- 1Department of Biology, Lund University, Lund, Sweden
- 2Department of Biology and Biological Engineering, Systems and Synthetic Biology, Chalmers University of Technology, Gothenburg, Sweden
- 3Department of Microbial Pathogenicity Mechanisms, Hans Knöll Institute, Jena, Germany
- 4Institute of Microbiology, Friedrich Schiller University, Jena, Germany
- 5Department of Nephrology, Lund University, Lund, Sweden
- 6Department of Molecular Genetics, Genomics and Microbiology, Strasbourg University, Strasbourg, France
- 7Department of Biology, Faculty of Science, University of Copenhagen, Copenhagen, Denmark
- 8Lund Protein Production Platform, Lund University, Lund, Sweden
The yeast Candida glabrata is a major opportunistic pathogen causing mucosal and systemic infections in humans. Systemic infections caused by this yeast have high mortality rates and are difficult to treat due to this yeast’s intrinsic and frequently adapting antifungal resistance. To understand and treat C. glabrata infections, it is essential to investigate the molecular basis of C. glabrata virulence and resistance. We established an RNA interference (RNAi) system in C. glabrata by expressing the Dicer and Argonaute genes from Saccharomyces castellii (a budding yeast with natural RNAi). Our experiments with reporter genes and putative virulence genes showed that the introduction of RNAi resulted in 30 and 70% gene-knockdown for the construct-types antisense and hairpin, respectively. The resulting C. glabrata RNAi strain was used for the screening of a gene library for new virulence-related genes. Phenotypic profiling with a high-resolution quantification of growth identified genes involved in the maintenance of cell integrity, antifungal drugs, and ROS resistance. The genes identified by this approach are promising targets for the treatment of C. glabrata infections.
Introduction
The yeast Candida glabrata is an opportunistic human pathogen that causes relatively benign mucosal or fatal systemic infections. The incidence of infections caused by this Candida species has significantly increased particularly in immune-deficient patients, in addition to those that undergo chemotherapy, treated with broad-spectrum antibiotics for prolonged time, or have undergone a higher number of invasive surgeries (Pfaller and Diekema, 2007). In healthy humans, Candida species colonize the oral cavity, vagina, and gut (Mårdh et al., 2002; Moyes and Naglik, 2011). In many studies, C. glabrata and C. albicans are considered the most common causes of candidiasis (Fidel et al., 1999; Brunke and Hube, 2013). While C. glabrata is a distant relative of C. albicans, it is a close relative of bakers’ yeast Saccharomyces cerevisiae (Dujon et al., 2004). In evolutionary time scales, C. glabrata only “recently” became pathogenic (Brunke and Hube, 2013; Ahmad et al., 2014). The mechanisms behind C. glabrata pathogenicity are thus far not well understood, but appear to differ greatly from C. albicans. Immune evasion strategies, possibly via intracellular survival and replication in macrophages contribute to the virulence of C. glabrata (Seider et al., 2011, 2014; Brunke and Hube, 2013; Kasper et al., 2014). C. glabrata also readily rearranges its genome (Ahmad et al., 2014) at a frequency one order of magnitude higher than in C. albicans (Gabaldón and Fairhead, 2018). Genome rearrangements could be evolutionary advantageous for this yeast, allowing it to adapt rapidly to its host environment and to antifungal treatments.
Research focused on C. glabrata pathogenicity can benefit greatly from the development of molecular tools. In S. cerevisiae, the strategy of gene deletion has been used for decades as an approach to study gene function. However, Giaever et al. (2002) found that approximately 1000 mutants (representing ∼17% of all S. cerevisiae genes) failed to grow as a result of a specific gene deletion in haploids, proving that these genes are essential for this yeast. The genome of C. glabrata has 5283 predicted coding sequences, and extensive research has been devoted to the creation of a whole genome deletion library for this haploid yeast (Schwarzmüller et al., 2014). Large-scale gene deletions for C. glabrata proved less feasible than for S. cerevisiae due to gene essentiality or technical reasons (lower homologous recombination rates). However it must be assumed that C. glabrata contains a similar number of essential genes as S. cerevisiae.
Other techniques, such as down-regulating gene expression can provide alternative ways to study genes’ functions. The RNA interference (RNAi), which relies on manipulating the levels of a gene’s transcript, has become a widespread tool to analyze the function of genes in organisms ranging from protozoa to human. Unlike gene deletions, RNAi can be applied to any gene, even those that are essential. It has, however, not found many applications in baker’s yeast, which naturally lack important components of the RNAi machinery (Drinnenberg et al., 2009). The RNAi approach relies on the activity of two proteins, Dicer (ribonuclease III) and Argonaute (the carrier of small interfering RNA [siRNAs]). The process is initiated by the cleavage of double stranded RNA (complementary to the target transcript) by Dicer into siRNAs, which are then loaded on Argonaute and, by base-pair interaction, target the gene’s transcript. This ultimately interferes with gene expression by down-regulation. RNAi is found widely in nature (plants, animals, and fungi), and provides evolutionary advantages by protecting these organisms from viruses, taking part in gene silencing, heterochromatin organization, and chromosome segregation (Tijsterman et al., 2002; Martienssen et al., 2005; Moazed, 2009).
Remarkably, although RNAi is well preserved among some fungi (Drinnenberg et al., 2009), it has been lost in several budding yeasts such as S. cerevisiae and C. glabrata. The aim of this study was to develop a RNAi system for C. glabrata and to use it as a tool to identify and functionally analyze genes known to be putatively required for virulence.
Results
Introduction of RNAi Into C. glabrata
For the reconstitution of an RNAi mechanism in C. glabrata, two heterologous Saccharomyces castellii genes, DCR1 and AGO1 (coding for Dicer and Argonaute, correspondingly) were introduced into the C. glabrata genome. For this purpose we first evaluated the activity of several constitutive and inducible promoters by their ability to induce expression of a functional enzyme, deoxyribonucleoside kinase of Drosophila melanogaster (Supplementary Figure S1, Methods). The dNK gene encoding this enzyme was successfully expressed in C. glabrata and different enzyme activity was observed when expressed from different promoters (Supplementary Figure S1). For the expression of DCR1 and AGO1 genes in the C. glabrata, we used the strong constitutive promoters TEF1 and TDH3 of C. glabrata (Figure 1A and Supplementary Figure S1). The plasmid carrying the DCR1 and AGO1 constructs (P1062, Supplementary Table S2) was linearized by SphI and integrated into the genome of three standard laboratory C. glabrata strains, BG2, CBS138 and BG14 (Table 1).
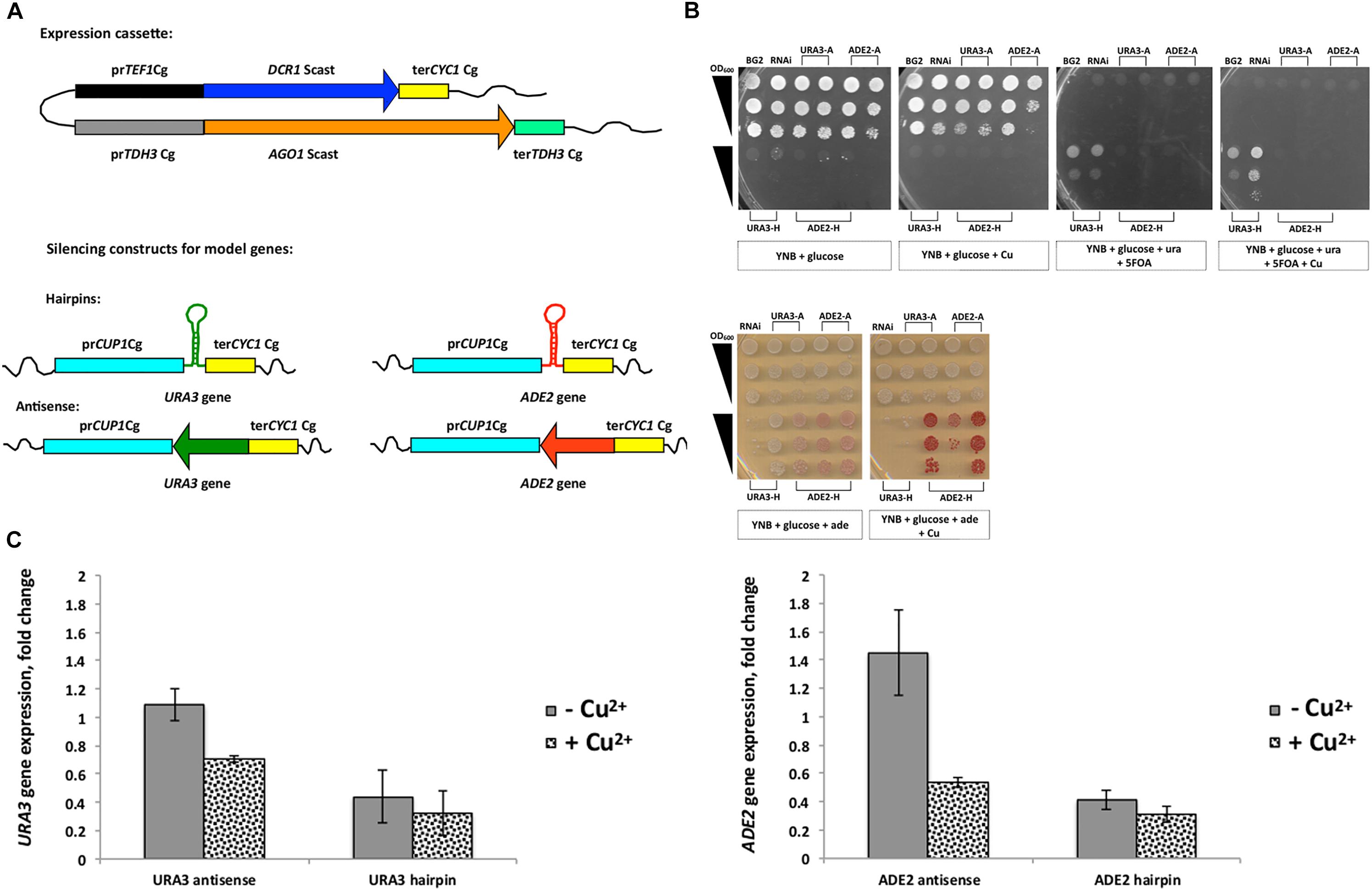
Figure 1. Introduction of RNA interference (RNAi) into C. glabrata. (A) Schematic view of the plasmid carrying the expression cassettes for the DCR1 and AGO1 genes of S. castellii (P1062), which was integrated into the genome; the DCR1 cassette consisted of the promoter TEF1 and the terminator CYC1 of C. glabrata; the AGO1 cassette consisted of the promoter TDH3 and the terminator TDH3 of C. glabrata; the silencing constructs (antisense and hairpins) targeting two model genes of the C. glabrata genome, URA3 and ADE2, were expressed under control of the inducible promoter CUP1 with the terminator CYC1, both of C. glabrata. (B) Growth phenotypes of C. glabrata transformant carrying RNAi constructs for URA3 and ADE2 genes (hairpin [H] and antisense [A]). Serial dilutions of cells (OD600 1; 0.1; 0.01) were plated on different media with and without copper (YNB; YNB with adenine; YNB with 5-FOA and uracil); Strains are as follows: 1 – BG2; 2 – Y1662 carrying P1028 (RNAi negative control); 3 – URA3 antisense-8; 4 – URA3 antisense-12; 5 – ADE2 antisense-5; 6 – ADE2 antisense-10; 7 – URA3 hairpin-4; 8 – URA3 hairpin-7; 9 – ADE2 hairpin-3; 10 – ADE2 hairpin-4; 11 – ADE2 hairpin-10. (C) Expression analysis of ADE2 and URA3 genes in RNAi transformants performed by qRT-PCR. Gene expression was induced by addition of copper to the medium. Strains: control (Y1662 carrying P1028); ADE2 antisense; ADE2 hairpin; URA3 antisense; URA3 hairpin. The error bars represent standard deviation of two replicates. Corresponding primer pairs were used with cDNA: ACT1 gene (OP33 and OP34), ADE2 (OP31 and OP32), URA3 (OP27 and OP28).
