- 1Programa de Pós-Graduação em Patologia Ambiental e Experimental, Universidade Paulista (UNIP), São Paulo, Brazil
- 2Departamento de Fisiopatologia, Instituto Butantan, São Paulo, Brazil
Microsporidia, including Encephalitozoon intestinalis, are emerging pathogens which cause opportunistic infections in immunocompromised patients, such as those with AIDS, cancer, the elderly and people on immunosuppressive drugs. Intestinal mucosa (IM) is crucial for developing an efficient adaptive immune response against pathogenic micro-organisms, thereby preventing their colonization and subsequent infection. As immunosuppressive drugs affect the intestinal immune response is little known. In the present study, we investigated the immune response to E. intestinalis infection in the IM and gut-associated lymphoid tissue (GALT) in cyclophosphamide (Cy) immunosuppressed mice, to mimic an immunocompromised condition. Histopathology revealed lymphoplasmacytic enteritis at 7 and 14 days-post-infection (dpi) in all infected groups, however, inflammation diminished at 21 and 28 dpi. Cy treatment also led to a higher number of E. intestinalis spores and lesions, which reduced at 28 dpi. In addition, flow cytometry analysis demonstrated CD4+ and CD8+ T cells to be predominant immune cells, with up-regulation in both Th1 and Th2 cytokines at 7 and 14 dpi, as demonstrated by histopathology. In conclusion, Cy treatment reduced GALT (Peyer’s plaques and mesenteric lymph nodes) and peritoneum populations but increased the T-cell population in the intestinal mucosa and the production of pro-and anti-inflammatory cytokines, which were able to eliminate this opportunistic fungus and reduced the E. intestinalis infection.
Introduction
Microsporidia are spore-forming intracellular pathogens, responsible for causing opportunistic infections in immunocompromised people, such as those with HIV infection and AIDS, cancer patients or individuals with autoimmune diseases taking immunosuppressive drugs, and in elderly and children (Didier and Weiss, 2011; Hinney et al., 2016; Bednarska et al., 2018; Wang et al., 2018; Ghoyounchi et al., 2019). Seroprevalence studies confirm that microsporidia spores easily infect humans, both immunocompetent and immunocompromised, and cause asymptomatic infection. Two species are responsible for gastrointestinal infections: Enterocytozoon bieneusi and Encephalitozoon intestinalis (Field and Milner, 2015.). Both microsporidia are transmitted by the oral route and cause abdominal cramping, diarrhea, malabsorption, and weight loss in patients with AIDS (Han and Weiss, 2017). E. intestinalis also infects and develops inside intestinal macrophages, allowing the infection to spread from the intestine to other organs (Didier, 2005). A very limited number of drugs are available for treating intestinal microsporidiosis; these include albendazole and fumagillin, which are at least partially effective in reducing the parasite count (Didier, 2005; Didier and Weiss, 2011). A strong immune response of an individual is mainly responsible for controlling the pathogen. The compartmentalized response against E. intestinalis infection is primarily mediated by CD8+ and CD4+ T cells together with interferon (IFN) and interleukin (IL)-12 cytokines (Salát et al., 2004, 2006).
The past decade has witnessed a surge in the use of immunosuppressive drugs for the treatment of neoplastic and autoimmune disease patients, as well as in patients undergoing transplantation. Cyclophosphamide (Cy) is one such immunosuppressive drug that has been recommended by the World Health Organization (WHO) and is on the list of drugs most frequently used by the Public and Private Health Systems owing to its efficacy, cost-effectiveness and lesser side-effects (World Health Organization, 2013). Cy is primarily used against autoimmune and alloimmune diseases (Brodsky, 2010; Dezern et al., 2013), in treating patients undergoing transplantation, such as bone marrow recipients (Colvin, 1999) and in cancer treatment (Emadi et al., 2009; Sistigu et al., 2011)
Cyclophosphamide is a cytotoxic alkylating agent that binds to DNA; its major effects on the body include cellular apoptosis and myelosuppression, and decreased lymphocyte, neutrophil, red blood cell, and platelet count. However, it also possesses immunomodulatory effects, which have not yet been clarified, such as (i) expansion of antigen-specific T cells, (ii) expansion of T cell-specific cytokines (IFNs, IL-7, and IL-15), (iii) decrease in regulatory T cells (Treg), and (iv) increased mobilization of dendritic cells from bone marrow, with activation of intracellular machinery for antigen processing and presentation (Sevko et al., 2013). Thus, this anticancer agent is known for inducing immunogenic cancer cell death, subverting immunosuppressive T cells, and promoting Th1 and Th17 cells that control cancer outgrowth (Schiavoni et al., 2011; Viaud et al., 2011). We have shown previously Cy to suppress the immune system of mice; intraperitoneal infection of mice with E. cuniculi resulted in a disseminated, acute, and fatal encephalitozoonosis. These results were associated with the immunosuppressive effects of Cy (Lallo and Hirschfeld, 2012).
The intestinal mucosa (IM) acts as a host to a variable microflora, which plays an important role in nutrition absorption and immune function, among others. The immune response of the IM plays a critical role in maintaining the commensal homeostasis and protecting the host against pathogens (Mangan and Fung, 2012; Allaire et al., 2018). The immune response of IM against E. cuniculi infection is majorly mediated by antigen-specific intraepithelial lymphocytes (IELs) (Moretto et al., 2012). Considering the entry point of microsporidia to be IM, there is a lack of knowledge of local immunity in individuals on immunosuppressive conditions.
This study was designed to describe how the immunosuppressive effects of cyclophosphamide compromise the intestinal immune response against E. intestinalis, one of the most prevalent microsporidia in opportunistic infections in humans. Herein, we show a higher number of CD8+ and CD4+ T lymphocytes in IM in association with pro-inflammatory cytokines to be responsible for resolution of E. intestinalis infection in both Cy immunosuppressed and immunocompetent mice, despite the immunosuppressive activity of Cy observed in cells populations of Peyer’s plaques, mesenteric lymph nodes, peritoneum and even part of the immune population of the IM.
Materials and Methods
Animals
Specific pathogen-free, 6–8-week-old C57BL/6 mice were purchased from the Federal University of São Paulo (CEDEME, UNIFESP), Brazil. Animals were housed under sterile conditions at the Animal Facility of Paulista University, São Paulo, Brazil, and given food and water ad libitum. All animal procedures were performed in strict accordance with the Paulista University Ethics Committee (CEUA - protocol number 313/15).
