- Centre d’Estudis Avançats de Blanes, CEAB-CSIC, Girona, Spain
Sponge diversity has been reported to decrease from well-preserved to polluted environments, but whether diversity and intra-species variation of their associated microbiomes also change as function of environmental quality remains unknown. Our study aimed to assess whether microbiome composition and structure are related to the proliferation of some sponges and not others under degraded conditions. We characterized the most frequent sponges and their associated bacteria in two close areas (impacted and well-preserved) of Nha Trang Bay (Indo-Pacific). Sponge assemblages were richer and more diverse in the well-preserved reefs, but more abundant (individuals/m. transect) in the impacted environments, where two species (Clathria reinwardti and Amphimedon paraviridis) dominated. Sponge microbiomes from the polluted zones had, in general, lower bacterial diversity and core size and consequently, higher intra-species dispersion than microbiomes of sponges from the well-preserved environments. Microbial communities reflect the reduction of diversity and richness shown by their host sponges. In this sense, sponges with less complex and more variable microbiomes proliferate under degraded environmental conditions, following the ecological paradigm that negatively correlates community diversity and environmental degradation. Thereby, the diversity and structure of sponge microbiomes might indirectly determine the presence and proliferation of sponge species in certain habitats.
Introduction
Sponges are key invertebrates in marine benthic ecosystems where they play essential functions in many ecological processes. Besides increasing benthic diversity by supplying ecological niches to other organisms, they contribute to benthic–pelagic coupling by exchanging particulate and dissolved organic matter with the water column (Richter et al., 2001; Morganti et al., 2017). Sponges also participate in marine biogeochemical fluxes and some species may also show detoxifying potential by transforming noxious products present in polluted waters through the interplay of symbiotic bacteria (Loredana et al., 2017).
Microbes have been intimate partners of sponges since the Pre-Cambrian (Wilkinson, 1984). They can represent up to ca. 50% in volume of the microbial-sponge holobiont in some sponge species (Uriz et al., 2012) and are taxonomically and metabolically diverse in most cases (Weisz et al., 2007; Thomas et al., 2016). Thus, it is hard to envisage the causes of sponge success or failure without considering its accompanying bacterial microbiome. Indeed, unbalancing of their microbial symbioses has been considered to trigger extensive mass mortalities of sponges in the Mediterranean (Webster et al., 2008; Cebrian et al., 2011), and Red Sea (Gao et al., 2014). In addition, some purported benefits that sponges may obtain from their associations with microbes have also been proposed such as, antifungal activity, production of bioactive compounds against predation, roles in nitrogen and carbon cycle and vitamin biosynthesis (Schmidt et al., 2000; Freeman and Thacker, 2011; Hentschel et al., 2012; Freeman et al., 2013), but rarely have been experimentally demonstrated (de Voogd et al., 2015; Garate et al., 2015).
In contrast to the spatial and temporal variations reported for microbial communities in seawater (Zeglin, 2015; Glasl et al., 2017), the structure of the sponge microbiome does not vary substantially in the same species along geographical and bathymetrical ranges or over temperature, eutrophication or irradiance shifts (Hentschel et al., 2002; Erwin et al., 2012; Pita et al., 2013a,b; Luter et al., 2014; Strand et al., 2017). Although several experimental studies reported microbiome shifts under strong environmental changes in some host species (Mohamed et al., 2008; Fan et al., 2013; Lesser et al., 2016; Webster et al., 2016; Pineda et al., 2017; Weigel and Erwin, 2017; Glasl et al., 2018; Ramsby et al., 2018) they only inform us about short term changes, which might reflect temporal responses to environmental stresses.
Sponges usually show a high species diversity in well-preserved ecosystems (Van Soest et al., 2012), which has been suggested to decrease under anthropogenic pressures (Easson et al., 2015). Shifts in nutrient cycling and ecosystem functioning, which occur in degraded reef systems (Bell et al., 2013, 2018; Easson et al., 2015), are considered to be responsible for decreases in sponge biodiversity. However, a few, likely opportunistic, sponge species have been reported to inhabit and even dominate degraded coral reefs (Maliao et al., 2008). How these sponges cope with the potentially noxious products of polluted waters and whether particular sponge-associated bacteria are involved or not in their ecological success are challenging issues poorly understood (Douglas, 2014). Indeed, little is known about the role (if any) that symbiotic bacteria may play in shaping the ecological distribution of sponge species and whether the decrease of sponge diversity observed in perturbed assemblages also involves a lower diversity of their associated bacterial communities.
In this study, we explored whether microbiomes of sponges inhabiting degraded environments show differential characteristics, in such a way that they might influence the sponge distribution and proliferation in those adverse conditions. With this goal, we characterized the most frequent sponges and their associated bacteria in two close areas of Nha Trang Bay (Indo-Pacific) subjected to contrasting environmental conditions: well-preserved and impacted coral systems. In particular, we looked for sponge-associated bacteria in the few sponge species able to proliferate in the polluted zones.
Nha Trang bay is located in central Vietnam and harbors one of the highest coral diversities in the area (Latypov, 2011). Unfortunately, the health of the local ecosystems is being threatened by an increase of human activities leading to an alarming degradation of the bay in some zones (Latypov, 2015). The islands located closer to the city and the port of Nha Trang show a higher degree of anthropogenic impact, with higher sedimentation fluxes and lower water transparency compared to the ones located farther from the coast line (Latypov, 2006; Tkachenko et al., 2016). Moreover, Vietnam has been ranked the world’s third largest producer of farmed food (Nguyen et al., 2016) and certain areas of Nha Trang bay are highly impacted by mariculture activities, which also produce eutrophication and release of xenobiotics to the water (Nguyen et al., 2016). Tkachenko et al. (2016) reported the concentration of nutrients in areas close to our sampling sites. The nutrient values were 2.63 μM ± 0.21 for dissolved inorganic nitrogen and 0.29 μM ± 0.11 for phosphorus at the impacted sites (culture cages) and 2.41 μM ± 0.2 and 0.28 μM ± 0.08 at the unperturbed sites. As a result, the native Acropora coral assemblages have been replaced by the more resistant to silting Millepora communities in these perturbed areas (Latypov, 2015; Tkachenko et al., 2016). Conversely, several outer areas of Nha Trang Bay, such as Hun Mun, receive a low anthropogenic impact and still present well-developed Acropora communities with high coral coverage (ca. 60–70%) (Tkachenko et al., 2016).
Materials and Methods
Sample Collection and DNA Extraction
Quantitative sponge sampling was approached by SCUBA diving by randomly placing a total of 13, 25 m-long transect lines between 3–9 m depth along both well-preserved and impacted areas of Nha Trang Bay (Supplementary Figure S1). This quantitative method has been traditionally used for biodiversity studies in coral reefs and other structurally complex habitats (Loya, 1978) and provides a good approach on the abundance (density or coverage) of the non-cryptic fraction of the reef benthos. Specimens that were crossed by the metric tape along the line transect were sampled. The well-preserved areas were coral reefs and rocky shores of the eastern part of Hun Mun Island and the southern part of Hun Tre Island, considered to be the most well preserved areas of the bay (Tkachenko et al., 2016). The impacted targeted zones were 2 km apart from the well-preserved areas, next to Dambay region, which harbors an intensive mariculture system (lobster caging) that causes chronic eutrophication in the area (Tkachenko et al., 2016). Overall, the quantitative sampling provided 203 sponge samples, from which 71 individuals were from the impacted areas and 132 individuals from the well-preserved environments.
Each sponge individual fitting within a line transect was photographed and a piece of ca. 3 cm2 (whenever possible) was collected in a 50 mL Falcon tube with seawater. Seawater was immediately replaced by 100% ethanol once on board. Back in the lab, the ethanol was replaced twice with fresh absolute ethanol again for a good sample preservation. DNA was extracted following the protocol of DNeasy Blood & Tissue Kit (Qiagen). Triplicate plankton samples were taken from the three sampling locations where the ecological transects were performed (i.e., Dambay, Hun Mun and Nock Island). Two liters of water were collected and sequentially filtered throughout 5-μm, to remove undesired plankton components, and then throughout 0.22 μm polycarbonate membranes. The size fraction between 5 and 0.22 μm was processed for DNA extraction. Membranes were enzymatically digested with lysozyme, proteinase K and sodium dodecylsulfate and afterward, DNA was extracted with phenol:chloroform-isoamyl alcohol (25:24:1, vol/vol/vol) and chloroform:isoamyl alcohol (24:1, vol/vol).
Sponge species were previously identified in Turon et al. (2018) by morphological features (Hooper and Van Soest, 2002) and molecular markers. Preparation of spicules and histological sections were made from specimens’ subsamples and observed under both light and scanning electron microscopes.
16S rRNA Gene Sequencing and Processing
Only representative sponge species (n = 18) at each environment, which appeared replicated in the quantitative sampling, were used for the study of microbial symbionts. Replicates varied from 2 to 9, depending on the species abundance (n. individuals) and material availability (sponge fragment size), as the sampling was intended to reflect the sponge diversity and abundance at each environment (Table 1). PCR and high-throughput multiplexed 16S rRNA gene amplicon Illumina MiSeq sequencing, were carried out following the genomic core facilities and methods of the MrDNA Lab (Texas, United States)1. The variable V4 region of the bacterial 16S rRNA gene was amplified using the primers 564F (5′AYTGGGYDTAAAGNG-3′) and 785R (5′TACNVGGGTATCTAATCC-3′) (c.a. 250 nt) (Klindworth et al., 2013). Raw rRNA gene sequences were processed separately using the UPARSE pipeline (Edgar, 2017). A quality check was applied to our dataset with the fastq_filter command and the arguments –fastq_trunclen 208 –fastq_maxee 0.25. Sequences were then dereplicated with the – derep_fulllength command and sorted by size (-sortbysize command) in Usearch 9.2 version. Denoising (error-correction) of amplicons was performed following the UNOISE pipeline (Edgar, 2016) using the - unosie2 command. This algorithm removed chimeras, reads with sequencing errors, PhiX, and low complexity sequences due to Illumina artifacts, and generates ZOTUs (“zero-radius” OTUs) with 100% identity sequences. Finally, -usearch_global command with identity threshold set at 0.97 was applied to our dataset. Taxonomic assignment was done with SINA v1.2.11 (Pruesse et al., 2012) using SILVA 128 database. Sequences with low identity (< 75%) and sequences identified as mitochondria or chloroplasts were removed from the analysis. In order to minimize biased effects for differences in sampling effort, the original bacterial ZOTU table was rarefied at a minimum reads threshold of 41000, using QIIME (Caporaso et al., 2010).
