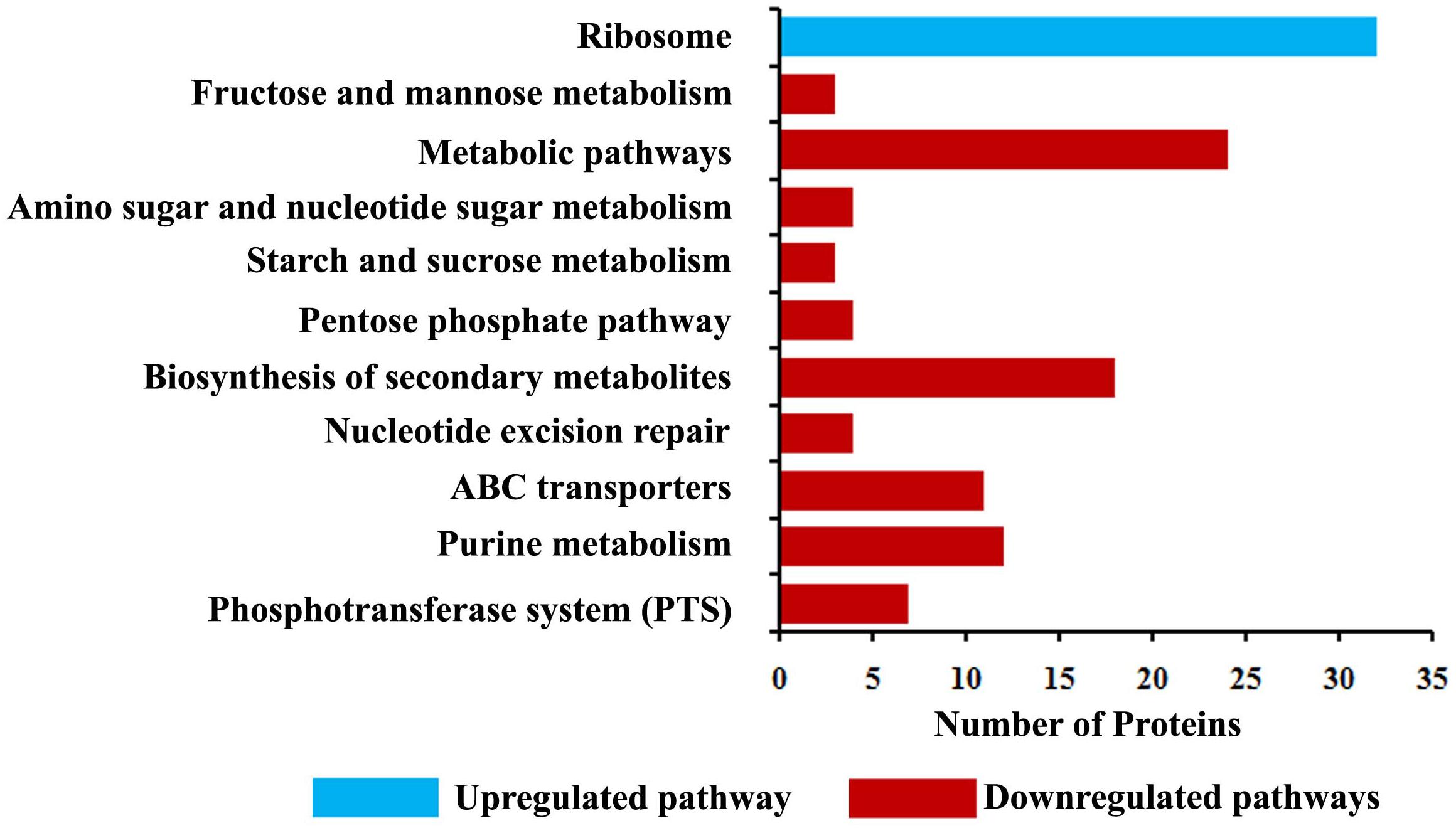
Figure 5. KEGG pathway analysis of differentially expressed proteins identified using LC-MS/MS analysis.
Validation of RNA-Sequencing and LC-MS/MS Data by qRT-PCR
The transcriptomic and proteomic results were further validated using qRT-PCR. qRT-PCR was performed with RNA isolated from bacteria under the similar experimental conditions used for the RNA-sequencing and LC-MS/MS analysis experiments. A total of 21 genes were selected randomly based on the functional categorization and KEGG pathway analysis. The obtained results were consistent with the transcriptomic and proteomic data (Figure 6), which not only validated the expression profiles of the selected genes, but also substantiated the reliability and accuracy of our transcriptome and proteome analyses. Based on the integrated transcriptome and proteome analyses and further validation through qRT-PCR, it was speculated that α-MG targets S. epidermidis through multifarious pathways viz., by principally targeting the cytoplasmic membrane integrity and by subsequently affecting fatty acid biosynthesis, DNA replication, DNA repair machinery, oxidative and cellular stress responses.
