- 1Division of Molecular Biology, Biocenter, Medical University of Innsbruck, Innsbruck, Austria
- 2Division of Human Genetics, Medical University of Innsbruck, Innsbruck, Austria
- 3Institute of Molecular Biosciences, Biophysics Division, University of Graz, Graz, Austria
- 4Institute of Zoology, University of Innsbruck, Innsbruck, Austria
The membrane sphingolipid glucosylceramide (GlcCer) plays an important role in fungal fitness and adaptation to most diverse environments. Moreover, reported differences in the structure of GlcCer between fungi, plants and animals render this pathway a promising target for new generation therapeutics. Our knowledge about the GlcCer biosynthesis in fungi is mainly based on investigations of yeasts, whereas this pathway is less well characterized in molds. We therefore performed a detailed lipidomic profiling of GlcCer species present in Neurospora crassa and comprehensively show that the deletion of genes encoding enzymes involved in GlcCer biosynthesis affects growth, conidiation and stress response in this model fungus. Importantly, our study evidences that differences in the pathway intermediates and their functional role exist between N. crassa and other fungal species. We further investigated the role of GlcCer in the susceptibility of N. crassa toward two small cysteine-rich and cationic antimicrobial proteins (AMPs), PAF and PAFB, which originate from the filamentous ascomycete Penicillium chrysogenum. The interaction of these AMPs with the fungal plasma membrane is crucial for their antifungal toxicity. We found that GlcCer determines the susceptibility of N. crassa toward PAF, but not PAFB. A higher electrostatic affinity of PAFB than PAF to anionic membrane surfaces might explain the difference in their antifungal mode of action.
Introduction
Fungi are pioneers in colonizing the most diverse habitats with access to organic and inorganic nutrients that enables a saprophytic and parasitic way of life. The barrier function of the plasma membrane is crucial for cellular homeostasis and cell environment interactions. The activity of enzymes, transporters and receptors embedded in and along this lipid bilayer ensures important vital functions, such as cell wall synthesis, growth, cell polarity establishment, reproduction and optimal adaptation to environmental changes (Los and Murata, 2004; Fontaine, 2017). As such, any alteration of the lipid composition of the plasma membrane might impact the distribution, regulation, activity and signaling function of membrane proteins, with adverse effects on the fungal cell fitness.
Our knowledge on GlcCer biosynthesis in fungi is based on investigations on yeasts, such as the human pathogenic Cryptococcus neoformans (Rhome et al., 2007; Munshi et al., 2018) and Candida albicans (Oura and Kajiwara, 2010) and on some few reports on filamentous ascomycetes, such as the human pathogen Scedosporium apiospermium (Rollin-Pinheiro et al., 2014), the model organisms Neurospora crassa (Park et al., 2005) and Aspergillus nidulans (Fernandes et al., 2016), the plant pathogen Fusarium graminearum (Ramamoorthy et al., 2007, 2009) and the post-harvest pathogen Penicillium digitatum (Zhu et al., 2014). Notably, Saccharomyces cerevisiae and some other yeast species lack the GlcCer synthesis gene and therefore, membranes do not contain this sphingolipid (Saito et al., 2006).
Glucosylceramide backbones of fungal origin show a characteristic consensus structure with less structural variations than GlcCer from plants. However, genus- and species-specific modifications of the sphingoid base and the fatty acid chains exist in this fungal lipid group (Warnecke and Heinz, 2003). Fungal GlcCer is a sugar-bound sphingolipid, composed of a long chain (C18) sphingoid base (methyl-branched and diunsaturated) linked to a long chain (C16 or C18) hydroxy fatty acid and a glucose moiety (Del Poeta et al., 2014).
Glucosylceramide is located mostly in the outer leaflet of the plasma membrane with the carbohydrate group exposed to the extracellular space and associates with sterols and proteins to form so called lipid rafts. These are microdomains that influence the membrane fluidity and regulate the organization and integrity of the membrane (Alvarez et al., 2007; Singh et al., 2012; Guimarães et al., 2014). Furthermore, intermediate compounds of the GlcCer pathway, e.g., sphingosine, ceramide and phosphorylated derivatives thereof, are involved in cell signaling related to cell growth and apoptosis (Ohanian and Ohanian, 2001).
As such, the GlcCer synthesis pathway has attracted increasing attention due to its reported role in fungal pathogenicity, growth, morphology, cell polarity establishment and alkali tolerance (Del Poeta et al., 2014; Guimarães et al., 2014). Differences in the structure of GlcCer between fungi, plants and animals/humans and a different set of key enzymes involved in the fungal GlcCer synthesis render this pathway a promising target for new generation therapeutics (Warnecke and Heinz, 2003; Nimrichter and Rodrigues, 2011; Del Poeta et al., 2014; Pan et al., 2018).
Small cysteine-stabilized and cationic AMPs are exceptionally interesting candidates for developing novel treatment strategies. Numerous studies generated detailed insight into the fast-growing group of fungal AMPs in respect to their specificity, activity, structure and function (Garrigues et al., 2016; Paege et al., 2016; Sonderegger et al., 2018; Tóth et al., 2018). We have been extensively studying the function and the structural properties of two AMPs, PAF and PAFB, that are secreted from the penicillin producer Penicillium chrysogenum (Marx et al., 2008; Fizil et al., 2015; Sonderegger et al., 2017; Huber et al., 2018). Importantly, their antifungal activity is closely linked with their internalization (Huber et al., 2018) and PAF triggers a rapid and sustained increase of the cytoplasmic calcium concentration in N. crassa (Binder et al., 2010). A detailed knowledge of the mode of action of AMPs and their way to interact with sensitive target fungi is supportive for their future exploitation.
In this study, we provide for the first time a comprehensive qualitative and quantitative analysis of sphingolipid species present in the plasma membrane of N. crassa. The availability of a gene deletion library of this model fungus prompted us to dissect in detail the GlcCer biosynthesis pathway using mutant strains defective in the distinct enzymatic steps. Phenotypic analysis evidenced that the intermediates of this pathway play an important role in growth, morphology, conidiogenesis and stress response in N. crassa. We further show that the GlcCer biosynthesis pathway mediates PAF but not PAFB susceptibility of the highly sensitive N. crassa, which suggests that the mode of action differs considerably between these two AMPs. This can be explained by the fact that membrane binding of both AMPs is electrostatically driven revealing a higher affinity of PAFB than PAF to anionic membrane surfaces, which at least in part may compensate the GlcCer depletion in the respective mutants.
Materials and Methods
Strains, Media and Cultivation Conditions
All strains and media used in this study are listed in Table 1 and Supplementary Data Sheet S1, Supplementary Table S1.
The strains P. chrysogenum paf and P. chrysogenum pafB were grown on solid minimal medium (MM) at 25°C for 48–72 h for conidia generation and in liquid MM at 25°C for 72 h for AMP production (Huber et al., 2018). All N. crassa strains were grown on Vogel’s agar [supplemented with 200 μg/mL hygromycin or 400 μg/mL phosphinothricin (PT)] at 37°C under continuous light for 48–72 h to generate conidia. Antibiotics were omitted from experiments performed for phenotype analysis. For all experiments, conidia were freshly harvested in spore buffer [0.9% NaCl (w/v), 0.01% Tween 80 (v/v)].
Fungal biomass of the N. crassa strains for dry weight determination and membrane lipid extraction was generated by cultivating 2.5 × 104 conidia in 50 mL liquid Vogel’s medium for 72 h at 25°C under continuous shaking.
Colony formation and growth of N. crassa strains was monitored on Vogel’s agar in petri dishes (VWR) after 72 h of incubation at 25°C. After 24 h of cultivation, the diameter of the colonies was determined.
For quantification of conidia production, a defined number of spores from each strain was plated in 6-well plates (VWR) on 4 mL Vogel’s agar/well and spores were harvested after 72 h of incubation at 25°C.
The growth inhibition assays and stress-tests with the N. crassa strains were performed in 24-well plates (VWR) on Vogel’s agar with the respective supplementations. Plates were incubated at 25°C for 72 h.
For the broth microdilution assays, the determination of germination efficiency and germ tube length as well as for protein uptake studies, the N. crassa strains were grown in 0.2 × Vogel’s medium in 96-well plates (VWR) at 25°C for the indicated time points.
Membrane Lipid Extraction
Freeze dried mycelia of the N. crassa strains were crashed for 1 min at 20 Hz using a Mixermill (MM400, Retsch). The total protein content of each sample was determined by Bradford protein assay (Bio-Rad), after dissolving the sample in dH2O.
Subsequently lipids were extracted from biomass corresponding to 500 μg total protein by adding 500 μL chloroform:methanol (2:1) containing 0.5 μM of the internal standards (tetramyristoylcardiolipin and N-heptadecanoyl-D-erythro-sphingosine [(C17 ceramide), Avanti Polar Lipids]. Mycelia were further homogenized 4 × 30 sec at 20 Hz and then incubated for 5 min in an ultrasonic bath. For phase separation, samples were centrifuged for 10 min at 14,000 rpm (4°C) and the organic phases were transferred to glass tubes. This chloroform:methanol extraction step was repeated once more. Combined organic phases were dried overnight and stored at -20°C until analysis.
HPLC-MS/MS Lipidomics
For lipid identification and quantification, lipid extracts of five biological replicates of each strain were used for analysis. Details about equipment and parameters used for lipidomics are provided in Supplementary Data Sheet S1, Supplementary Table S2. The detection of phospholipids and sphingolipids was performed according to a method adapted from Oemer et al. (2018). Briefly, lipid extracts were dissolved in 100 μL mobile phase and 10 μL were injected into an Ultimate 3000 HPLC system (Dionex). Chromatographic separation of analytes was achieved by using gradient elution as indicated (Supplementary Data Sheet S1, Supplementary Table S2). Lipid components were detected with an online coupled LTQ Velos mass spectrometer (Thermo Fisher Scientific) operated in positive ESI mode and within a range of 460–1650 m/z. Raw data were converted into the mzML open format and analyzed with MZmine2 (V2.30) (Pluskal et al., 2010). A manually curated peaklist (Supplementary Data Sheet S1, Supplementary Table S3) was used for targeted peak extraction on basis of cropped (1–22 min, 460–1650 m/z) and baseline corrected data (Parameters: TIC, 0.2 m/z bin with, asymmetric baseline corrector: 109 smoothing, 0.1 asymmetry factor, Rserve). Integrated peaks were further analyzed in R (R Development Core Team, 2015). Lipids were quantified according to their class by linear regression using an external standard dilution series, including three ceramide standards, dihydro-ceramide (dhCer:35:0), ceramide (Cer:36:1) and GlcCer (GlcCer:34:1) and two phospholipids, phosphatidylethanolamine (PE:28:0) and phosphatidylcholine (PC:28:0) and then normalized via the internal standard dihydro-ceramide (18:0/17:0). Absolute quantities were referred to the total protein amount measured in each sample. Please note that the nomenclature of lipid species in this manuscript also reflects the level of their structural characterization by tandem MS/MS in accordance to Liebisch et al. (2013).
Determination of Conidiation and Colony Establishment
To quantify conidia production, 15 μL aliquots of strain specific spore suspension (2 × 104 per mL) were plated on Vogel’s agar in 6-well plates (VWR). The spores were harvested from the colonies after 72 h of incubation at 25°C and counted. Uni- and multinuclear conidia were quantified by fluorescence microscopy after fluorescence staining with 5 μM of the nuclei-specific dye Hoechst-33342 (Thermo Fisher Scientific) for 5 min at room temperature. One hundred conidia were analyzed for spore type discrimination. The germination efficiency and germ tube length of 6 h old N. crassa germlings was determined as described (Sonderegger et al., 2017). For the examination of conidial anastomosis tubes (CAT) fusion, 100 μL of a conidia suspension (5 × 105 per mL) were cultivated in 0.2 × Vogel’s medium for 6 h at 25°C before microscopic validation. Experiments were done in duplicates and repeated at least twice.
Investigation of Stress Response
Five μL spore suspension (2 × 104 – 2 × 105 spores/mL) were point inoculated in 24-well plates (VWR) and cultivated for 72 h at 25°C on 500 μL Vogel’s agar/well, supplemented with inducers of ROS: hydrogen peroxide (H2O2, 0–3.5 mM, hydroxyl radicals), menadione (0–0.06 mM; superoxide radicals), tert-butylhydroperoxide (t-BOOH, 0–1.75 mM; organic hydroperoxide). Experiments were done in duplicates and repeated at least twice.
Susceptibility Testing With PAF and PAFB
The antifungal proteins PAF and PAFB were produced and purified from P. chrysogenum cell-free supernatant as described earlier (Huber et al., 2018). Susceptibility tests were performed in 24-well plates (VWR) with 500 μL Vogel’s agar/well, supplemented with increasing concentrations of PAF or PAFB (0–16 μM), respectively. Five μL of a 2 × 105/mL spore suspension were dotted on the agar and the plates were incubated at 25°C. Images were taken after 72 h, as described below.