Sponge and Bacterial Community Analysis
Statistical analyses were run in the R environment (R Core Team, 2013). Community ecology related parameters were calculated using the vegan v2.5-1 (Oksanen et al., 2017) and iNEXT v2.0.15 packages (Hsieh et al., 2018), and figures were drawn with ggplot2 3.0.0 (Wickham, 2009).
We determined sponge species composition on impacted and well-preserved environments: the number of sponge individuals was standardized per meter of sampled transect to minimize the bias of the sampling effort in the two habitats. Shannon diversity indices and species richness were calculated for both environments using the ChaoEntropy and ChaoSpecies functions, respectively, and integrated curves that smoothly link rarefaction (interpolation) and prediction (extrapolation) were computed for both variables (Chao et al., 2014) to facilitate their comparison of between habitats (Supplementary Figure S2). Additionally, we used the specaccum function with 1000 permutation of the vegan package to represent the accumulated number of species per sampled meter in each habitat (Supplementary Figure S3).
A Venn diagram of total species richness in both environments was generated using the eulerr package (Larson et al., 2018) and t-tests were conducted to assess differences in community metrics between sites. We calculated the Bray–Curtis dissimilarity of species composition of all transects to assess the beta diversity patterns between environments.
We performed a hierarchical cluster analysis (Ward method) based on Bray–Curtis dissimilarity matrix using the rarefied ZOTU table to determine whether sponge bacterial communities were more similar among replicates from the same species than between impacted and well-preserved environments. To test the effects of host identity and environment on structuring the sponge bacterial communities, we used PERMANOVA (Anderson, 2001) based on 999 permutations as implemented in adonis function. A heatmap was generated for the most abundant bacterial families (>1% relative abundance in any of the samples). We performed and Indicator Value (IndVal) (Dufrêne and Legendre, 1997) analysis to detect if particular microbial taxa were differentially found at each site using the labdsv package (Roberts and Roberts, 2016) in R. We set the Indval threshold to 0.7 and the p-value for significance at 0.01.
We compared three main bacterial community features between the sponge assemblages from impacted and well-preserved environments: (i) intra-species dispersion (beta diversity), (ii) Shannon diversity and (iii) core size (explained below). For these comparisons, we included Amphimedon sulcata and Mycale (Arenochalina) sp., for which we had replicates in the impacted and well-preserved environments, within the category of sponges able to survive in impacted sites.
Only two species inhabited both environments [A. sulcata and Mycale (Arenochalina) sp.]. However, only one individual of Mycale (Arenochalina) sp. was found in the well-preserved habitats, preventing statistically based comparisons between environments for this species. Betadisper and Shannon functions were used to calculate beta and alpha diversity measures, respectively. We defined the bacterial core of each sponge species considering the ZOTUs present across all species replicates. The core size of each individual represented the percentage of core bacteria (of the sponge species) with respect to the total bacteria present in that individual. T-test and Kruskal–Wallis test were used to detect significant differences between sponge species from impacted and well-preserved environments. Pearson correlation was used to detect the relationship between intra-species dispersion and bacterial core size for each sponge species.
Results
Sponge Assemblages in Two Contrasting Environments
A total of 71 sponge species were identified in the overall sampling area (Figure 1). The impacted and well-preserved environments showed strong differences in terms of sponge richness, diversity, and density (Figure 1 and Supplementary Figure S2). Sponge richness and diversity were much lower in the impacted sites than in the well-preserved environments (t-test: p-value = 0.039, p-value = 0.037, respectively), although the respective rarefaction curves per number of individuals sampled did not reach the saturation point, in particular for species richness (Supplementary Figure S2). The rarefaction curves of accumulated species per sampled area neither reached the saturation point (Supplementary Figure S3).
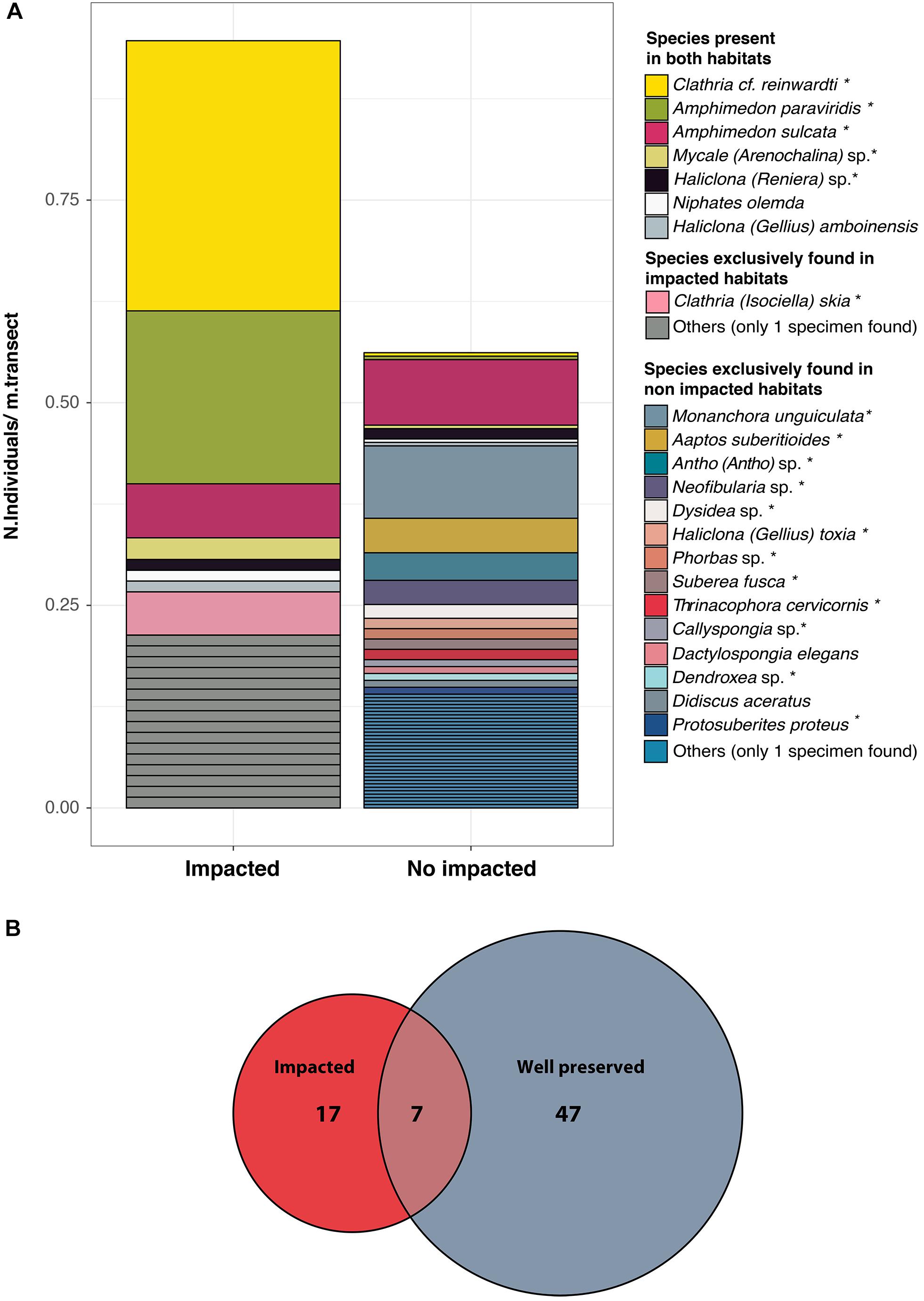
Figure 1. (A) Bar plots of sponge species composition and abundance at the well-preserved and impacted habitats. Y-axis represents the numbers of individuals per meter of transect. (B) Venn diagram of total species richness and the species overlap between well-preserved (gray) and impacted (red) environments. ∗ Indicates species for which microbiome has been analyzed.
Conversely, the overall sponge density was higher (t-test: p-value = 0.05) in the impacted (0.94 individuals/m) than in the well-preserved environments (0.56 individuals/m). Most species were environment-specific with only seven species found in both environments, though all of them but A. sulcata were much more abundant at the polluted sites (Figure 1). The well-preserved habitats harbored a more diverse and evenly distributed sponge assemblage with Monanchora unguiculata, A. sulcata, Antho (Antho) sp., Aaptos suberitoides, and Neofibularia sp. (Figures 1, 2) being the most abundant species. Conversely, the impacted habitats showed a community mostly dominated by Clathria reinwardti and A. paraviridis (Figures 1, 2) (>50% of the specimens sampled). Moreover, significant differences in beta diversity for species composition were found between sites (adonis, F = 2.72, df = 1, R2 = 0.19, P = 0.01).