Encephalitozoon intestinalis Cultivation and Experimental Infection
Spores of E. intestinalis were purchased from Waterborne Inc., New Orleans, LA, United States. These were cultivated in rabbit kidney cells (RK–13) in Dulbecco’s Modified Eagle’s medium (DMEM) supplemented with 10% fetal bovine serum (FBS), pyruvate, non-essential amino acids, and gentamicin followed by incubation at 37°C and 5% CO2. Spores were collected from the supernatant, washed thrice in phosphate-buffered saline (PBS), and counted using a Neubauer chamber.
Experimental Design
Mice were divided into four experimental groups: infected, mice infected with E. intestinalis; uninfected, non-infected and non-treated mice; Cy-infected, mice treated with cyclophosphamide and infected with E. intestinalis; and Cy-uninfected, mice treated with cyclophosphamide. The Cy-treatment protocol was previously established (Lallo and Hirschfeld, 2012) and consisted of intraperitoneal injection of 100 mg/kg twice a week (Genuxal; Asta Medica Oncologia, São Paulo, Brazil). The treatment started at the day of infection until 28 days post infection (dpi). Mice were orally infected by gavage with 5 × 107 E. intestinalis spores. Non-infected mice served as control.
Necropsy and Tissue Sampling
At 7, 14, 21, and 28 dpi, five animals from each group were euthanized with a mixture of ketamine (100 mg/mL), xylazine (20 mg/mL), and fentanyl (0.05 mg/mL). The samples of the intestine (duodenum, ileum), liver, kidneys, and lungs were collected and fixed in 10% buffered formalin for 72 h, routinely processed for histopathology, and stained with hematoxylin-eosin (HE) and Giemsa.
Ultrastructural Analysis by Transmission Electronic Microscopy
Ileum samples of 1 mm thickness from Cy-infected mice were fixed in 2% glutaraldehyde in 0.2 M cacodylate buffer (pH 7.2) at 4°C for 10 h. These were then fixed in buffered 1% OsO4 for 3 h. Subsequently, the samples were embedded in EPON resin, sliced into semi-thin cuts, and stained with toluidine blue. Then, ultrathin sections were double stained using uranyl and lead citrate and observed under the TEM LEO EM 906 at 80 kV at the Butantan Institute.
Fungal Burden
The paraffinized ileum was cut into 5 μm thick sections and evaluated histopathologically using HE staining to determine the fungal number. Fungal spores were counted randomly in at least 10 fields under the light microscope (40× objective magnification). The average number of spores from each mouse was recorded and statistically analyzed.
Phenotypic Analysis of Immune Intestinal Mucosal Cells
Cells of the IM were obtained as previously described by Guy-Grand et al. (1978) with minor modifications. The small intestine was washed with 50 mL of Hanks’ balanced salt solution (HBSS)–2% FBS solution, longitudinally cut and separated into 2 cm segments. The mucosa was grated and submerged in HBSS–2% FBS solution supplemented with 0.1 M ethylenediaminetetraacetic acid (EDTA) at 37°C for 20 min. Then, samples were vortexed for 15 s and filtered using a cell strainer to remove the cell debris. Percoll gradient (70% and 40%) centrifugation was used to isolate the IM cells. After centrifugation, cells were washed with HBSS–2% FBS and resuspended in 100 μL of PBS–1% bovine serum albumin (BSA).
Mesenteric lymph nodes (MLN) and Peyer’s patches (PP) were isolated from the intestines using a scalped blade and washed in a cell strainer with HBSS–2% FBS. In addition, cells from the peritoneal cavity (PerC) were obtained by successive washes with at least 10 mL of HBSS–2% FBS. Finally, cell suspensions from PerC, MLN, and IM were washed with HBSS–2% FBS and resuspended in 100 μL of PBS–1%BSA. After centrifugation at 500 × g for 5 min, each sample was incubated at 4°C for 20 min with the anti-CD16/CD32 antibody. After incubation, cells were divided into two aliquots and resuspended in PBS–1%BSA, followed by incubation with monoclonal antibodies: APC-conjugated anti-mouse CD19, FITC, or PE-conjugated anti-mouse CD23, PerCP-conjugated anti-mouse CD4, FITC-conjugated anti-mouse CD8, APC-Cy7-conjugated anti-mouse CD11b, and PE-conjugated anti-mice CD11c (BD Pharmingen; San Diego, CA, United States). Finally, cell suspensions were run on the flow cytometer FACS Canto II (BD Biosciences; Mountain View, CA, United States). The cells were characterized according to their phenotypes in CD8 T (CD19– CD4–CD8+), CD4 T (CD19– CD8– CD4+), NKT/NK cells (CD19– CD4+ NK1.1+), B-1 (CD23– CD19+), B-2 cells (CD23+ CD19+) and dendritic cells (CD11c+); and analyzed with the software FlowJo (FlowJo LLC; data analysis software, Ashland, OR, United States).
Cytokine Quantification
For quantification of intestinal cytokines, 100 mg of ileum was sampled and treated with 1 mL of protease inhibitor (Sigma-Aldrich; St. Louis, MO, United States) at −80°C. The sample was thawed and processed using a Precellys homogenizer for three cycles, 20 s each, filtering the homogenate using a cell strainer to remove the debris. Cytokines (IL-2, IL-4, IL-6, IL-10, IL-17, IFN-γ, and TNF-α) in the homogenate were measured using the CBA Mouse Th1/Th2/Th17 cytokine kit (BD Biosciences; CA, United States) according to manufacturer’s instructions. The kit consists of fluorescent beads coated with antibodies specific to cytokines. Briefly, 25 μL of each sample was added to capture beads and PE-labeled secondary antibodies. The samples were incubated for 2 h at room temperature in the dark, following which two-color flow cytometry analysis was performed on the FACS Canto II flow cytometer (BD Biosciences; Mountain View, CA, United States) and analyzed using the FCAP Array 1.0 software (BD Biosciences; CA, United States).
Statistical Analysis
Comparisons were made by One Way ANOVA followed by Tukey’s or Bonferroni’s test. All values are expressed as mean ± standard error of mean (SEM) with significance of α = 0.05 (p < 0.05). All graphs were generated using the “GraphPad Prism” version 5.0 for Windows (GraphPad Software Inc; La Jolla, CA, United States).
