- 1Key Laboratory of Systems Bioengineering (MOE), Frontier Science Center for Synthetic Biology, School of Chemical Engineering and Technology, Tianjin University, Tianjin, China
- 2Department of Brewing Engineering, Moutai Institute, Renhuai, China
- 3Tianjin Engineering Research Center of Microbial Metabolism and Fermentation Process Control, Tianjin University of Science and Technology, Tianjin, China
- 4College of Food Science and Technology, Hainan University, Haikou, China
Microbial fuel cells (MFCs) are eco-friendly bio-electrochemical reactors that use exoelectrogens as biocatalyst for electricity harvest from organic biomass, which could also be used as biosensors for long-term environmental monitoring. Glucose and xylose, as the primary ingredients from cellulose hydrolyzates, is an appealing substrate for MFC. Nevertheless, neither xylose nor glucose can be utilized as carbon source by well-studied exoelectrogens such as Shewanella oneidensis. In this study, to harvest the electricity by rapidly harnessing xylose and glucose from corn stalk hydrolysate, we herein firstly designed glucose and xylose co-fed engineered Klebsiella pneumoniae-S. oneidensis microbial consortium, in which K. pneumoniae as the fermenter converted glucose and xylose into lactate to feed the exoelectrogens (S. oneidensis). To produce more lactate in K. pneumoniae, we eliminated the ethanol and acetate pathway via deleting pta (phosphotransacetylase gene) and adhE (alcohol dehydrogenase gene) and further constructed a synthesis and delivery system through expressing ldhD (lactate dehydrogenase gene) and lldP (lactate transporter gene). To facilitate extracellular electron transfer (EET) of S. oneidensis, a biosynthetic flavins pathway from Bacillus subtilis was expressed in a highly hydrophobic S. oneidensis CP-S1, which not only improved direct-contacted EET via enhancing S. oneidensis adhesion to the carbon electrode but also accelerated the flavins-mediated EET via increasing flavins synthesis. Furthermore, we optimized the ratio of glucose and xylose concentration to provide a stable carbon source supply in MFCs for higher power density. The glucose and xylose co-fed MFC inoculated with the recombinant consortium generated a maximum power density of 104.7 ± 10.0 mW/m2, which was 7.2-folds higher than that of the wild-type consortium (12.7 ± 8.0 mW/m2). Lastly, we used this synthetic microbial consortium in the corn straw hydrolyzates-fed MFC, obtaining a power density 23.5 ± 6.0 mW/m2.
Introduction
Microbial electrochemical technologies are green and sustainable that enabled many practical applications in environments and energy fields (Wang and Ren, 2013; Wang et al., 2015), including microbial fuel cells (MFCs) to harvest electricity production from organic wastes treatment (Bond et al., 2002; Lovley, 2006; Logan, 2009; Logan and Rabaey, 2012), microbial electrolysis cells to product hydrogen (Cheng and Logan, 2007; Kadier et al., 2016), microbial electrosynthesis to produce value-added chemicals and biofuels from CO2 bio-reduction (Rabaey and Rozendal, 2010; Li et al., 2012; Liu et al., 2016), and MFC-based biosensors for remote environmental monitoring in long-term (Golitsch et al., 2013), and so on. Meanwhile, MFCs are interesting devices to study interspecies extracellular electron transfer in mixed microbial consortia (Katoa et al., 2012; Ha et al., 2017). A large amount of efforts had been made to design MFCs that could harvest electricity from brewery wastewaters (Wen et al., 2010), human feces (Du et al., 2011), marine putrefaction (Tender et al., 2002), natural gas (McAnulty et al., 2017; Yamasaki et al., 2018), and lignocellulose biomass (Mohan et al., 2016), etc. Cellulose is the most abundant biomass in the world, the hydrolyzates of which are rich in glucose and xylose (Payne et al., 2015; Kracher et al., 2016; Taylor et al., 2018). Thus, the conversion of xylose and glucose to electricity energy by designing novel MFCs has received much attention in recent years (Yang et al., 2015b; Lin et al., 2016; Li et al., 2017; Liu et al., 2017).
Shewanella oneidensis, one of the most well-studied exoelectrogens (Hau and Gralnick, 2007; Fredrickson et al., 2008; Li et al., 2018a,c), is capable of conducting extracellular electrons transfer (EET) to anodes via direct electron transfer pathways mediated by c-type cytochromes, and electron transfer pathways mediated by diffusible electron shuttles (Shi et al., 2016; Kumar et al., 2017). Nevertheless, the wild-type (WT) S. oneidensis could only utilize very limited substrates (i.e., acetate, lactate, and pyruvate) as their carbon and energy sources, while common pentoses or hexoses from cellulose could not be utilized by the WT S. oneidensis due to its incomplete sugar metabolism (Pinchuk et al., 2009; Flynn et al., 2012), which enormously restricted the wide applications of S. oneidensis. To use xylose and glucose as substrates in MFC for harvesting electricity, a number of synthetic biology and microbial consortium strategies were developed. Li et al. successfully constructed engineered S. oneidensis by assembling the xylose transporters from Candida intermedia with one of intracellular oxidoreductase pathway from Schefersomyces stipites (Li et al., 2017). Furthermore, the glucose metabolic pathways from Zymomonas mobilis were heterogeneously expressed into S. oneidensis, allowing it to use glucose as the sole carbon and energy source (Choi et al., 2014). However, these efforts only used either glucose or xylose for harvesting energy, not achieving co-utilization of pentoses and hexoses from cellulose hydrolyzates for electricity production. Another efficient strategy was to employ mixed microbial cultures to derive electricity from cellulose hydrolyzates in MFCs. Undefined mixed electricity-producing microbial communities in MFCs with various biomass hydrolysates including wheat straw hydrolysate (Zhang et al., 2009), rapeseed straw hydrolysate (Jablonska et al., 2016), rice straw hydrolysate (Wang et al., 2014) were used for electricity generation. However, it is hard to further improve the power generation of such undefined microbial mixture due to the complicated mechanisms of the microbial metabolic interactions and ambiguous EET among fermenters and exoelectrogens. However, recent studies demonstrated that K. pneumonia, one of the most well-established model microorganisms (Kumar and Park, 2017), was capable of utilizing a wide spectrum of substrates as carbon sources (such as xylose, glucose, and glycerol, etc.) to synthesize lactate (Feng et al., 2014). Also, K. pneumonia exhibited high growth rate, which endowed it more metabolic advantages over other fermentative strains used in electrochemical systems. Furthermore, biotechnological applications of K. pneumonia will also require attenuation of its pathogenicity and reduction of biosafety concerns. Many virulence factors that contribute to its pathogenicity including lipopolysaccharide (LPS), capsular antigens, fimbrial adhesins, siderophores, and O antigens, have been inactivated by genetically engineering approaches (Kumar and Park, 2017).