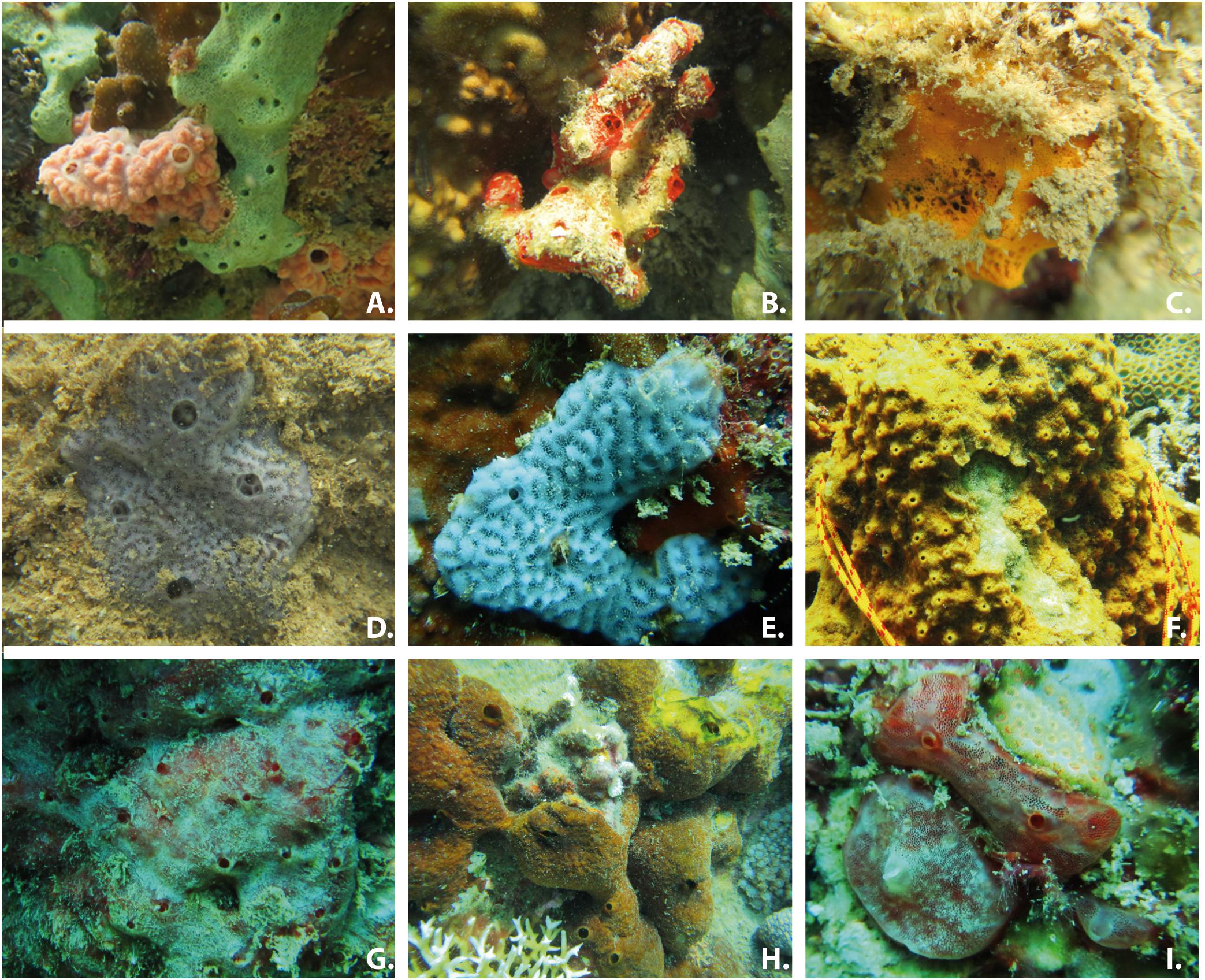
Figure 2. Pictures of the most common sponges of the impacted (A–D) and the well-preserved (E–I) habitats: (A) Amphimedon paraviridis (greenish) and Clathria reinwardti (pinkish), (B) Clathria (Isociella) skia, (C) Mycale (Arenochalina) sp., (D) Amphimedon sulcata (in the impacted environment), (E) A. sulcata (in the well-preserved environment), (F) Neofibularia sp., (G) Antho (Antho) sp., (H) Aaptos suberitioides and (I) Monanchora unguiculata.
Sponge-Associated Bacterial Communities
We obtained a total of 15,712 high-quality ZOTUs (Zero-radius Operational Taxonomic Unit, 100% identity) corresponding to 48 bacterial phyla, with Proteobacteria (52.9%), Actinobacteria (2.8%), Acidobacteria (2.4%), Chloroflexi (2%), and Planctomycetes (1.7%) being the most abundant taxa. Bacterial communities of HMA sponges (Neofibularia sp., A. suberitoides, and Suberea fusca clearly differed from those of LMA sponges (Figure 3). Chloroflexi, PAUC34f, Caldilineaceae and Sva0996 marine group were consistently associated to the HMA sponges.
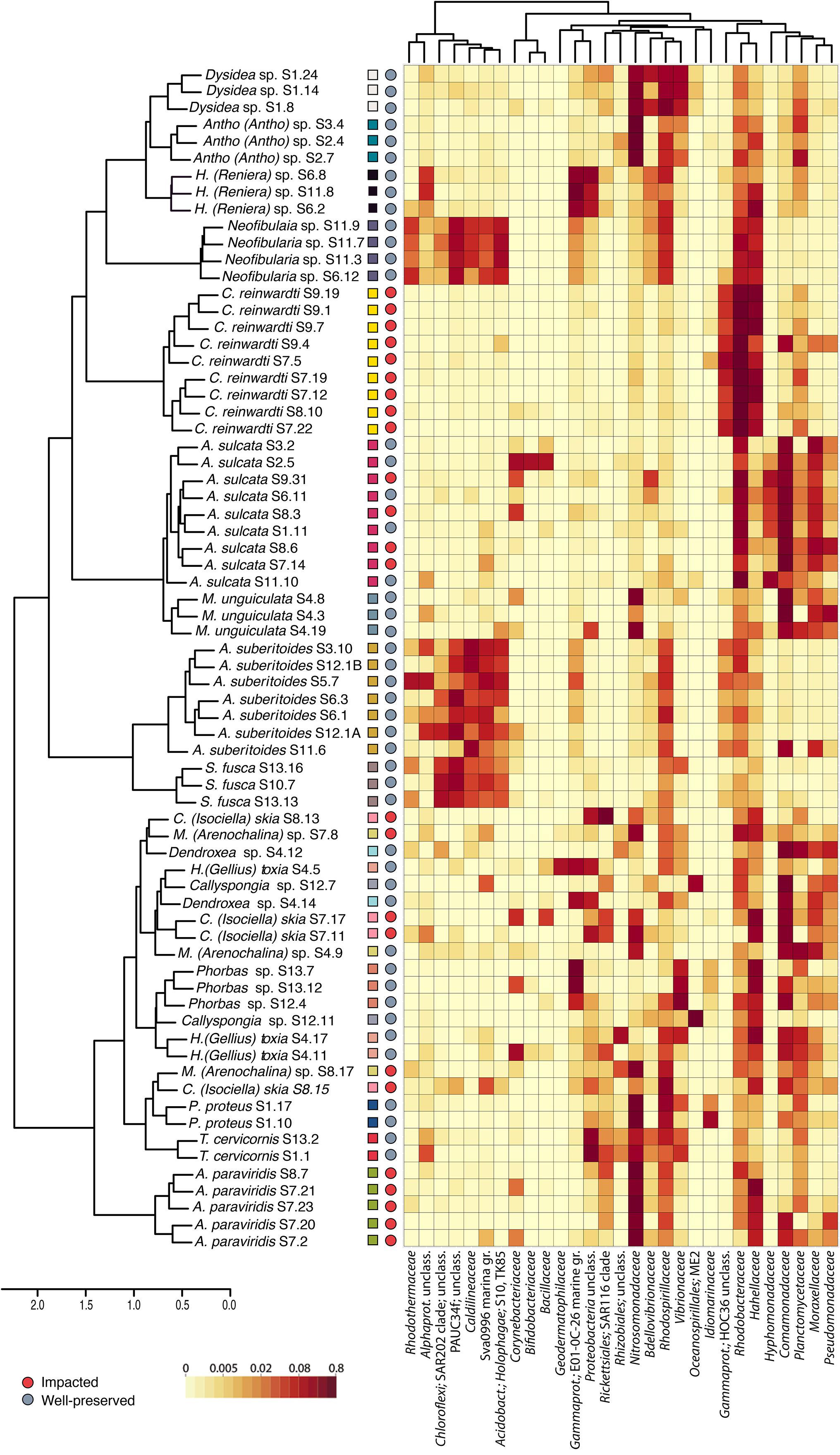
Figure 3. Heatmap of the sponge bacterial composition at the family level. Only taxa with relative abundances higher than 1% in any of the samples are shown, with abundance represented in the color temperature bar. Sponge samples (y-axis) and bacterial taxa (x-axis) are organized according to a hierarchical clustering based on Bray-Curtis dissimilarity matrices (at the level of ZOTU and family, respectively). Square colors correspond to different sponge species, and circle colors indicate the site of collection: impacted (red) or well-preserved (gray).
The cluster analysis showed that the sponge-associated bacterial communities were closely related to their host-species regardless of the environment (impacted vs. well-preserved) where the sponges were living, with, in general, low variation among replicates of a species (Figure 3). Indeed, we found a strong effect of host identity on the composition of the sponge microbiomes (adonis: Pseudo-F: 4.46, R2 = 0.593, p < 0.001), but a negligible effect of the environment (adonis: Pseudo-F: 1.07, R2 = 0.008, p > 0.05). Thus, each sponge species presented a unique microbiome that differed from that of other sponges and from that of the surrounding seawater (Supplementary Figure S4), even if they were living in the same environment (Figure 3). Planktonic communities showed significant differences in bacterial composition between types of habitats (adonis: Pseudo-F: 2.2, R2 = 0.23, p < 0.05, Supplementary Figure S4A). Shannon diversity was higher for the seawater bacterial communities in the well-preserved habitat than in the impacted habitat, but differences were only statistically significant at an alpha value of 0.07 (Kruskal–Wallis test: p-value = 0.07), likely due to the unbalanced design (3 vs. 6 replicates) and the large variation in the Shannon diversity Index across replicates at the polluted environment (Supplementary Figure S4B).