To first prove that the RNAi pathway can function in C. glabrata, we generated silencing constructs targeting the two genes URA3 (encoding orotidine 5′-phosphate decarboxylase) and ADE2 (encoding phosphoribosylaminoimidazole carboxylase) in the C. glabrata genome. They are part of the uracil (URA3 gene) and adenine (ADE2 gene) biosynthesis pathway and are popular auxotrophic markers in yeast genetics. Lack or loss of function of these genes products result in following phenotypes: requirement of uracil/adenine for growth, accumulation of p-ribosylaminoimidazolecarboxylate (red pigment) in the absence of ADE2 transcript, and resistance to 5-FOA without URA3. We used two different silencing constructs for these genes (antisense and hairpin), which we introduced into the lys2 auxotrophic variant of BG2 carrying RNAi genes (Y1662, Table 1) by transforming it with integrative plasmids. The design of silencing constructs was similar to that used by Drinnenberg et al. (2009), were the antisense constructs consisted of 339 bp of antisense DNA strand of the gene and hairpins had 339 bp of both antisense and sense DNA strand separated by 79 bp “loop” (Supplementary Data). SalI linearized the plasmids with antisense constructs, and SmaI linearized hairpin plasmids before the transformation. For the expression of silencing constructs we selected the strong inducible C. glabrata promoter CUP1 (Figure 1A and Supplementary Figure S1).
Two to three selected transformants of the RNAi strain carrying different silencing constructs were grown on different selective media (Figure 1B). We observed a decreased growth among transformants carrying antisense constructs (URA3, ADE2) under conditions of CUP1 promoter induction (medium supplemented with copper) on minimal medium lacking uracil or adenine. For hairpin constructs (URA3, ADE2), a growth inhibition by several orders of magnitudes was observed with and without CUP1 promoter induction. Apparently, the CUP1 promoter was leaky (Supplementary Figure S1) and the hairpin constructs had a strong effect on gene expression. In agreement with this, silencing of the URA3 gene with the hairpins allowed growth on 5-FOA (Figure 1B), a compound, which is toxic to cells with an active URA3 gene. Further, ADE2 silencing with hairpins resulted in accumulation of the red pigment P-ribosylamino imidazole on adenine-limited media (10 mg/L), which indicates a block in adenine synthesis (Figure 1B). These results show a strong silencing of model target genes by both URA3 and ADE2 hairpin constructs.
To confirm our phenotypic observations, the expression of URA3 and ADE2 genes was studied by qRT-PCR in transformants carrying either antisense or hairpin silencing constructs (Figure 1C). Compared to the control strain, both ADE2 antisense and URA3 antisense constructs caused 1.4-times and 2-times down-regulation, respectively, of their target genes specifically in the medium with copper (Figure 1C). In contrast, gene expression in transformants with hairpin constructs was repressed further than that even in the medium without copper, with only slight additional increase in the presence of Cu ions (Figure 1C).
The experiment showed that the reconstituted RNAi pathway in C. glabrata is functional and can be applied for gene silencing in this yeast.
Knock-Down of a Known Virulence Gene
In addition to model genes, we designed silencing constructs for PUP1/CAGL0M12947g gene of C. glabrata to be tested in our RNAi system in C. glabrata CBS 138 strain. The deletion of this gene, encoding a mitochondria localized protein, decreases the virulence of azole resistant strain DSY565 of C. glabrata in an immuno-compromised mouse model (Vermitsky et al., 2006; Ferrari et al., 2011). Both DSY565 and CBS 138 strains are fluconazole resistant. As estimated in our lab, the CBS138 strain has fluconazole MIC of 129 mg/L, which is higher than that of DSY565 (fluconazole MIC of 64 mg/L, Vale-Silva et al., 2017). To achieve a stable integration for silencing constructs in the genome, we targeted the 18S rDNA locus of C. glabrata CBS 138 strain with RNAi. For a constitutive expression level, the promoter PGK1 of C. glabrata was selected (Supplementary Figure S1).
We constructed two recombinant plasmids carrying antisense or hairpin constructs for the PUP1 (Supplementary Table S2). These vectors (P1125 and P1151) were linearized with SacII (this restriction site is present in the 18S rDNA sequence) and transformed into CBS 138-based C. glabrata master strain Y1699 carrying both DCR1 and AGO1 genes (Table 1). The plasmid linearization in 18S rDNA region generated homologous regions for ends-in homologous recombination with 18S rDNA loci. To check the stability of the plasmids’ integration into the genome, the recombinant colonies were grown for several generations on non-selective YPD medium. The strains were analyzed by PCR to confirm the presence of antisense or hairpin “bullets” and integration of both DCR1 and AGO1. Then 90% of all strains carrying the inserts were stable for 60 generations.
Using this approach, we constructed two strains, Y1843 and Y2172, which carry antisense and hairpin constructs for the C. glabrata PUP1 gene, respectively. As survival in macrophages may be one important virulence determinant of C. glabrata (Brunke and Hube, 2013), we used a macrophage-confrontation assay for the analysis of fitness of our mutant. In this macrophage confrontation assay, the strains carrying the PUP1 antisense and hairpin constructs showed decreased intracellular survival of 42% (for antisense construct) and 29% (for hairpin construct) of their mean values compared to the empty-vector control strain Y1848 (Figure 2). While the silencing constructs reduced survival comparing to the control (ANOVA, p = 0.02 and p = 0.1), the difference between the antisense and hairpin transformants survival was not significantly different (ANOVA, p = 0.42). Compared to the control strain, the PUP1 gene expression was 10-fold lower in the PUP1 antisense strain, and 1000-fold lower in PUP1 hairpin strain (Supplementary Figure S2). The ratio of gene size to antisense region was ∼2. Our results of the down regulation of PUP1 gene by silencing constructs prove that our RNAi system can be used to study gene functions in an infection model and show that PUP1 is required for C. glabrata survival in macrophages.
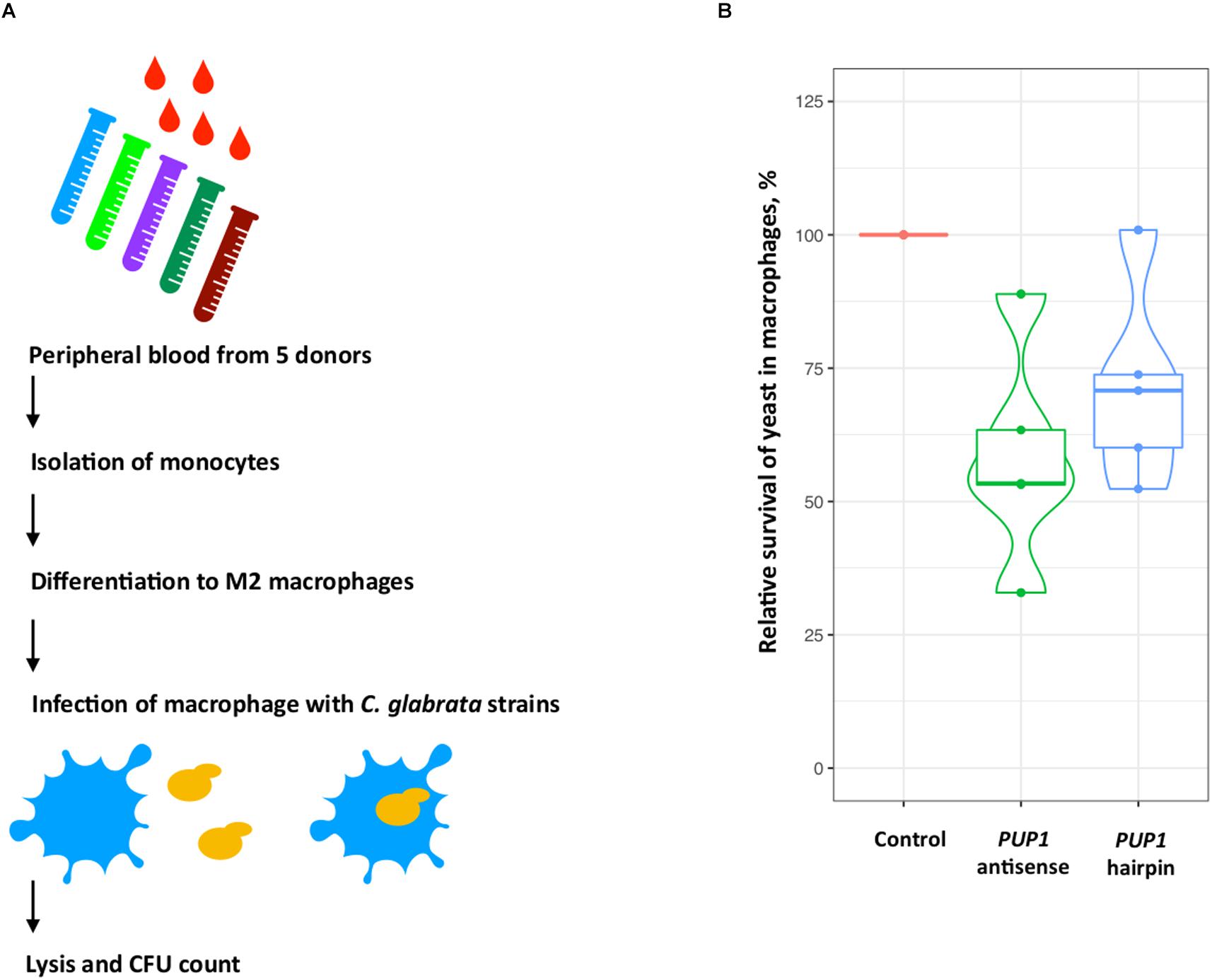
Figure 2. In C. glabrata, silencing-constructs of the putative virulence-associated gene PUP1 (CAGL0M12947g) inhibit yeast-survival in the macrophage model. (A) M2 macrophages differentiated from peripheral-blood monocytes of five donors were infected with the three C. glabrata strains. Yeast survival was assessed by CFU count. (B) The relative survival-rates of C. glabrata strains carrying antisense and hairpin constructs of the PUP1 gene were determined and normalized to the control strain carrying an empty vector (Y1848, set as 100% survival and used to normalize data). The plot is based on the data of five replicates for each construct.
