- 1Child Health Division, Menzies School of Health Research, Charles Darwin University, Darwin, NT, Australia
- 2GeneCology Research Centre, University of the Sunshine Coast, Sippy Downs, QLD, Australia
- 3Department of Respiratory and Sleep Medicine, Children’s Health Queensland, Queensland University of Technology, Brisbane, QLD, Australia
Non-typeable Haemophilus influenzae (NTHi), an opportunistic pathogen of the upper airways of healthy children, can infect the lower airways, driving chronic lung disease. However, the molecular basis underpinning NTHi transition from a commensal to a pathogen is not clearly understood. Here, we performed comparative genomic and transcriptomic analyses of 12 paired, isogenic NTHi strains, isolated from the nasopharynx (NP) and bronchoalveolar lavage (BAL) of 11 children with chronic lung disease, to identify convergent molecular signatures associated with lung adaptation. Comparative genomic analyses of the 12 NP-BAL pairs demonstrated that five were genetically identical, with the remaining seven differing by only 1 to 3 mutations. Within-patient transcriptomic analyses identified between 2 and 58 differentially expressed genes in 8 of the 12 NP-BAL pairs, including pairs with no observable genomic changes. Whilst no convergence was observed at the gene level, functional enrichment analysis revealed significant under-representation of differentially expressed genes belonging to Coenzyme metabolism, Function unknown, Translation, ribosomal structure, and biogenesis Cluster of Orthologous Groups categories. In contrast, Carbohydrate transport and metabolism, Cell motility and secretion, Intracellular trafficking and secretion, and Energy production categories were over-represented. This observed trend amongst genetically unrelated NTHi strains provides evidence of convergent transcriptional adaptation of NTHi to pediatric airways that deserves further exploration. Understanding the pathoadaptative mechanisms that NTHi employs to infect and persist in the lower pediatric airways is essential for devising targeted diagnostics and treatments aimed at minimizing disease severity, and ultimately, preventing NTHi lung infections and subsequent chronic lung disease in children.
Introduction
Non-typeable Haemophilus influenzae (NTHi) is a Gram-negative bacterium that frequently colonizes the respiratory tract mucosa of humans. NTHi is part of the upper airway flora and is found in 20–50% of healthy children and 20–30% of healthy adults (Slack, 2015). The upper airways may act as a reservoir for seeding the lung environment (Fothergill et al., 2014), where NTHi switches to an opportunistic pathogenic lifestyle, a transition driven by multiple, poorly understood factors (Duell et al., 2016).
NTHi facilitates the colonization of other bacterial species in the lower airways (Van Eldere et al., 2014) and is associated with exacerbations in several respiratory diseases (Duell et al., 2016). Indeed, its presence in the lower airways is associated with increased risk of future development of bronchiectasis (Wurzel et al., 2016). In children with chronic suppurative lung disease (CSLD) or bronchiectasis, NTHi is the most commonly detected bacterium in the lower airways (Byun et al., 2017). In adults, the bacterium can chronically and repeatedly colonize the lungs of people with chronic obstructive pulmonary disease (COPD), bronchiectasis, or COPD with bronchiectasis (Martinez-Garcia et al., 2011; Sriram et al., 2018), resulting in increased airway inflammation (Tufvesson et al., 2015). NTHi also has the capacity to form biofilms (Murphy and Kirkham, 2002; Swords, 2012) resulting in a decreased susceptibility to antibiotics and increased inflammatory and defense responses in the host (Starner et al., 2006). Unsurprisingly, NTHi is a pathogen with increasing public health recognition (Van Eldere et al., 2014; Slack, 2017).
NTHi has evolved several adaptive mechanisms that enable the bacterium to survive and persist in the human host, including modification of its genome via homologous recombination and phase variation (Erwin et al., 2005; Power et al., 2012). Modulation of gene expression through phase variation enables NTHi to rapidly adapt to and colonize new environments (Wong and Akerley, 2012). Analysis of the NTHi genome has identified several phase-variable virulence factors involved in persistence, nutrient uptake, cellular adherence, and host immune response evasion (Pettigrew et al., 2018), with direct modulation of gene expression in response to environmental stimuli and host milieu (Baddal et al., 2015). NTHi is naturally competent and can undergo high rates of recombination, resulting in greater genetic and phenotypic diversity than serotypeable H. influenzae (Connor et al., 2012); however, unlike other chronic respiratory infections (Smith et al., 2006; Price et al., 2013), no significant gene loss or gain has yet been observed in chronic H. influenzae infections (Pettigrew et al., 2018).
Identifying signals of NTHi adaptation to the lung would aid in unmasking the mechanisms facilitating pathogenesis, which is critical for improving diagnosis, treatment and prevention of NTHi-associated lower airway diseases. However, the mechanisms involved in the transition from a commensal state in the nasopharynx (NP) to a pathogenic lifestyle in the lower airways is not well understood (De Chiara et al., 2014; Pettigrew et al., 2018). In this study, we investigated genome- and transcriptome-wide differences between 12 isogenic NTHi pairs obtained from the NP and lower airways (via bronchoalveolar lavage; BAL) of patients with bronchiectasis or CSLD. We hypothesized that NTHi isolated from the airways of patients with lung disease would exhibit characteristic genetic or transcriptional profiles that signaled a change from a commensal to a pathogen.
Materials and Methods
Isolate Collection
NP swabs and BAL specimens were collected concurrently from Australian children (mean, 3.2 years; range: 1.1–13.9 years) diagnosed with bronchiectasis or CSLD (Table 1) at participating hospitals in the Northern Territory and Queensland (Hare et al., 2010). All children had an active lower airway infection, as defined by > 104 colony-forming units of respiratory bacteria/mL BAL fluid (Hare et al., 2010). Following collection, specimens were transported on ice in 1 mL skim milk, tryptone, glucose and glycerol broth (STGGB) (Gibson and Khoury, 1986; Hare et al., 2010) and stored at −80°C before processing. All subsequent cultures were also stored in STGGB at −80°C. Following selective culture on chocolate blood agar (CBA) supplemented with bacitracin, vancomycin, and clindamycin (Oxoid, Scoresby, VIC, Australia), NTHi was isolated and confirmed as previously described (Hare et al., 2010; Price et al., 2017). Antibiotic sensitivity was determined by disk diffusion as per the Calibrated Dichotomous Susceptibility (CDS) testing guidelines (8th Edition) (Bell et al., 2016) for ampicillin, amoxicillin-clavulanate, cefaclor, ceftriaxone, chloramphenicol, tetracycline, and azithromycin.
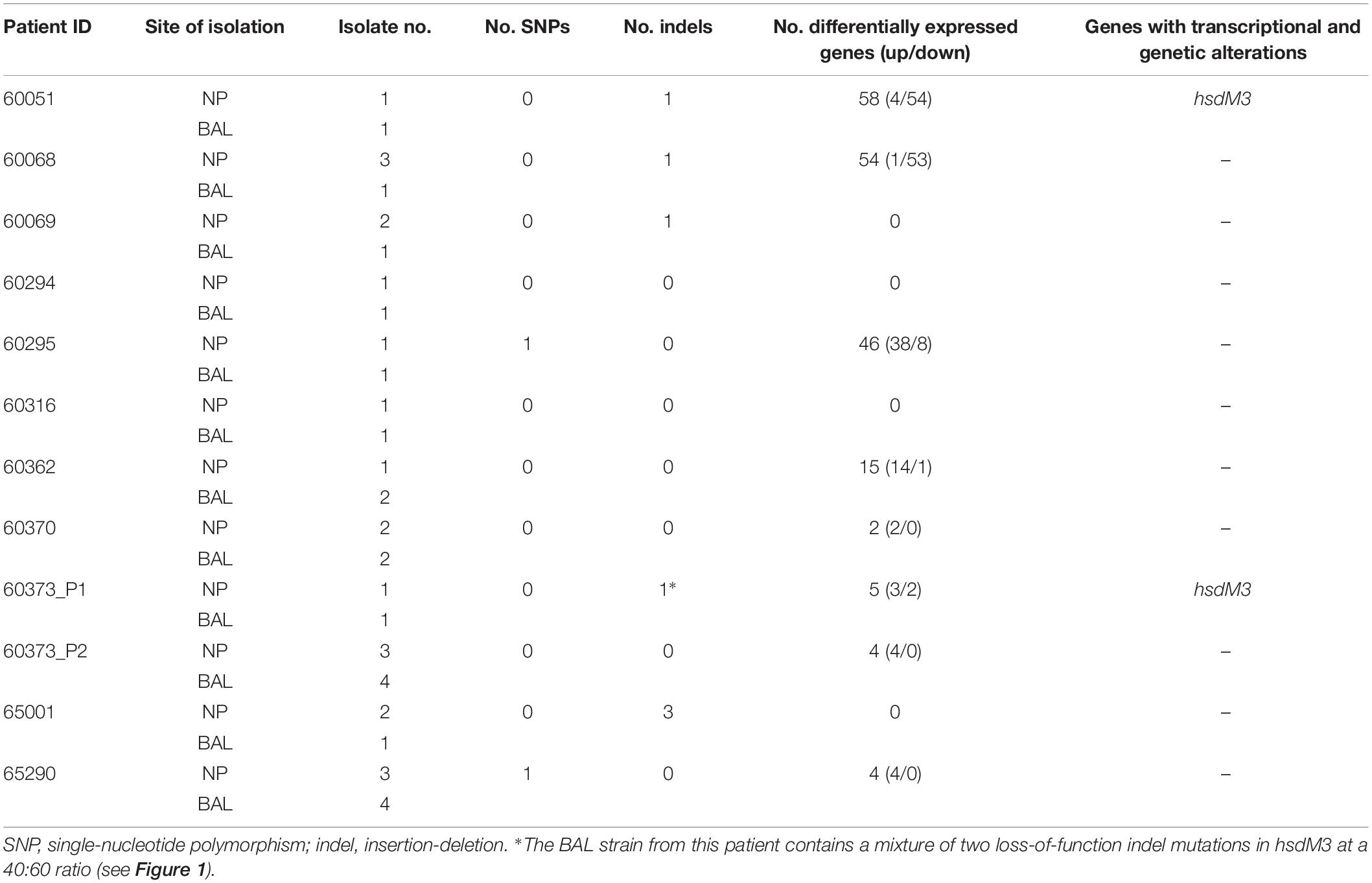
Table 1. Summary of genomic and transcriptomic differences between paired, isogenic NTHi isolates obtained from the nasopharynx (NP) and lower airways (bronchoalveolar lavage; BAL) of 11 Australian children with chronic lung disease.
