- 1Department of Phytopathology, Centre for BioSystems, Land Use and Nutrition, Justus Liebig University, Giessen, Germany
- 2Department of Crop Sciences, Molecular Phytopathology and Mycotoxin Research, University of Göttingen, Göttingen, Germany
In filamentous fungi, gene silencing through RNA interference (RNAi) shapes many biological processes, including pathogenicity. We explored the requirement of key components of fungal RNAi machineries, including DICER-like 1 and 2 (FgDCL1, FgDCL2), ARGONAUTE 1 and 2 (FgAGO1, FgAGO2), AGO-interacting protein FgQIP (QDE2-interacting protein), RecQ helicase (FgQDE3), and four RNA-dependent RNA polymerases (FgRdRP1, FgRdRP2, FgRdRP3, FgRdRP4), in the ascomycete mycotoxin-producing fungal pathogen Fusarium graminearum (Fg) for sexual and asexual multiplication, pathogenicity, and its sensitivity to double-stranded (ds)RNA. We corroborate and extend earlier findings that conidiation, ascosporogenesis, and Fusarium head blight (FHB) symptom development require an operable RNAi machinery. The involvement of RNAi in conidiation is dependent on environmental conditions as it is detectable only under low light (<2 μmol m−2 s−1). Although both DCLs and AGOs partially share their functions, the sexual ascosporogenesis is mediated primarily by FgDCL1 and FgAGO2, while FgDCL2 and FgAGO1 contribute to asexual conidia formation and germination. FgDCL1 and FgAGO2 also account for pathogenesis as their knockout (KO) results in reduced FHB development. Apart from KO mutants Δdcl2 and Δago1, mutants Δrdrp2, Δrdrp3, Δrdrp4, Δqde3, and Δqip are strongly compromised for conidiation, while KO mutations in all RdPRs, QDE3, and QIP strongly affect ascosporogenesis. Analysis of trichothecenes mycotoxins in wheat kernels showed that the relative amount of deoxynivalenol (DON), calculated as [DON] per amount of fungal genomic DNA was reduced in all spikes infected with RNAi mutants, suggesting the possibility that the fungal RNAi pathways affect Fg’s DON production. Moreover, silencing of fungal genes by exogenous target gene-specific double-stranded RNA (dsRNA) (spray-induced gene silencing, SIGS) is dependent on DCLs, AGOs, and QIP, but not on QDE3. Together these data show that in F. graminearum, different key components of the RNAi machinery are crucial in different steps of fungal development and pathogenicity.
Introduction
RNA interference (RNAi) is a conserved mechanism triggered by double-stranded (ds)RNA that mediates resistance to exogenous nucleic acids, regulates the expression of protein-coding genes on the transcriptional and post-transcriptional level, and preserves genome stability by transposon silencing (Fire et al., 1998; Mello and Conte, 2004; Hammond, 2005; Baulcombe, 2013). Many reports have demonstrated that this natural mechanism for sequence-specific gene silencing also holds promise for experimental biology and offers practical applications in functional genomics, therapeutic intervention, and agriculture (Nowara et al., 2010; Koch and Kogel, 2014; Cai et al., 2018; Zanini et al., 2018). Core RNAi pathway components are conserved in eukaryotes, including most parasitic and beneficial fungi (Cogoni and Macino, 1999; Dang et al., 2011; Carreras-Villaseñor et al., 2013; Torres-Martínez and Ruiz-Vázquez, 2017): DICER-like (DCL) enzymes, which belong to the RNase III superfamily, generate double-stranded small interfering (si)RNAs and micro (mi)RNAs (Meng et al., 2017; Song and Rossi, 2017); ARGONAUTE (AGO) superfamily proteins bind small RNA duplexes to form an RNA-induced silencing complex (RISC) for transcriptional and post-transcriptional gene silencing (PTGS) (Zhang et al., 2015; Nguyen et al., 2018); and RNA-dependent RNA polymerases (RdRPs) are involved in the production of double-stranded RNA (dsRNA) that initiate the silencing mechanism as well as in the amplification of the silencing signals through the generation of secondary siRNAs (Calo et al., 2012).
Fungal RNAi pathways contribute to genome protection (Meng et al., 2017), pathogenicity (Weiberg et al., 2013; Kusch et al., 2018; Zanini et al., 2019), development (Carreras-Villaseñor et al., 2013), and antiviral defense (Segers et al., 2007; Campo et al., 2016; Wang et al., 2016a). In Aspergillus flavus (Bai et al., 2015), Magnaporthe oryzae (Raman et al., 2017), and Penicillium marneffei (Lau et al., 2013), sRNAs were shown to be responsive to environmental stress. In Trichoderma atroviride, both light-dependent asexual reproduction and light-independent hyphal growth require an operational RNAi machinery (Carreras-Villaseñor et al., 2013). Similarly, in Mucor circinelloides, defects in the RNAi machinery resulted in various developmental defects such as dysfunction during sexual and asexual reproduction (Torres-Martínez and Ruiz-Vázquez, 2017).
Neurospora crassa, a model organism for studying RNAi in filamentous fungi, has different silencing pathways, including quelling (Romano and Macino, 1992) and meiotic silencing by unpaired DNA (MSUD) (Shiu et al., 2001). In the vegetative stage, the introduction of transgenes results in PTGS of the transgenes and cognate endogenous mRNAs, an RNAi silencing phenomenon known as quelling. The process requires QDE3 (Quelling defective 3), which encodes a RecQ helicase, and RPA (subunit of replication protein A), which recognizes aberrant DNA structures. Interaction of these proteins recruits Quelling defective 1 (QDE1), a protein with dual function as DNA-dependent RNA polymerase (DdRP) and RdPR, to the single-stranded (ss)DNA locus, resulting in production of aberrant ssRNAs and its conversion to dsRNAs. Subsequently, the dsRNA is processed into small RNAs by DCL1. sRNAs duplexes are loaded onto QDE2 (Quelling defective 2), which encodes an AGO homolog. QDE2 cleaves the passenger strand and the exonuclease QIP (QDE2-interacting protein) assists to remove it to form an active RISC that targets complementary mRNA for degradation (Chang et al., 2012). MSUD occurs during sexual development in prophase I of meiosis, when unpaired homologous DNA sequences have been detected during the pairing of the homologous chromosomes, which then also leads to the production of aberrant RNA transcripts (Chang et al., 2012). Genes required for MSUD are SAD1 (Suppressor of ascus dominance 1), a paralog of QDE-1, and SAD2. SAD2 recruits SAD1 to the perinuclear region, where aberrant RNA is converted to dsRNA. Upon silencing by DCL1, the small RNA duplexes are loaded onto SMS2 (Suppressor of meiotic silencing 2), an AGO homolog in Neurospora, which also is assisted by QIP. In contrast, QDE-2 and DCL2 are not required for MSUD in Neurospora, indicating that there are two parallel RNAi pathways functioning separately in the vegetative and meiotic stages.
Fusarium graminearum (Fg) is one of the devastating pathogens of cereals causing Fusarium head blight (FHB) and Crown Rot (FCR) (Dean et al., 2012; Harris et al., 2016). The pathogen belongs to the filamentous ascomycetes. Ascospores are the primary inoculum for FHB epidemics as these spores are forcibly shot into the environment and also can pass long distances (Maldonado-Ramirez et al., 2005). Moreover, the sexual development ensures the formation of survival structures necessary for overwintering (Dill-Macky and Jones, 2000) and the genetic diversity of the population (Cuomo et al., 2007). Of note, spike infections can be symptomless or symptomatic (Urban et al., 2015; Brown et al., 2017). In both cases, Fusarium fungi contaminate the grain with mycotoxins and thus decrease grain quality. Among the mycotoxins, the B group trichothecenes, including deoxynivalenol (DON), nivalenol (NIV), and their acetylated derivatives (3A-DON, 15A-DON, and 4A-NIV) influence the virulence of the fungus (Desjardins et al., 1993; Jansen et al., 2005; Ilgen et al., 2009). Mycotoxins such as DON trigger an oxidative burst in the host plants, resulting in cell necrosis and disintegration of the defense system, which then favors colonization of the plant tissues by a necrotrophic fungus (Audenaert et al., 2014). Importantly, Fg possesses a functional MSUD mechanism (Son et al., 2011) and AGO genes FgSMS2 or FgAGO2 are necessary for sexual reproduction (Kim et al., 2015). A recent work discovered that the sex-induced RNAi mechanism has important roles in sexual reproduction (Son et al., 2017). siRNAs produced from exonic gene regions (ex-siRNAs) participate in PTGS at a genome-wide level in the late stages of sexual reproduction. The sex-specific RNAi pathway is primarily governed by FgDCL1 and FgAGO2. Thus, Fg primarily utilizes ex-siRNA-mediated RNAi for ascospore formation. Consistent with the key role of FgDCL1 in generative development, the combination of sRNA and transcriptome sequencing predicted 143 novel microRNA-like RNAs (milRNAs) in wild-type perithecia, of which most were dependent on FgDCL1. Given that 117 potential target genes were predicted, these perithecium-specific milRNAs may play roles in sexual development (Zeng et al., 2018).
To develop RNAi-based plant protection strategies such as host-induced gene silencing (HIGS) (Koch et al., 2013) and spray-induced gene silencing (SIGS) (Koch et al., 2016, 2018) against Fusarium species, it is required to bank on knowledge about the RNAi components involved in Fusarium development and pathogenicity. A report of Chen and colleagues (Chen et al., 2015) demonstrated that, in Fg, a hairpin RNA (hpRNA) can efficiently silence the expression of a target gene, and that the RNAi components FgDCL2 and FgAGO1 are required for silencing. This finding is consistent with reports showing that a Fg wild-type (WT) strain, but not Fg RNAi mutants, is amenable to SIGS-mediated target gene silencing, when it grows on a plant sprayed with exogenous dsRNA directed against the fungal Cytochrome P450 lanosterol C-14α-demethylase (CYP51) genes (Koch et al., 2016). In the present study study, we expanded previous studies to address the requirement of an extended set of Fg RNAi genes in growth, reproduction, virulence, toxin production, and SIGS-mediated inhibition of fungal infection cereal hosts.
