- 1Aix-Marseille Université, Université de Toulon, CNRS, IRD, MIO UM110, Marseille, France
- 2INRA, US 1426, GeT-PlaGe, Genotoul, Castanet-Tolosan, France
- 3Université de Paris, Institut de Physique du Globe de Paris, CNRS UMR 7154, Paris, France
Rock-hosted subseafloor habitats are very challenging for life, and current knowledge about microorganisms inhabiting such lithic environments is still limited. This study explored the cultivable microbial diversity in anaerobic enrichment cultures from cores recovered during the International Ocean Discovery Program (IODP) Expedition 357 from the Atlantis Massif (Mid-Atlantic Ridge, 30°N). 16S rRNA gene survey of enrichment cultures grown at 10–25°C and pH 8.5 showed that Firmicutes and Proteobacteria were generally dominant. However, cultivable microbial diversity significantly differed depending on incubation at atmospheric pressure (0.1 MPa), or hydrostatic pressures (HP) mimicking the in situ pressure conditions (8.2 or 14.0 MPa). An original, strictly anaerobic bacterium designated 70B-AT was isolated from core M0070C-3R1 (1150 meter below sea level; 3.5 m below seafloor) only from cultures performed at 14.0 MPa. This strain named Petrocella atlantisensis is a novel species of a new genus within the newly described family Vallitaleaceae (order Clostridiales, phylum Firmicutes). It is a mesophilic, moderately halotolerant and piezophilic chemoorganotroph, able to grow by fermentation of carbohydrates and proteinaceous compounds. Its 3.5 Mb genome contains numerous genes for ABC transporters of sugars and amino acids, and pathways for fermentation of mono- and di-saccharides and amino acids were identified. Genes encoding multimeric [FeFe] hydrogenases and a Rnf complex form the basis to explain hydrogen and energy production in strain 70B-AT. This study outlines the importance of using hydrostatic pressure in culture experiments for isolation and characterization of autochthonous piezophilic microorganisms from subseafloor rocks.
Introduction
The subseafloor biosphere remains largely unexplored, although estimated as a huge reservoir for prokaryotic life (Whitman et al., 1998; Orcutt et al., 2011; Kallmeyer et al., 2012). Exploration of the rock-hosted subseafloor biosphere is especially very challenging and carried out through costly ocean drilling programs, which still must face several technical difficulties, such as poor core recovery and microbial contamination. In addition, the very low microbial cell density, around 104 cells per gram of rock at North Pond (Jørgensen and Zhao, 2016) or even less in the Atlantis Massif (Früh-Green et al., 2018) (respectively 22 and 30°N along the Mid-Atlantic Ridge), the usually low growth rate, and the lack of knowledge on the physiology and metabolism of the prokaryotes living in these extreme environments, hamper the attempt at cultivating them. As a result, the subseafloor prokaryotic cultivability was estimated below 0.1% of total microscopically counted cells (D’Hondt et al., 2004). Moreover, the hydrostatic pressure is an important physical parameter of these deep-sea environments but was often neglected in previous subseafloor cultivation studies. To date, most of the enrichment tests performed on rocks recovered during ocean drilling programs were made at atmospheric pressure and failed to obtain microbial growth after first incubations or subcultures (Santelli et al., 2010; Hirayama et al., 2015). Both hydrostatic and lithostatic pressures in deep-sea and deep subseafloor environments (increasing by about 10 and 30 MPa km-1, respectively (Schrenk et al., 2010)) have an impact on microbial growth, metabolism and physiology, thus on the cultivability of microorganisms (Bartlett et al., 2007; Lauro and Bartlett, 2007; Parkes et al., 2009; Takai, 2011; Picard and Daniel, 2013). Moreover, the importance of high HP for deep-sea microorganisms cultivation is now well established (Tamburini et al., 2013). In this work, HP incubation systems were used to cultivate and study physiology of microorganisms inhabiting the underexplored oceanic crustal biosphere.
The Atlantis Massif, a prominent underwater oceanic core complex of nearly 4 000 m high, hosts the famous Lost City Hydrothermal Field (LCHF) (Kelley et al., 2005). It is composed of deep crustal (gabbro) and upper mantle rocks (peridotite) that have been exposed at the ocean floor as a result of tectonic plates drifting and large active faulting (Früh-Green et al., 2018). Serpentinization of ultramafic mantle rocks by deeply circulating seawater produces heat and generates alkaline fluids enriched in hydrogen (H2), methane (CH4), short-chain alkanes and small organic acids, representing possible carbon and energy sources to fuel life in the absence of light and contributing to global biogeochemical cycles (Levin et al., 2016). Such environmental conditions and ecosystems may have prevailed on early Earth or other planets (Martin et al., 2008). In this context, the main objective of the IODP Expedition 357 “Atlantis Massif Serpentinization and Life” was to explore the extent and activity of the subseafloor biosphere in a young ultramafic substratum (Früh-Green et al., 2016, Früh-Green et al., 2017d). During this expedition, series of boreholes were drilled across the Atlantis Massif at different water depths and distances of LCHF (Figure 1), in order to evaluate how subseafloor microbial communities may vary depending on the age of the lithosphere, its alteration and the hydrothermal activity.
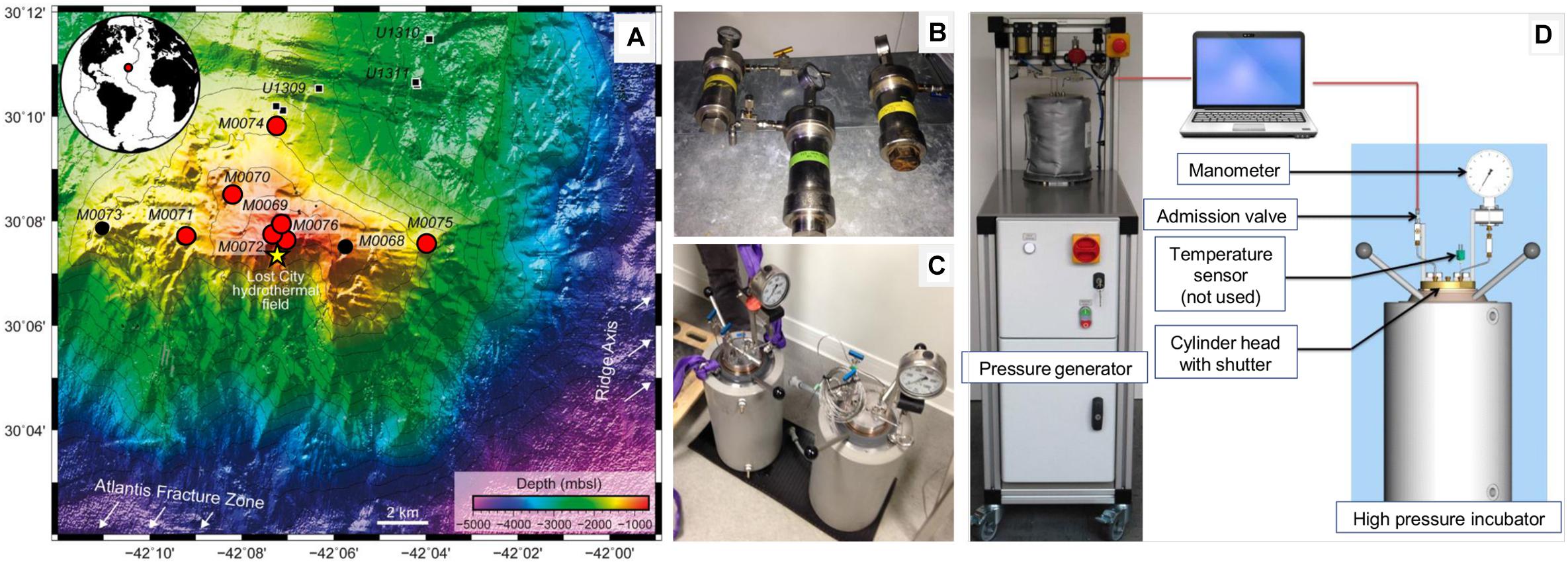
Figure 1. Bathymetric map of the Atlantis Massif southern wall showing the location of the sites drilled during the IODP Expedition 357 [A; modified from (Rouméjon et al., 2018)] and the high-pressure devices used in this study (B–D). On the map, the red circles indicate the sites considered in this study, the small black circles correspond to other holes drilled during the expedition, the small black squares show the Holes drilled during IODP Expeditions 304-305 (Site U1309) and the yellow star indicates the Lost City Hydrothermal Field. On the right, the photographs show high-pressure vessels (0.2 l in B or 5 l in C) used for storage and incubation, respectively. High-pressure vessels were connected to a piloted pressure generator (D) allowing linear increase for pressurization (or decrease for depressurization) of the hydrostatic pressure (0.5 MPa sec-1) by the programmable computer-driven system [see details in (Tamburini et al., 2009)].
The emblematic LCHF located on top of the Atlantis Massif, at 800 meters below sea level (mbsl), exhibits high carbonate chimneys discharging alkaline hydrothermal fluids at moderate temperature (∼ pH 11 and 90°C) and high levels of dissolved H2 (1-15 mM) and CH4 (1-2 mM) as the most obvious manifestation of underground serpentinization reactions (Schrenk et al., 2013). The unique microbial communities living inside the porous chimney structures are dominated by a single Methanosarcinales phylotype (Archaea), with Proteobacteria and Firmicutes (Bacteria) (Schrenk et al., 2013). This peculiar ecosystem is assumed to picture an “open window on deep serpentinizing hydrothermal system” (Lang et al., 2018). However, due to the lack of core samples from the LCHF basement, direct experimental evidences supporting this assumption are missing. Only one study on the microbiology of the Atlantis Massif (IODP Expeditions 304-305, Hole U1309D) reports that the gabbroic layers host a low diversity of proteobacterial lineages (Alpha-, Beta- and Gammaproteobacteria) hypothetically degrading hydrocarbons and fixing carbon and nitrogen with the potential for anaerobic respiration of nitrate, sulfate and metals (Mason et al., 2010). To date no cultivated microorganism has been reported from these ecosystems to attest the occurrence of these metabolisms, and most of the deep-subsurface microorganisms detected so far were refractory to cultivation.
The primary goal of this study was to explore the diversity of cultivable prokaryotic communities of the Atlantis Massif subseafloor from a unique collection of rock cores composed in various proportions of carbonate, basalt, serpentinized peridotite and gabbro (Figure 1 and Supplementary Figure 1). To increase our chance of success, we used a high-pressure incubation system (Figure 1) to mimic in situ HP at the sampling sites and the various anaerobic metabolisms likely to be present were targeted based on literature surveys (Mason et al., 2010; Schrenk et al., 2013). We used next-generation sequencing (NGS) of 16S rRNA gene amplicons to explore the cultivable microbial diversity of Atlantis Massif core samples incubated at atmospheric or in situ HP. We report novel anaerobes isolated from rock-hosted subseafloor ecosystems and describe the phenotypic and genomic features of strain 70B-AT, the first isolate from the Atlantis Massif subseafloor obtained from high-pressure cultures. Finally, we propose it to represent a novel species of a new bacterial genus within the newly described family Vallitaleaceae (order Clostridiales, phylum Firmicutes).
Materials and Methods
Rock Sample Collection
A unique set of rock samples was recovered during IODP Expedition 357 “Atlantis Massif Serpentinization and Life,” which took place from October to December 2015 onboard the RRS James Cook (Früh-Green et al., 2016). Nine core samples were investigated in this study and were collected using two seabed rock drills (i.e., the British Geological Survey RockDrill2 (RD2) and the Meeresboden-Bohrgerät 70 (Mebo) from the Center for Marine Environmental Sciences, MARUM, University of Bremen, Germany). Drilling sites were located at varying distances away from LCHF and the ridge axis: one site drilled on the eastern part of the southern wall of the Atlantis Massif (Site M0075), three sites located in the central section <1 km north of Lost City (Sites M0069, M0072, and M0076), two sites located north toward the central dome of the massif (Sites M0070 and M0074), and one site on the western end (Site M0071) (Table 1 and Figure 1). Drilled sites were located at a water depth ranging from 820 to 1 568 mbsl (Table 1). Sites M0070 and M0074 targeted the mafic, plutonic domain drilled during IODP Expeditions 304-305 at Site U1309 while the other sites targeted the serpentinite basement of the Atlantis Massif. Lithologies associated with each core sample are described in Table 1 (photographs are shown in Supplementary Figure 1). During drilling, potential contamination was quantified using perfluorocarbon tracer and sampling was achieved under strict contamination controls onboard and offshore (Orcutt et al., 2017). Only samples with no detectable contamination were used for this study (Früh-Green et al., 2017c). Primary procedures, as well as core handling and processing used during the offshore and onshore phases of IODP Expedition 357 are detailed in (Früh-Green et al., 2017c). Details on studied sites according to their location on the Atlantis Massif are provided in (Früh-Green et al., 2017a,b,e,f).