Whole-Genome Sequencing and Comparative Genomics
DNA extraction was performed as previously described (Price et al., 2015). Whole-genome sequencing (WGS) was carried out at the Australian Genome Research Facility (Parkville, VIC, Australia) using the Illumina (San Diego, CA, United States) HiSeq 2500 platform. As a criterion for our study, all isolate pairs were required to be highly genetically similar (i.e., isogenic). To identify isogenic NP-BAL pairs from each patient, multilocus sequence typing (MLST) (Jolley and Maiden, 2010) followed by phylogenetic analysis was performed. Further details on isolate selection is described in Supplementary Methods. Based on this analysis, we selected 12 paired NP-BAL isolates from 11 patients for further investigation; two genetically distinct NP-BAL pairs were selected from one patient (designated 60373_P1 and 60373_P2).
To catalog the mutations separating each NP-BAL pair, SPANDx v3.2 (default settings) (Sarovich and Price, 2014) was used. Where possible, genetic variants were also confirmed using transcriptomic (RNA-seq) data. For each analysis, the NP isolate draft assembly was used as the reference for variant calling. Further details are provided in Supplementary Methods.
NTHi Liquid Media Growth Conditions for RNA Harvest
Due to the reliance of NTHi growth on the presence of X (haemin/haematin) and V (nicotinamide-adenine-dinucleotide) blood factors (Holt, 1962), we initially attempted to culture isolates in brain heart infusion (BHI) broth supplemented with Haemophilus Test Medium (HTM; Oxoid), and subsequently, a HTM-supplemented artificial sputum medium formulated to mimic the mucosal environment in the airways of cystic fibrosis patients (Fung et al., 2010; Price et al., 2017). However, due to inconsistent optical density (OD) readings and poor growth or flocculation of some or all isolates in these media, we investigated an alternative liquid medium for the growth of NTHi for RNA-seq (see Supplementary Methods for further details). CBA is widely used for culturing NTHi and other fastidious bacteria (Artman et al., 1983). Thus, we used this medium as the basis for formulating liquid chocolate medium (LCM). Formulation of LCM and growth conditions are provided in Supplementary Methods.
RNA Extraction
All isolates were cultured in LCM and in duplicate to account for any technical replicability issues. RNA was extracted using TRIzol® as described in Supplementary Methods. After DNA digest, absence was verified using real-time PCR assays that detect H. influenzae DNA (Aziz et al., 2017) and defibrinated horse blood DNA (Humair et al., 2007). For further details see Supplementary Methods.
Differential Expression (DE) Analysis
RNA-seq processing and DE analysis was performed as described in Supplementary Methods. Briefly, RNA-seq was carried out using the Illumina HiSeq 2500 platform. In total, 24 isolates were sequenced in duplicate, generating 48 transcriptomes.
To identify molecular signatures of lung adaptation on a within-patient basis, DE analysis was first conducted by comparing BAL with NP isolates from individual patients. Subsequently, convergence analysis was performed by comparing BAL with NP isolates from all 12 isolate pairs. The following additional analyses with different sample sets were performed to ensure robustness: (a) those NP-BAL pairs with DE detected in the within-patient analysis (eight in total), and (b) the three NP-BAL pairs with > 45 DE genes (60051, 60068, and 60295). For all convergence analyses, the two NP-BAL pairs from patient 60373 were treated either as independent NP-BAL pairs or grouped as a single patient. DE was conducted using edgeR (v3.18.1) (Robinson et al., 2010), implemented in R (v3.4.1) (R Core Team, 2014). For all analyses, DE was determined using the quasi-likelihood method (glmQLFit function).
Genes with significant DE were annotated using Clusters of Orthologous Groups (COG) (Galperin et al., 2015), with categories retrieved from a previous study (Santana et al., 2014) with minor corrections (Supplementary Table S1). For genes with multiple COG categories, all assigned categories were included in the analysis. To assess for significant enrichment of functional groups, COG categories of the reference genome 86-028NP were compared with those identified in the within-patient DE analyses. Statistical assessment comparing the proportion of categories was performed in R using a two-tailed Fisher’s exact test with a false discovery rate corrected p-value of ≤ 0.05.
Results
Comparative Genomic Analysis of Paired Isolates
To assess diversity and to identify potential clustering of NP or BAL isolates, a maximum parsimony phylogenetic tree was constructed using 124,262 biallelic, core-genome SNPs identified among the paired isolates and a global NTHi isolate set comprising 157 strains (Supplementary Figure S1). Phylogenomic analysis confirmed that pairs from individual patients clustered closely together yet were distinct from other NP-BAL pairs. Consistent with previous studies (De Chiara et al., 2014; Price et al., 2015; Pettigrew et al., 2018), there was no evidence of NP- or BAL-specific clades. Isolate pairs were identical sequence types (STs), with 5 of the 12 pairs possessing novel STs (ST-1906 to ST-1910; Supplementary Table S2). Patient 60373 had two distinct isolate pairs (NP-BAL pairs 60373_P1 and 60373_P2) that were separated by > 17,000 SNPs, demonstrating the presence of at least two NTHi lineages colonizing this patient. This finding was also reflected in the MLST data, with 60373_P1 isolates belonging to ST-1910 whereas 60373_P2 isolates were ST-139 (Supplementary Table S2). Antibiotics are routinely used to treat exacerbation events in pediatric chronic lung disease, and resistance to clinically relevant antibiotics can arise during such infections. In the current work, no NTHi isolate exhibited resistance toward any of the tested antibiotics, ruling out antibiotic resistance-driven mutations arising between isolate pairs.
Next, within-patient comparative genomic analyses were performed to identify variants separating the paired NP-BAL isolates. Pairs were highly genetically similar, with a maximum of three mutations observed between any one pair (Table 1). In total, two non-synonymous SNPs and seven indels (six in open-reading frames) were identified among seven NP-BAL pairs, with the remaining five NP-BAL pairs being genetically identical (Table 2). No copy-number variants, large deletions or inversions were observed between any of the pairs. One non-synonymous SNP occurred in the exogenous haem acquisition-encoding gene, hgpB (HgpBArg407Lys; 60295 BAL Hi1), and the second affected the outer membrane protein assembly factor, bamA (BamAGlu508Gly; 65290 NP Hi3). Of the seven indels, four were simple sequence repeats (SSRs) that were predicted to result in frameshift mutations, leading to truncated proteins in phase-variable genes (HsdM3Glu9fs, HsdM3Glu12fs, HgpBThr35fs, and Lic3ASer18fs in 60051 BAL Hi1, 60373_P1 BAL Hi1, 60068 BAL Hi1, and 65001 BAL Hi1, respectively). The remaining three indels were in: ompP2 (OmpP2Ala269_Gly270insValGlyAla; 65001 BAL Hi1), hsdS2 (HsdS2Thr173_Leu176del; 65001 BAL Hi1), and an intergenic region 62 bp upstream of hxuC (60069 BAL Hi1). Notably, hsdM3 (in 60051 and 60373_P1) and hgpB (in 60068 and 60295) were mutated in isolates from two patients. When compared with 86-028NP, all mutations were shown to deleteriously affect the BAL isolates (Table 2).