Materials and Methods
Fungal Material, Generation of Gene Deletion Mutants in Fusarium graminearum
The Fg strain PH1 and the PH1 dcl1 dcl2 double mutant were a gift of Dr. Martin Urban, Rothamsted Research, England. RNAi gene deletion mutants were generated in the Fg strain IFA65 (IFA, Department for Agrobiotechnology, Tulln, Austria), hereafter termed IFA WT. They were generated by homolog recombination using the pPK2 binary vector. Fg RNAi genes were identified by blasting Neurospora crassa genes against the Fusarium genome sequence in the Broad institute database. Disruption vectors were constructed by inserting two flanking fragments (~1,000 bp) upstream and downstream of the corresponding genes in the pPK2 vector as follows: RdRP1, AGO1, QDE3, QIP, AGO2, DCL1, RdRP2, RdRP3, RdRP4, and DCL2 upstream flanking sequences were inserted in the plasmid between PacI-KpnI restriction sites, and the downstream flanking sequences were inserted between XbaI-HindIII restriction sites, except the AGO2 downstream flanking sequence which was inserted in XbaI restriction site (primers used in disruption plasmid construction are listed in Supplementary Table S1). Disruption vectors were introduced into Agrobacterium tumefaciens (LBA440 and AGL1 strains) by electroporation. A single colony of Agrobacterium containing the pPK2 plasmid was grown in 10 ml of YEB medium (Vervliet et al., 1975) containing the appropriate antibiotics (5 μg/ml tetracycline +25 μg/ml rifampicin +50 μg/ml kanamycin for LBA440, and 25 μg/ml carbenicillin +25 μg/ml rifampicin +50 μg/ml kanamycin for AGL1) and incubated at 28°C till OD600nm 0.7 was reached. T-DNA was mobilized in Agrobacterium with 200 μM acetosyringone, and Agrobacterium and fungal recipient IFA WT were co-cultivated on black filter paper (DP 551070, Albert LabScience, Hahnemühle, Dassel, Germany), respectively. Putative fungal mutants were selected on potato extract glucose (PEG, Roth, Germany) medium containing 150 μg/ml hygromycin +150 μg/ml ticarcillin and grown for 5 days. For genotyping, genomic DNA of putative Fusarium mutants was extracted from mycelia.
Genotyping of Fusarium Mutants
Fg IFA mutants were confirmed by genotyping using primers located in hygromycin and corresponding gene flanking sequences (located after the cloned flanking sequence in the genome) (Supplementary Table S2). Upon amplification, the samples were sequenced. Additionally, mRNA expression levels in mutants vs. levels in IFA WT were measured by quantitative real-time PCR (qRT-PCR) using primers pairs listed in (Supplementary Table S3). The mRNA transcripts were measured using 1 × SYBR Green JumpStart Taq Ready Mix (Sigma-Aldrich, Germany) according to manufacturer’s instructions and assayed in 7500 Fast Real-Time PCR cycler (Applied Biosystems Inc., CA, USA) under the following thermal cycling conditions: initial activation step at 95°C for 5 min, 40 cycles (95°C for 30 s, 53°C for 30 s, and 72°C for 30 s). The Ct values were determined with the software in the qRT-PCR instrument and the transcript levels of the genes were determined according to the 2-ΔΔCt method (Livak and Schmittgen, 2001).
Colony Morphology
The RNAi mutants were cultured on PEG, starch agar (SA) and synthetic nutrient agar (SNA) (Leslie and Summerell, 2006). The cultures were incubated at 25°C in 12-h light/12-h dark (52 μmol m−2 s−1, Philips Master TL-D HF 16 W/840). The growth was documented after 5 days. For growth in liquid cultures, agar blocks (1 cm in diameter) from 2-week-old fungal cultures were incubated in liquid PEG for 5 days at room temperature (RT) under light (2 μmol m−2 s−1) with shaking. Each mutant was grown in flask containing medium supplemented with hygromycin (100 μg/ml) and flask containing medium without hygromycin. Photos were taken to document the growth pattern after 5 days of incubation.
Production of Fungal Biomass
Fifty milligram mycelia (fresh mycelia from 4-day-old fungal cultures grown on Aspergillus complete medium (CM) plates in the dark; Leslie and Summerell, 2006) were incubated in a 100-ml flask containing 20 ml of PEG medium incubated at RT with shaking under 12-h light (2 μmol m−2 s−1). Fungal mycelium was harvested after 3 days by filtration through filter paper (Munktell, Germany), washed with distilled water twice, and dried at 75°C overnight. The dry weight was calculated by using the following formula: Dry weight = (weight of filter paper + mycelium) − (weight of filter paper).
Conidiation Assay
Production of conidia was done according to Yun et al. (2015) with slight modification. Four-day-old cultures of each mutant and IFA WT growing in CM agar plates in the dark at 25°C were used for fresh mycelia preparation. The mycelia were scraped from the plate surface using a sterile toothpick; then, 50 mg mycelia were inoculated in a 100-ml flask containing 20 ml of SN medium. The flasks were incubated at RT for 5 days in light (2 μmol m−2 s−1) on a shaker (100 rpm). Subsequently, the conidia produced from each mutant and WT were counted using a hemocytometer (Fuchs Rosenthal, Superior Marienfeld, Germany).
Viability Test of Conidia
Fourteen milliliters from the same cultures used in conidiation assay were centrifuged at 4,000 rpm for 10 min to precipitate conidia. The conidia were resuspended in 5 ml 2% sucrose water and incubated in dark for 2 days at 23°C. Germinated and non-germinated conidia were visualized and counted under an inverse microscope. Conidia germination rate was determined as percentage of germinated conidia of the total conidia number.
Perithecia Production and Ascospore Discharge Assay
Fungi were grown on carrot agar prepared under bright fluorescent light at RT (18–24°C) for 5 days (Klittich and Leslie, 1988). Aerial mycelia were removed with a sterile toothpick. To stimulate sexual reproduction and perithecia formation, 1 ml of 2.5% Tween 60 was applied to the plates with a sterile glass rod after scraping the mycelia (Cavinder et al., 2012). The plates were incubated under fluorescent light at RT for 9 days. Subsequently, agar blocks (1.5 cm in diameter) were cut from the plates containing the mature perithecia using a cork borer. Agar blocks were sliced in half, placed on glass microscope slides, and incubated in boxes under high humidity for 2 days under 24 h of light (52 μmol m−2 s−1 Philips Master TL-D HF 16 W/840). During this time, ascospores discharged from the perithecia accumulated on the slide. For the quantification of discharged ascospores, slides were washed off by 2 ml of an aqueous Tween 20 (0.002%) solution and counted using a hemocytometer.
Viability Test of the Discharged Ascospores
Mycelia with mature perithecia (13 days after sexual induction) on carrot agar were incubated in a humid box at RT under light for 4 days according to Son et al. (2017). The discharged ascospores were washed from the plate cover using SN liquid medium and incubated in the dark for 24 h in a humid box. The germinated and non-germinated ascospores were visualized under an inverse microscope and counted.
Pathogenicity Assay on Wheat Ears
The susceptible wheat cultivar Apogee was used. Plants were grown in an environmentally controlled growth chamber (24°C, 16 h light, 180 μmol m−2 s−1 photon flux density, 60% rel. humidity) till anthesis. Point inoculations to the second single floret of each spike were performed at mid-anthesis with 5 μl of a 40,000 conidia/ml suspension amended with 0.002% v/v Tween 20 (Gosman et al., 2010). Control plants were treated with sterile Tween 20. For each Fg genotype, 10 wheat heads were inoculated and incubated in plastic boxes misted with water to maintain high humidity for 2 days. Incubation continued at 22°C in 60% rel. humidity. Infected wheat heads were observed nine and 13 dpi and infection percentage was determined as the ratio of infected spikelets to the total spikelet number per ear.
Thousand Grain Weight of Infected Wheat Kernels
One hundred kernels from two biological experiments with 10 wheat heads point-inoculated with IFA WT and mutants were counted and weighed. TGW was calculated in grams per 1,000 kernels of cleaned wheat seeds.
Quantification of Fungal DNA in Infected Wheat Kernels
Fungal genomic DNA in kernels was quantified using qPCR as described (Brandfass and Karlovsky, 2008). Dried grains were ground and DNA was extracted from 30 mg of flour and dissolved in 50 μl of TE buffer. One microliter of 50x diluted DNA was used as template for RT-PCR with primers amplifying a 280-bp fragment specific for Fg. The PCR mix consisted of reaction buffer [16 mM (NH4)2SO4, 67 mM Tris-HCl, 0.01% Tween-20, pH 8.8 at 25°C; 3 mM MgCl2, 0.3 μM of each primer, 0.2 mM of each dATP, dTTP, dCTP and dGTP (Bioline), 0.03 U/μl Taq DNA polymerase (Bioline, Luckenwalde, Germany) and 0.1x SYBR Green I solution (Invitrogen, Karlsruhe, Germany)]. The PCR was performed in CFX384 thermocycler (BioRad, Hercules, CA, USA) according to the following cycling condition: initial denaturation for 2 min at 95°C, 35 cycles with 30 s at 94°C, 30 s at 61°C, 30 s at 68°C, and final elongation for 5 min at 68°C. No matrix effects were detectable with 50-fold diluted DNA extracted from grains. Standards were prepared from pure Fg DNA in 3-fold dilution steps from 100 pg to 0.4 pg/well.
Analysis of Mycotoxins in Infected Wheat Kernels
The content of mycotoxins in wheat kernels infected with Fg RNAi mutants and IFA WT was determined using high-performance liquid chromatography coupled to tandem mass spectrometry (HPLC-MS/MS). Mycotoxins were extracted from ground grains with mixture containing 84% acetonitrile, 15% water, and 1% acetic acid and the extracts were defatted with cyclohexane. Chromatographic separation was carried out on a C18 column eluted with a water/methanol gradient and the analytes were ionized by electrospray and detected by MS/MS in multiple reaction monitoring (MRM) mode essentially as described (Sulyok et al., 2006).