Broth microdilution assays were performed in 96-well microtiter plates (Thermo Fisher Scientific) using 0.2 × Vogel’s medium as recently described (Huber et al., 2018). The growth was determined spectrophotometrically by measuring the optical density at 620 nm (OD620) with a Fluostar Omega microplate reader (BMG Labtech). The MIC was defined as the protein concentration that inhibited growth by ≥90% compared to the untreated control which was set to be 100%.
The germination efficiency and germ tube length of 6 h old N. crassa conidia in the presence of 1 × MIC PAF (0.06 μM) or PAFB (0.25 μM) was determined as described previously (Sonderegger et al., 2017).
All experiments were done in duplicates and repeated at least twice.
Protein Uptake Studies
Protein labeling using the green fluorophore BODIPY (BP; Life Technologies) was performed as described (Sonderegger et al., 2017). To study the protein uptake in the N. crassa strains, 8 μM BP-labeled PAF or PAFB was added to 70 μL conidia (5 × 105 conidia/mL) in 0.2 × Vogel’s medium and incubated for 12 h at 25°C. To monitor the viability of the fungal cells, co-staining with propidium iodide (5 μg/mL) for 10 min at room temperature was performed. Experiments were done in duplicates and repeated at least twice.
Microscopy and Imaging
The validation of germination efficiency, colony establishment, CAT fusion and AMP susceptibility testing was done with an inverted Leica DM IL LED microscope (Leica Microsystems) and imaging was performed with an AxioCam MR3 camera (Carl Zeiss GmbH). This microscope and imaging system was also used to visualize the edge of colonies grown on solid medium (Supplementary Data Sheet S1, Supplementary Methods). To monitor fungal growth on solid medium, images of samples were taken with a Nikon D5100 SLR camera or a Zeiss DSM950 scanning electron microscope (Carl Zeiss GmbH) (Supplementary Data Sheet S1, Supplementary Methods). For fluorescence microscopy, an Axioplan microscope (Carl Zeiss GmbH), equipped with an AxioCam 503 monochrome camera (excitation/emission filters 365/420 nm for blue fluorescence, 500/535 nm for green fluorescence, 546/590 or 565/620 nm for red fluorescence, Carl Zeiss GmbH) was used for imaging.
Image processing and editing was achieved with the programs ZEN (Carl Zeiss GmbH), Axio Vision software (Carl Zeiss GmbH), GIMP (GNU Image Manipulation Program, version 2.8.201) and Microsoft Power Point (Microsoft Corp.).
Zeta-Potential Measurements
Large unilamellar vesicles (LUVs) were prepared from isolated lipid extracts from wt, Δlac, and Δgcs, respectively, as described (Supplementary Data Sheet S1, Supplementary Methods). Lipids dissolved in chloroform:methanol (2:1, v/v) were evaporated under a stream of nitrogen. After removal of any residual traces of solvent in vacuum overnight, the dried lipid films were hydrated in HEPES buffer at 30°C by intermittent vigorous vortexing. LUVs were obtained by extrusion of the lipid dispersions through a polycarbonate filter (Millipore-IsoporeTM) of 100 nm pore size resulting in LUVs of 100–110 nm in diameter as determined by Zetasizer.
Zeta-potential measurements were performed using the Zetasizer NANO (Malvern Instruments) as described previously (Freire et al., 2011). Briefly, 50 μM of LUVs mixed with defined aliquots of PAF and PAFB, respectively, were injected into the Zeta capillary cell DTS1070 (Malvern Instruments) and equilibrated before measurements for 120 s at 25°C at a constant voltage of 40 V. All samples were prepared in HEPES buffer (10 mM HEPES, 10 mM NaCl, pH 6.8, filtrated through 0.02 μm). Data analysis was processed using the instrumental Malvern’s DTS software to obtain the mean Zeta-potential value calculated from the means of 30 measurements in two experimental repetitions.
Statistical Analysis
If not otherwise stated, statistical analysis was performed using Microsoft Excel 2010 software (Microsoft Corp.). A two-sample t-test with equal variance and one-tailed distribution was applied.
Results
Gene Identification and Verification of Respective Gene Deletion Mutants
Gene candidates involved in the GlcCer biosynthesis were identified in the N. crassa genome2 using the respective C. albicans genes as queries3. All genes investigated in this study and coding for enzymes of the GlcCer pathway in C. albicans were identified in the N. crassa genome by bidirectional NCBI-blast analysis4 (Table 2): NCU02468 (lac) coding for the longevity-assurance protein (ceramide synthase activity), NCU08927 (des-1) encoding the dihydroceramide Δ4-desaturase (Plesofsky et al., 2008), NCU02408 (des-2) encoding the Δ8-sphingolipid desaturase, NCU07859 (smt) encoding the C9-methyltransferase (Ramamoorthy et al., 2009) and NCU01116 (gcs) coding for the GlcCer synthase (Plesofsky et al., 2008). The predicted protein functions were verified with UniProt5. Generally, protein identities and similarities were >30% and >50%, respectively. The open reading frames (ORFs) of the N. crassa genes were analyzed for intron/exon structure, chromosome localization and gene product length. A summary is given in Table 2 and Supplementary Data Sheet S1, Supplementary Table S4. A proposed scheme of the N. crassa GlcCer pathway is presented in Figure 1.
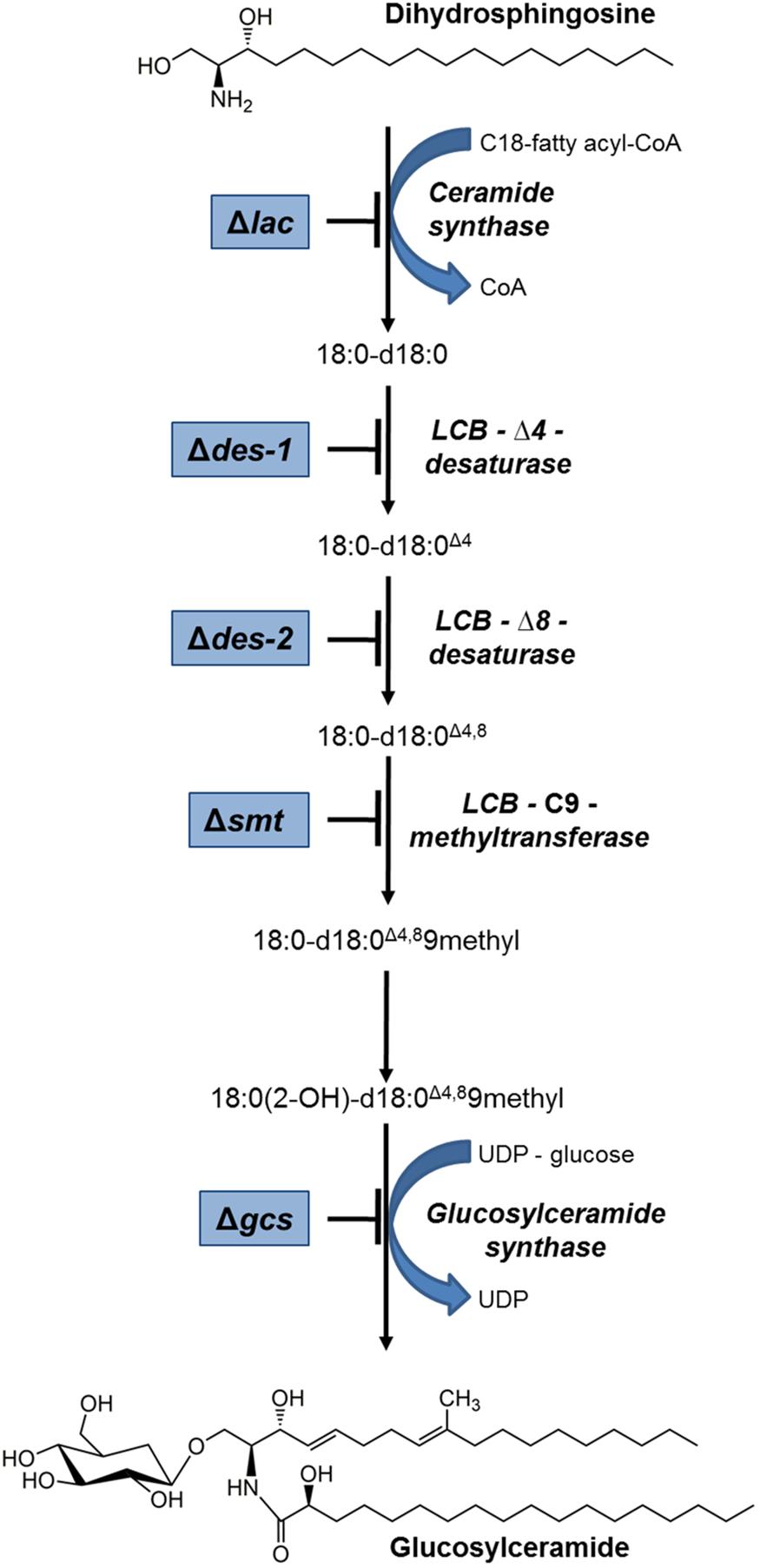
Figure 1. Schematic overview of the GlcCer pathway in N. crassa. Mutants that were used in this study are framed in blue and their defective enzyme function are indicated. Structures were plotted with ChemBioDraw (ChemBioOffice).
To analyze the membrane lipids and correlate the distinct lipid patterns with the overall fitness of N. crassa, we took advantage of the genome-wide deletion library of this model fungus to analyze mutant strains with deleted genes that code for the enzymes involved in the GlcCer biosynthesis: Δlac, Δdes-1, Δdes-2, Δsmt, and Δgcs (Dunlap et al., 2007). These genes were deleted by replacement with the hygR cassette (Colot et al., 2006; Park et al., 2011). Respective reference strains were the wildtype (wt) strain mating-type mat a [isogenic strain ORS-SL6a (FGSC4200) for Δlac, Δdes-2, and Δsmt] and mat A [isogenic strain 74-OR23-1VA (FGSC2489) for Δdes-1 and Δgcs] (Table 1). The replacement of the respective genes by the hygR cassette was verified in all mutants with PCR (Supplementary Data Sheet S1, Supplementary Methods, Supplementary Figure S1, Supplementary Table S5). All mutants were homokaryons, except for Δsmt, which exists only as heterokaryon. The genetic background of the Δsmt was confirmed by analysis of smt gene expression and growth in the presence of PT and hygromycin (Supplementary Data Sheet S1, Supplementary Figure S2). The existence of Δsmt as heterokaryon suggests that the C9-methyltransferase is an essential enzyme for N. crassa viability, similar to the situation reported for the unicellular fungi P. pastoris and C. neoformans (Ternes et al., 2006; Ramamoorthy et al., 2009), but contrasting with A. nidulans and F. graminearum, which possess two sphingolipid C9-methyltransferases, which are suggested to exhibit redundant enzymatic functions (Ramamoorthy et al., 2009; Fernandes et al., 2016). For completeness, we want to mention here that we have also tested the N. crassa NCU00008 strain (FGSC13263), which is defective in gsl-4, a second ceramide synthase encoding gene present in the genome of this model fungus (Case et al., 2014). The gene gsl-4 is a lac-1 paralog and ortholog of the gene LAG1 from Saccharomyces cerevisiae (Schorling et al., 2001) and lagA from Aspergillus sp. (Cheng et al., 2001; Fontaine, 2017), and is implicated in the inositol phosphorylceramide (IPC) synthesis pathway. But since this mutant showed no difference in its phenotype compared to the wt in respect to vegetative growth, conidiation and susceptibility to AMPs (data not shown), we decided to focus in this study on the characterization of the mutants of the GlcCer biosynthesis pathway rather than the IPC pathway.
Impaired Lipid Composition in N. crassa GlcCer Mutants
To characterize the lipidomic phenotypes caused by the deletion of individual genes involved in GlcCer biosynthesis in N. crassa, we performed liquid chromatography (LC-) MS/MS lipid analysis with a special focus on ceramides and glycosphingolipids (putative chemical structures are shown in Supplementary Data Sheet S1, Supplementary Figure S3). Additionally, abundant features related to lipid classes including PC, PE, phosphatidylinositol (PI) and cardiolipin (CL) were also covered (Figure 2 and Supplementary Data Sheet S1, Supplementary Figure S4, Supplementary Table S3). This analysis revealed a clear knock-out specific alteration of the ceramide and GlcCer state of cells, allowing to verify all mutant strains, respectively (Figure 2A). Notably, total levels of unrelated phospholipids (including PC, PE, PI and CL) and their respective species compositions remained unchanged (Figure 2B). The quantification of the lipids can be found in Supplementary Table S1.
