- Department of Isotope Biogeochemistry, Helmholtz Centre for Environmental Research – UFZ, Leipzig, Germany
We determined 2H stable isotope fractionation at natural abundances associated with hydrogenase activity by whole cells of Desulfovibrio vulgaris strain Miyazaki F expressing a NiFe(Se) hydrogenase. Inhibition of sulfate reduction by molybdate inhibited the overall oxidation of hydrogen but still facilitated an equilibrium isotope exchange reaction with water. The theoretical equilibrium isotope exchange δ2H-values of the chemical exchange reaction were identical to the hydrogenase reaction, as confirmed using three isotopically different waters with δ2H-values of – 62, +461, and + 1533‰. Expected kinetic isotope fractionation of hydrogen oxidation by non-inhibited cells was also superimposed by an equilibrium isotope exchange. The isotope effects were solely catalyzed biotically as hydrogen isotope signatures did not change in control experiments without cells of D. vulgaris Miyazaki.
Introduction
Many microorganisms use hydrogen (H2) or protons (H+) as electron donors or acceptors, coupled to the oxidation or production of H2. The enzyme catalyzing H2 oxidation or production is a metalloenzyme termed hydrogenase, for which several differently structured isoenzymes are known (Vignais and Billoud, 2007; Greening et al., 2016). The most abundant and commonly studied type of hydrogenase contains a NiFe(Se)-active center (Vignais and Billoud, 2007). The reaction catalyzed by hydrogenases can be formulated as follows (Eq. 1).
Such a reaction usually leads to a kinetic isotope fractionation, which is defined as the ratio of the rate constants for light and heavy isotopes within the unidirectional reaction of H2 to two protons releasing two electrons. The isotope fractionation is a result of the slightly lower activation energy needed to cleave and form bonds of lighter isotopes compared to heavy isotopes in this (bio-) chemical reaction. The kinetic isotope fractionation leads to the predominant reaction of light isotopomers, which implies that the remaining fraction would get heavier during the reaction. For the oxidation of molecular hydrogen at natural abundances, this would result in accumulation of 2H (deuterium) in the remaining fraction. However, during the oxidation of H2 (Eq. 1), an isotopic exchange with water (Eq. 2) was observed simultaneously to the kinetic isotope effect (Arp and Burris, 1982; Vignais et al., 1997, 2002; Yang et al., 2012).
During the isotope exchange of gaseous hydrogen with water, the heavy isotopes of molecular hydrogen (2H) exchange with the light hydrogen isotopes (1H) of the water, until an equilibrium isotope value for molecular hydrogen is reached. This is an inverse isotope effect compared to kinetic isotope fractionation, where the H2 will become enriched in deuterium over time and thus, the δ2H-value will become more positive. If kinetic and equilibrium isotope fractionation take place in parallel, the oxidation and the isotope exchange reaction cannot be separated from each other. In order to understand overall isotope effects during H2-consumption, the equilibrium isotope fractionation of the isotopic exchange reaction must be studied separately. Therefore we designed experiments to study both H2-oxidation and isotope exchange with cell suspensions of Desulfovibrio vulgaris strain Miyazaki F, which expresses one of the best studied NiFe hydrogenases, as well as a NiFe(Se) suited for H2 oxidation (Yagi et al., 1976; Deckers et al., 1990; Ogata et al., 2002; Foerster et al., 2003; Fichtner et al., 2006; Pandelia et al., 2010; Nonaka et al., 2013; Riethausen et al., 2013). We hypothesized that the addition of molybdate will inhibit electron flow to sulfate (Peck, 1959; Wolin and Miller, 1980) and thus, only the isotopic exchange reaction would be observable.
The aim of our study was to analyze the equilibrium isotope effect of the isotope exchange reaction and the kinetic isotope effect of the unidirectional oxidation reaction of hydrogen, in order to eventually monitor hydrogenase activity in environmental samples and settings, e.g., during hydrogen underground storage. This method, based on natural abundant stable hydrogen isotopes of gaseous samples, would allow in situ assessment of hydrogenase activity without further treatment of samples.
Materials and Methods
Chemicals
All chemicals until otherwise stated were purchased from Merck Chemicals GmbH (Darmstadt, Germany). Deuterium-enriched waters were prepared by mixing 1 l sterilized tap water water (Merck Millipore, Germany) with either 250 μl or 100 μl 2H2O (99.9%; Merck Chemicals, Germany).
Culture and Cultivation Conditions
Desulfovibrio vulgaris strain Miyazaki F (DSM 19637) was obtained from the DSMZ (Deutsche Sammlung von Mikroorganismen und Zellkulturen, Braunschweig, Germany). The strain was grown in a mineral medium for sulfate-reducers, which consisted of NH4Cl (0.3 g/l), KH2PO4 (0.4 g/l), CaCl2 (0.075 g/l), Na2SO4 (2 g/l), MgSO4 ∗ 7 H2O (1 g/l), 1 ml trace element solution SL-10, 0.1 ml selenite-tungstate solution, 4 ml 50 % Na-DL-lactate, 2 ml vitamin solution, 10 ml 1 M NaHCO3 solution, 2 ml 1 M Na-acetate solution. 1 M L-cysteine solution was used for reduction. 0.1 mg/l resazurin was used as redox indicator. The selenite-tungstate solution contained per 100 ml: 0.5 g/l NaOH, 3 mg/l Na2SeO3 ∗ 5 H2O and 4 mg/l Na2WO4 ∗ 2 H2O. The vitamin solution contained per 100 ml: 1 mg biotin, 1 mg folic acid, 25 mg pyridoxine-HCl, 25 mg thiamine-HCl ∗ 2 H2O, 5 mg riboflavin, 25 mg nicotinic acid, 25 mg D-Ca-pantothenate, 0.5 mg vitamin B12, 25 mg p-aminobenzoic acid and 25 mg lipoic acid. All components, except the last four, were mixed in sterilized tap water (Merck Millipore, Germany) and purged with 75% N2 and 25% CO2 until they became virtually oxygen-free and were autoclaved subsequently. The remaining components were added within an anaerobic glovebox (Toepffer Lab Systems, Germany).
Experiments with cell suspensions were performed with cells pre-grown on 28 mM lactate and 22 mM sulfate at 30°C and 120 rpm on a Multitron incubation shaker (Infors, Germany). At optical densities above 0.2 absorbance with no further increase in cell density, 50 ml each were transferred into 120 ml serum bottles in the glovebox, which was filled with a mixture of N2 (97–98%) and H2 (2–3%) and crimped close with PTFE-coated chlorobutyl-isoprene septa (Thermo Fisher Scientific, Germany) before the start of the experiment.
Three sets of isotope fractionation experiments were designed with these cells: one set in which cells were inhibited by molybdate, one in which the headspace had been purged to reduce sulfide load, and one without further treatment. For the inhibited setups, six of the bottles each were treated with 20 mM molybdate. Therefore, 2 ml of a 0.5 M sodium molybdate solution was added to each bottle, yielding yellow-orange molybdo-sulfide-complexes (Wolin and Miller, 1980; Biswas et al., 2009). Both the purged and untreated setups consisted of five bottles of cell suspension each. The headspace in the purged setups was exchanged with N2 to eliminate H2S-burden in the headspace and reduce potential inhibition by sulfides.