To further promote bioelectricity generation efficiency from cellulose hydrolyzates, we herein designed a rationally engineered microbial consortium based on the principle of “division-of-labor” (Liu et al., 2017), in which metabolic interaction modes between the fermenter (K. pneumoniae) and the exoelectrogen (S. oneidensis) were increased to achieve an efficient conversion of cellulose hydrolyzates to bioelectricity energy. As shown in Figure 1, we rationally engineered the consortium strains by redirecting carbon flux distribution of the K. pneumoniae and improving extracellular electron transfer of the S. oneidensis, respectively. To promote more carbon flux to lactate biosynthesis of K. pneumoniae, we firstly knocked out the phosphotransacetylase gene (pta) after deleting the alcohol dehydrogenase gene (adhE) to simultaneously eliminate the ethanol and acetate pathway (Guo et al., 2014). Then, a synthesis and delivery system, including a lactate dehydrogenase encoded by ldhD gene from Lactobacillus bulgaricus and a lactate transporter encoded by lldP gene from Escherichia coli (Li et al., 2015), was expressed to accelerate the supply of lactate. To improve the electrons transfer of S. oneidensis, a biosynthetic flavins pathway from Bacillus subtilis was expressed in a highly hydrophobic S. oneidensis strain CP-S1, which not only improved direct-contacted EET via enhancing S. oneidensis adhesion to the carbon electrode but also accelerated the flavins-mediated EET via increasing flavins synthesis. As a result, the mixed sugars (glucose and xylose) and cellulose hydrolyzates-fed MFC, inoculated with the engineered K. pneumonia-S. oneidensis, produced a maximum power density of 104.7 ± 10.0 mW/m2 and 23.5 ± 6.0 mW/m2. This synthetic microbial consortium successfully utilized cellulose hydrolyzates to harvest electricity, laying the foundation for the efficient conversion of biomass to generate power in MFCs.
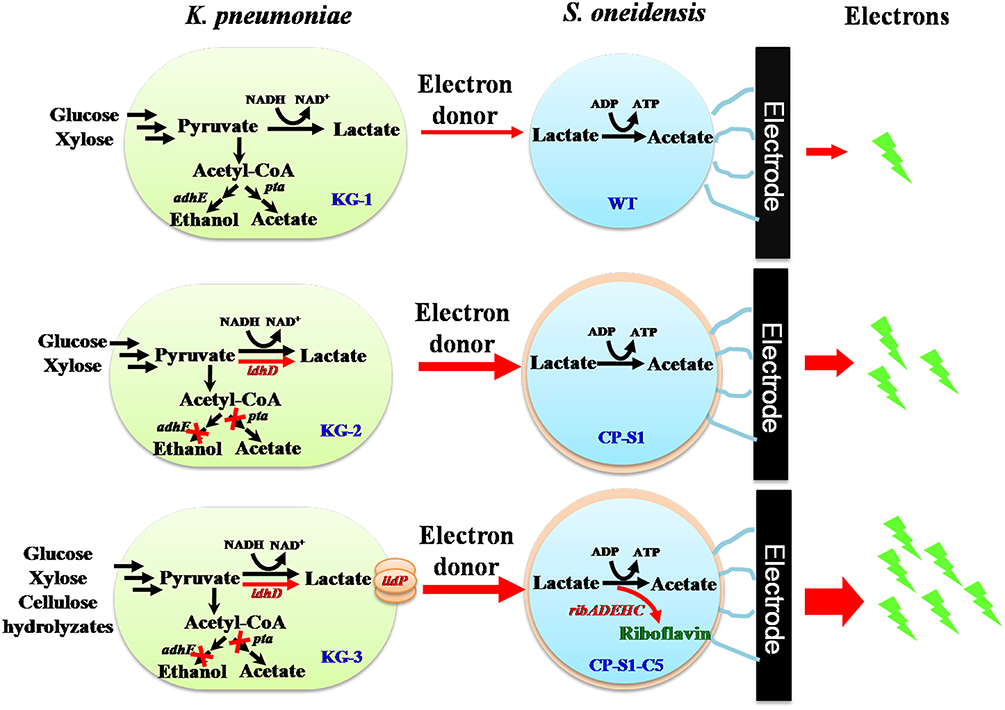
Figure 1. Schematic of the metabolic interaction of the engineered microbial consortia with K. pneumoniae S. oneidensis in a hierarchical way. Lactate was produced by K. pneumoniae from glucose and xylose which was fed to S. oneidensis as carbon source and electron donor to generate electricity in MFCs. To direct more carbon flux to lactate biosynthesis of K. pneumoniae, we eliminated the ethanol and acetate pathway via deleting phosphotransacetylase gene (pta) and alcohol dehydrogenase gene (adhE) and further constructed a synthesis and delivery system through expressing a lactate dehydrogenase gene (ldhD) and a lactate transporter gene (lldP). To increase S. oneidensis adhesion to the carbon electrode to compete the surface of electrode and further facilitate flavin-mediated electron transfer, a biosynthetic flavins pathway (from Bacillus subtilis) was expressed in a highly hydrophobic S. oneidensis strain CP-S1.
Materials and Methods
In vitro Gene Synthesis
The gene sequences of ldhD from L. bulgaricus ATCC11842 and the lldP from E. coli K-12 strain MG1655 were extracted from the NCBI database (www.ncbi.nlm.nih.gov/gene/) (Table S1). To prevent shortage of tRNAs for rare codons, we used a Java codon adaption tool (JCAT) to obtain the optimal sequences for K.pneumoniae (Yang et al., 2015a). The ldhD gene and lldP gene were then in vitro synthesized. Subsequently, the sequences of ldhD and lldP were linked in vector pUC18K, obtaining recombinant plasmid pUC18K-ldhD-lldP. The designed gene sequences were synthesized and verified in vitro. All the plasmid construction was performed in E. coli Trans T1.
Strain Construction and Culture
The KG-2 strain was obtained by deleting the adhE gene and the pta gene from KG-1 by following a previously established procedure (Guo et al., 2014). The recombinant K. pneumoniae strain KG-3 was engineered by electro-transferring the plasmid pUC18K-ldhD-lldP into KG-2. Two millimeter electroporate chamber and pulser voltage selected 1.8 kV were used in this electro-transferring process. The plasmid pYYDT-C5 harboring the flavin synthesis genes (ribADEHC) from B. subtilis (Yang et al., 2015a) was transformed into E. coli WM3064, and then was transferred into a highly hydrophobic S. oneidensis strain CP-S1 by conjugation. All stains and plasmids used in this study are listed in Table 1.