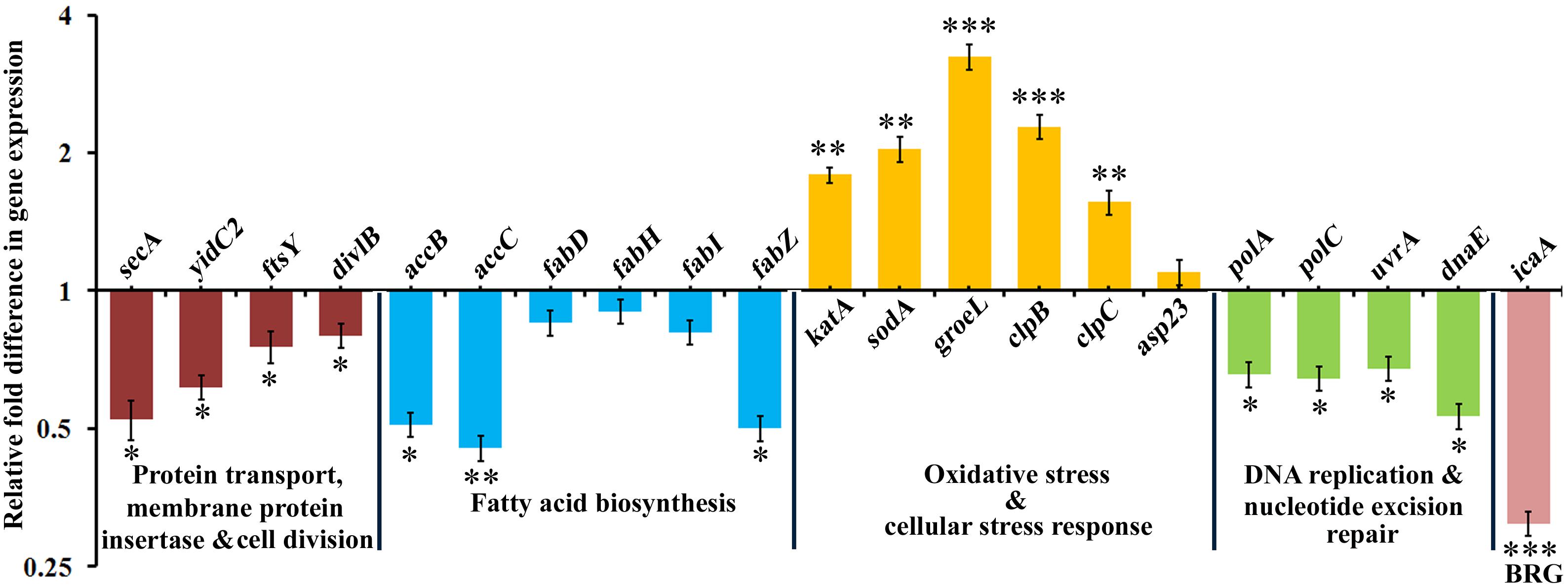
Figure 6. The qRT-PCR validation of differentially expressed genes which are randomly selected based on transcriptomic and proteomic approaches. BRG stands for biofilm regulatory gene. The data represented the mean ± standard deviation of three independent experiments. The statistical significance of data was determined by unpaired Student’s t-test. ∗P < 0.05, ∗∗P < 0.005, ∗∗∗P < 0.001.
Discussion
The combination of transcriptome and proteome analyses demonstrated a panoramic view of the antibacterial mechanism of α-MG on S. epidermidis. Koh et al. (2013) reported that the rapid bactericidal action of α-MG is comparable with cell membrane lytic cationic antimicrobial peptides (CAMPs). The presence of carbonyl and hydroxyl groups might confer the negative charge to α-MG (Ahmad et al., 2013). Unlike conventional antibiotics that interact with specific targets, many CAMPs bind to anionic bacterial surface and perturb the cyotplasmic membrane (Hancock, 2001). In addition, α-MG is known to interact with transmembrane precursor proteins via hydrogen bonding (Wang et al., 2012). In parallel with this report, α-MG strongly downregulated the yidC2 gene (Table 1), which is involved in the integration of nascent membrane protein with dependent from or independent from the Sec-translocase (van der Laan et al., 2003). The proper membrane biogenesis depends on YidC, wherein it acts as a protein insertase, facilitates protein folding, ensues proper topology and an assembly factor for transmembrane proteins (Wang and Dalbey, 2011). The downregulated gene yidC2 (encoding a membrane protein insertase) was found to interact with the Sec pathway proteins such as SecA, SecY and FtsY, and the cell division protein FtsQ (encoded by divlB). The data from qRT-PCR revealed that secA, ftsY and divlB were downregulated upon α-MG treatment (Figure 6). Though data on the Sec system of Staphylococcus spp. is scarce, secA has been shown to be essential for cell viability (Ji et al., 2001; Jin et al., 2015). The small analogs of Rose Bengal inhibited the secA1 and secA2 of S. aureus thereby reducing the toxin secretion and bypassing the negative effect of efflux pumps (Ji et al., 2001). In addition, MscL (large conductance mechanosensitive channel protein) plays a vital role in protecting cells from osmotic downshock (Booth and Louis, 1999), which was downregulated upon α-MG treatment (Table 1). Facey et al. (2007) suggested that signal recognition particle (SRP) plays an important role in targeting of MscL protein to the cytoplasmic membrane and hence, the deletion of SRP receptor FtsY had a significant effect on the insertion of MscL. Moreover, the depletion of YidC2 inhibits the translocation of protein across the membrane. This confirms that downregulation of YidC2 and FtsY expression could inhibit the insertion of membrane protein MscL. Hence, the downregulation of these very important cytoplasmic membrane associated genes/proteins further corroborate that α-MG elicits bacterial killing by affecting the integrity of cytoplasmic membrane.