In all samples 12 ml H2 were hereafter exchanged with the existing headspace with a syringe and an empty needle while bottles were tilted to remove headspace only. Bottles were kept at 30°C and 120 rpm (Multitron incubation shaker, Infors, Germany). For abiotic controls cell suspensions were inactivated by incubation at 80°C in a water bath for 20 min and subsequent addition of 0.3 ml 10 M NaOH, yielding pH 12.
All experimental setups were performed in two replicates using water at natural abundance (δ2H-H2O = -62‰) and water enriched in 2H by adding 100 μl 2H2O resulting in an isotope composition of δ2H-H2O = +461‰ One experiment with molybdate for inhibition of sulfate reduction was conducted with enriched water (250 μl 2H2O) to yield a final isotope composition of δ2H-H2O = + 1533‰. Bottles were sacrificed at different time points in a water bath at 80°C for 20 min and stored upside down after the addition of 10 M NaOH before hydrogen isotopes of H2 in the headspace were analyzed. The setup with enriched water (δ2H-H2O = + 1533‰) was sacrificed in different periods of time than the other experiments, in order to gain insight into the initial reaction. NaOH was added to terminate microbial activity and to remove CO2 from the headspace by precipitation of sodium bicarbonate. CO2-removal was necessary for subsequent isotope analysis, as the column used for gas chromatography (GC) would retain it. 100 to 300 μl sample volume were transferred via syringe into 1 ml 3% (w/v) Zn-acetate and stored at -20°C for downstream processing. Sulfides in the headspace of the bottles were eliminated due to precipitation as ZnS by the addition of 3 ml 3 (w/v) % Zn-acetate.
Analytical Methods
Sulfide Measurements
Sulfides were measured as previously described (Cline, 1969; Kleinsteuber et al., 2008) against a blank control. Previously frozen samples were thawed and mixed with 4 ml water and 0.4 ml of Cline’s solution. The mixtures were stored in the dark for 20 min before photometric measurement on an UV-1800 (Shimadzu, Germany) at 670 nm. Due to the high concentrations of sulfides, samples were diluted before measurement. Optical densities of cell suspensions were determined on an UV-1800 (Shimadzu, Germany) at 600 nm. 1 ml culture solution was transferred via syringe into a cuvette filled with a few mg of Na-dithionite, closed with parafilm and shaken until dissolved and then measured using water as control.
Isotope Measurement
Hydrogen isotope measurements were performed on a GC-isotope ratio mass spectrometer (IRMS) system (Supplementary Figure 1) (7890A, Agilent Technologies, Germany; GC-IsoLink II Thermo Fisher Scientific, Germany; Conflo IV Thermo Fisher Scientific, Germany; Finnigan MAT 253, Thermo Fisher Scientific, Germany) equipped with a J&W CP-Molsieve 5Å GC Column (50 m, 0.32 mm, 30 μm, Agilent Technologies, Germany). An empty ceramic tube was kept isothermal at 500°C inside the GC-IsoLink and the GC column was kept at 40°C during measurements. An additional cold trap operated with liquid nitrogen was installed after the GC to remove water to keep the water background within the ion source of the IRMS low. After 2 h of runtime, the column was heated to 250°C and held for at least 10 min remove residual water vapor and residual CO2 from the GC column.
0.2 ml headspace injections were made manually at split-ratio of 1:25 with a gas-tight syringe in an interval of roughly eight minutes needed for elution of H2 and permanent gases. The analytical system was evaluated for reproducibility and isotope artifacts. It was found to be reproducible and deliver true values within the uncertainty of 0.7 ± 0.4‰.
Both the normal and the δ2H-enriched waters were measured with an elemental analysis-chromium/high temperature conversion (EA-Cr/HTC)-IRMS system (HEKAtech, Germany) coupled via the Conflo IV (Thermo Fisher Scientific, Germany) to the same IRMS instrument (Gehre et al., 2017). All results are reported in the delta notation (Eq. 3) and according to the guidelines for stable isotope measurements (Coplen, 2011).
The ratio of heavy to light isotopes (Rsample) in a compound is reported in δ-notation; for hydrogen, samples will be compared to Vienna Standard Mean Ocean Water (VSMOW) as standard with an isotope ratio of 155.76 ± 0.1 ppm (Rstandard). A laboratory standard, made of 10% H2 in N2, with a δ2H-value of -205‰ was used for reference. The auto-protonation factor (H3+ factor) was determined daily and remained stable at 8.24 ± 0.05. Theoretical equilibrium isotope values, mainly dependent on temperature and the isotope signature of water, were calculated according to a formula previously described (Horibe and Craig, 1995).
Assessment of H2 Concentrations
The concentration of H2 was measured with the GC-IRMS. For concentration measurements a defined volume of 10% H2 was prepared using 120 ml serum bottles which were purged with N2 before. Twelve ml H2 were exchanged with the gaseous phase by a syringe and an empty needle while the bottle was held upside down. This sample was prepared daily and used for external calibration of the concentration measurement. Therefore the sample was injected three times at the start of each run, and all following samples were normalized to the average area under the curve of all controls in this measurement period. Intensities of external calibration were stable over 20 days, leading to overall variations in H2-concentration of ± 1.5%. Initial concentrations of H2 between setups and replicates varied about 5% of the response, probably dependent on handling speed and temperature in the laboratory during exchange of H2, as well as gas-tightness of the syringe used for injections.
Results
Measurement of Hydrogen Stable Isotopes
The isotope signatures of the different waters were determined to be δ2H-H2O = -62 ± 2‰, +461 ± 1‰, and +1533 ± 2‰, respectively. For the sterilized tap water in Leipzig (δ2H-H2O = -62‰), an equilibrium δ2H-value for H2 of – 744‰ was calculated. The enriched water setups (δ2H-H2O = + 461‰ and δ2H-H2O = + 1533‰) were calculated to equilibrate with a theoretical value for H2 of δ2H = -606‰ and δ2H = -317‰, respectively.
Control experiments with H2 in the headspace and sterilized tap water or culture medium showed no significant change in the isotopic signature over the experimental timeframe of 18 days (Figure 1), with stable δ2H-values of δ2H = -141.2‰ and δ2H = -142.2‰. These were identical to the isotope value of the pure H2 used (δ2H = -139‰), taking usual uncertainties into account. The δ2H signature of the hydrogen gas used in the anaerobic glove box was substantially lighter (approx. δ2H = -645‰) than the signature of the H2 gas used in the whole cell experiments, leading to a shift of Δ2H = 65‰ (Figure 1) when mixed.