Construction of RNAi Gene Library to Detect New Virulence-Associated Genes of C. glabrata
To use our RNAi system as a basis for the identification of infection-relevant C. glabrata genes, we constructed a library of C. glabrata genome fragments on a plasmid vector (P1226) carrying an ARS-like sequence, which results in about 10 copies per cell (Hanic-Joyce and Joyce, 1998) (Figure 3A). The expression cassette for genomic fragments contained the strong constitutive promoter TEF1 and the terminator CYC1 of C. glabrata. The resulting gene-library plasmids were isolated from 400,000 bacterial transformants, and 40% of these carried inserts ranging from 1 to 5 kb in size as estimated by Sau3AI enzyme digestion of the plasmid DNA. The constructed gene library therefore represented at least a 10× genome coverage of C. glabrata as estimated by the number of clones and their plasmid insert sizes. This library was used to transform the BG2-based C. glabrata strain with the reconstituted RNAi pathway, Y1662 (Figure 3). In this case, both sense and antisense DNA fragments (depending on the orientation of the ligated genomic fragments) could determine the transformant’s phenotype. We expected that the sense fragments lead to gene up-regulation, while the antisense fragments lead to gene down-regulation (silencing).
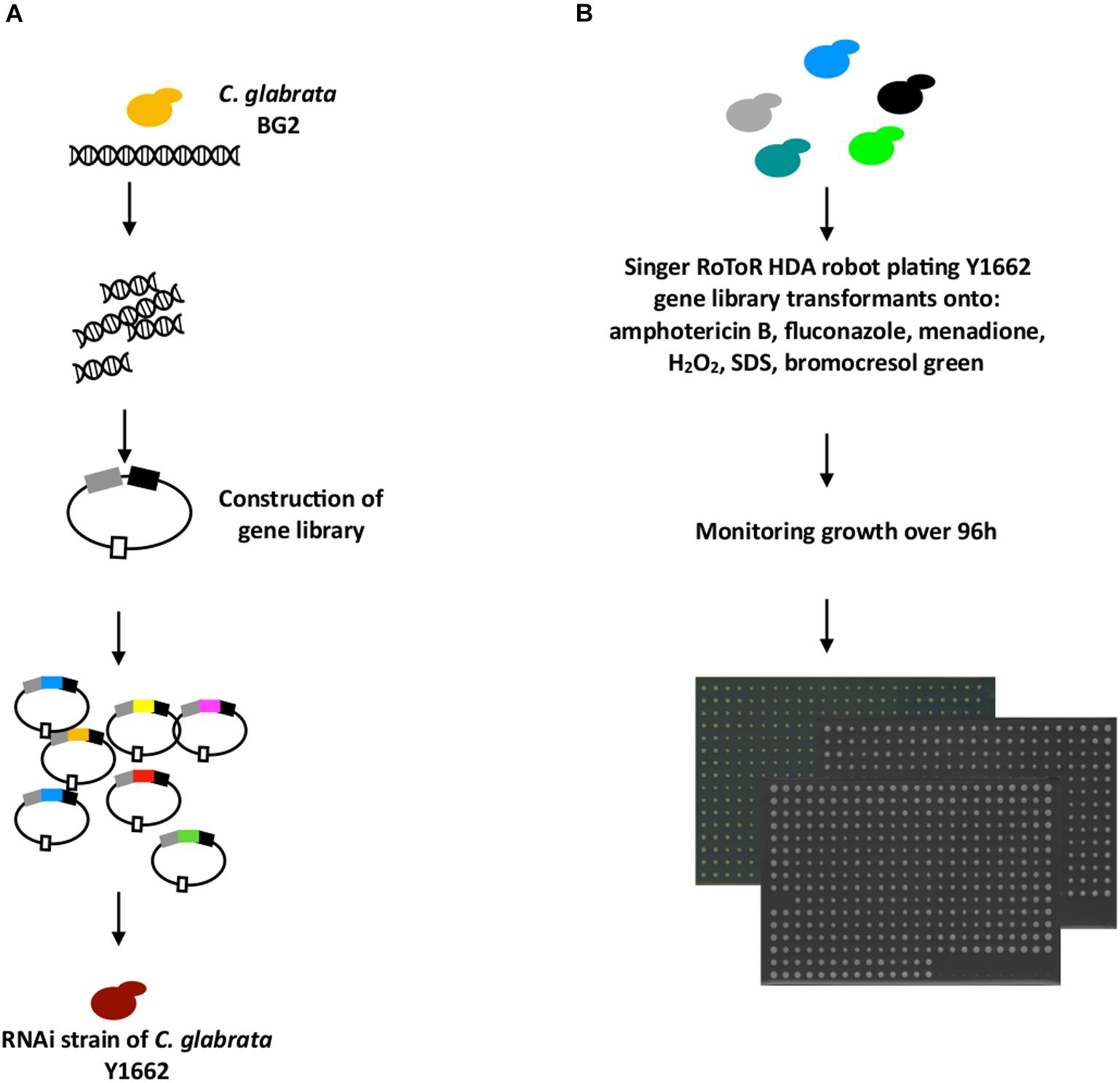
Figure 3. Cloning of putative virulence genes from a gene library. (A) Genomic DNA for gene library construction was isolated from the C. glabrata BG2 strain. The DNA was partially digested with Sau3AI and cloned into the C. glabrata multi-copy expression vector P1226 under control of the TEF1 promoter and with CYC1 terminator. This library was transformed into the RNAi-capable C. glabrata strain Y1662. (B) 1080 transformants of Y1662 containing gene library plasmids were plated from liquid YNB onto different media of interest (amphotericin B, fluconazole, menadione, hydrogen peroxide, SDS, bromocresol green) using a robotic system in 384-colony format plates. The growth of colonies was monitored by the digital imaging over 96 h.
RNAi Gene Library Phenotypic Screening Identifies Putative Virulence-Associated Genes
A total of 1080 C. glabrata strains with the gene library were selected randomly from ∼5000 colonies obtained after transformation, and subjected to phenotypic profiling in search of novel virulence-related genes involved in ROS tolerance, and antifungal drug resistance (Figure 3), attributes that help C. glabrata survive in the host. In addition, we selected medium with SDS for the large-scale cloning of genes responsible for C. glabrata cell integrity, and pH indicator for monitoring the colonies surface pH (Figure 3). Later can be explored further as targets for the development of new antifungals to destroy the cell or studied for its pH alteration properties in macrophages.
In the first round of screening, 1080 transformants were analyzed for their growth capacity in two replicates at high resolution using a robotic platform. The plating and growth screening were carried out on solid media containing fluconazole, amphotericin B, menadione, hydrogen peroxide, SDS, or the pH indicator bromocresol green. As shown in Figure 4, the C. glabrata transformant colonies varied in their growth phenotypes with a high correlation between two replicates. In comparison to the control medium (YNB with 2% glucose), the addition of selected compounds in most conditions resulted in more divergent transformant phenotypes (colony sizes) relative to the control transformant with an empty vector (used for the normalization and has value 1). Transformants with colony size different to the control strain under the conditions studied are of interest for further study, as the genes, which can contribute to this superior or diminished growth, are potential antifungal targets. Several transformants were resistant or sensitive to different stress conditions (Figure 4).
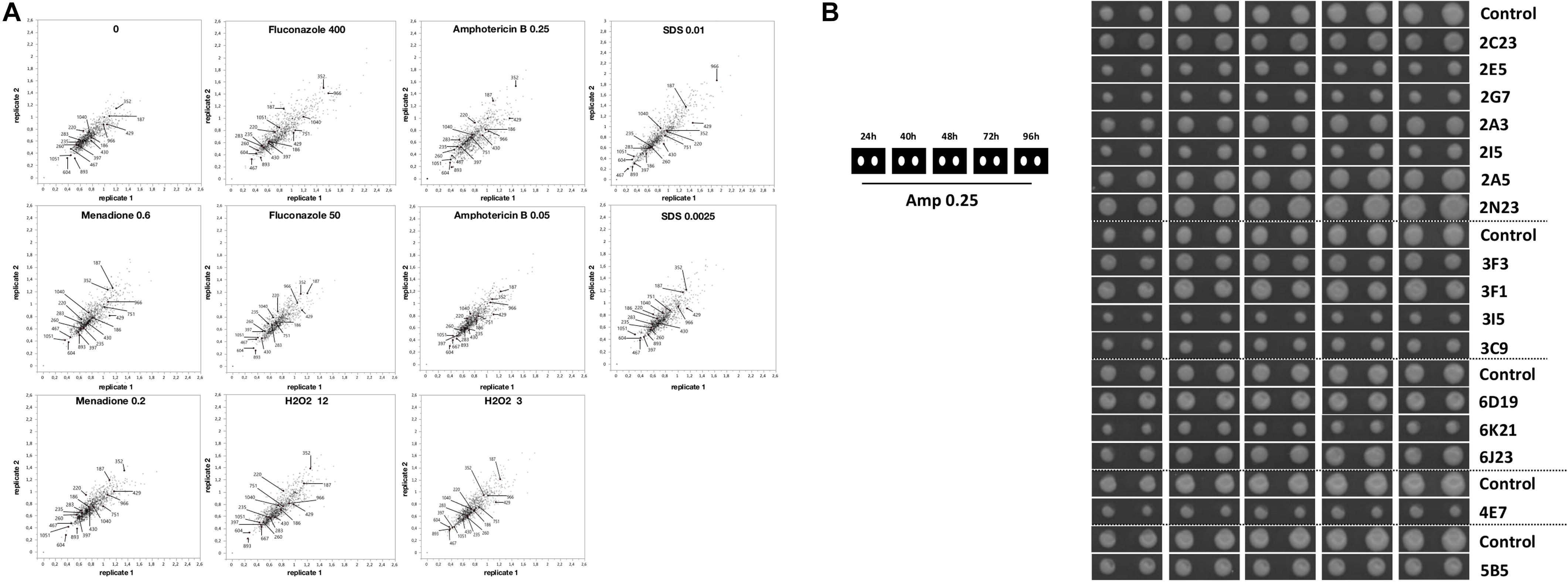
Figure 4. The gene library transformants had different growth phenotypes. (A) Relative colony size of two replicates of 1080 gene library transformants of the RNAi-capable strain under different conditions estimated at 48 h of incubation. Colony size of transformants with the library was normalized to the control (Y1662 with empty vector, which was set to 1.0) on each plate. Conditions: 0 (no treatment), antifungals (fluconazole [50 and 400 μg/ml], amphotericin B [0.05 and 0.25 μg/ml]), ROS-generating compounds (hydrogen peroxide [3 and 12 mM], menadione [0.02 and 0.06 mM]), and surfactant (SDS, 0.0025 and 0.01%). (B) Growth for 96 h of transformants (two replicates each) selected for sequencing on the medium with AmB (0.25 μg/ml).