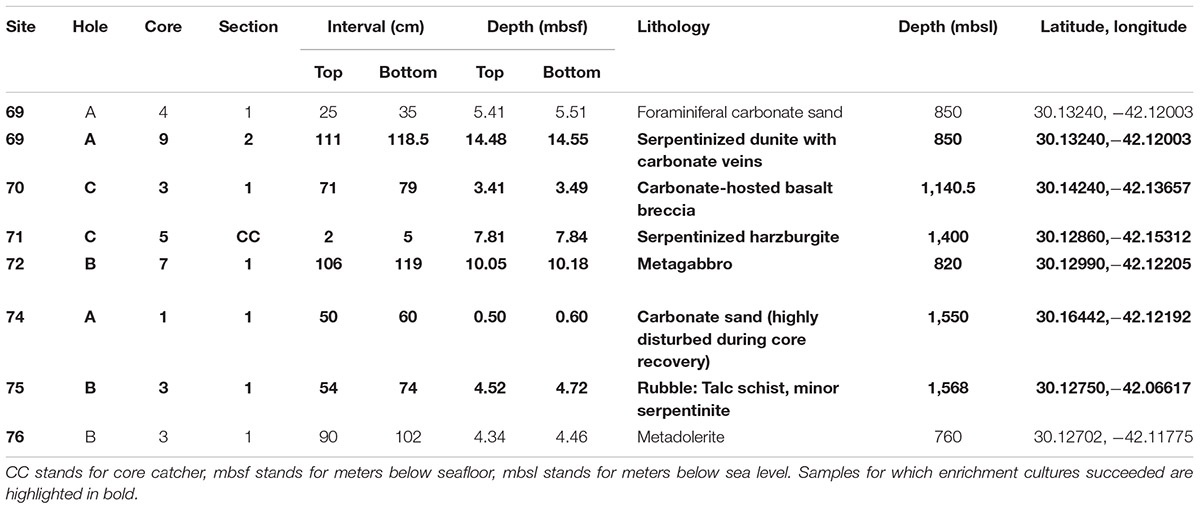
Table 1. IODP expedition 357 samples used in this study (see photographs in Supplementary Figure 1).
Processing and Storage of Core Samples Used in This Study
Onboard, the processing of the core sample was done at 10°C. The exterior of the core sample was flamed to eliminate potential contaminants, then sliced and smashed into small pieces using a flamed chisel. These subsamples were crushed using a sterile stainless steel mortar and pestle, under sterile and anaerobic conditions. Approximately 5 cm3 of the powdered rock sample were transferred into Hungate screw tubes, fully filled (∼17 ml) with sterile artificial seawater (40 g l-1 solution of Sea Salts, Sigma Aldrich) and stored in high pressure vessels (Top Industrie SA, France) at in situ HP conditions (from 8 to 17 MPa, depending on the water depths of the sites) by means of manual hydraulic pump and stored at 4°C until processing in the shore-based laboratory (Figure 2). Extra powder samples were transferred in 100 ml Schott bottles under a N2 gas atmosphere, then stored at 4°C and atmospheric pressure (0.1 MPa) until processing in the onboard and shore-based laboratories (Figure 2). Details on HP devices are provided in (Tamburini, 2006; Tamburini et al., 2009, 2013).
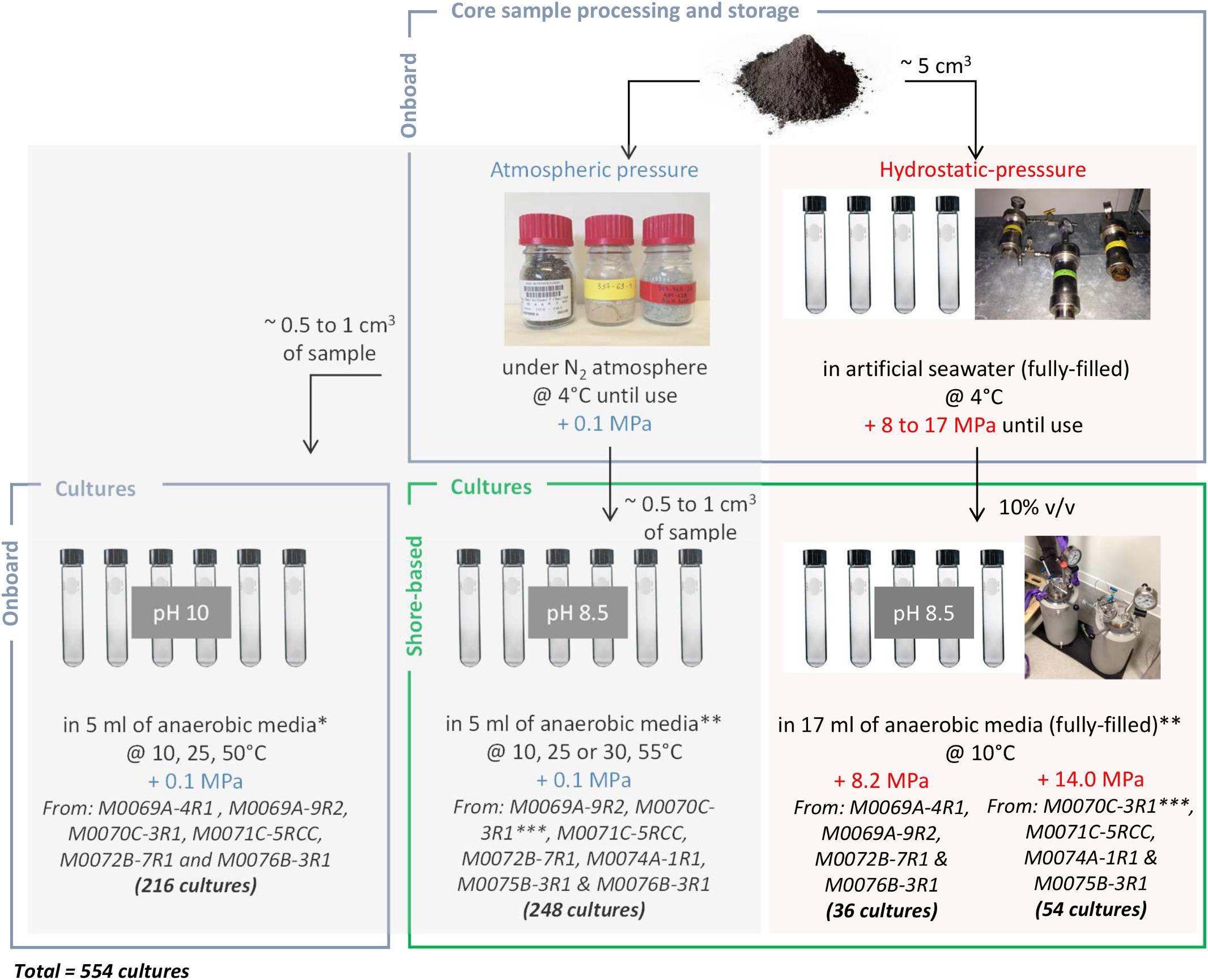
Figure 2. Schematic view of the sampling processing and the cultures initiated at 0.1, 8.2 and 14.0 MPa, onboard and onshore. A total of 554 cultures was performed, in duplicate (except if too small rock quantity); controls were uninoculated tubes. ∗Fermentation (F); acetotrophic (MAcH2), hydrogenotrophic (MH2), and methylotrophic (MMet) methanogenesis; acetotrophic (SAc) and hydrogenotrophic (SH2) sulfate reduction. ∗∗Fermentation (F1), oligotrophy (F2), sulfate reduction from acetate or lactate (SRB1) and formate or H2/CO2 (SRB2), methanogenesis by acetoclasty or methylotrophy (MET1) and hydrogenotrophy (MET2), and iron reduction (FE) (Supplementary Table 2). ∗∗∗Black basaltic and white carbonated parts dissociated.
Culture Media Preparation and Enrichment Culture Conditions
The composition of the culture media is detailed in the Supplementary Table 1. After dissolution of its components, the culture medium was boiled under a stream of O2-free N2 gas, cooled to room temperature, dispensed into Hungate tubes, flushed under N2/CO2 (80/20, v/v) and then sterilized by autoclaving. The media were completed by addition of sterile stock solutions of Na2CO3 8% (w/v), MgCl26H2O 50 g l-1, Balch oligo elements solution, and Na2S9H2O 3% (w/v) injected through the tube septum (volumes are indicated in Supplementary Table 1).
Onboard, culture media were inoculated in duplicates in Hungate tubes at 0.1 MPa headspace of N2/CO2 or H2/CO2 (80/20, v/v) using approximately 0.5–1 cm3 of rock as inoculum in 5 ml of anaerobic media (with the following abbreviations: F, for fermenters; MAcH2, MH2, MMet, for acetotrophic, hydrogenotrophic and methylotrophic methanogens; SAc and SH2, for acetotrophic and hydrogenotrophic sulfate reducers; Supplementary Table 1). Culture media pH were adjusted to 10 with 1M KOH as detailed in (Früh-Green et al., 2017c) and incubations were done at three temperatures: 10°C (the average temperature of sea bottom waters), 25 and 50°C (as we hypothesized a temperature gradient between hot hydrothermal fluid and cold subseafloor) A total of 216 tubes were inoculated with core samples M0069A-4R1, M0069A-9R2, M0070C-3R1, M0071C-5RCC, M0072B-7R1 and M0076B-3R1 (6 samples × 2 replicates × 3 temperatures × 6 media) (Figure 2).
In the shore-based laboratory (MIO), series of culture media (composition in Supplementary Table 1) were used to target the following metabolisms: fermentation (F1), oligotrophy (F2), sulfate reduction from acetate or lactate (SRB1) and formate or H2/CO2 (SRB2), methanogenesis by acetoclasty or methylotrophy (MET1) and hydrogenotrophy (MET2), and iron reduction (FE). The ISO 10390:2005 protocol, dedicated to measure soil pH, was used here for rock samples: powdered rocks were suspended in KCl 1M (1/5, v/v), and pH of the suspension was measured. The mean pH was 8.5 (below a pH of 10, which was the first assumption for onboard enrichment cultures) thus the pH of the shore-based culture media was accordingly adjusted to pH 8.5. Hungate tubes for incubation at 0.1 MPa contained 5 ml of medium, thus about 12 ml of gas headspace (N2/CO2 or H2/CO2, 80/20, v/v), while those used in HP incubation were filled with 17 ml of medium (no headspace) (Khelaifia et al., 2011). Cultures were run in duplicates, except for samples M0069A-4R1 and M0074A-1R1 due to the low amount of available material. 248 enrichment cultures at 0.1 MPa were incubated at 10°C, 25°C (or 30°C) and 55°C from core samples M0069A-9R2, M0070C-3R1 (black basaltic and white carbonated parts dissociated), M0071C-5RCC, M0072B-7R1, M0074A-1R1, M0075B-3R1, and M0076B-3R1 (8 samples × 2 replicates × 3 temperatures × 6 media -all except SRB2-, MET2 without replicate and only at 30°C). 36 enrichment cultures were launched at 8.2 MPa and 10°C from core samples M0069A-4R1, M0069A-9R2, M0072B-7R1 and M0076B-3R1 (the last two in duplicate) using the 6 onshore media (all except F2), while 54 enrichment cultures were launched at 14.0 MPa and 10°C from core samples M0070C-3R1 (black basaltic and white carbonated parts dissociated), M0071C-5RCC, M0074A-1R1 and M0075B-3R1 using the 6 onshore media (all except F2).
All cultures carried out under HP were incubated at 10°C (corresponding to the average temperature of sea bottom waters) (Figure 2). Shortly, culture tubes were placed for incubations in two HP 5 l inox incubators which were totally filled with distilled water, the septum transmitting the hydrostatic pressure inside the culture, before pressure was increased. A piloted pressure generator (see details in (Tamburini et al., 2009)) was used for accurate pressurization and depressurization (ramping rate = 3 MPa/min) (Figure 1D).
For each culture condition, a tube of medium not inoculated was incubated in parallel as negative control, to verify the absence of microbial contamination. The experimental procedure is summarized in Figure 2.
After 1 week incubation onboard and 1 month incubation (and more) in shore-lab experiments, the enrichment cultures were checked for growth by observation of cells stained with SYBR® Green I (Molecular Probes) 1X using an epifluorescence microscope Nikon ECLIPSE E600. The headspace gas, in the cultures that have one, were analyzed by gas chromatography (Shimadzu GC 8A instrument) to evaluate H2 production or consumption and CH4 production, as described in (Mei et al., 2014).
Strain Isolation and Analyses
Positive cultures were serially diluted at 1/10 of the volume up to 10-9 dilution in Hungate screw tubes containing 4.5 ml of anaerobic growth media plus agar (1.6% final) maintained liquid at 55°C in a water-bath. Inoculated tubes were then quickly cooled down while spinning using a tube spinner according to the roll-tube technique (Hungate, 1969). Colonies were picked up in an anaerobic gloves-box and used as an inoculum for the next series of dilution. This procedure was repeated three times before the strain culture was deemed pure. Morphological features of the isolates were examined using a microscope Nikon ECLIPSE E600 under phase contrast condition. Procedure for analyzing cell structures, the G+C content of genomic DNA, as well as cellular fatty acids and polar lipids composition, are given in Supplementary Methods.
Determination of Optimal Growth Conditions of Strain 70B-AT
Experiments to determine growth ranges of strain 70B-AT were performed in duplicates or in triplicates at 0.1 MPa using Hungate tubes containing 5 ml of F1 medium (for pH, temperature and salinity) or at 14.0 MPa using BM medium (i.e., mineral base of the F1 medium) supplemented with 0.2 g l-1 yeast extract and 20 mM glucose as sole carbon source. Potential substrates were tested in duplicates by addition into the BM medium (containing 0.5 g l-1 yeast extract). Details on experimental procedures are given in Supplementary Methods. Growth was determined by measuring optical density (OD) at 600 nm (Cary 50 UV-Vis spectrophotometer; Varian). End-products of metabolism were measured by high-performance liquid chromatography (HPLC) and gas phase chromatography after 2-weeks of incubation at 30°C (instruments details in (Mei et al., 2014)). Graphical and statistical analyzes of growth curves (OD versus time) of strain 70B-AT were performed with XLSTAT 2018.6 (Microsoft Excel add-in program). After checking data normality, the parametric Student’s t-test or non-parametric Mann-Whitney test were respectively used to estimate if the differences observed in maximum OD values (ODmax) or maximal growth rates between the cultures performed at 0.1 and 14.0 MPa were significant.