Results
Immunosuppressed Mice Showed Transitory Enteritis With Fast Resolution
All infected mice survived the oral infection by E. intestinalis, with no evidence of symptoms and macroscopic lesions in the gut. Microscopically, lymphoplasmacytic enteritis was observed in infected animals (infected and Cy-infected), mostly affecting the duodenum and ileum, especially at 7 and 14 dpi (Figures 1a,b). In addition to the inflammatory infiltrate, mucosal ulceration caused exposure of the underlying lamina propria (Figure 1b), apical and mural multifocal necrosis, and villus-based epithelial proliferation. At 21 and 28 dpi, young cells and an increased number of mitoses were observed in the small intestine, suggesting a restoration of tissue integrity, via resolution of the inflammatory process and tissue remodeling. E. intestinalis spore clusters were observed on the glandular base (Figure 1c), with a higher fungal burden observed in the Cy-infected than the infected (Figure 1d) group. However, in both groups, the fungal burden and the lesions progressively decreased from 7 to 28 dpi (Figure 1d). No E. intestinalis spores were observed in uninfected mice. Thus, infection by E. intestinalis via oral route caused lymphoplasmacytic enteritis, accompanied by favorable proliferation in both immunosuppressed and non-immunosuppressed groups, however, the fungal burden was higher in the Cy-treated mice. Macroscopically, the infection led to the enlargement of the spleen and intestinal lymph nodes in the infected animals.
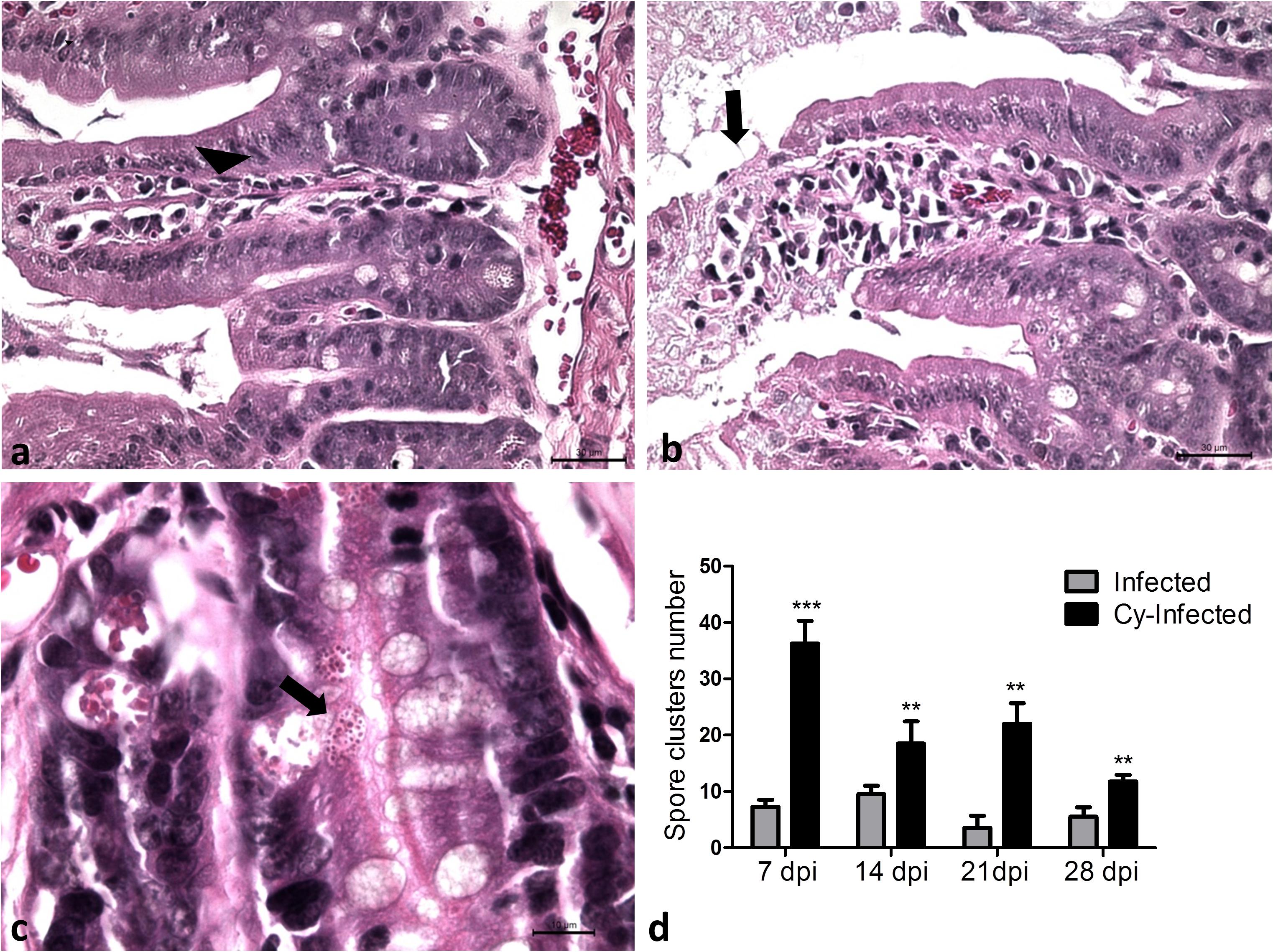
Figure 1. (a) Photomicrograph of lymphoplasmacytic enteritis in the small intestine (arrowhead) in Infected mice. (b) Ulceration (arrow) of intestinal mucosa of Cy-Infected mice. (c) Clusters of E. intestinalis (arrow) at the glandular region in the mucosa of the ileum of Cy-Infected mice. (d) Spores counting in the Infected and Cy-Infected mice. HE staining. ANOVA test with Tukey’s posttest showed ∗∗p < 0.01 and ∗∗∗p < 0.001.
CD8+ T Lymphocytes Increased in Intestinal Mucosa in Infected Mice
The infected and Cy-infected groups showed a significant increase in CD8+ T lymphocyte population as compared to controls at 7, 14, and 28 dpi (Figure 2A). This is in line with a previous study that demonstrated CD4+ T and CD8+ T lymphocyte subpopulations to play a substantive role in protecting against peroral infection of E. intestinalis (Salát et al., 2004). Another study reported a significant increase in the CD8αα subset of IELs in response to oral E. cuniculi infection (Moretto et al., 2004).