Spray Application of Double-Stranded RNA on Barley Leaves
Second leaves of 3-week-old barley cultivar Golden Promise were detached and transferred to square Petri plates containing 1% agar. dsRNA spray applications and leaf inoculation were done as described (Koch et al., 2016). For the TE control, TE buffer was diluted in 500 μl of water corresponding to the amount used for dilution of the dsRNA. Typical RNA concentration after elution was 500 ng μl−1, representing a buffer concentration of 400 μM Tris-HCL and 40 μM EDTA (TE buffer) in the final dilution. TE buffer was indistinguishable from treatments with control dsRNA generated from the GFP or GUS gene, respectively (Koch et al., 2016, 2018). Thus, we used TE buffer as control to save costs. Spraying of the leaves was carried out in the semi-systemic design (Koch et al., 2016), where the lower parts of the detached leaf segments were covered by a tinfoil to avoid direct contact of dsRNA with the leaf surface that was subsequently inoculated.
Statistics and Analysis
Data obtained from two or three repetitions were subjected to the Student’s t test in Microsoft office Excel 2010. Significance was determined as p ≤ 0.05, 0.01, or 0.001 and indicated by *, **, or ***, respectively. Unless specified otherwise, data are presented as mean ± standard error or mean ± standard deviation of the mean. Sequence analysis was performed on the ApE plasmid editor free tool. Basic Local Alignment Search Tool (BLAST) NCBI BLAST1 was used for sequence search and alignment.
Results
Requirement of RNA Interference Pathway Core Components Under Different Light Regimes
The Fg genome obtained from the Broad Institute2 contains many functional RNAi machinery components (Chen et al., 2015; Son et al., 2017). We generated Fg gene replacement mutants for several major RNAi genes by homolog recombination using the pPK2 binary vector (Table 1). Disruption vectors for FgDCL1, FgDCL2, FgAGO1, FgAGO2, FgRdRP1, FgRdRP2, FgRdRP3, FgRdRP4, FgQDE3, and FgQIP were constructed by inserting two flanking fragments (~1,000 bp) upstream and downstream of the corresponding genes in pPK2 vector (Supplementary Table S1; Supplementary Figure S1). The vectors were introduced into Agrobacterium tumefaciens, followed by agro-transformation of the Fg strain IFA. Transformants were transferred to Petri dishes of PEG medium, containing 150 μg/ml hygromycin and 150 μg/ml ticarcillin. Mutants were verified by PCR analysis with genomic DNA as template (Figure 1) and by expression analysis of the respective RNAi gene (Supplementary Figure S2). Colony morphology of PCR-verified mutants (12 h light, see section “Methods”) was inspected in axenic cultures of three different media, PEG, SNA, and SA. In the PEG agar medium, all mutants showed slightly reduced radial growth, while there were no clear differences as compared with the IFA WT strain in SN and SA media (Supplementary Figures S3A–C). In liquid PEG medium under day light conditions, all mutants produced comparable amounts of mycelium biomass, though different amounts of the red pigment aurofusarin (Frandsen et al., 2006): Δdcl1, Δdcl2, Δrdrp1, Δqde3, and Δqip1 showed reduced pigmentation, while Δago1, Δrdrp2, Δrdrp3, and Δrdrp4 showed higher pigmentation compared to IFA WT (Supplementary Figure S3D; Table 2). Under light induction conditions (12-h light; 52 μmol m−2 s−1), conidia grown in 96-well plate liquid SN cultures showed normal germ tube emergence (not shown). All RNAi mutants formed an elongated hyphal cell type, producing abundant conidia on conidiophores and directly from hyphae. Conidia were moderately curved with clear septations.
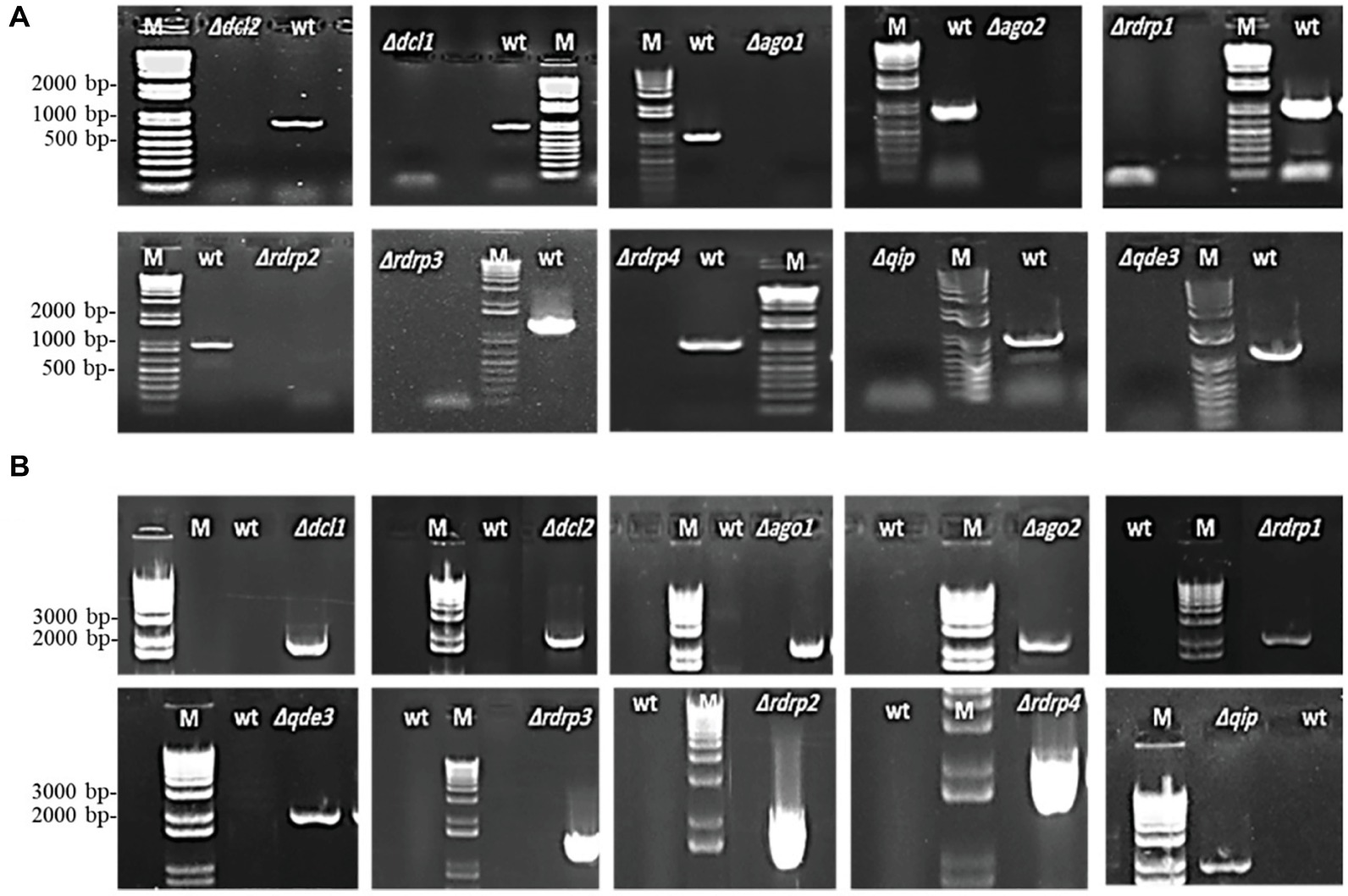
Figure 1. PCR verification of targeted gene replacement in Fusarium graminearum. (A) Amplification of an internal part of the targeted genes DCL1, DCL2, AGO1, AGO2, RdRP1, RdRP4, RdRP2, RdRP3, QIP, and QDE3 is positive in IFA WT and negative in corresponding mutants. (B) PCR with primer pairs in the right recombination sequence and hygromycin, showing that the antibiotic resistance gene had integrated into the target gene locus. PCR products were analyzed on 1.5% agarose gel electrophoresis. M, DNA marker; WT, wild type.
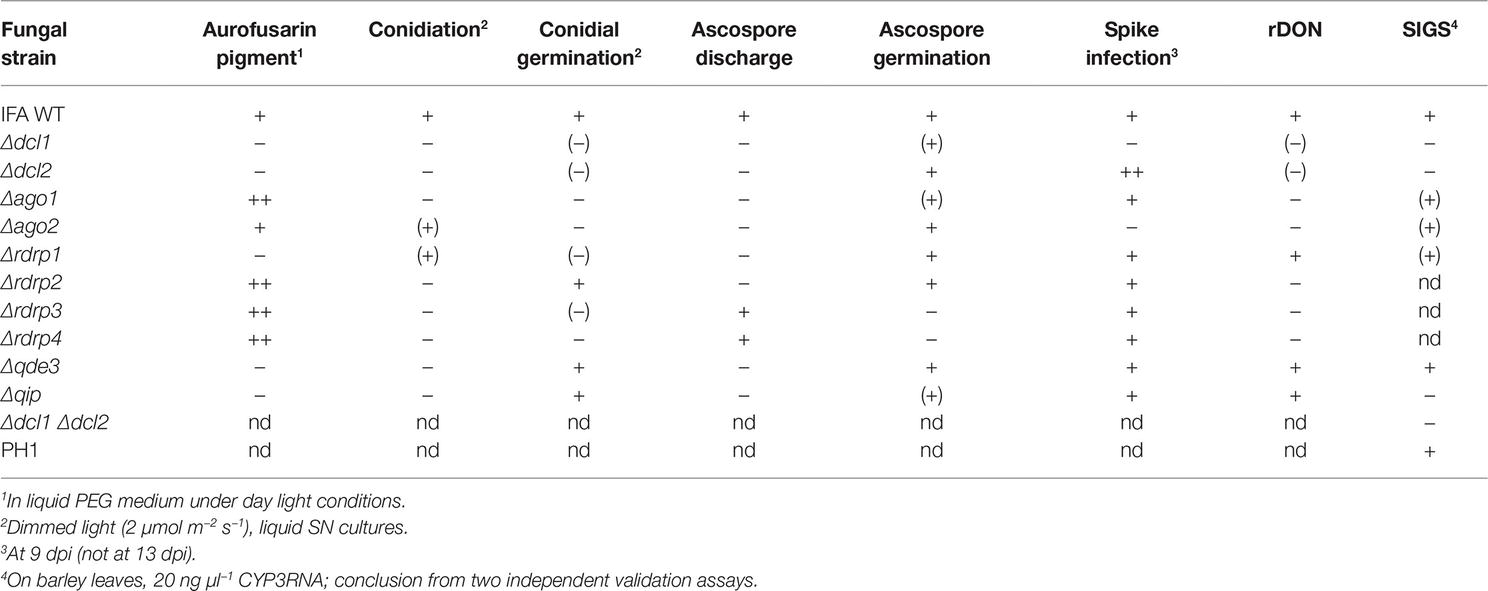
Table 2. Function of Fusarium graminearum RNAi mutants in various developmental and pathogenic processes.