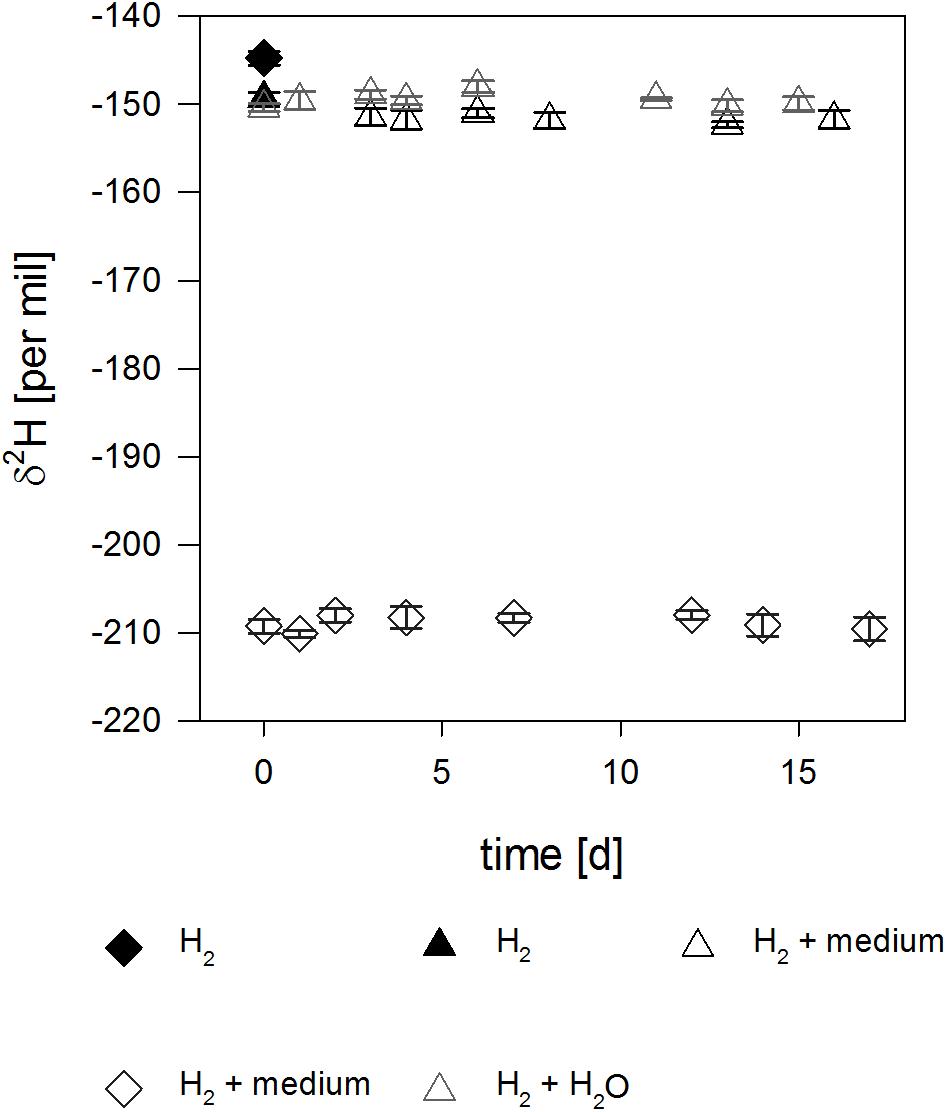
Figure 1. Isotope signal over time in the abiotic controls. Filled symbols correspond to pure H2 used for the experiment and empty symbols correspond to H2 in the headspace of serum bottles filled with either water (gray) or medium (black). Please note the depleted δ2H-values of H2 (empty diamonds) for the medium prepared in the anaerobe glovebox due to mixing of two isotopically different H2 sources (see text).
H2-Oxidation by Non-inhibited Cells
Experiments With Reduced Sulfide Concentration
To reduce concentrations of sulfide which may affect the metabolism of D. vulgaris, the headspace of culture bottles were purged with N2. The sulfide concentrations after purging were typically roughly 6 mM.
13.9 ± 0.7% and 12.2 ± 0.3% H2 were oxidized within 4 days (Table 1 and Figure 2D). Sulfide concentrations increased from 6.0 ± 2.2 mM and 6.4 ± 0.1 mM to 17.9 mM and 16.7 ± 0.2 mM, respectively, in 3 days (Figure 2A). Hydrogen isotope values changed slowly toward depletion in the beginning from δ2H = -309.0 ± 0.7‰ and δ2H = -163.2 ± 0.9‰, before the reaction gained speed (Table 2 and Figure 3), yielding δ2H = -678.6 ± 1.5‰ and δ2H = -475.3 ± 4.1‰ after 3 days.
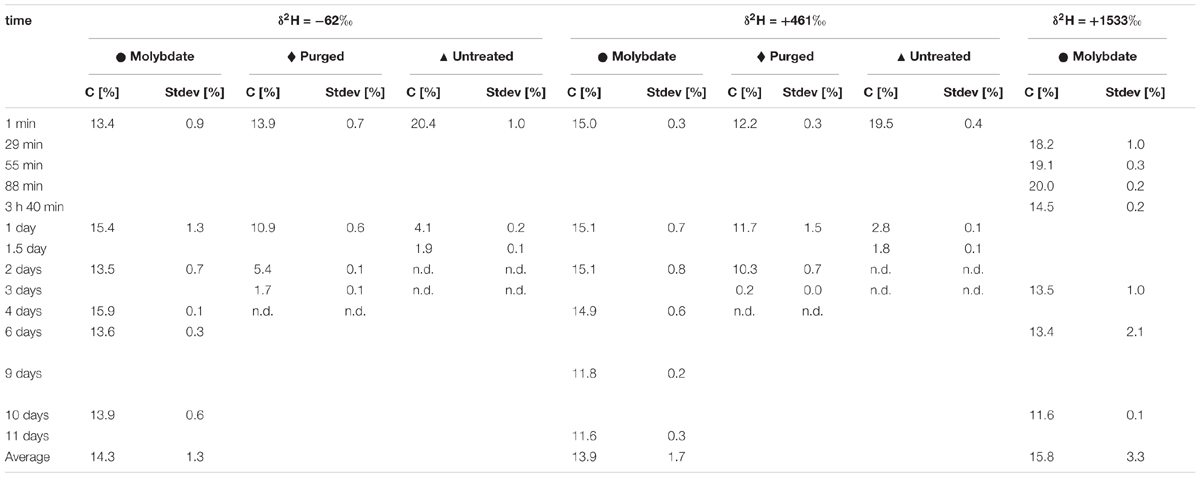
Table 1. Concentration of H2 in all experimental setups: molybdate-inhibited, headspace purged with N2 to reduce sulfides and without additional treatment.