MFC Setup
The H-type MFCs were separated by Nafion 117 membranes, which were pretreated as those used in the previous study. The anode and cathode were composed of carbon cloth for anode (2.5 cm × 2.5 cm) and cathode (2.5 cm × 3 cm). The anodic electrolyte consisted of M9 buffer (Table S2) supplemented with definite carbon source and 5% (v/v) LB broth. The cathodic electrolyte was made of 50 mM KH2PO4, 50 mM K2HPO4, and 50 mM K3[Fe(CN)6] solution. The amplification cultured cells (OD600 = 2.0) were centrifuged, collected, and dispersed the anode chambers. To ensure consistent culture condition for different MFCs, 50 μg/mL kanamycin and 0.2 mM IPTG were supplemented in anodic electrolyte. All the MFCs were incubated at 30°C, and the voltage generation was measured by a digital multimeter (DT9205A) across the external loading a 2 kΩ resistor in MFCs.
Quantification of Metabolites
The metabolites in the shake flasks were analyzed using a high performance liquid chromatography (HPLC) system equipped with a refractive index detector. All samples and standard solutions were diluted and filtered by a 0.22 μm filter prior to HPLC assay. To analyses glucose, xylose, lactate, acetate, and ethanol, 5 mM sulfuric acid was used as the mobile phase (0.6 mL/min) through the Aminex HPX-87H ion-exchange column (Bio-Rad) which was incubated at 45°C, and detection was performed with the Waters 2414 refractive index detector. In addition, the determination of riboflavin concentration can refer to our previous method (Li et al., 2018a).
Bio-Electrochemical Analysis
Electrochemical analyses were performed on a CHI 1000C multichannel potentiostat (CH Instrument, Shanghai, China), when MFCs reached the pseudo-steady state. Cyclic voltammetry (CV) was conducted on a three-electrode configuration with the cathode as the counter electrode, the anode as the working electrode, and the Ag/AgCl (vs. SHE) as the reference electrode. To obtain the maximum power density and the polarization curves, linear sweep voltammetry (LSV) analysis was also performed on the CHI 1000C multichannel potentiostat.
The actually recovered coulombs were determined by integrating the current (I) over a period of batch cycle (tb). Such that the coulombic efficiency can be evaluated as Yan et al. (2015); Li et al. (2018a,c):
where MS (g/mol) is the molecular weight of the substrate, F is the Faraday's constant (98.485 C/mol of electrons), C is the symbol of coulomb, I (A) is the current, tb (s) is the time period of a batch cycle, bES is the stoichiometric number of moles of electrons produced per mole of substrate (glucose: bES = 16 when acetate was used; xylose: bES = 12 when acetate was used), VAn (L) is the volume of liquid in the anode compartment, and Δc (g/L) is the change in substrate concentration over a bath cycle time.
Biofilms Characterization
To measure the composition of microbial consortium, anode carbon were sampled and assayed by colony forming unit (CFU) counting. The anode was placed in a 50 mL test tube containing 5 mL of PBS (pH = 7.2) solution, vortexed oscillation for 2 min. A series of dilutions were spread onto LB agar plates, and incubated at 30°C for 36 h. The colony of S. oneidensis and K. pneumoniae was dark orange and white, respectively.
Preparation of Corn Straw Hydrolysates
Corn straw (CS) used in this study was harvested in the suburb of Tianjin, China. CS was milled coarsely using a beater pulverizer and the fractions between 20 and 80 meshes were screened. The CS was further transformed to hydrolysates upon pretreatment and enzymatic hydrolysis. The procedure of pretreatment was carried out by following a previously published procedure with minor modifications (Li et al., 2016, 2018c). And the enzymatic hydrolysis experiments were performed in accordance with the NREL standard protocol (LAP-009). After enzymatic hydrolysis at 30°C and 200 rpm for 72 h in an orbital incubator, the mixture was centrifuged at 12,000 rpm for 5 min to separate the hydrolysates from solid residues. The hydrolysates were frozen at −20°C for subsequent sugar analysis.
Results and Discussion
Increase Lactate Production From K. pneumoniae and Strengthen Flavins Biosynthesis From S. oneidensis
As the fermenter, K. pneumoniae could utilize glucose or xylose as a carbon source to produce lactate, concurrently producing a considerable amount of by-products, i.e., ethanol and acetate (Guo et al., 2014). Therefore, to drive more glucose or xylose to synthesize lactate, we eliminated the ethanol and acetate biosynthesis pathways by knocking out the gene adhE encoding the alcohol dehydrogenase and gene pta encoding phosphotransacetylase in K. pneumoniae. However, interruption of ethanol biosynthesis would accumulate abundant intracellular reducing equivalent (NADH), causing unbalancing of intracellular redox, which further inhibited cell viability. To solve this redox unbalance and increase lactate synthesis, we expressed the ldhD gene encoding lactate dehydrogenase from L. bulgaricus, thus consuming the excess NADH. Concurrently, a lactate transporter encoded by lldP gene from E. coli was introduced in K. pneumonia to increase the lactate transportation across the hydrophobic cell membrane. Thus, as shown in Figures 2A–C, compared with the wild K. pneumonia KG-1, the recombinant K. pneumoniae strain KG-3 expressed lactate biosynthesis pathway, deleted by-products synthesis and produced a significant amount of lactate, almost in the absence of ethanol and acetate.