When grown continuously under dimmed light (2 μmol m−2 s−1), liquid SN cultures of RNAi mutants showed significantly reduced conidiation compared to IFA WT, except Δago2 and Δrdrp1, which were only slightly affected (Figure 2A). Under this non-inductive condition, some RNAi mutants also were compromised in conidial germination: Δago1, Δago2, and Δrdrp4 showed significantly reduced germination, while Δrdrp3, Δdcl1, Δrdrp1, and Δdcl2 showed a slight reduction, and rdrp2, Δqip, and Δqde3 showed normal conidial germination (Figure 2B; see Table 2). All RNAi mutants had a normal germ tube morphology, except Δrdrp4, which tends to develop multiple germ tubes (Figure 2C). These results suggest a requirement for Fg RNAi genes in the control of asexual development depending on the environmental conditions.
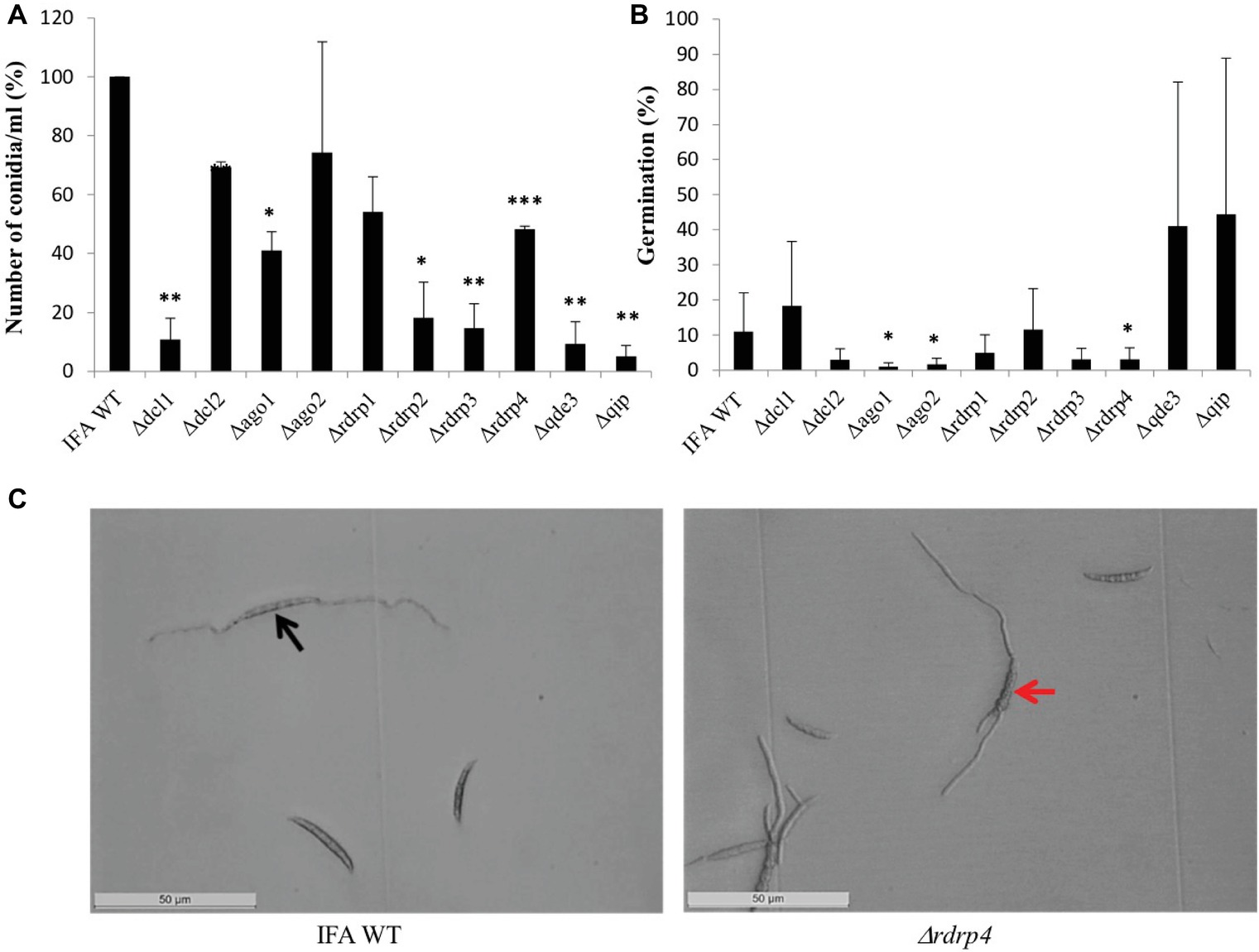
Figure 2. The RNAi pathway is required for asexual development of Fusarium graminearum in the absence of inductive light. (A) Number of conidia produced: means ± SEs of the percentage of conidia numbers from three repeated experiments. Significant differences are marked: *p < 0.05; **p < 0.01; ***p < 0.001 (Student’s t test). (B) Percent of conidial germination: means± SEs of the percentage of germinated spores from three biological repetitions. Significant differences are marked: *p < 0.05 (Student’s t test). (C) Microscopic observation of germinated and non-germinated conidia of IFA WT and Δrdrp4. Imaging after 48 h of incubation in the dark, scale bar: 50 μm. Black arrow, conidia forming a bipolar germ tube. Red arrow, conidia forming multiple germ tubes.
F. graminearum RNA Interference Components Are Required for Sexual Development
Because there were contrasting data in the literature, we resumed asking the question of whether RNAi components are required for sexual reproduction of Fg. To this end perithecia (fruiting bodies) formation was induced in axenic cultures on carrot agar (Cavinder et al., 2012). All RNAi mutants produced melanized mature perithecia to the same extent as compared to IFA WT (not shown). Next, we assessed the forcible discharge of ascospores by a spore discharge assay (Figure 3). Discharge of ascospores from perithecia into the environment results from turgor pressure within the asci; the dispersal of ascospores by forcible discharge is a proxy for fungal fitness as it is important for dissemination of the disease. To this end, half circular agar blocks covered with mature perithecia were placed on glass slides and images from forcibly fired ascospores (white cloudy) were taken after 48 h of incubation in boxes under high humidity and fluorescent light. We found that the forcible discharge of ascospores was severely compromised in Δdcl1, Δago2, Δrdrp1, Δrdrp2, Δqde3, and less severe in Δdcl2, Δago1, Δqip1, while Δrdrp3 and Δrdrp4 were indistinguishable from IFA WT (Figures 3A,B). Microscopic observation of the discharged ascospores revealed that their morphology was not affected (not shown). However, the percentage of discharged ascospores that retained the ability to germinate varied in the mutants with Δrdrp3 and Δrdrp4, showing strong reduction in the ascospore germination (Figure 3C; see Table 2). Together, these results confirm that the RNAi pathway is involved in sexual reproduction, though the requirement of individual RNAi components greatly varies in the different developmental stages.