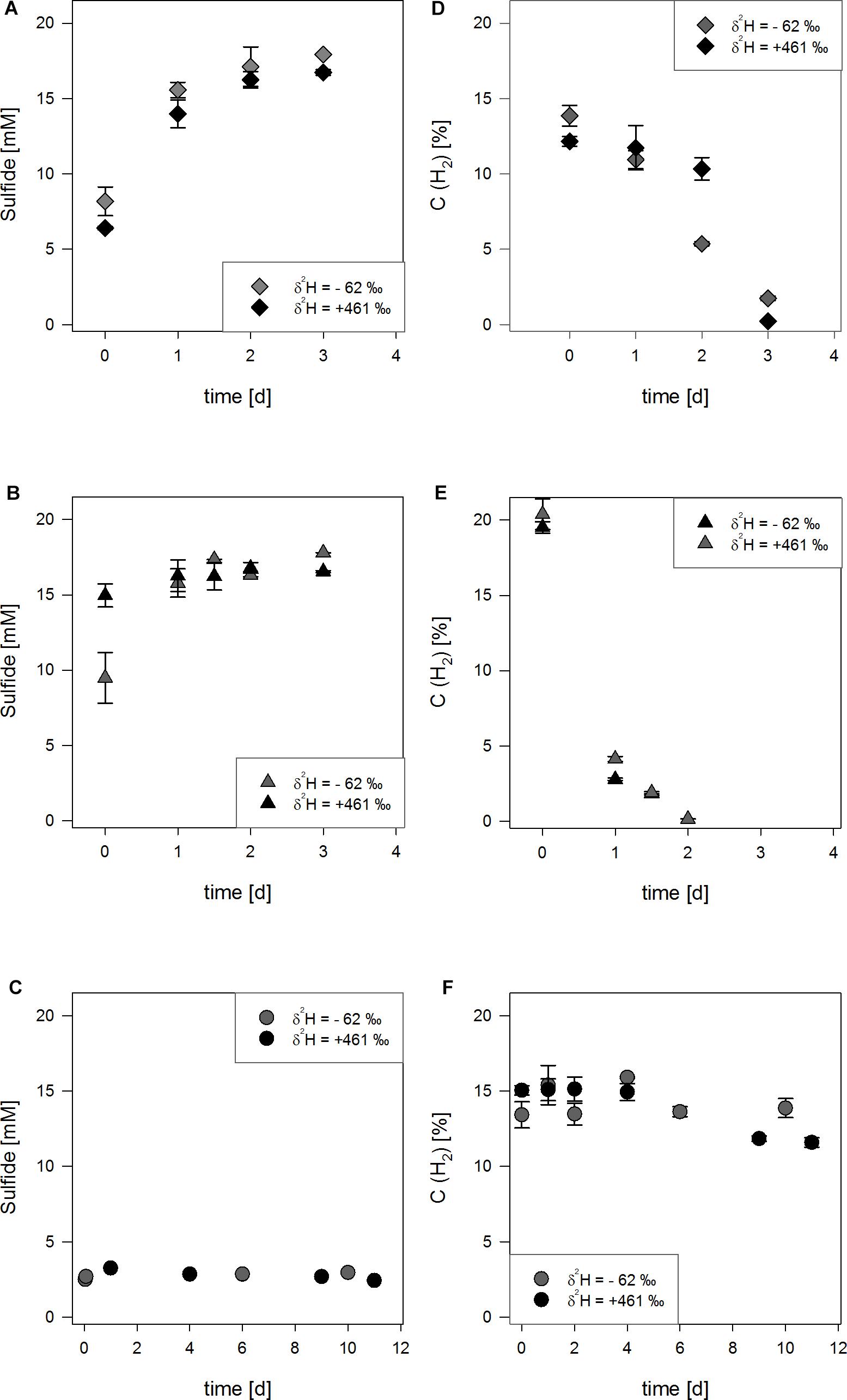
Figure 2. Concentration of H2 (empty symbols) and sulfides (filled symbols) for three experimental setups (A,D): setups with reduced sulfide burden, (B,E):
setups without further treatment, (C,F):
inhibition by molybdate) in either water with δ2H2O = -62‰ (gray) or with δ2H2O = +461‰ (black). The uncertainty of 2σ is shown.
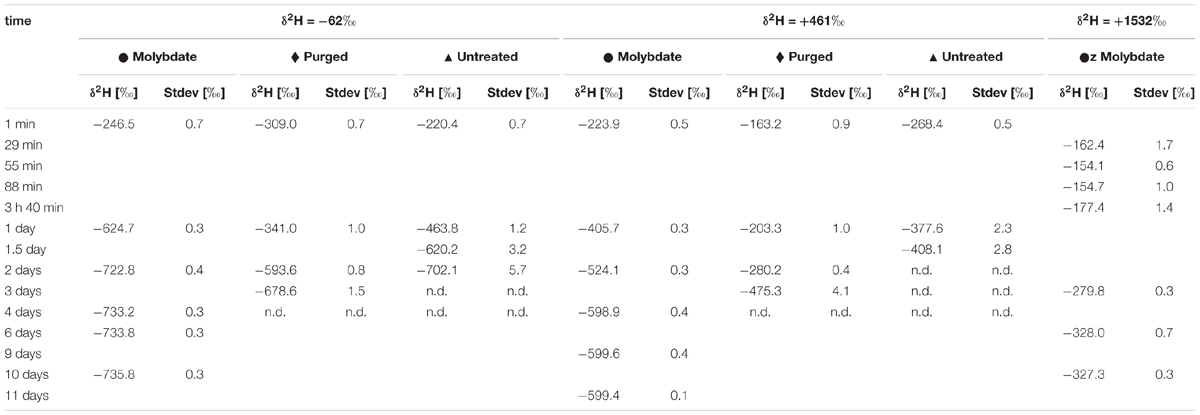
Table 2. δ2H-values of H2 in all experimental setups: molybdate-inhibited, headspace purged with N2 to reduce sulfides and without additional treatment.
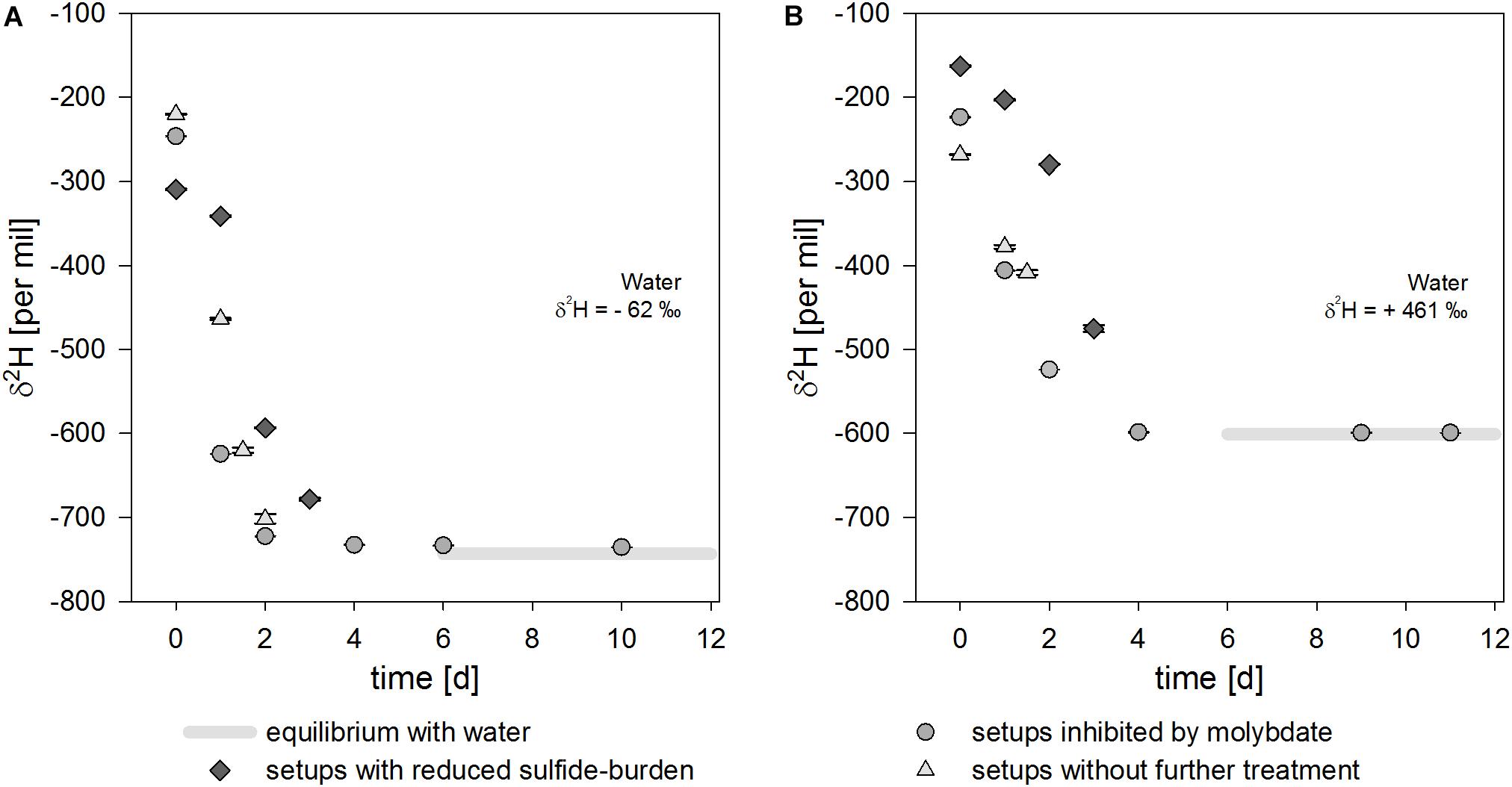
Figure 3. Isotope signal over time for three experimental setups in either water with δ2 H2O = –62‰ (A) or with δ2 H2O = +461‰ (B). The isotope equilibrium is reached after about 6 days and indicated by light gray bars. The uncertainty of 2σ is shown and often smaller than the symbol.