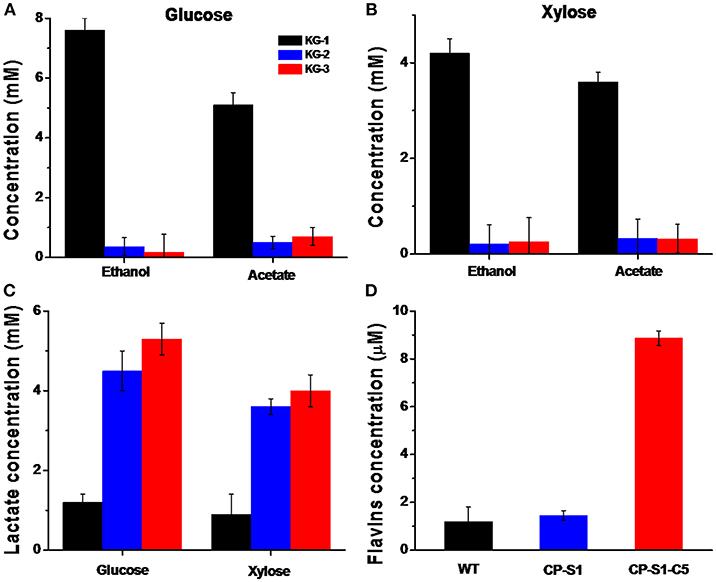
Figure 2. Quantification of metabolites of K.pneumoniae and S.oneidensis. The metabolites concentration in the fermentation media (K. pneumoniae was inoculated in the anolyte with 20 mM glucose or 20 mM xylose, and S. oneidensis was cultured with 20 mM of sodium L-lactate) was quantified by HPLC after 24 hours' fermentation, respectively. (A) Quantification of metabolites when K. pneumoniae was inoculated in the anolyte with 20 mM glucose. The concentration of lactate produced by KG-3 increased by 2.1 times than KG-1(from 2.8 ± 0.2 mM to 8.8 ± 0.4 mM); the ethanol level produced by KG-3 decreased by 97% (from 7.6 ± 0.5 mM to 0.2 ± 0.1 mM); and the acetate level produced by KG-3 decreased by 86% (from 5.1 ± 0.4 mM to 0.7 ± 0.3 mM). (B) Quantification of metabolites when K. pneumoniae was inoculated in the anolyte with 20 mM xylose. The concentration of lactate produced by KG-3 increased by 2.4 times than KG-1(from 1.8 ± 0.4 mM to 6.2 ± 0.5 mM); the ethanol level produced by KG-3 decreased by 94% than KG-1 (from 5.2 ± 0.3 mM to 0.3 ± 0.2 mM); the acetate level produced by KG-3 decreased by 81% (from 3.6 ± 0.2 mM to 0.7 ± 0.3 mM). (C) The concentration of lactate produced by KG-3 increased by 3.4 times than KG-1(from 1.2 ± 0.2 mM to 5.3 ± 0.4 mM) when inoculated in the anolyte with 20 mM glucose; The concentration of lactate produced by KG-3 increased by 3.4 times than KG-1 (from 0.9 ± 0.5 mM to 4.0 ± 0.5 mM) when inoculated in the anolyte with 20 mM xylose. (D) The concentration of flavins obtained from CP-S1-C5 increased ~6.4 times (from 1.2 ± 0.1 μM to 8.9 ± 0.3 μM) compared with the wild-type strain (MR-1). Statistics were calculated from three independent replicates of experiments.
In addition, based on different electron transfer mechanisms, two strategies were adopted to facilitate extracellular electron transport. To improve the direct contact-based electron transfer pathway of exoelectrogens, the CP-S1 S. oneidensis mutant was used to further improve the adhesion of S. oneidensis to the carbon electrode surfaces (Figure S2). To enhance the shuttles-mediated electrons transfer, a flavin biosynthesis pathway was assembled in CP-S1 to further accelerate electron transfer. As a result, the flavins produced by the CP-S1-C5 increased by 6.4-folds compared with that of the WT S. oneidensis MR-1 (from 1.2 ± 0.1 μM to 8.9 ± 0.3 μM) (Figure 2D).
Optimize K. pneumonia-S. oneidensis Microbial Consortium in the Mixed Sugar Co-fed MFC
To realize a continuous and stable supply of carbon sources for exoelectrogen and achieve a maximum MFC power generation, we optimized the seeding ratio of the microorganisms and the feed-in glucose and xylose concentration, respectively. Firstly, the seeding ratio of the K. pneumoniae and S. oneidensis was optimized by a serial of orthogonal experiments to achieve a maximum MFC power generation in anolytes. The result indicated that the seeding OD600 ratio between K. pneumoniae and S. oneidensis being 1 to 5 would reach a maximum bioelectricity generation in MFCs (Figure S1). Secondly, to ensure that S. oneidensis had a suitable continuous supply of lactate produced by K. pneumoniae for the maximum MFC power generation, we further optimized the initial feed-in glucose and xylose concentrations. Three different concentration ratios of glucose and xylose, i.e., 30 mM (glucose): 10 mM (xylose), 20 mM: 20 mM, and 10 mM: 30mM xylose were chosen to determine the optimal substrate concentration for our engineered strains. As shown in Figures 3A–C, the results showed that the feed-in ratio of 20 mM (glucose): 20 mM (xylose) reached a highest output voltage (268.5 ± 10 mV), while 10 mM: 30 mM and 30 mM: 10 mM generated a lowest MFC output voltage, 120.2 ± 7.0 mV and 210.3 ± 6.0 mV, respectively. Furthermore, as shown in Figure 3D, the coulomb efficiency of the MFC inoculated with KG-3: CP-S1-C5 and substrates (glucose: xylose = 20 mM: 20 mM) was 33.3%, which was higher than in the other scenarios.
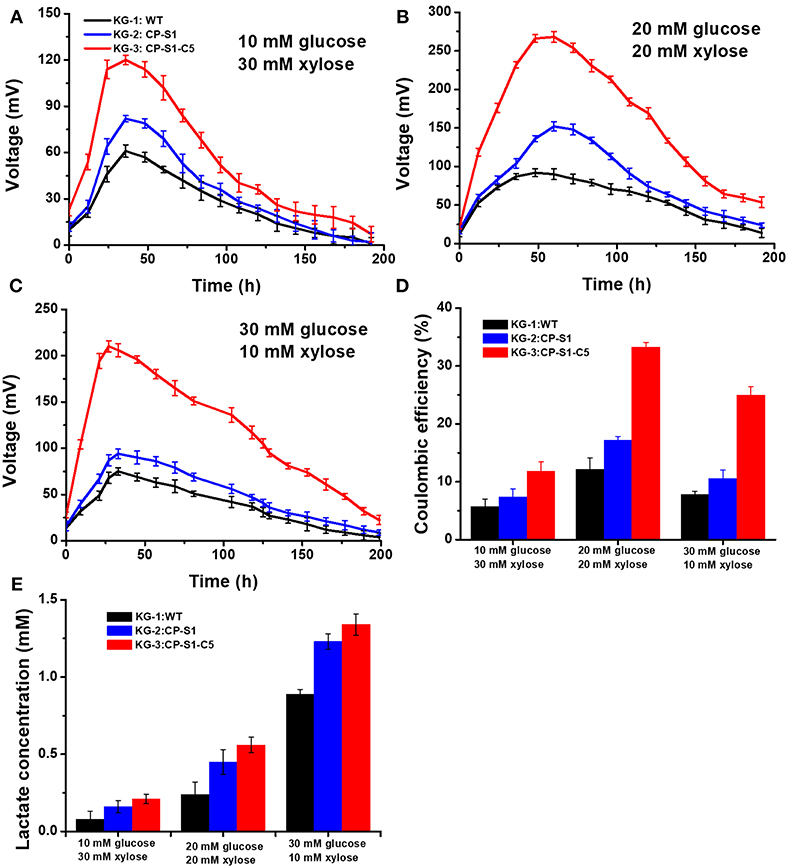
Figure 3. Optimizing glucose and xylose concentration of the K.pneumoniae S.oneidensis consortia to maximize power output in MFCs. The MFC voltage output of K.pneumoniae S.oneidensis consortia with different concentrations of feed-in glucose and xylose. (A) Corresponded to feed-in 10 mM glucose and 30 mM xylose. (B) Corresponded to feed-in 20 mM glucose and 20 mM xylose. (C) Corresponded to feed-in 30 mM glucose and 10 mM xylose. (D) The coulomb efficiency of the K. pneumoniae S. oneidensis consortia-inoculated MFCs with different feed-in glucose and xylose concentrations. (E) Analysis of lactate level at 24 th h under different feed-in glucose and xylose concentrations.