We looked in further detail into the bacterial composition of the two purportedly opportunistic species that dominate the perturbed (polluted) environments (C. reinwardti and Amphimedon paraviridis). A common feature of these two species was that their bacterial communities were dominated by a few taxa such as Candidatus Branchiomonas (42.85 ± 12.5%) and Endozoicomonas (12.74 ± 12.1%) in A. paraviridis, and members of the family Rhodobacteraceae (58.54 ± 22%) and Endozoicomonas (21.63 ± 12.9%) in C. reinwardti (Figure 3). In both sponges, only two bacterial taxa achieved >60% of the whole microbiome. Moreover, these two taxa were consistently found across all species replicates (i.e., they belong to the sponge core). A single C. Branchiomonas ZOTU made up 56% of the total core reads of A. paraviridis and 6 ZOTUs belonging to Rhodobacteraceae family made up 64% of the total core reads in C. reinwardti. Moreover, both species had in their core communities ZOTUs belonging to Endozoicomonas and Shewanella at abundances higher than 10 and 1%, respectively. Indval analysis (Supplementary Figure S5) showed that some bacteria were significantly associated to the impacted environment (Indval > 0.7, p-value < 0.01) but only Rhodobacteraceae and Shewanella were found at high abundances in the sponges of that habitat.
Between Environment Comparisons: General Microbiome Ecological Descriptors
To test for microbiome differences among replicates of the same species living in the two environments, we focused on the unique species equally found in both habitats: Amphimedon sulcata. No influence of the environment could be detected for inter-individual variation (PERMANOVA: R2 = 0.128, p > 0.05) and no differences (t-test, p-value > 0.05) were detected for intra-species dispersion, core size and Shannon diversity of the A. sulcata bacterial communities between individuals from both environments.
Intra-species dispersion of the sponge microbiomes was species-specific in all the species tested, with Neofibularia sp., Thrinacophora cervicornis, and A. suberitoides having the lowest dispersions and Mycale (Arenochalina) sp., Clathria (Isociella) skia, and A. paraviridis having the highest (Supplementary Figure S6A). Interestingly, intra-species dispersions were higher in impacted than in well-preserved environments (t-test: p-value < 0.001; Figure 4A). That is, the bacterial communities of sponge replicates from well-preserved environments are more similar to each other than seen for those sponge replicates from impacted environments. Consequently, intra-species dispersion was negatively correlated to the size of the sponge bacterial core (Rp = −0.65, p-value < 0.01: Supplementary Figure S7): the higher the intra-species dispersion, the lower the size of the core and the larger the variable bacterial community of the sponge species. C. reinwardti, Mycale (Arenochalina) sp. and A. paraviridis had the smallest core sizes (10.55, 17.34 and 18.44%, respectively), whereas T. cervicornis, Protosuberites proteus, Monanchora unguiculata, and Neofibularia sp. (43.71, 41.78, 41.73, and 41.72%, respectively) had the largest ones (Supplementary Figure S6B). Overall, the bacterial core sizes of the sponge species living in the impacted environments were lower (Kruskal–Wallis test: p-value < 0.001) than those of the sponges living in the well-preserved environments (Figure 4B). Shannon diversity indices (H′) of the sponge microbiomes were also species-specific and ranged from 2.7 to 4.7 (mean values for C. reinwardti and S. fusca, respectively) (Supplementary Figure S6C), which were lower in impacted than in well-preserved environments (Kruskal–Wallis test: p-value < 0.001) (Figure 4C).
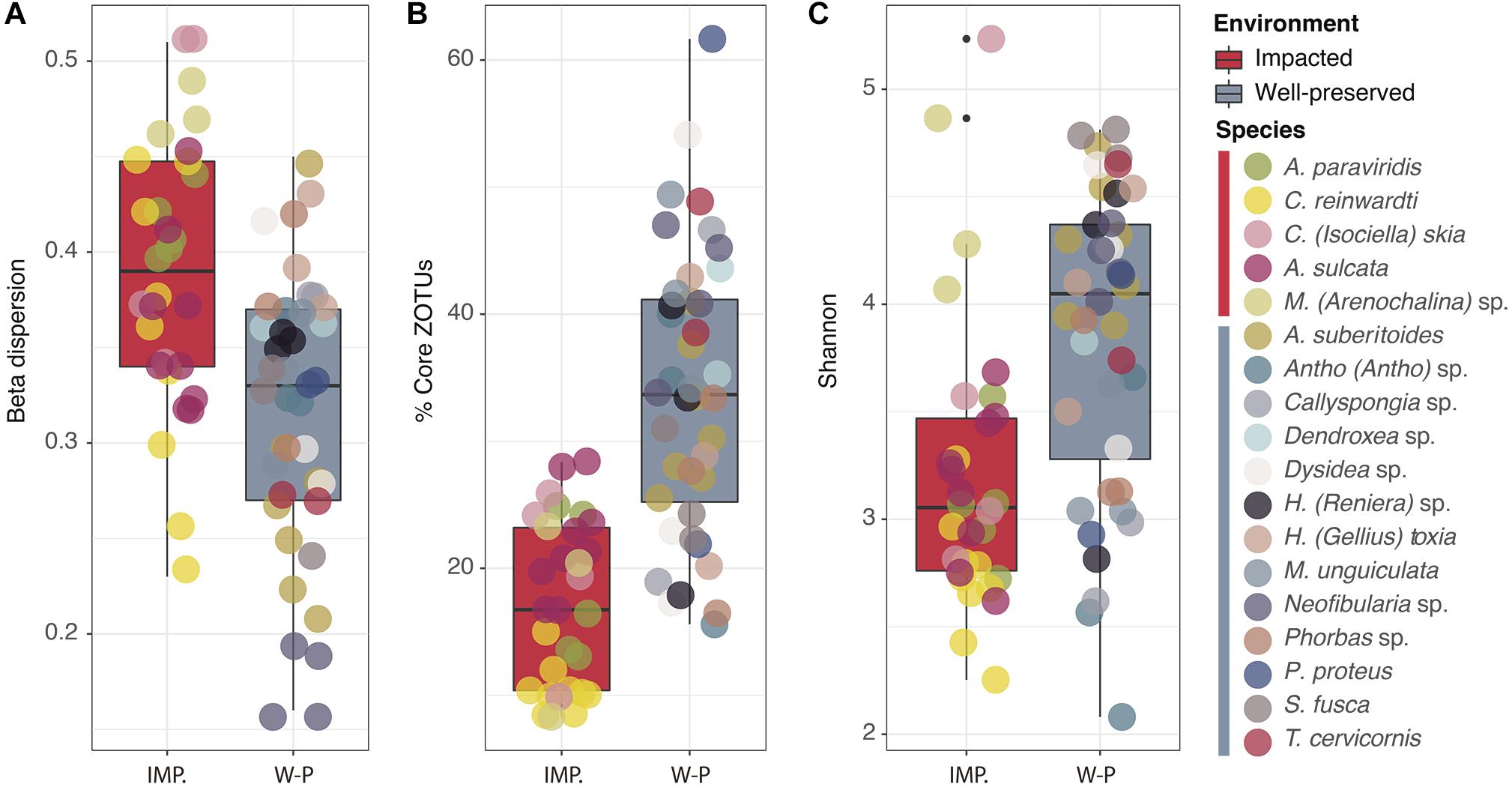
Figure 4. Box plots comparing the intra-species dispersion (A), core size (B) and Shannon diversity (C) of the sponge microbiomes between impacted (red) and well-preserved (gray) environments. Replicates of the same species are depicted in the same dot color.
To sum up, microbiomes of sponge species living in the impacted environment had in general, higher intra-species dispersion, lower core size and lower bacterial diversity, than the microbiomes of sponges living in the well-preserved environments (Figure 5).
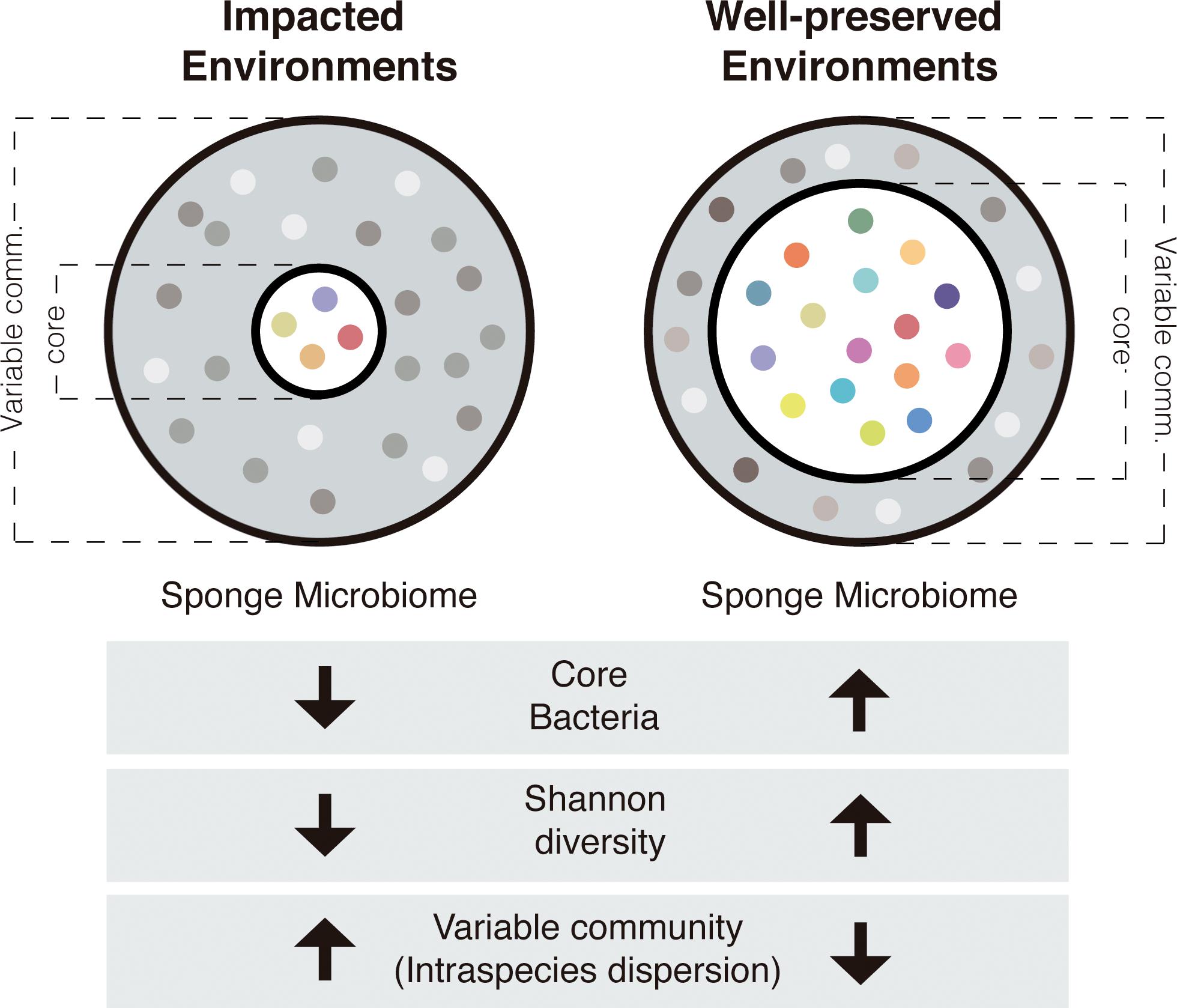
Figure 5. Schematic representation of the sponge microbiomes in impacted and well-preserved environments. Gray fraction represents the variable community and white fraction represents the core community. Each colored dot corresponds to a single bacterial species.