Experiments With High Sulfide Concentration
In the experiments without further treatment to reduce the amount of sulfides present, 20.4 ± 1.0% H2 and 19.5 ± 0.4% H2, respectively were consumed within 2 days in normal water (Table 1 and Figure 2E). 17.8 mM and 16.5 mM sulfides were produced starting from 9.48 ± 1.7 mM and 11.2 ± 0.6 mM until day three (Figure 2B). The δ2H of hydrogen in the bottles’ headspace was rapidly decreasing from δ2H = -220.4 ± 0.7‰ and δ2H = -268.4 ± 0.5‰ to δ2H = -702.1 ± 5.7‰ and δ2H = -408.1 ± 2.8‰ after 1.5 to 2 days (Table 2 and Figure 3). Concentrations at the last measureable time-points were 1.9 ± 0.1% and 1.8 ± 0.1% H2.
H2 Isotope Exchange by Cells Inhibited With Molybdate
Molybdate was used to inhibit the electron flow to the electron acceptor sulfate. In molydate-amended cultures, the H2 concentrations were almost constant with 14.3 ± 1.3%, 13.9 ± 1.7% and 15.8 ± 3.3% H2. Thus, no consumption of H2 in the molybdate setups was observed (Table 1 and Figure 2F). Furthermore, the sulfide concentrations did not increase and were stable between 2.5 to 3.5 mM (Figure 2C), indicating that sulfate reduction to sulfide was completely inhibited. In all experimental setups inhibited by molybdate, hydrogen isotopes in the headspace were depleting in deuterium starting from δ2H = -246.5 ± 0.7‰, δ2H = -223.9 ± 0.5‰, and δ2H = -162.4 ± 1.7‰ (Table 2) and stabilized at δ2H = -735.8 ± 0.4‰, δ2H = -599.4 ± 0.1‰, and δ2H = -327.3 ± 0.3‰ for the differently enriched waters after 6 days (Figure 3).
Discussion
No changes in the isotope signature of H2 for both culture medium and water in the abiotic controls could be observed, even though H2-concentrations decreased with continuous sampling of the same bottles (Supplementary Figure 2). Most studies on hydrogen isotope exchange with water used platinum or palladium as a catalyst and subsequent equilibration times of a few hours were reported at, e.g., 20°C (Crist and Dalin, 1934; Farkas and Farkas, 1934; Horiuti and Polanyi, 1934; Farkas, 1936; Suess, 1949; Rolston et al., 1976). It is therefore reasonable to assume that isotope exchange without a catalyst is too slow to be assessable in the experimental timeframe used in this study. Subsequently, it is unlikely that the isotope signal in environmental samples could be significantly affected by minerals, as catalysts are needed for accelerating the exchange reaction. But sampling itself might lead to bias, as it has been shown that use of steel can lead to the generation of molecular H2 from water, with which it is equilibrated (Chapelle et al., 1997).
Addition of gaseous hydrogen to water or culture medium leads to an immediate shift in the isotope signature from δ2H = -139.0 ± 0.8‰ to δ2H = -141.2 ± 0.3‰ for water and δ2H = -142.2 ± 0.7‰ for culture medium. This effect is probably due to a relatively higher solubility of 2H in water, which leads to an isotope fractionation (Muccitelli and Wen, 1978). The observed shift in isotopic signature lies within usual standard deviations for hydrogen isotope measurements, and no further change in the isotope signal was observed after the initial shift (Figure 1). Albeit negligible compared to biocatalysis, isotope exchange takes place in abiotic controls within 18 days at 30°C in the absence of a catalyst.
The addition of a cell suspension leads to changes in the isotope signature due to hydrogenase activity. An inverse isotope effect was observed in all three experimental setups. This is consistent with previously reported δ2H -values for H2-production from water for Shewanella oneidensis MR-1, which can express a NiFe- or a FeFe-hydrogenase (Kreuzer et al., 2014). Upon inhibition of dissimilatory sulfate reduction by molybdate, observable isotope effects should be limited to isotope exchange, since H2 was not consumed. Hydrogenases were previously described to facilitate isotope exchange of 2H2 and H2O or H2 and 2H2O (Hoberman and Rittenberg, 1943; Jouanneau et al., 1980; Arp and Burris, 1982; Vignais et al., 2000, 2002). We therefore assume that the hydrogenase in our experiments is solely responsible for the isotope exchange.
After four to 6 days the isotope exchange reaction approximately reached equilibrium with differences of Δ2H = 9‰ and Δ2H = 6‰ and Δ2H = 10‰ compared to the theoretical values (Figure 4) in cultures inhibited by molybdate addition. Deviations from the theoretical values could be due to the higher measurement error and standard deviations of the water measurements used for calculation, as well as the high sensitivity of the equilibrium equation toward fluctuations in temperature.