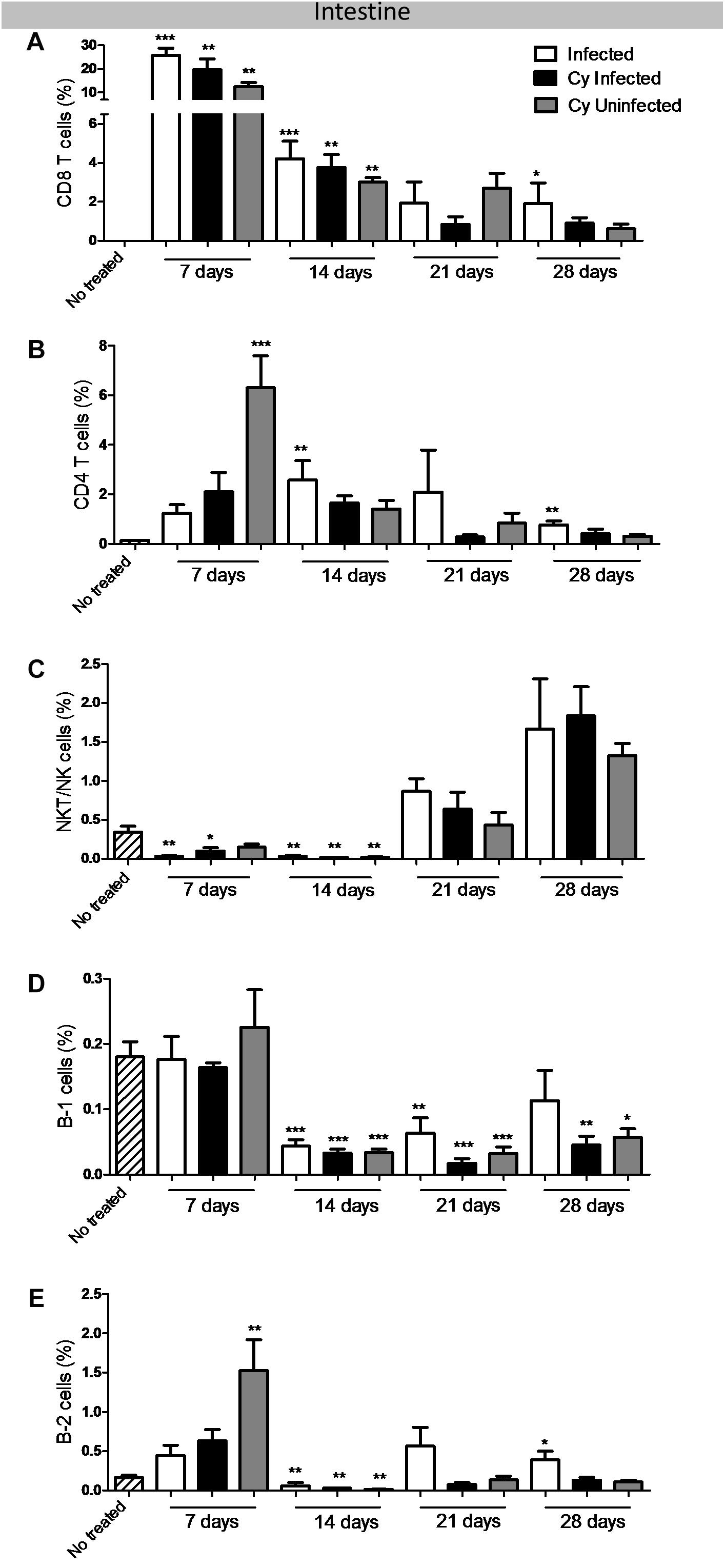
Figure 2. Evaluation of T and B cell population in the intestinal mucosa of the mice infected with E. intestinalis and treated or not with Cy at 7, 14, 21 and 28 dpi. Percentage of (A) CD8 T lymphocytes (CD19– CD4–CD8+); (B) CD4 T lymphocytes (CD19– CD8– CD4+); (C) NKT/NK cells (CD19– CD4+ NK1.1+); (D) B-1 cells (CD23– CD19+), and (E) B-2 cells (CD23+ CD19+). ANOVA test with Tukey’s post-test showed ∗p < 0.05, ∗∗p < 0.01, and ∗∗∗p < 0.001 compared to non-infected controls.
Immunosuppressive therapy with Cy could not cause a significant difference in CD8+ T lymphocyte population among the infected mice. Moreover, CD8+ T lymphocyte population witnessed a 10-time decrease, when compared 7 with 28 dpi (Supplementary Figure S1). Together, the results showed that CD8+ T lymphocyte peak was observed at 7 dpi and was associated with a high number of E. intestinalis spores and lymphoplasmacytic enteritis. At the same time, there was a gradual reduction in the CD8+ T cell population, fungal burden, and histological lesions in infected (infected and Cy-infected) animals at 14, 21, and 28 dpi, indicating the resolution of infection in both immunosuppressed and non-immunosuppressed groups. The treatment with Cy resulted in a significant increase in the CD4+ T lymphocyte population in the uninfected group. In addition, infected and Cy-infected groups also showed an increase in this population at 14 and 28 dpi as compared to the untreated control (Figure 2B), suggesting that E. intestinalis stimulated CD4+ T expansion.
Further, the NKT/NK cells decreased significantly in the infected, Cy-infected, and Cy-uninfected groups as compared to the uninfected control at 7 and 14 dpi (Figure 2C). However, these cells increased in the infected and Cy-infected groups in the later stages of infection (Supplementary Figure S1).
A significant reduction in B-1 cells was reported in the infected, Cy-infected, and Cy-uninfected groups as compared to the uninfected control at 14, 21, and 28 dpi (Figure 2D). Moreover, B-2 cells decreased in the infected and Cy-infected groups as compared to the uninfected control at 14 dpi but increased significantly in the infected group at 28 dpi (Figure 2E). Another notable observation was a decrease in the dendritic cells in PP in the infected and Cy-infected groups (Figure 3A). PP in the infected group was more evident, with a marked increase in the germinal center activity, thereby expanding the lymphoid center (Figure 3B). Moreover, PP from Cy-infected mice showed a rarified lymphoid tissue (Figure 3B), enlarged lymphatics, and clusters of E. intestinalis spores (data not shown).