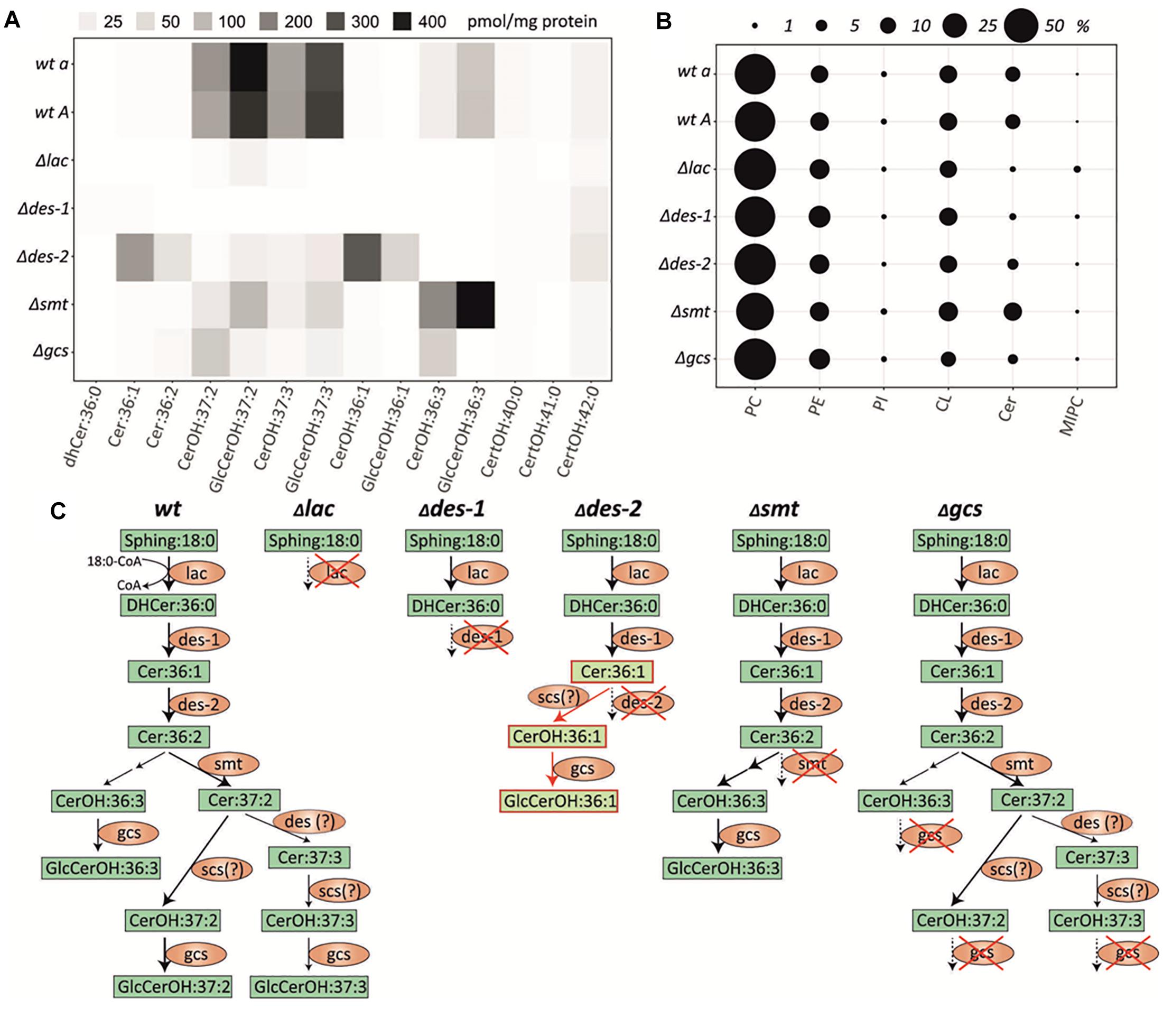
Figure 2. Lipid quantification in the N. crassa GlcCer knockout mutants and wildtype strains. (A) Heatmap of individual quantifiable ceramide species (means of n = 5, for values see Supplementary Data Sheet S1, Supplementary Table S3). (B) Total abundance of quantified lipid classes in each strain, respectively. PC, phosphatidylcholin; PE, phosphatidylethanolamine; PI, phosphatidylinositol; CL, cardiolipin; Cer, ceramide; MIPC, mannosylinositol phosphorylceramide. (C) Schematic depiction of the ceramide synthesis pathway in N. crassa wt and GlcCer mutants Δlac, Δdes-1, Δdes-2, Δsmt, Δgcs based on the results shown in (A). Fatty acid hydroxylation of ceramides by the putative C. albicans scs7 orthologous gene product (NCU03492) of N. crassa. A respective N. crassa scs deletion mutant was not included in this study. Green box, lipid species; Orange ellipse, genes/enzymes; Red framed light green box, lipids from alternative pathway; Red cross, respective gene knock-out; Question mark: putative enzymatic step.
Both wt strains (FGSC4200 and FGSC2489) were able to generate GlcCerOH:37:2, GlcCerOH:37:3, CerOH:37:2, and CerOH:37:3 (Figure 2C). These ceramide species were already identified in other fungal species, as reported by Leipelt et al. (2001).
Furthermore, non-hydroxylated ceramide levels were below the limit of detection in the wt. In most cases the deletion of GlcCer biosynthetic genes resulted in blockage of the pathway resulting in the absence of downstream products. For example, the missing ceramide synthase function in the Δlac knockout caused a complete deficiency of all pathway specific ceramides and GlcCer species. At the same time the phytoCeramide pathway, that utilizes hydroxylated very-long chain fatty acids (C22:0-C24:0) remained unaffected, but further downstream the mannosylinositol phosphorylceramide (MIPC) were accumulated (Figure 2B and Supplementary Data Sheet S1, Supplementary Figure S4). A very similar phenotype was found in the LCB-Δ4-desaturase mutant (Δdes-1), where the subsequent Δ4-desaturation step is blocked. However, we found no alteration of the MIPC pathway and no accumulation of its dihydro-ceramide precursor in this mutant. In contrast, blockage of the Δ8-desaturation step in the LCB-Δ8-desaturase mutant (Δdes-2) caused an accumulation of its direct precursor Cer:36:1 and enabled flux into a second alternative metabolic route by hydroxylation – but not methylation – and subsequent glycosylation to form GlcCerOH:36:1, which was undetectable in the wt strains (Figure 2A). Additionally, small amounts of the regular GlcCerOH:37:2 and GlcCerOH:37:3 products were detected that could potentially be generated by an unspecific desaturase activity. Interestingly, the missing methyltransferase activity in the LCB-C9-methyltransferase knockout (Δsmt) caused a strong diversion into the GlcCerOH:36:3 forming pathway, which we found only slightly active in the wt. Finally, the GlcCer synthase mutant (Δgcs) almost completely prevented the glycosylation of the CerOH species detected in this study, suggesting a broad recognition of hydroxylated, but not necessarily methylated, ceramides in this step. We further analyzed the mass spectrometric data for the presence of potentially relevant ceramide species reported elsewhere (Costantino et al., 2011; Tian et al., 2015; Singh et al., 2017), but could not detect any additional species.
In summary, while all mutants could be characterized by their specific lipid phenotype, none of them was able to generate the final GlcCer:OH37:2 and GlcCer:OH37:3 products in significant amounts compared to the wt strains. The quantity and composition of most other lipid classes including phytoCeramide predominantly Cer[t18:0/24:0(2OH)] was not significantly altered in any mutant (Supplementary Data Sheet S1, Supplementary Figure S4), however, MIPC were elevated in the Δlac mutant.
Phenotypical Characterization of GlcCer Depletion Mutants
Both wt strains (FGSC4200 and FGSC2489) were included as controls in all experiments described below. No difference in the phenotype between these reference strains could be observed. For simplicity reasons, we therefore refer in the following to wt as control/reference strain.
GlcCer Depletion Affects Growth, Asexual Development and Colony Establishment
We first studied the effect of depletion of intermediates of the GlcCer synthesis pathway on N. crassa strains grown on solid medium (Figure 3). While the reference strain colonized the whole surface, the mutant strains showed disturbed growth with limited radial expansion (Table 3), resulting in small, compact, sharp-edged colonies, except for the mutants Δsmt and Δgcs, which had a frayed appearance (Figure 3). Microscopy of the colony edges revealed a hyperbranched morphology of all mutants compared to the wt (Supplementary Data Sheet S1, Supplementary Figure S5).
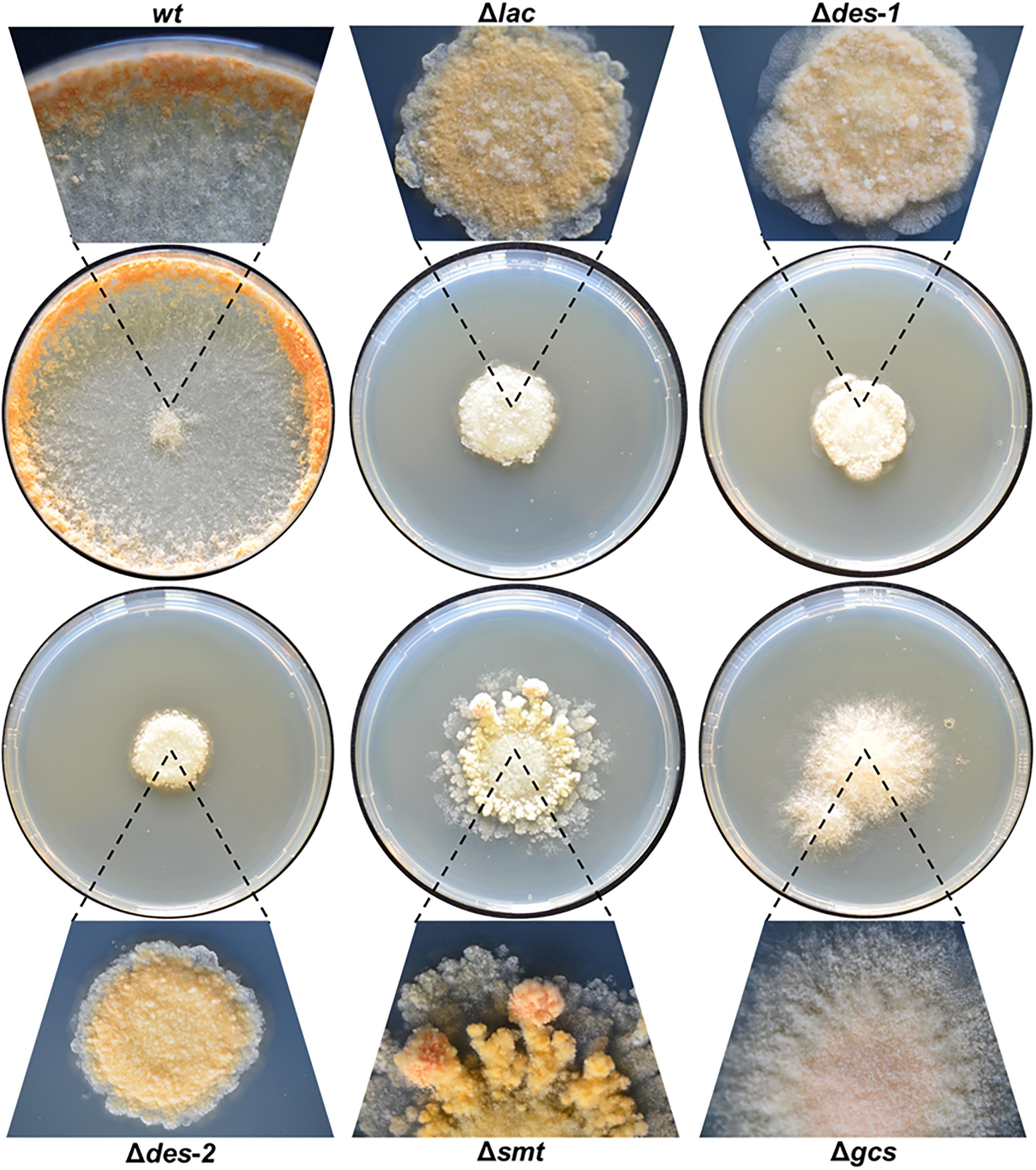
Figure 3. Effect of GlcCer depletion on colony formation of N. crassa. Conidia (103) were point inoculated on Vogel’s agar and grown for 72 h at 25°C. Magnifications of the colonies are presented for each strain.
The conidiation efficiency was significantly reduced in the mutants Δlac, Δdes-1, Δdes-2, and Δsmt in contrast to the Δgcs strain that generated a similar amount of conidia compared to the wt strain (Table 4 and Supplementary Data Sheet S1, Supplementary Figure S6). Importantly, N. crassa produces two different types of asexual reproductive organs: the uninucleate microconidia and the multinucleate macroconidia (Springer and Yanofsky, 1989; Bistis et al., 2003). When analyzing the conidia types we observed a prominent shift from 33% microconidia and 67% macroconidia in the reference strain toward more than 90% macroconidia in the mutant strains (Table 4). Among these macroconidia, the mutants Δdes-1 and Δgcs produced predominantly arthroconidia, whereas mostly blastoconidia were found in the wt and the other mutants (Figure 4).