Discussion
Sponge Ecology: Well-Preserved vs. Impacted Environments
We have found clear differences between the sponge assemblages from well-preserved and impacted environments of Nha Trang Bay, with different species, lower sponge richness and diversity but higher sponge density (individuals/m.) in the perturbed zones. Similar decrease in species richness (S) and Shannon diversity index (H′) due to anthropogenic impacts has been previously reported for the coral communities in the study area (Tkachenko et al., 2016) and for sponge diversity in different oceans (Powell et al., 2014; Easson et al., 2015). Indeed, low diversity but high abundance with dominance of a few species is a common feature of many polluted habitats (Piola and Johnston, 2008; Powell et al., 2014). However, although our sampling captured differences in diversity between habitats quite acceptably, rarefaction curves for accumulated species predict an insufficient sampling effort to completely catch the true species richness.
In the Nha Trang region, marine cultures are producing chronic eutrophication and high sedimentation rates in some areas. As expected, the composition of sponge communities severely changes in those perturbed areas, with only a few sponge species inhabiting there. Body architecture and physiological traits, such as sediment removing mechanisms through mucus production (Bell et al., 2015; Schönberg, 2015, 2016) have been reported and allow sponges to survive under anomalous sedimentation rates (Pineda et al., 2017; Strehlow et al., 2017), as those resulting from severe eutrophication (Ralph et al., 2006). Some of these mechanisms are indeed displayed by the most abundant sponge species inhabiting the polluted habitat of Nha Trang (e.g., C. reinwardti, A. paraviridis, A. sulcata), as the sponge surfaces appear completely clean whereas a dense layer of sediment covers the remaining substrate (Figure 2). However, a series of xenobiotic compounds, resulting from an intense mariculture, are also released to the water at the impacted study area (Nguyen et al., 2016), so that biological/ecological sponge traits other than resistance to sedimentation, are expected to contribute to the observed abundance of a few species in those areas.
Sponge Microbiomes: Shannon Diversity, Intra-Species Dispersion and Core Community
The bacterial communities of the study sponges show a high fidelity to the sponge species independently of the environmental conditions where the host species are living, as reported for many other sponge species (Luter et al., 2012, 2014; Simister et al., 2012; Bosch and Miller, 2016; Webster and Thomas, 2016; Gantt et al., 2017; Glasl et al., 2018).
Experimental studies repeatedly show a high resilience of the sponge microbiota in species exposed to a range of environmental variables, such as irradiance, temperature, salinity, acidification, and contrasting habitats (Erwin et al., 2012; Pita et al., 2013a, b; Cárdenas et al., 2014; Luter et al., 2014; Ribes et al., 2016; Glasl et al., 2017). A similar absence of effects on microbiome composition, and diversity was reported for the sponge Gelliodes obtusa under eutrophic conditions from mariculture (Baquiran and Conaco, 2018). As in the mentioned study, similar microbiomes were found in our study sponge A. sulcata, inhabiting either well-preserved or polluted environments. Thus, some haplosclerid sponges seem to tolerate eutrophication pressures although they can also inhabit well-preserved habitats, suggesting that they are sponges able to grow in a broad range of environmental conditions.
Most of the above-mentioned experimental studies focus on ecological adaptation of the sponge microbiomes to the assayed conditions but instead they found microbiome stability across treatments. Although surprising at first sight, microbiome resilience is indeed logical if we consider the concept of hologenome evolution (Rosenberg and Zilber-Rosenberg, 2018) and that these microbial-eukaryote symbioses have been evolutionarily fixed thousands of years ago (Sirová et al., 2018). Seemingly, structural microbiome stability has also been reported for few coral species across environmental gradients, which suggests resilience of microbiomes to environmental fluctuations or stress also in corals (Sawall et al., 2014; Grottoli et al., 2018; Pogoreutz et al., 2018).
Sponge microbiomes are species-specific in both environments, with no differences between individuals of the same species (i.e., A. sulcata) living at both habitats. However, significant differences in community metrics are revealed when the microbiomes of the sponge assemblages, as a whole, were compared between environments, with some particular bacterial groups proliferating in the polluted sites. Thus, even though the conditions of the perturbed environment cannot modify the evolutionarily fixed microbial communities of the sponge species (i.e., microbiomes do not change as a result of ecological adaptation to environmental conditions in species living at both habitats), they might influence the ecological distribution of the sponge species.
The sponge microbiomes of the polluted sites show a significantly lower Shannon diversity than sponge microbiomes in well-preserved environments. This is in accordance with the agreed general loss of biodiversity in impacted environments (Rygg, 1985). However, community success does not seem to directly depend on its diversity, but on its ability to respond to particular environmental conditions (McCann, 2000; Evans et al., 2017, 2018; Glasl et al., 2018). Indeed, the few species proliferating in the polluted environments of Nha Trang Bay (i.e., C. reinwardti and A. paraviridis) show a high density and a large coverage in the area, regardless of their low diversity microbiomes.
Moreover, the microbiomes of the sponges inhabiting the polluted sites show a higher intra-species dispersion than those of the sponges from well-preserved environments. The high microbiome dispersion in the former might indicate that the sponges living in the polluted environments, despite being visibly healthy are subjected to some stress. The Anna Karenina principle (Zaneveld et al., 2017), which proposes that variability is higher in dysbiotic than in healthy individuals of the same species, might be extended to species assemblages, according to our results. That is, bacterial communities of sponges in general, would be more variable in impacted than in well-preserved reefs. Similar trends in alpha and beta diversity metrics to those found in these contrasting environments have been also observed in successional stages of bacterial communities from primary, supposedly more stressed, to late or more mature (Ortiz-Álvarez et al., 2018).
The bacterial cores (Astudillo-García et al., 2017), predicted to play crucial roles in the sponge functioning (Cárdenas et al., 2014; Pita et al., 2018), represent a low percentage of the total microbiome in the study sponges inhabiting the polluted site. Consequently, most bacteria in these species are transient and thus, may be site-variable. This is confirmed by a negative correlation between the core size and the intra-species dispersion (variable community) of sponge microbiomes. Conversely, sponges inhabiting the well-preserved study sites contain large diverse bacterial cores that might be difficult to maintain in perturbed habitats, as both, theory and empirical evidence point to the simplification of ecological communities in those habitats (Piola and Johnston, 2008). As for the seawater bacteria communities, Shannon diversity was lower and variation between replicates was higher at the polluted habitats than at the well-preserved environments. Thus, seawater bacteria communities follow a similar pattern as for among replicates variation and alpha diversity than sponge microbiomes at both environments.
Moreover, the simplified microbial systems of the perturbed study habitats contain certain bacterial species that might play a role in the holobiont success.
Rhodobacteraceae members and C. Branchiomonas are major members of core communities of the two dominant sponges in the polluted sites (C. reinwardti and A. paraviridis), pointing to a potential function of these bacteria in the sponge success by facilitating them to cope with some pollutants. The family Rhodobacteraceae includes key players in biogeochemical cycling (Simon et al., 2017) and several members with chemotrophic anaerobic metabolisms, which are able to oxidize the noxious hydrogen sulfide present in eutrophic environments (Zhang et al., 2013). Members of the Nitrosomonadaceae family (i.e., C. Branchiomonas) are reported to be ammonia oxidizers (Prosser et al., 2014) and have been found to be dominant symbionts in sponges, suggesting that they may represent a source of bioavailable nitrogen for their hosts (Matcher et al., 2017). Also remarkable is the high abundance of Endozoicomonas in these sponge species. This genus, with various marine species distributed worldwide (Neave et al., 2016), is commonly found in close associations with sponges (Nishijima et al., 2013). Functions related to sponge health (Gardères et al., 2015), bromopyrrole production (Haber and Ilan, 2014), carbohydrate fermentation/nitrate reduction (Nishijima et al., 2013), and antibiotic production (Rua et al., 2014) in sponge hosts have been proposed (Neave et al., 2016). Thus, sponge-associated Endozoicomonas might play biological functions in the study sponges by participating in the nitrogen and sulfur cycles, influencing the inter-species interactions of the microbial community by producing antimicrobial compounds or signaling molecules, as reported for other sponges (Morrow et al., 2015). Finally, Shewanella is also found in the core of the two species at relatively high abundances at the impacted habitats. Members of this genus have been reported to reduce heavy metals, sulfates, nitrates, and chromates (Fredrickson et al., 2008), which are expected to be abundant in the polluted study habitats due to the antifouling paints containing heavy metals and residuals from industrial activities. The purported functions of the bacteria associated with the dominant sponges in the impacted habitats suggest a plausible role of the sponge microbiomes in detoxification of the seawater at a local scale. Indeed, sponges have been reported to exert a bioremediation effect (Milanese et al., 2003) in zones where they are abundant as they process high volumes of water per day (Leys et al., 2011), but further studies would be needed to confirm the real functionality of these bacteria in our study sponges.