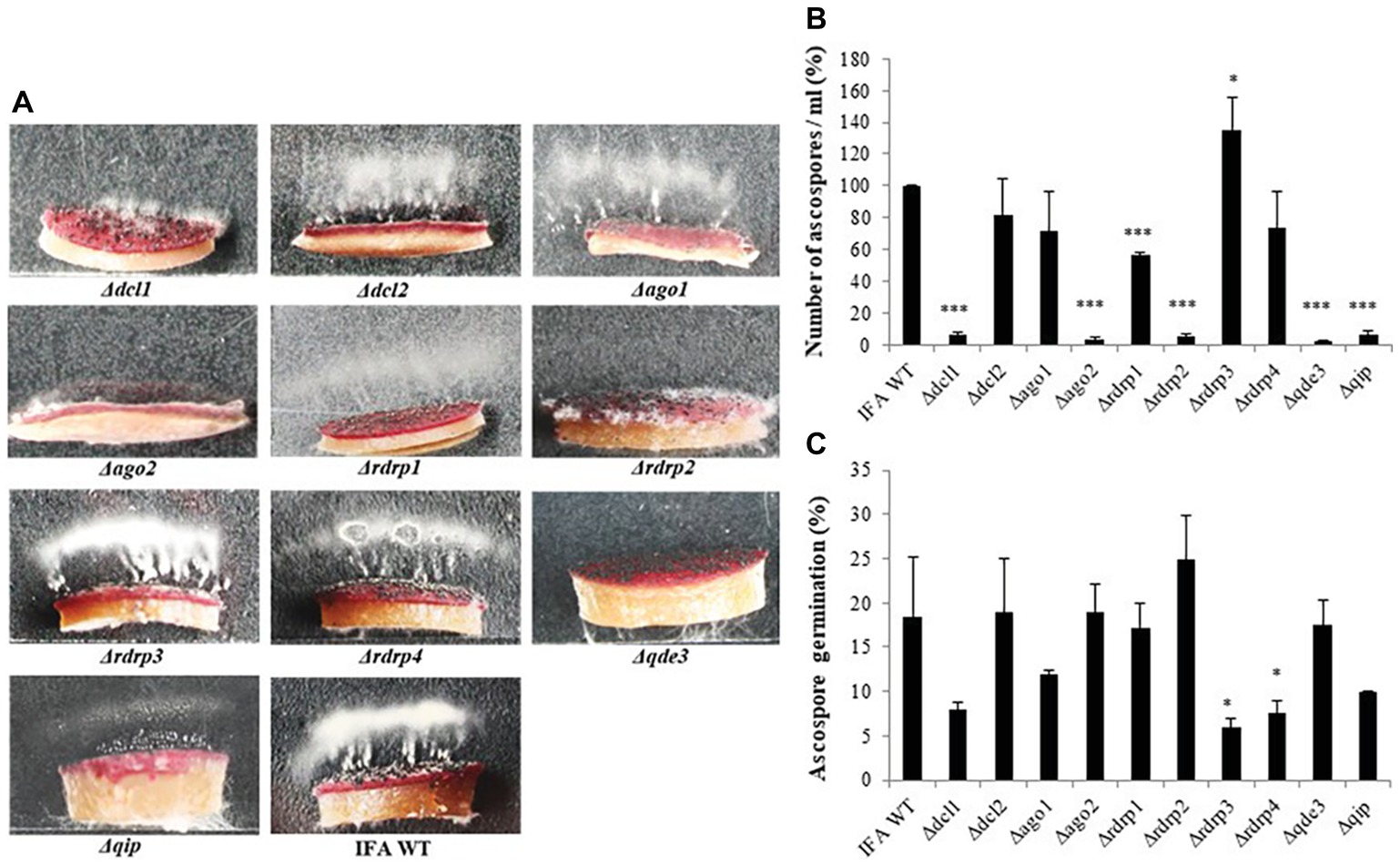
Figure 3. Forcible ascospore discharge in Fusarium graminearum RNAi mutants and IFA WT. (A) Forcible ascospore firing. Half circular carrot agar blocks covered with mature perithecia were placed on glass slides. Photos from forcibly fired ascospores (white cloudy) were taken after 48 h of incubation in boxes under high humidity and fluorescent light. (B) Fired ascospores were washed off and counted. Means± SDs of the counted spores is presented from three biological repetitions. Significant differences are marked: *p < 0.05; ***p < 0.001 (Student’s t test). (C) Ascospore germination. Discharged ascospores were incubated at 100% RT in the dark for 24 h at 23°C in SN liquid medium. The percentage of germination was assessed by examining the ascospore number in three random squares in the counting chamber. Means ± SEs of the percentage of germinated spores from three biological repetitions. Significant differences are marked: *p < 0.05 (Student’s t test).
F. graminearum RNA Interference Mutants Show Variation in Kernel Infection
It has been reported that Fg mutants defective in DCL, AGO, or RdRP were not compromised in virulence on wheat spikes (Chen et al., 2015). We extended this previous study by testing additional Fg RNAi mutants. Conidia were point-inoculated to a single spikelet at the bottom of a spike of the susceptible wheat cultivar Apogee. Fungal colonization was quantified 9 and 13 days post inoculation (dpi) by determining the infection strength. Infected parts of a spike bleached out, whereas the non-inoculated spikes remained greenish. At late infection stages (13 dpi), all RNAi mutants caused strong FHB symptoms comparable with IFA WT. However, we found differences in the severity of infections at earlier time points (9 dpi), with Δdcl1 and Δago2 showing most compromised FHB development (Figure 4A; see Table 2). At 13 dpi, RNAi mutants also showed considerable variation in Fg-infected kernel morphology (Supplementary Figure S4A). Thousand-grain-weight (TGW) of kernels infected with RNAi mutants showed slight, though not significant. Differences in the total weights compared to IFA WT infection (Supplementary Figure S4B).
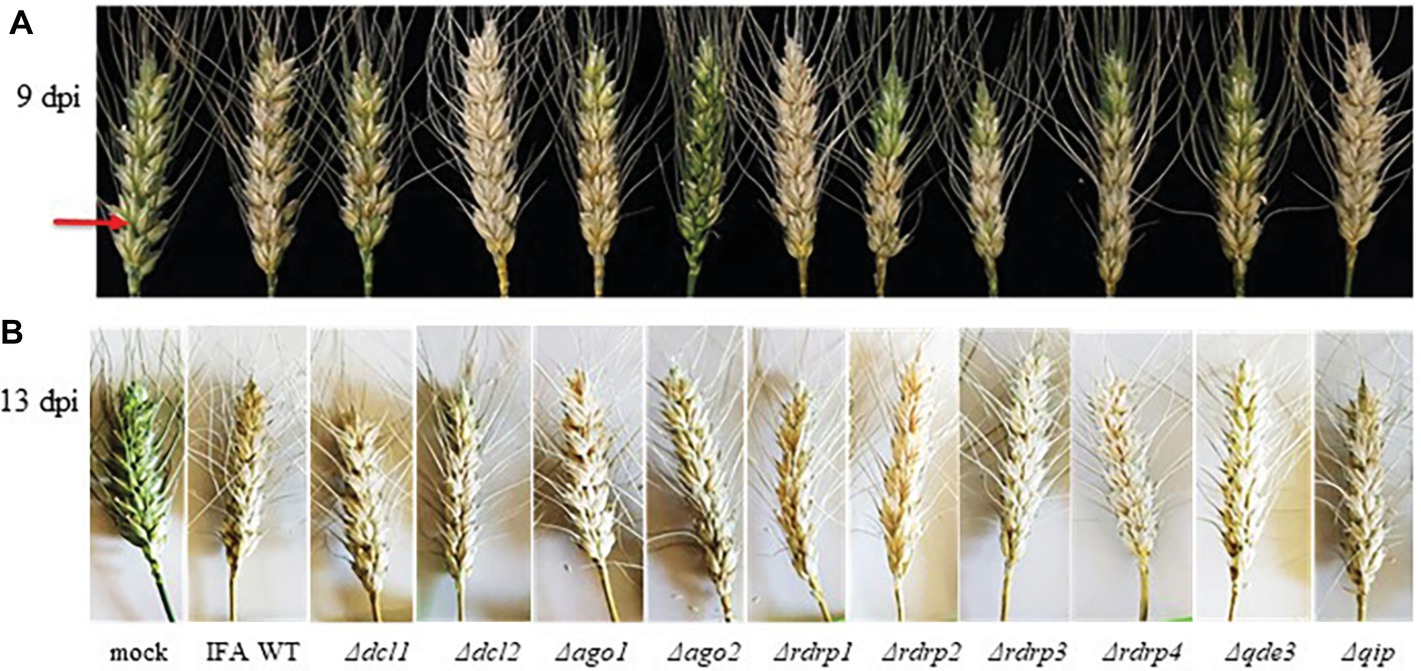
Figure 4. Infection of Apogee wheat spikes with Fusarium graminearum RNAi mutants and IFA WT. (A) Representative samples of spikes at 9 dpi. One spikelet at the bottom of each spike (red arrow) was point-inoculated with 5 μl of 0.002% Tween 20 water containing 40,000 conidia/ml. The assay was repeated two times with 10 spikes per fungal genotype and experiment. (B) Wheat spikes at 13 dpi.
Deoxynivalenol Production Is Compromised in F. graminearum Mutants That Show Reduced Pathogenicity on Wheat Kernels
We quantified the amount of DON in Fg-infected wheat spikes at 13 dpi (point inoculation using 5 μl of 0.002% Tween 20 water containing 40,000 conidia/ml) at mid-anthesis. Of note, the relative amount of DON [rDON], calculated as [DON] relative to the amount of fungal genomic DNA, was reduced in virtually all spikes infected with RNAi mutants, whereby spikes infected by Δqip and Δdcl2 showed the lowest toxin reduction as compared with the other mutants (Table 3). The data suggest that fungal RNAi pathways affect Fg’s DON production in wheat spikes. Moreover, while [rDON] changed, the ratio of [DON] and [A-DON] (comprising 3A-DON and 15A-DON) remained constant in all mutants vs. IFA WT, suggesting that the fungal RNAi pathways do not affect the trichothecene metabolism.
F. graminearum RecQ Helicase Mutant Δqde3 Is Insensitive to Double-Stranded RNA
Spraying plant leaves or fruits with dsRNA targeting essential fungal genes can reduce fungal infections by a mechanism called spray-induced gene silencing (SIGS) (Dalakouras et al., 2016; Koch et al., 2016; Wang et al., 2016b; McLoughlin et al., 2018). We addressed the question which RNAi mutants of our set are compromised in SIGS upon treatment with dsRNA. To this end, we conducted a SIGS experiment on detached barley leaves that were sprayed with 20 ng μl−1 CYP3RNA, a 791 nt-long dsRNA that targets the three fungal genes FgCYP51A, FgCYP51B, and FgCYP51C (Koch et al., 2016). By 48 h after spraying, leaves were drop inoculated with 5 × 104 conidia ml−1 of Fg RNAi mutants and IFA WT. Five days later, infected leaves were scored for disease symptoms and harvested to measure the expression of the fungal target genes by qRT-PCR (Figure 5). As revealed by reduced disease symptoms, leaves sprayed with CYP3RNA vs. TE (buffer control), only Δqde3 was equally sensitive to dsRNA like the IFA WT, while all other mutants tested in this experiment were slightly or strongly compromised in SIGS and less sensitive to CYP3RNA (Figure 5A, see Table 2). Consistent with this, strong down-regulation of all three CYP51 target genes was observed only in IFA WT and Δqde3. In Δdcl1, Δdcl2, and Δqip1, the inhibitory effect of CYP3RNA on FgCYP51A, FgCYP51B, and FgCYP51C expression was completely abolished (Figure 5B). To further substantiate this finding, we tested a dcl1/dcl2 double mutant in Fg strain PH1. As anticipated from the experiments with IFA WT, the PH1 dcl1/dcl2 mutant was fully compromised in SIGS (Figures 5A,B).