DNA Extraction and 16S rRNA Gene Sequencing
Fast DNA® kit for soil and FastDNA® kit were used respectively to extract DNA from enrichment cultures and isolated strain, according to the manufacturer recommendations (MP Biomedical). DNA from enrichment cultures was used for PCR amplification targeting the V3-V4 region of the 16S rRNA gene using the primers Pro341F and Pro805R (Takahashi et al., 2014); amplicons were sequenced by Illumina MiSeq at MrDNA (TX, United States). 16S rRNA genes from isolated strains were amplified using the primers 27F and 1492R (Lane, 1991) and the PCR products were sent to GATC Biotech AG (Germany) for Sanger sequencing.
Whole Genome Sequencing of Strain 70B-AT
For sequencing the genome of strain 70B-AT, high molecular weight DNA was extracted from a 500 mL culture cell pellet by using a phenol-chloroform-isoamyl alcohol-based method as previously described (Marteinsson et al., 1995). Genomic sequencing was performed at the GenoToul platform (Toulouse, France) by combining long reads technology of Oxford Nanopore to ease assembling (GridION) and high coverage provided by short paired-end reads obtained with Illumina (MiSeq) technology. The methodological details on sequencing, read processing and de novo assembly of genome are provided in Supplementary Methods. The genome was annotated using the MicroScope plateform (Vallenet et al., 2017).
Bacterial Community Composition and Phylogenetic Analysis
Raw reads were first merged with PEAR v0.9.6 and trimmed when the quality scores were less than 20 for two consecutive bases. Only sequences with a length greater than 35 bp were retained for further analyses. Chimera sequences were identified and removed using vsearch (v2.3.4). The clustering to Operational Taxonomic Units (OTUs) (>97%) and their quality filtration were carried out using QIIME 1.9.1 (Caporaso et al., 2010) as described in (Frouin et al., 2018). Taxonomic assignment of the filtered OTUs was performed using the Uclust method against the SILVA database release for QIIME (v.123). Heatmap.3 package in R was used to build the heatmap (R Core Team, 2017).
Phylogenetic analyses and trees based on 16S rRNA gene sequences were conducted in MEGA7 (Kumar et al., 2016) using MUSCLE program (Edgar, 2004) for multiple alignment, as described by (Postec et al., 2015).
Data Deposition
MiSeq V3-V4 amplicons raw data were submitted to the Sequence Read Archive under SRA Study SRP148750, as part of the BioProject PRJNA448822. The GenBank/EMBL/DDBJ accession number for the 16S rRNA gene sequence (1490 bp) of strain 70B-AT is KY969626. The genome sequence was deposited to the European Nucleotide Archive under study accession number PRJEB285851, and Genbank accession number LR130778. The type strain 70B-AT was deposited in DSMZ and JCM culture collections under accession numbers DSM 105309T = JCM 32078T respectively.
Results
Summary of Enrichment Cultures
A total of 554 anaerobic enrichment cultures were performed from IODP 357 core samples and monitored for about 1 year (Figure 2). Onboard, the first positive enrichment cultures were obtained after 2-week incubation from M0069A-9R2 using MAcH2 media at 25°C, 0.1 MPa and pH 10 (in the range of low temperature serpentinizing fluids pH values). No microbial growth was observed in other alkaliphilic cultures initiated onboard after 1-year regular monitoring, whatever the temperature and medium conditions tested. Back to the home lab, the first enrichment cultures at 0.1 MPa were obtained after 1-month incubation from M0070C-3R1 at 25°C in F1 and FE media, but only scarce cells were detected by epifluorescence microscopy from M0069A-9R2, M0071C-5RCC, M0072B-7R1 and M0074A-1R1 cultures after 2 months under similar conditions. Dividing cells were observed at 10°C in the same media than at 25°C but after longer incubation (≥2 months). No microbial growth was detected in any of the enrichment cultures at 55°C, neither from M0069A-4R1 (carbonate sand) or M0076B-3R1 (serpentinite) samples, in any conditions. At 14.0 MPa, active cells were observed from M0070C-3R1 sample after 1 month with all the tested media (more cells with F1 one), while scarce active cells were observed with M0075B-3R1 in F1 and SRB1 media. At 8.2 MPa, a slight growth was detected after 1-year incubation from M0072B-7R1 (using F1 and SRB1 media).
Phyla Diversity in Enrichment Cultures
Microbial diversity was analyzed by MiSeq 16S rRNA gene sequencing for a selection of 51 enrichment cultures, listed and described in Supplementary Table 2. A total of 462,162 sequences clustered in 415 OTUs was obtained.
Firmicutes and Proteobacteria were largely dominant in most conditions tested (Figure 3). Actinobacteria, Bacteroidetes, Cyanobacteria and Deinococcus-Thermus were also abundant in M0069A-9R2 cultures (MAcH2 and FE media, 10 and 25°C, 0.1 MPa) while Acidobacteria, Chloroflexi, Saccharibacteria and Verrucomicrobia were abundant in a M0071C-5RCC culture (FE medium, 25°C, 0.1 MPa). Enrichment cultures from M0072B-7R1, M0074A-1R1 and M0075B-3R1 contained only Firmicutes and Proteobacteria, except for sample M0072B-7R1 in FE medium at 25°C and 0.1 MPa culture containing also Acidobacteria, Actinobacteria and Cyanobacteria. In M0070C-3R1 cultures at 14.0 MPa, Firmicutes accounted for 74% to 99% of the microbial community (with the second most abundant phylum being Proteobacteria), while a more diverse community exhibiting Bacteroidetes, Actinobacteria, Tennericutes and Cyanobacteria members was detected at 0.1 MPa.
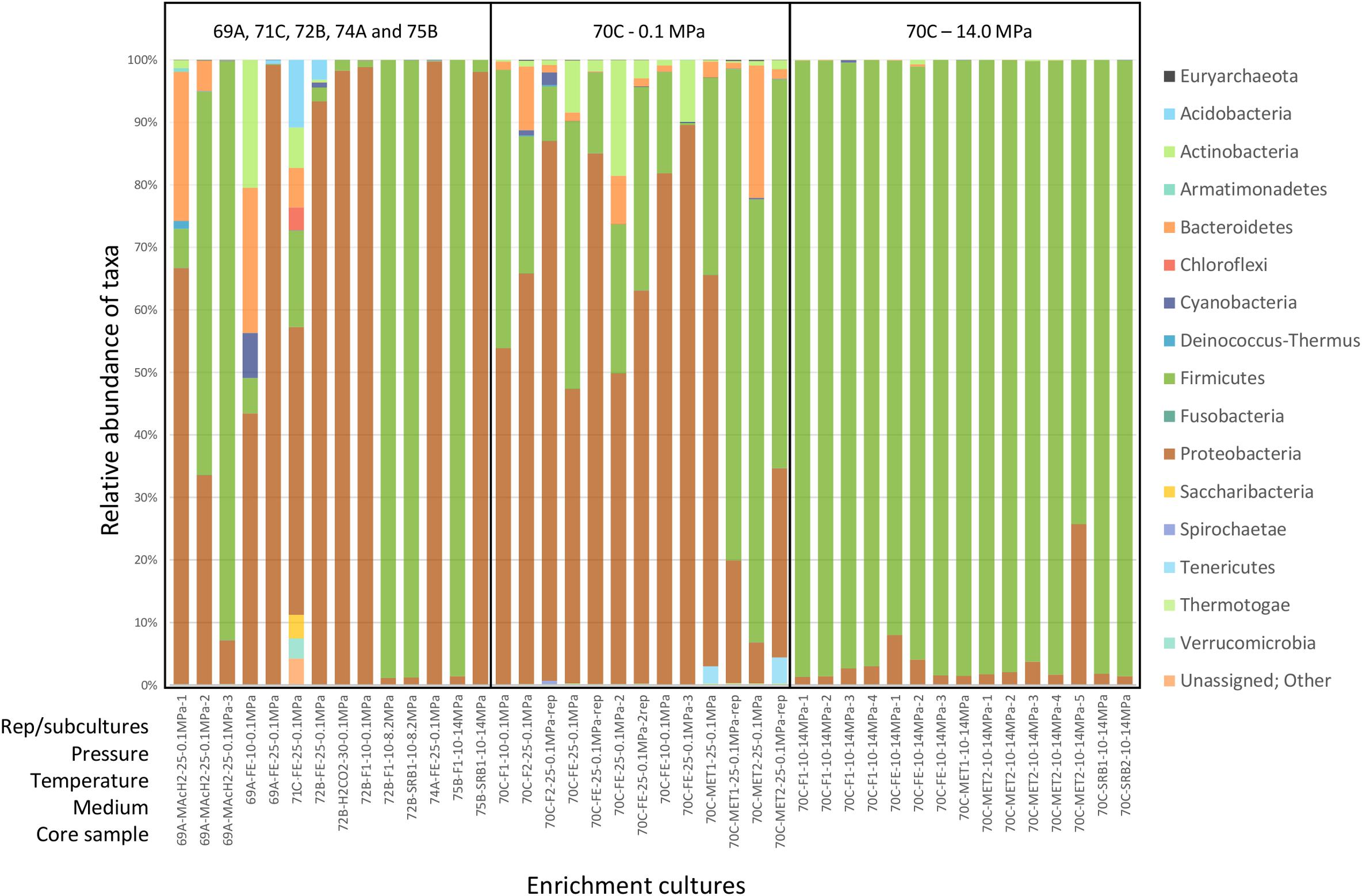
Figure 3. Phylum-level microbial diversity in enrichment cultures from Atlantis Massif core samples. Analysis based on 16S rRNA gene sequences (V3–V4 region). 69A, 70C, 71C, 72B, 74A and 75B respectively stand for M0069A-9R2, M0070C-3R1, M0071C-5RCC, M0072B-7R1, M0074A-1R1 and M0075B-3R1 core samples. The sample names used in this study and their description in SRA (study SRP148750) are shown in Supplementary Table 2. Rep stands for replicate. Pressure is expressed in MPa, temperature in °C. F, SRB, FE, MET & MAcH2 correspond to culture media targeting fermenters, sulfate reducers, iron reducers and methanogens, respectively (Supplementary Table 1).
Archaea represented less than 0.2% of the total reads and were not detected in all cultures. Methanosarcinales affiliated to ANaerobic MEthane oxidizing Archaea (ANME-3) were found in M0069A-9R2, M0070C-3R1 and M0074A-1R1 [and already recognized in Lost City Hydrothermal chimneys (Brazelton et al., 2006)], together with rare OTUs assigned to the hyperthermophilic sulfur-reducing genus Thermococcus and the thermoacidophilic genus Thermoplasma (Table 2).
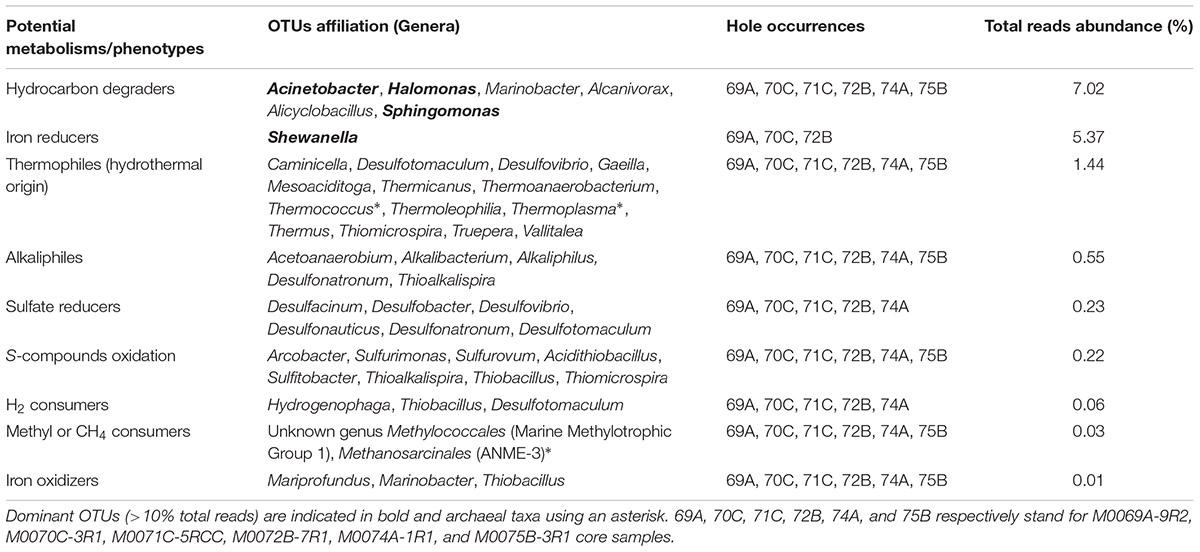
Table 2. Relevant potential metabolisms/phenotypes of operational taxonomic units (OTUs) retrieved from anaerobic enrichment cultures from Atlantis Massif core samples (based on 16S rRNA gene analysis).