Conclusion
Overall, the microbiomes might play a certain role in determining the presence and proliferation of sponge species in polluted environments. Low core size, low diversity, and high intra-species dispersion are microbiome features shared by the study sponges inhabiting the prospected polluted environments, which suggests that less complex microbiomes are favored under degraded environmental conditions. Thus, the microbial communities associated with sponges mimic the reduction of diversity showed by animal or plant assemblages at the ecosystem scale.
Under the context of climate change, it has been proposed that coral reefs may change to sponge dominated reefs (Bell et al., 2013) due to the lower sensitivity of sponges to ocean acidification and eutrophication. However, few sponges are favored by these stressful conditions and, as shown in the present study, we may expect to face a scenario with less diverse but highly abundant sponge assemblages with low complexity microbiomes.
Data Availability
Raw 16S rRNA gene amplicon sequences are available in the SRA archive under the project number PRJNA453898 (https://www.ncbi.nlm.nih.gov/sra/?term=PRJNA453898). Accession numbers for sponge eukaryotic sequences are reported in the author’s previous publication (Turon et al., 2018).
Author Contributions
MT and MU conceived the study. MT, JC, and XT-M performed the analyses. MT and MU wrote the manuscript. JC, XT-M, and EC commented on later versions of the manuscript.
Funding
This research was partially funded by projects MarSymbiOmics (CTM2013-43287-P) and PopCOmics (CTM2017-88080 and MCIU/AEI/FEDER/UE) from the Spanish MINECO. JC, XT-M, and EC were supported by the project INTERACTOMA (RTI2018-101205-B-I00).
Conflict of Interest Statement
The authors declare that the research was conducted in the absence of any commercial or financial relationships that could be construed as a potential conflict of interest.
Acknowledgments
We thank Daniel Martin (CEAB, Blanes), Temir A. Britayev (RAS, Russia) and Elena S. Mekhova (RAS, Russia) for the help provided in the sponge sampling.
Supplementary Material
The Supplementary Material for this article can be found online at: https://www.frontiersin.org/articles/10.3389/fmicb.2019.01961/full#supplementary-material
Footnotes
References
Anderson, M. J. (2001). A new method for non-parametric multivariate analysis of variance. Austral Ecol. 26, 32–46. doi: 10.1111/j.1442-9993.2001.01070.pp.x
Astudillo-García, C., Bell, J. J., Webster, N. S., Glasl, B., Jompa, J., Montoya, J. M., et al. (2017). Evaluating the core microbiota in complex communities: a systematic investigation. Environ. Microbiol. 19, 1450–1462. doi: 10.1111/1462-2920.13647
Baquiran, J. I. P., and Conaco, C. (2018). Sponge-microbe partnerships are stable under eutrophication pressure from mariculture. Mar. Pollut. Bull. 136, 125–134. doi: 10.1016/j.marpolbul.2018.09.011
Bell, J. J., Davy, S. K., Jones, T., Taylor, M. W., and Webster, N. S. (2013). Could some coral reefs become sponge reefs as our climate changes? Glob. Chang. Biol. 19, 2613–2624. doi: 10.1111/gcb.12212
Bell, J. J., McGrath, E., Biggerstaff, A., Bates, T., Bennett, H., Marlow, J., et al. (2015). Sediment impacts on marine sponges. Mar. Pollut. Bull. 94, 5–13. doi: 10.1016/j.marpolbul.2015.03.030
Bell, J. J., Rovellini, A., Davy, S. K., Taylor, M. W., Fulton, E. A., Dunn, M. R., et al. (2018). Climate change alterations to ecosystem dominance: how might sponge-dominated reefs function? Ecology 99, 1920–1931. doi: 10.1002/ecy.2446
Bosch, T. C. G., and Miller, D. J. (2016). The Holobiont Imperative: Perspectives from Early Emerging Animals. Berlin: Springer.
Caporaso, J. G., Kuczynski, J., Stombaugh, J., Bittinger, K., Bushman, F. D., Costello, E. K., et al. (2010). QIIME allows analysis of high- throughput community sequencing data. Nat. Methods 7, 335–336.
Cárdenas, C. A., Bell, J. J., Davy, S. K., Hoggard, M., and Taylor, M. W. (2014). Influence of environmental variation on symbiotic bacterial communities of two temperate sponges. FEMS Microbiol. Ecol. 88, 516–527. doi: 10.1111/1574-6941.12317
Cebrian, E., Uriz, M. J., Garrabou, J., and Ballesteros, E. (2011). Sponge mass mortalities in a warming mediterranean sea: are cyanobacteria-harboring species worse off? PLoS One 6:e20211. doi: 10.1371/journal.pone.0020211
Chao, A., Gotelli, N., Hsieh, T., Sander, E., Ma, K. H., Colwell, R. K., et al. (2014). Rarefactionand extrapolation with Hill numbers: a framework for sampling and estimation in species diversity studies. Ecol. Monogr. 84, 45–67. doi: 10.1890/13-0133.1
de Voogd, N. J., Cleary, D. F. R., Polónia, A. R. M., and Gomes, N. C. M. (2015). Bacterial community composition and predicted functional ecology of sponges, sediment and seawater from the thousand islands reef complex, West Java, Indonesia. FEMS Microbiol. Ecol. 91:fiv019. doi: 10.1093/femsec/fiv019
Douglas, A. E. (2014). Symbiosis as a general principle in eukaryotic evolution. Cold Spring Harb. Perspect. Biol. 6, 1–14. doi: 10.1101/cshperspect.a016113
Dufrêne, M., and Legendre, P. (1997). Species assemblages and indicator species: the need for a flexible asymmetrical approach. Ecol. Monogr. 67, 345–366.
Easson, C. G., Matterson, K. O., Freeman, C. J., Archer, S. K., and Thacker, R. W. (2015). Variation in species diversity and functional traits of sponge communities near human populations in Bocas del Toro, Panama. PeerJ 3:e1385. doi: 10.7717/peerj.1385
Edgar, R. C. (2016). UNOISE2: improved error-correction for Illumina 16S and ITS amplicon sequencing. bioRxiv 081257.
Edgar, R. C. (2017). Updating the 97% identity threshold for 16S ribosomal RNA OTUs. bioRxiv 192211. doi: 10.1093/bioinformatics/bty113
Erwin, P. M., Pita, L., López-Legentil, S., and Turon, X. (2012). Stability of sponge-associated bacteria over large seasonal shifts in temperature and irradiance. Appl. Environ. Microbiol. 78, 7358–7368. doi: 10.1128/aem.02035-12
Evans, J. S., Erwin, P. M., Shenkar, N., and López-Legentil, S. (2017). Introduced ascidians harbor highly diverse and host-specific symbiotic microbial assemblages. Sci. Rep. 7:11033.
Evans, J. S., Erwin, P. M., Shenkar, N., and López-Legentil, S. (2018). A comparison of prokaryotic symbiont communities in nonnative and native ascidians from reef and harbor habitats. FEMS Microbiol. Ecol. 94:fiy139. doi: 10.1093/femsec/fiy139
Fan, L., Liu, M., Simister, R., Webster, N. S., and Thomas, T. (2013). Marine microbial symbiosis heats up: the phylogenetic and functional response of a sponge holobiont to thermal stress. ISME J. 7, 991–1002. doi: 10.1038/ismej.2012.165
Fredrickson, J. K., Romine, M. F., Beliaev, A. S., Auchtung, J. M., Driscoll, M. E., Gardner, T. S., et al. (2008). Towards environmental systems biology of Shewanella. Nat. Rev. Microbiol. 6, 592–603. doi: 10.1038/nrmicro1947
Freeman, C. J., and Thacker, R. W. (2011). Complex interactions between marine sponges and their symbiotic microbial communities. Limnol. Oceanogr. 56, 1577–1586. doi: 10.4319/lo.2011.56.5.1577
Freeman, C. J., Thacker, R. W., Baker, D. M., and Fogel, M. L. (2013). Quality or quantity: is nutrient transfer driven more by symbiont identity and productivity than by symbiont abundance? ISME J. 7, 1116–1125. doi: 10.1038/ismej.2013.7
Gantt, S. E., López-Legentil, S., and Erwin, P. M. (2017). Stable microbial communities in the sponge Crambe crambe from inside and outside a polluted Mediterranean harbor. FEMS Microbiol. Lett. 364, 1–7. doi: 10.1093/femsle/fnx105
Gao, Z. M., Wang, Y., Lee, O. O., Tian, R. M., Wong, Y. H., Bougouffa, S., et al. (2014). Pyrosequencing reveals the microbial communities in the red sea sponge Carteriospongia foliascens and their impressive shifts in abnormal tissues. Microb. Ecol. 68, 621–632. doi: 10.1007/s00248-014-0419-0
Garate, L., Blanquer, A., and Uriz, M. J. (2015). Calcareous spherules produced by intracellular symbiotic bacteria protect the sponge Hemimycale columella from predation better than secondary metabolites. Mar. Ecol. Prog. Ser. 523, 81–92. doi: 10.3354/meps11196
Gardères, J., Bedoux, G., Koutsouveli, V., Crequer, S., Desriac, F., and Le Pennec, G. (2015). Lipopolysaccharides from commensal and opportunistic bacteria: characterization and response of the immune system of the host sponge Suberites domuncula. Mar. Drugs 13, 4985–5006. doi: 10.3390/md13084985
Glasl, B., Smith, C. E., Bourne, D. G., and Webster, N. S. (2018). Exploring the diversity-stability paradigm using sponge microbial communities. Sci. Rep. 8:8425. doi: 10.1038/s41598-018-26641-9
Glasl, B., Webster, N. S., and Bourne, D. G. (2017). Microbial indicators as a diagnostic tool for assessing water quality and climate stress in coral reef ecosystems. Mar. Biol. 164, 1–18.