The second round of screening was performed for the sensitivity to the potent new antifungal peptides 6, 9, and 11 developed by Larsen et al. (2015). For this, we used selected transformants with stress-related phenotypes (identified in the first round, 139 transformants) and 54 previously untested randomly picked up transformants. Transformants were tested in replicate and 11 transformants proved to be sensitive or resistant to one or all of the antifungal peptides tested (Table 2).
In total, we randomly selected 82 gene library transformants for sequencing, all of which showed increased or decreased stress sensitivity, and/or changed resistance to antifungal drugs tested, for sequencing. The inserts of the isolated plasmids were sequenced from the promoter TEF1 and the terminator CYC1 and 24 inserts sequences, which were obtained in full, contained one gene (or portion of a gene) in either the sense or anti-sense orientation (Table 2). The C. glabrata CAGL0G05335g gene, (TPS2) encoding a subunit of trehalose-6-phosphate synthase/phosphatase complex and known to be associated with virulence in C. albicans (Van Dijck et al., 2002), was found on two plasmids. Most of the genes isolated were so far uncharacterized (Table 2). Potential gene functions predicted form orthologs of identified genes in other species cover numerous cellular processes including RNA processing and transcription regulation, adhesins, trehalose biosynthesis, ethanol production, protein folding, chromosome maintenance and recombination, and metabolite transport. We also found two clones with antisense fragments to genes, whose orthologs in S. cerevisiae are essential: CAGL0H00891g (S. cerevisiae IQG1 encodes protein required cytokinesis (Epp and Chant, 1997), and CAGL0A01430g [S. cerevisiae TRP5 gene encodes tryptophan synthase and whose mutations results in tryptophan auxotrophy (Braus, 1991)].
Seven transformants proved to be sensitive to one or to all antifungal peptides tested (Table 2). For example, transformant 81C6 (5N2) was sensitive to peptide 6, 9, and 11 (Table 2 and Figure 5), and was found to carry the plasmid with antisense fragment to the C. glabrata CAGL0J02464g gene, which encodes a putative isopeptidase, that is possibly able to directly cleave or modify the tested peptides. Four transformants proved to be resistant to one or to all antifungal peptides tested (Table 2). Transformant 81E12 (2A3) was resistant to peptide 9 (Table 2 and Figure 5), and was found to carry the plasmid with sense fragment of the C. glabrata CAGL0B01683g/TMN2 gene’s open reading frame, which ortholog in S. cerevisiae is a membrane protein, which take part in endosome-vacuolar trafficking. The degradation of other antimicrobial peptides by targeting to yeast vacuole was reported by other studies (Lis et al., 2009; Muñoz et al., 2013).
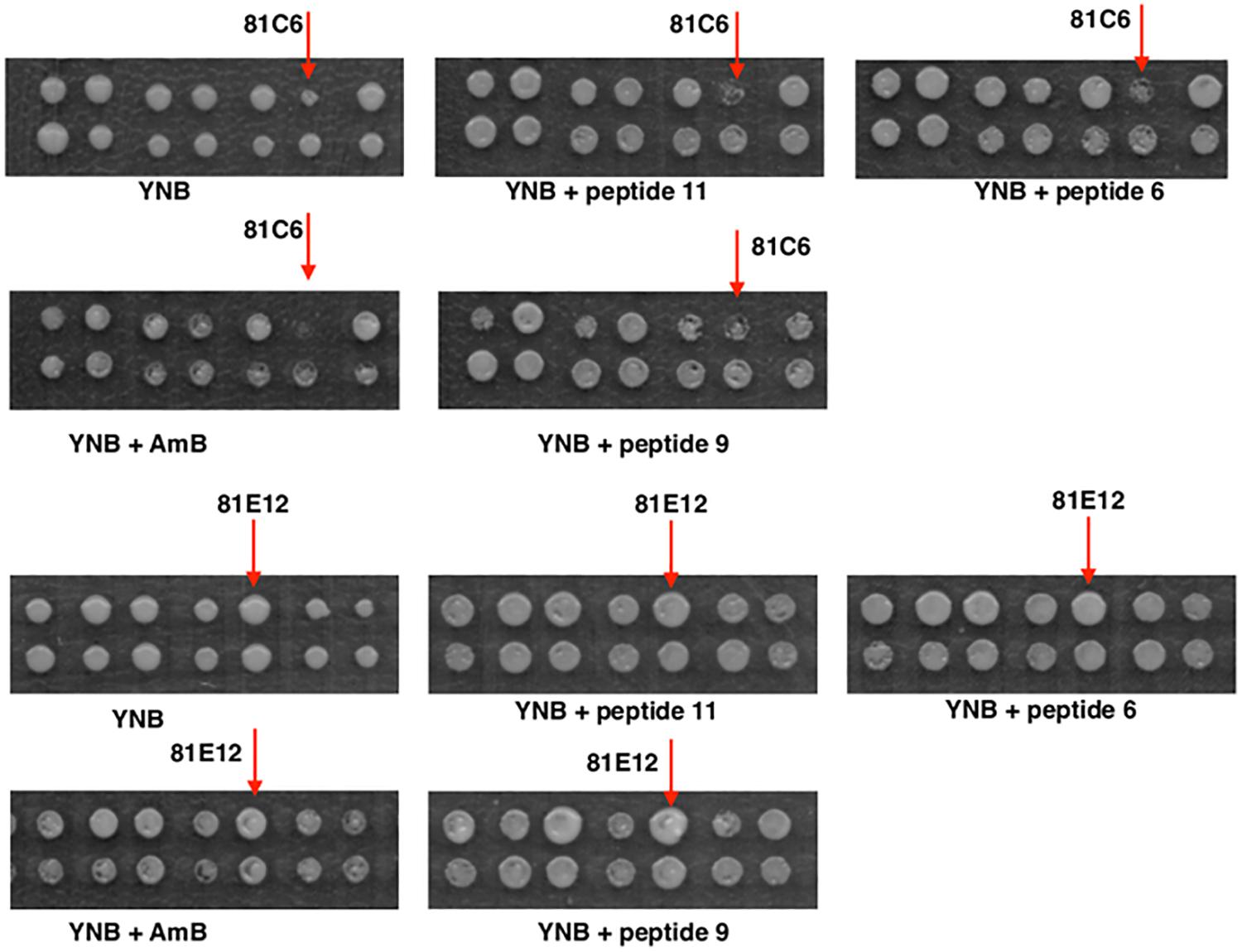
Figure 5. Growth of gene library transformants of RNAi strain on antifungal peptides (#6, #9, #11) and amphotericin B (AmB). The transformants, which displayed sensitivity or resistance to the compounds tested were selected for sequencing (arrowed).
In addition sensitivity to antifungal drugs, the transformant 3F3 displayed more acidic colony surface on medium with pH indicator bromocresol green, as the colonies of this strain had darker yellow color than the control strain (Figure 6A), indicating acid pH (≤3) of 3F3 colonies. The observed properties of 3F3 on bromocresol green could affect its survival after phagocytosis. Sequencing of the insert of the gene library plasmid isolated from 3F3 showed that it carried an antisense insert of the uncharacterized gene of CAGL0K11968g of C. glabrata. In S. cerevisiae, the gene-ortholog has a 3-hydroxyisobutyryl-CoA hydrolase activity and a role in the stress-activated MAPK cascade with its mutation affecting the fluid-phase endocytosis1. Indeed, vacuolar staining revealed that as in deletion mutants of S. cerevisiae, the down-regulation of this gene in C. glabrata affects the vacuolar morphology (Figure 6B). We tested the 3F3 strain in our macrophage model, where it was 50% more susceptible to killing after macrophage phagocytosis (Figure 6C). The macrophages phagosome maturation is accompanied with high level of ROS, and one of C. glabrata strategies to proliferate inside the macrophages is lowering ROS (Seider et al., 2011). Our study of 3F3 strain showed that it had lowered proliferating properties inside the macrophages (lowered survival). The expression of the 3F3 plasmid (antisense) was also found to confer hydrogen peroxide sensitivity in RNAi strain but not in wild type (Supplementary Figure S3).
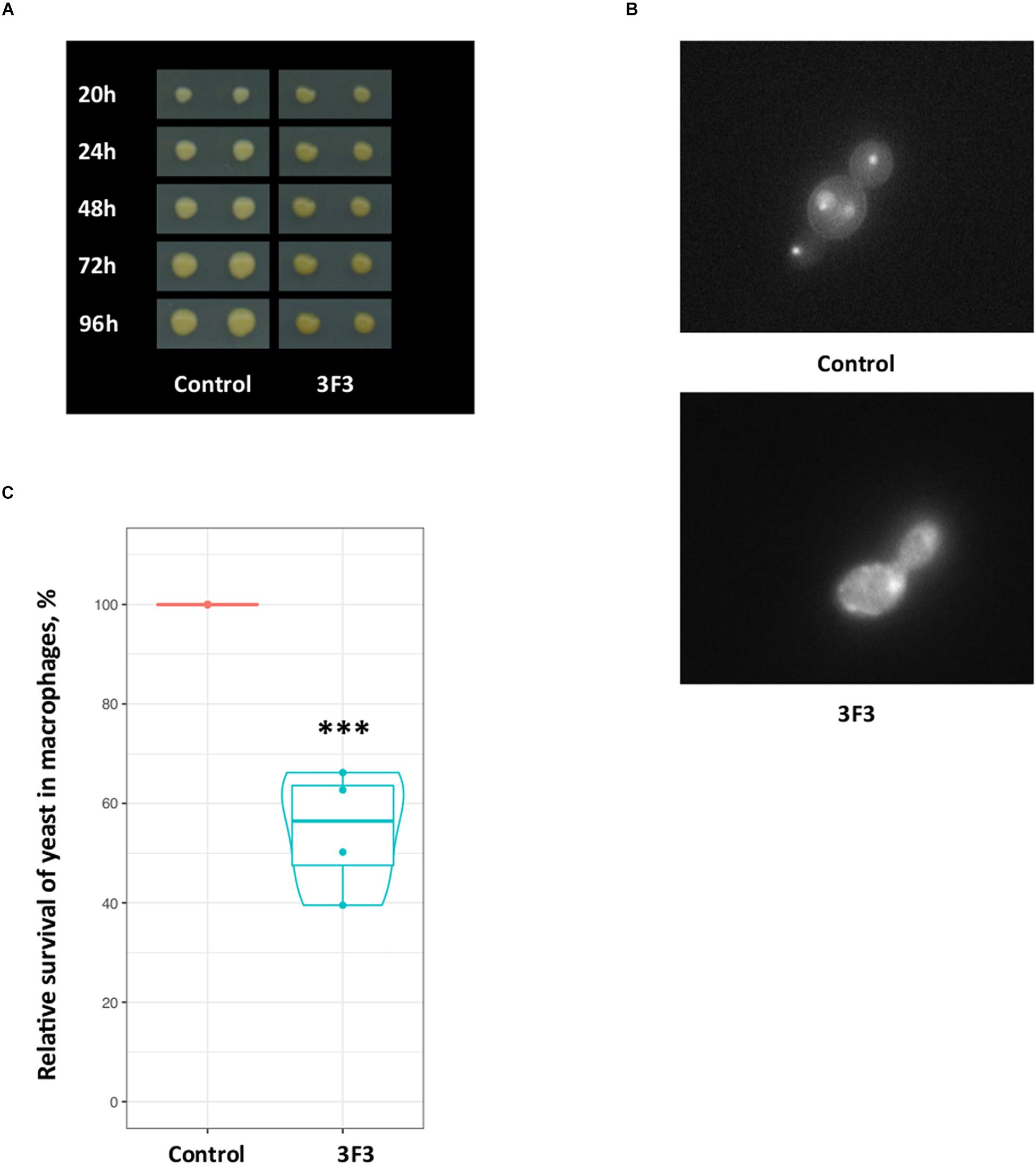
Figure 6. Candida glabrata CAGL0K11968g contributes to vacuolar morphology and survival in macrophages. (A) On the medium with pH indicator bromocresol green the 3F3 strains had darker yellow color than control strain, indicating more acidic pH. (B) Fluorescent microscopy images of C. glabrata strains after staining showing abnormal vacuolar morphology of the 3F3 strain. (C) The transformant 3F3, which carries an antisense CAGL0K11968g gene, displayed 50% loss of viability in our macrophage model. The experiment was performed in four replicates with cells originating from blood of two donors. The plot is based on data of four replicates. The control strain data (CFU) was set as 100% and used to normalize data. According to Poisson Regression analysis of raw data, the 3F3 strains CFU response was significantly different (∗∗∗p-value ≤ 0.0005).