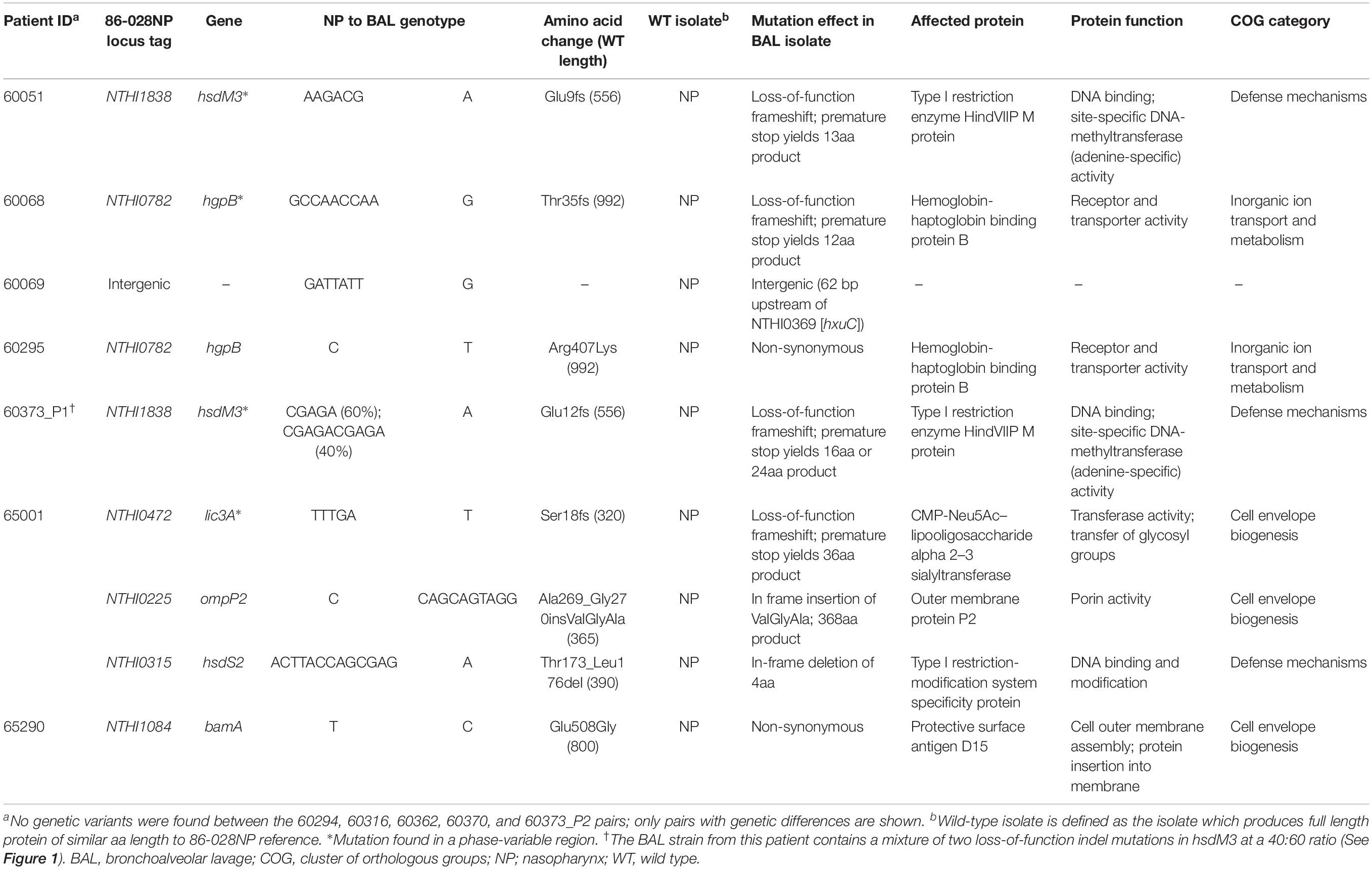
Table 2. Summary of genetic variants identified between 12 paired, isogenic non-typeable Haemophilus influenzae isolates retrieved from the nasopharynx vs. lower airways (bronchoalveolar lavage; BAL).
The hsdM3 indels in both the 60051 and 60373_P1 BAL isolates occurred in the same pentanucleotide SSR tract, which comprises variable copy number of a “CGAGA” motif at the 5′ end of this gene. Phase variation in hsdM3 is driven by changes in this highly mutable SSR tract and is influenced by a lack of adenine methylation (Zaleski et al., 2005). A 5 bp AGACG deletion in this SSR tract in 60051 BAL Hi1 (Figures 1A,C) is predicted to produce a truncated protein of just 13 residues. Similarly, a 5 bp CGAGA deletion was observed in 60373_P1 BAL Hi1; however, it was only supported by ∼60% of aligned reads. Upon closer inspection, a 10 bp deletion (CGAGACGAGA) was present in the remaining ∼40% of aligned reads (Figure 1B). This mixture of hsdM3 alleles is supported by the RNA-seq reads. The 5 bp (∼60%) and 10 bp (∼40%) components were both predicted to produce a truncated protein of 16 and 24 residues, respectively (Figure 1C). Given that isolates were likely subjected to a genetic bottleneck prior to sequencing due to growth on artificial media after isolation, these mixtures suggest that the hsdM3 SSR undergoes a rapid mutation rate, with mutations potentially occurring during laboratory passage.
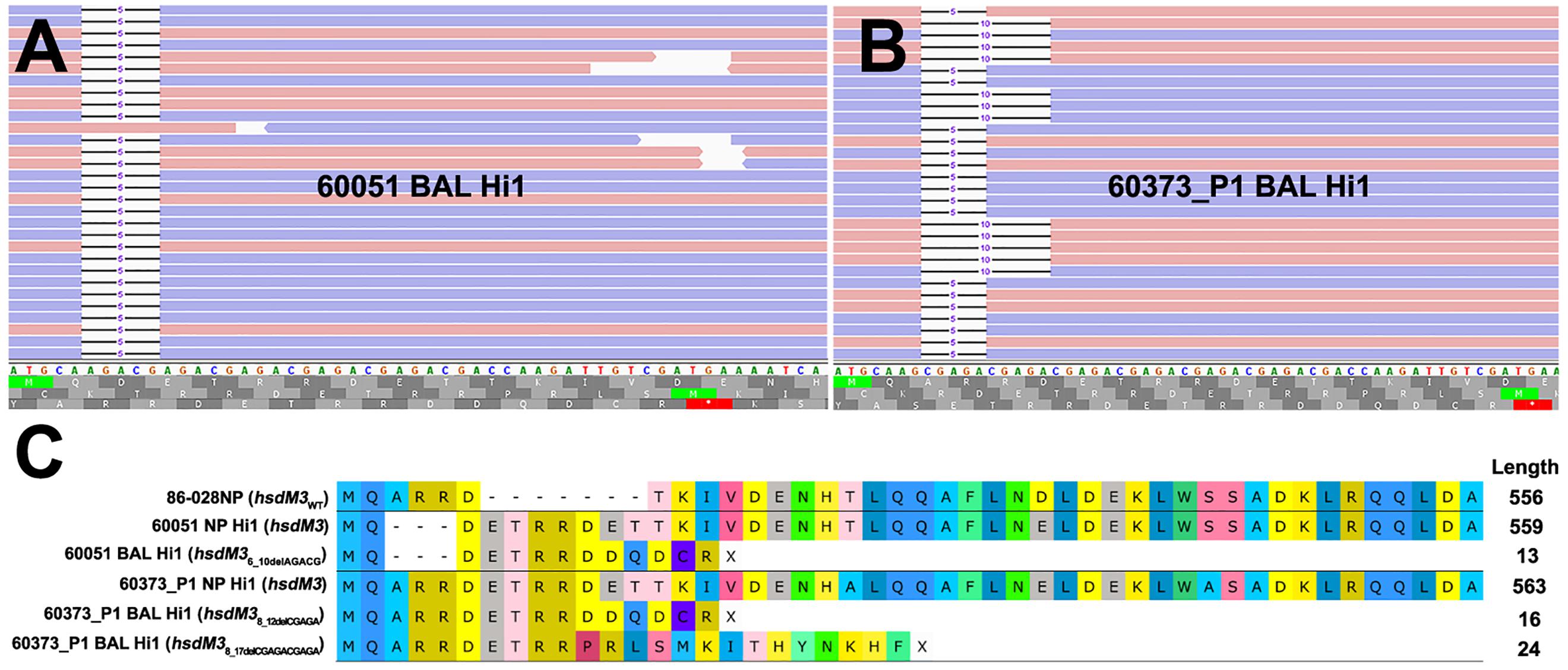
Figure 1. Read mapping analysis of the highly mutable hsdM3 pentanucleotide simple sequence repeat (SSR) tract in 60051 and 60373_P1 NP-BAL pairs. (A) Graphical representation of 60051 BAL Hi1 Illumina reads aligned against the hdsM3 region of the 60051 NP Hi1 reference assembly. One allele consisting of a 5 bp deletion (AGACG) was observed. (B) Graphical representation of 60373_P1 BAL Hi1 Illumina reads aligned against the 60373_P1 NP Hi1 reference assembly. Two different alleles consisting of 5 bp (AGACG) and 10 bp (AGACGx2) deletions were observed in the BAL isolate. Blue and red bars represent forward and reverse reads (respectively). (C) Amino acid alignment of HsdM3 against reference strain 86-028NP (wild-type). NP isolates from 60051 and 60373_P1 encode the full-length protein, with minor differences resulting from the variable SSR tract. Indels in the same SSR tract of both BAL isolates, including the two alleles observed in 60373_P1, result in a loss-of-function frameshift that is predicted to yield a truncated protein of 13-24aa.
Liquid Chocolate Medium for Reproducible NTHi Growth
As NTHi has an absolute growth requirement for X and V blood factors (Holt, 1962), commonly used culture media for this fastidious bacterium typically includes lysed blood in a nutrient-rich agar base (e.g., CBA) (Artman et al., 1983), or BHI base supplemented with HTM (Coleman et al., 2003). However, we found uniform and reproducible growth of NTHi in HTM-supplemented BHI problematic; variable growth rates were observed both among isolates and between technical replicates, indicating inconsistent bacterial growth. Additionally, the microbial load was insufficient for RNA-seq, even after 24 h incubation. We also observed inconsistent OD readings between replicates and a tendency toward flocculation in certain strains. Similar issues were observed with HTM-supplemented artificial sputum medium, which is designed to mimic cystic fibrosis sputum (Fung et al., 2010; Supplementary Figure S2A).
To overcome these issues, we developed liquid chocolate medium (LCM), a medium that is straightforward to formulate, inexpensive and produces high-quantity NTHi cellular density for downstream analysis. LCM yielded NTHi cultures with reproducible growth, consistent growth rates, little to no flocculation, and sufficient microbial load to support sequencing of late-log phase RNA. LCM was thus used to determine the growth curves of four isolates to identify an appropriate culture duration for RNA extraction. A 7.5-h post-inoculation time point was found to represent late-log phase growth in all four cultures tested (Supplementary Figure S2B). This time point, which was chosen to reflect non-logarithmic bacterial growth rates in vivo (Yang et al., 2008; Kasi et al., 2017), was subsequently used for RNA extraction of all isolates.