α-MG induced the expression level of certain heat-shock proteins (ClpB, ClpC, and GroEL) that ultimately rescue the cells from the lethal effect of α-MG (Table 1 and Figure 6). In parallel with our observation, the enhanced expression of the groES and groEL genes was observed upon oxacillin treatment, suggesting that upregulation of these heat-shock protein encoding genes is an integral part of cell wall active-antibiotic stress response (Singh et al., 2001). Under stress conditions, the chaperons and heat-shock proteins are capable of reactivating and refolding denatured proteins. Acyldepsipeptides, a new class of antibiotics, activate ClpP to degrade the cell division protein FtsZ, thereby arresting cell division (Sass et al., 2011). In line with this report, it was hypothesized that α-MG decrease the expression level of cell division related proteins (FtsY and DivlB) by increasing the Clp protease activity. Further, α-MG treatment increased the expression level of asp23 encoding the alkaline shock protein (Table 1 and Figure 6). In S. aureus, the transcription of asp23 is used as a marker for the activity of alternative sigma factor SigmaB, which responds to various stress conditions by rapidly increasing its activity (Bischoff et al., 2001; Senn et al., 2005; Cebrian et al., 2009). Further, increased expression levels of the oxidative stress response genes, katA and sodA (encoding catalase and superoxide dismutase, respectively) were observed on α-MG treatment (Table 1 and Figure 6).
In addition to cytoplasmic membrane perturbation, α-MG seems to target very basic metabolic processes in order to kill the bacteria. Enzymes responsible for DNA replication play an indispensable role in cell growth and are therefore appropriate targets for antimicrobial agents. DNA polymerase I is encoded by the gene polA and two other important DNA replication specific enzymes, DNA polymerase III C and DNA polymerase III E are encoded by polC and dnaE. Both polC and dnaE are essential for chromosomal DNA replication in Gram-positive bacteria (Dervyn et al., 2001; Inoue et al., 2001). As reported by Inoue et al. (2001), inactivation of either polC or dnaE has a bactericidal effect on S. aureus. In addition, the downregulated genes uvrA and mfd have been shown to be involved in nucleotide excision repair machinery. UvrA detects the damaged DNA and the transcription repair coupling factor mfd attract the DNA-excision repair machinery to the damaged DNA (Ambur et al., 2009). Our transcriptomic and proteomic data shows the downregulation of polA, polC, dnaE, uvrA, and mfd genes upon α-MG treatment (Table 2 and Figure 6). Besides, the transcriptomic data shows the downregulation of the accB gene encoding a protein that interacts with AccC, AccB, AccA, AccD, FabF, FabG, FabH, FabI, and FabZ proteins (Table 1). These proteins are involved in fatty acid biosynthetic pathway (FASII), in which AccB is involved in the first committed step of FASII, catalyzing the formation of malonyl-CoA from acetyl-CoA. The qRT-PCR analysis also demonstrated the downregulation of all the selected genes involved in FASII pathway (Figure 6). In line with our report, platensimycin and platencin were shown to inhibit key FASII enzymes such as FabB/F and/or FabH against S. aureus, Streptococcus pneumonia, Enterococcus faecalis and Enterococcus faecium, which resulted in bacterial growth inhibition (Wang et al., 2006, 2007; Wright and Reynolds, 2007). In general, it is hypothesized that α-MG subsided the DNA replication, DNA repair machinery and fatty acid biosynthesis pathway which might further augment the bactericidal property of α-MG against S. epidermidis.
Furthermore, our transcriptomic and proteomic data shows the downregulation of tagG (encoding the teichoic acid ABC transporter protein) known to be involved in teichoic acids biosynthesis pathway (Tables 1, 2). The cell wall of gram-positive bacteria is mainly composed of peptidoglycan and Teichoic acids, both of which are important for maintaining the shape and structural integrity of the bacterial cell. Teichoic acids are anomeric polyglycerophosphate chains, which are exported via TagGH, a two component ABC transporter and then, either coupled with peptidoglycan (wall teichoic acids) or anchored from the cytoplasmic membrane (lipoteichoic acids). However, the D-alanylation modification regulates the interactions between bacterial cell membrane and CAMPs. For instance, increased D-alanylation of TAs provides resistance to CAMPs, possibly by increasing positive surface charge density. Our transcriptomic data suggested that the expression of dltA was clearly downregulated upon α-MG treatment (Table 1). The D-alanylation of TAs is directed by the dlt operon, where DltA begins the biosynthetic pathway by activating the D-alanine from aminoacyl adenylate. May et al. (2005) reported the analog of D-Ala aminoacyl adenylate as the inhibitor of DltA, that enhanced the susceptibility of Bacillus subtilis against cationic antibiotic vancomycin. In line with this report, our previous study signifies that the continuous exposure of α-MG does not induce resistance in planktonic as well as biofilm cells of S. epidermidis. This is possibly due to the downregulation of dltA which in turn averts positive surface charge density on bacterial membrane and facilitates the interaction between α-MG and bacterial cell membrane. Moreover, dltA mutant strain of S. aureus is unable to form biofilm on glass or polystyrene, which has been attributed by the inhibition of initial adherence and not accumulation, as the levels of polysaccharide intercellular adhesin (PIA) were unaltered (Gross et al., 2001). S. epidermidis and S. aureus have shown to possess icaABCD operon, wherein icaA encodes for N-acetylglucosaminyltransferase, an enzyme which is important for synthesis of PIA. The proteomic and qRT-PCR analyses showed downregulation of icaA which goes in parallel with our previous report (Sivaranjani et al., 2017), in which a less pronounced biofilm inhibition was observed at sub-MIC of α-MG. Of note, the mode of action of α-MG appears to involve compromising the cytoplasmic membrane integrity of Gram-positive bacteria. The cytoplasmic membrane perturbing ability of α-MG may also add credits in restricting the acquisition of bacterial resistance, as there is evidence suggesting that bacteria do not easily acquire resistance against membrane active antibacterial agents (Przybylski et al., 1998; Ooi et al., 2009).