To illuminate the underlying reason, the lactate concentrations sampled from the anolyte were measured after 24 h cultivation. The data indicated that feed-in ratio 30 mM (glucose) and 10 mM (xylose) could cause a higher level accumulation of lactate in MFCs (Figure 3E), however, the MFC output voltage was not the optimum. This result was caused by the fact that the rapidly accumulated lactate resulted in an acidic condition (pH = 5), in which the growth activity and flavins biosynthesis of cell would be inhibited, reducing the power generation capability of S. onedensis (Yong et al., 2013; Lin et al., 2016). When feeding in 10 mM glucose and 30 mM xylose in MFC, the voltage output maintained at a low level all the time. This could be explained as the substrate could be exhausted rapidly, leading to a shortage of lactate to feed S. oneidensis. However, upon feeding 20 mM glucose and 20 mM xylose, the recombinant consortium enabled higher and longer voltage output than other concentrations. In this scenario, K. pneumoniae can continuously supply lactate to S. oneidensis, and the lactate from this feed-in mix sugar concentration was neither too high to generate cellular toxicity to S. oneidensis, nor too low as substrates to feed S. oneidensis. In addition, the substrates consumption results demonstrated that the engineered strain KG-3 showed a superior performance of substrate consumption than that of the KG-1 and the KG-2 (Table S3). Meanwhile, these strains preferred to utilize glucose than xylose for the production of lactate.
MFC Performance and Bio-Electrochemical Analysis
The cyclic voltammetry (CV) was conducted to reveal the redox reaction kinetics at the interfaces of bacterial cells and anodes with a scan rate of 1 mV/s (Li et al., 2018b,c). As shown in Figure 4A, there were typical redox peaks of favins in the CV curves starting from around ~-0.4 V (vs. Ag/AgCl), revealing that the dominating mechanism for bioelectricity production in this microbial consortium was the favins-mediated EET. Furthermore, another catalytic current arose at ~-0.27 V (vs. Ag/AgCl) was observed in these strains, which was caused by the direct involvement of conductive c-type cytochromes. To further investigate the MFCs' performance inoculated with these microbial consortia, the linear sweep voltammetry (LSV) and the polarization curves at a low scan rate of 0.1 mV/s were obtained (Baron et al., 2009). As shown in Figure 4B, the maximum catalytic current of KG-3: CP-S1-C5 was the highest (408.2 ± 8.0 mA/m2), which was higher than the maximum catalytic current of KG-1: WT (119.6 ± 6.0 mA/m2) and KG-2: CP-S1 (167.84 mA/m2). Notably, the dropping slope of the polarization curve obtained from the engineered KG-3: CP-S1-C5 was smaller than those obtained from the other two microbial consortium (KG-1: WT and KG-2: CP-S1), implying that the internal charge transfer resistance of the MFC inoculated with KG-3: CP-S1-C5 was relatively smaller (Figure 4C). The power densities were calculated for each; the engineered KG-3: CP-S1-C5 microbial consortium obtained a maximum power density of 104.7 ± 10.0 mW/m2, which was 7.2- and 2.2-folds higher than that of the KG-1:WT (12.7 ± 3.0 mW/m2) and KG-2:CP-S1 (32.7 ± 6.0 mW/m2), respectively.
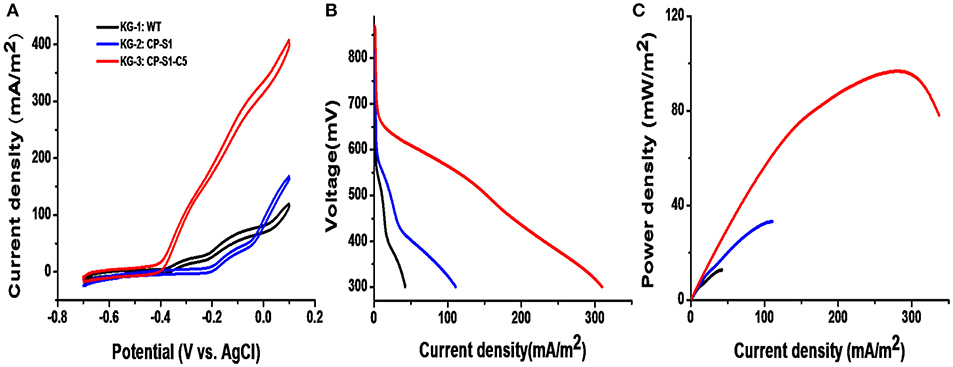
Figure 4. Electrochemical analysis of the performances of microbial consortia in the 20 mM glucose and 20 mM xylose-fed MFCs. (A) Turnover cyclic voltammetry (CV) curves at a scan rate of 1 mV/s. (B) MFC polarization curves obtained by linear sweep voltammetry (LSV) with a slow scan rate of 0.1 mV/s. (C) MFC power density output curves calculated on the basis of the corresponding polarization curves.
To evaluate the electricity generation capacity of utilizing cellulose hydrolyzates, this synergistic microbial consortium (KG-3: CP-S1-C5) was further inoculated to the sterile or non-sterile corn straw hydrolyzates-fed MFC. As shown in Figure 5, the maximum output voltage of the sterile cellulosic hydrolyzates-fed MFCs could reach 152.3 ± 8.0 mV, the maximum current density was 72.8 ± 5.0 mA/m2, and the maximum power density of the sterile cellulosic hydrolyzates-fed MFC could reach 23.5 ± 6.0 mW/m2.