Grottoli, A. G., Wilkins, M. J., Johnston, M. D., Levas, S., Schoepf, V., Dalcin, M. P., et al. (2018). Coral physiology and microbiome dynamics under combined warming and ocean acidification. PLoS One 13:e0191156. doi: 10.1371/journal.pone.0191156
Haber, M., and Ilan, M. (2014). Diversity and antibacterial activity of bacteria cultured from Mediterranean Axinella spp. sponges. J. Appl. Microbiol. 116, 519–532. doi: 10.1111/jam.12401
Hentschel, U., Hopke, J., Horn, M., Anja, B., Wagner, M., Hacker, J., et al. (2002). Molecular evidence for a uniform microbial community in sponges from different oceans. Appl. Environ. Microbiol. 68, 4431–4440. doi: 10.1128/aem.68.9.4431-4440.2002
Hentschel, U., Piel, J., Degnan, S. M., and Taylor, M. W. (2012). Genomic insights into the marine sponge microbiome. Nat. Rev. Microbiol. 10, 641–654. doi: 10.1038/nrmicro2839
Hooper, J. N. A., and Van Soest, R. W. M. (2002). “Order poecilosclerida topsent, 1928,” in Systema Porifera: A Guide to the Classification of Sponges, eds J. N. A. Hooper, R. W. M. Van Soest, and P. Willenz (Boston, MA: Springer), 403–408. doi: 10.1007/978-1-4615-0747-5_49
Hsieh, T. C., Ma, K. H., Chao, A., and Hsieh, M. T. C. (2018). Interpolation and Extrapolation for Species Diversity Version 2.0.19.
Klindworth, A., Pruesse, E., Schweer, T., Peplies, J., Quast, C., Horn, M., et al. (2013). Evaluation of general 16S ribosomal RNA gene PCR primers for classical and next-generation sequencing-based diversity studies. Nucleic Acids Res. 41, 1–11. doi: 10.1093/nar/gks808
Larson, J., Jonathan, A., Godfry, R., Kelley, T., Eberly, D. H., Gustafsson, P., et al. (2018). Area-Proportional Euler and Venn Diagrams with Circles or Ellipses. R Packag. version 4.1.0.
Latypov, Y. (2006). Changes in the composition and structure of coral communities of Mju and Moon islands, Nha Trang Bay, South China Sea. Russ. J. Mar. Biol. 32, 269–275. doi: 10.1134/s1063074006050014
Latypov, Y. (2011). Scleractinian corals and reefs of vietnam as a part of the pacific reef ecosystem. Open J. Mar. Sci. 01, 50–68. doi: 10.4236/ojms.2011.12006
Latypov, Y. (2015). The spatial-temporal variability and stability of Vietnamese reef communities. Russ. J. Mar. Biol. 41, 103–110. doi: 10.1134/s1063074015020066
Lesser, M. P., Fiore, C., Slattery, M., and Zaneveld, J. (2016). Climate change stressors destabilize the microbiome of the Caribbean barrel sponge, Xestospongia muta. J. Exp. Mar. Bio. Ecol. 475, 11–18. doi: 10.1016/j.jembe.2015.11.004
Leys, S. P., Yahel, G., Reidenbach, M. A., Tunnicliffe, V., Shavit, U., and Reiswig, H. M. (2011). The sponge pump: the role of current induced flow in the design of the sponge body plan. PLoS One 6:e27787. doi: 10.1371/journal.pone.0027787
Loredana, S., Graziano, P., Antonio, M., Carlotta, N. M., Caterina, L., Maria, A. A., et al. (2017). Lindane bioremediation capability of bacteria associated with the demosponge Hymeniacidon perlevis. Mar. Drugs 15, 1–15. doi: 10.3390/md15040108
Loya, Y. (1978). “Plotless and transect methods,” in Coral Reefs: Research Methods, eds D. R. Stoddart and R. E. Johannes (Paris: Unesco), 197–217.
Luter, H. M., Gibb, K., and Webster, N. S. (2014). Eutrophication has no short-term effect on the Cymbastela stipitata holobiont. Front. Microbiol. 5:216. doi: 10.3389/fmicb.2014.00216
Luter, H. M., Whalan, S., and Webster, N. S. (2012). Thermal and sedimentation stress are unlikely causes of brown spot syndrome in the Coral Reef sponge, Ianthella basta. PLoS One 7:e39779. doi: 10.1371/journal.pone.0039779
Maliao, R. J., Turingan, R. G., and Lin, J. (2008). Phase-shift in coral reef communities in the Florida Keys National Marine Sanctuary (FKNMS), USA. Mar. Biol. 154, 841–853. doi: 10.1007/s00227-008-0977-0
Matcher, G. F., Waterworth, S. C., Walmsley, T. A., Matsatsa, T., Parker-Nance, S., Davies-Coleman, M. T., et al. (2017). Keeping it in the family: coevolution of latrunculid sponges and their dominant bacterial symbionts. Microbiologyopen 6, 1–13. doi: 10.1002/mbo3.417
Milanese, M., Chelossi, E., Manconi, R., Sarà, A., Sidri, M., and Pronzato, R. (2003). The marine sponge Chondrilla nucula schmidt, 1862 as an elective candidate for bioremediation in integrated aquaculture. Biomol. Eng. 20, 363–368. doi: 10.1016/s1389-0344(03)00052-2
Mohamed, N. M., Rao, V., Hamann, M. T., Kelly, M., and Hill, R. T. (2008). Monitoring bacterial diversity of the marine sponge Ircinia strobilina upon transfer into aquaculture. Appl. Environ. Microbiol. 74, 4133–4143. doi: 10.1128/AEM.00454-08
Morganti, T., Coma, R., Yahel, G., and Ribes, M. (2017). Trophic niche separation that facilitates co-existence of high and low microbial abundance sponges is revealed by in situ study of carbon and nitrogen fluxes. Limnol. Oceanogr. 62, 1963–1983. doi: 10.1002/lno.10546
Morrow, K. M., Bourne, D. G., Humphrey, C., Botté, E. S., Laffy, P., Zaneveld, J., et al. (2015). Natural volcanic CO2 seeps reveal future trajectories for host-microbial associations in corals and sponges. ISME J. 9, 894–908. doi: 10.1038/ismej.2014.188
Neave, M. J., Apprill, A., Ferrier-Pagès, C., and Voolstra, C. R. (2016). Diversity and function of prevalent symbiotic marine bacteria in the genus Endozoicomonas. Appl. Microbiol. Biotechnol. 100, 8315–8324. doi: 10.1007/s00253-016-7777-0
Nguyen, H. N. K., Van, T. T. H., and Coloe, P. J. (2016). Antibiotic resistance associated with aquaculture in Vietnam. Microbiol. Aust. 37, 108–111.
Nishijima, M., Adachi, K., Katsuta, A., Shizuri, Y., and Yamasato, K. (2013). Endozoicomonas numazuensis sp. nov., a gammaproteobacterium isolated from marine sponges, and emended description of the genus Endozoicomonas Kurahashi and Yokota 2007. Int. J. Syst. Evol. Microbiol. 63, 709–714. doi: 10.1099/ijs.0.042077-0
Oksanen, J., Blanchet, F. G., Friendly, M., Kindt, R., Legendre, P., McGlinn, D., et al. (2017). vegan: Community Ecology Package. R Packag. version 2.5–1.
Ortiz-Álvarez, R., Fierer, N., De Los Ríos, A., Casamayor, E. O., and Barberán, A. (2018). Consistent changes in the taxonomic structure and functional attributes of bacterial communities during primary succession. ISME J. 12, 1658–1667. doi: 10.1038/s41396-018-0076-2
Pineda, M. C., Strehlow, B., Sternel, M., Duckworth, A., Haan, J. D., Jones, R., et al. (2017). Effects of sediment smothering on the sponge holobiont with implications for dredging management. Sci. Rep. 7:5156. doi: 10.1038/s41598-017-05243-x
Piola, R. F., and Johnston, E. L. (2008). Pollution reduces native diversity and increases invader dominance in marine hard-substrate communities. Divers. Distrib. 14, 329–342. doi: 10.1111/j.1472-4642.2007.00430.x
Pita, L., Erwin, P. M., Turon, X., and López-Legentil, S. (2013a). Till death do us part: stable sponge-bacteria associations under thermal and food shortage stresses. PLoS One 8:e80307. doi: 10.1371/journal.pone.0080307
Pita, L., Turon, X., López-Legentil, S., and Erwin, P. M. (2013b). Host rules: spatial stability of bacterial communities associated with marine sponges (Ircinia spp.) in the western mediterranean sea. FEMS Microbiol. Ecol. 86, 268–276. doi: 10.1111/1574-6941.12159
Pita, L., Rix, L., Slaby, B. M., Franke, A., and Hentschel, U. (2018). The sponge holobiont in a changing ocean: from microbes to ecosystems. Microbiome 6:46. doi: 10.1186/s40168-018-0428-1
Pogoreutz, C., Rädecker, N., Cárdenas, A., Gärdes, A., Wild, C., and Voolstra, C. R. (2018). Dominance of Endozoicomonas bacteria throughout coral bleaching and mortality suggests structural inflexibility of the Pocillopora verrucosa microbiome. Ecol. Evol. 8, 2240–2252. doi: 10.1002/ece3.3830
Powell, A., Smith, D. J., Hepburn, L. J., Jones, T., Berman, J., Jompa, J., et al. (2014). Reduced diversity and high sponge abundance on a sedimented indo-pacific reef system: implications for future changes in environmental quality. PLoS One 9:e85253. doi: 10.1371/journal.pone.0085253
Prosser, J. I., Head, I. M., and Stein, L. Y. (2014). “The family Nitrosomonadaceae,” in The Prokaryotes: Alphaproteobacteria and Betaproteobacteria, eds E. Rosenberg, E. F. DeLong, S. Lory, E. Stackebrandt, and F. Thompson (Berlin: Springer), 901–918. doi: 10.1007/978-3-642-30197-1_372
Pruesse, E., Peplies, J., and Glöckner, F. O. (2012). SINA: accurate high-throughput multiple sequence alignment of ribosomal RNA genes. Bioinformatics 28, 1823–1829. doi: 10.1093/bioinformatics/bts252
R Core Team (2013). R: A Language and Environment for Statistical Computing. Vienna: R Foundation on Statistical Computing.