Within-Patient DE and Comparison With Genetic Mutations
Culture and RNA extraction of 24 isolates was performed in duplicate. On average, 89.2% (87.1–92.0%) of sequence reads aligned uniquely to the 86-028NP reference genome, and of these, an average of 70% (68.2–74.8%) were assigned to known genomic features (Supplementary Table S2). Due to this high mapping percentage, within-patient DE analysis was performed using 86-028NP as the reference genome. This approach also greatly simplified comparisons among patients. DE was observed between eight NP-BAL pairs, ranging from 2 to 58 DE genes per pair. Three pairs (60051, 60068, and 60295) had > 45 DE genes, five pairs (60362, 60370, 60373_P1, 60373_P2, and 65290) had 15 or fewer DE genes (Supplementary Dataset S1), and the remaining four pairs (60069, 60294, 60316, and 65001) did not exhibit any DE.
In total, 189 DE genes, representing a wide functional spectrum, were detected across the eight NP-BAL pairs. Eight genes were DE in three pairs (comE, comM, glgC, ligA, pilB, pilC, smf and tnaA), 39 were DE in two pairs (arcC, argF, asnA, comABCDF, dmsC, glgABPX, glpK, hitA, hsdMS3, licBC, mao2, nagAB, nanAEK, NTHI0229, NTHI0232, NTHI0235, NTHI0646, NTHI0647, NTHI1183, NTHI1243, nrfCD, pckA, pilA, sdaA, siaT, and tnaB), and the remaining 87 were DE in single NP-BAL pairs (Figure 2 and Supplementary Dataset S1). However, only ten genes exhibited concordant downregulation (mao2, NTHI0646, NTHI0647, pckA, sdaA) or upregulation (asnA, hitA, hsdMS3, hsdS3, and tnaB) in the BAL isolates. In all other DE genes, discordance in the directionality of expression was observed, including the eight shared DE genes in three NP-BAL pairs (Figure 2). Additionally, genes in the 60295 pair were predominantly upregulated, whereas genes were predominantly downregulated in pairs 60051 and 60068. Overall, there was little overlap amongst patients.
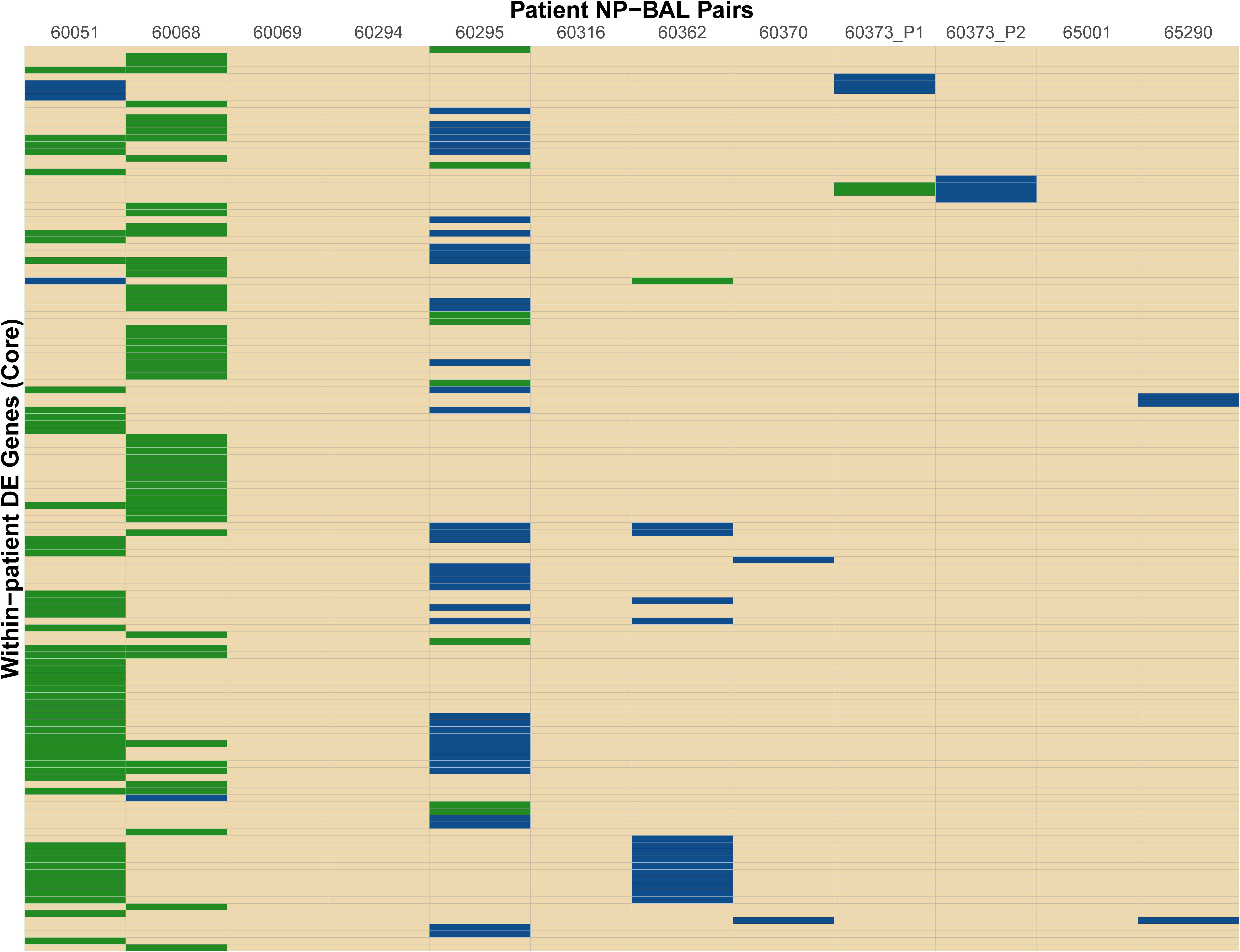
Figure 2. Heat map of differentially expressed (DE) genes identified between the NP-BAL pairs from 11 pediatric chronic lung disease patients. In total 134 non-redundant DE genes were detected across eight NP-BAL pairs. DE was observed in eight NP-BAL pairs; the remaining four pairs contained no DE. Genes were predominantly upregulated in the 60295 NP-BAL pair but predominately downregulated in the 60051 and 60068 NP-BAL pairs. The direction of log2 fold change of DE genes in BAL is color-coded as follows: upregulation (blue), downregulation (green), no DE (tan).
There was also minimal correlation between the number of genetic mutations and the number of DE genes detected between NP-BAL pairs (Table 1). For example, the 60295 NP-BAL pair exhibited 46 DE genes, but only a single non-synonymous SNP (HgpBArg407Lys) was observed in the BAL isolate. In contrast, no DE was detected between the 65001 NP-BAL pair (Supplementary Dataset S1), despite three indels being identified in the BAL isolate, including a loss-of-function frameshift in the phase-variable sialyltransferase gene, lic3A, which truncates the Lic3A protein from 320 to just 36 residues (Table 2). Two NP-BAL pairs (60294 and 60316) contained no mutations and no DE genes. Conversely, two NP-BAL pairs (60051 and 60373_P1) contained both genetic and transcriptional alterations affecting a single phase-variable gene, hsdM3 (Table 2 and Supplementary Dataset S1). For both pairs, hsdM3 and hsdS3 were upregulated in the BAL isolates, with an average log2 fold change of 2.5 and 4.6, respectively. Additionally, hsdR3 was upregulated by 2.2-fold in 60373_P1. However, both BAL isolates from 60051 and 60373_P1 encode truncated HsdM proteins of 13 and 16–24 residues, respectively (Figure 1C), indicating that hsdM3 upregulation in these isolates is unlikely to have a functional consequence. Notably, the two isolates with mutations in hgpB, 60068 BAL Hi1 and 60295 BAL Hi1, both had a relatively high amount of DE, with 55 and 46 DE genes, respectively.
DE Convergence and Functional Enrichment Analysis of NP-BAL Isolates
We compared BAL with NP isolates from all patients to identify genes with convergent gene expression. However, no single convergent genetic mutations or DE genes were identified, suggesting more complex pathways involved in lung adaptation. Therefore, we conducted a functional enrichment analysis of the 134 non-redundant DE genes using COG assignments to identify enriched categories. Statistical analysis identified 4/21 COG categories that were significantly over-represented in the functional enrichment analysis (Carbohydrate transport and metabolism, Energy production and conversion, Intracellular trafficking and secretion, and Cell motility, and secretion), and 3/21 categories (Translation, ribosomal structure and biogenesis, Coenzyme metabolism, and Function unknown) that were under-represented (p ≤ 0.05, Figure 3). Of note, the Function unknown category encompasses 20.2% genes in the 86-028NP genome, yet DE was only observed in 3.5% of these genes. The basis for this under-representation may partly reflect an artificially high number of gene annotations in the NTHi genome. In support of this notion, 47/397 (11.8%) genes assigned to the Function unknown category exhibited zero reads across our 48 transcriptomes (Supplementary Dataset S2, gray shaded rows), representing 37.9% of all genes with zero reads. Approximately 51.8% of the other genes with zero reads corresponded with paralogous ribosomal and transfer RNA species (Supplementary Dataset S2).