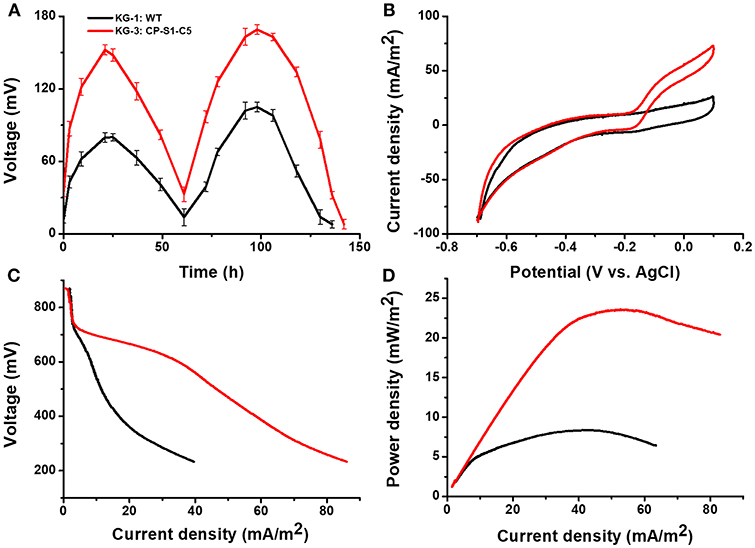
Figure 5. Electrochemical analysis of the performances of microbial consortia in the cellulose hydrolyzates-fed MFCs. MFC output voltage profiles produced by KG-3: CP-S1-C5 under cellulose hydrolyzates feedstock anolyte. (A) Voltage output in the multiple operational cycles. (B) Turnover cyclic voltammetry (CV) curves at a scan rate of 1 mV/s. (C) MFC polarization curves obtained by linear sweep voltammetry (LSV) with a slow scan rate of 0.1 mV/s. (D) MFC power density output curves calculated on the basis of the corresponding polarization curves.
To further verify the stability of the engineered co-cultures, particularly over long term and in the presence of non-sterile feeds, we further used non-sterile corn straw hydrolyzates as the carbon source to evaluate the electricity generation capability. As shown in Figure S3, the output voltage of MFCs with a multi-cycle operation revealed that the engineered co-culture enabled a stable power generation. And the results of bioelectrochemical characterization also demonstrated that the engineered co-culture (KG-3: CP-S1-C5) showed a superior performance of electricity generation than that of co-culture (KG-1: WT). In all, our engineered co-culture showed stability of power generation in MFCs with either sterile or non-sterile feeds.
Conclusions
To efficiently harvest electricity from glucose and xylose in cellulosic hydrolyzates, we rationally designed and constructed synergistic microbial consortia including K. pneumoniae and S. oneidensis in a hierarchical way, in which K. pneumonia could convert glucose and xylose into lactate to feed S. oneidensis continuously as the sole carbon source and electron donor.
To promote power generation of the microbial consortium in cellulosic hydrolyzates-fed MFCs, we rationally engineered the co-culturing strains in the consortium by redirecting carbon flux distribution toward lactate biosynthesis in K. pneumoniae and enhancing flavins-mediated EET efficiency of S. oneidensis, respectively. In this synthetic microbial consortium, the by-products (i.e., ethanol and acetate) were eliminated, and a lactate biosynthesis pathway was assembled into K. pneumonia for overproducing lactate. To facilitate extracellular electron transfer (EET) of exoelectrogens, a biosynthetic flavins pathway from B. subtilis was expressed in a highly hydrophobic S. oneidensis CP-S1, which improved both the direct-contacted and the flavins-mediated EET. Subsequently, the co-culturing conditions and substrate concentrations were further optimized to obtain higher and more stable electricity output. The glucose and xylose co-fed MFC inoculated with the recombinant K. pneumonia S. oneidensis generated a maximum power density of 104.7 ± 10.0 mW/m2. Furthermore, we used this synthetic microbial consortium in the corn straw hydrolyzates-fed MFC, obtaining a power density 23.5 ± 6.0 mW/m2. This rationally constructed a “fermenter-exoelectrogen” synthetic microbial consortium, that successfully utilized cellulosic hydrolyzates to harvest electricity, laying the foundation for the conversion of other biomass that was difficult to utilize in MFCs for power generation.
Author Contributions
FL and XA designed the project, performed experiments, analyzed data, and drafted the manuscript. DW, JX, YuC, WL, YiC, XG, XL, CL, and SL helped to design some experiments and drafted the manuscript. HS designed and supervised the project, analyzed data, and critically revised the manuscript.
Funding
This research was supported by the National Natural Science Foundation of China (21621004), and the Foundation of Tianjin Engineering Research Center of Microbial Metabolism and Fermentation Process Control (No. ZXKF20180102).
Conflict of Interest Statement
The authors declare that the research was conducted in the absence of any commercial or financial relationships that could be construed as a potential conflict of interest.