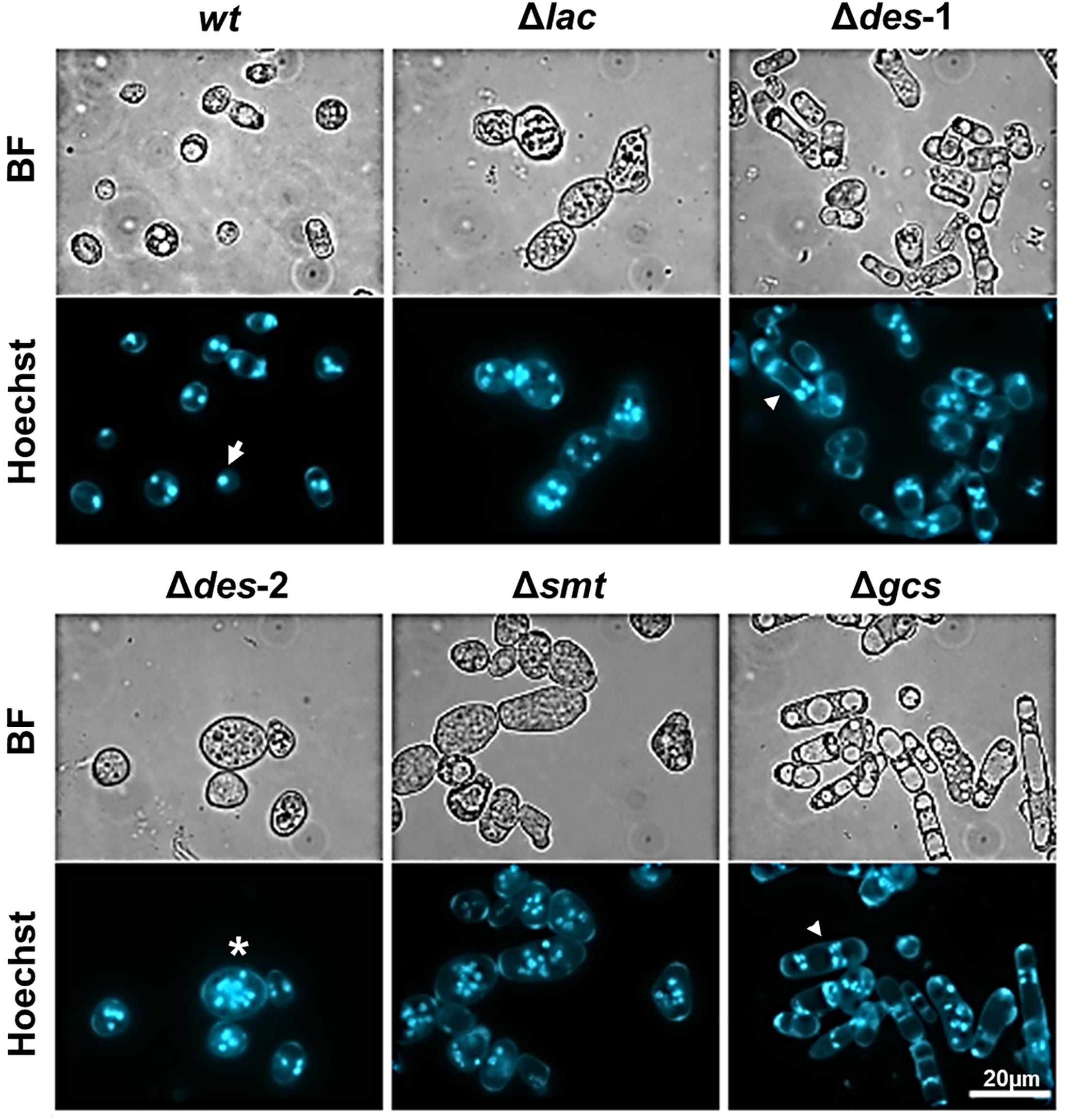
Figure 4. Effect of GlcCer depletion on the formation of uninuclear and multinuclear conidia. Samples were stained for 5 min with Hoechst 33342 before imaging. The white arrow shows an example for uninuclear conidia, the asterisk indicates an example for multinuclear blastoconidia and the arrowhead for multinuclear arthroconidia. BF, Brightfield. Scale bar 20 μm.
We next determined the phenotype of the mutants in submerse growth conditions. The germination efficiency of all strains was similar (Table 5, control). The germ tube length reached 53.4 ± 6.9% in the control strain and was similar in the mutants, except for the Δgcs strain, which suffered from a significant growth retardation (36.7 ± 2.8%; p ≤ 0.05) compared to the control (Table 6 and Figure 5A). The overall dry biomass, however, was similar in all tested strains after 72 h of cultivation, which indicates that the Δgcs mutant is able to overcome this early growth defect (Supplementary Data Sheet S1, Supplementary Figure S7). Notably, all mutants were impaired in polarized germination and exhibited mostly two germ tubes compared to predominantly one in the reference strain (Table 7). No defects in CAT fusion could be observed in any of the strains tested (Figure 5B).
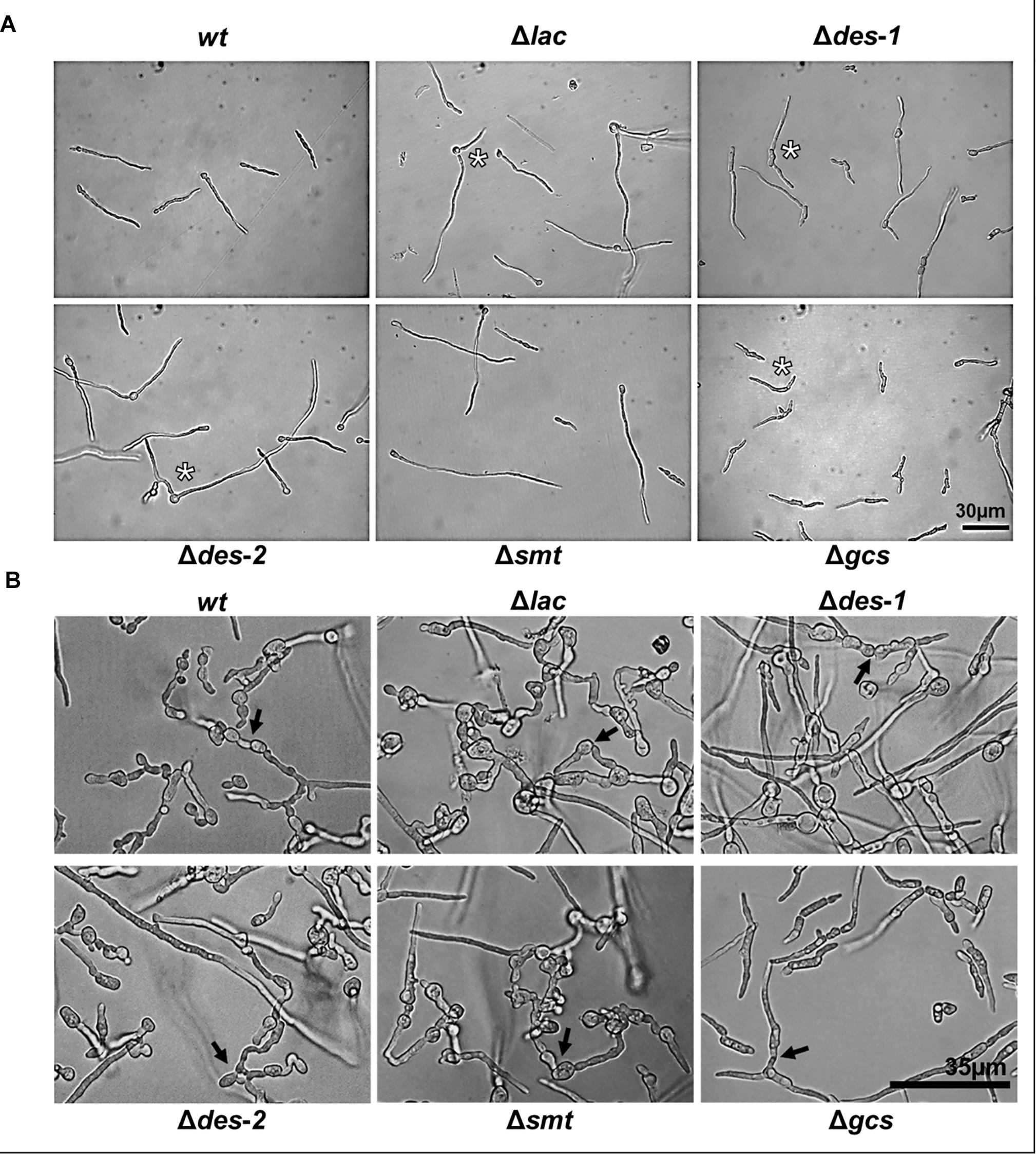
Figure 5. Effect of GlcCer depletion on germination and CAT-fusion of N. crassa. (A) Phenotypes of 6 h old germlings: 104 conidia/mL were incubated in 0.2 × Vogel’s medium. Asterisks indicate multiple germ tubes in Δlac, Δdes-1, Δdes-2, and Δgcs mutants. (B) CAT-fusion: (5 × 105 conidia/mL were cultivated for 6 h in 0.2 × Vogel’s medium). Black arrows indicate CAT-fusion. Scale bar 30 μm in (A), scale bar 35 μm in (B).
Defective GlcCer Synthesis Affects Sensitivity to Oxidative Stress
Ceramide is an important signaling molecule in the induction of ROS and of apoptosis (Ueda, 2015). Depletion in ceramide was referred to lower ROS levels and higher survival rates (Portt et al., 2011). To investigate if the GlcCer synthesis pathway plays a role in oxidative stress response in N. crassa, we exposed the mutant strains to increasing concentrations of ROS generators: menadione (superoxide radicals), hydrogen peroxide (H2O2; hydroxyl radicals) and tert-butylhydroperoxide (t-BOOH; organic hydroperoxide). Figure 6 shows that the reference strain and the Δgcs mutant tolerated 0.0025 mM menadione, 2 mM H2O2 and 1 mM t-BOOH. The Δlac strain, in contrast, was resistant within the tested concentration ranges of menadione (up to 0.05 mM), H2O2 (up to 3 mM), but showed the same sensitivity toward t-BOOH as the reference strain. The mutants Δdes-1, Δdes-2, and Δsmt were menadione resistant, but H2O2 and t-BOOH sensitive (Figure 6). These results indicate that distinct intermediates of the GlcCer synthesis pathway regulate the sensitivity toward superoxide and hydroxyl radicals but not toward organic hydroperoxides.
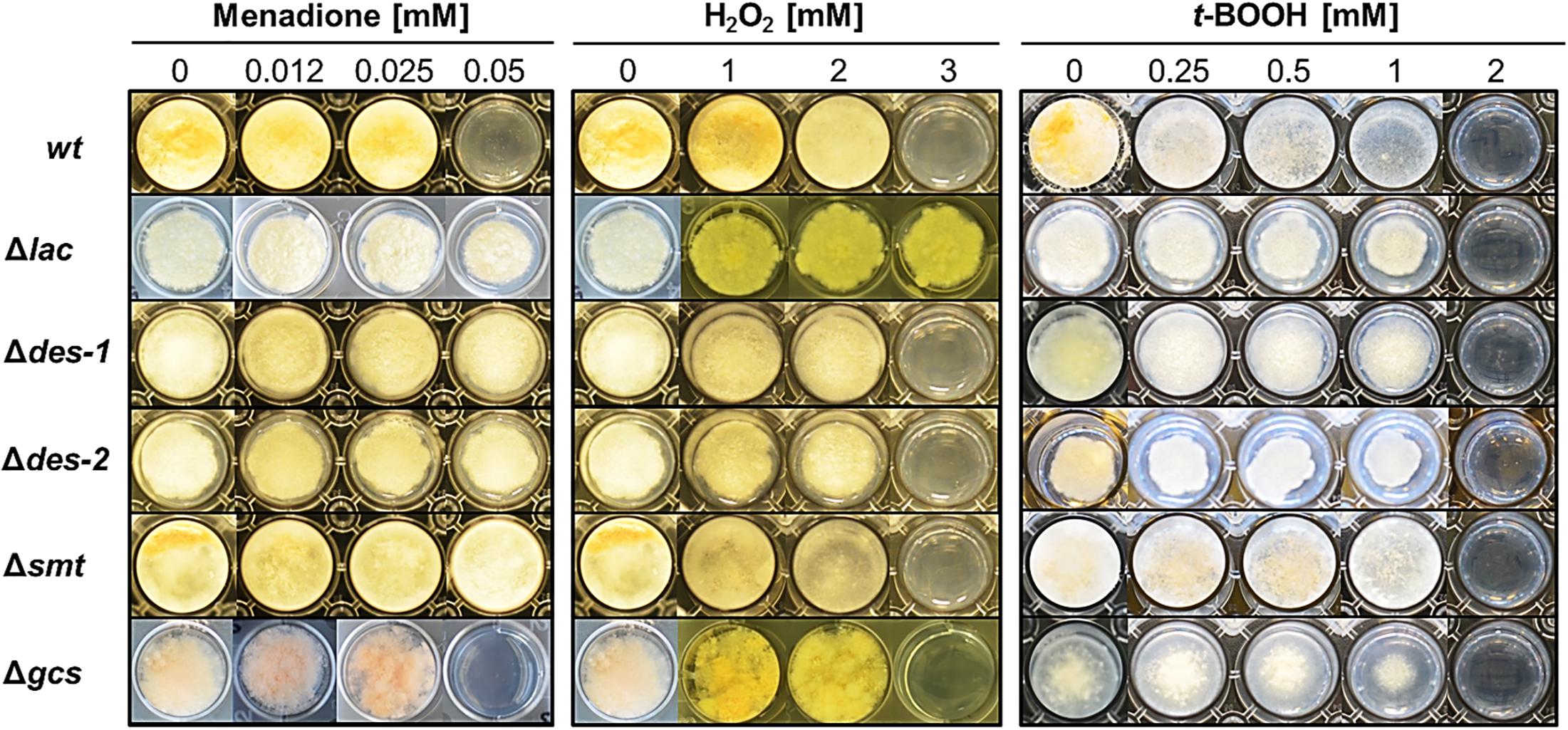
Figure 6. Impact of GlcCer depletion on oxidative stress response. Conidia (103) were point inoculated on Vogel’s agar (0.75%) containing increasing concentrations of oxidative stress inducers menadione (superoxide radical), H2O2 (hydroxyl radicals) and tert-butylhydroperoxide (t-BOOH; organic hydroperoxide). Samples were cultivated for 72 h at 25°C.