Ralph, P. J., Tomasko, D., Moore, K., Seddon, S., and Macinnis-Ng, C. M. O. (2006). “Human impacts on seagrasses: eutrophication, sedimentation, and contamination,” in Seagrasses: Biology, Ecology and Conservation, eds A. W. D. Larkum, R. J. Orth, and C. M. Duarte (Dordrecht: Springer), 567–593. doi: 10.1007/1-4020-2983-7_24
Ramsby, B. D., Hoogenboom, M. O., Whalan, S., and Webster, N. S. (2018). Elevated seawater temperature disrupts the microbiome of an ecologically important bioeroding sponge. Mol. Ecol. 27, 2124–2137. doi: 10.1111/mec.14544
Ribes, M., Calvo, E., Movilla, J., Logares, R., Coma, R., and Pelejero, C. (2016). Restructuring of the sponge microbiome favors tolerance to ocean acidification. Environ. Microbiol. Rep. 8, 536–544. doi: 10.1111/1758-2229.12430
Richter, C., Wunsch, M., Rasheed, M., Kötter, I., and Badran, M. I. (2001). Endoscopic exploration of Red Sea coral reefs reveals dense populations of cavity-dwelling sponges. Nature 413, 726–730. doi: 10.1038/35099547
Roberts, A. D. W., and Roberts, M. D. W. (2016). labdsv: Ordination and Multivariate Analysis for Ecology. R Packag. version 1.8–0.
Rosenberg, E., and Zilber-Rosenberg, I. (2018). The hologenome concept of evolution after 10 years. Microbiome 6:78. doi: 10.1186/s40168-018-0457-9
Rua, C. P. J., Trindade-Silva, A. E., Appolinario, L. R., Venas, T. M., Garcia, G. D., Carvalho, L. S., et al. (2014). Diversity and antimicrobial potential of culturable heterotrophic bacteria associated with the endemic marine sponge Arenosclera brasiliensis. PeerJ 2:e419. doi: 10.7717/peerj.419
Rygg, B. (1985). Distribution of species along pollution-induced diversity gradients in benthic communities in Norwegian fjords. Mar. Pollut. Bull. 16, 469–474. doi: 10.1016/0025-326x(85)90378-9
Sawall, Y., Al-Sofyani, A., Banguera-Hinestroza, E., and Voolstra, C. R. (2014). Spatio-temporal analyses of symbiodinium physiology of the coral Pocillopora verrucosa along large-scale nutrient and temperature gradients in the Red Sea. PLoS One 9:e103179. doi: 10.1371/journal.pone.0103179
Schmidt, E. W., Obraztsova, A. Y., Davidson, S. K., Faulkner, D. J., and Haygood, M. (2000). Identification of the antifungal peptide- containing symbiont of the marine sponge Theonella swinhoei as a novel d-proteobacterium, “Candidatus Entotheonella palauensis”. Mar. Biol. 136, 969–977. doi: 10.1007/s002270000273
Schönberg, C. H. L. (2015). Self-cleaning surfaces in sponges. Mar. Biodivers. 45, 623–624. doi: 10.1007/s12526-014-0302-8
Schönberg, C. H. L. (2016). Happy relationships between marine sponges and sediments-a review and some observations from Australia. J. Mar. Biol. Assoc. United Kingdom 96, 493–514. doi: 10.1017/s0025315415001411
Simister, R., Taylor, M. W., Tsai, P., Fan, L., Bruxner, T. J., Crowe, M. L., et al. (2012). Thermal stress responses in the bacterial biosphere of the great barrier reef sponge, rhopaloeides odorabile. Environ. Microbiol. 14, 3232–3246. doi: 10.1111/1462-2920.12010
Simon, M., Scheuner, C., Meier-Kolthoff, J. P., Brinkhoff, T., Wagner-Döbler, I., Ulbrich, M., et al. (2017). Phylogenomics of Rhodobacteraceae reveals evolutionary adaptation to marine and non-marine habitats. ISME J. 11, 1483–1499. doi: 10.1038/ismej.2016.198
Sirová, D., Karel, Š, Posch, T., Stone, J., Borovec, J., Adamec, L., et al. (2018). Hunters or farmers? Microbiome characteristics help elucidate the diet composition in an aquatic carnivorous plant. Microbiome 6:225. doi: 10.1186/s40168-018-0600-7
Strand, R., Whalan, S., Webster, N. S., Kutti, T., Fang, J. K. H., Luter, H. M., et al. (2017). The response of a boreal deep-sea sponge holobiont to acute thermal stress. Sci. Rep. 7:1660. doi: 10.1038/s41598-017-01091-x
Strehlow, B. W., Pineda, M., Duckworth, A., Kendrick, G. A., Renton, M., Azmi, M., et al. (2017). Sediment tolerance mechanisms identified in sponges using advanced imaging techniques. PeerJ 5, 1–26. doi: 10.7717/peerj.3904
Thomas, T., Moitinho-Silva, L., Lurgi, M., Björk, J. R., Easson, C., Astudillo-García, C., et al. (2016). Diversity, structure and convergent evolution of the global sponge microbiome. Nat. Commun. 7, 1–12.
Tkachenko, K. S., Britayev, T. A., Huan, N. H., Pereladov, M. V., and Latypov, Y. Y. (2016). Influence of anthropogenic pressure and seasonal upwelling on coral reefs in Nha Trang Bay (Central Vietnam). Mar. Ecol. 37, 1131–1146. doi: 10.1111/maec.12382
Turon, M., Cáliz, J., Garate, L., Casamayor, E. O., and Uriz, M. J. (2018). Showcasing the role of seawater in bacteria recruitment and microbiome stability in sponges. Sci. Rep. 8:15201. doi: 10.1038/s41598-018-33545-1
Uriz, M. J., Agell, G., Blanquer, A., Turon, X., and Casamayor, O. (2012). Endosymbiotic calcifying bacteria: a new cue to the origin of calcification in Metazoa? Evolution 66, 2993–2999. doi: 10.1111/j.1558-5646.2012.01676.x
Van Soest, R. W. M., Boury-Esnault, N., Vacelet, J., Dohrmann, M., Erpenbeck, D., de Voogd, N. J., et al. (2012). Global diversity of sponges (Porifera). PLoS One 7:e35105. doi: 10.1371/journal.pone.0035105
Webster, N. S., Negri, A. P., Botté, E. S., Laffy, P. W., Flores, F., Noonan, S., et al. (2016). Host-associated coral reef microbes respond to the cumulative pressures of ocean warming and ocean acidification. Sci. Rep. 6:19324. doi: 10.1038/srep19324
Webster, N. S., Xavier, J. R., Freckelton, M., Motti, C. A., and Cobb, R. (2008). Shifts in microbial and chemical patterns within the marine sponge Aplysina aerophoba during a disease outbreak. Environ. Microbiol. 10, 3366–3376. doi: 10.1111/j.1462-2920.2008.01734.x
Weigel, B. L., and Erwin, P. M. (2017). Effects of reciprocal transplantation on the microbiome and putative nitrogen cycling functions of the intertidal sponge, Hymeniacidon heliophila. Sci. Rep. 7:43247. doi: 10.1038/srep43247
Weisz, J. B., Hentschel, U., Lindquist, N., and Martens, C. S. (2007). Linking abundance and diversity of sponge-associated microbial communities to metabolic differences in host sponges. Mar. Biol. 152, 475–483. doi: 10.1007/s00227-007-0708-y
Wilkinson, C. R. (1984). Immunological evidence for the precambrian origin of bacterial symbioses in marine sponges. Proc. R. Soc. Lond. Ser. B Biol. Sci. 220, 509–518. doi: 10.1111/j.1558-5646.2012.01676.x
Zaneveld, J. R., McMinds, R., and Thurber, R. V. (2017). Stress and stability: applying the Anna Karenina principle to animal microbiomes. Nat. Microbiol. 2:17121. doi: 10.1038/nmicrobiol.2017.121
Zeglin, L. H. (2015). Stream microbial diversity in response to environmental changes: review and synthesis of existing research. Front. Microbiol. 6:454. doi: 10.3389/fmicb.2015.00454
Keywords: ecology, sponges, microbiomes, diversity, resilience, contrasting environments, eutrophication
Citation: Turon M, Cáliz J, Triadó-Margarit X, Casamayor EO and Uriz MJ (2019) Sponges and Their Microbiomes Show Similar Community Metrics Across Impacted and Well-Preserved Reefs. Front. Microbiol. 10:1961. doi: 10.3389/fmicb.2019.01961
Received: 30 April 2019; Accepted: 09 August 2019;
Published: 22 August 2019.
Edited by:
Russell T. Hill, University of Maryland, Baltimore County, United StatesReviewed by:
Cole G. Easson, Middle Tennessee State University, United StatesHeidi M. Luter, Australian Institute of Marine Science (AIMS), Australia
Copyright © 2019 Turon, Cáliz, Triadó-Margarit, Casamayor and Uriz. This is an open-access article distributed under the terms of the Creative Commons Attribution License (CC BY). The use, distribution or reproduction in other forums is permitted, provided the original author(s) and the copyright owner(s) are credited and that the original publication in this journal is cited, in accordance with accepted academic practice. No use, distribution or reproduction is permitted which does not comply with these terms.
*Correspondence: Maria J. Uriz, aW9zdW5lQGNlYWIuY3NpYy5lcw==