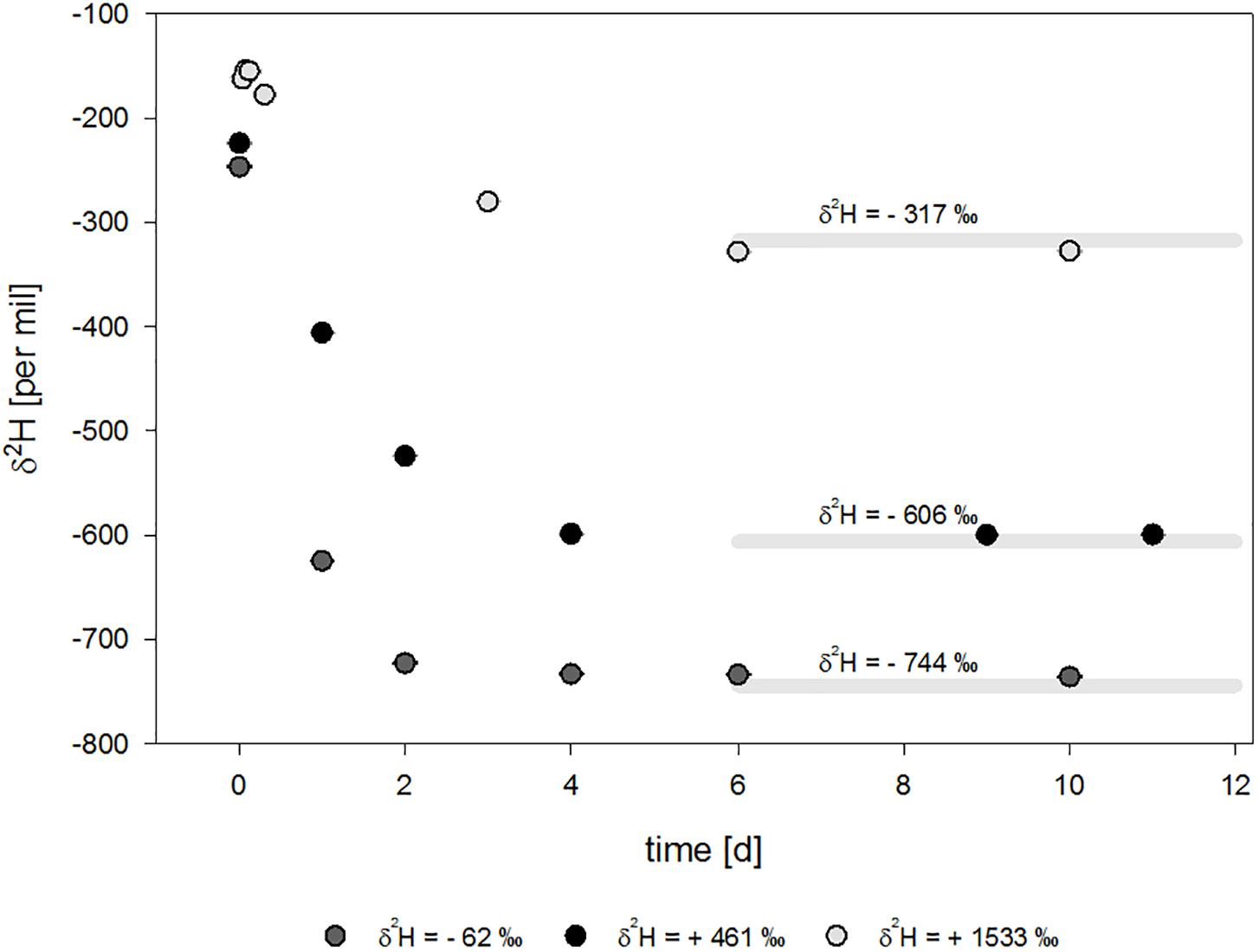
Figure 4. Isotope signature over time for molybdate-inhibited setups with water of different isotope composition. Theoretical equilibrium values ± approx. 5‰ standard deviation are indicated by light gray lines.
It has been shown for the NiFe-hydrogenase from D. vulgaris Miyazaki that hydrogen oxidation is a two-step process, during which an enzyme-hydride-state forms (Lubitz et al., 2014). First, H2 diffuses into the active center. Then, it is heterolytically cleaved, forming a proton and an enzyme-hydride-complex. Afterward, electrons and protons are shuffled out of the protein structure (Lubitz et al., 2014). A study using D. vulgaris Hildenborough suggested that H2 can be caged by the protein structure surrounding the active site when selenocysteine replaces cysteine in the active center of the protein structure (Gutiérrez-Sanz et al., 2013). In this case, substrate and products can accumulate within the protein structure of the NiFeSe-hydrogenase near the catalytic center and the two transfers of protons proceed faster than in NiFe-hydrogenases. The authors suggested that the accumulation and subsequent availability of substrate would correspond to a fast isotope exchange reaction (Gutiérrez-Sanz et al., 2013). This hypothesis connects protein structure and isotope effects. D. vulgaris Miyazaki expresses a structurally similar NiFeSe-hydrogenase best suited for hydrogen oxidation (Nonaka et al., 2013; Riethausen et al., 2013), and the corresponding fast isotope exchange reaction was measured in this study, where the isotope equilibrium was reached within 6 days. It might be possible to compare the equilibrium isotope effect of structurally different types of hydrogenases, such as NiFe- and FeFe-hydrogenases, in order to characterize the isotope exchange rate in future studies in more detail. For this, a different inhibition of electron flow might be needed, as molybdo-sulfide-complexes might not work for all microorganisms, as it specifically inhibits sulfate reduction. Even though it has been shown to also inhibit H2 production from glucose (Wolin and Miller, 1980), it has not been further or sufficiently studied. Information on structurally different hydrogenases is crucial for using stable hydrogen isotopes as a monitoring tool to track in situ hydrogenase activity, e.g., during storage of hydrogen in underground reservoirs.
Even though H2 was consumed in the experiment with purged headspaces, only minimal changes in the δ2H-values were observed in the beginning. During the substrate consumption, the hydrogen bond is cleaved, which is expected to result in a normal kinetic isotope effect. However, in our experiments, the isotope values approximate isotope equilibrium values with increasing time. We therefore suggest that the kinetic isotope effect of the hydrogen bond cleavage is superimposed by the equilibrium isotope exchange reaction. Only in the beginning of hydrogen oxidation, an effect of the kinetic isotope effect can be observed. Here, the equilibrium isotope effect has not yet completely superimposed the isotope signature, which results in seemingly stable isotope values.
The observed kinetic isotope fractionation effects could be due to shuffling of protons into the cell. Hydrogenase and cytochrome complexes are able to translocate protons (Ide et al., 1999; Dolla et al., 2000; Chang et al., 2004). For example, the reduction of sulfate needs two additional protons (Eq. 4).
During this process a slight isotope fractionation is expected, due to different diffusivity according to their molecular mass and tunneling effects in the hydrogenase structure (Cukier, 2004). Then, more 2H+ than 1H+ would be released from the hydrogenase, which would equivalent the expected kinetic isotope fractionation. This effect would counteract an equilibrium isotope exchange reaction. Not only the rate of equilibrium isotope exchange, but also the kinetic isotope fractionation could be affected by the protein structure. Therefore, further studies on both kinetic isotope effects and equilibrium isotope effects and their superimposition using structurally different hydrogenases are needed in order to use this concept as a monitoring or diagnostic tool.
Interestingly, cultures containing the highest sulfide concentrations tested in this study (10 mM) showed consumption of H2, but lacked the “isotopic lag phase” of the purged experiments with 6 mM starting concentration (Figure 3). This might be an indication that the amount of sulfides could affect the electron and proton flow, as H2 is still consumed but the normal kinetic isotope fractionation of the H-H bond cleavage is immediately superimposed by the equilibrium isotope effect of the exchange reaction. During growth on lactate and sulfate, up to 52% of electrons flow into the production of H2 and the remaining 48 % of electrons are coupled to sulfate reduction in D. vulgaris, yielding the potential to reduce approx. 8.9 mM sulfate from lactate and H2 (Noguera et al., 1998) or 14 mM sulfate from lactate alone before the start of the hydrogen oxidation experiments. And with concentrations of 9.48 up to 11.2 mM sulfide in the setups without further treatment to reduce sulfides at the start of the experiment, inhibition is a consequential hypothesis.