In summary, using high resolution of mitotic growth screening of gene library in RNAi strain we cloned several putative virulence-related genes.
RNAi Gene Library Antisense Plasmids Inhibit the Expression of Their Target Genes
Further studies are needed to verify each isolated gene by the gene overexpression studies or gene deletions. Moreover, the antisense and truncated gene constructs may have off target effects on other gene expression as observed in mammalian cells (El-Brolosy and Stainier, 2017; Ma et al., 2019; Wilkinson, 2019), and studying the global gene expression of these strains could give more information on this in future studies. In this study, to rule-out the possibility of off-target gene inhibitions we have investigated the target gene expression of 10 antisense constructs in both wild type and RNAi strains.
For this purpose we took ten gene library antisense plasmids (2C23, 2E5, 81E20, 6D19, 3F3, 2I5, 82N22, 81N8, 83K11, and 2G7) and transformed them into the wild type and RNAi strains. Two transformants of each plasmid were selected for the analysis. The RNA was extracted from their cultures grown in YNB with 2% glucose and gene expression was studied by qRT-PCR (Figure 7 and Supplementary Figure S4). Plasmids 2C23, 2E5, 81E20, 2I5, and 82N22 had stronger gene expression inhibition in RNAi strain than in WT. Plasmids 6D19, 3F3, 81N8, 83K11, and 2G7 proved to inhibit the target genes in RNAi strain but not in the WT strain (Figure 7). This suggests that the gene inhibition is indeed mediated through RNAi. Phenotypes observed during the robotics screening were re-confirmed for most plasmids after the re-transformation (Supplementary Figure S3). While some of the phenotypes were unique to RNAi transformants (H2O2 sensitivity in 2I5, 3F3, 82N22, and 81E20), 2I5 conferred the amphotericin resistance to both RNAi and wild type strain (Supplementary Figure S3).
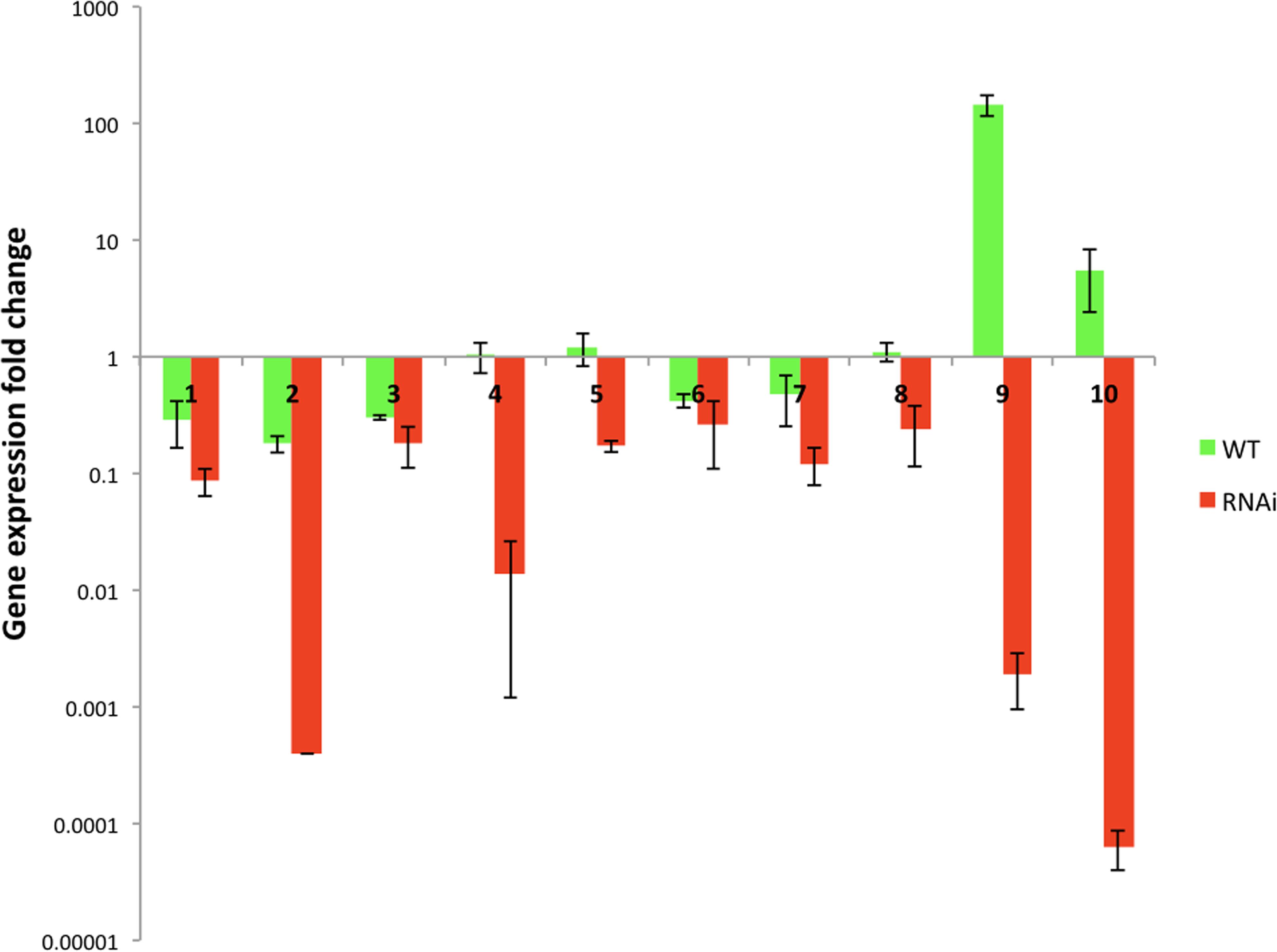
Figure 7. The target genes expression studies of the transformants of RNAi and wild type strain carrying antisense gene library plasmids. Y-axis – logarithmic scale. RNAi strain was Y1662, and the wild type was Y1637, which were newly re-transformed with plasmids. Antisense plasmids: 1 – 2C23 (CAGL0L00157g gene), 2 – 2E5 (CAGL0E00231g gene), 3 – 81E20 (CAGL0G05335g gene), 4 – 6D19 (CAGL0E00539g gene), 5 – 3F3 (CAGL0K11968g gene), 6 – 2I5 (CAGL0G05335g gene), 7 – 82N22 (CAGL0H00891g gene), 8 – 81N8 (CAGL0A01430g gene), 9 – 83K11 (CAGL0H07623g gene), and 10 – 2G7 (CAGL0I11011g gene). The untreated controls were used to calculate gene expression fold change: RNAi and wild type carrying empty vector (P1226). Primer pair ACT1-1 and ACT1-2 was used for ACT1 gene.
Discussion
The yeast C. glabrata is an important opportunistic human pathogen, which has become of interest over recent decades, particularly due to its increasing occurrence and resistance to antifungal drugs.
Unlike for S. cerevisiae and C. albicans, there are no extensive genetics toolboxes available for C. glabrata and thus this yeast remains poorly studied. Gene deletions in this yeast for gene function elucidations are not easy to obtain. With a higher rate of non-homologous recombination in C. glabrata than that in S. cerevisiae (Cormack and Falkow, 1999; Corrigan et al., 2013), longer homology regions are required for more efficient gene knockouts in C. glabrata; haploid asexual nature of C. glabrata prohibits many techniques based on mating developed for S. cerevisiae. In this study, we developed a new tool for C. glabrata based on RNA interference (RNAi), which relies on gene silencing through double-stranded RNA intermediates and siRNA. This ancient mechanism of protection from foreign DNA and chromatin organization is lost in Hemiascomycetes (Axelson-Fisk and Sunnerhagen, 2006) with the exception of Saccharomyces castellii (Drinnenberg et al., 2009). We therefore cloned the DCR1 and AGO1 genes from S. castellii and overexpressed them in C. glabrata together with silencing constructs. Judging from our data on silencing of metabolic genes (endogenous ADE2 and URA3 genes), the introduced RNAi pathway is functional and can be applied to additional genes of interest in this yeast. We found that gene silencing was stronger with hairpin compared to antisense constructs in this study similarly to Drinnenberg et al. (2009). Although our data indicate that the CUP1 promoter used for RNAi is leaky, the antisense constructs resulted in decreased gene expression and detectable phenotypes only upon its induction by copper. In contrast, the hairpin constructs displayed high inhibition of gene expression regardless of the CUP1 promoter induction, which suggests that the designed hairpins are very effective in our system.
Our RNAi tool proved to be active when we tested it with an established virulence-associated gene CAGL0M12947g (PUP1) of C. glabrata, which was shown by deletion to be important for a survival in a mouse model (Ferrari et al., 2011). Here, we showed that down-regulation of this gene affects survival in human macrophages. Its ortholog (RCI37/YIL077C) is poorly studied in S. cerevisiae. Although it is known to localize in the mitochondria and interact with the respiratory chain (Morgenstern et al., 2017), the mechanistic connections of its C. glabrata ortholog to virulence remain unknown.