GlcCer Mutants Are Resistant Against PAF but Not PAFB
Next, the GlcCer mutants were tested for their susceptibility to the P. chrysogenum AMPs, PAF and PAFB, to investigate whether their antifungal mode of action is regulated by this specific pathway. A concentration dependent growth reduction of the point inoculated wt strains on solid medium was observed at a MIC of 1 μM PAF and 4 μM PAFB, respectively (Supplementary Data Sheet S1, Supplementary Figure S8). The growth of the Δlac strain was visibly reduced at 0.5 μM PAF, but reduction was not further aggravated with increasing PAF concentrations (up to 16 μM) (Supplementary Data Sheet S1, Supplementary Figure S9A). The same resistant phenotype within this concentration range was detected for the mutants Δdes-1, Δdes-2, Δsmt, and Δgcs (Supplementary Data Sheet S1, Supplementary Figure S9A). In contrast, a concentration dependent PAFB sensitive phenotype was observed for all mutant strains (Supplementary Data Sheet S1, Supplementary Figure S9B).
Broth microdilution assays reflected the results obtained with cultures grown on solid medium. Generally, the growth of N. crassa in submerged cultures is inhibited with lower AMP concentrations than on solid media (Huber et al., 2018). The MIC for the wt strain was 0.06 μM for PAF and 0.25 μM for PAFB, respectively (Figure 7). All mutant strains proliferated within a PAF concentration range of 0.06–1 μM, though the hyphae were short and hyperbranched as observed in the PAF-treated wt (Figure 7A). The germination efficiency of the mutants was similar to that of the wt (84.1 ± 3.1%) irrespective of PAF treatment (Table 5) and the germ tube length was only mildly affected - if at all - by PAF (Table 6). In contrast, the mutants were as susceptible to PAFB as the wt (MIC 0.25 μM) (Figure 7B). The inhibitory activity of PAFB was also reflected in significantly reduced germination efficiency (Table 5) and significantly shorter germ tubes of all strains tested (Table 6).
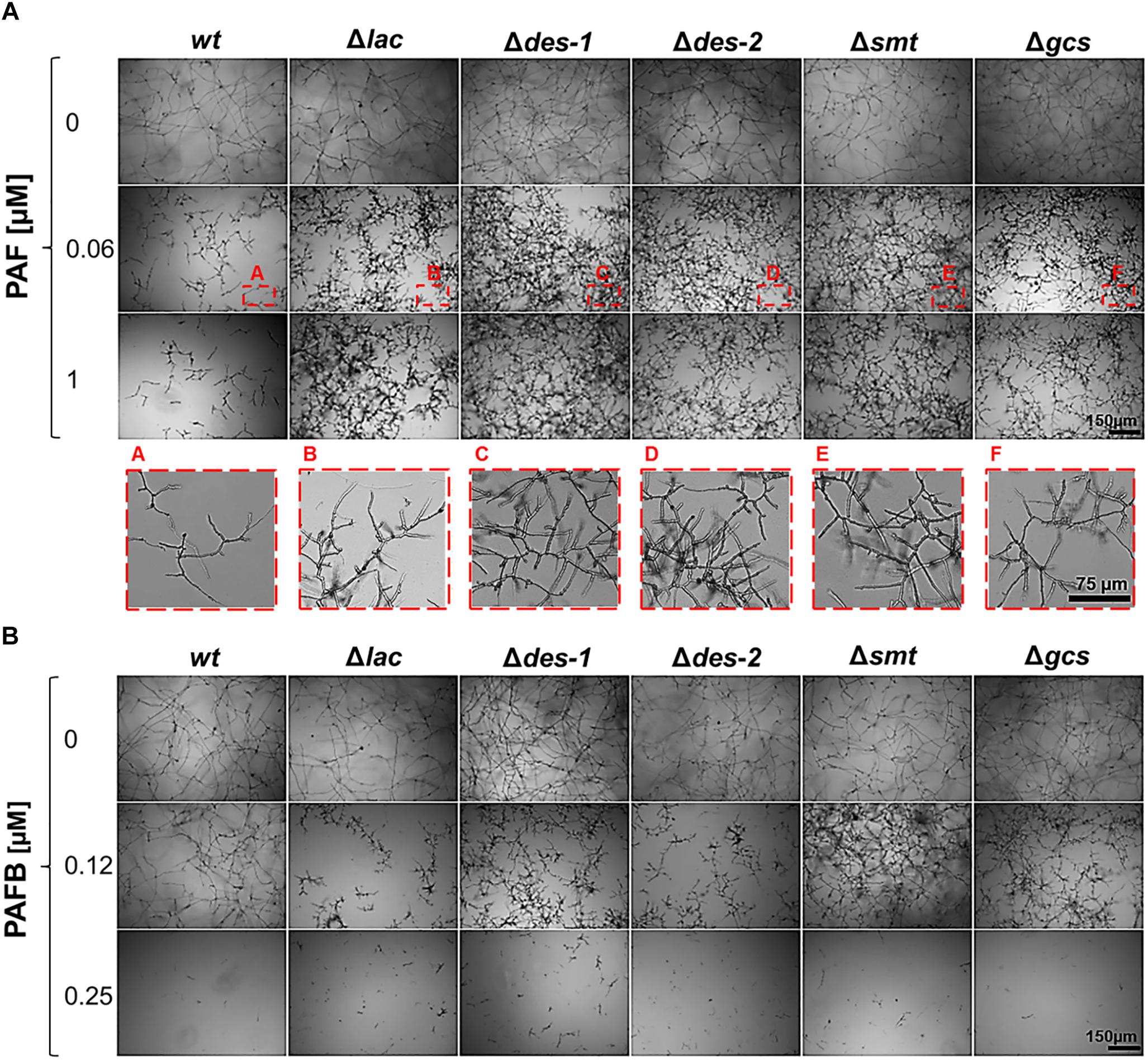
Figure 7. Impact of GlcCer depletion on the AMP susceptibility. (A) Sensitivity toward PAF: conidia were grown in the presence of increasing PAF concentrations (0–1 μM) for 30 h. To visualize the mycelial growth at 0.06 μM PAF (corresponding to the MIC of the wt strain), magnifications of the insets A–F (framed in red) are presented in the lower panel. (B) Sensitivity toward PAFB: conidia were grown in the presence of increasing PAFB concentrations (0–0.25 μM). Scale bar 150 μm and 75 μm.
The GlcCer Mutants Are Defective in PAF Interaction
In previous studies, we reported that the uptake of P. chrysogenum AMPs and their cytoplasmic localization in sensitive fungi is a regulated process and triggers fungal cell death (Oberparleiter et al., 2003; Sonderegger et al., 2017; Huber et al., 2018). In this study we investigated, to which extent the GlcCer synthesis pathway regulates the interaction of PAF with N. crassa. Since PAFB acted in a GlcCer independent way, we used this AMP as a control. To visualize their cellular localization, the N. crassa strains were exposed to both BP labeled AMPs (BP-PAF and BP-PAFB) (Huber et al., 2018). Figure 8 shows that BP-PAF and BP-PAFB were taken up in the reference strain where they localized intracellularly. Co-staining with the cell death dye propidium iodide coincided with positive AMP staining in the treated cells and reflected membrane permeabilization and cell death as reported previously (Huber et al., 2018). Interestingly, no or only a very weak BP-PAF and propidium iodide signal could be observed when mutant strains were treated with BP-PAF (applying the same exposure time as for the reference strain) (Figure 8A). When increasing the signal intensity, the residual BP-PAF signals were localized in cellular compartments but not in the cytoplasm (Figure 8A). In contrast, in all mutant strains a wt-like localization of BP-PAFB could be confirmed (Figure 8B).
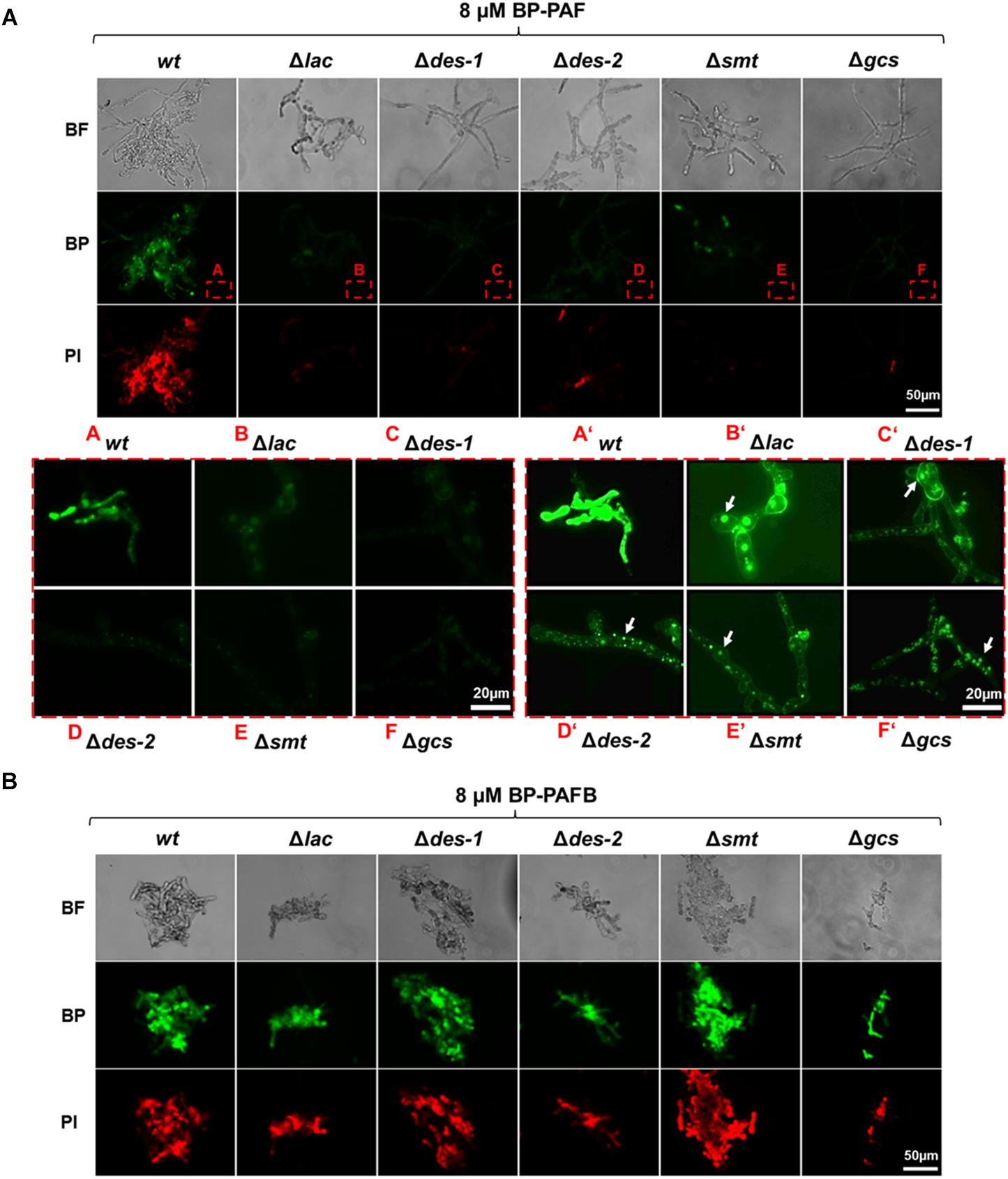
Figure 8. Uptake of BP-PAF and BP-PAFB in germlings of N. crassa mutants. Samples were taken after 12 h of incubation of conidia with BP-labeled AMPs. Co-staining with propidium iodide was performed 10 min before imaging. Images were taken with the same exposition time (1500 ms). (A) Upper panel: Overview of BP-PAF treated germlings; Red framed lower panel left A–F: Magnification of BP-PAF treated germlings; Framed lower panel right A’–F’: Magnification of BP-PAF treated germlings with increased signal intensity. White arrows indicate BP-PAF uptake into intracellular compartments. (B) Overview of BP-PAFB treated samples. BF, Brightfield; BP, BODIPY-labeled proteins; PI, Propidium iodide. Scale bar 50 and 20 μm in (A), scale bar 50 μm in (B).