Conclusion
Based on the integrated high throughput transcriptomic and proteomic analyses, the antibacterial mode of action of α-MG was identified to be more complex that targets multiple metabolic pathways. It was speculated that the principal target of α-MG seems to be cytoplasmic membrane which further orchestrate the downregulation of genes important for various metabolic pathways. α-MG downregulated the genes involved in FASII pathway, cell division, DNA replication, homologous recombination, mismatch repair, resistance development, biofilm, oxidative stress and cellular stress response, which are believed to be secondary targets of α-MG (Figure 7). As α-MG targets several metabolic pathways, resistance development by the bacterium will be minimal and thus α-MG may be further exploited for clinical trials. To our knowledge, this is one of the very first reports that potentiate the antibacterial activity of α-MG through an integrated transcriptomic and proteomic approach.
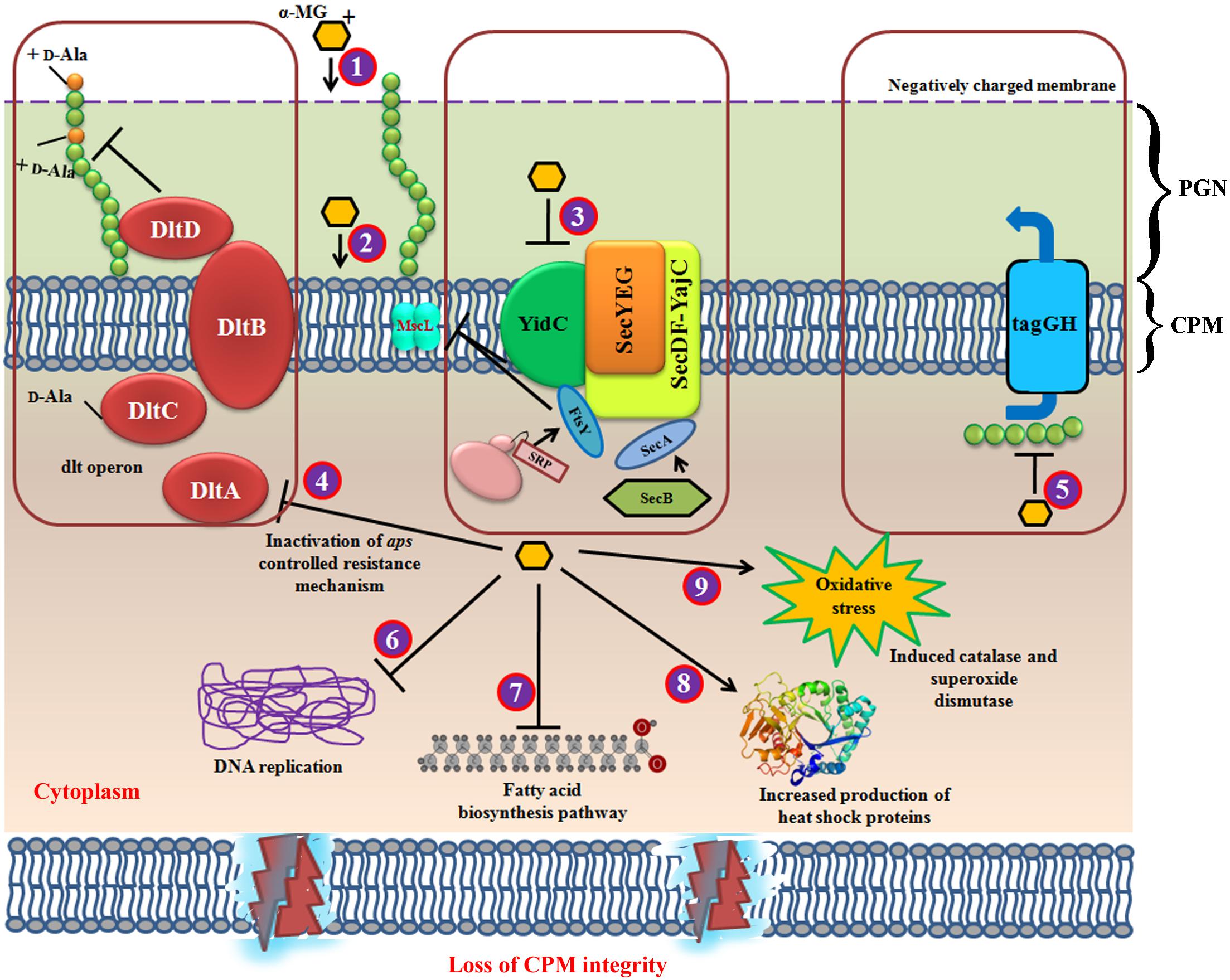
Figure 7. Schematic representation of multifarious antibacterial mode of action for α-MG. (1) α-MG shows electrostatic interaction with negatively charged bacterial membrane, where it binds with the bacterial inner membrane (2) in which, the strong hydrophobic interaction with lipid alkyl chain of cytoplasmic membrane might be the major driving force for the rapid bactericidal property. Further, α-MG interacts with the transmembrane precursor proteins via hydrogen bonding. (3) The downregulation of YidC2, SecA, FtsY, and MscL has evidenced that α-MG thwart the cytoplasmic membrane integrity. (4) The downregulation of dltA inhibits D-alanylation of anomeric teichoic acids and hence, the development of resistance against α-MG has been aborted. (5) TagG, a part of two component ABC transporter which exports the anomeric polyglycerophosphate chains (TAs) from cytoplasm. Inhibition of tagG shows that teichoic acid biosynthetic pathway has also been affected, because tagG is localized in cytoplasmic membrane. This might possibly due to the loss of cytoplasmic membrane integrity which resulted in decreased export of teichoic acids from cytoplasm. (6) DNA replication and mismatch repair mechanism has been downregulated upon α-MG treatment. (7) α-MG also inhibited fatty acid biosynthesis pathway (8) α-MG also enhanced the expression of heat shock proteins. (9) α-MG has also shown to increase the genes involved in oxidative stress response. CPM, cytoplasmic membrane; PGN, peptidoglycan.