We were interested to apply the developed RNAi for the discovery of new virulence-associated genes of C. glabrata. We did this by using the RNAi strain with a gene library as the basis for screening of virulence-related phenotypes and the identification of responsible genes. To this end we created a library of random genome fragments in the expression vector. In contrast to hairpin constructs, this approach is feasible on the genomic level (the library represents 10-fold genome coverage), and we can assume that a significant portion of clones will carry the antisense regions of genes to induce RNAi. Since the inhibitory effect of the antisense constructs was less efficient than hairpins in our model and required a high expression level, the gene library was constructed using the strong constitutive TEF1 promoter and a multi-copy vector.
Our primary interest was to discover genes, which affect C. glabrata resistance to antifungal drugs, and to stress conditions that C. glabrata may face during survival in macrophages. Therefore, we selected the following conditions for cell growth in vitro, which have overlap in their targets in yeast. In our screening we selected fluconazole and amphotericin B, which are both antifungal drugs currently in use against candidiasis, and which target the plasma membrane. Although the mechanism of action of many azole drugs and resistance to them are quite extensively studied (Vale-Silva and Sanglard, 2015), the molecular mechanism of amphotericin B action and resistance remains poorly understood. We also tested hydrogen peroxide and menadione since ROS are elevated upon amphotericin B exposure (Mesa-Arango et al., 2014), and are produced inside the macrophages phagosomes (Seider et al., 2014), and resistance against ROS is important for pathogen survival. SDS was tested to mimic damage to the plasma membrane. In addition, we used new antifungal drugs for the C. glabrata gene library screening including three peptidomimetics with an arginine-[β-(2,5,7-tri-tert-butylindol-3-yl)alanine]-arginine motif, which were recently developed (Larsen et al., 2015), and are effective against S. cerevisiae as well as Zygosaccharomyces bailii, known to spoil food. Interestingly, C. glabrata was previously shown to be resistant to these peptides (Larsen et al., 2015) and finding the resistance genes in pathogenic yeasts can help to develop potent antifungals further.
The frequent and large size differences of transformants colonies in our screening indicated that a broad range of genes was covered by the RNAi library strains. We selected representative resistant and sensitive transformants for sequencing of their plasmid inserts to determine the original genome loci. Using this approach, we found that several positive clones of our RNAi library corresponded to putative virulence-associated genes. This shows that our approach allows us to recover genes with relevance for C. glabrata pathobiology, which can be exploited further for the development of treatments for C. glabrata infections.
The gene down-regulation and expression of truncated genes might affect the expression of other genes in the genome, their genes network as described for mammalian cells (El-Brolosy and Stainier, 2017; Ma et al., 2019; Wilkinson, 2019). This effect, genetic compensation, is also common for gene knockouts in yeasts (He and Zhang, 2006; Teng et al., 2013). The expression of antisense constructs spanning the coding regions in S. cerevisiae from the plasmid vectors were reported to inhibit the ATH1 and CAR1 gene expression (Park et al., 2001; Jung and Park, 2005) suggesting the natural antisense interference without RNAi (Donaldson and Saville, 2012). The whole genome sequencing and global gene expression profiling can give more information on any off-target effects of isolated gene library clones in the future studies. When we analyzed ten antisense constructs, all 10 down-regulated the target genes, and 5 of 10 worked through the RNAi because they did not down-regulate the gene expression in the wild type strain without RNAi pathway.
Three of the genes identified in our screen were predicted glycosylphosphatidylinositol (GPI)-anchored proteins and adhesins (CAGL0L00157g, CAGL0E00231g, and CAGL0I11011g), and the expression of their antisense regions leads to sensitivity to fluconazole, amphotericin B, menadione, hydrogen peroxide, and SDS. GPI-anchored proteins are abundant membrane and cell wall proteins with multiple roles, and their biosynthesis is reportedly linked ergosterol biosynthesis and azole drug response, Ras signaling (Yadav et al., 2014). The GPI-anchored proteins are therefore important antifungal targets, and are in the focus of drug discovery studies (Mann et al., 2015). It is important to note that the antisense region of the 2C23 isolate of our study included a part of the GPI-anchored protein transmembrane domain (CAGL0L00157g), which is a fragment of 17 other GPI-genes sequences in the C. glabrata genome. This suggests that the construct affected several GPI proteins, likely resulting in altered plasma membrane structure.
The 6K21 strain, which was sensitive to antifungals and ROS, carried the overexpression plasmid for a sense fragment of transporter gene CAGL0J01661g, whose ortholog in S. cerevisiae (YPR011C) encodes a mitochondrial transporter for adenosine 5′-phosphosulfate (APS) and 3′-phospho-adenosine 5′-phosphosulfate (PAPS), the deletion of whish causes the decreased glutathione and methionine levels and temperature sensitive phenotype (Todisco et al., 2014), and glutathione is known ROS scavenger (Jamieson, 1998).
Our results point at the TPS2 gene, encoding trehalose-6 phosphate phosphatase of C. glabrata, as a potential drug target with virulence-associated roles in this yeast. The TPS2 antisense construct expression resulted in sensitivity to fluconazole, amphotericin B, and other stressors. The deletion of TPS2 in both S. cerevisiae and C. albicans causes the accumulation of increased amounts of trehalose-6 phosphate upon stress, which is toxic to the cell, and results in thermo-sensitive phenotype (De Virgilio et al., 1993; Van Dijck et al., 2002). In C. albicans, it was also found to reduce the virulence as the survival of infected mice in systemic infection model was increased (Van Dijck et al., 2002). Indeed, the deletion of this gene leads to impaired growth in C. albicans, and other species, and the gene product itself is considered as a potential target for antifungal therapy (Van Dijck et al., 2002; Pianalto and Alspaugh, 2016).
The transformant 3I5 carried a gene library plasmid with a complete ADH1 open reading frame in the sense direction. It proved to be sensitive to fluconazole, amphotericin B, peptides, SDS, and reactive oxygen species. The ADH1 gene is encoding alcohol dehydrogenase, responsible for the conversion of acetaldehyde to ethanol. In C. albicans, the expression of the ADH1 and azole resistance is inversely correlated in clinical isolates (Siikala et al., 2011). On the contrary, increased abundance of the Adh1 protein was previously observed in an azole resistant strain of C. glabrata (Rogers et al., 2006). Since we do not have the data on the ADH1 expression in 3I5 strain, further study is needed.
Due to their low toxicity, the peptidomimetics are potent antifungal drugs (Larsen et al., 2015). A mode of action of these peptides is likely the interaction with sphingolipids, as determined by the analysis of deletion mutants’ library in S. cerevisiae (Larsen et al., 2015). Larsen et al. (2015) pointed out that higher resistance in pathogenic yeasts (C. albicans and C. glabrata) to these compounds might be due to the secretion of extracellular proteases, which sequester or degrade the antifungals. One of the isolated C. glabrata antisense plasmids carried part of the CAGL0J02464g gene, whose orthologs have SUMO (Small Ubiquitin-like Modifier)-specific isopeptidase and protein deSUMOylation activities. The encoded isopeptidase could be important for the resistance to peptidomimetics in this yeast and could be involved in their direct cleavage, in support of the hypothesis proposed by Larsen et al. (2015). In addition, fragments of two putative aminoacyl-tRNA genes were found with our system affecting the peptidomimetics resistance. We hypothesize that they could directly interact with peptidomimetics or their targets by acylating them. Aminoacyl-tRNA dependent acylation is involved in several cellular processes and resistance to antifungal peptides reported in other species (Raina and Ibba, 2014). Acylation of membrane lipids is known to change the membrane surface and subsequently the affinity of the membrane to antifungal peptides in other species (Ernst and Peschel, 2011; Raina and Ibba, 2014). The direct acylation of macromolecules using aminoacyl-tRNA can change their activity, recognition or directs them to degradation (Katz et al., 2016).
Taking a step further, we validated the importance of one of the identified target in the macrophage model. We confirmed the vital importance of the C. glabrata CAGL0K11968g gene affecting colony pH and vacuolar function with this screening method. With the antisense construct for this gene the C. glabrata strain was less viable upon exposure to human macrophages.
We have thus established a working RNAi system for the investigation of C. glabrata pathobiology. Our initial testing showed that the system can be used to interfere with the expression of a broad range of genes, including those assumed to be essential for C. glabrata. The RNAi strains can be used in in vitro stress tests and in interaction with immune cells, which will be invaluable in attributing functions to the many genes of C. glabrata, which are unannotated so far. Our screening of a RNAi library with genome fragments is a first step into that direction. Especially, as this method enables us to tackle new genes, which are central for fungal growth and survival, we believe that it will allow us to find important new potential targets for C. glabrata antifungals, a yeast that is notorious for its inherent and acquired resistances.
Materials and Methods
Growth Conditions
All strains used in this study were grown in rich medium (YPD [yeast extract 1%, peptone 2%, glucose 2%, Bactoagar 2%] or synthetic minimal medium (YNB [yeast nitrogen base without amino acid and ammonium sulfate] 1.9 g/L, glucose 2%, ammonium sulfate 0.5%) at 25°C, unless stated otherwise. For the uracil-deficient mutant BG14, 50 mg/L uracil was added to the YNB medium. For the lysine-deficient mutants, 40 mg/L lysine was added to the minimal medium. C. glabrata transformants carrying plasmids with the LYS2 gene as a selectable marker were selected and propagated on YNB medium. For C. glabrata transformants carrying the Streptoalloteichus hindustanus ble gene as a selective marker, 200 μg/ml of zeocin was added to the YPD medium. For the induction of the CUP1 promoter, 0.05 mM CuSO4 was added to the YNB medium.
For gene library yeast transformants different compounds were added to YNB medium as follows: amphotericin B (0.05 and 0.25 μg/ml), fluconazole (50 and 400 mg/L), SDS (0.01 and 0.0025%), hydrogen peroxide (3 and 12 mM), menadione (0.02 and 0.06 mM), and antifungal peptidomimetics (peptide 6 [H-Arg-Tbt-Arg-Phe-NH2], 9 [H-Arg-Tbt-Arg-hPhe-NH2], and 11 [H-Arg-Tbt-Arg-[NPhe]-NH2] (Larsen et al., 2015) at 25 μg/ml. Gene library transformants were propagated at 37°C.
RNA Extraction and qRT-PCR
Total RNA was extracted from the C. glabrata cultures grown in selective media (supporting plasmids propagation) using the PureLink RNA Mini Kit (Thermo Fisher Scientific). The concentration and purity of RNA were determined by NanoDrop spectrophotometer. The isolated RNA was treated with DNase I (RNase-Free DNase Set, Qiagen) according to the manufacturer’s recommendations. The RNA integrity was checked by electrophoresis using precast RNA MOPS agarose gels (Sigma-Aldrich). Five microgram of pure RNA was used for the synthesis of cDNA. The SuperScript III Reverse Transcriptase kit with RNaseOUT Ribonuclease Inhibitor and random primers (Thermo Fisher Scientific) was used. The cDNA produced was used as a template with gene-specific primers in qRT-PCR reactions with the SYBR GreenER qPCR SuperMix (Thermo Fisher Scientific). qRT-PCRs were run in duplicate in the RotorGene 2000 cycler (Corbett Research) under the conditions specified by Thermo Fisher Scientific. The take off and amplification values were obtained using the RotorGene 2000 software. The β-actin gene was treated as the endogenous reference gene (housekeeping gene), while Y1848 was used as untreated strain. Primer pairs for the 3′-region of the gene ORFs of interest of C. glabrata were used in qRT-PCR experiments as follows for the: ACT1 (OP33 and OP34; ACT1-1 and ACT1-2), ADE2 (OP31 and OP32), URA3 (OP27 and OP28), PUP1 (947-1 and 947-2), CAGL0L00157g (157-1 and 157-2), CAGL0E00231g (231-3 and 231-4), CAGL0G05335g (5335-3 and 5335-4), CAGL0E00539g (539-1 and 539-2), CAGL0K11968g (968-1 and 968-2), CAGL0H00891g (891-1 and 891-2), CAGL0A01430g (1430-3 and 1430-4), CAGL0H07623g (7623-1 and 7623-2), and CAGL0I11011g (11-1 and 11-2) (Supplementary Table S1). The gene expression fold change was calculated by the ΔΔCt method (Livak and Schmittgen, 2001).