In vitro Protein-Lipid Interaction Is Electrostatically Driven
Zeta-potential measurements were performed to investigate the interaction between PAF and PAFB with membrane lipids as this method reflects on electrostatic interactions, which presumably plays an important role in the binding of the cationic AMPs with the anionic lipid membranes. Thus, titration of LUVs with AMPs was performed in order to monitor if the accessible surface charge is affected, which in turn influences the Zeta-potential (Domingues et al., 2008). Lipid analysis showed that the various mutant strains mainly differed in the amount of PIs and the amount and nature of ceramides (Supplementary Data Sheet S1, Supplementary Figure S4). In addition to lipid extracts of the wt, extracts of Δlac and Δgcs were selected. Besides the lack of glycosylated ceramides both mutants had reduced levels of anionic PIs, whereas the Δlac exhibited markedly increased levels of anionic MIPCs. This difference in lipid composition is reflected in the negative Zeta-potential of the lipid extracts being lowest (-39 mV) for Δlac and highest (-29 mV) for Δgcs. The Zeta-potential for wt was in between the lipid extracts of the two mutants (-34 mV).
Addition of PAF to the lipid extracts of both wt and Δlac progressively altered the Zeta-potential toward a neutral threshold, which was reached at a lipid-to-peptide molar ratio (L:P) of about 3:1 (Figure 9). Adding PAF to lipid extracts of Δgcs only induced a minor change of the Zeta-potential leveling of around -20 mV. In contrast, PAFB induced a dramatic increase of the Zeta-potential to a value of about -5 mV already at a lipid-to-peptide molar ratio of 100:1 leading to neutralization of the surface charge around L:P 25:1 (Figure 9). A further increase of the protein concentration led to an overcompensation of the surface charge. The effect of PAFB on LUVs of Δgcs was less pronounced, but led to a stronger decrease of the Zeta-potential as compared to PAF (-11 vs. -19 mV). In summary, binding of the AMPs to lipid extracts of Δlac was prominent owing to the higher amount of anionic lipids emphasizing the role of electrostatic interactions.
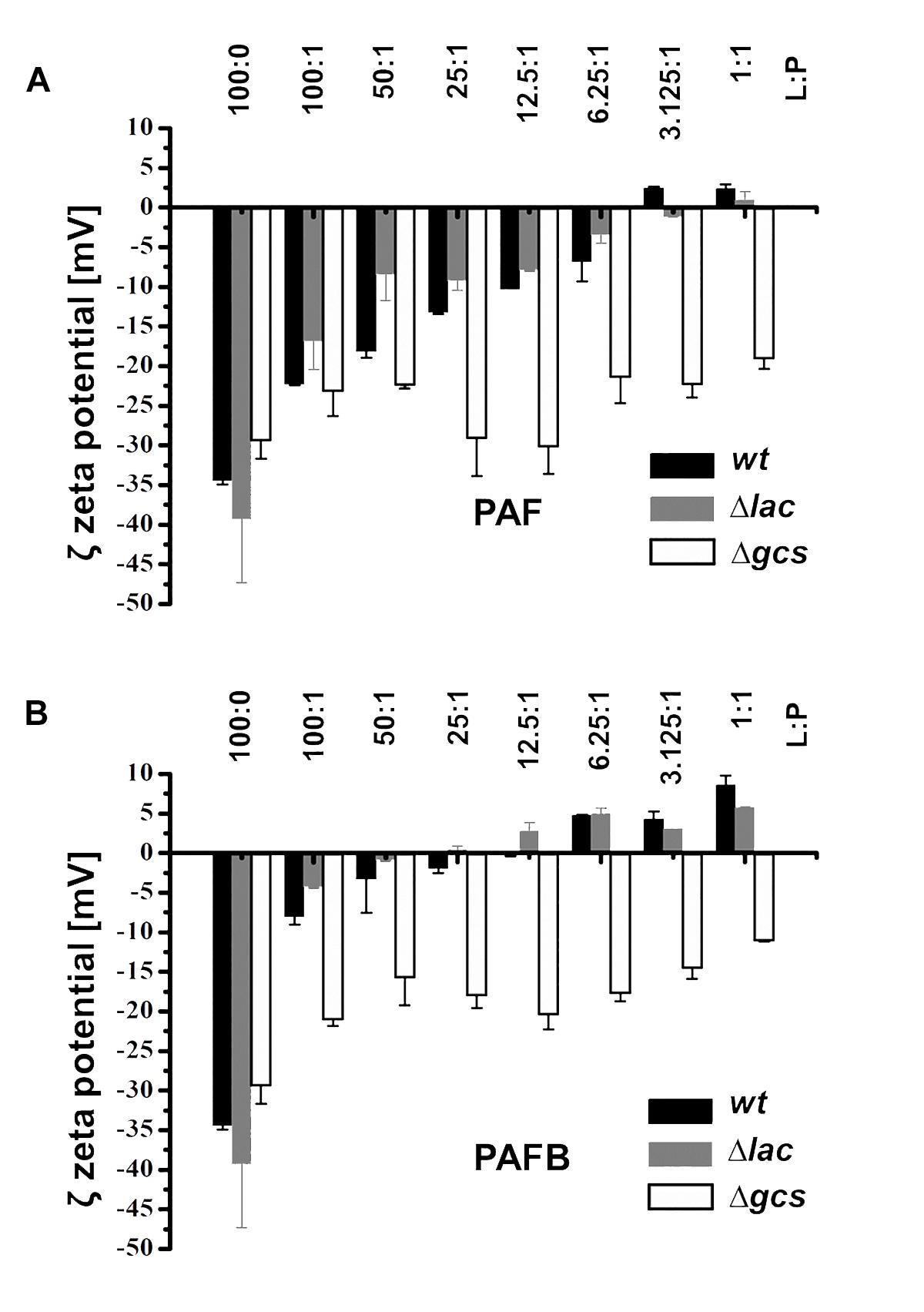
Figure 9. Impact of PAF and PAFB on the surface charge of N. crassa lipid vesicles. Zeta potential (mV) of N. crassa LUVs obtained from lipid extracts of wt (black bars), Δlac (gray bars) and Δgcs (white bars) in the absence and presence of (A) PAF and (B) PAFB. The AMP concentration was used in relation to 50 μM of LUVs and is expressed as lipid-to-protein molar ratio (L:P). Results are means of 30 measurements in two replicates.
Discussion
In this study, we carefully investigated the role of the enzymes involved in the GlcCer synthesis pathway in the N. crassa membrane lipid composition, growth, conidiation, morphology, stress sensitivity and susceptibility to the P. chrysogenum AMPs, PAF and PAFB.
To our best knowledge, this is the first comprehensive report of the contribution of enzymes involved in the GlcCer synthesis pathway to the phenotype of this model fungus. The lack of downstream lipid intermediates and differences in sphingolipid types fully reflected the respective gene deletions in the five analyzed N. crassa mutant strains (Δlac, Δdes-1, Δdes-2, Δsmt, Δgcs) and confirmed the identity of the mutants.
Whole-cell lipid extracts analyzed in an untargeted lipidomics approach yielded lipid levels independently of their subcellular location including for example the mitochondria-specific CLs (Schlame and Greenberg, 2017). Extracted features contained all known ceramides and phytoCeramides including their intermediates that were described by Leipelt et al. (2001). Interestingly, phytoCeramides with long chain fatty acids were far less abundant, as were their downstream MIPC products, except for the Δlac strain, where they were slightly accumulated. Non-detectable lipids could still be present in trace amounts at levels below the limit of detection. In all mutant strains as well as the control strains, the phospholipid content as well as its lipid profile remained unchanged and were thus not affected by any of the GlcCer biosynthesis gene deletions.
The determination of GlcCer abundance in null mutant strains provided new insights into this synthesis pathway, which shows in part similarities but also differences to that of other fungi. The mutants Δlac, Δdes-1, and Δgcs were completely impaired in GlcCer synthesis, whereas the Δdes-2 and Δsmt mutants exhibited alternative branching of the pathway ending up in the production of the glycosylated sphingolipids, GlcCerOH:36:1 and GlcCerOH:36:3, respectively. Interestingly, such alternative branching patterns have not been described so far in the A. nidulans C9-methyltransferase mutant (ΔsmtA), which produced wt-like GlcCer, whereas in the ΔsmtB mutant increased levels of unmethylated GlcCer have been detected. The A. nidulans ΔsdeA mutant (lacking the sphingolipid Δ8-desaturase) accumulated C8-saturated and unmethylated GlcCer (Fernandes et al., 2016).
The change in the membrane sphingolipid composition resulted in a growth defect of N. crassa: the mutants showed severe growth retardation and disruption of polarized hyphal extension when grown on solid medium. Similar defects have been repeatedly observed in other fungal species defective in ceramide synthase (A. nidulans, barA1), sphingolipid Δ8-desaturase (A. nidulans, ΔsdeA; C. albicans, Δsld), C9-methyltransferase (F. graminearum, ΔFgmt2; C. albicans, Δmts1), and GlcCer synthase (A. nidulans, ΔgcsA; C. albicans, Δhsx11) (Li et al., 2006; Oura and Kajiwara, 2008, 2010; Ramamoorthy et al., 2009; Fernandes et al., 2016).
Similar to the F. graminearum mutant ΔBAR1 lacking the ceramide synthase (Rittenour et al., 2011), all N. crassa mutants – except for the Δgcs mutant – produced less conidia than the reference strain. Interestingly, all N. crassa mutants exhibited a pronounced shift toward multinuclear macroconidia, which suggests the interference of GlcCer synthesis products in conidial development. Specifically, the observation that the mutants Δdes-2 and Δgcs produced predominantly arthroconidia and little blastoconidia lets us speculate that these enzymatic steps play an important role in conidiophore development and/or in the developmental timeline of conidiation (Springer and Yanofsky, 1989). In contrast, the A. nidulans Δgcs suffered from a severe reduction of conidia (Fernandes et al., 2016). Our results thus allow us further to conclude that glycosylated sphingolipids play a variable role in the conidiation efficiency of different fungal species.
Defects in polarized germination, but not in germination efficiency, could be observed in all N. crassa mutants, which mostly exhibited two germ tubes compared to predominantly one of the wt strain. A slight reduction in the germ tube length of the Δgcs mutant after 6 h of incubation pointed toward a mild defect in germ tube elongation during early growth phase. We conclude that the glycosylation of CerOH:36:3, CerOH:37:2 and CerOH:37:3 supports fast and efficient colony establishment under liquid conditions (Araujo-Palomares et al., 2007), though the GlcCer depletion had no adverse impact on the fungal biomass after longer growth times. The CAT fusion ability in the N. crassa mutants was not affected by any of the gene deletions, which also matched a previous report for the N. crassa Δgcs mutant (Chu, 2013).
It is well known that ceramides and other sphingolipids play an important role in cell signaling (Epstein and Riezman, 2013), e.g., they are involved in oxidative stress response and cell death mechanisms (Barth et al., 2010). Until now, the contribution of GlcCer in oxidative stress response in fungi has been only sparsely characterized. Our study demonstrates that the GlcCer pathway plays no role in organic hydroperoxide (t-BOOH) sensitivity, whereas all N. crassa mutants were resistant to superoxide radicals (menadione), except for the Δgcs mutant, which was comparably susceptible to menadione as the wt. This indicates that the modification of the ceramide fatty acid rather than the presence of a sugar residue regulates the stress response to menadione. The Δlac mutant, however, was the only strain resistant to H2O2. This stands in contrast to reports on a respective C. neoformans (Δcer1) mutant lacking the ceramide synthase, which developed a hypersensitive phenotype when grown in the presence of H2O2 (Munshi et al., 2018). Additionally, a connection between MIPCs and oxidative stress has been reported for S. cerevisiae: reduced M(IP)2C levels rendered yeast cells more resistant to ROS induced by H2O2 (Aerts et al., 2006). Surprisingly and in contrast to these findings, the amount of MIPCs was increased in the H2O2 resistant N. crassa Δlac mutant. Due to the lack of respective data from other filamentous fungi, we assume that the total loss of GlcCer intermediates differently affects the stress response of N. crassa compared to yeasts. This, however, awaits further investigation.