In addition, as α-MG targets multifarious pathway, further in depth analysis viz., mutational and in vivo studies on each pathway can be evaluated to identify the primary and secondary targets of α-MG. This will pave the way to develop α-MG as one of the vital drug candidates to treat the infections caused by S. epidermidis. Further, combination of any known bioactive compounds or with effective antibiotics not only increases the activity of known bioactive compounds but also can possibly assist the clinical development of these bioactive compounds. Further, combinatorial therapy avoids the resistant development by the bacterial pathogens as it involves the usage of lower/shorter dosing regimens. Hence, combination of α-MG with other well-known phytochemicals or conventional antibiotics can be carried out to evaluate the bactericidal property on mature biofilms.
Author Contributions
MgS and CA designed the study. MgS, KL, and MkS performed the experiments and interpreted the data. MkS, PS, AR, and SP contributed to materials and reagents. MgS and CA drafted the manuscript. All authors approved the final manuscript.
Funding
This study was supported by Funding Academy of Finland (Grant No. 1288701).
Conflict of Interest Statement
The authors declare that the research was conducted in the absence of any commercial or financial relationships that could be construed as a potential conflict of interest.
Acknowledgments
Financial support to MgS by the Department of Science and Technology (DST), New Delhi in the form of DST-INSPIRE Fellowship [No.: DST/INSPIRE Fellowship/2013/461] and EMBO Short Term Fellowship (No. 7169) to carry out the work at Prof. Skurnik’s Lab, University of Helsinki, Finland is gratefully acknowledged. The authors are grateful to Computational and Bioinformatics Facility provided by the Alagappa University Bioinformatics Infrastructure Facility (funded by Department of Biotechnology, Government of India; File No. BT/BI/25/012/2012,BIF). Instrumentations facility provided by DST-PURSE [SR/PURSE Phase 2/38 (G)], DST-FIST [Grant No. SR/FST/LSI-639/2015(C)], RUSA 2.0 [F. 24-51/2014-U, Policy (TN Multi-Gen), Department of Education, GoI], and UGC-SAP [Grant No. F.5-1/2018/DRS-II (SAP-II)] are sincerely acknowledged.
Supplementary Material
The Supplementary Material for this article can be found online at: https://www.frontiersin.org/articles/10.3389/fmicb.2019.00150/full#supplementary-material
Footnotes
References
Ahmad, M., Yamin, B. M., and Lazim, A. M. (2013). A study on dispersion and characterisation of α-mangostin loaded pH sensitive microgel systems. Chem. Cent. J. 7:85. doi: 10.1186/1752-153X-7-85
Ambur, O. H., Davidsen, T., Frye, S. A., Balasingham, S. V., Lagesen, K., Rognes, T., et al. (2009). Genome dynamics in major bacterial pathogens. FEMS Microbiol. Rev. 33, 453–470. doi: 10.1111/j.1574-6976.2009.00173.x
Bischoff, M., Entenza, J. M., and Giachino, P. (2001). Influence of a functional sigB operon on the global regulators sar and agr in Staphylococcus aureus. J. Bacteriol. 183, 5171–5179. doi: 10.1128/JB.183.17.5171-5179.2001
Booth, I. R., and Louis, P. (1999). Managing hypoosmotic stress: aquaporins and mechanosensitive channels in Escherichia coli. Curr. Opin. Microbiol. 2, 166–169. doi: 10.1016/S1369-5274(99)80029-0
Carvalhais, V., Franca, A., Cerca, F., Vitorino, R., Pier, G. B., Vilanova, M., et al. (2014). Dormancy within Staphylococcus epidermidis biofilms: a transcriptomic analysis by RNA-seq. Appl. Microbiol. Biotechnol. 98, 2585–2596. doi: 10.1007/s00253-014-5548-3
Cebrian, G., Sagarzazu, N., Aertsen, A., Pagan, R., Condon, S., and Manas, P. (2009). Role of the alternative sigma factor sigma on Staphylococcus aureus resistance to stresses of relevance to food preservation. J. Appl. Microbiol. 107, 187–196. doi: 10.1111/j.1365-2672.2009.04194.x
Center for Disease Control Prevention [CDC] (2013). Antibiotic Resistance Threats in the United States, 2013. Atlanta: CDC.
Clinical Laboratory Standards Institute [CLSI] (2006). Methods for Dilution Antimicrobial Susceptibility Tests for Bacteria that Grow Aerobically; Approved Standard, CLSI Document M7-A7, 7th Edn. Wayne: Clinical and Laboratory Standards Institute.