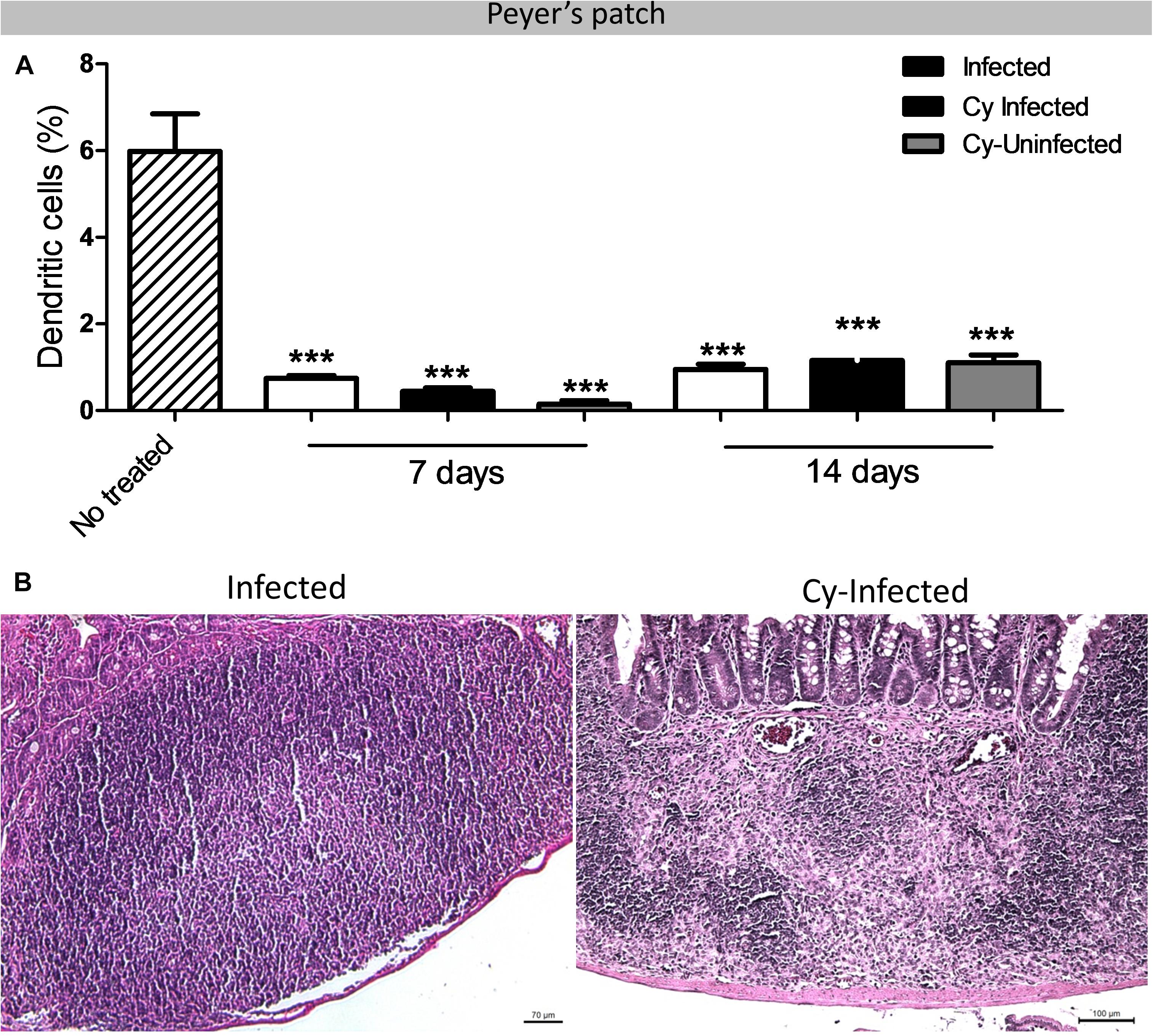
Figure 3. Peyer’s patch analyzed of the mice infected with E. intestinalis and treated or not with Cy for 7, 14, 21 and 28 dpi. (A) Percentage of dendritic cells (CD11c+) in Peyer’s Patch. (B) Photomicrograph of Peyer’s Patch with lymphoid expansion in Infected group or rarified lymphoid tissue in Cy-Infected group. HE staining. ANOVA test with Tukey’s post-test showed ∗p < 0.05, ∗∗p < 0.01, and ∗∗∗p < 0.001 compared to non-infected controls.
Interestingly, Cy treatment (Cy-uninfected) showed an immunomodulatory effect on lymphocyte populations in the intestinal mucosa. Overall, elevated levels of CD8+ T, CD4+ T, and B-2 cell populations were observed as compared to other groups (Figure 2).
T and B Lymphocytes Decreased in Mesenteric Lymph Nodes of Cy-Infected Mice
The populations of B-2, CD4+ T, CD8+ T, and NKT/NK cells decreased at 21 and 28 dpi in the Cy-infected group as compared to the infected group, suggesting the immunosuppressive effect of Cy. At 14 dpi, a higher percentage of macrophages was present in the Cy-infected group as compared to the infected group, however, this witnessed a decrease at 21 and 28 dpi (Figure 4).