Dominant Cultivated Microorganisms: Abundant OTUs
Only the most abundant OTUs (>10% of total reads), representing the most likely cultivated members, are presented in Figure 4. Enrichment cultures from M0069A-9R2 were dominated at 25°C and 0.1 MPa by Acinetobacter (Gammaproteobacteria), Bacillus (Bacilli), Sphingomonas (Alphaproteobacteria) and Sphingobacteriia (Bacteroidetes). Interestingly, an effect of the HP was observed for M0072B-7R1 cultures since Tissierella (Clostridia) largely dominated at 8.2 MPa while Thalassospira (Alphaproteobacteria; using H2/CO2), Shewanella and Acinetobacter (Gammaproteobacteria) were dominant at 0.1 MPa. Besides, Marinilactibacillus and Halomonas, also found in other deep ecosystems and including some piezophilic representatives (Kaye et al., 2004; Toffin et al., 2005), were cultivated at 14.0 MPa from M0075B-3R1 (Figure 4).
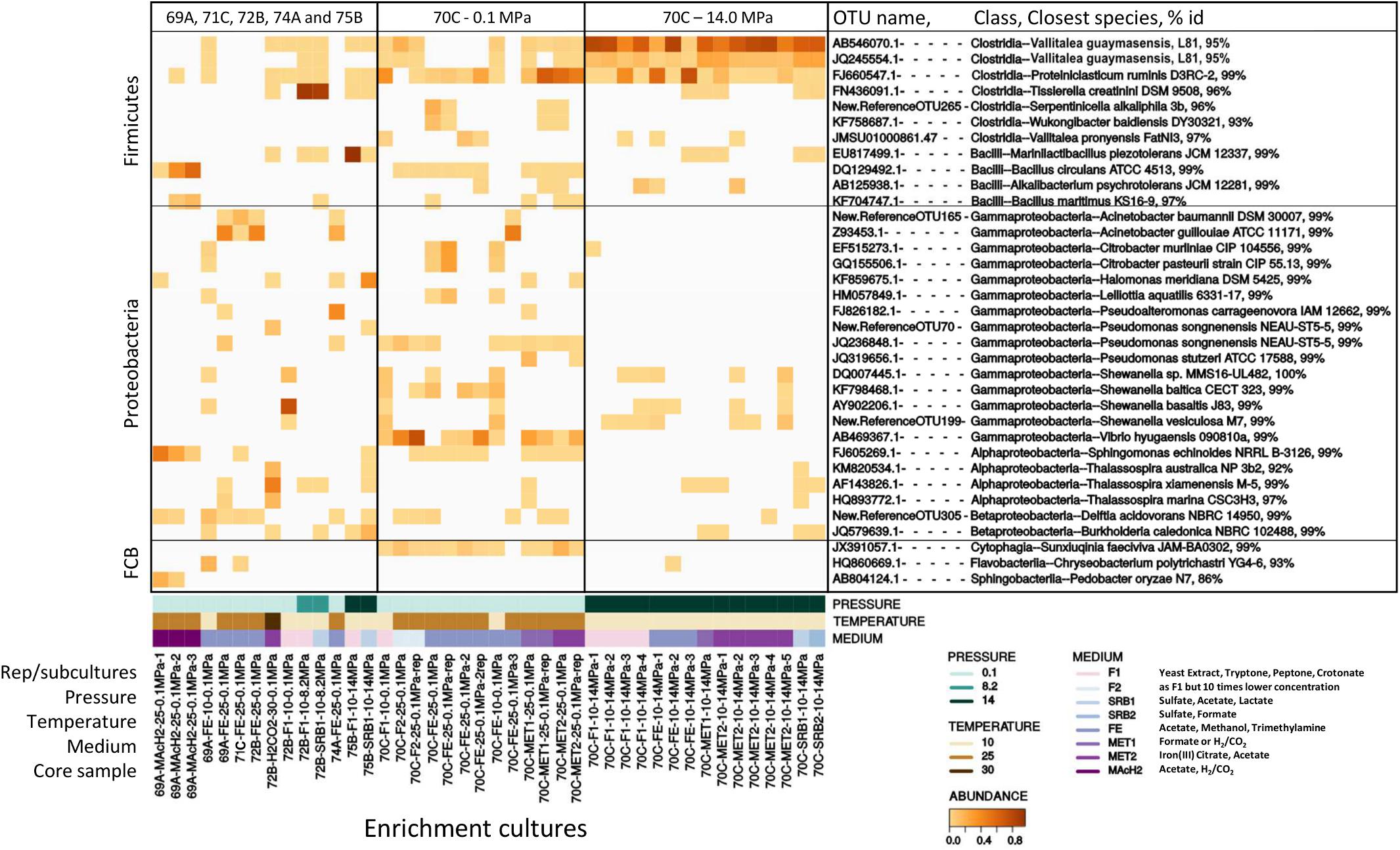
Figure 4. Heatmap showing the distribution of dominant OTUs (>10% total reads) in anaerobic enrichment cultures from Atlantis Massif subseafloor rocks. OTU name, its class affiliation, name of its closest species and 16S rRNA gene percentage identity (% id) with this closest homolog are indicated on the right. 69A, 70C, 71C, 72B, 74A and 75B respectively stand for M0069A-9R2, M0070C-3R1, M0071C-5RCC, M0072B-7R1, M0074A-1R1 and M0075B-3R1 core samples. Rep stands for replicate. Pressure is expressed in MPa, temperature in °C. F, SRB, FE, MET & MAcH2 correspond to culture media targeting fermenters, sulfate reducers, iron reducers and methanogens, respectively (Supplementary Table 1). FCB stands for Fibrobacteres/Bacteroidetes/Chlorobi group.
Most of the successful enrichment cultures were obtained from carbonate-hosted basalt breccia samples of M0070C-3R1 core (1150 mbsl, north of the Atlantis Massif). Marked differences in the microbial diversity of the cultures were observed depending on the incubation pressure. At 0.1 MPa, the dominant genera in M0070C-3R1 cultures were Proteiniclasticum (Clostridia), Vibrio, Shewanella, Acinetobacter, Citrobacter and Pseudomonas (belonging to Gammaproteobacteria) (Figure 4). Sunxiuqinia (Cytophagia) and Tessaracoccus (Actinobacteria; not shown, <10%), less abundant in the cultures, have been previously reported in deep biosphere environments, as well as Shewanella (Toffin et al., 2004; Finster et al., 2009; Picard and Daniel, 2013; Takai et al., 2013). In contrast, cultures from M0070C-3R1 incubated at 14.0 MPa were dominated mainly by 2 OTUs of an unknown genus, related to Vallitalea genus (Clostridia), representing up to 98% of the reads in a culture (Figure 4). Proteiniclasticum, Alkalibacterium (Firmicutes) with Shewanella were also found as dominant OTUs in M0070C-3R1 cultures at 14.0 MPa (also found at 0.1 MPa).
Most of the identified microorganisms seem to develop on heterotrophy in our enrichment cultures. Additionally, potential iron reducers (e.g., Shewanella) were identified as dominant, while potential H2-consumers (Hydrogenophaga, Thiobacillus) and/or sulfate-reducers (Desulfotomaculum) were only observed as rare OTUs (Table 2).
Strain Isolation
In this study, a total of fourteen strains were isolated and affiliated to Gammaproteobacteria, Actinobacteria, Bacillales and Clostridiales (Supplementary Figure 2).
Three very similar isolates (100% 16S rRNA gene identity) affiliated to Tissierella genus (97% 16S rRNA gene identity with T. creatinophila, Clostridiales) were obtained from M0072B-7R1 cultures at 8.2 MPa using media SRB1 and F1 after 1-year incubation. At 25°C and 0.1 MPa, Sphingobacteriia initially enriched from M0069A-9R2 (in MAcH2 medium) and accounting for 48% of the microbial community, was outcompeted by Bacillus after only two subcultures. A strain affiliated to Bacillus circulans (99% 16S rRNA gene identity) was finally isolated with LB-Tris medium from M0069A-9R2 (Supplementary Figure 2B).
The rest of the strains (10/14) were isolated from M0070C-3R1 subcultures. Among them, the strain 70-CrotoS2-10-2 isolated at 25°C and 0.1 MPa using F1 medium was closely affiliated to Tessaracoccus oleiagri and T. profundii (99% 16S rRNA gene identity). A positive enrichment culture at 25°C and 0.1 MPa with FE medium was also used as an inoculum for serial dilution on roll-tubes from which dark brown colonies, likely indicating iron reduction, formed after 4 days up to the dilution 10-7. Four of these colonies were assigned to Citrobacter freundii (99% 16S rRNA gene identity) in the Enterobacteriaceae family (class Gammaproteobacteria). One of them, strain 70O-Fer25-B, is a motile coccobacillus shown in Supplementary Figure 2A.
Within Firmicutes, two strains closely affiliated to Proteiniclasticum ruminis (99% identity; type strain from yak rumen) were isolated at 10°C and 0.1 MPa in F1 medium. Finally, six isolates very closely related (sharing >99% 16S rRNA gene identity), were obtained from M0070C-3R1 cultures incubated at 14.0 MPa and 10°C in MET1, MET2 and F1 media. First colonies were obtained in roll-tubes after about 1 month incubation at 10°C and further purified by two additional series end-point dilution in roll-tubes before deemed pure. They were related to Vallitalea genus at <93% 16S rRNA gene identity and therefore represente new cultivated members of Clostridiales without close described species. One of them, called strain 70B-AT, was chosen for complete characterization.
Characterization of Strain 70B-AT
The 16S rRNA gene sequence of strain 70B-AT was most closely related to members of Vallitalea and Natranaerovirga genera, with V. guaymasensis (Lakhal et al., 2013) and N. pectinivora (Sorokin et al., 2012) sharing respectively 92.6 and 90.2% 16S rRNA gene sequence identity with strain 70B-AT (Figure 5A). Strain 70B-AT genomic DNA contained 37.7 mol % of G+C.
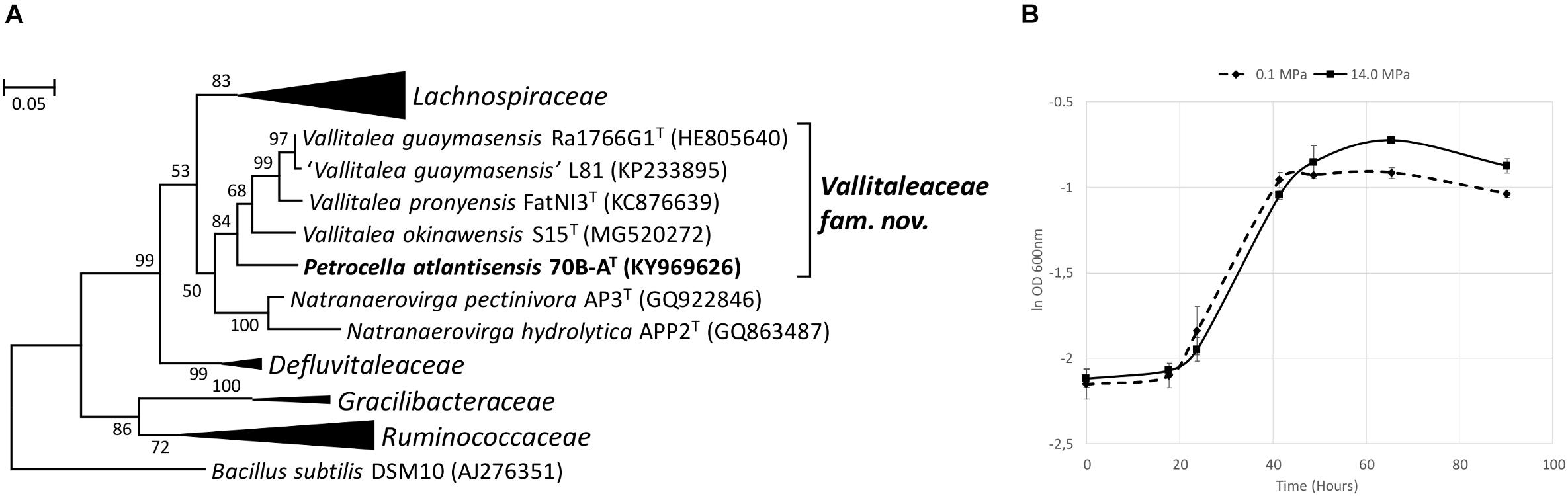
Figure 5. Phylogenetic and physiological characteristics of strain 70B-AT. (A) Maximum Likelihood phylogenetic tree based on 1203 aligned base pairs of 16S rRNA gene sequences showing the position of strain 70B-AT in the Clostridiales order. The Kimura 2-parameter was used, and the analysis involved 34 sequences. Bacillus subtilis (AJ276351) was used as an outgroup. Bootstrap values higher than 50% (based on 1 000 replicates) are shown at branch nodes. Accession numbers of type species are indicated in brackets. Bar, 0.05 substitutions per nucleotide. (B) Growth curves of strain 70B-AT at 0.1 and 14.0 MPa. Apart of hydrostatic pressure, all other culture conditions were identical (in Hungate tube with no headspace). The BM medium was supplemented with 0.2 g l-1 yeast extract and 20 mM glucose. The ln OD 600nm average from triplicates is shown here. Error bars are the associated standard deviation.
The major cellular fatty acids of strain 70B-AT were C16:1 w7c (35.6%), C16:0 (22.1%) and C16:1 w7c DMA (14.3%) (Supplementary Table 3). The main polar lipids consisted of nine phospholipids, one glycolipid, one phosphatidylglycerol and one phosphoglycolipid (Table 3).