Conclusion
The hydrogenase of Desulfovibrio vulgaris Miyazaki facilitates an equilibrium isotope exchange when consumption of H2 is inhibited. Resulting δ2H-values of H2 corresponds to theoretical thermodynamic isotope equilibria. During H2, the kinetic isotope fractionation, which should be observed due to bond-cleavage, is superimposed by the equilibrium isotope exchange. These results might differ for other microorganisms and structurally different hydrogenases. Equilibrium isotope exchange in the experiments with starting concentration of about 10 mM sulfides also indicates a possibility that sulfides could inhibit electron flow. This research is fundamental in nature and aims to build a better understanding of the isotope effects and processes associated with hydrogenases. The results of this study serve as a basis for future research on a simple monitoring tool for environmental, gaseous samples based on stable isotopes of hydrogen.
Data Availability
All datasets generated for this study are included in the manuscript and/or the Supplementary Files.
Author Contributions
ML planned and performed the experiments and data analyses and wrote the manuscript. SK helped to establish a GC-IRMS method for H2. CV and H-HR supervised the research and edited the manuscript.
Funding
This work was supported by the Federal Ministry for Economic Affairs and Energy (BMWi) within the funding initiative “Energiespeicher,” project ANGUS II, Grant Number 03ET6122B.
Conflict of Interest Statement
The authors declare that the research was conducted in the absence of any commercial or financial relationships that could be construed as a potential conflict of interest.
Acknowledgments
We would like to acknowledge Florian Tschernikl for help in the cultivation and Matthias Gehre for helpful inputs during method development and work in the isotope lab.
Supplementary Material
The Supplementary Material for this article can be found online at: https://www.frontiersin.org/articles/10.3389/fmicb.2019.01545/full#supplementary-material
References
Arp, D. J., and Burris, R. H. (1982). Isotope exchange and discrimination by the H2-oxidizing hydrogenase from soybean root nodules. Biochim. Biophys. Acta Protein Struct. Mol. Enzymol. 700, 7–15. doi: 10.1016/0167-4838(82)90285-0
Biswas, K. C., Woodards, N. A., Xu, H., and Barton, L. L. (2009). Reduction of molybdate by sulfate-reducing bacteria. BioMetals 22, 131–139. doi: 10.1007/s10534-008-9198-8
Chang, C. J., Chang, M. C., Damrauer, N. H., and Nocera, D. G. (2004). Proton-coupled electron transfer: a unifying mechanism for biological charge transport, amino acid radical initiation and propagation, and bond making/breaking reactions of water and oxygen. Biochim. Biophys. Acta Bioenerget. 1655, 13–28. doi: 10.1016/j.bbabio.2003.08.010
Chapelle, F. H., Vroblesky, D. A., Woodward, J. C., and Lovley, D. R. (1997). Practical considerations for measuring hydrogen concentrations in groundwater. Environ. Sci. Technol. 31, 2873–2877. doi: 10.1021/es970085c
Cline, J. D. (1969). Spectrophotometric determination of hydrogen sulfide in natural waters 1. Limnol. Oceanogr. 14, 454–458. doi: 10.4319/lo.1969.14.3.0454
Coplen, T. B. (2011). Guidelines and recommended terms for expression of stable-isotope-ratio and gas-ratio measurement results. Rapid Commun. Mass Spectrom. 25, 2538–2560. doi: 10.1002/rcm.5129
Crist, R., and Dalin, G. (1934). “Isotopic Equilibria” in the Hydrogen-Hydrogen Oxide system. J. Chem. Phys. 2, 735–738. doi: 10.1063/1.1749388
Cukier, R. I. (2004). Theory and simulation of proton-coupled electron transfer, hydrogen-atom transfer, and proton translocation in proteins. Biochim. Biophys. Acta Bioenerget. 1655, 37–44. doi: 10.1016/j.bbabio.2003.06.011
Deckers, H. M., Wilson, F. R., and Voordouw, G. (1990). Cloning and sequencing of a [NiFe] hydrogenase operon from Desulfovibrio vulgaris Miyazaki F. Microbiology 136, 2021–2028. doi: 10.1099/00221287-136-10-2021
Dolla, A., Pohorelic, B. K. J., Voordouw, J. K., and Voordouw, G. (2000). Deletion of the hmc operon of Desulfovibrio vulgaris subsp. vulgaris Hildenborough hampers hydrogen metabolism and low-redox-potential niche establishment. Arch. Microbiol. 174, 143–151. doi: 10.1007/s002030000183
Farkas, A. (1936). The mechanism of the catalytic exchange reaction between deuterium and water. Trans. Faraday Soc. 32, 922–932.
Farkas, L., and Farkas, A. (1934). The equilibrium H 2 O+ HD = HDO+ H 2. Trans. Faraday Soc. 30, 1071–1079.
Fichtner, C., Laurich, C., Bothe, E., and Lubitz, W. (2006). Spectroelectrochemical characterization of the [NiFe] hydrogenase of Desulfovibrio vulgaris miyazaki F. Biochemistry 45, 9706–9716.
Foerster, S., Stein, M., Brecht, M., Ogata, H., Higuchi, Y., and Lubitz, W. (2003). Single crystal EPR studies of the reduced active site of [NiFe] Hydrogenase from Desulfovibrio vulgaris miyazaki F. J. Am. Chem. Soc. 125, 83–93. doi: 10.1021/ja027522u
Gehre, M., Renpenning, J., Geilmann, H., Qi, H., Coplen, T. B., Kümmel, S., et al. (2017). Optimization of on-line hydrogen stable isotope ratio measurements of halogen- and sulfur-bearing organic compounds using elemental analyzer–chromium/high-temperature conversion isotope ratio mass spectrometry (EA-Cr/HTC-IRMS). Rapid Commun. Mass Spectrom. 31, 475–484. doi: 10.1002/rcm.7810
Greening, C., Biswas, A., Carere, C. R., Jackson, C. J., Taylor, M. C., Stott, M. B., et al. (2016). Genomic and metagenomic surveys of hydrogenase distribution indicate H 2 is a widely utilised energy source for microbial growth and survival. ISME J. 10:761. doi: 10.1038/ismej.2015.153
Gutiérrez-Sanz, O., Marques, M. C., Baltazar, C. S., Fernández, V. M., Soares, C. M., Pereira, I. A., et al. (2013). Influence of the protein structure surrounding the active site on the catalytic activity of [NiFeSe] hydrogenases. J. Biol. Inorg. Chem. 18, 419–427. doi: 10.1007/s00775-013-0986-4
Hoberman, H. D., and Rittenberg, D. (1943). Biological catalysis of the exchange reaction between water and hydrogen. J. Biol. Chem. 147, 211–227.
Horibe, Y., and Craig, H. (1995). DH fractionation in the system methane-hydrogen-water. Geochim. Cosmochim. Acta 59, 5209–5217. doi: 10.1016/0016-7037(95)00391-6
Horiuti, I., and Polanyi, M. (1934). Exchange reactions of hydrogen on metallic catalysts. Trans. Faraday Soc. 30, 1164–1172.