Supplementary Material
The Supplementary Material for this article can be found online at: https://www.frontiersin.org/articles/10.3389/fmicb.2019.00409/full#supplementary-material
References
Baron, D., LaBelle, E., Coursolle, D., Gralnick, J. A., and Bond, D. R. (2009). Electrochemical measurement of electron transfer kinetics by Shewanella oneidensis MR-1. J. Biol. Chem. 284, 28865–28873. doi: 10.1074/jbc.M109.043455
Bond, D. R., Holmes, D. E., Tender, L. M., and Lovley, D. R. (2002). Electrode-reducing microorganisms that harvest energy from marine sediments. Science 295, 483–485. doi: 10.1126/science.1066771
Cheng, S., and Logan, B. E. (2007). Sustainable and efficient biohydrogen production via electrohydrogenesis. Proc. Natl. Acad. Sci. U.S.A. 104, 18871–18873. doi: 10.1073/pnas.0706379104
Choi, D., Lee, S. B., Kim, S., Min, B., Choi, I. G., and Chang, I. S. (2014). Metabolically engineered glucose-utilizing Shewanella strains under anaerobic conditions. Bioresour. Technol. 154, 59–66. doi: 10.1016/j.biortech.2013.12.025
Du, F., Li, Z., Yang, S., Xie, B., and Liu, H. (2011). Electricity generation directly using human feces wastewater for life support system. Acta Astronaut. 68, 1537–1547. doi: 10.1016/j.actaastro.2009.12.013
Feng, X., Ding, Y., Mo, X., Xin, X., Zhang, R., and Zhao, G. (2014). Production of optically pure D-lactate from glycerol by engineered Klebsiella pneumoniae strain. Bioresour. Technol. 172, 269–275. doi: 10.1016/j.biortech.2014.09.074
Flynn, C. M., Hunt, K. A., Gralnick, J. A., and Srienc, F. (2012). Construction and elementary mode analysis of a metabolic model for Shewanella oneidensis MR-1. BioSystems 107, 120–128. doi: 10.1016/j.biosystems.2011.10.003
Fredrickson, J. K., Romine, M. F., Beliaev, A. S., Auchtung, J. M., Driscoll, M. E., Gardner, T. S., et al. (2008). Towards environmental systems biology of Shewanella. Nat. Rev. Microbiol. 6, 592–603. doi: 10.1038/nrmicro1947
Golitsch, F., Bücking, C., and Gescher, J. (2013). Proof of principle for an engineered microbial biosensor based on Shewanella oneidensis outer membrane protein complexes. Biosens. Bioelectron. 47, 285–291. doi: 10.1016/j.bios.2013.03.010
Guo, X. W., Cao, C. C., Wang, Y., Li, C., Wu, M. Y., Chen, Y., et al. (2014). Effect of the inactivation of lactate dehydrogenase, ethanol dehydrogenase, and phosphotransacetylase on 2,3-butanediol production in Klebsiella pneumoniae strain. Biotechnol. Biofuels 7, 1–11. doi: 10.1186/1754-6834-7-44
Ha, P. T., Lindemann, S. R., Shi, L., Dohnalkova, A. C., Fredrickson, J. K., Madigan, M. T., et al. (2017). Syntrophic anaerobic photosynthesis via direct interspecies electron transfer. Nat. Commun. 8:13924. doi: 10.1038/ncomms13924
Hau, H. H., and Gralnick, J. A. (2007). Ecology and biotechnology of the genus Shewanella. Annu. Rev. Microbiol. 61, 237–258. doi: 10.1146/annurev.micro.61.080706.093257
Jablonska, M. A., Rybarczyk, M. K., and Lieder, M. (2016). Electricity generation from rapeseed straw hydrolysates using microbial fuel cells. Bioresour. Technol. 208, 117–122. doi: 10.1016/j.biortech.2016.01.062
Kadier, A., Kalil, M. S., Abdeshahian, P., Chandrasekhar, K., Mohamed, A., Azman, N. F., et al. (2016). Recent advances and emerging challenges in microbial electrolysis cells (MECs) for microbial production of hydrogen and value-added chemicals. Renew. Sust. Energ. Rev. 61, 501–525. doi: 10.1016/j.rser.2016.04.017
Katoa, S., Hashimotoa, K., and Watanabe, K. (2012). Microbial interspecies electron transfer via electric currents through conductive minerals. Proc. Natl. Acad. Sci. U.S.A. 109, 10042–10046. doi: 10.1073/pnas.1117592109
Kracher, D., Scheiblbrandner, S., Felice, A. K., Breslmayr, E., Preims, M., Ludwicka, K., et al. (2016). Extracellular electron transfer systems fuel cellulose oxidative degradation. Science 352, 1098–1101. doi: 10.1126/science.aaf3165
Kumar, A., Hsu, H. H., Kavanagh, P., Barrière, F., Lens, P. N. L., Lapinsonnière, L., et al. (2017). The ins and outs of microorganism–electrode electron transfer reactions. Nat. Rev. Chem. 1:0024. doi: 10.1038/s41570-017-0024
Kumar, V., and Park, S. (2017). Potential and limitations of Klebsiella pneumoniae as a microbial cell factory utilizing glycerol as the carbon source. Biotechnol. Adv. 36, 150–167. doi: 10.1016/j.biotechadv.2017.10.004
Li, C., Tao, F., Ni, J., Wang, Y., Yao, F., and Xu, P. (2015). Enhancing the light-driven production of D-lactate by engineering cyanobacterium using a combinational strategy. Sci. Rep. 5:9777. doi: 10.1038/srep09777
Li, F., Li, Y., Sun, L., Li, X., Yin, C., An, X., et al. (2017). Engineering Shewanella oneidensis enables xylose-fed microbial fuel cell. Biotechnol. Biofuels 10:196. doi: 10.1186/s13068-017-0881-2
Li, F., Li, Y., Sun, L. M., Chen, X., An, X., Yin, C., et al. (2018b). Modular engineering intracellular NADH regeneration boosts extracellular electron transfer of Shewanella oneidensis MR-1. ACS Synth. Biol. 7, 885–895. doi: 10.1021/acssynbio.7b00390
Li, F., Li, Y.-X., Cao, Y.-X., Wang, L., Liu, C.-G., Shi, L., et al. (2018a). Modular engineering to increase intracellular NAD(H/+) promotes rate of extracellular electron transfer of Shewanella oneidensis. Nat. Commun. 9:3637. doi: 10.1038/s41467-018-05995-8
Li, H. L., Opgenorth, P., Wernick, D., Rogers, S., Wu, T. Y., Higashide, W., et al. (2012). Integrated electromicrobial conversion of CO2 to higher alcohols. Science 335:1596. doi: 10.1126/science.1217643
Li, W. C., Li, X., Qin, L., Zhu, J. Q., Han, X., Li, B. Z., et al. (2016). Reducing sugar loss in enzymatic hydrolysis of ethylenediamine pretreated corn stover. Bioresour. Technol. 224:405. doi: 10.1016/j.biortech.2016.11.031
Li, W. C., Li, X., Zhu, J. Q., Qin, L., Li, B. Z., and Yuan, Y. J. (2018c). Improving xylose utilization and ethanol production from dry dilute acid pretreated corn stover by two-step and fed-batch fermentation. Energy 157, 877–885. doi: 10.1016/j.energy.2018.06.002
Lin, T., Bai, X., Hu, Y., Li, B., Yuan, Y., Song, H., et al. (2016). Synthetic Saccharomyces cerevisiae-Shewanella oneidensis consortium enables glucose-fed high-performance microbial fuel cell. AIChE J. 63, 1830–1838. doi: 10.1002/aic.15611