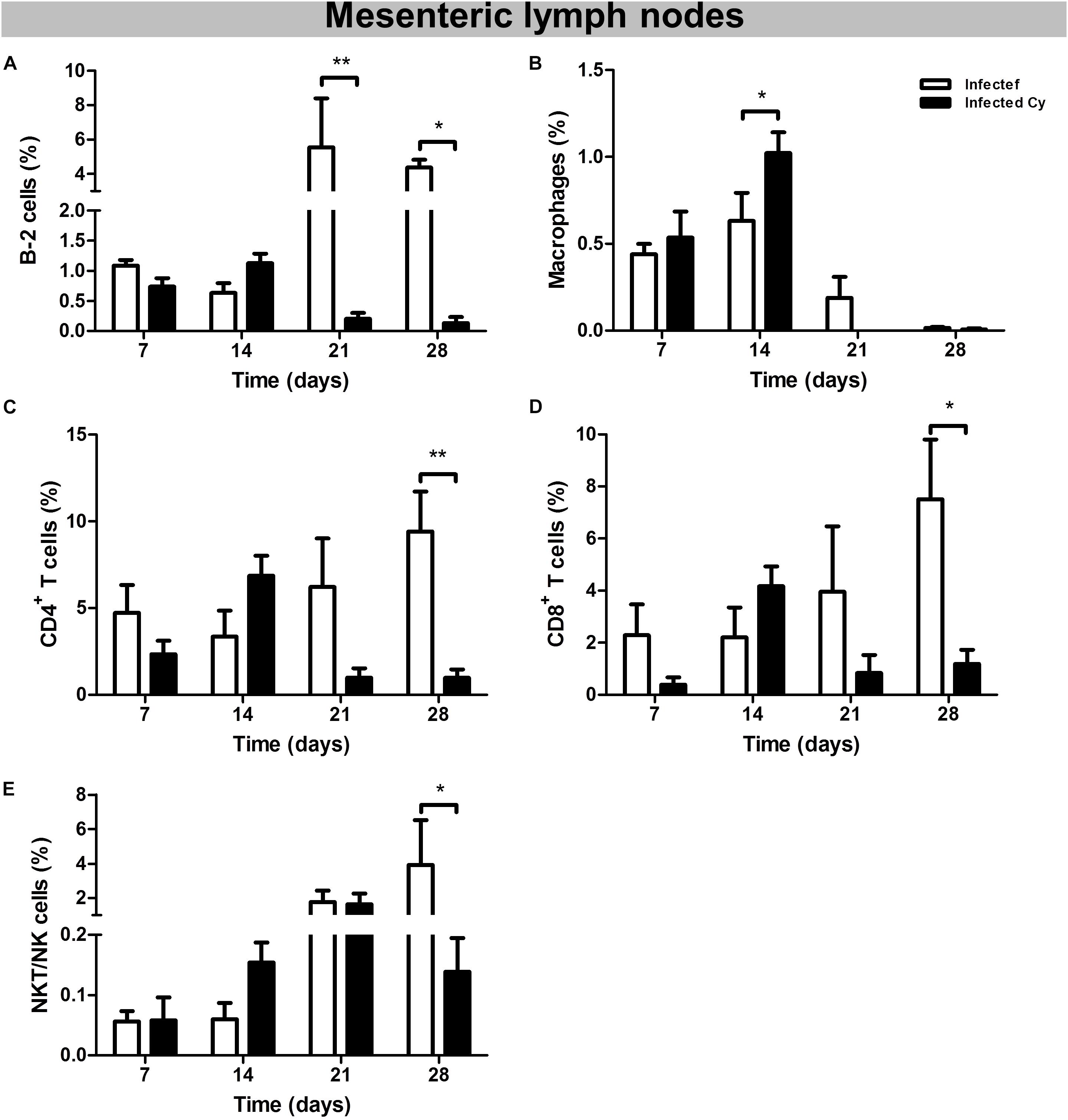
Figure 4. Cell population in the mesenteric lymph nodes of the mice infected with E. intestinalis and treated or not with Cy for 7, 14, 21 and 28 dpi. Percentage of: (A) B-2 cells (CD23+ CD19+); (B) macrophages (CD19– CD11b+); (C) CD4 T lymphocytes (CD19– CD8– CD4+); (D) CD8 T lymphocytes (CD19– CD4– CD8+), (E) NKT/NK cells (CD19– CD4+ NK1.1+). ANOVA test with Tukey’s post-test showed ∗p < 0.05 and ∗∗p < 0.01.
T and B Lymphocytes Decreased in the Peritoneum of E. intestinalis-Infected Mice
The CD4+ T, CD8+ T, and NKT/NK cells decreased significantly in all infected groups as compared to the uninfected control (Figure 5). Moreover, CD8+ T, B-1, and B-2 lymphocytes decreased significantly in all Cy-treated groups at 7 and 28 dpi (Figure 5). At 14 dpi, both B-1 and B-2 cell populations and macrophages increased in the infected group as compared to the Cy-infected group (Figure 5). However, the macrophage number decreased in the infected, Cy-infected, and Cy-uninfected groups at 14 and 28 dpi (Figure 5). Overall, the infected mice showed a decreased number of B-1, B-2, CD4+ T, and CD8+ T cells, with a statistical difference at 14 dpi (Supplementary Figure S2). This difference was maintained at 21 and 28 dpi for B cells, but not for T cells.
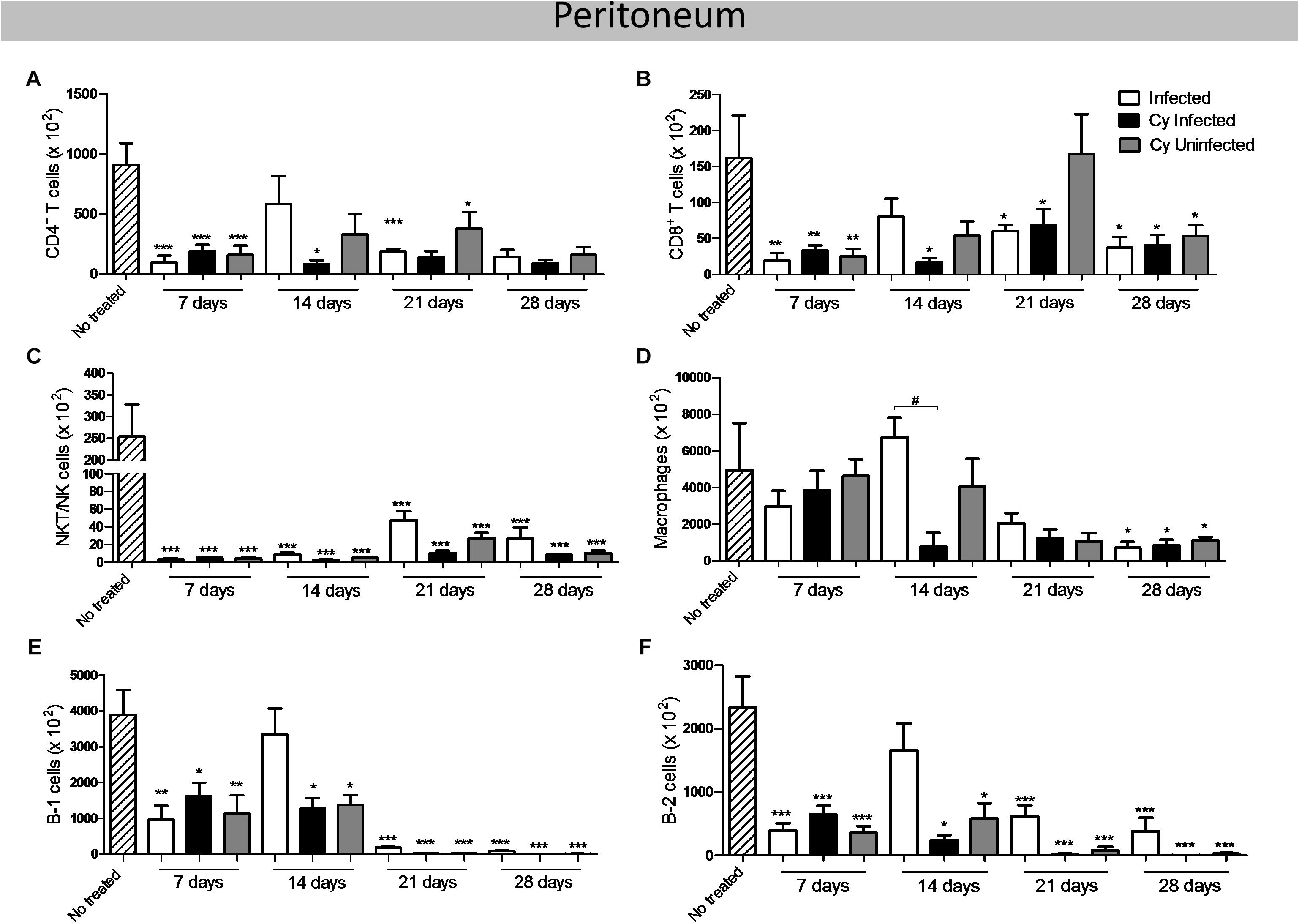
Figure 5. T and B population in the peritoneum of the mice infected with E. intestinalis and treated or not with Cy for 7, 14, 21 and 28 dpi. The number of: (A) CD8 T cells (CD19– CD4– CD8+); (B) CD4 T cells (CD19– CD8– CD4+); (C) NKT/NK cells (CD19– CD4+ NK1.1+); (D) macrophages (CD19– CD11b+); (E) B-1 cells (CD23– CD19+), (F) B-2 cells (CD23+ CD19+). ANOVA test with Tukey’s post-test showed ∗p < 0.05, ∗∗p < 0.01, and ∗∗∗p < 0.001 compared to non-infected controls.