Macrophage Culture and Infection With Yeast
Human monocyte-derived macrophages (hMDMs) were prepared according to the protocol used before (Seider et al., 2011, 2014). Monocytes were isolated from human peripheral blood (donated by healthy volunteers with written consent) with CD14 magnetic beads by automated cell sorting (autoMACs, MiltenyiBiotec). CD14-positive monocytes differentiated to M2 macrophages for 7 days in RPMI1640 media with L-glutamine (Thermo Fisher Scientific) with 10% heat-inactivated FCS (Bio&Sell GmbH), and with 50 ng/ml recombinant human macrophage colony stimulating factor (rh M-CSF; Immunotools). Adherent MDMs were detached with 10 mM EDTA in PBS and seeded in 96-well plates (4 × 104 hMDMs/well) in RPMI with 50 ng/ml rh M-CSF and 10% FCS and incubated overnight. Prior macrophage infection yeast cells from a stationary YNB culture were washed three times with PBS. M2 macrophages were infected by adding the yeast cells at multiplicity of infection of one (MOI 1) in RPMI w/o FCS. The cells were further diluted and incubated for 3 h at 5% CO2 and 37°C. Unattached yeast cells were removed by washing the macrophages two times with 60 μl PBS. Next, 20 μl of 0.5% Triton X-100 was added to lysate the macrophages, and incubated 10 min under gentle shaking. After the incubation, cells were diluted, plated on YPD, and incubated at 37°C for 1 day. After incubation, the yeast CFU were counted. The reference strain Y1848 carrying empty vector was used to normalize obtained data and was set to 100% survival.
Robotics Screening
Individual transformants of the C. glabrata RNAi strain (Y1662) containing the gene library plasmids were grown overnight in flat bottom polystyrene 96-well plates in 200 μl of liquid YNB at 37°C (12 plates in total). The next day, yeast transformants were plated onto the solid media YNB supplemented with antifungal drugs [amphotericin B (0.05 and 0.25 μg/ml) or fluconazole (50 and 400 μg/ml)], ROS generating compounds [menadione (0.02 and 1 0.06 mM) and hydrogen peroxide (3 and 12 mM)], surfactant (SDS 0.01 and 0.0025%) and a pH indicator (bromocresol green 0.01 g/ml, YNB medium pH was adjusted to 4.5) using a robotic system (Siger RoToR HDA robot). Each 96-well plate liquid culture was plated on solid medium in duplicate, and four were combined onto one 384 format solid medium plate. The colonies growth was scored by the colony size in pixels from the digital images of the plates during 96 h of incubation.
Vacuolar Staining and Fluorescent Microscopy
Cells were re-suspended in 10 mM HEPES buffer pH 7.4 supplemented with 5% glucose. The fluorescent dye CMAC-Ala-Pro (7-amino-4-chloromethylcoumarin, l-alanyl-l-proline amide, Yeast Vacuolar Marker Sampler Kit [Thermo Fisher Scientific]) was added to the cell suspension at 100 μM and then incubated in the dark for 30 min. The staining was visualized by fluorescent microscopy (automated inverted wide-field microscope Observer Z1 [Carl Zeiss] equipped with a sCMOS camera).
Statistical Analysis
The software packages R (Version 1.1.463– ©2009–2018 RStudio, Inc.), JMP®, Pro 13.0.0 (SAS Institute Inc., Cary, NC, United States, 1989–2019) and Minitab®18.1, were used to analyze the obtained data.
Dedication
In loving memory of JP who sadly deceased on May 18, 2014.
Data Availability
All datasets generated for this study are included in the manuscript and/or the Supplementary Files.
Ethics Statement
The protocols for the experiments with macrophages were approved by the ethical commissions of the Lund University and the Hans Knöll Institute, Jena.
Author Contributions
OI and JP designed the study. OI, KA, KK, KB, and TS developed the RNAi tool. LK, SB, MS, BH, TH, BG, and OI performed the macrophage experiments. CB, KF, JS, BR, and OI analyzed the gene library. OI, KA, WK, and JP wrote the manuscript. All authors commented on and reviewed the manuscript.
Funding
This study was supported by grants from the Swedish Research Council (2012-02842 and 2016-01164), the Lawsky Foundation, the Royal Physiographic Society of Lund, the Erik Philip-Sörensen Foundation, and the Jörgen Lindström Foundation.
Conflict of Interest Statement
The authors declare that the research was conducted in the absence of any commercial or financial relationships that could be construed as a potential conflict of interest.
Acknowledgments
We thank Paul Joyce (Concordia University, Canada) for providing a plasmid vector carrying ARS-like sequence, pMIR4, Brendan Cormack (The Johns Hopkins University School of Medicine, United States) for providing the BG2 and BG14 strains, and Kate Campbell (Chalmers University of Technology, Sweden) for the help with R-script. JP passed away before the submission of the final version of this manuscript. OI accepts responsibility for the integrity and validity of the data collected and analyzed.
Supplementary Material
The Supplementary Material for this article can be found online at: https://www.frontiersin.org/articles/10.3389/fmicb.2019.01679/full#supplementary-material
Footnotes
References
Ahmad, K. M., Kokošar, J., Guo, X., Gu, Z., Ishchuk, O. P., and Piškur, J. (2014). Genome structure and dynamics of the yeast pathogen Candida glabrata. FEMS Yeast Res. 14, 529–535. doi: 10.1111/1567-1364.12145
Axelson-Fisk, M., and Sunnerhagen, P. (2006). “Comparative genomics and gene finding in fungi,” in Comparative Genomics Using Fungi as Models. Topics in Current Genetics, Vol. 15, eds P. Sunnerhagen and J. Piškur (Berlin: Springer), doi: 10.1007/4735_111
Braus, G. H. (1991). Aromatic amino acid biosynthesis in the yeast Saccharomyces cerevisiae: a model system for the regulation of a eukaryotic biosynthetic pathway. Microbiol Rev. 55, 349–370.
Brunke, S., and Hube, B. (2013). Two unlike cousins: Candida albicans and C. glabrata infection strategies. Cell. Microbiol. 15, 701–708. doi: 10.1111/cmi.12091
Cormack, B. P., and Falkow, S. (1999). Efficient homologous and illegitimate recombination in the opportunistic yeast pathogen Candida glabrata. Genetics 151, 979–987.
Corrigan, M. W., Kerwin-Iosue, C. L., Kuczmarski, A. S., Amin, K. B., and Wykoff, D. D. (2013). The fate of linear DNA in Saccharomyces cerevisiae and Candida glabrata: the role of homologous and non-homologous end joining. PLoS One 8:e69628. doi: 10.1371/journal.pone.0069628
De Virgilio, C., Bürckert, N., Bell, W., Jenö, P., Boller, T., and Wiemken, A. (1993). Disruption of TPS2, the gene encoding the 100-kDa subunit of the trehalose-6-phosphate synthase/phosphatase complex in Saccharomyces cerevisiae, causes accumulation of trehalose-6-phosphate and loss of trehalose-6-phosphate phosphatase activity. Eur. J. Biochem. 212, 315–323. doi: 10.1074/jbc.M113.528802
Donaldson, M. E., and Saville, B. J. (2012). Natural antisense transcripts in fungi. Mol. Microbiol. 85, 405–417. doi: 10.1111/j.1365-2958.2012.08125.x
Drinnenberg, I. A., Weinberg, D. E., Xie, K. T., Mower, J. P., Wolfe, K. H., Fink, G. R., et al. (2009). RNAi in budding yeast. Science 326, 544–550. doi: 10.1126/science.1176945
Dujon, B., Sherman, D., Fischer, G., Durrens, P., Casaregola, S., Lafontaine, I., et al. (2004). Genome evolution in yeasts. Nature 430, 35–44. doi: 10.1038/nature02579
El-Brolosy, M. A., and Stainier, D. Y. R. (2017). Genetic compensation: a phenomenon in search of mechanisms. PLoS Genet. 13:e1006780. doi: 10.1371/journal.pgen.1006780
Epp, J. A., and Chant, J. (1997). An IQGAP-related protein controls actin-ring formation and cytokinesis in yeast. Curr. Biol. 7, 921–929. doi: 10.1016/s0960-9822(06)00411-8
Ernst, C. M., and Peschel, A. (2011). Broad-spectrum antimicrobial peptide resistance by MprF-mediated aminoacylation and flipping of phospholipids. Mol. Microbiol. 80, 290–299. doi: 10.1111/j.1365-2958.2011.07576.x
Ferrari, S., Sanguinetti, M., Torelli, R., Posteraro, B., and Sanglard, D. (2011). Contribution of CgPDR1-regulated genes in enhanced virulence of azole-resistant Candida glabrata. PLoS One 6:e17589. doi: 10.1371/journal.pone.0017589
Fidel, P. L. Jr., Cutright, J. L., Tait, L., and Sobel, J. D. (1996). A murine model of Candida glabrata vaginitis. J. Infect. Dis. 173, 425–431. doi: 10.1093/infdis/173.2.425
Fidel, P. L. Jr., Vazquez, J. A., and Sobel, J. D. (1999). Candida glabrata: review of epidemiology, pathogenesis, and clinical disease with comparison to C. albicans. Clin. Microbiol. Rev. 12, 80–96. doi: 10.1128/CMR.12.1.80
Gabaldón, T., and Fairhead, C. (2018). Genomes shed light on the secret life of Candida glabrata: not so asexual, not so commensal. Curr. Genet. 65, 93–98. doi: 10.1007/s00294-018-0867-z
Giaever, G., Chu, A. M., Ni, L., Riles, L., Véronneau, S., Dow, S., et al. (2002). Functional profiling of the Saccharomyces cerevisiae genome. Nature 418, 387–391. doi: 10.1038/nature00935
Hanic-Joyce, P. J., and Joyce, P. B. (1998). A high-copy-number ADE2-bearing plasmid for transformation of Candida glabrata. Gene 211, 395–400. doi: 10.1016/s0378-1119(98)00157-7