Danzmann, L., Gastmeier, P., Schwab, F., and Vonberg, R. P. (2013). Health care workers causing large nosocomial outbreaks: a systematic review. BMC. Infect. Dis. 13:98. doi: 10.1186/1471-2334-13-98
Dervyn, E., Suski, C., Daniel, R., and Vonberg, R. P. (2001). Two essential DNA polymerases at the bacterial replication fork. Mol. Genet. Genomics 266, 564–571. doi: 10.1126/science.1066351
Dobin, A., Davis, C. A., Schlesinger, F., Drenkow, J., Zaleski, C., Jha, S., et al. (2013). STAR: ultrafast universal RNA-seq aligner. Bioinformatics 29, 15–21. doi: 10.1093/bioinformatics/bts635
Donlan, R. M., and Costerton, J. W. (2002). Biofilms: survival mechanisms of clinically relevant microorganisms. Clin. Microbiol. Rev. 15, 167–193. doi: 10.1128/CMR.15.2.167-193.2002
Dos Santos, B. S., da Silva, L. C., da Silva, T. D., Rodrigues, J. F., Grisotto, M. A., Correia, M. T., et al. (2016). Application of omics technologies for evaluation of antibacterial mechanisms of action of plant-derived products. Front. Microbiol. 7:1466. doi: 10.3389/fmicb.2016.01466
Facey, S. J., Neugebauer, S. A., Krauss, S., and Kuhn, A. (2007). The mechanosensitive channel protein MscL is targeted by the SRP to the novel YidC membrane insertion pathway of Escherichia coli. J. Mol. Biol. 365, 995–1004. doi: 10.1016/j.jmb.2006.10.083
Fei, X., Jo, M., Lee, B., Han, S.-B., Lee, K., Jung, J.-K., et al. (2014). Syntheis and xnthone derivatives based on α-mangostin and their biological evaluation for anti-cancer agents. Bioorg. Med. Chem. Lett. 24, 2062–2065. doi: 10.1016/j.bmcl.2014.03.047
Gomes, F., Teixeira, P., and Oliveira, R. (2014). Mini-review: Staphylococcus epidermidis as the most frequent cause of nosocomial infections: old and new fighting strategies. Biofouling 30, 131–141. doi: 10.1080/08927014.2013.848858
Gross, M., Cramton, S. E., Gotz, F., and Peschel, A. (2001). Key role of teichoic acid net charge in Staphylococcus aureus colonization of artificial surfaces. Infect. Immun. 69, 3423–3426. doi: 10.1128/IAI.69.5.3423-3426.2001
Ha, L. D., Hansen, P. E., Vang, O., Pham, H. D., and Nguyen, L. H. (2009). Cytotoxic geranylated xanthones and O-alkylated derivatives of alpha-mangostin. Chem. Pharm. Bull. 57, 830–834. doi: 10.1248/cpb.57.830
Hancock, R. E. (2001). Cationic peptides: effectors in innate immunity and novel antimicrobials. Lancet Infect. Dis. 1, 156–164. doi: 10.1016/S1473-3099(01)00092-5
Ibrahim, M. Y., Hashim, N. M., Mariod, A. A., Mohan, S., Abdulla, M. A., Abdelwahab, S. I., et al. (2016). α-Mangostin from Garcinia mangostana Linn: an updated review of its pharmacological properties. Arab. J. Chem. 9, 317–329. doi: 10.1016/j.arabjc.2014.02.011
Inoue, R., Kaito, C., Tanabe, M., Kamura, K., Akimitsu, N., and Sekimizu, K. (2001). Genetic identification of two distinct DNA polymerases, DnaE and PolC, that are essential for chromosomal DNA replication in Staphylococcus aureus. Science 294, 1716–1719.
Ji, Y., Zhang, B., Van, S. F., Warren, P., Woodnutt, G., Burnham, M. K. R., et al. (2001). Identification of critical staphylococcal genes using conditional phenotypes generated by antisense RNA. Science 293, 2266–2269. doi: 10.1126/science.1063566
Jin, J., Cui, J., Chaudhary, A. S., Hsieh, Y. H., Damera, K., Zhang, H., et al. (2015). Evaluation of small molecule SecA inhibitors against methicillin-resistant Staphylococcus aureus. Bioorg. Med. Chem. 23, 7061–7068. doi: 10.1016/j.bmc.2015.09.027
Khan, H., Jawad, M., Kamal, M. A., Baldi, A., Xiao, J., Nabavi, S. M., et al. (2018). Evidence and prospective of plant derived flavonoids as antiplatelet agents: strong candidates to be drugs of future. Food Chem. Toxicol. 119, 355–367. doi: 10.1016/j.fct.2018.02.014
Kinghorn, A. D., Pan, L., Fletcher, J. N., and Chai, H. (2011). The relevance of higher plants in lead compound discovery programs. J. Nat. Prod. 74, 1539–1555. doi: 10.1021/np200391c
Koh, J., Qiu, S., Zou, H., Lakshminarayanan, R., Li, J., Zhou, X., et al. (2013). Rapid bactericidal action of alpha-mangostin against MRSA as an outcome of membrane targeting. Biochim. Biophys. Acta 1828, 834–844. doi: 10.1016/j.bbamem.2012.09.004
Koh, J. J., Lin, S., Aung, T. T., Lim, F., Zou, H., Bai, Y., et al. (2015). Amino acid modified xanthone derivatives: novel, highly promising membrane-active antimicrobials for multidrug resistant gram-positive bacterial infections. J. Med. Chem. 58, 739–752. doi: 10.1021/jm501285x
Koh, J.-J., Zou, H., Lin, S., Lin, H., Soh, R. T., Lim, F. H., et al. (2016). Nonpeptidic amphiphilic xanthone derivatives: structure-activity relationship and membrane-targeting properties. J. Med. Chem. 59, 171–193. doi: 10.1021/acs.jmedchem.5b01500