Ide, T., Bäumer, S., and Deppenmeier, U. (1999). Energy conservation by the H2: heterodisulfide oxidoreductase from Methanosarcina mazei Gö1: identification of two proton-translocating segments. J. Bacteriol. 181, 4076–4080.
Jouanneau, Y., Kelley, B. C., Berlier, Y., Lespinat, P. A., and Vignais, P. M. (1980). Continuous monitoring, by mass spectrometry, of H2 production and recycling in Rhodopseudomonas capsulata. J. Bacteriol. 143, 628–636.
Kleinsteuber, S., Schleinitz, K. M., Breitfeld, J., Harms, H., Richnow, H. H., and Vogt, C. (2008). Molecular characterization of bacterial communities mineralizing benzene under sulfate-reducing conditions. FEMS Microbiol. Ecol. 66, 143–157. doi: 10.1111/j.1574-6941.2008.00536.x
Kreuzer, H. W., Hill, E. A., Moran, J. J., Bartholomew, R. A., Yang, H., and Hegg, E. L. (2014). Contributions of the [NiFe]-and [FeFe]-hydrogenase to H2 production in Shewanella oneidensis MR-1 as revealed by isotope ratio analysis of evolved H2. FEMS Microbiol. Lett. 352, 18–24. doi: 10.1111/1574-6968.12361
Lubitz, W., Ogata, H., Rüdiger, O., and Reijerse, E. (2014). Hydrogenases. Chem. Rev. 114, 4081–4148.
Muccitelli, J., and Wen, W.-Y. (1978). Solubilities of hydrogen and deuterium gases in water and their isotope fractionation factor. J. Solution Chem. 7, 257–267. doi: 10.1007/bf00644273
Noguera, D. R., Brusseau, G. A., Rittmann, B. E., and Stahl, D. A. (1998). A unified model describing the role of hydrogen in the growth of Desulfovibrio vulgaris under different environmental conditions. Biotechnol. Bioeng. 59, 732–746. doi: 10.1002/(sici)1097-0290(19980920)59:6<732::aid-bit10>3.3.co;2-2
Nonaka, K., Nguyen, N. T., Yoon, K.-S., and Ogo, S. (2013). Novel H2-oxidizing [NiFeSe]hydrogenase from Desulfovibrio vulgaris Miyazaki F. J. Biosci. Bioeng. 115, 366–371. doi: 10.1016/j.jbiosc.2012.10.011
Ogata, H., Mizoguchi, Y., Mizuno, N., Miki, K., Adachi, S.-I., Yasuoka, N., et al. (2002). Structural Studies of the Carbon Monoxide Complex of [NiFe]hydrogenase from Desulfovibrio vulgaris Miyazaki F: suggestion for the initial activation site for dihydrogen. J. Am. Chem. Soc. 124, 11628–11635. doi: 10.1021/ja012645k
Pandelia, M.-E., Ogata, H., Currell, L. J., Flores, M., and Lubitz, W. (2010). Inhibition of the [NiFe] hydrogenase from Desulfovibrio vulgaris Miyazaki F by carbon monoxide: An FTIR and EPR spectroscopic study. Biochim. Biophys. Acta Bioenerget. 1797, 304–313. doi: 10.1016/j.bbabio.2009.11.002
Peck, H. D. (1959). The ATP-dependent reduction of sulfate with hydrogen in extracts of Desulfovibrio desulfuricans. Proc. Natl. Acad. Sci. 45, 701–708. doi: 10.1073/pnas.45.5.701
Riethausen, J., Rüdiger, O., Gärtner, W., Lubitz, W., and Shafaat, H. S. (2013). Spectroscopic and electrochemical characterization of the [NiFeSe] Hydrogenase from Desulfovibrio vulgaris Miyazaki F: reversible redox behavior and interactions between electron transfer centers. ChemBioChem 14, 1714–1719. doi: 10.1002/cbic.201300120
Rolston, J., Den Hartog, J., and Butler, J. (1976). The deuterium isotope separation factor between hydrogen and liquid water. J. Phys. Chem. 80, 1064–1067. doi: 10.1021/j100551a008
Suess, H. E. (1949). Das Gleichgewicht H2 + HDO HD + H2O und die weiteren Austauschgleichgewichte im System H2, D2 und H2O. Z. Naturforsch. A 4, 328–332.
Vignais, P. M., and Billoud, B. (2007). Occurrence, classification, and biological function of hydrogenases: an overview. Chem. Rev. 107, 4206–4272. doi: 10.1021/cr050196r
Vignais, P. M., Cournac, L., Hatchikian, E. C., Elsen, S., Serebryakova, L., Zorin, N., et al. (2002). Continuous monitoring of the activation and activity of [NiFe]-hydrogenases by membrane-inlet mass spectrometry. Int. J. Hydrogen Energy 27, 1441–1448. doi: 10.1016/s0360-3199(02)00114-3
Vignais, P. M., Dimon, B., Zorin, N. A., Colbeau, A., and Elsen, S. (1997). HupUV proteins of Rhodobacter capsulatus can bind H2: evidence from the H-D exchange reaction. J. Bacteriol. 179, 290–292. doi: 10.1128/jb.179.1.290-292.1997
Vignais, P. M., Dimon, B., Zorin, N. A., Tomiyama, M., and Colbeau, A. (2000). Characterization of the hydrogen-deuterium exchange activities of the energy-transducing HupSL hydrogenase and H2-signaling HupUV hydrogenase in Rhodobacter capsulatus. J. Bacteriol. 182, 5997–6004. doi: 10.1128/jb.182.21.5997-6004.2000
Wolin, M., and Miller, T. L. (1980). Molybdate and sulfide inhibit H2 and increase formate production from glucose by Ruminococcus albus. Arch. Microbiol. 124, 137–142. doi: 10.1007/bf00427718
Yagi, T., Kimura, K., Daidoji, H., Sakai, F., Tamura, S., and Inokuchi, H. (1976). Properties of purified hydrogenase from the particulate fraction of Desulfovibrio vulgaris, Miyazaki. J. Biochem. 79, 661–671. doi: 10.1093/oxfordjournals.jbchem.a131111
Keywords: hydrogenase, D. vulgaris strain Miyazaki, monitoring, GC-IRMS, equilibrium isotope fractionation, kinetic isotope fractionation, hydrogen isotopes
Citation: Löffler M, Kümmel S, Vogt C and Richnow H-H (2019) H2 Kinetic Isotope Fractionation Superimposed by Equilibrium Isotope Fractionation During Hydrogenase Activity of D. vulgaris Strain Miyazaki. Front. Microbiol. 10:1545. doi: 10.3389/fmicb.2019.01545
Received: 30 April 2019; Accepted: 20 June 2019;
Published: 10 July 2019.
Edited by:
Eric Boyd, Montana State University, United StatesReviewed by:
Wolfgang Buckel, University of Marburg, GermanyChristopher L. Hemme, University of Rhode Island, United States
Copyright © 2019 Löffler, Kümmel, Vogt and Richnow. This is an open-access article distributed under the terms of the Creative Commons Attribution License (CC BY). The use, distribution or reproduction in other forums is permitted, provided the original author(s) and the copyright owner(s) are credited and that the original publication in this journal is cited, in accordance with accepted academic practice. No use, distribution or reproduction is permitted which does not comply with these terms.
*Correspondence: Carsten Vogt, Y2Fyc3Rlbi52b2d0QHVmei5kZQ==