He, X., and Zhang, J. (2006). Toward a molecular understanding of pleiotropy. Genetics 173, 1885–1891. doi: 10.1016/S0378-1119(98)00157-7
Jamieson, D. J. (1998). Oxidative stress responses of the yeast Saccharomyces cerevisiae. Yeast 14, 1511–1527. doi: 10.1002/(SICI)1097-0061(199812)14:16<1511::AID-YEA356<3.0.CO;2-S
Jung, Y. J., and Park, H. D. (2005). Antisense-mediated inhibition of acid trehalase (ATH1) gene expression promotes ethanol fermentation and tolerance in Saccharomyces cerevisiae. Biotechnol. Lett. 27, 1855–1859. doi: 10.1007/s10529-005-3910-3
Kasper, L., Seider, K., Gerwien, F., Allert, S., Brunke, S., Schwarzmüller, T., et al. (2014). Identification of Candida glabrata genes involved in pH modulation and modification of the phagosomal environment in macrophages. PLoS One 9:e96015. doi: 10.1371/journal.pone.0096015
Katz, A., Elgamal, S., Rajkovic, A., and Ibba, M. (2016). Non-canonical roles of tRNAs and tRNA mimics in bacterial cell biology. Mol. Microbiol. 101, 545–558. doi: 10.1111/mmi.13419
Larsen, C. E., Larsen, C. J., Franzyk, H., and Regenberg, B. (2015). Antifungal properties of peptidomimetics with an arginine-[β-(2,5,7-tri-tert-butylindol-3-yl)alanine]-arginine motif against Saccharomyces cerevisiae and Zygosaccharomyces bailii. FEMS Yeast Res. 15:fov011. doi: 10.1093/femsyr/fov011
Lis, M., Fuss, J. R., and Bobek, L. A. (2009). Exploring the mode of action of antimicrobial peptide MUC7 12-mer by fitness profiling of Saccharomyces cerevisiae genomewide mutant collection. Antimicrob. Agents Chemother. 53, 3762–3769. doi: 10.1128/AAC.00668-09
Livak, K. J., and Schmittgen, T. D. (2001). Analysis of relative gene expression data using real-time quantitative PCR and the 2-ΔΔCT Method. Methods 25, 402–408. doi: 10.1006/meth.2001.1262
Ma, Z., Zhu, P., Shi, H., Guo, L., Zhang, Q., Chen, Y., et al. (2019). PTC – bearing mRNA elicits a genetic compensation response via Upf3a and COMPASS components. Nature 568, 259–263. doi: 10.1038/s41586-019-1057-y
Mann, P. A., McLellan, C. A., Koseoglu, S., Si, Q., Kuzmin, E., Flattery, A., et al. (2015). Chemical genomics-based antifungal drug discovery: targeting glycosylphosphatidylinositol (GPI) precursor biosynthesis. ACS Infect. Dis. 1, 59–72. doi: 10.1021/id5000212
Mårdh, P. A., Rodrigues, A. G., Genç, M., Novikova, N., Martinez-de-Oliveira, J., and Guaschino, S. (2002). Facts and myths on recurrent vulvovaginal candidosis–a review on epidemiology, clinical manifestations, diagnosis, pathogenesis and therapy. Int. J. STD. AIDS. 13, 522–539. doi: 10.1258/095646202760159639
Martienssen, R. A., Zaratiegui, M., and Goto, D. B. (2005). RNA interference and heterochromatin in the fission yeast Schizosaccharomyces pombe. Trends Genet. 21, 450–456. doi: 10.1016/j.tig.2005.06.005
Mesa-Arango, A. C., Trevijano-Contador, N., Román, E., Sánchez-Fresneda, R., Casas, C., Herrero, E., et al. (2014). The production of reactive oxygen species is a universal action mechanism of Amphotericin B against pathogenic yeast and contributes to the fungicidal effect of this drug. Antimicrob. Agents Chemother. 58, 6627–6638. doi: 10.1128/AAC.03570-14
Moazed, D. (2009). Molecular biology. Rejoice–RNAi for yeast. Science 326, 533–534. doi: 10.1126/science.1182102
Morgenstern, M., Stiller, S. B., Lübbert, P., Peikert, C. D., Dannenmaier, S., Drepper, F., et al. (2017). Definition of a high-confidence mitochondrial proteome at quantitative scale. Cell Rep. 19, 2836–2852. doi: 10.1016/j.celrep.2017.06.014
Moyes, D. L., and Naglik, J. R. (2011). Mucosal immunity and Candida albicans infection. Clin. Dev. Immunol. 2011:346307. doi: 10.1155/2011/346307
Muñoz, A., Harries, E., Contreras-Valenzuela, A., Carmona, L., Read, N. D., and Marcos, J. F. (2013). Two functional motifs define the interaction internalization and toxicity of the cell-penetratingantifungal peptide PAF26 on fungal cells. PLoS One 8:e54813. doi: 10.1371/journal.pone.0054813
Park, H., Shin, M., and Woo, I. (2001). Antisense-mediated inhibition of arginase (CAR1) gene expression in Saccharomyces cerevisiae. J. Biosci. Bioeng. 92, 481–484. doi: 10.1263/jbb.92.481
Pfaller, M. A., and Diekema, D. J. (2007). Epidemiology of invasive candidiasis: a persistent public health problem. Clin. Microbiol. Rev. 20, 133–163. doi: 10.1128/CMR.00029-06
Pianalto, K. M., and Alspaugh, J. A. (2016). New horizons in antifungal therapy. J. Fungi. 2:26. doi: 10.3390/jof2040026
Raina, M., and Ibba, M. (2014). tRNAs as regulators of biological processes. Front. Genet. 5:171. doi: 10.3389/fgene.2014.00171
Rogers, P. D., Vermitsky, J. P., Edlind, T. D., and Hilliard, G. M. (2006). Proteomic analysis of experimentally induced azole resistance in Candida glabrata. J. Antimicrob. Chemother. 58, 434–438. doi: 10.1093/jac/dkl221
Schwarzmüller, T., Ma, B., Hiller, E., Istel, F., Tscherner, M., Brunke, S., et al. (2014). Systematic phenotyping of a large-scale Candida glabrata deletion collection reveals novel antifungal tolerance genes. PLoS Pathog. 10:e1004211. doi: 10.1371/journal.ppat.1004211
Seider, K., Brunke, S., Schild, L., Jablonowski, N., Wilson, D., Majer, O., et al. (2011). The facultative intracellular pathogen Candida glabrata subverts macrophage cytokine production and phagolysosome maturation. J. Immunol. 187, 3072–3086. doi: 10.4049/jimmunol.1003730
Seider, K., Gerwien, F., Kasper, L., Allert, S., Brunke, S., Jablonowski, N., et al. (2014). Immune evasion, stress resistance, and efficient nutrient acquisition are crucial for intracellular survival of Candida glabrata within macrophages. Eukaryot. Cell. 13, 170–183. doi: 10.1128/EC.00262-13
Siikala, E., Bowyer, P., Richardson, M., Saxen, H., Sanglard, D., and Rautemaa, R. (2011). ADH1 expression inversely correlates with CDR1 and CDR2 in Candida albicans from chronic oral candidosis in APECED (APS-I) patients. FEMS Yeast Res. 11, 494–498. doi: 10.1111/j.1567-1364.2011.00739.x
Teng, X., Dayhoff-Brannigan, M., Cheng, W. C., Gilbert, C. E., Sing, C. N., Diny, N. L., et al. (2013). Genome-wide consequences of deleting any single gene. Mol. Cell. 52, 485–494. doi: 10.1016/j.molcel.2013.09.026
Tijsterman, M., Ketting, R. F., and Plasterk, R. H. (2002). The genetics of RNA silencing. Annu. Rev. Genet 36, 489–519. doi: 10.1146/annurev.genet.36.043002.091619
Todisco, S., Di Noia, M. A., Castegna, A., Lasorsa, F. M., Paradies, E., and Palmieri, F. (2014). The Saccharomyces cerevisiae gene YPR011c encodes a mitochondrial transporter of adenosine 5′-phosphosulfate and 3′-phospho-adenosine 5′-phosphosulfate. Biochim. Biophys. Acta. 1837, 326–334. doi: 10.1016/j.bbabio.2013.11.013
Vale-Silva, L., Beaudoing, E., Tran, V. D. T., and Sanglard, D. (2017). Comparative genomics of two sequential Candida glabrata clinical isolates. G3. 7, 2413–2426. doi: 10.1534/g3.117.042887
Vale-Silva, L. A., and Sanglard, D. (2015). Tipping the balance both ways: drug resistance and virulence in Candida glabrata. FEMS Yeast Res. 15:fov025. doi: 10.1093/femsyr/fov025
Van Dijck, P., De Rop, L., Szlufcik, K., Van Ael, E., and Thevelein, J. M. (2002). Disruption of the Candida albicans TPS2 gene encoding trehalose-6 phosphate phosphatase decreases infectivity without affecting hypha formation. Infect. Immunol 70, 1772–1782. doi: 10.1128/IAI.70.4.1772-1782.2002
Vermitsky, J. P., Earhart, K. D., Smith, W. L., Homayouni, R., Edlind, T. D., and Rogers, P. D. (2006). Pdr1 regulates multidrug resistance in Candida glabrata: gene disruption and genome-wide expression studies. Mol. Microbiol. 61, 704–722. doi: 10.1111/j.1365-2958.2006.05235.x
Wilkinson, M. F. (2019). Genetic paradox explained by nonsense. Nature 568, 179–180. doi: 10.1038/d41586-019-00823-5
Keywords: Candida glabrata, pathogenic yeast, RNA interference, RNAi, gene library, antifungal drugs, virulence factors, macrophages
Citation: Ishchuk OP, Ahmad KM, Koruza K, Bojanovič K, Sprenger M, Kasper L, Brunke S, Hube B, Säll T, Hellmark T, Gullstrand B, Brion C, Freel K, Schacherer J, Regenberg B, Knecht W and Piškur J (2019) RNAi as a Tool to Study Virulence in the Pathogenic Yeast Candida glabrata. Front. Microbiol. 10:1679. doi: 10.3389/fmicb.2019.01679
Received: 05 March 2019; Accepted: 08 July 2019;
Published: 24 July 2019.
Edited by:
Ana Traven, Monash University, AustraliaReviewed by:
Aaron Mitchell, Carnegie Mellon University, United StatesAlessia Buscaino, University of Kent, United Kingdom
Copyright © 2019 Ishchuk, Ahmad, Koruza, Bojanovič, Sprenger, Kasper, Brunke, Hube, Säll, Hellmark, Gullstrand, Brion, Freel, Schacherer, Regenberg, Knecht and Piškur. This is an open-access article distributed under the terms of the Creative Commons Attribution License (CC BY). The use, distribution or reproduction in other forums is permitted, provided the original author(s) and the copyright owner(s) are credited and that the original publication in this journal is cited, in accordance with accepted academic practice. No use, distribution or reproduction is permitted which does not comply with these terms.
*Correspondence: Olena P. Ishchuk, ishchuk@chalmers.se; olenkaishchuk@gmail.com