Liu, C., Colon, B. C., Ziesack, M., Silver, P. A., and Nocera, D. G. (2016). Water splitting-biosynthetic system with CO2 reduction efficiencies exceeding photosynthesis. Science 352, 1210–1213. doi: 10.1126/science.aaf5039
Liu, Y., Ding, M.-Z., Ling, W., Yang, Y., Zhou, X., Li, B.-Z., et al. (2017). A three-species microbial consortium for power generation. Energy Environ. Sci. 10, 1600–1609. doi: 10.1039/C6EE03705D
Logan, B. E. (2009). Exoelectrogenic bacteria that power microbial fuel cells. Nat. Rev. Microbiol. 7, 375–381. doi: 10.1038/nrmicro2113
Logan, B. E., and Rabaey, K. (2012). Conversion of wastes into bioelectricity and chemicals by using microbial electrochemical technologies. Science 337, 686–690. doi: 10.1126/science.1217412
Lovley, D. R. (2006). Bug juice: harvesting electricity with microorganisms. Nat. Rev. Microbiol. 4, 497–508. doi: 10.1038/nrmicro1442
McAnulty, M. J., Poosarla, V. G., Kim, K. Y., Jasso-Chávez, R., Logan, B. E., and Wood, T. K. (2017). Electricity from methane by reversing methanogenesis. Nat. Commun. 8:15419. doi: 10.1038/ncomms15419
Mohan, S. V., Butti, S. K., Amulya, K., Dahiya, S., and Modestra, J. A. (2016). Waste biorefinery:a new paradigm for a sustainable bioelectro economy. Trends Biotechnol. 34, 852–855. doi: 10.1016/j.tibtech.2016.06.006
Payne, C. M., Knott, B. C., Mayes, H. B., Hansson, H., Himmel, M. E., Sandgren, M., et al. (2015). Fungal cellulases. Chem. Rev. 115, 1308–1448. doi: 10.1021/cr500351c
Pinchuk, G. E., Rodionov, D. A., Yang, C., Li, X., Osterman, A. L., Dervyn, E., et al. (2009). Genomic reconstruction of Shewanella oneidensis MR-1 metabolism reveals a previously uncharacterized machinery for lactate utilization. Proc. Natl. Acad. Sci. U.S.A. 106, 2874–2879. doi: 10.1073/pnas.0806798106
Rabaey, K., and Rozendal, R. A. (2010). Microbial electrosynthesis - revisiting the electrical route for microbial production. Nat. Rev. Microbiol. 8, 706–716. doi: 10.1038/nrmicro2422
Shi, L., Dong, H., Reguera, G., Beyenal, H., Lu, A., Liu, J., et al. (2016). Extracellular electron transfer mechanisms between microorganisms and minerals. Nat. Rev. Microbiol. 14, 651–662. doi: 10.1038/nrmicro.2016.93
Taylor, L. E., Knott, B. C., Baker, J. O., Alahuhta, P. M., Hobdey, S. E., Linger, J. G., et al. (2018). Engineering enhanced cellobiohydrolase activity. Nat. Commun. 9:1186. doi: 10.1038/s41467-018-03501-8
Tender, L. M., Reimers, C. E., Stecher, H. A. 3rd, Holmes, D. E., Bond, D. R., Lowy, D. A., et al. (2002). Harnessing microbially generated power on the seafloor. Nat. Biotechnol. 20, 821–825. doi: 10.1038/nbt716
Wang, H., Luo, H., Fallgren, P. H., Jin, S., and Ren, Z. J. (2015). Bioelectrochemical system platform for sustainable environmental remediation and energy generation. Biotechnol. Adv. 33, 317–334. doi: 10.1016/j.biotechadv.2015.04.003
Wang, H., and Ren, Z. J. (2013). A comprehensive review of microbial electrochemical systems as a platform technology. Biotechnol. Adv. 31, 1796–1807. doi: 10.1016/j.biotechadv.2013.10.001
Wang, Z., Lee, T., Lim, B., Choi, C., and Park, J. (2014). Microbial community structures differentiated in a single-chamber air-cathode microbial fuel cell fueled with rice straw hydrolysate. Biotechnol. Biofuels 7:9. doi: 10.1186/1754-6834-7-9
Wen, Q., Wu, Y., Zhao, L., and Sun, Q. (2010). Production of electricity from the treatment of continuous brewery wastewater using a microbial fuel cell. Fuel 89, 1381–1385. doi: 10.1016/j.fuel.2009.11.004
Yamasaki, R., Maeda, T., and Wood, T. K. (2018). Electron carriers increase electricity production in methane microbial fuel cells that reverse methanogenesis. Biotechnol. Biofuels 11:211. doi: 10.1186/s13068-018-1208-7
Yan, D., Yang, X., and Yuan, W. (2015). Electricity and H2 generation from hemicellulose by sequential fermentation and microbial fuel/electrolysis cell. J. Power Sources 289, 26–33. doi: 10.1016/j.jpowsour.2015.04.164
Yang, Y., Ding, Y., Hu, Y., Cao, B., Rice, S. A., Kjelleberg, S., et al. (2015a). Enhancing bidirectional electron transfer of Shewanella oneidensis by a synthetic flavin pathway. ACS Synth. Biol. 4, 815–823. doi: 10.1021/sb500331x
Yang, Y., Wu, Y., Hu, Y., Cao, Y., Poh, C. L., Cao, B., et al. (2015b). Engineering electrode-attached microbial consortia for high-performance xylose-fed microbial fuel cell. ACS Catal. 5, 6937–6945. doi: 10.1021/acscatal.5b01733
Yong, Y. C., Cai, Z., Yu, Y. Y., Chen, P., Jiang, R., Cao, B., et al. (2013). Increase of riboflavin biosynthesis underlies enhancement of extracellular electron transfer of Shewanella in alkaline microbial fuel cells. Bioresour. Technol. 130, 763–768. doi: 10.1016/j.biortech.2012.11.145
Keywords: microbial fuel cell, synthetic biology, microbial consortia, cellulosic hydrolyzates, Shewanella oneidensis
Citation: Li F, An X, Wu D, Xu J, Chen Y, Li W, Cao Y, Guo X, Lin X, Li C, Liu S and Song H (2019) Engineering Microbial Consortia for High-Performance Cellulosic Hydrolyzates-Fed Microbial Fuel Cells. Front. Microbiol. 10:409. doi: 10.3389/fmicb.2019.00409
Received: 30 November 2018; Accepted: 18 February 2019;
Published: 18 March 2019.
Edited by:
Pascal E. Saikaly, King Abdullah University of Science and Technology, Saudi ArabiaReviewed by:
Enrico Marsili, Nanyang Technological University, SingaporeRaghu Sapireddy, King Abdullah University of Science and Technology, Saudi Arabia
Copyright © 2019 Li, An, Wu, Xu, Chen, Li, Cao, Guo, Lin, Li, Liu and Song. This is an open-access article distributed under the terms of the Creative Commons Attribution License (CC BY). The use, distribution or reproduction in other forums is permitted, provided the original author(s) and the copyright owner(s) are credited and that the original publication in this journal is cited, in accordance with accepted academic practice. No use, distribution or reproduction is permitted which does not comply with these terms.
*Correspondence: Hao Song, aHNvbmdAdGp1LmVkdS5jbg==
†These authors have contributed equally to this work