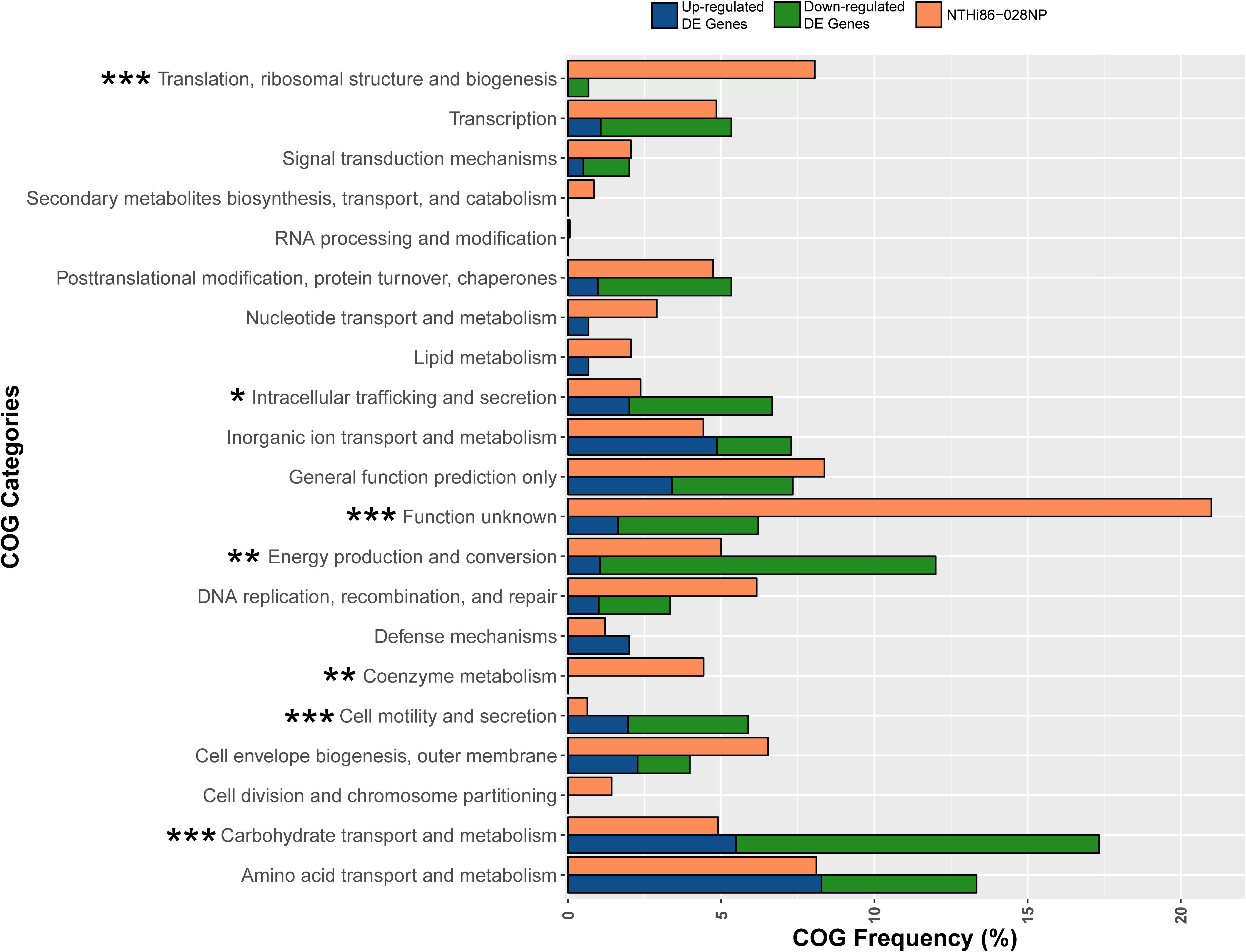
Figure 3. Clusters of Orthologous Group (COG) analysis comparing the frequency of categories in the 86-028NP genome with all DE genes in the 12 NP-BAL pairs. Seven COG categories were significantly enriched in DE genes from BAL-derived isolates, comprising four over-represented categories (Carbohydrate transport and metabolism, Cell motility and secretion, Energy production and conversion and Intracellular trafficking, and secretion) that were majority downregulated and three under-represented categories (Coenzyme metabolism, Function unknown, and Translation, ribosomal structure and biogenesis). ∗∗∗p < 0.001, ∗∗p < 0.01, *p < 0.05.
The most significant over-represented COGs (p < 0.001) were Carbohydrate transport and metabolism, which is represented by 4.9% of 86-028NP genes, yet 17.3% of DE genes belonged to this category, and Cell motility and secretion, with only 0.6% genes in 86-028NP but with 5.8% of DE genes belonging to this COG. The most significant under-represented COGs were Translation, ribosomal structure and biogenesis, comprising 8.0% of 86-028NP genes but only 0.6% of the DE genes, and Function unknown, which comprises 20.2% of 86-028NP genes yet only 6.2% of DE genes belonged to this category (Figure 3 and Supplementary Dataset S1). Other significant COG categories (p < 0.01) were Energy production and conversion (5.0% of 86-028NP genes but 12.0% DE genes) and Coenzyme metabolism (4.4% 86-028NP genes but 0% DE genes).
Discussion
Non-typeable H. influenzae is a pathogen of emerging public health importance (Erwin and Smith, 2007; Van Eldere et al., 2014). Conventionally considered a commensal of the upper airways, it is now recognized that NTHi is associated with increased severity and progression of multiple polymicrobial diseases of the lower airways such as COPD, bronchiectasis, and CSLD (Murphy, 2003; Purcell et al., 2014). In this study, we aimed to identify signals of NTHi pathoadaptation to the lower airways using 12 paired isogenic strains retrieved from the upper (NP) and the lower (BAL fluid) airways of 11 pediatric patients with CLSD or bronchiectasis. Previous studies have employed WGS to document the genome-wide changes leading to chronic adaptation and persistence of longitudinal NTHi isolates retrieved from COPD sputum in adults (Moleres et al., 2018; Pettigrew et al., 2018). The current work expands on these prior studies by examining both the genomic and transcriptomic profiles of NTHi adaptation in children’s airways. To ensure isolates were retrieved from the lower airways, we obtained NTHi isolates from the lower airways (BAL fluid) rather than sputum, the latter of which is fraught with upper respiratory tract contamination issues (Grønseth et al., 2017).
As part of our study criteria, only isogenic strains were selected to simplify the identification of convergent pathoadaptative mechanisms. As expected, comparative genomic analyses found minimal genetic differences between all isogenic NP-BAL pairs, with only nine total variants in our dataset, ranging from zero to three variants per pair. Five pairs were genetically identical, with the remaining seven encoding a total of two SNPs and seven indels. Notably, four of these indels occurred in tandem repeat regions, or SSR tracts, of phase-variable genes, which are abundant in the NTHi genome (Gilsdorf et al., 2004; Pettigrew et al., 2018). SSRs provide a rapid mechanism for gene expression modulation and adaptation (Vasu and Nagaraja, 2013; De Ste Croix et al., 2017). Two SSR indels occurred in the hypervariable Type I restriction-modification (RM) system methyltransferase gene, hsdM3. The remaining SSR indels occurred in the hemoglobin-haptoglobin binding protein B-encoding gene hgpB, which is involved in NP colonization and iron acquisition (Poole et al., 2013), and lic3A, which encodes a lipooligosaccharide (LOS) sialyltransferase that facilitates NTHi colonization in different anatomical sites (Hosking et al., 1999; Hood et al., 2001).
Our genetic findings concur with the recent longitudinal studies, which found that phase variation in lic3A, hsdM3, and hgpB are common in NTHi isolates retrieved from COPD airways (Moleres et al., 2018; Pettigrew et al., 2018). In addition to the hgpB SSR variant in 60068 BAL Hi1 that truncates the protein from 992 to 12 residues, a non-synonymous SNP (HgpBArg407Lys) was seen in 60295 BAL Hi1. Likewise, the SSR region of hsdM3 was deleteriously mutated in the 60051 and 60373_P1 BAL isolates, yielding truncated proteins of ≤ 24 residues (Figure 1C). The hsdM3 gene is part of a Type I RM system that acts as a defense system against foreign DNA invasion by, e.g., bacteriophages (Murray, 2000), fine-tuning of competence through variable methylation patterns, and in the methylation of self-DNA that may alter gene expression (Vasu and Nagaraja, 2013). While the hdsM3 mutations in the pediatric BAL isolates would render their corresponding Type I RM systems non-functional, the phase-variable nature of hsdM3 enables rapid reverse switching back to an active state. Others have shown that phase-variable RM systems allow NTHi to rapidly generate genomic and phenotypic diversity, which may enhance survival and persistence, especially in new environments (Zaleski et al., 2005; Atack et al., 2015). Taken together, the commonality of SSR tract mutants affecting hgpB, hsdM3, and lic3A in airway-derived isolates demonstrates that phase variation of these loci is driven by strong diversifying selection, with this variation being key in the long-term persistence of NTHi within the hostile lung environment.
Outer membrane porin (OmpP1 and OmpP2) mutations are also common in COPD-derived NTHi isolates. Previous studies have identified null ompP1 mutations in ∼33% of COPD isolates, and in-frame ompP2 mutations (i.e., missense SNPs and indels) in ∼20% of COPD isolates (Moleres et al., 2018; Pettigrew et al., 2018). Similarly, we identified an in-frame ompP2 mutation in the 65001 BAL Hi1 isolate that occurred in the extracellular loop six motif (Sikkema and Murphy, 1992) and increased OmpP2 length by three amino acids. However, we did not observe any genomic or transcriptomic ompP1 variation between our 12 NP and BAL pairs. Although our study is cross-sectional in nature and our sample size is modest, this result suggests that ompP1 variants may be much less common in pediatric NTHi lung-adapted strains than COPD lung-adapted strains. Biochemical profiles in COPD airways (e.g., fatty acids with bactericidal activity against NTHi wild-type strains) (Moleres et al., 2018) are potentially different to those present in pediatric bronchiectasis or CSLD airways, which may explain the greater selective pressure for OmpP1 inactivation in COPD airways. Further work is needed to document the prevalence of ompP1 mutations in a larger pediatric lung isolate cohort to examine whether diseased pediatric airways do in fact impart different OmpP1 pressures on NTHi compared with isolates from COPD airways.