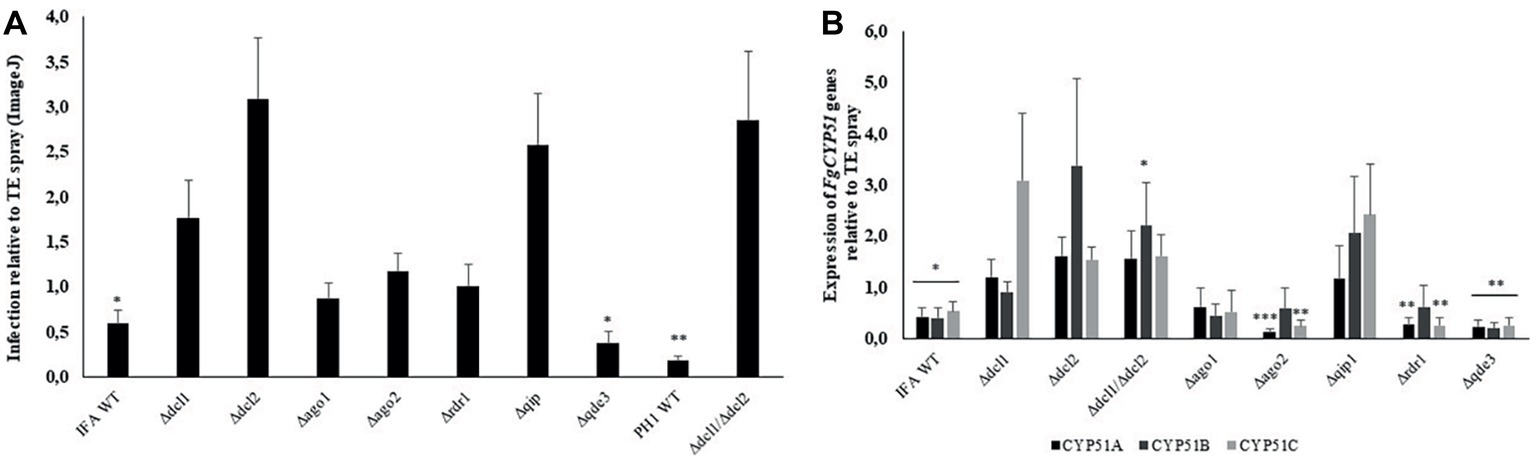
Figure 5. Infection symptoms of Fg RNAi mutants on barley leaves sprayed with the dsRNA CYP3RNA. (A) Detached leaves of 3-week-old barley plants were sprayed with 20 ng μl−1 CYP3RNA or TE buffer, respectively. After 48 h, leaves were drop-inoculated with 5 × 104 conidia ml−1 of indicated Fg RNAi mutants and evaluated for infection symptoms at 5 dpi. Values show relative infection area as calculated from TE- vs. CYP3RNA-treated plants for each RNAi mutant with 10 leaves and three biological repetitions. Asterisks indicate statistical significant reduction of the infection area on CYP3RNA- vs. TE-treated plants measured by ImageJ for each mutant (*p < 0.05; **p < 0.01; Student’s t test). The dcl1 dcl2 double mutant is generated in Fg strain PH1. (B) Down-regulation of the three CYP51 genes in Fg mutants upon colonization of CYP3RNA- vs. TE-treated barley leaves. Asterisks indicate statistically significant down-regulation of CYP51 genes on CYP3RNA vs. TE-treated plants. (*p < 0.05; **p < 0.01; ***p < 0.001; Student’s t test). Error bars indicate SE of three independent experiments in (A) and (B).
Discussion
We generated a broad collection of knockout mutants for RNAi genes in the necrotrophic, mycotoxin-producing pathogen Fusarium graminearum to demonstrate their involvement in vegetative and generative growth, disease development, mycotoxin production, and sensitivity to environmental RNAi. A summary of the mutants’ performance in the various processes is shown in Table 2. While all RNAi mutants show normal vegetative development in axenic cultures, there were differences in pigments production in liquid potato extract glucose cultures. This suggests that in Fg, an RNAi pathway regulates the gene cluster responsible for the biosynthesis of pigments, including aurofusarin. Aurofusarin is a secondary metabolite belonging to the naphthoquinone group of polyketides that shows antibiotic properties against filamentous fungi and yeast (Medentsev et al., 1993). The function of the compound in the fungus is unknown because white mutants have even higher growth rates and are equally pathogenic than a WT fungus on wheat and barley (Malz et al., 2005).
Overall, the contribution of the RNAi pathways to vegetative fungal development and conidiation varies among different fungi and must be considered case by case. Under low light (<2 μmol m−2 s−1), all Fg RNAi mutants showed reduced conidia production and some showed aberrant germination compared to IFA WT. This suggests that in the absence of light induction, the RNAi pathway is required for conidiation. RNAi may play a role in regulation of light-responsive genes affecting conidiation as shown for T. atroviride, where DCL2 and RdRP3 control conidia production under light induction (Carreras-Villaseñor et al., 2013). The authors claimed that Δdcl2 and Δrdrp3 are impaired in perception and/or transduction of the light signal affecting the transcriptional response of light-responsive genes. Similarly, Metarhizium robertsii dcl and ago mutants show reduced abilities to produce conidia under light, though the light quantity was not described (Meng et al., 2017).
Perithecia development has been used to study sexual development and transcription of genes related to sexual development (Trail and Common, 2000; Qi et al., 2006; Hallen et al., 2007). In field situations, ascospores serve as the primary inoculum for FHB epidemics because these spores are shot into the environment and can spread over long distances (Maldonado-Ramirez et al., 2005). We found that all RNAi mutants could produce mature perithecia. However, corroborating and extending the exemplary work of Son et al. (2017), we also found that, besides FgDCL1 and FgAGO2, other RNAi genes such as RdRP1, RdRP2, RdRP3, RdRP4, QDE3, QIP contribute to the sexual reproduction. Mutations in these genes either showed severe defect in forcible ascospore discharge or significantly reduced germination. The Son et al. (2017) study showed that Fgdcl1 and Fgago2 are severely defective in forcible ascospore discharge, while Fgdcl2 and Fgago1 show indistinguishable phenotypes compared to the WT. Active roles for FgDCL1 and FgAGO2 are supported by the finding that expression levels of many genes, including those closely related to the mating-type (MAT)-mediated regulatory mechanism during the late stages of sexual development, was compromised in the respective mutants after sexual induction (Kim et al., 2015). Moreover, FgDCL1 and FgAGO2 participate in the biogenesis of sRNAs and perithecia-specific miRNA-like RNAs (milRNAs) also are dependent on FgDCL1 (Zeng et al., 2018). Most of the produced sRNA originated from gene transcript regions and affected expression of the corresponding genes at a post-transcriptional level (Son et al., 2017).
While our data show that, in addition to FgDCL1 and FgAGO2, more Fg RNAi-related proteins are required for sex-specific RNAi, further transcriptomic analysis and sRNA characterization are needed for a mechanistic explanation. Of note, ex-siRNA functions are important for various developmental stages and stress responses in the fungus M. circinelloides, while Fg utilizes ex-siRNAs for a specific developmental stage. Thus, ex-siRNA-mediated RNAi might occur in various fungal developmental stages and stress responses depending on the fungal species.
We investigated the involvement of RNAi in pathogenicity and FHB development by infecting wheat spikes of the susceptible cultivar Apogee with fungal conidia. At earlier time points of infection (9 dpi), clear differences in virulence between RNAi mutants were observed, though all mutants could spread within a spike and caused typical FHB symptoms at later time points (13 dpi). Despite full FHB symptom development in all mutants at 13 dpi, we observed various effects of fungal infection on the kernel morphology, corresponding to the different aggressiveness of mutants at early time points. Since this phenomenon may account for differences in producing mycotoxins during infection, we quantified mycotoxins in the kernels. Of note, [rDON] was reduced in virtually all spikes infected with RNAi mutants, though most strongly in spikes colonized with mutants Δago1, Δrdrp1, Δrdrp2, Δrdrp3, Δrdrp4, and Δqde3 as compared with IFA WT (see Tables 2 and 3). The data suggest that fungal RNAi pathways affect Fg’s DON production in wheat spikes. Interestingly, while [rDON] changed, the ratio of [DON] and [3A-DON] remained constant in all mutants vs. IFA WT, suggesting that the fungal RNAi pathways do not affect the trichothecene chemotype.
Our work also identifies additional Fg RNAi proteins associated with sensitivity to dsRNA treatments. For the validation of dsRNA effects, we used two independent tests: infection phenotyping and qRT-PCR analysis of fungal target genes. Δdcl1 and Δdcl2 as well as Δqip showed compromised SIGS phenotypes in either test, strongly suggesting that these proteins are required for environmental RNAi in Fg. Further substantiating our finding, the fungal dcl1/dcl2 double mutant of Fg strain PH1 also showed complete insensitivity to dsRNA and thus is fully compromised to environmental RNAi.
Taken together, our results further substantiate the involvement of RNAi pathways in conidiation, ascosporogenesis, and pathogenicity of Fg. Nevertheless, further studies must explore the mechanistic roles of Fg RNAi genes in these processes.
Author Contributions
FG generated the Fusarium mutants and conducted the experiments related to Figures 1–4. K-HK and JI designed the research, analyzed the data, and wrote the manuscript. PK oversaw the DON experiments. AK oversaw the experiments related to Figure 5.
Funding
This research was supported by the German Research Council (DFG) to K-HK and AK in the project GRK2355. FG was supported by the German Academic Exchange Service (DAAD).
Conflict of Interest Statement
The authors declare that the research was conducted in the absence of any commercial or financial relationships that could be construed as a potential conflict of interest.
Acknowledgments
The authors thank Mrs. E. Stein for excellent technical assistance, Dr. A. Rathgeb for mycotoxin analysis, and Ms. C. Birkenstock for taking care of the plants. They also thank Dr. Martin Urban, Rothamsted Research, England for providing the Fg strains PH1 and PH1 dcl1 dcl2.