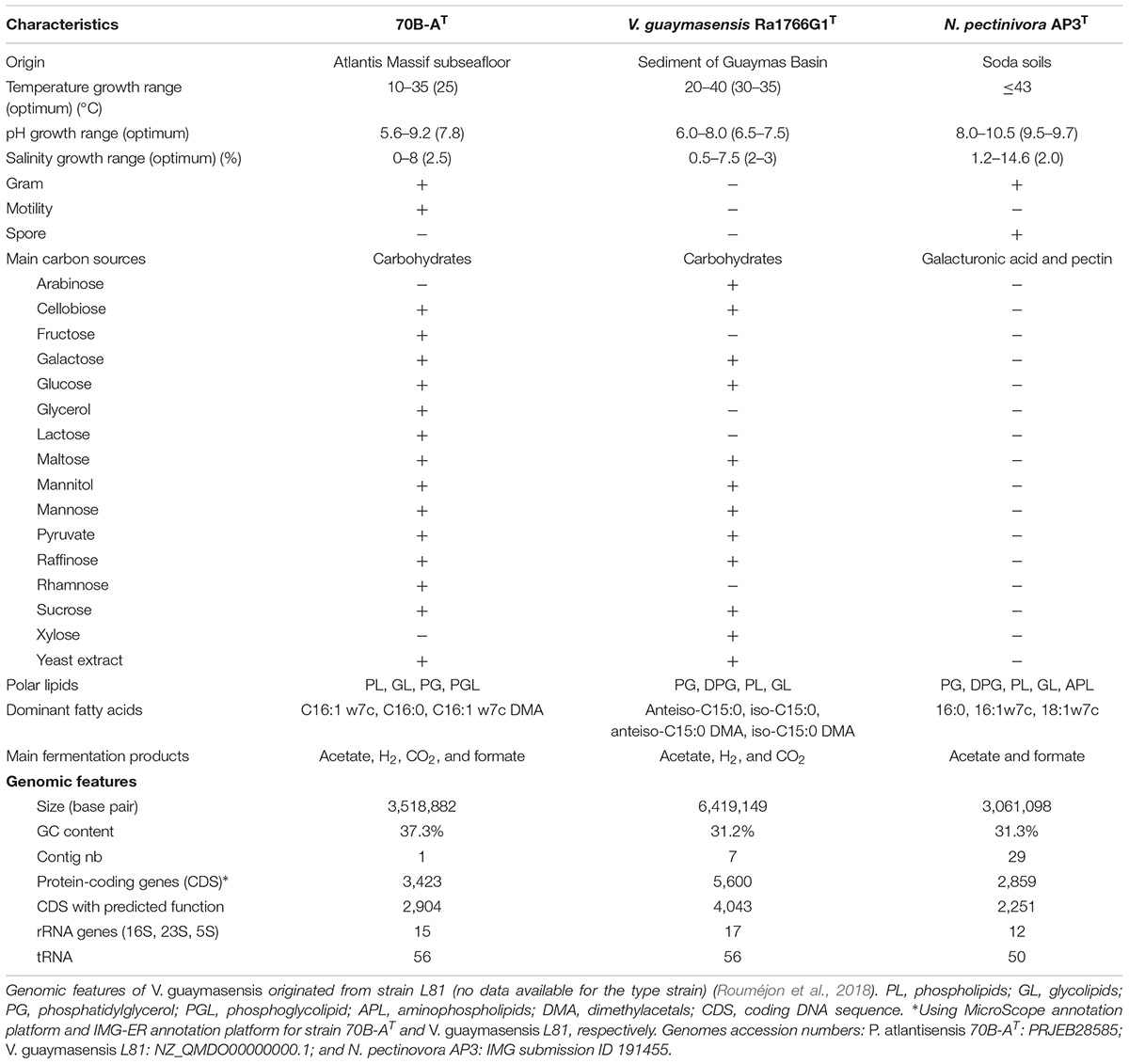
Table 3. Differential phenotypic and genotypic characteristics between strain 70B-AT and type strains of recognized Vallitalea species: V. guaymasensisT (Kumar et al., 2016), Natranaerovirga pectinivoraT (Schrenk et al., 2010).
Strain 70B-AT cells rod shaped (0.4–0.7 μm in diameter and 2.0–12.0 μm in length) during the exponential growth phase (Supplementary Figure 3A) where it is motile through the action of observed polar and lateral monotrich flagella (data not shown). No spore formation was observed. Under unfavorable conditions (e.g., pH 11), morphology changed and showed inflated and deformed cells (Supplementary Figure 3B). The cell wall structure observed by transmission electron microscopy revealed a Gram-positive structure with the presence of a multilayered cell wall without an outer membrane as confirmed by Gram-staining (Supplementary Figure 3C).
Strain 70B-AT grows under strict anaerobic conditions from 0 to 8% (w/v) NaCl (optimum at 2.5% (w/v) of NaCl; Supplementary Figure 4B), 10 to 35°C (optimum at 25°C; Supplementary Figure 4A), and pH 5.6 to 9.2 (optimum at pH 7.4-8.0). HP did not significantly improve its maximum specific growth rate: μmax was in average 0.048 h-1 at 0.1 MPa and 0.045 h-1 at 14.0 MPa (Figure 5B). However, the maximum OD was significantly higher at 14.0 MPa (0.476 ± 0.016) than at 0.1 MPa (0.422 ± 0.011; Student t-test, p = 0.003).
Strain 70B-AT was able to use cellobiose, fructose, galactose, glucose, glycerol, lactose, maltose, mannitol, mannose, pyruvate, raffinose, rhamnose, ribose, sucrose, trehalose, tryptone, yeast extract (YE), but not acetate, arabinose, butyrate, casaminoacids, citrate, crotonate, ethanol, formate, lactate, methanol, pectine, peptone, propionate, succinate, TMA, xylose. No growth was observed with only H2/CO2 or H2/CO2 and acetate as sole carbon sources. Strain 70B-AT is strictly chemoorganotrophic as confirmed by HPLC analysis of substrate utilization. Growth on carbohydrates resulted in acetate as the main metabolic product, along with formate, H2 and CO2 (Table 3). Strain 70B-AT was not able to use thiosulfate, sulfate, sulfite, nitrite, nitrate, Fe(III) citrate and elemental sulfur as electron acceptors. Under optimal conditions, the maximal growth rate (on BM medium plus glucose) was 0.048 h-1.
Strain 70B-AT has a lower growth temperature range than the type species of Vallitalea and Natranaerovirga (Table 3). While its pH and salinity ranges as well as its use of carbon source (mainly carbohydrates) are similar to that of V. guaymasensis, strain 70B-AT differs by its capacity to utilize fructose, glycerol, lactose and rhamnose (Table 3). Fatty acids profile resembles more that of N. pectinovora with abundant C16 and the absence of C15. Unlike the two other strains, 70B-AT displays cell motility and a singular profile of polar lipids and a higher DNA G + C content (Table 3).
Genome Analysis of Strain 70B-AT
The complete genome (one single contig) of strain 70B-AT consists of a circular chromosome of 3 518 882 bp, with 37.3% GC content (Table 3). The chromosome contains 3 536 predicted genes, of which 3 423 are protein-coding. The size of the strain 70B-AT genome is considerably smaller than that of V. guaymasensis L81, 6.42 Mb, and V. okinawensis, 5.86 Mb, but in the range of those of the Natranaerovirga genomes, 3.06 Mb for N. pectinivora and 2.98 Mb for N. hydrolytica (Schouw et al., 2018; Sun et al., 2018). Of the 3 423 protein-coding genes, 2 904 were given a predicted function (Figure 6). Carbohydrates and amino acids transport and metabolisms were among the most represented functional categories with respectively 6.3 and 6.1% of the function-predicted genes. In agreement with the culture-based conclusions on the metabolism, the genome of strain 70B-AT encodes several glycosyl hydrolases (β-galactosidase, β-glucosidase, and α-amylase), proteases and peptidases. It contains numerous ABC-transporters of multi-sugars (pentose, hexose, and di-saccharides) and several sugars (mannose, fructose, lactose) phosphotransferase systems (PTS). A complete glycolysis (Embden-Meyerhof) and gluconeogenesis pathways were identified, as well as uncomplete Entner-Doudoroff and pentose phosphate pathways as observed in some Gram positive bacteria and archaea (Conway, 1992). The genes for the complete biosynthesis pathways of all the amino acids are present, except for the L-lysine and L-methionine which are incomplete and thus maybe not operational. Nevertheless, the genome encodes two oligo-peptides and, at least 12 amino-acids ABC transporters with all kind of specificity for polar amino-acids, branched-amino acids, lysine or arginine, and proline-betaine, suggesting these served as substrates for growth. The degradation pathways identified for L-alanine, L-cysteine, L-tryptophan, L-serine and L-threonine lead to pyruvate while those of L-aspartate, L-asparagine, L-Glutamate, and L-Glutamine generate oxaloacetate, fumarate or acetyl-CoA which could aliment an uncomplete citric acid (TCA) cycle (the succinyl-CoA synthase gene is lacking). Pyruvate could also be directly fermented in lactate (by a lactate dehydrogenase), acetate or ethanol (by an alcohol dehydrogenase) or via the common mixed acid fermentation pathway. A putative pyruvate: ferredoxin oxidoreductase catalyzes the formation of acetyl-CoA from the pyruvate, coupled to the reduction of ferredoxin (Fd). Thus, most of the reducing power of the diverse fermentations is transferred to NADH and reduced Fd electron carriers, and we postulate, as recently proposed for V. guaymasensis (Schouw et al., 2018), this fuels a hydrogenase to generate H2. Indeed, genes for a tetrameric [Fe-Fe] hydrogenase are found as an operon (PATL70BA_1019 to PATL70BA_1022) homologous to hndA, hndB, hndC, hndD, encoding a cytosolic NADP-reducing hydrogenase in Desulfovibrio fructosivorans (Dermoun et al., 2002). In this sulfate-reducing bacterium, this enzyme could either catalyzes H2 oxidation during growth on H2 as a sole energy source or produces H2 when growing by fermentation of fructose in the absence of sulfate as a terminal electron acceptor. We assume that this tetrameric hydrogenase utilizes the mechanism of flavin-based electron bifurcation (FBEB) (Schuchmann et al., 2018): the exergonic electron flow from reduced Fd to H+ drives endergonic electron flow from NADH to H+. The archetype of such so-called bifurcative/confurcative hydrogenase was first described in Thermotoga maritima (Schut and Adams, 2009). Two additional hydrogenases-encoding genes are present, PATL70BA_1241 is homologous to a 4Fe-4S dicluster domain-containing, poorly characterized, periplasmic hydrogenase found in many anaerobic Firmicutes, and PATL70BA_2847, another homolog of the hndA subunit gene. Besides, the genes encoding a [FeFe] hydrogenase maturase (HydEFG), involved in the synthesis and incorporation of the di-iron center, were identified. The genome of strain 70B-AT also contains an operon (rnfC, rnfD, rnfG, rsxE, rsxA, rnfB) encoding an Rnf complex coupling the translocation of proton/sodium ion across the membrane with the reversible oxidation of reduced ferredoxin with NAD+ (Westphal et al., 2018). The Rnf complex could, therefore, serve to generate a proton/sodium gradient while equilibrating the pool of reductants (NADH) produced during sugars or amino-acids fermentation (Buckel and Thauer, 2018; Westphal et al., 2018).
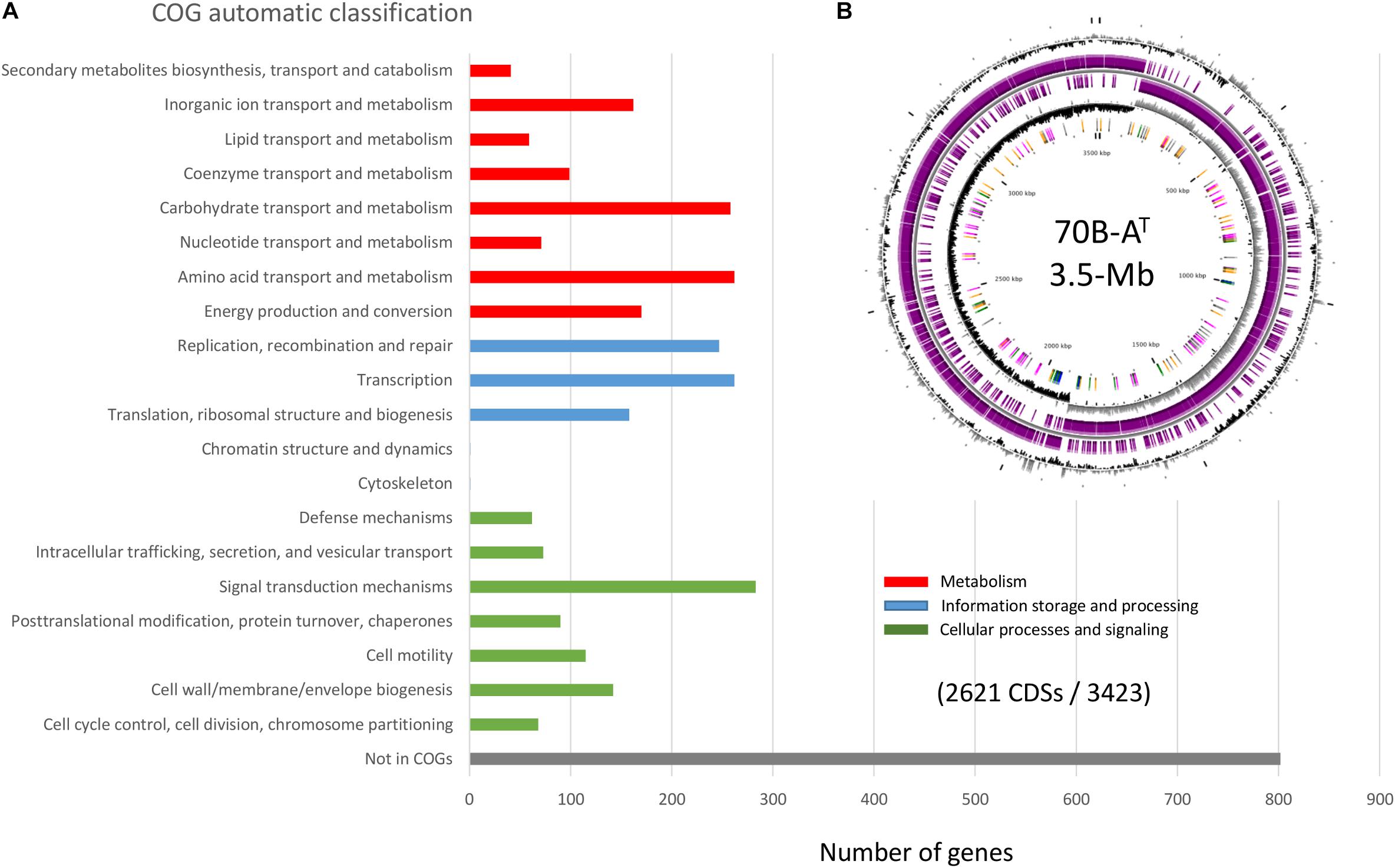
Figure 6. Strain 70B-AT genome analysis. (A) Functional categorization of 2621 Coding DNA Sequences (CDS) (out of 3423) based on the Clusters of Orthologous Groups (COGs) database (Galperin et al., 2015), (B) Circular genome view.