Across the 12 within-patient DE analyses, a total of 134 non-redundant genes (range: 0–58) were DE between NP-BAL pairs, with no correlation between the number of mutations and the number of DE genes. Indeed, NP-BAL pairs 60362, 60370 and 60373_P2 were devoid of mutations, yet DE was observed in 15, 2, and 4 genes, respectively. Likewise, 65001 BAL Hi1 encoded mutations in lic3A, ompP2, and a Type I RM system specificity gene, hsdS2, yet no DE was detected in this strain. This lack of correlation suggests that DE between isolate pairs is influenced by epigenetic factors [e.g., altered DNA methylation (Fox et al., 2007)], or by unidentified genetic mutations due to inherent limitations with short-read sequencing approaches (e.g., insertion sequence-mediated rearrangements or variants in paralogs). Future studies employing methylation signature detection and long-read sequencing will enable a deeper understanding of the basis for DE in these strains.
We did not identify any DE gene(s) common to all isolates, indicating no universal expression profile associated with lung adaptation (i.e., the BAL isolates). However, some degree of gene convergence was observed, with eight DE genes common to three isolate pairs, and 39 common to two pairs (Supplementary Dataset S1). Of these, the com and pil operons, required for the biogenesis and function of the type IV pilus, were DE in three pairs. The type IV pilus is involved in multiple biological roles including adherence to epithelial cells, competence, twitching motility, biofilm formation and long-term NP colonization (Bakaletz et al., 2005; Jurcisek et al., 2007). The pathway responsible for uptake, transport and incorporation of N-Acetylneuraminic acid (Neu5Ac) into LOS (Apicella, 2012; Wong and Akerley, 2012), was DE in two pairs. Incorporation of Neu5Ac into the LOS enhances immune evasion and increases resistance to killing by human serum (Mandrell et al., 1992). Most DE genes (n = 87) occurred in single pairs, suggesting multiple, parallel strategies toward lower airway adaptation. In addition, the directionality of DE was inconsistent, with gene upregulation in some pairs and downregulation in others (Figure 2). Lack of a convergent transcriptional signal across all strains may be due to sampling biases, for example, the inclusion of BAL isolates that have yet to adapt to the lower airways, the re-seeding of the NP environment with lung-adapted strains, or inherent differences in NP carriage time prior to lower airway infection. Greater breadth and depth of strains is needed to determine whether a convergent signal of lung adaptation can be identified in the NTHi transcriptome. Additionally, sampling of other disease sites (e.g., middle ear) may help to resolve NTHi adaptation strategies in the lung environment.
To identify parallel signatures of adaptation to the lung environment on a broader scale, we categorized the functions of within-patient DE genes based on COG categories. Overall, strains isolated from the lower airways showed significant enrichment of DE genes in 7/21 COG categories. Four categories were over-represented in the DE gene dataset when compared with their prevalence in the 86-028NP genome, with Carbohydrate transport and metabolism, Cell motility and secretion, Intracellular trafficking and secretion, and Energy production and conversion being significant (p < 0.05 or p < 0.001). In comparison, the COG categories Translation, ribosomal structure and biogenesis, Function unknown and Coenzyme metabolism were significantly under-represented (p < 0.01 or p < 0.001) in the DE gene dataset, suggesting that altered expression of these gene categories is negatively associated with lung adaptation. However, analysis of the 48 transcriptomes revealed that 11.8% genes in the Function unknown category had zero reads mapping to the 86-028NP genome (Supplementary Dataset S2, gray-shaded rows), suggesting that many of these so-called genes likely do not produce messenger RNA or proteins under any growth conditions, and may represent incorrect gene annotations. Further RNA-seq studies of NTHi cultures grown under different conditions will help to resolve these potential annotation issues. Another noteworthy observation was that Carbohydrate transport and metabolism and Energy production and conversion collectively contained ∼35% of all DE genes and were primarily downregulated. This observation is consistent with Baddal et al. (2015), who observed the downregulation of genes involved in NTHi central metabolism in response to environmental stimuli. Intracellular trafficking and secretion and Cell motility and secretion were also over-represented in the BAL isolates and predominately downregulated (Figure 3 and Supplementary Dataset S1). Taken together, these results suggest that NTHi alters certain functional pathways in response to the lower airway environment, suggesting a degree of predictability in adapting to this new niche. Although further work is needed to consolidate these findings, our work sets the stage for identifying key functional pathways that may be exploited for targeted treatment and eradication of this pathogen.
Our study chose not to assess the wider polymicrobial community of the lower airways, as accurate transcriptional characterization of entire bacterial communities remains bioinformatically challenging (Lim et al., 2013). We instead chose to focus on an in-depth genomic and transcriptional analysis of NTHi, a species known to play a crucial role in the progression and severity of lung disease (Erwin and Smith, 2007; Van Eldere et al., 2014; Slack, 2017). Genetically diverse NTHi isolates from different patients were selected to enable identification of convergent adaptation strategies to the lung environment of pediatric patients. Due to the necessary passaging of strains to ensure purity and to obtain sufficient RNA material for sequencing, we cannot discount that these procedures may have influenced the NTHi expression profiles so that they no longer represent their in vivo counterparts. However, as previously demonstrated with other bacterial species, isolates appear to maintain their expression profiles even after microbiological handling and culturing in vitro (Viberg et al., 2017). For future studies, we recommend maximizing the number of strains isolated from individual patients together with longitudinal sampling to better understand pathogen adaptation over time.
In conclusion, our study provides new insights into NTHi adaptation to the lower airways in pediatric chronic lung diseases. Through genomic and transcriptomic characterization of 12 paired NTHi isolates from the NP and the lung, we found that NTHi employs several avenues of pathoadaptation that enables this pathogen to persist in the lower airways. Although we did not identify evidence of convergent pathoadaptive mechanisms at the single-gene level, our study identified parallel DE at the functional level, suggesting that NTHi adaptation to the pediatric airways is a complex but ultimately predictable process. The next steps require characterizing larger isolate panels using genomic and transcriptomic methods, particularly the elucidation of within-host NTHi population diversity from NP and lower airway sites across multiple patients, to better understand the pathoadaptive mechanisms that enable NTHi to persist and cause disease. Such findings will be crucial for the informed development of effective therapeutic interventions to prevent NTHi-driven chronic lung diseases.
Data Availability
The datasets generated for this study can be found in the NCBI database under the BioProject PRJNA484075. Genome assemblies for the 24 NTHi strains can be found in the NCBI GenBank (QWLW00000000-QWMT00000000), and Illumina WGS and RNA-seq data are available on the Sequence Read Database (SRR7719256-SRR7719303).
Ethics Statement
This study was carried out in accordance with the recommendations of the National Health and Medical Research Council’s (NHMRC) National Statement on Ethical Conduct in Human Research (2007) with written informed consent from all subjects. All subjects gave written informed consent in accordance with the Declaration of Helsinki. The protocol was approved by the Human Research Ethics Committee of the Northern Territory Department of Health and Menzies School of Health Research, with the approval numbers HREC 07/63 and 07/85.
Author Contributions
AC conducted the patient recruitment and specimen collection. EN and JB conducted the specimen processing, genotyping, and DNA extractions. AA, JB, HS-V, EP, and TH conducted the isolate selection. AA conducted the culturing, RNA extraction, and bioinformatics analysis with supervision and assistance from DS, EP, and TH. AA wrote the initial manuscript draft. DS, EP, and TH critically reviewed and edited the manuscript. DS, HS-V, AC, and EP conceived of the study. DS, HS-V, and EP obtained the funding. All authors reviewed and approved the final manuscript.
Funding
This work was funded by the Channel 7 Children’s Research Foundation (151068) and NHMRC Project Grant (1023781). AA was supported by an RTP scholarship from the Australian government and an NHMRC CRE scholarship (1078557). DS was supported by an Advance Queensland fellowship (AQRF13016-17RD2). EP was supported by a University of the Sunshine Coast fellowship and an Advance Queensland fellowship (AQIRF0362018). TH was supported by an NHMRC CRE (1040830). AC was supported by an NHMRC Practitioner Fellowship (1154302). HS-V was supported by an NHMRC CRE Postdoctoral Fellowship (1040830) and NHMRC-funded HOT NORTH collaboration (1131932).
Conflict of Interest Statement
The authors declare that the research was conducted in the absence of any commercial or financial relationships that could be construed as a potential conflict of interest.
Acknowledgments
We wish to thank the Menzies School of Health Research Child Health team for assistance in microbiological aspects of this study, and Roisin Ure (NHS Greater Glasgow and Clyde, Glasgow, United Kingdom) for assistance in H. influenzae MLST database submission. This manuscript has been released as a preprint at bioRxiv (Aziz et al., 2019).
Supplementary Material
The Supplementary Material for this article can be found online at: https://www.frontiersin.org/articles/10.3389/fmicb.2019.01622/full#supplementary-material
References
Apicella, M. A. (2012). Nontypeable Haemophilus influenzae: the role of N-acetyl-5-neuraminic acid in biology. Front. Cell Infect. Microbiol. 2:19. doi: 10.3389/fcimb.2012.00019
Artman, M., Domenech, E., and Weiner, M. (1983). Growth of Haemophilus influenzae in simulated blood cultures supplemented with hemin and NAD. J. Clin. Microbiol. 18, 376–379.