Many reports handled fungal sphingolipids as possible binding targets for plant defensins. In vitro experiments indicated that the Raphanus sativus antifungal protein 2 (RsAFP2) or the Pisum sativum defensin 1 (Psd1) interact with fungal GlcCer (Thevissen et al., 2004; de Medeiros et al., 2014). Defects in GlcCer synthesis were associated with lower susceptibility or even resistance of fungi against certain plant defenins (Ramamoorthy et al., 2007, 2009; Fernandes et al., 2016).
Here we could show that the intermediates and the end products of the GlcCer pathway determine the susceptibility of N. crassa toward PAF, but not PAFB. All mutants lacking enzymes in the GlcCer biosynthesis exhibited a PAF-resistant phenotype. The energy-dependent uptake and cytoplasmic localization in PAF/PAFB-exposed sensitive fungi is a prerequisite for antifungal activity as reported previously (Oberparleiter et al., 2003; Sonderegger et al., 2017; Huber et al., 2018). The GlcCer mutants showed a disturbed interaction with PAF resulting in significantly diminished protein internalization. In contrast, we could not detect any GlcCer-dependent resistance toward PAFB and its intracellular localization coincided with cell death in N. crassa. This is not impossible, since different mechanisms of action have been also described for plant defensins that share high structural similarities (Cools et al., 2017).
Zeta-potential measurements performed with LUVs composed of lipid extracts derived from wt, Δlac and Δgcs emphasize that electrostatic interaction is a driving force for the affinity between the P. chrysogenum derived AMPs and lipid membranes. PAFB, exhibiting a higher net positive charge than PAF (+5.2 vs. +4.7, respectively) (Huber et al., 2018; Sonderegger et al., 2018), neutralized the membrane surface charge at much lower concentrations and both AMPs bound more efficiently to LUVs of the Δlac than the wt strain. One may speculate that this is due to the increase of anionic MIPC in this mutant, exhibiting a high affinity for PAFB. This in turn could partially compensate for the lack of GlcCerOH:36:3, GlcCerOH:37:2, and GlcCerOH:37:3 and is in line with the uptake of PAFB in the N. crassa GlcCer mutants. This finding supports a recent computational modeling approach, which showed that adsorption of the antifungal protein AFP from Aspergillus giganteus is mainly driven by electrostatic attraction between the cationic protein and the acidic membrane surface (Utesch et al., 2018). Interestingly, none of the two P. chrysogenum AMPs was able to neutralize the negative Zeta-potential of LUVs derived from the mutant Δgcs within the investigated concentration range proposing a role of the sugar moiety for high affinity binding of AMPs as suggested also by Utesch et al. (2018).
Importantly, our study evidences that differences in the GlcCer pathway intermediates and their functional role exist between N. crassa and other fungal species. Furthermore, we show that the antifungal mode of action of PAF and PAFB differs despite their considerable sequence and structural similarity. Both AMPs follow diverging underlying molecular mechanisms: a GlcCer-dependent route for PAF and a GlcCer-independent route for PAFB. Our data exclude a direct interaction of PAF with GlcCer or other pathway specific intermediates. Instead, our findings allow us to hypothesize that sphingolipids regulate the membrane attraction and stabilization of PAF and PAFB binding, and suggest the necessity of other interaction molecules, whose presence or activity might be influenced by the GlcCer dependent lipid raft organization in the plasma membrane (Santos et al., 2018).
Author Contributions
FM, MAK, and JZ conceived, supervised and financed the study. FM, MAK, and KL designed the experiments and edited the manuscript. AH performed membrane lipid extractions, phenotypically characterized the mutants, conducted protein localization studies and analyzed the data. MAK and GO performed the lipidomic profiling of membrane extracts and analyzed the data. AH and LK determined the antimicrobial activity. WS performed scanning electron microscopy. NM and KL performed zeta-potential measurements and analyzed the data. All authors contributed to manuscript writing, and read and approved the submitted version.
Funding
This study was financially supported by the Austrian Science Fund (FWF) with the projects FWF P25894-B20 and FWF I1644-B20 to FM and a MUI Start grant of the Medical University of Innsbruck to MAK.
Conflict of Interest Statement
The authors declare that the research was conducted in the absence of any commercial or financial relationships that could be construed as a potential conflict of interest.
Acknowledgments
We thank Doris Bratschun-Khan for technical support.
Supplementary Material
The Supplementary Material for this article can be found online at: https://www.frontiersin.org/articles/10.3389/fmicb.2019.00605/full#supplementary-material
Abbreviations
AMP, antimicrobial protein; BP, BODIPY FL-EDA; CAT, conidial anastomosis tubes; CL, cardiolipin; ESI, electrospray ionization; GlcCer, glucosylceramide; H2O2, hydrogen peroxide; HPLC, high-performance liquid chromatography; (LC-) MS/MS, (liquid chromatography) tandem mass spectrometry; LCB, long chain base; LUV, large unilamellar vesicle; MIC, minimal inhibitory concentration; MIPC, mannosylinositol phosphorylceramides; MS, mass spectrometry; PAF, Penicillium chrysogenum antifungal protein; PAFB, Penicillium chrysogenum antifungal protein B; PC, phosphatidylcholines; PE, phosphatidylethanolamines; PI, phosphatidylinositols; PT, phosphinothricin; ROS, reactive oxygen species; t-BOOH, tert-butylhydroperoxide.
Footnotes
- ^ www.gimp.org
- ^ www.broadinstitute.org
- ^ www.candidagenome.org
- ^ https://blast.ncbi.nlm.nih.gov/Blast.cgi
- ^ http://www.uniprot.org/
References
Aerts, A. M., Francois, I. E., Bammens, L., Cammue, B. P., Smets, B., Winderickx, J., et al. (2006). Level of M(IP)2C sphingolipid affects plant defensin sensitivity, oxidative stress resistance and chronological life-span in yeast. FEBS Lett. 580, 1903–1907. doi: 10.1016/j.febslet.2006.02.061
Alvarez, F. J., Douglas, L. M., and Konopka, J. B. (2007). Sterol-rich plasma membrane domains in fungi. Eukaryot. Cell 6, 755–763. doi: 10.1128/EC.00008-07
Araujo-Palomares, C. L., Castro-Longoria, E., and Riquelme, M. (2007). Ontogeny of the Spitzenkörper in germlings of Neurospora crassa. Fungal Genet. Biol. 44, 492–503. doi: 10.1016/j.fgb.2006.10.004
Barth, B. M., Gustafson, S. J., Young, M. M., Fox, T. E., Shanmugavelandy, S. S., Kaiser, J. M., et al. (2010). Inhibition of NADPH oxidase by glucosylceramide confers chemoresistance. Cancer Biol. Ther. 10, 1126–1136. doi: 10.4161/cbt.10.11.13438
Binder, U., Chu, M., Read, N. D., and Marx, F. (2010). The antifungal activity of the Penicillium chrysogenum protein PAF disrupts calcium homeastasis in Neurospora crassa. Eukaryot. Cell 9, 1374–1382. doi: 10.1128/EC.00050-10
Bistis, G. N., Perkins, D. D., and Read, N. D. (2003). Different cell types in Neurospora crassa. Fungal Genet. Newsl. 50, 17–19. doi: 10.4148/1941-4765.1154
Case, M. E., Griffith, J., Dong, W., Tigner, I. L., Gaines, K., Jiang, J. C., et al. (2014). The aging biological clock in Neurospora crassa. Ecol. Evol. 4, 3494–3507. doi: 10.1002/ece3.1202
Cheng, J. J., Park, T. S., Fischl, A. S., and Ye, X. S. (2001). Cell cycle progression and cell polarity require sphingolipid biosynthesis in Aspergillus nidulans. Mol. Cell. Biol. 21, 6198–6209. doi: 10.1128/MCB.21.18.6198-6209.2001
Cheon, S. A., Bal, J., Song, Y., Hwang, H. M., Kim, A. R., Kang, W. K., et al. (2012). Distinct roles of two ceramide synthases, CaLag1p and CaLac1p, in the morphogenesis of Candida albicans. Mol. Microbiol. 83, 728–745. doi: 10.1111/j.1365-2958.2011.07961.x
Chu, M. (2013). Ca2+ Signalling and Homeostasis During Colony Initiation in Neurospora crassa. Ph.D. thesis, The University of Edinburgh, Edinburgh.
Colot, H. V., Park, G., Turner, G. E., Ringelberg, C., Crew, C. M., Litvinkova, L., et al. (2006). A high-throughput gene knockout procedure for Neurospora reveals functions for multiple transcription factors. Proc. Natl. Acad. Sci. U.S.A. 103, 10352–10357. doi: 10.1073/pnas.0601456103
Cools, T. L., Struyfs, C., Cammue, B. P., and Thevissen, K. (2017). Antifungal plant defensins: increased insight in their mode of action as a basis for their use to combat fungal infections. Fut. Microbiol. 12, 441–454. doi: 10.2217/fmb-2016-0181
Costantino, V., Mangoni, A., Teta, R., Kra-Oz, G., and Yarden, O. (2011). Neurosporaside, a tetraglycosylated sphingolipid from Neurospora crassa. J. Nat. Prod. 74, 554–558. doi: 10.1021/np1009493
de Medeiros, L. N., Domitrovic, T., de Andrade, P. C., Faria, J., Bergter, E. B., Weissmuller, G., et al. (2014). Psd1 binding affinity toward fungal membrane components as assessed by SPR: the role of glucosylceramide in fungal recognition and entry. Biopolymers 102, 456–464. doi: 10.1002/bip.22570
Del Poeta, M., Nimrichter, L., Rodrigues, M. L., and Luberto, C. (2014). Synthesis and biological properties of fungal glucosylceramide. PLoS Pathog. 10:e1003832. doi: 10.1371/journal.ppat.1003832
Domingues, M. M., Santiago, P. S., Castanho, M. A., and Santos, N. C. (2008). What can light scattering spectroscopy do for membrane-active peptide studiesΔ J. Pept. Sci. 14, 394–400. doi: 10.1002/psc.1007
Dunlap, J. C., Borkovich, K. A., Henn, M. R., Turner, G. E., Sachs, M. S., Glass, N. L., et al. (2007). Enabling a community to dissect an organism: overview of the Neurospora functional genomics project. Adv. Genet. 57, 49–96. doi: 10.1016/S0065-2660(06)57002-6
Epstein, S., and Riezman, H. (2013). Sphingolipid signaling in yeast: potential implications for understanding disease. Front. Biosci. 5:97–108. doi: 10.2741/E599
Fernandes, C. M., de Castro, P. A., Singh, A., Fonseca, F. L., Pereira, M. D., Vila, T. V. M., et al. (2016). Functional characterization of the Aspergillus nidulans glucosylceramide pathway reveals that LCB Δ8-desaturation and C9-methylation are relevant to filamentous growth, lipid raft localization and Psd1 defensin activity. Mol. Microbiol. 102, 488–505. doi: 10.1111/mmi.13474
Fizil, Á, Gáspári, Z., Barna, T., Marx, F., and Batta, G. (2015). “Invisible” conformers of an antifungal disulfide protein revealed by constrained cold and heat unfolding, CEST-NMR experiments, and molecular dynamics calculations. Chemistry 21, 5136–5144. doi: 10.1002/chem.201404879
Fontaine, T. (2017). Sphingolipids from the human fungal pathogen Aspergillus fumigatus. Biochimie 141, 9–15. doi: 10.1016/j.biochi.2017.06.012
Freire, J. M., Domingues, M. M., Matos, J., Melo, M. N., Veiga, A. S., Santos, N. C., et al. (2011). Using zeta-potential measurements to quantify peptide partition to lipid membranes. Eur. Biophys. J. 40, 481–487. doi: 10.1007/s00249-010-0661-4
Garrigues, S., Gandía, M., and Marcos, J. F. (2016). Occurrence and function of fungal antifungal proteins: a case study of the citrus postharvest pathogen Penicillium digitatum. Appl. Microbiol. Biotechnol. 100, 2243–2256. doi: 10.1007/s00253-015-7110-3
Guimarães, L. L., Toledo, M. S., Ferreira, F. A., Straus, A. H., and Takahashi, H. K. (2014). Structural diversity and biological significance of glycosphingolipids in pathogenic and opportunistic fungi. Front. Cell. Infect. Microbiol. 4:138. doi: 10.3389/fcimb.2014.00138