Pro- and Anti-inflammatory Cytokines Detected in the Ileum of Infected Mice
E. intestinalis infection was associated with upregulation of various pro- and anti-inflammatory cytokines, including IFN-γ, TNF-α, IL-2, IL-6, IL-17a, IL-4, and IL-10 at 14 dpi (Figure 6). TNF-α levels persisted at 21 and 28 dpi, whereas other cytokines were not detected at these dpi (Figure 6).
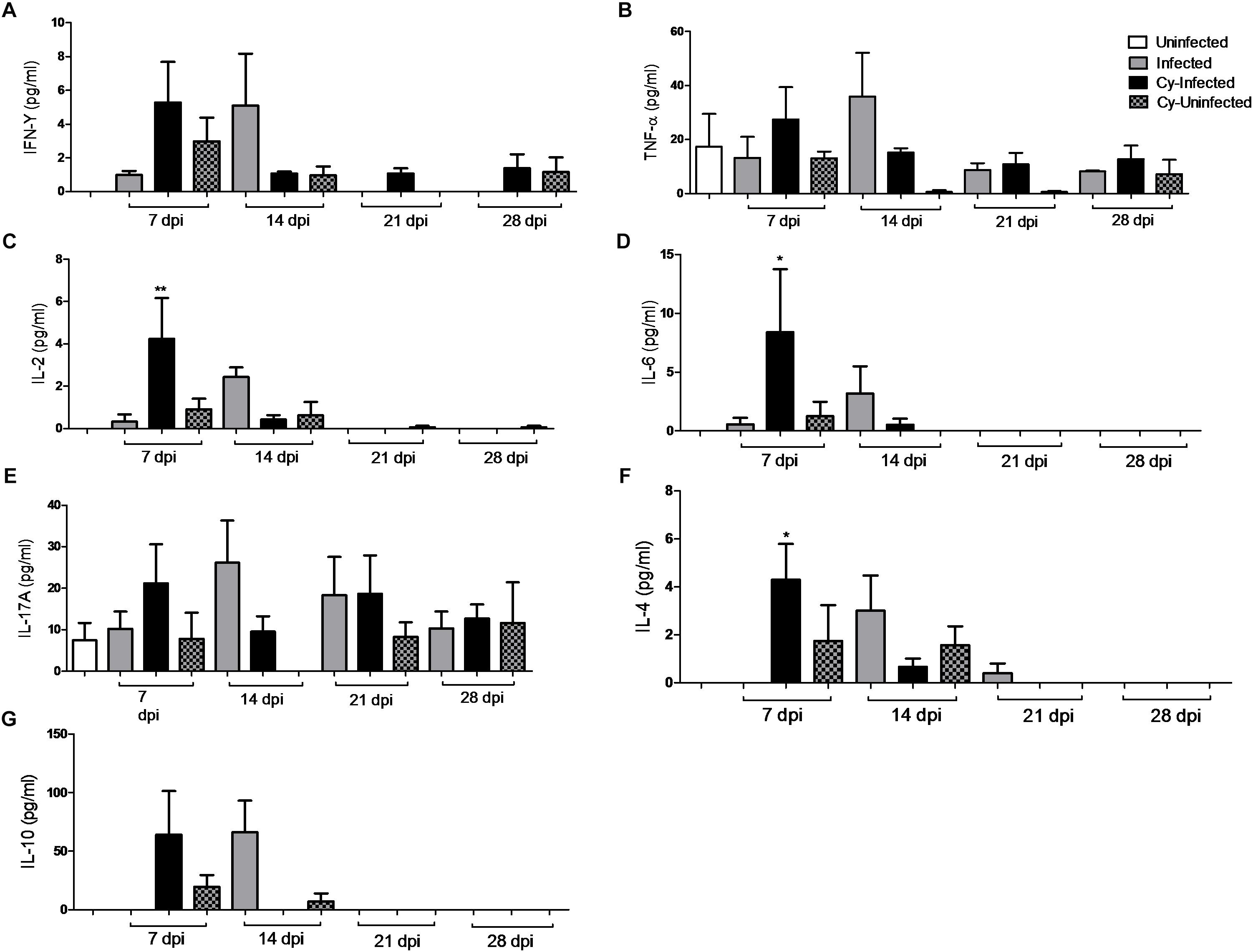
Figure 6. Proinflammatory cytokines (IL-2, IL-6, IFN-γ, and TNF-α) and anti-inflammatory cytokines (IL-4, IL-10, and IL-17A) levels in the intestine of mice infected with E. intestinalis and treated or not with Cy for 7, 14, 21, and 28 dpi. (A) IFN-γ; (B) TNF-α; (C) IL-2; (D) IL-6; (E) IL-17a; (F) IL-4, g) IL-10. ANOVA test with Tukey’s post-test showed ∗p < 0.05 and ∗∗p < 0.01 compared to non-infected controls
These results implied that Cy treatment increased the production of IL-10 and IFN-γ at 7 dpi in the Cy-uninfected group as compared to the uninfected group. The highest level of cytokines was observed in the Cy-infected mice and included mostly IL-2, IL-6 and IL-4. The cytokine IL-17a was detected in all groups in all experimental animals although no statistical difference was observed between them. Overall, the cytokines levels in the present study after E. intestinalis infection corroborate with the histopathological results obtained in all infected groups at 7 and 14 dpi, reflecting the immune system-mediated attempt to counter the intestinal infection.