Atack, J. M., Srikhanta, Y. N., Fox, K. L., Jurcisek, J. A., Brockman, K. L., Clark, T. A., et al. (2015). A biphasic epigenetic switch controls immunoevasion, virulence and niche adaptation in non-typeable Haemophilus influenzae. Nat. Commun. 6:7828. doi: 10.1038/ncomms8828
Aziz, A., Sarovich, D. S., Harris, T. M., Kaestli, M., McRobb, E., Mayo, M., et al. (2017). Suspected cases of intracontinental Burkholderia pseudomallei sequence type homoplasy resolved using whole-genome sequencing. Microb. Genom 3:e000139 doi: 10.1099/mgen.0.000139
Aziz, A., Sarovich, D. S., Nosworthy, E., Beissbarth, J., Chang, A. B., Smith-Vaughan, H., et al. (2019). Molecular signatures of non-typeable Haemophilus influenzae lung adaptation in paediatric chronic lung disease. bioRxiv 614214. doi: 10.1101/614214
Baddal, B., Muzzi, A., Censini, S., Calogero, R. A., Torricelli, G., Guidotti, S., et al. (2015). Dual RNA-seq of Nontypeable Haemophilus influenzae and host cell transcriptomes reveals novel insights into host-pathogen cross talk. mBio 6:e01765-15. doi: 10.1128/mBio.01765-15
Bakaletz, L. O., Baker, B. D., Jurcisek, J. A., Harrison, A., Novotny, L. A., Bookwalter, J. E., et al. (2005). Demonstration of type IV pilus expression and a twitching phenotype by Haemophilus influenzae. Infect. Immun. 73, 1635–1643. doi: 10.1128/IAI.73.3.1635-1643.2005
Bell, S. M., Pham, J. N., Rafferty, D. L., and Allerton, J. K. (2016). Antibiotic Susceptibility Testing By the CDS Method, 8th Edn. Available: http://cdstest.net/wordpress/wp-content/uploads/Antibiotic-Susceptibility-Testing-by-the-CDS-Method-8th-Edition.pdf (accessed 25/03/2019).
Byun, M. K., Chang, J., Kim, H. J., and Jeong, S. H. (2017). Differences of lung microbiome in patients with clinically stable and exacerbated bronchiectasis. PLoS One 12:e0183553. doi: 10.1371/journal.pone.0183553
Coleman, H. N., Daines, D. A., Jarisch, J., and Smith, A. L. (2003). Chemically defined media for growth of Haemophilus influenzae strains. J. Clin. Microbiol. 41, 4408–4410. doi: 10.1128/JCM.41.9.4408-4410.2003
Connor, T. R., Corander, J., and Hanage, W. P. (2012). Population subdivision and the detection of recombination in non-typable Haemophilus influenzae. Microbiology 158, 2958–2964. doi: 10.1099/mic.0.063073-0
De Chiara, M., Hood, D., Muzzi, A., Pickard, D. J., Perkins, T., Pizza, M., et al. (2014). Genome sequencing of disease and carriage isolates of nontypeable Haemophilus influenzae identifies discrete population structure. Proc. Natl. Acad. Sci. U.S.A. 111, 5439–5444. doi: 10.1073/pnas.1403353111
De Ste Croix, M., Vacca, I., Kwun, M. J., Ralph, J. D., Bentley, S. D., Haigh, R., et al. (2017). Phase-variable methylation and epigenetic regulation by type I restriction-modification systems. FEMS Microbiol. Rev. 41, S3–S15. doi: 10.1093/femsre/fux025
Duell, B. L., Su, Y. C., and Riesbeck, K. (2016). Host-pathogen interactions of nontypeable Haemophilus influenzae: from commensal to pathogen. FEBS Lett. 590, 3840–3853. doi: 10.1002/1873-3468.12351
Erwin, A. L., Nelson, K. L., Mhlanga-Mutangadura, T., Bonthuis, P. J., Geelhood, J. L., Morlin, G., et al. (2005). Characterization of genetic and phenotypic diversity of invasive nontypeable Haemophilus influenzae. Infect. Immun. 73, 5853–5863. doi: 10.1128/IAI.73.9.5853-5863.2005
Erwin, A. L., and Smith, A. L. (2007). Nontypeable Haemophilus influenzae: understanding virulence and commensal behavior. Trends Microbiol. 15, 355–362. doi: 10.1016/j.tim.2007.06.004
Fothergill, J. L., Neill, D. R., Loman, N., Winstanley, C., and Kadioglu, A. (2014). Pseudomonas aeruginosa adaptation in the nasopharyngeal reservoir leads to migration and persistence in the lungs. Nat. Commun. 5:4780. doi: 10.1038/ncomms5780
Fox, K. L., Dowideit, S. J., Erwin, A. L., Srikhanta, Y. N., Smith, A. L., and Jennings, M. P. (2007). Haemophilus influenzae phasevarions have evolved from type III DNA restriction systems into epigenetic regulators of gene expression. Nucleic Acids Res. 35, 5242–5252. doi: 10.1093/nar/gkm571
Fung, C., Naughton, S., Turnbull, L., Tingpej, P., Rose, B., Arthur, J., et al. (2010). Gene expression of Pseudomonas aeruginosa in a mucin-containing synthetic growth medium mimicking cystic fibrosis lung sputum. J. Med. Microbiol. 59(Pt 9), 1089–1100. doi: 10.1099/jmm.0.019984-0
Galperin, M. Y., Makarova, K. S., Wolf, Y. I., and Koonin, E. V. (2015). Expanded microbial genome coverage and improved protein family annotation in the COG database. Nucleic Acids Res. 43, D261–D269. doi: 10.1093/nar/gku1223
Gibson, L. F., and Khoury, J. T. (1986). Storage and survival of bacteria by ultra-freeze. Lett. Appl. Microbiol. 3, 127–129. doi: 10.1111/j.1472-765X.1986.tb01565.x
Gilsdorf, J. R., Marrs, C. F., and Foxman, B. (2004). Haemophilus influenzae: genetic variability and natural selection to identify virulence factors. Infect. Immun. 72, 2457–2461. doi: 10.1128/iai.72.5.2457-2461.2004
Grønseth, R., Drengenes, C., Wiker, H. G., Tangedal, S., Xue, Y., Husebø, G. R., et al. (2017). Protected sampling is preferable in bronchoscopic studies of the airway microbiome. ERJ Open Res. 3, 00019–2017. doi: 10.1183/23120541.00019-2017
Hare, K. M., Grimwood, K., Leach, A. J., Smith-Vaughan, H., Torzillo, P. J., Morris, P. S., et al. (2010). Respiratory bacterial pathogens in the nasopharynx and lower airways of Australian indigenous children with bronchiectasis. J. Pediatr. 157, 1001–1005. doi: 10.1016/j.jpeds.2010.06.002
Holt, L. B. (1962). The growth-factor requirements of Haemopiius influenzae. J. Gen. Microbiol. 27, 317–322. doi: 10.1099/00221287-27-2-317
Hood, D. W., Cox, A. D., Gilbert, M., Makepeace, K., Walsh, S., Deadman, M. E., et al. (2001). Identification of a lipopolysaccharide alpha-2,3-sialyltransferase from Haemophilus influenzae. Mol. Microbiol. 39, 341–350. doi: 10.1046/j.1365-2958.2001.02204.x
Hosking, S. L., Craig, J. E., and High, N. J. (1999). Phase variation of lic1A, lic2A and lic3A in colonization of the nasopharynx, bloodstream and cerebrospinal fluid by Haemophilus influenzae type b. Microbiology 145, 3005–3011. doi: 10.1099/00221287-145-11-3005
Humair, P. F., Douet, V., Moran Cadenas, F., Schouls, L. M., Van De Pol, I., and Gern, L. (2007). Molecular identification of bloodmeal source in Ixodes ricinus ticks using 12S rDNA as a genetic marker. J. Med. Entomol. 44, 869–880. doi: 10.1093/jmedent/44.5.869
Jolley, K. A., and Maiden, M. C. (2010). BIGSdb: scalable analysis of bacterial genome variation at the population level. BMC Bioinformatics 11:595. doi: 10.1186/1471-2105-11-595
Jurcisek, J. A., Bookwalter, J. E., Baker, B. D., Fernandez, S., Novotny, L. A., and Munson, R. S. Jr., et al. (2007). The PilA protein of non-typeable Haemophilus influenzae plays a role in biofilm formation, adherence to epithelial cells and colonization of the mammalian upper respiratory tract. Mol. Microbiol. 65, 1288–1299. doi: 10.1111/j.1365-2958.2007.05864.x
Kasi, A. S., Neubauer, C., Kato, R. M., and Newman, D. K. (2017). Bacterial growth rate in cystic fibrosis pulmonary exacerbation. Am. J. Respir. Crit. Care Med. 195:A4856. doi: 10.1164/ajrccm-conference.2017.195.1_MeetingAbstracts.A4856
Lim, Y. W., Schmieder, R., Haynes, M., Willner, D., Furlan, M., Youle, M., et al. (2013). Metagenomics and metatranscriptomics: windows on CF-associated viral and microbial communities. J. Cyst. Fibros 12, 154–164. doi: 10.1016/j.jcf.2012.07.009
Mandrell, R. E., McLaughlin, R., Aba Kwaik, Y., Lesse, A., Yamasaki, R., Gibson, B., et al. (1992). Lipooligosaccharides (LOS) of some Haemophilus species mimic human glycosphingolipids, and some LOS are sialylated. Infect. Immun. 60, 1322–1328.