The genome encodes both a V-type ATPase and an F-type of ATPase. The V-type ATPases use ATP to translocate protons across membranes, while F-type ATPases generate ATP via proton translocation (Perzov et al., 2001). The genome also contains a cluster of 9 genes (PATL70BA_2417 to 2426) encoding a nitrogenase (nifH, nifD, nifK, nifE, nifB, nifV) conferring this bacterium the ability to fix N2, which may represent a selective advantage to survive in its challenging habitat. Several clusters of metal or metalloids (Fe, Zn, Cr, Ni, Ar, Hg) transporters genes were also found which could be involved in either import (iron siderophores) or export (detoxication) processes. Finally, the genome contains numerous transposases and integrases genes, of which many belong to putative prophages and two large conjugative integrated plasmids, also called integrons (Mazel, 2006), known as essential agents of bacterial genome evolution.
Discussion
Today, our knowledge of subseafloor microbial ecosystems is mainly based on data from culture-independent methods such as 16S rRNA barcoding or metagenomics. Although these approaches have been proved incomparable to explore and describe the extent of microbial diversity and model the functioning of these ecosystems, they present only a partial view of the real picture since most of the microorganisms inhabiting these environments are uncultivated (Jungbluth et al., 2016). Subseafloor microorganisms have been mostly recalcitrant to cultivation in laboratory, making their physiological characterization complicated. Most of these microorganisms were isolated from sedimentary rock cores and included mostly aerobes or facultative anaerobes but a few strict anaerobes even though, passed the first tens centimeters depth below the seafloor, these environments are essentially anoxic (Toffin and Alain, 2014). Previous attempts at cultivating anaerobes from various subseafloor sediments often led to the isolation of the same few ‘generalist’ bacteria belonging to genera counting numerous species and strains encompassing a broad spectrum of environmental conditions (Ciobanu et al., 2014; Parkes et al., 2014).
In this study, our main goal was to cultivate anaerobic microorganisms inhabiting the rocky subseafloor associated with active serpentinization characterized by circulation of high pH fluids enriched in H2, CH4 and other organic molecules abiotically produced. To the best of our knowledge, the only cultivated anaerobes were isolated by our team from a shallow submarine serpentinite-hosted hydrothermal system, analogous to the LCHF, located in the Prony bay (New Caledonia) (Ben Aissa et al., 2014, 2015; Mei et al., 2014, 2016; Bes et al., 2015). In the present study, putative moderately halo-alkaliphilic bacteria (related to Alkalibacterium, Halomonas, Tissierella and the proposed genus Petrocella) were enriched and in some case isolated in cultures carried out at pH 8.5, while the only isolate obtained from the cultures at pH 10 (in the common range of pH values observed in low temperature serpentinizing systems) was a strain of Bacillus circulans, a facultative anaerobe, spore-forming, frequently detected in soils, but no obligate alkaliphile as targeted by the pH conditions. These results may be explained by the relatively low pH values (7.82–7.87) measured in the hole bottom waters, with maximum values of pH 7.98 recorded in Hole M0070C (Früh-Green et al., 2018). Anaerobic H2-consumers, sulfate-reducers or methanogens (main metabolisms detected at LCHF) were not abundant in cultures despite the use of various culture conditions targeting these metabolisms and the elevated H2 and CH4 levels measured in bottom waters (5-73 and 2-5 nM, respectively, at Site M0070 where gas bubbles were observed issuing from the hole, see Figure 13 in Früh-Green et al. (2018)).
In such rock-hosted subseafloor ecosystems, cell abundance is well known to be exceptionally low making contamination a crucial issue, and cultivation of indigenous microorganism very challenging. Cell abundance in the IODP 357 core samples was very low ranging from tens to thousands of cells cm-3 with a maximum in Hole M0069A sediments (up to 1.6 × 104 cells cm-3), before decreasing rapidly to <102 cells cm-3 in the underlying basement rocks (Früh-Green et al., 2018). In this study, growth was observed after a relatively short incubation time (1 month) in M0069A-9R2 and M0070C-3R1 enrichment cultures at 0.1 MPa and 25°C which may be due to contamination as suggested by the affiliation (at 99% identity) of the dominant OTU to genus or species corresponding to ubiquitous bacteria (Citrobacter freundii, Proteiniclasticum ruminis, Bacillus circulans; Figure 4 and Supplementary Figures 2A,B) found in a wide range of habitats not specific of the deep biosphere or serpentinizing systems. In contrast with cultures at 0.1 MPa, utilization of HP induced a drastic change in the structure of the bacterial communities for all combination of samples and cultures conditions (medium and incubation temperature), even at modest HP of 8.2 MPa, when compared to their counterpart incubated at atmospheric pressure (0.1 MPa gas headspace) as shown in Figure 3. Such phenomena has been since confirmed in our lab (Garel et al., 2019). Thus, one may assume that even moderately elevated HP inhibits growth of surface waters microorganisms and other contaminants that in these conditions are outcompeted by true deep sea/rock inhabitants. HP allowed the cultivation of novel microorganisms such as a potential new Tissierella species obtained at 8.2 MPa from M0072B-7R1 core (metagabbro) and a new Clostridiales lineage obtained at 14.0 MPa with M0070C-3R1 core sample (carbonate-cemented basaltic breccia) (Figure 4 and Supplementary Figures 2A,B). Moreover, the isolate 70B-AT, representing this new lineage, exhibits a strict anaerobic growth at 14.0 MPa making it very unlikely to originate from seawater or drilling equipment, but instead it may come from anoxic subseafloor. Strain 70B-AT, as well as its closest neighbors belonging to the Vallitalea genus, were adapted to live in the deep-sea and subseafloor environments, both impacted by various kinds of hydrothermal activity in different geographical and geodynamic area (Arctic Mid-Ocean Ridge, Northeast and Southwest Pacific Ocean, East China Sea and now Mid-Atlantic Ridge). Here, strain 70B-AT displayed maximum cell density (ODmax) under HP conditions (at 14 MPa). Knowing that the maximum population density (ODmax) can strongly influence the definition of the optimal growth conditions (Martini et al., 2013), strain 70B-AT can therefore be considered as a piezophile even if further studies are needed to characterize its lifestyle under HP.
Mason et al. (2010) gave the first insight into the microbial community inhabiting the Atlantis Massif subseafloor using molecular approaches. They identified bacteria usually related to hydrocarbon-rich environment and recognized hydrocarbon degraders (Mason et al., 2010). Here, potential hydrocarbon degraders (Halomonas, Sphingomonas) were also detected as dominant in our anaerobic enrichments. The hydrocarbons detected in rocks of the Atlantis Massif may have a marine origin but might also originate from abiotic organic synthesis associated with serpentinization (Delacour et al., 2008; Hickok et al., 2018; Ménez et al., 2018), which may potentially sustain the endolithic microbial community. Interestingly, the closest neighbors of strain 70B-AT belonging to Vallitalea genus originated from (i) the serpentinizing hydrothermal system of Prony (PHF; New Caledonia) (Ben Aissa et al., 2014), (ii) the Guaymas basin, a deep hydrothermally influenced ecosystem rich in hydrocarbons (Lakhal et al., 2013) and (iii) Loki’s Castle, a hydrocarbon-rich hydrothermal field (Schouw et al., 2016). However, the role of these microorganisms in hydrocarbon degradation (Schouw et al., 2016) has not yet been established (Schouw et al., 2018). Culture experiments showed that strain 70B-AT, like strains V. guaymasensis Ra1766G1T and L81, and V. pronyensis strain FatNI3T, does not use alkanes for growth but, instead, can degrade various carbohydrates and proteinaceous compounds, suggesting this is a metabolic feature common to members of the novel Vallitaleaceae family. Genome analysis of strain 70B-AT confirmed the utilization of sugars which are fermented mostly in acetate, CO2 and H2 via the glycolysis and suggests that strain 70B-AT is able to import oligo-peptides and amino acids and to convert them to acetate via oxidation to pyruvate and acetyl-CoA. The presence of a putative electron bifurcative/confurcative hydrogenase and an ion-motive Rnf complex, enabling ferredoxin-based pathways, seems to be crucial for energy conservation in members of the proposed family Vallitaleaceae. Interestingly, the closest homologs of this novel hydrogenase, and Rnf complex were detected in the same metagenome-assembled genomes (MAG) of dominant uncultivated Firmicutes from deep terrestrial subsurface sediments (Hernsdorf et al., 2017).
In conclusion, this study shows that hydrostatic pressure helps to enrich novel anaerobes from subseafloor rocks. The new isolate Petrocella atlantisensis 70B-AT is considered to represent a novel species of a novel genus within a novel proposed family Vallitaleaceae. It is a halotolerant, psychrotolerant, mesophilic and piezophilic chemoheterotroph able to use carbohydrates, proteinaceous compounds and potentially amino acids. Strain 70B-AT represents the first cultivated and characterized representative of the Atlantis Massif and will constitute a model strain to further study microbial adaptation to the deep subseafloor in a context of serpentinizing ultramafic rocks.
Description of Vallitaleaceae fam. nov.
Cells were rod shaped, obligately anaerobic, mesophilic, neutrophilic and marine. Capable of fermentation (no electron acceptor identified), using cellobiose, galactose, glucose, maltose, mannose, raffinose, sucrose and yeast extract, but not acetate (contrarily to both Natranaerovirga species). Originating from hydrothermal fields and/or deep oceanic subsurface habitats rich in hydrocarbon or related to serpentinization process. The family contains the type genus Vallitalea and the genus Petrocella.
Description of Petrocella gen. nov.
Petrocella (Pe.tro.cel’la N. L. n. petra a rock, stone; L. fem. n. cella a cell; N. L. fem. n. Petrocella a rod isolated from a rock). Gram-positive, motile, non-sporulating, marine and mesophilic rods. Fermentative and obligate anaerobe, able to use a wide range of sugars. It belongs to the order Clostridiales (phylum Firmicutes). The DNA G+C content of the type strain of the type species is 37.7 mol%. The type species is Petrocella atlantisensis.
Description of Petrocella atlantisensis sp. nov.
Petrocella atlantisensis (a.tlan.tis.en’sis. N. L. fem. adj. atlantisensis originated from the Atlantis Massif). It displays the following features in addition to those listed for the genus description. Cells are approximately 2–12 μm long and 0.4–0.7 μm wide, occurring singly or in pairs. Cells are motile and possess polar or lateral monotrich flagella. No spore was observed. Growth occurs at 10–35°C (optimum 25°C), at pH 5.6–9.2 (optimum pH 7.4–8.0) and with 0–8% (w/v) NaCl (optimum 2.5%). Yeast extract is required for growth. Cellobiose, fructose, galactose, glucose, glycerol, lactose, maltose, mannitol, mannose, pyruvate, raffinose, rhamnose, ribose, sucrose, trehalose, tryptone and yeast extract are used as electron donors, but not acetate, arabinose, butyrate, casamino acids, citrate, crotonate, ethanol, formate, H2/CO2, H2/CO2 + acetate, lactate, methanol, pectine, peptone, propionate, succinate, TMA, xylose. None of the following electron acceptors was used: elemental sulfur, sulfate, thiosulfate, sulfite, nitrate and nitrite. The major fatty acids are C16:1 w7c, C16:0 and C16:1 w7c DMA. The type strain, 70B-AT, ( = DSM 105309T = JCM 32078T) was isolated from rocks drilled in the Atlantis Massif (Mid-Atlantic Ridge, 30° N).
Data Availability
The datasets generated for this study can be found in SRA, and ENA or Genbank, SRP148750 (MiSeq V3-V4 amplicons raw data), and PRJEB28585 or LR130778 (whole genome sequence of strain 70B-AT).