Huber, A., Hajdu, D., Bratschun-Khan, D., Gáspári, Z., Varbanov, M., Philippot, S., et al. (2018). New antimicrobial potential and structural properties of PAFB: a cationic, cysteine-rich protein from Penicillium chrysogenum Q176. Sci. Rep. 8:1751. doi: 10.1038/s41598-018-20002-2
Leipelt, M., Warnecke, D., Zähringer, U., Ott, C., Müller, F., Hube, B., et al. (2001). Glucosylceramide synthases, a gene family responsible for the biosynthesis of glucosphingolipids in animals, plants, and fungi. J. Biol. Chem. 276, 33621–33629. doi: 10.1074/jbc.M104952200
Li, S., Du, L., Yuen, G., and Harris, S. D. (2006). Distinct ceramide synthases regulate polarized growth in the filamentous fungus Aspergillus nidulans. Mol. Biol. Cell. 17, 1218–1227. doi: 10.1091/mbc.e05-06-0533
Liebisch, G., Vizcaíno, J. A., Köfeler, H., Trötzmüller, M., Griffiths, W. J., Schmitz, G., et al. (2013). Shorthand notation for lipid structures derived from mass spectrometry. J. Lipid Res. 54, 1523–1530. doi: 10.1194/jlr.M033506
Los, D. A., and Murata, N. (2004). Membrane fluidity and its roles in the perception of environmental signals. Biochim. Biophys. Acta 1666, 142–157. doi: 10.1016/j.bbamem.2004.08.002
Marx, F., Binder, U., Leiter,É, and Pócsi, I. (2008). The Penicillium chrysogenum antifungal protein PAF, a promising tool for the development of new antifungal therapies and fungal cell biology studies. Cell. Mol. Life Sci. 65, 445–454. doi: 10.1007/s00018-007-7364-8
Munshi, M. A., Gardin, J. M., Singh, A., Luberto, C., Rieger, R., Bouklas, T., et al. (2018). The role of ceramide synthases in the pathogenicity of Cryptococcus neoformans. Cell Rep. 22, 1392–1400. doi: 10.1016/j.celrep.2018.01.035
Nimrichter, L., and Rodrigues, M. L. (2011). Fungal glucosylceramides: from structural components to biologically active targets of new antimicrobials. Front. Microbiol. 2:212. doi: 10.3389/fmicb.2011.00212
Oberparleiter, C., Kaiserer, L., Haas, H., Ladurner, P., Andratsch, M., and Marx, F. (2003). Active internalization of the Penicillium chrysogenum antifungal protein PAF in sensitive Aspergilli. Antimicrob. Agents Chemother. 47, 3598–3601. doi: 10.1128/AAC.47.11.3598-3601.2003
Oemer, G., Lackner, K., Muigg, K., Krumschnabel, G., Watschinger, K., Sailer, S., et al. (2018). Molecular structural diversity of mitochondrial cardiolipins. Proc. Natl. Acad. Sci. U.S.A. 115, 4158–4163. doi: 10.1073/pnas.1719407115
Ohanian, J., and Ohanian, V. (2001). Sphingolipids in mammalian cell signalling. Cell. Mol. Life Sci. 58, 2053–2068. doi: 10.1007/PL00000836
Oura, T., and Kajiwara, S. (2008). Disruption of the sphingolipid Δ8-desaturase gene causes a delay in morphological changes in Candida albicans. Microbiology 154, 3795–3803. doi: 10.1099/mic.0.2008/018788-0
Oura, T., and Kajiwara, S. (2010). Candida albicans sphingolipid C9-methyltransferase is involved in hyphal elongation. Microbiology 156, 1234–1243. doi: 10.1099/mic.0.033985-0
Paege, N., Jung, S., Schäpe, P., Müller-Hagen, D., Ouedraogo, J. P., Heiderich, C., et al. (2016). A transcriptome meta-analysis proposes novel biological roles for the antifungal protein AnAFP in Aspergillus niger. PLoS One 11:e0165755. doi: 10.1371/journal.pone.0165755
Pan, J., Hu, C., and Yu, J. H. (2018). Lipid biosynthesis as an antifungal target. J. Fungi 4:E50. doi: 10.3390/jof4020050
Park, C., Bennion, B., Francois, I. E., Ferket, K. K., Cammue, B. P., Thevissen, K., et al. (2005). Neutral glycolipids of the filamentous fungus Neurospora crassa: altered expression in plant defensin-resistant mutants. J. Lipid Res. 46, 759–768. doi: 10.1194/jlr.M400457-JLR200
Park, G., Colot, H. V., Collopy, P. D., Krystofova, S., Crew, C., Ringelberg, C., et al. (2011). High-throughput production of gene replacement mutants in Neurospora crassa. Methods Mol. Biol. 722, 179–189. doi: 10.1007/978-1-61779-040-9_13
Plesofsky, N. S., Levery, S. B., Castle, S. A., and Brambl, R. (2008). Stress-induced cell death is mediated by ceramide synthesis in Neurospora crassa. Eukaryot. Cell 7, 2147–2159. doi: 10.1128/EC.00147-08
Pluskal, T., Castillo, S., Villar-Briones, A., and Orešič, M. (2010). MZmine 2: modular framework for processing, visualizing, and analyzing mass spectrometry-based molecular profile data. BMC Bioinformatics 11:395. doi: 10.1186/1471-2105-11-395
Portt, L., Norman, G., Clapp, C., Greenwood, M., and Greenwood, M. T. (2011). Anti-apoptosis and cell survival: a review. Biochim. Biophys. Acta 1813, 238–259. doi: 10.1016/j.bbamcr.2010.10.010
R Development Core Team (2015). R: A Language and Environment for Statistical Computing. Austria: R Foundation for Statistical Computing.
Ramamoorthy, V., Cahoon, E. B., Li, J., Thokala, M., Minto, R. E., and Shah, D. M. (2007). Glucosylceramide synthase is essential for alfalfa defensin-mediated growth inhibition but not for pathogenicity of Fusarium graminearum. Mol. Microbiol. 66, 771–786. doi: 10.1111/j.1365-2958.2007.05955.x
Ramamoorthy, V., Cahoon, E. B., Thokala, M., Kaur, J., Li, J., and Shah, D. M. (2009). Sphingolipid C-9 methyltransferases are important for growth and virulence but not for sensitivity to antifungal plant defensins in Fusarium graminearum. Eukaryot. Cell 8, 217–229. doi: 10.1128/EC.00255-08
Rhome, R., McQuiston, T., Kechichian, T., Bielawska, A., Hennig, M., Drago, M., et al. (2007). Biosynthesis and immunogenicity of glucosylceramide in Cryptococcus neoformans and other human pathogens. Eukaryot. Cell 6, 1715–1726. doi: 10.1128/EC.00208-07
Rittenour, W. R., Chen, M., Cahoon, E. B., and Harris, S. D. (2011). Control of glucosylceramide production and morphogenesis by the Bar1 ceramide synthase in Fusarium graminearum. PLoS One 6:e19385. doi: 10.1371/journal.pone.0019385
Rollin-Pinheiro, R., Liporagi-Lopes, L. C., de Meirelles, J. V., Souza, L. M., and Barreto-Bergter, E. (2014). Characterization of Scedosporium apiospermum glucosylceramides and their involvement in fungal development and macrophage functions. PLoS One 9:e98149. doi: 10.1371/journal.pone.0098149
Saito, K., Takakuwa, N., Ohnishi, M., and Oda, Y. (2006). Presence of glucosylceramide in yeast and its relation to alkali tolerance of yeast. Appl. Microbiol. Biotechnol. 71, 515–521. doi: 10.1007/s00253-005-0187-3
Santos, F. C., Lobo, G. M., Fernandes, A. S., Videira, A., and de Almeida, R. F. M. (2018). Changes in the biophysical properties of the cell membrane are involved in the response of Neurospora crassa to staurosporine. Front. Physiol. 9:1375. doi: 10.3389/fphys.2018.01375
Schlame, M., and Greenberg, M. L. (2017). Biosynthesis, remodeling and turnover of mitochondrial cardiolipin. Biochim. Biophys. Acta Mol. Cell. Biol. Lipids 1862, 3–7. doi: 10.1016/j.bbalip.2016.08.010
Schorling, S., Vallée, B., Barz, W. P., Riezman, H., and Oesterhelt, D. (2001). Lag1p and Lac1p are essential for the Acyl-CoA-dependent ceramide synthase reaction in Saccharomyces cerevisae. Mol. Biol. Cell 12, 3417–3427. doi: 10.1091/mbc.12.11.3417
Singh, A., MacKenzie, A., Girnun, G., and Del Poeta, M. (2017). Analysis of sphingolipids, sterols, and phospholipids in human pathogenic Cryptococcus strains. J. Lipid Res. 58, 2017–2036. doi: 10.1194/jlr.M078600
Singh, A., Wang, H., Silva, L. C., Na, C., Prieto, M., Futerman, A. H., et al. (2012). Methylation of glycosylated sphingolipid modulates membrane lipid topography and pathogenicity of Cryptococcus neoformans. Cell. Microbiol. 14, 500–516. doi: 10.1111/j.1462-5822.2011.01735.x
Sonderegger, C., Fizil,Á, Burtscher, L., Hajdu, D., Muñoz, A., Gáspári, Z., et al. (2017). D19S mutation of the cationic, cysteine-rich protein PAF: novel insights into its structural dynamics, thermal unfolding and antifungal function. PLoS One 12:e0169920. doi: 10.1371/journal.pone.0169920
Sonderegger, C., Galgóczy, L., Garrigues, S., Fizil,Á, Borics, A., Manzanares, P., et al. (2016). A Penicillium chrysogenum-based expression system for the production of small, cysteine-rich antifungal proteins for structural and functional analyses. Microb. Cell Fact. 15:192. doi: 10.1186/s12934-016-0586-4
Sonderegger, C., Váradi, G., Galgóczy, L., Kocsubé, S., Posch, W., Borics, A., et al. (2018). The evolutionary conserved γ-core motif influences the anti-Candida activity of the Penicillium chrysogenum antifungal protein PAF. Front. Microbiol. 9:1655. doi: 10.3389/fmicb.2018.01655
Springer, M. L., and Yanofsky, C. (1989). A morphological and genetic analysis of conidiophore development in Neurospora crassa. Genes Dev. 3, 559–571. doi: 10.1101/gad.3.4.559
Ternes, P., Sperling, P., Albrecht, S., Franke, S., Cregg, J. M., Warnecke, D., et al. (2006). Identification of fungal sphingolipid C9-methyltransferases by phylogenetic profiling. J. Biol. Chem. 281, 5582–5592. doi: 10.1074/jbc.M512864200
Thevissen, K., Warnecke, D. C., Francois, I. E., Leipelt, M., Heinz, E., Ott, C., et al. (2004). Defensins from insects and plants interact with fungal glucosylceramides. J. Biol. Chem. 279, 3900–3905. doi: 10.1074/jbc.M311165200
Tian, Y., Zhao, G. Y., Fang, W., Xu, Q., and Tan, R. X. (2015). Delta10(E)-Sphingolipid desaturase involved in fusaruside mycosynthesis and stress adaptation in Fusarium graminearum. Sci. Rep. 5:10486. doi: 10.1038/srep10486
Tóth, L., Váradi, G., Borics, A., Batta, G., Kele, Z., Vendrinszky, A., et al. (2018). Anti-Candidal activity and functional mapping of recombinant and synthetic Neosartorya fischeri antifungal protein 2 (NFAP2). Front. Microbiol. 9:393. doi: 10.3389/fmicb.2018.00393
Ueda, N. (2015). Ceramide-induced apoptosis in renal tubular cells: a role of mitochondria and sphingosine-1-phoshate. Int. J. Mol. Sci. 16, 5076–5124. doi: 10.3390/ijms16035076
Utesch, T., de Miguel Catalina, A., Schattenberg, C., Paege, N., Schmieder, P., Krause, E., et al. (2018). A computational modeling approach predicts interaction of the antifungal protein AFP from Aspergillus giganteus with fungal membranes via its γ-core motif. mSphere 3:e377-18. doi: 10.1128/mSphere.00377-18
Warnecke, D., and Heinz, E. (2003). Recently discovered functions of glucosylceramides in plants and fungi. Cell. Mol. Life Sci. 60, 919–941. doi: 10.1007/s00018-003-2243-4
Keywords: sphingolipids, glucosylceramide, lipidomics, Neurospora crassa, antimicrobial proteins, Penicillium chrysogenum
Citation: Huber A, Oemer G, Malanovic N, Lohner K, Kovács L, Salvenmoser W, Zschocke J, Keller MA and Marx F (2019) Membrane Sphingolipids Regulate the Fitness and Antifungal Protein Susceptibility of Neurospora crassa. Front. Microbiol. 10:605. doi: 10.3389/fmicb.2019.00605
Received: 30 November 2018; Accepted: 11 March 2019;
Published: 11 April 2019.
Edited by:
Sven Krappmann, University of Erlangen-Nuremberg, GermanyReviewed by:
Dilip Shah, Donald Danforth Plant Science Center, United StatesCarmit Ziv, Agricultural Research Organization (ARO), Israel
Copyright © 2019 Huber, Oemer, Malanovic, Lohner, Kovács, Salvenmoser, Zschocke, Keller and Marx. This is an open-access article distributed under the terms of the Creative Commons Attribution License (CC BY). The use, distribution or reproduction in other forums is permitted, provided the original author(s) and the copyright owner(s) are credited and that the original publication in this journal is cited, in accordance with accepted academic practice. No use, distribution or reproduction is permitted which does not comply with these terms.
*Correspondence: Markus A. Keller, bWFya3VzLmtlbGxlckBpLW1lZC5hYy5hdA== Florentine Marx, ZmxvcmVudGluZS5tYXJ4QGktbWVkLmFjLmF0