Supplementary Material
The Supplementary Material for this article can be found online at: https://www.frontiersin.org/articles/10.3389/fmicb.2019.01662/full#supplementary-material
Abbreviations
AGO, ARGONAUTE; CYP51, Cytochrome P450 lanosterol C-14α-demethylase; DCL, DICER-like l; DON, deoxynivalenol; Fg, Fusarium graminearum; FHB, Fusarium head blight; HIGS, host-induced gene silencing; hpRNA, hairpin RNA; MSUD, meiotic silencing by unpaired DNA; NIV, nivalenol; PEG, potato extract glucose; QDE 2,3, Quelling defective 2,3; QIP, QDE-interacting protein; RdRp, RNA-dependent RNA polymerase; RISC, RNA-dependent silencing complex; RNAi, RNA interference; RPA, subunit of replication protein A; siRNA, small interfering RNA; SN, synthetic nutrient agar; ssDNA, single-stranded DNA; TGW, thousand grain weight.
Footnotes
References
Audenaert, K., Vanheule, A., Höfte, M., and Haesaert, G. (2014). Deoxynivalenol: a major player in the multifaceted response of Fusarium to its environment. Toxins 6, 1–19. doi: 10.3390/toxins6010001
Bai, Y., Lan, F., Yang, W., Zhang, F., Yang, K., Li, Z., et al. (2015). sRNA profiling in Aspergillus flavus reveals differentially expressed miRNA-like RNAs response to water activity and temperature. Fungal Genet. Biol. 81, 113–119. doi: 10.1016/j.fgb.2015.03.004
Baulcombe, D. C. (2013). Small RNA-the secret of noble rot. Science 342, 45–46. doi: 10.1126/science.1245010
Brandfass, C., and Karlovsky, P. (2008). Upscaled CTAB-based DNA extraction and real-time PCR assays for Fusarium culmorum and F. graminearum DNA in plant material with reduced sampling error. Int. J. Mol. Sci. 9, 2306–2321. doi: 10.3390/ijms9112306
Brown, N., Evans, A. J., Mead, A., and Hammond-Kosack, K. E. (2017). A spatial temporal analysis of the Fusarium graminearum transcriptome during symptomless and symptomatic wheat infection. Mol. Plant Pathol. 18, 1295–1312. doi: 10.1111/mpp.12564
Cai, Q., He, B., Kogel, K. H., and Jin, H. (2018). Cross-kingdom RNA trafficking and environmental RNAi – nature’s blueprint for modern crop protection strategies. Curr. Opin. Microbiol. 46, 58–64. doi: 10.1016/j.mib.2018.02.003
Calo, S., Nicolás, F. E., Vila, A., Torres-Martínez, S., and Ruiz-Vázquez, R. M. (2012). Two distinct RNA-dependent RNA polymerases are required for initiation and amplification of RNA silencing in the basal fungus Mucor circinelloides. Mol. Microbiol. 83, 379–394. doi: 10.1111/j.1365-2958.2011.07939.x
Campo, S., Gilbert, K. B., and Carrington, J. C. (2016). Small RNA-based antiviral defense in the phytopathogenic fungus Colletotrichum higginsianum. PLoS Pathog. 12:e1005640. doi: 10.1371/journal.ppat.1005640
Carreras-Villaseñor, N., Esquivel-Naranjo, E. U., Villalobos-Escobedo, J. M., Abreu-Goodger, C., and Herrera-Estrella, A. (2013). The RNAi machinery regulates growth and development in the filamentous fungus Trichoderma atroviride. Mol. Microbiol. 89, 96–112. doi: 10.1111/mmi.12261
Cavinder, B., Sikhakolli, U., Fellows, K. M., and Trail, F. (2012). Sexual development and ascospore discharge in Fusarium graminearum. J. Vis. Exp. pii: 3895 doi: 10.3791/3895
Chang, S. S., Zhang, Z., and Liu, Y. (2012). RNA interference pathways in fungi: mechanisms and functions. Annu. Rev. Microbiol. 66, 305–323. doi: 10.1146/annurev-micro-092611-150138
Chen, Y., Gao, Q., Huang, M., Liu, Y., Liu, Z., Liu, X., et al. (2015). Characterization of RNA silencing components in the plant pathogenic fungus Fusarium graminearum. Sci. Rep. 5:12500. doi: 10.1038/srep12500
Cogoni, C., and Macino, G. (1999). Gene silencing in Neurospora crassa requires a protein homologous to RNA-dependent RNA polymerase. Nature 399, 166–169.
Cuomo, C. A., Güldener, U., Xu, J. R., Trail, F., Turgeon, B. G., Di Pietro, A., et al. (2007). The Fusarium graminearum genome reveals a link between localized polymorphism and pathogen specialization. Science 317, 1400–1402. doi: 10.1126/science.1143708
Dalakouras, A., Wassenegger, M., McMillan, J. N., Cardoza, V., Maegele, I., Dadami, E., et al. (2016). Induction of silencing in plants by high-pressure spraying of in vitro-synthesized small RNAs. Front. Plant Sci. 7:1327. doi: 10.3389/fpls.2016.01327
Dang, Y., Yang, Q., Xue, Z., and Liu, Y. (2011). RNA interference in fungi: pathways, functions, and applications. Eukaryot. Cell 10, 1148–1155. doi: 10.1128/EC.05109-11
Dean, R., Van Kan, J. A., Pretorius, Z. A., Hammond-Kosack, K. E., Di Pietro, A., Spanu, P. D., et al. (2012). The top 10 fungal pathogens in molecular plant pathology. Mol. Plant Pathol. 13, 414–430. doi: 10.1111/j.1364-3703.2011.00783.x
Desjardins, A. E., Hohn, T. M., and McCormick, S. P. (1993). Trichothecene biosynthesis in Fusarium species: chemistry, genetics and significance. Microbiol. Rev. 57, 595–604.
Dill-Macky, R., and Jones, R. K. (2000). The effect of previous crop residues and tillage on Fusarium head blight of wheat. Plant Dis. 84, 71–76. doi: 10.1094/PDIS.2000.84.1.71
Fire, A., Xu, S., Montgomery, M. K., Kostas, S. A., Driver, S. E., and Mello, C. C. (1998). Potent and specific genetic interference by double-stranded RNA in Caenorhabditis elegans. Nature 391, 806–811. doi: 10.1038/35888
Frandsen, R. J., Nielsen, N. J., Maolanon, N., Sørensen, J. C., Olsson, S., Nielsen, J., et al. (2006). The biosynthetic pathway for aurofusarin in Fusarium graminearum reveals a close link between the naphthoquinones and naphthopyrones. Mol. Microbiol. 61, 1069–1080. doi: 10.1111/j.1365-2958.2006.05295.x
Gosman, N., Steed, A., Chandler, E., Thomsett, M., and Nicholson, P. (2010). Evaluation of type I Fusarium head blight resistance of wheat using non-deoxynivalenol-producing fungi. Plant Pathol. 59, 147–157. doi: 10.1111/j.1365-3059.2009.02202.x
Hallen, H., Huebner, M., Shiu, S. H., Guldener, U., and Trail, F. (2007). Gene expression shifts during perithecium development in Gibberella zeae (anamorph Fusarium graminearum), with particular emphasis on ion transport proteins. Fungal Genet. Biol. 44, 1146–1156. doi: 10.1016/j.fgb.2007.04.007
Hammond, S. M. (2005). Dicing and slicing: the core machinery of the RNA interference pathway. FEBS Lett. 579, 5822–5829. doi: 10.1016/j.febslet.2005.08.079
Harris, L. J., Balcerzak, M., Johnston, A., Schneiderman, D., and Ouellet, T. (2016). Host-preferential Fusarium graminearum gene expression during infection of wheat, barley, and maize. Fungal Biol. 120, 111–123. doi: 10.1016/j.funbio.2015.10.010
Ilgen, P., Hadeler, B., Maier, F. J., and Schäfer, W. (2009). Developing kernel and rachis node induce the trichothecene pathway of Fusarium graminearum during wheat head infection. Mol. Plant Microbe Interact. 22, 899–908. doi: 10.1094/MPMI-22-8-0899
Jansen, C., von Wettstein, D., Schäfer, W., Kogel, K. H., Felk, F., and Maier, F. J. (2005). Infection patterns in barley and wheat spikes inoculated with wild type and trichodiene synthase gene disrupted Fusarium graminearum. Proc. Natl. Acad. Sci. USA 102, 16892–16897. doi: 10.1073/pnas.0508467102
Kim, H. K., Jo, S. M., Kim, G. Y., Kim, D. W., Kim, Y. K., and Yun, S. H. (2015). A large-scale functional analysis of putative target genes of mating-type loci provides insight into the regulation of sexual development of the cereal pathogen Fusarium graminearum. PLoS Genet. 11:e1005486. doi: 10.1371/journal.pgen.1005486
Klittich, C. J. R., and Leslie, J. F. (1988). Nitrate reduction mutants of Fusarium moniliforme (Gibberella fujikuroi). Genetics 118, 417–423.
Koch, A., Biedenkopf, D., Furch, A., Weber, L., Rossbach, O., Abdellatef, E., et al. (2016). An RNAi-Based Control of Fusarium graminearum infections through spraying of Long dsRNAs involves a plant passage and is controlled by the fungal silencing machinery. PLoS Pathog. 12:e1005901. doi: 10.1371/journal.ppat.1005901
Koch, A., and Kogel, K. H. (2014). New wind in the sails: improving the agronomic value of crop plants through RNAi-mediated gene silencing. Plant Biotechnol. J. 12, 821–831. doi: 10.1111/pbi.12226
Koch, A., Kumar, N., Weber, L., Keller, H., Imani, J., and Kogel, K. H. (2013). Host-induced gene silencing of cytochrome P450 lanosterol C14α-demethylase–encoding genes confers strong resistance to Fusarium species. Proc. Natl. Acad. Sci. USA 110, 19324–19329. doi: 10.1073/pnas.1306373110
Koch, A., Stein, E., and Kogel, K.-H. (2018). RNA-based disease control as a complementary measure to fight Fusarium fungi through silencing of the Azole target cytochrome P450 lanosterol C-14 α-demethylase. Eu. J. Plant Pathol. 152, 1003–1010. doi: 10.1007/s10658-018-1518-4
Kusch, S., Frantzeskakis, L., Thieron, H., and Panstruga, R. (2018). Small RNAs from cereal powdery mildew pathogens may target host plant genes. Fungal Biol. 122, 1050–1063. doi: 10.1016/j.funbio.2018.08.008
Lau, S. K., Tse, H., Chan, J. S., Zhou, A. C., Curreem, S. O., Lau, C. C., et al. (2013). Proteome profiling of the dimorphic fungus Penicillium marneffei extracellular proteins and identification of glyceraldehyde-3-phosphate dehydrogenase as an important adhesion factor for conidial attachment. FEBS J. 280, 6613–6626. doi: 10.1111/febs.12566
Leslie, J. F., and Summerell, B. A. (2006). The Fusarium laboratory manual. Ames, IA, USA: Blackwell Professional. ISBN: 978-0-813-81919-8.