Author Contributions
AP wrote the manuscript in collaboration with MQ. MQ, GE, and AP designed the experiments. MQ collected the samples. MQ, AP, EZ, MG, and CT carried out the high-pressure experiments. EF performed the microbial diversity analyses. CV performed the genome sequencing. All authors contributed to the discussion and the writing of the manuscript.
Funding
This project was financially supported by the deepOASES ANR project (ANR-14-CE01-0008-06 and ANR-14-CE01-0008-01). Samples have been obtained from the International Ocean Discovery Program (IODP). Sequencing work in GeT Toulouse was funded as part of “Investissement d’avenir” program managed by Agence Nationale pour la Recherche (contract ANR-10-INBS-09). The project leading to this publication has received funding from European FEDER Fund under project 1166-39417.
Conflict of Interest Statement
The authors declare that the research was conducted in the absence of any commercial or financial relationships that could be construed as a potential conflict of interest.
Acknowledgments
This research used samples and data provided by the International Ocean Discovery Program (IODP). We thank the co-chief scientists Prof. Gretchen Früh-Green and Dr. Beth Orcutt of IODP 357 “Atlantis Massif Serpentinization and Life” expedition that took place in 2015 (26 October–11 December 2015), and the staff of the drillship RRS James Cook carrying the BGS Rockdrill2 and the MARUM-MeBo seafloor drills. We also thank the other members of the shipboard scientific team (especially Y. Morono, M. O. Schrenk, and K. I. Twing) who enabled the collection and photography of the samples used in this study. We also thank M. Bartoli (MIO) for the electron microscopy photographs of strain 70B-AT and H. Gaussier for correcting English in the text. This work was performed in collaboration with the GeT core facility, Toulouse, France (http://get.genotoul.fr) and was supported by France Génomique National Infrastructure; C. Roques and A. Castinel are especially thanked.
Supplementary Material
The Supplementary Material for this article can be found online at: https://www.frontiersin.org/articles/10.3389/fmicb.2019.01497/full#supplementary-material
Footnotes
References
Bartlett, D. H., Lauro, F. M., and Eloe, E. A. (2007). “Microbial adaptation to high pressure,” in Physiology and Biochemistry of Extremophiles, eds C. Gerday and N. Glandsdorf (Washington, DC: American Society for Microbiology Press), 333–348. doi: 10.1128/9781555815813.ch25
Ben Aissa, F., Postec, A., Erauso, G., Payri, C., Pelletier, B., Hamdi, M., et al. (2014). Vallitalea pronyensis sp nov., isolated from a marine alkaline hydrothermal chimney. Int. J. Syst. Evol. Microbiol. 64, 1160–1165. doi: 10.1099/ijs.0.055756-0
Ben Aissa, F., Postec, A., Erauso, G., Payri, C., Pelletier, B., Hamdi, M., et al. (2015). Characterization of Alkaliphilus hydrothermalis sp. nov., a novel alkaliphilic anaerobic bacterium, isolated from a carbonaceous chimney of the prony hydrothermal field, New Caledonia. Extremophiles 19, 183–188. doi: 10.1007/s00792-014-0697-y
Bes, M., Merrouch, M., Joseph, M., Quemeneur, M., Payri, C., Pelletier, B., et al. (2015). Acetoanaerobium pronyense sp. nov., an anaerobic alkaliphilic bacterium isolated from a carbonate chimney of the prony hydrothermal field (New Caledonia). Int. J. Syst. Evol. Microbiol. 65, 2574–2580. doi: 10.1099/ijs.0.000307
Brazelton, W. J., Schrenk, M. O., Kelley, D. S., and Baross, J. A. (2006). Methane- and sulfur-metabolizing microbial communities dominate the lost city hydrothermal field ecosystem. Appl. Environ. Microbiol. 72, 6257–6270. doi: 10.1128/AEM.00574-06
Buckel, W., and Thauer, R. K. (2018). Flavin-based electron bifurcation, ferredoxin, flavodoxin, and anaerobic respiration with protons (Ech) or NAD+ (Rnf) as electron acceptors: a historical review. Front. Microbiol. 9:401. doi: 10.3389/fmicb.2018.00401
Caporaso, J. G., Kuczynski, J., Stombaugh, J., Bittinger, K., Bushman, F. D., Costello, E. K., et al. (2010). QIIME allows analysis of high-throughput community sequencing data. Nat. Methods 7, 335–336. doi: 10.1038/nmeth.f.303
Ciobanu, M.-C., Burgaud, G., Dufresne, A., Breuker, A., Redou, V., Ben Maamar, S., et al. (2014). Microorganisms persist at record depths in the subseafloor of the canterbury Basin. ISME J. 8, 1370–1380. doi: 10.1038/ismej.2013.250
Conway, T. (1992). The entner-doudoroff pathway: history, physiology and molecular biology. FEMS Microbiol. Rev. 9, 1–27. doi: 10.1111/j.1574-6968.1992.tb05822.x
Delacour, A., Früh-Green, G. L., Bernasconi, S. M., Schaeffer, P., and Kelley, D. S. (2008). Carbon geochemistry of serpentinites in the lost city hydrothermal system (30 degrees N, MAR). Geochim. Cosmochim. Acta 72, 3681–3702. doi: 10.1016/j.gca.2008.04.039
Dermoun, Z., De Luca, G., Asso, M., Bertrand, P., Guerlesquin, F., and Guigliarelli, B. (2002). The NADP-reducing hydrogenase from Desulfovibrio fructosovorans: functional interaction between the C-terminal region of HndA and the N-terminal region of HndD subunits. Biochim. Biophys. Acta 1556, 217–225. doi: 10.1016/S0005-2728(02)00364-X
D’Hondt, S., Jørgensen, B. B., Miller, D. J., Batzke, A., Blake, R., Cragg, B. A., et al. (2004). Distributions of microbial activities in deep subseafloor sediments. Science 306, 2216–2221. doi: 10.1126/science.1101155
Edgar, R. C. (2004). MUSCLE: multiple sequence alignment with high accuracy and high throughput. Nucliec Acids Res. 32, 1792–1797. doi: 10.1093/nar/gkh340
Finster, K. W., Cockell, C. S., Voytek, M. A., Gronstal, A. L., and Kjeldsen, K. U. (2009). Description of Tessaracoccus profundi sp.nov., a deep-subsurface actinobacterium isolated from a chesapeake impact crater drill core (940 m depth). Antonie van Leeuwenhoek 96, 515–526. doi: 10.1007/s10482-009-9367-y
Frouin, E., Bes, M., Ollivier, B., Quéméneur, M., Postec, A., Debroas, D., et al. (2018). Diversity of rare and abundant prokaryotic phylotypes in the prony hydrothermal field and comparison with other serpentinite-hosted ecosystems. Front. Microbiol. 9:102. doi: 10.3389/fmicb.2018.00102
Früh-Green, G. L., Orcutt, B. N., Green, S., Cotterill, C., and the Expedition 357 Scientists (2016). “Expedition 357 preliminary report: atlantis massif serpentinization and Life,” in Proceedings of the International Ocean Discovery Program, College Station, TX.
Früh-Green, G. L., Orcutt, B. N., Green, S. L., Cotterill, C., and the Expedition 357 Scientists (2017a). “Central sites,” in Proceedings of the International Ocean Discovery Program, College Station, TX.
Früh-Green, G. L., Orcutt, B. N., Green, S. L., Cotterill, C., and the Expedition 357 Scientists (2017b). “Eastern sites,” in Proceedings of the International Ocean Discovery Program, College Station, TX.
Früh-Green, G. L., Orcutt, B. N., Green, S. L., Cotterill, C., and the Expedition 357 Scientists (2017c). “Expedition 357 methods,” in Proceedings of the International Ocean Discovery Program, College Station, TX.
Früh-Green, G. L., Orcutt, B. N., Green, S. L., Cotterill, C., and the Expedition 357 Scientists (2017d). “Expedition 357 summary,” in Proceedings of the International Ocean Discovery Program, College Station, TX.
Früh-Green, G. L., Orcutt, B. N., Green, S. L., Cotterill, C., and the Expedition 357 Scientists (2017e). “Northern sites,” in Proceedings of the International Ocean Discovery Program, College Station, TX.
Früh-Green, G. L., Orcutt, B. N., Green, S. L., Cotterill, C., and the Expedition 357 Scientists (2017f). “Western sites,” in Proceedings of the International Ocean Discovery Program, College Station, TX.
Früh-Green, G. L., Orcutt, B. N., Rouméjon, S., Lilley, M. D., Morono, Y., Green, S., et al. (2018). Magmatism, serpentinization and life: insights through drilling the Atlantis massif (IODP expedition 357). Lithos 323, 137–155. doi: 10.1016/j.lithos.2018.09.012
Galperin, M. Y., Makarova, K. S., Wolf, Y. I., and Koonin, E. V. (2015). Expanded microbial genome coverage and improved protein family annotation in the COG database. Nucleic Acids Res. 43, D261–D269. doi: 10.1093/nar/gku1223
Garel, M., Bonin, P., Martini, S., Guasco, S., Roumagnac, M., Nagib, B., et al. (2019). Pressure-retaining sampler and high-pressure systems to study deep-sea microbes under in situ conditions. Front. Microbiol. 10:453. doi: 10.3389/fmicb.2019.00453
Hernsdorf, A. W., Amano, Y., Miyakawa, K., Ise, K., Suzuki, Y., Anantharaman, K., et al. (2017). Potential for microbial H2 and metal transformations associated with novel bacteria and archaea in deep terrestrial subsurface sediments. ISME J. 11, 1915–1929. doi: 10.1038/ismej.2017.39
Hickok, K. A., Nguyen, T. B., and Lang, S. Q. (2018). Assessment of apolar lipids in subseafloor rocks and potential contaminants from the Atlantis Massif (IODP expedition 357). Org. Geochem. 122, 68–77. doi: 10.1016/j.orggeochem.2018.05.003
Hirayama, H., Abe, M., Miyazaki, J., Sakai, S., Nagano, Y., and Takai, K. (2015). “Data report: cultivation of microorganisms from basaltic rock and sediment cores from the north pond on the western flank of the mid-atlantic ridge, IODP expedition 336,” in Proceedings of the IODP (Integrated Ocean Drilling Program Management International, Inc.), eds K. J. Edwards, W. Bach, A. Klaus, and the Expedition 336 Scientists Tokyo. doi: 10.2204/iodp.proc.336.204.2015
Hungate, R. E. (1969). “A roll tube method for the cultivation of strict anaerobes,” in Methods in Microbiology, eds J. R. Norris and D. W. Ribbons (London: Academic Press), 117–132. doi: 10.1016/s0580-9517(08)70503-8
Jørgensen, S. L., and Zhao, R. (2016). Microbial inventory of deeply buried oceanic crust from a young ridge flank. Front. Microbiol. 7:820. doi: 10.3389/fmicb.2016.00820
Jungbluth, S. P., Bowers, R. M., Lin, H.-T., Cowen, J. P., and Rappé, M. S. (2016). Novel microbial assemblages inhabiting crustal fluids within mid-ocean ridge flank subsurface basalt. ISME J. 10, 2033–2047. doi: 10.1038/ismej.2015.248
Kallmeyer, J., Pockalny, R., Adhikari, R. R., Smith, D. C., and D’Hondt, S. (2012). Global distribution of microbial abundance and biomass in subseafloor sediment. Proc. Natl. Acad. Sci. U.S.A. 109, 16213–16216. doi: 10.1073/pnas.1203849109
Kaye, J. Z., Márquez, M. C., Ventosa, A., and Baross, J. A. (2004). Halomonas neptunia sp. nov., Halomonas sulfidaeris sp. nov., Halomonas axialensis sp. nov. and Halomonas hydrothermalis sp. nov.: halophilic bacteria isolated from deep-sea hydrothermal-vent environments. Int. J. Syst. Evol. Microbiol. 54, 499–511. doi: 10.1099/ijs.0.02799-0
Kelley, D. S., Karson, J. A., Früh-Green, G. L., Yoerger, D. R., Shank, T. M., Butterfield, D. A., et al. (2005). A serpentinite-hosted ecosystem: the lost city hydrothermal field. Science 307, 1428–1434. doi: 10.1126/science.1102556
Khelaifia, S., Fardeau, M.-L., Pradel, N., Aussignargues, C., Garel, M., Tamburini, C., et al. (2011). Desulfovibrio piezophilus sp. nov., a piezophilic, sulfate-reducing bacterium isolated from wood falls in the mediterranean Sea. Int. J. Syst. Evol. Microbiol. 61, 2706–2711. doi: 10.1099/ijs.0.028670-0
Kumar, S., Stecher, G., and Tamura, K. (2016). MEGA7: molecular evolutionary genetics analysis version 7.0 for bigger datasets. Mol. Biol. Evol. 33, 1870–1874. doi: 10.1093/molbev/msw054
Lakhal, R., Pradel, N., Postec, A., Hamdi, M., Ollivier, B., Godfroy, A., et al. (2013). Vallitalea guaymasensis gen. nov., sp. nov., isolated from marine sediment. Int. J. Syst. Evol. Microbiol. 63(Pt 8), 3019–3023. doi: 10.1099/ijs.0.045708-0
Lane, D. J. (1991). “16S/23S rRNA sequencing,” in Nucleic Acid Techniques in Bacterial Systematics, eds E. Stackebrandt and M. Goodfellow (New York, NY: John Wiley and Sons), 115–175.