Laallam, H., Boughediri, L., Bissati, S., Menasria, T., Mouzaoui, M. S., Hadjadj, S., et al. (2015). Modeling the synergistic antibacterial effects of honey characteristics of different botanical origins from the Sahara desert of Algeria. Front. Microbiol. 6:1239. doi: 10.3389/fmicb.2015.01239
Li, J., Liu, S., Koh, J. J., Zou, H., Lakshminarayanan, R., Bai, Y., et al. (2015). A novel fragment based strategy for membrane active antimicrobials against MRSA. Biochim. Biophys. Acta 1848, 1023–1031. doi: 10.1016/j.bbamem.2015.01.001
Macosko, E. Z., Basu, A., Satija, R., Nemesh, J., Shekhar, K., Goldman, M., et al. (2015). Highly parallel genome-wide expression profiling of individual cells using nanoliter droplets. Cell 161, 1202–1214. doi: 10.1016/j.cell.2015.05.002
Matsumoto, K., Akao, Y., Yi, H., Ohguchi, K., Ito, T., Tanaka, T., et al. (2004). Preferential target is mitochondria in alpha-mangostin-induced apoptosis in human leukemia HL60 cells. Bioorg. Med. Chem. 12, 5799–5806. doi: 10.1016/j.bmc.2004.08.034
May, J. J., Finking, R., Wiegeshoff, F., Weber, T. T., Bandur, N., Koert, U., et al. (2005). Inhibition of the D-alanine:D-alanyl carrier protein ligase from Bacillus subtilis increases the bacterium’s susceptibility to antibiotics that target the cell wall. FEBS J. 272, 2993–3003. doi: 10.1111/j.1742-4658.2005.04700.x
Newman, D. J., and Cragg, G. M. (2012). Natural products as sources of new drugs over the 30 years from 1981 to 2010. J. Nat. Prod. 75, 311–335. doi: 10.1021/np200906s
Nguyen, P. T., Falsetta, M. L., Hwang, G., Gonzalez-Begne, M., and Koo, H. (2014). α-Mangostin disrupts the development of Streptococcus mutans biofilms and facilitates its mechanical removal. PLoS One 9:e111312. doi: 10.1371/journal.pone.0111312
Nguyen, P. T. M., and Marquis, R. E. (2011). Antimicrobial actions of α-mangostin against oral streptococci. Can. J. Microbiol. 57, 217–225. doi: 10.1139/W10-122
Ooi, N., Miller, K., Hobbs, J., Rhys-Williams, W., Love, W., and Chopra, I. (2009). XF-73, a novel antistaphylococcal membrane-active agent with rapid bactericidal activity. J. Antimicrob. Chemother. 64, 735–740. doi: 10.1093/jac/dkp299
Parra-Ruiz, J., Bravo-Molina, A., Pena-Monje, A., and Hernandez-Quero, J. (2012). Activity of linezolid and high-dose daptomycin, alone or in combination, in an in vitro model of Staphylococcus aureus biofilm. J. Antimicrob. Chemother. 67, 2682–2685. doi: 10.1093/jac/dks272
Pohlit, A. M., Lima, R. B., Frausin, G., Silva, L. F., Lopes, S. C., Moraes, C. B., et al. (2013). Amazonian plant natural products: perspectives for discovery of new antimalarial drug leads. Molecules 18, 9219–9240. doi: 10.3390/molecules18089219
Przybylski, R., Lee, Y. C., and Eskin, N. A. M. (1998). Antioxidant and radical scavenging activities of buckwheat seed components. J. Am. Oil Chem. Soc. 75, 1595–1601. doi: 10.1007/s11746-998-0099-3
Reddy, P. J., Ray, S., Sathe, G. J., Prasad, T. S., Rapole, S., Panda, D., et al. (2015). Proteomics analyses of Bacillus subtilis after treatment with plumbagin, a plant-derived naphthoquinone. OMICS 19, 12–23. doi: 10.1089/omi.2014.0099
Reiter, K. C., Sant’Anna, F. H., and d’Azevedo, P. A. (2014). Upregulation of icaA, atlE and aap genes by linezolid but not vancomycin in Staphylococcus epidermidis RP62A biofilms. Int. J. Antimicrob. Agents 43, 248–253. doi: 10.1016/j.ijantimicag.2013.12.003
Robinson, M. D., McCarthy, D. J., and Smyth, G. K. (2010). edgeR: a Bioconductor package for differential expression analysis of digital gene expression data. Bioinformatics 26, 139–140. doi: 10.1093/bioinformatics/btp616
Roper, D. I., Huyton, T., Vagin, A., and Dodson, G. (2000). The molecular basis of vancomycin resistance in clinically relevant enterococci: crystal structure of D-alanyl-D-lactate ligase (VanA). Proc. Natl. Acad. Sci. U.S.A. 97, 8921–8925. doi: 10.1073/pnas.150116497
Sass, P., Josten, M., Famulla, K., Schiffer, G., Sahl, H. G., Hamoen, L., et al. (2011). Antibiotic acyldepsipeptides activate ClpP peptidase to degrade the cell division protein FtsZ. Proc. Natl. Acad. Sci. U.S.A. 108, 17474–17479. doi: 10.1073/pnas.1110385108
Satthakarn, S., Chung, W. O., Promsong, A., and Nittayananta, W. (2015). Houttuynia cordata modulates oral innate immune mediators: potential role of herbal plant on oral health. Oral Dis. 21, 512–518. doi: 10.1111/odi.12313
Senn, M. M., Giachino, P., Homerova, D., Steinhuber, A., Strassner, J., Kormanec, J., et al. (2005). Molecular analysis and organization of the sigma B operon in Staphylococcus aureus. J. Bacteriol. 187, 8006–8019. doi: 10.1128/JB.187.23.8006-8019.2005