Livak, K. J., and Schmittgen, T. D. (2001). Analysis of relative gene expression data using real-time quantitative PCR and the 2∆∆C(T). Methods 25, 402–408. doi: 10.1006/meth.2001.1262
Maldonado-Ramirez, S. L., Schmale, D. G. Jr., Shields, E. J., and Bergstrom, G. C. (2005). The relative abundance of viable spores of Gibberella zeae in the planetary boundary layer suggests the role of long-distance transport in regional epidemics of Fusarium head blight. Agric. For. Meteorol. 132, 20–27. doi: 10.1016/j.agrformet.2005.06.007
Malz, S., Grell, M. N., Thrane, C., Maier, F. J., Rosager, P., Felk, A., et al. (2005). Identification of a gene cluster responsible for the biosynthesis of aurofusarin in the Fusarium graminearum species complex. Fungal Genet. Biol. 42, 420–433. doi: 10.1016/j.fgb.2005.01.010
McLoughlin, A. G., Wytinck, N., Walker, P. L., Girard, I. J., Rashid, K. Y., de Kievit, T., et al. (2018). Identification and application of exogenous dsRNA confers plant protection against Sclerotinia sclerotiorum and Botrytis cinerea. Sci. Rep. 8:7320. doi: 10.1038/s41598-018-25434-4
Medentsev, A. G., Kotik, A. N., Trufanova, V. A., and Akimenko, V. K. (1993). Identification of aurofusarin in Fusarium graminearum isolates, causing a syndrome of worsening of egg quality in chickens. Prikl. Biokhim. Mikrobiol. 29, 542–546.
Mello, C. C., and Conte, D. Jr. (2004). Revealing the world of RNA interference. Nature 431, 338–342. doi: 10.1038/nature02872
Meng, H., Wang, Z., Wang, Y., Zhu, H., and Huang, B. (2017). Dicer and Argonaute genes involved in RNA interference in the entomopathogenic fungus Metarhizium robertsii. Appl. Environ. Microbiol. 83:e03230-16. doi: 10.1128/AEM.03230-16
Nguyen, Q., Iritani, A., Ohkita, S., Vu, B. V., Yokoya, K., Matsubara, A., et al. (2018). A fungal Argonaute interferes with RNA interference. Nucleic Acids Res. 46, 2495–2508. doi: 10.1093/nar/gkx1301
Nowara, D., Gay, A., Lacomme, C., Shaw, J., Ridout, C., Douchkov, D., et al. (2010). HIGS: host-induced gene silencing in the obligate biotrophic fungal pathogen Blumeria graminis. Plant Cell 22, 3130–3141. doi: 10.1105/tpc.110.077040
Qi, W., Kwon, C., and Trail, F. (2006). Microarray analysis of transcript accumulation during perithecium development in Gibberella zeae (anamorph Fusarium graminearum). Mol. Gen. Genomics. 276, 87–100. doi: 10.1007/s00438-006-0125-9
Raman, V., Simon, S. A., Demirci, F., Nakano, M., Meyers, B. C., and Donofrio, N. M. (2017). Small RNA functions are required for growth and development of Magnaporthe oryzae. Mol. Plant Microbe Interact. 30, 517–530. doi: 10.1094/MPMI-11-16-0236-R
Romano, N., and Macino, G. (1992). Quelling: transient inactivation of gene expression in Neurospora crassa by transformation with homologous sequences. Mol. Microbiol. 6, 3343–3353. doi: 10.1111/j.1365-2958.1992.tb02202.x
Segers, G. C., Zhang, X., Deng, F., Sun, Q., and Nuss, D. L. (2007). Evidence that RNA silencing functions as an antiviral defense mechanism in fungi. Proc. Natl. Acad. Sci. USA 104, 12902–12906. doi: 10.1073/pnas.0702500104
Shiu, P. K., Raju, N. B., Zickler, D., and Metzenberg, R. L. (2001). Meiotic silencing by unpaired DNA. Cell 107, 905–916. doi: 10.1016/S0092-8674(01)00609-2
Son, H., Min, K., Lee, J., Raju, N. B., and Lee, Y. W. (2011). Meiotic silencing in the homothallic fungus Gibberella zeae. Fungal Biol. 115, 1290–1302. doi: 10.1016/j.funbio.2011.09.006
Son, H., Park, A. R., Lim, J. Y., Shin, C., and Lee, Y. W. (2017). Genome-wide exonic small interference RNA-mediated gene silencing regulates sexual reproduction in the homothallic fungus Fusarium graminearum. PLoS Genet. 13:e1006595. doi: 10.1371/journal.pgen.1006595
Song, M. S., and Rossi, J. J. (2017). Molecular mechanisms of Dicer: endonuclease and enzymatic activity. Biochem. J. 474, 1603–1618. doi: 10.1042/BCJ20160759
Sulyok, M., Berthiller, F., Krska, R., and Schuhmacher, R. (2006). Development and validation of a liquid chromatography/tandem mass spectrometric method for the determination of 39 mycotoxins in wheat and maize. Rapid Commun. Mass Spectrom. 20, 2649–2659. doi: 10.1002/rcm.2640
Torres-Martínez, S., and Ruiz-Vázquez, R. M. (2017). The RNAi universe in fungi: a varied landscape of small RNAs and biological functions. Annu. Rev. Microbiol. 71, 371–391. doi: 10.1146/annurev-micro-090816-093352
Trail, F., and Common, R. (2000). Perithecial development by Gibberella zeae: a light microscopy study. Mycologia 92, 130–138. doi: 10.2307/3761457
Urban, M., King, R., Hassani-Pak, K., and Hammond-Kosack, K. E. (2015). Whole-genome analysis of Fusarium graminearum insertional mutants identifies virulence associated genes and unmasks untagged chromosomal deletions. BMC Genomics 16:261. doi: 10.1186/s12864-015-1412-9
Vervliet, G., Holsters, M., Teuchy, H., Van Montagu, M., and Schell, J. (1975). Characterization of different plaque-forming and defective temperate phages in Agrobacterium strains. J. Gen. Virol. 26, 33–48. doi: 10.1099/0022-1317-26-1-33
Wang, S., Li, P., Zhang, J., Qiu, D., and Guoa, L. (2016a). Generation of a high resolution map of sRNAs from Fusarium graminearum and analysis of responses to viral infection. Sci. Rep. 6:26151. doi: 10.1038/srep26151
Wang, M., Weiberg, A., Lin, F. M., Thomma, B. P., Huang, H. D., and Jin, H. (2016b). Bidirectional cross-kingdom RNAi and fungal uptake of external RNAs confer plant protection. Nature Plants 2:16151. doi: 10.1038/nplants.2016.151
Weiberg, A., Wang, M., Lin, F.-M., Zhao, H., Zhang, Z., Kaloshian, I., et al. (2013). Fungal small RNAs suppress plant immunity by hijacking host RNA interference pathways. Science 342, 118–123. doi: 10.1126/science.1239705
Yun, Y., Liu, Z., Yin, Y., Jiang, J., Chen, Y., Xu, J. R., et al. (2015). Functional analysis of the Fusarium graminearum phosphatome. New Phytol. 207, 119–134. doi: 10.1111/nph.13374
Zanini, S., Šečić, E., Busche, T., Kalinowski, T., and Kogel, K.- H. (2019). Discovery of interaction-related sRNAs and their targets in the Brachypodium distachyon and Magnaporthe oryzae pathosystem. BioRxiv [Preprint]. doi: 10.1101/631945
Zanini, S., Šečić, E., Jelonek, L., and Kogel, K.-H. (2018). A bioinformatics pipeline for the analysis and target prediction of RNA effectors in bidirectional communication during plant-microbe interactions. Front. Plant Sci. 9:1212.doi: 10.3389/fpls.2018.01212
Zeng, W., Wang, J., Wang, Y., Lin, J., Fu, Y., Xie, J., et al. (2018). Dicer-like proteins regulate sexual development via the biogenesis of perithecium-specific microRNAs in a plant pathogenic fungus Fusarium graminearum. Front. Microb. 9:818. doi: 10.3389/fmicb.2018.00818
Keywords: Argonaute, double-stranded RNA, small RNA, Fusarium graminearum, spray-induced gene silencing, wheat
Citation: Gaffar FY, Imani J, Karlovsky P, Koch A and Kogel K-H (2019) Different Components of the RNA Interference Machinery Are Required for Conidiation, Ascosporogenesis, Virulence, Deoxynivalenol Production, and Fungal Inhibition by Exogenous Double-Stranded RNA in the Head Blight Pathogen Fusarium graminearum. Front. Microbiol. 10:1662. doi: 10.3389/fmicb.2019.01662
Edited by:
David B. Collinge, University of Copenhagen, DenmarkReviewed by:
Liao Yucai, Huazhong Agricultural University, ChinaLi-Jun Ma, University of Massachusetts Amherst, United States
Copyright © 2019 Gaffar, Imani, Karlovsky, Koch and Kogel. This is an open-access article distributed under the terms of the Creative Commons Attribution License (CC BY). The use, distribution or reproduction in other forums is permitted, provided the original author(s) and the copyright owner(s) are credited and that the original publication in this journal is cited, in accordance with accepted academic practice. No use, distribution or reproduction is permitted which does not comply with these terms.
*Correspondence: Karl-Heinz Kogel, a2FybC1oZWluei5rb2dlbEBhZ3Jhci51bmktZ2llc3Nlbi5kZQ==
†These authors have contributed equally to this work