Lang, S. Q., Früh-Green, G. L., Bernasconi, S. M., Brazelton, W. J., Schrenk, M. O., and McGonigle, J. M. (2018). Deeply-sourced formate fuels sulfate reducers but not methanogens at lost city hydrothermal field. Sci. Rep. 8:755. doi: 10.1038/s41598-017-19002-5
Lauro, F., and Bartlett, D. (2007). Prokaryotic lifestyles in deep sea habitats. Extremophiles 12, 15–25. doi: 10.1007/s00792-006-0059-5
Levin, L. A., Baco, A. R., Bowden, D. A., Colaco, A., Cordes, E. E., Cunha, M. R., et al. (2016). Hydrothermal vents and methane seeps: rethinking the sphere of influence. Front. Mar. Sci. 3:72. doi: 10.3389/fmars.2016.00072
Marteinsson, V., Watrin, L., Prieur, D., Caprais, J.-C., Raguenes, G., and Erauso, G. (1995). Sulfur-metabolizing hyperthermophilic anaerobic archaea isolated from hydrothermal vents in the southwestern pacific ocean. Int. J. Syst. Bacteriol. 45, 623–632. doi: 10.1099/00207713-45-4-623
Martini, S., Al Ali, B., Garel, M., Nerini, D., Grossi, V., Pacton, M., et al. (2013). Effects of hydrostatic pressure on growth and luminescence of a moderately-piezophilic luminous bacteria Photobacterium phosphoreum ANT-2200. PLoS One 8:e66580. doi: 10.1371/journal.pone.0066580
Martin, W., Baross, J., Kelley, D., and Russell, M. J. (2008). Hydrothermal vents and the origin of life. Nat. Rev. Microbiol. 6, 805–814. doi: 10.1038/nrmicro1991
Mason, O. U., Nakagawa, T., Rosner, M., Van Nostrand, J. D., Zhou, J., Maruyama, A., et al. (2010). First investigation of the microbiology of the deepest layer of ocean crust. PLoS One 5:e15399. doi: 10.1371/journal.pone.0015399
Mazel, D. (2006). Integrons: agents of bacterial evolution. Nat. Rev. Microbiol. 4:608. doi: 10.1038/nrmicro1462
Mei, N., Postec, A., Erauso, G., Joseph, M., Pelletier, B., Payri, C., et al. (2016). Serpentinicella alkaliphila gen. nov., sp. nov., a novel alkaliphilic anaerobic bacterium isolated from the serpentinite-hosted prony hydrothermal field, New Caledonia. Int. J. Syst. Evol. Microbiol. 66, 4464–4470. doi: 10.1099/ijsem.0.001375
Mei, N., Zergane, N., Postec, A., Erauso, G., Oilier, A., Payri, C., et al. (2014). Fermentative hydrogen production by a new alkaliphilic Clostridium sp (strain PROH2) isolated from a shallow submarine hydrothermal chimney in prony bay, New Caledonia. Int. J. Hydrogen Energy 39, 19465–19473. doi: 10.1016/j.ijhydene.2014.09.111
Ménez, B., Pisapia, C., Andreani, M., Jamme, F., Vanbellingen, Q. P., Brunelle, A., et al. (2018). Abiotic synthesis of amino acids in the recesses of the oceanic lithosphere. Nature 564, 59–63. doi: 10.1038/s41586-018-0684-z
Orcutt, B. N., Bergenthal, M., Freudenthal, T., Smith, D., Lilley, M. D., Schnieders, L., et al. (2017). Contamination tracer testing with seabed drills: IODP expedition 357. Sci. Dril. 23, 39–46. doi: 10.5194/sd-23-39-2017
Orcutt, B. N., Sylvan, J. B., Knab, N. J., and Edwards, K. J. (2011). Microbial ecology of the dark ocean above, at, and below the seafloor. Microbiol. Mol. Biol. Rev. 75, 361–422. doi: 10.1128/MMBR.00039-10
Parkes, R. J., Cragg, B., Roussel, E., Webster, G., Weightman, A., and Sass, H. (2014). A review of prokaryotic populations and processes in sub-seafloor sediments, including biosphere:geosphere interactions. Mar. Geol. 352,409–425. doi: 10.1016/j.margeo.2014.02.009
Parkes, R. J., Sellek, G., Webster, G., Martin, D., Anders, E., Weightman, A. J., et al. (2009). Culturable prokaryotic diversity of deep, gas hydrate sediments: first use of a continuous high-pressure, anaerobic, enrichment and isolation system for subseafloor sediments (DeepIsoBUG). Environ. Microbiol. 11, 3140–3153. doi: 10.1111/j.1462-2920.2009.02018.x
Perzov, N., Padler-Karavani, V., Nelson, H., and Nelson, N. (2001). Features of V-ATPases that distinguish them from F-ATPases. FEBS Lett. 504, 223–228. doi: 10.1016/S0014-5793(01)02709-0
Picard, A., and Daniel, I. (2013). Pressure as an environmental parameter for microbial life — a review. Biophys. Chem. 183, 30–41. doi: 10.1016/j.bpc.2013.06.019
Postec, A., Quemeneur, M., Bes, M., Mei, N., Benaissa, F., Payri, C., et al. (2015). Microbial diversity in a submarine carbonate edifice from the serpentinizing hydrothermal system of the Prony Bay (New Caledonia) over a 6-year period. Front. Microbiol. 6:857. doi: 10.3389/fmicb.2015.00857
R Core Team (2017). A Language and Environment for Statistical Computing. Available at: https://www.R-project.org/ (accessed July, 2018).
Rouméjon, S., Früh-Green, G. L., Orcutt, B. N., and Zhao, R. (2018). Alteration heterogeneities in peridotites exhumed on the southern wall of the atlantis massif (IODP expedition 357). J. Petrol. 59, 1329–1358. doi: 10.1093/petrology/egy065
Santelli, C. M., Banerjee, N., Bach, W., and Edwards, K. J. (2010). Tapping the subsurface ocean crust biosphere: low biomass and drilling-related contamination calls for improved quality controls. Geomicrobiol. J. 27, 158–169. doi: 10.1080/01490450903456780
Schouw, A., Leiknes Eide, T., Stokke, R., Pedersen, R. B., Steen, I. H., and Bødtker, G. (2016). Abyssivirga alkaniphila gen. nov., sp. nov., an alkane-degrading, anaerobic bacterium from a deep-sea hydrothermal vent system, and emended descriptions of Natranaerovirga pectinivora and Natranaerovirga hydrolytica. Int. J. Syst. Evol. Microbiol. 66, 1724–1734. doi: 10.1099/ijsem.0.000934
Schouw, A., Vulcano, F., Roalkvam, I., Hocking, W., Reeves, E., Stokke, R., et al. (2018). Genome analysis of Vallitalea guaymasensis strain L81 isolated from a deep-sea hydrothermal vent system. Microorganisms 6:63. doi: 10.3390/microorganisms6030063
Schrenk, M. O., Brazelton, W. J., and Lang, S. Q. (2013). “Serpentinization, carbon, and deep life,” in Reviews Mineral Geochem, eds R. M. Hazen, A. P. Jones, and J. A. Baross (Chantilly, VA: Mineralogical Society of America), 575–606. doi: 10.1515/9781501508318-020
Schrenk, M. O., Huber, J. A., and Edwards, K. J. (2010). Microbial provinces in the subseafloor. Annu. Rev. Mar. Sci. 2, 279–304. doi: 10.1146/annurev-marine-120308-081000
Schuchmann, K., Chowdhury, N. P., and Müller, V. (2018). Complex multimeric [FeFe] hydrogenases: biochemistry, physiology and new opportunities for the hydrogen economy. Front. Microbiol. 9:2911. doi: 10.3389/fmicb.2018.02911
Schut, G. J., and Adams, M. W. W. (2009). The iron-hydrogenase of Thermotoga maritima utilizes ferredoxin and NADH synergistically: a new perspective on anaerobic hydrogen production. J. Bact. 191, 4451–4457. doi: 10.1128/JB.01582-08
Sorokin, D., Tourova, T., Panteleeva, A., Kaparullina, E., and Muyzer, G. (2012). Anaerobic utilization of pectinous substrates at extremely haloalkaline conditions by Natranaerovirga pectinivora gen. nov., sp. nov., and Natranaerovirga hydrolytica sp. nov., isolated from hypersaline soda lakes. Extremophiles 16, 307–315. doi: 10.1007/s00792-012-0431-6
Sun, Y.-T., Zhou, N., Wang, B.-J., Liu, X.-D., Jiang, C.-Y., Ge, X., et al. (2018). Vallitalea okinawensis sp. nov., isolated from okinawa trough sediment and emended description of the genus Vallitalea. Int. J. Syst. Evol. Microbiol. 69, 404–410. doi: 10.1099/ijsem.0.003158
Takahashi, S., Tomita, J., Nishioka, K., Hisada, T., and Nishijima, M. (2014). Development of a prokaryotic universal primer for simultaneous analysis of Bacteria and Archaea using next-generation sequencing. PLoS One 9:e105592. doi: 10.1371/journal.pone.0105592
Takai, K. (2011). “Limits of life and the biosphere: lessons from the detection of microorganisms in the deep sea and deep subsurface of the Earth,” in Origins and Evolution of Life: An Astrobiological Perspective, eds M. Gargaud, P. López-Garcìa, and H. Martin (Cambridge: Cambridge Univeristy Press), 469–486. doi: 10.1017/cbo9780511933875.030
Takai, K., Abe, M., Miyazaki, M., Koide, O., Nunoura, T., Imachi, H., et al. (2013). Sunxiuqinia faeciviva sp. nov., a facultatively anaerobic organoheterotroph of the Bacteroidetes isolated from deep subseafloor sediment. Int. J. Syst. Evol. Microbiol. 63, 1602–1609. doi: 10.1099/ijs.0.044065-0
Tamburini, C. (2006). “Life under pressure. Deep-sea microbial ecology,” in Life As We Know It, ed. J. Seckbach (Dordrecht: Springer), 650.
Tamburini, C., Boutrif, M., Garel, M., Colwell, R. R., and Deming, J. W. (2013). Prokaryotic responses to hydrostatic pressure in the ocean – a review. Environ. Microbiol. 15, 1262–1274. doi: 10.1111/1462-2920.12084
Tamburini, C., Goutx, M., Guigue, C., Garel, M., Lefèvre, D., Charrière, B., et al. (2009). Effects of hydrostatic pressure on microbial alteration of sinking fecal pellets. Deep Sea Res. Part 2 Top. Stud. Oceanogr. 56, 1533–1546. doi: 10.1016/j.dsr2.2008.12.035
Toffin, L., and Alain, K. (2014). “Technological state of the art and challenges. Cultivation of marine subseafloor microorganisms: state-of-the-art solutions and major issues,” in Microbial Life of the Deep Biosphere, eds J. Kallmeyer and D. Wagner (Berlin: Walter De Gruyter), 83–89.
Toffin, L., Bidault, A., Pignet, P., Tindall, B. J., Slobodkin, A., Kato, C., et al. (2004). Shewanella profunda sp. nov., isolated from deep marine sediment of the nankai trough. Int. J. Syst. Evol. Microbiol. 54, 1943–1949. doi: 10.1099/ijs.0.03007-0
Toffin, L., Zink, K., Kato, C., Pignet, P., Bidault, A., Bienvenu, N., et al. (2005). Marinilactibacillus piezotolerans sp. nov., a novel marine lactic acid bacterium isolated from deep sub-seafloor sediment of the nankai trough. Int. J. Syst. Evol. Microbiol. 55, 345–351. doi: 10.1099/ijs.0.63236-0
Vallenet, D., Calteau, A., Cruveiller, S., Gachet, M., Lajus, A., Josso, A., et al. (2017). MicroScope in 2017: an expanding and evolving integrated resource for community expertise of microbial genomes. Nucleic Acids Res. 45, D517–D528. doi: 10.1093/nar/gkw1101
Westphal, L., Wiechmann, A., Baker, J., Minton, N. P., and Müller, V. (2018). The Rnf complex is an energy coupled transhydrogenase essential to reversibly link cellular NADH and ferredoxin pools in the acetogen Acetobacterium woodii. J. Bacteriol. 200:e357-18. doi: 10.1128/jb.00357-18
Keywords: Atlantis Massif, subseafloor, oceanic crust, serpentinization, anaerobic culture, hydrostatic pressure
Citation: Quéméneur M, Erauso G, Frouin E, Zeghal E, Vandecasteele C, Ollivier B, Tamburini C, Garel M, Ménez B and Postec A (2019) Hydrostatic Pressure Helps to Cultivate an Original Anaerobic Bacterium From the Atlantis Massif Subseafloor (IODP Expedition 357): Petrocella atlantisensis gen. nov. sp. nov. Front. Microbiol. 10:1497. doi: 10.3389/fmicb.2019.01497
Received: 22 February 2019; Accepted: 14 June 2019;
Published: 16 July 2019.
Edited by:
Isabelle Daniel, Université Claude Bernard Lyon 1, FranceReviewed by:
Ida Helene Steen, University of Bergen, NorwayLotta Purkamo, Geological Survey of Finland, Finland
Copyright © 2019 Quéméneur, Erauso, Frouin, Zeghal, Vandecasteele, Ollivier, Tamburini, Garel, Ménez and Postec. This is an open-access article distributed under the terms of the Creative Commons Attribution License (CC BY). The use, distribution or reproduction in other forums is permitted, provided the original author(s) and the copyright owner(s) are credited and that the original publication in this journal is cited, in accordance with accepted academic practice. No use, distribution or reproduction is permitted which does not comply with these terms.
*Correspondence: Anne Postec, YW5uZS5wb3N0ZWNAdW5pdi1hbXUuZnI=