Singh, V. K., Jayaswal, R. K., and Wilkinson, B. J. (2001). Cell wall-active antibiotic induced proteins of Staphylococcus aureus identified using a proteomic approach. FEMS Microbiol. Lett. 199, 79–84. doi: 10.1016/S0378-1097(01)00163-X
Sivaranjani, M., Prakash, M., Gowrishankar, S., Rathna, J., Pandian, S. K., and Ravi, A. V. (2017). In vitro activity of alpha-mangostin in killing and eradicating Staphylococcus epidermidis RP62A biofilms. Appl. Microbiol. Biotechnol. 101, 3349–3359. doi: 10.1007/s00253-017-8231-7
van der Laan, M., Urbanus, M. L., Ten Hagen-Jongman, C. M., Nouwen, N., Oudega, B., Harms, N., et al. (2003). A conserved function of YidC in the biogenesis of respiratory chain complexes. Proc. Natl. Acad. Sci. U.S.A. 100, 5801–5806. doi: 10.1073/pnas.0636761100
Varjosalo, M., Keskitalo, S., Van Drogen, A., Nurkkala, H., Vichalkovski, A., Aebersold, R. et al. (2013). The protein interaction landscape of the human CMGC kinase group. Cell Rep. 3, 1306–1320. doi: 10.1016/j.celrep.2013.03.027
Veeresham, C. (2012). Natural products derived from plants as a source of drugs. J. Adv. Pharm. Technol. Res. 3, 200–201. doi: 10.4103/2231-4040.104709
Wang, J., Kodali, S., Lee, S. H., Galgoci, A., Painter, R., Dorso, K., et al. (2007). Discovery of platencin, a dual FabF and FabH inhibitor with in vivo antibiotic properties. Proc. Natl. Acad. Sci. U.S.A. 104, 7612–7616. doi: 10.1073/pnas.0700746104
Wang, J., Soisson, S. M., Young, K., Shoop, W., Kodali, S., Galgoci, A., et al. (2006). Platensimycin is a selective FabF inhibitor with potent antibiotic properties. Nature 441, 358–361. doi: 10.1038/nature04784
Wang, P., and Dalbey, R. E. (2011). Inserting membrane proteins: the YidC/Oxa1/Alb3 machinery in bacteria, mitochondria, and chloroplasts. Biochim. Biophys. Acta 1808, 866–875. doi: 10.1016/j.bbamem.2010.08.014
Wang, Y., Xia, Z., Xu, J. R., Wang, Y. X., Hou, L. N., Qiu, Y., et al. (2012). Alpha-mangostin, a polyphenolic xanthone derivative from mangosteen, attenuates beta-amyloid oligomers-induced neurotoxicity by inhibiting amyloid aggregation. Neuropharmacology 62, 871–881. doi: 10.1016/j.neuropharm.2011.09.016
Wright, H. T., and Reynolds, K. A. (2007). Antibacterial targets in fatty acid biosynthesis. Curr. Opin. Microbiol. 10, 447–453. doi: 10.1016/j.mib.2007.07.001
Xu, D., Nie, Y., Liang, X., Ji, L., Hu, S., You, Q., et al. (2013). A concise and efficient total synthesis of α-mangostin and β-mangostin from Garcinia mangostana. Nat. Prod. Commun. 8, 1101–1103.
Yu, N. Y., Wagner, J. R., Laird, M. R., Melli, G., Rey, S., Lo, R., et al. (2010). PSORTb 3.0: improved protein subcellular localization prediction with refined localization subcategories and predictive capabilities for all prokaryotes. Bioinformatics 26, 1608–1615. doi: 10.1093/bioinformatics/btq249
Yu, C. S., Cheng, C.-W., Su, W.-C., Chang, K.-C., Huang, S.-W., Hwang, J.-K., et al. (2014). CELLO2GO: a web server for protein subCELlular localization prediction with functional gene ontology annotation. PLoS One 9:e99368. doi: 10.1371/journal.pone.0099368
Keywords: RNA-sequencing, LC-MS/MS, bactericidal, alpha-mangostin, cytoplasmic membrane
Citation: Sivaranjani M, Leskinen K, Aravindraja C, Saavalainen P, Pandian SK, Skurnik M and Ravi AV (2019) Deciphering the Antibacterial Mode of Action of Alpha-Mangostin on Staphylococcus epidermidis RP62A Through an Integrated Transcriptomic and Proteomic Approach. Front. Microbiol. 10:150. doi: 10.3389/fmicb.2019.00150
Received: 27 June 2018; Accepted: 21 January 2019;
Published: 06 February 2019.
Edited by:
Santi M. Mandal, Indian Institute of Technology Kharagpur, IndiaReviewed by:
Nagendran Tharmalingam, Alpert Medical School, United StatesPiyush Baindara, University of Arkansas for Medical Sciences, United States
Copyright © 2019 Sivaranjani, Leskinen, Aravindraja, Saavalainen, Pandian, Skurnik and Ravi. This is an open-access article distributed under the terms of the Creative Commons Attribution License (CC BY). The use, distribution or reproduction in other forums is permitted, provided the original author(s) and the copyright owner(s) are credited and that the original publication in this journal is cited, in accordance with accepted academic practice. No use, distribution or reproduction is permitted which does not comply with these terms.
*Correspondence: Mikael Skurnik, bWlrYWVsLnNrdXJuaWtAaGVsc2lua2kuZmk= Arumugam Veera Ravi, YXZlZXJhcmF2aUByZWRpZmZtYWlsLmNvbQ==