Martinez-Garcia, M. A., Soler-Cataluna, J. J., Donat Sanz, Y., Catalan Serra, P., Agramunt Lerma, M., Ballestin Vicente, J., et al. (2011). Factors associated with bronchiectasis in patients with COPD. Chest 140, 1130–1137. doi: 10.1378/chest.10-1758
Moleres, J., Fernandez-Calvet, A., Ehrlich, R. L., Marti, S., Perez-Regidor, L., Euba, B., et al. (2018). Antagonistic pleiotropy in the bifunctional surface protein FadL (OmpP1) during adaptation of Haemophilus influenzae to chronic lung infection associated with chronic obstructive pulmonary disease. mBio 9:e1176-18. doi: 10.1128/mBio.01176-18
Murphy, T. F. (2003). Respiratory infections caused by non-typeable Haemophilus influenzae. Curr. Opin. Infect. Dis. 16, 129–134. doi: 10.1097/01.aco.0000065079.06965.e0
Murphy, T. F., and Kirkham, C. (2002). Biofilm formation by nontypeable Haemophilus influenzae: strain variability, outer membrane antigen expression and role of pili. BMC Microbiol. 2:7. doi: 10.1186/1471-2180-2-7
Murray, N. E. (2000). Type I restriction systems: sophisticated molecular machines (a legacy of Bertani and Weigle). Microbiol. Mol. Biol. Rev. 64, 412–434. doi: 10.1128/MMBR.64.2.412-434.2000
Pettigrew, M. M., Ahearn, C. P., Gent, J. F., Kong, Y., Gallo, M. C., Munro, J. B., et al. (2018). Haemophilus influenzae genome evolution during persistence in the human airways in chronic obstructive pulmonary disease. Proc. Natl. Acad. Sci. U.S.A. 115, E3256–E3265. doi: 10.1073/pnas.1719654115
Poole, J., Foster, E., Chaloner, K., Hunt, J., Jennings, M. P., Bair, T., et al. (2013). Analysis of nontypeable Haemophilus influenzae phase-variable genes during experimental human nasopharyngeal colonization. J. Infect. Dis. 208, 720–727. doi: 10.1093/infdis/jit240
Power, P. M., Bentley, S. D., Parkhill, J., Moxon, E. R., and Hood, D. W. (2012). Investigations into genome diversity of Haemophilus influenzae using whole genome sequencing of clinical isolates and laboratory transformants. BMC Microbiol. 12:273. doi: 10.1186/1471-2180-12-273
Price, E. P., Harris, T. M., Spargo, J., Nosworthy, E., Beissbarth, J., Chang, A. B., et al. (2017). Simultaneous identification of Haemophilus influenzae and Haemophilus haemolyticus using real-time PCR. Future Microbiol. 12, 585–593. doi: 10.2217/fmb-2016-0215
Price, E. P., Sarovich, D. S., Mayo, M., Tuanyok, A., Drees, K. P., Kaestli, M., et al. (2013). Within-host evolution of Burkholderia pseudomallei over a twelve-year chronic carriage infection. mBio 4:e388-13. doi: 10.1128/mBio.00388-13
Price, E. P., Sarovich, D. S., Nosworthy, E., Beissbarth, J., Marsh, R. L., Pickering, J., et al. (2015). Haemophilus influenzae: using comparative genomics to accurately identify a highly recombinogenic human pathogen. BMC Genomics 16:641. doi: 10.1186/s12864-015-1857-x
Purcell, P., Jary, H., Perry, A., Perry, J. D., Stewart, C. J., Nelson, A., et al. (2014). Polymicrobial airway bacterial communities in adult bronchiectasis patients. BMC Microbiol. 14:130. doi: 10.1186/1471-2180-14-130
R Core Team (2014). R: A Language and Environment for Statistical Computing. Vienna: R Foundation for Statistical Computing.
Robinson, M. D., McCarthy, D. J., and Smyth, G. K. (2010). edgeR: a Bioconductor package for differential expression analysis of digital gene expression data. Bioinformatics 26, 139–140. doi: 10.1093/bioinformatics/btp616
Santana, E. A., Harrison, A., Zhang, X. J., Baker, B. D., Kelly, B. J., White, P., et al. (2014). HrrF is the fur-regulated small RNA in nontypeable Haemophilus influenzae. PLoS One 9:e105644. doi: 10.1371/journal.pone.0105644
Sarovich, D. S., and Price, E. P. (2014). SPANDx: a genomics pipeline for comparative analysis of large haploid whole genome re-sequencing datasets. BMC Res. Notes 7:618. doi: 10.1186/1756-0500-7-618
Sikkema, D. J., and Murphy, T. F. (1992). Molecular analysis of the P2 porin protein of nontypeable Haemophilus influenzae. Infect. Immun. 60, 5204–5211.
Slack, M. P. E. (2015). A review of the role of Haemophilus influenzae in community-acquired pneumonia. Pneumonia 6, 26–43. doi: 10.15172/pneu.2015.6/520
Slack, M. P. E. (2017). The evidence for non-typeable Haemophilus influenzae as a causative agent of childhood pneumonia. Pneumonia 9:9. doi: 10.1186/s41479-017-0033-2
Smith, E. E., Buckley, D. G., Wu, Z., Saenphimmachak, C., Hoffman, L. R., D’Argenio, D. A., et al. (2006). Genetic adaptation by Pseudomonas aeruginosa to the airways of cystic fibrosis patients. Proc. Natl. Acad. Sci. U.S.A. 103, 8487–8492. doi: 10.1073/pnas.0602138103
Sriram, K. B., Cox, A. J., Clancy, R. L., Slack, M. P. E., and Cripps, A. W. (2018). Nontypeable Haemophilus influenzae and chronic obstructive pulmonary disease: a review for clinicians. Crit. Rev. Microbiol. 44, 125–142. doi: 10.1080/1040841X.2017.1329274
Starner, T. D., Zhang, N., Kim, G., Apicella, M. A., and McCray, P. B. Jr. (2006). Haemophilus influenzae forms biofilms on airway epithelia: implications in cystic fibrosis. Am. J. Respir. Crit. Care Med. 174, 213–220. doi: 10.1164/rccm.200509-1459OC
Swords, W. E. (2012). Nontypeable Haemophilus influenzae biofilms: role in chronic airway infections. Front. Cell Infect. Microbiol. 2:97. doi: 10.3389/fcimb.2012.00097
Tufvesson, E., Bjermer, L., and Ekberg, M. (2015). Patients with chronic obstructive pulmonary disease and chronically colonized with Haemophilus influenzae during stable disease phase have increased airway inflammation. Int. J. Chronic Obstr. 10, 881–889. doi: 10.2147/Copd.S78748
Van Eldere, J., Slack, M. P. E., Ladhani, S., and Cripps, A. W. (2014). Non-typeable Haemophilus influenzae, an under-recognised pathogen. Lancet Infect. Dis. 14, 1281–1292. doi: 10.1016/s1473-3099(14)70734-0
Vasu, K., and Nagaraja, V. (2013). Diverse functions of restriction-modification systems in addition to cellular defense. Microbiol. Mol. Biol. Rev. 77, 53–72. doi: 10.1128/MMBR.00044-12
Viberg, L. T., Sarovich, D. S., Kidd, T. J., Geake, J. B., Bell, S. C., Currie, B. J., et al. (2017). Within-host evolution of Burkholderia pseudomallei during chronic infection of seven australasian cystic fibrosis patients. mBio 8:e356-17. doi: 10.1128/mBio.00356-17
Wong, S. M., and Akerley, B. J. (2012). Genome-scale approaches to identify genes essential for Haemophilus influenzae pathogenesis. Front. Cell Infect. Microbiol. 2:23. doi: 10.3389/fcimb.2012.00023
Wurzel, D. F., Marchant, J. M., Yerkovich, S. T., Upham, J. W., Petsky, H. L., Smith-Vaughan, H., et al. (2016). Protracted bacterial bronchitis in children: natural history and risk factors for bronchiectasis. Chest 150, 1101–1108. doi: 10.1016/j.chest.2016.06.030
Yang, L., Haagensen, J. A., Jelsbak, L., Johansen, H. K., Sternberg, C., Hoiby, N., et al. (2008). In situ growth rates and biofilm development of Pseudomonas aeruginosa populations in chronic lung infections. J. Bacteriol. 190, 2767–2776. doi: 10.1128/JB.01581-07
Keywords: non-typeable Haemophilus influenzae, NTHi, RNA-seq, transcriptomics, comparative genomics, convergence, bacterial evolution, adaptation
Citation: Aziz A, Sarovich DS, Nosworthy E, Beissbarth J, Chang AB, Smith-Vaughan H, Price EP and Harris TM (2019) Molecular Signatures of Non-typeable Haemophilus influenzae Lung Adaptation in Pediatric Chronic Lung Disease. Front. Microbiol. 10:1622. doi: 10.3389/fmicb.2019.01622
Received: 07 May 2019; Accepted: 01 July 2019;
Published: 16 July 2019.
Edited by:
Daniel Yero, Autonomous University of Barcelona, SpainReviewed by:
Timothy Murphy, University at Buffalo, United StatesKristian Riesbeck, Lund University, Sweden
Copyright © 2019 Aziz, Sarovich, Nosworthy, Beissbarth, Chang, Smith-Vaughan, Price and Harris. This is an open-access article distributed under the terms of the Creative Commons Attribution License (CC BY). The use, distribution or reproduction in other forums is permitted, provided the original author(s) and the copyright owner(s) are credited and that the original publication in this journal is cited, in accordance with accepted academic practice. No use, distribution or reproduction is permitted which does not comply with these terms.
*Correspondence: Erin P. Price, eprice@usc.edu.au
†These authors have contributed equally to this work