Transmission Electron Microscopy
Transmission electron microscopy (TEM) results showed E. intestinalis spores of varying shapes, from oval to piriform, to be present close to the microvillosities and in the cytoplasm of enterocytes (Figure 7) of the Cy-infected group. Additionally, two patterns of the morphological invasion were observed: endocytosis (Figures 7a,b) and injection of sporoplasm by polar tubule into the host cells (Figures 7c,d). The discontinuous microvillosities, present next to spores, showed invaginations, manifesting their attempt to engulf spores, a mechanism similar to phagocytosis. However, no phagocytic vacuoles were observed (Figures 7a–c). The cytoplasm surrounding the spores was found to be electrodense with a high number of mitochondria (Figures 7a–c). Moreover, surrounding the E. intestinalis sporoplasm injection demonstrated a loss of microvillosities and cytoplasmic membrane projections involving the extruded polar tubule that appeared to form a channel for its entry into the electrodense cytoplasm (Figure 7d).
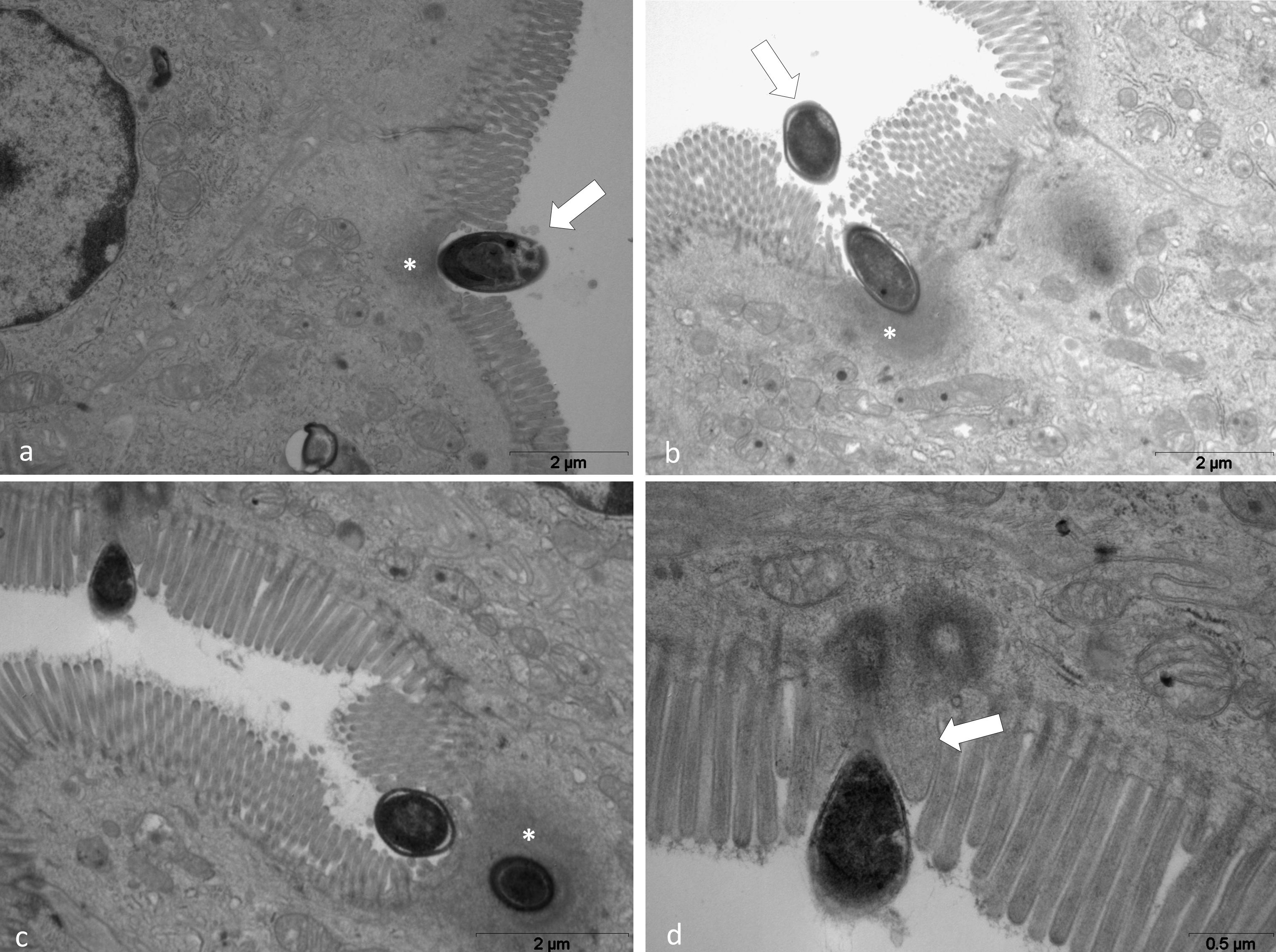
Figure 7. TEM from the intestine of Cy-Infected mice. (a–c) E. intestinalis spores adhered to and enterocyte (∗). Note that microvillosities disappeared, the membrane shows invaginations associated with an electrodense area in the cytoplasm close to the spore (∗). (d) Injection of the sporoplasm with loss of microvillosities (arrow). Projections of cytoplasmic membrane involving the extruded polar tubule (arrow).
Discussion
Encephalitozoon intestinalis invades and develops in the cytoplasm of enterocytes, causing persistent diarrhea in humans with AIDS (Didier, 2005) or in individuals immunocompromised by cancer or chemotherapy (Chabchoub et al., 2009; Hamamci et al., 2015). Microsporidia also infect the macrophages of the lamina propria that help in its dissemination to kidneys and the hepatobiliary tract (El Fakhry et al., 2001). In the present study, we observed oral infection with E. intestinalis not to be associated with clinical signs, such as diarrhea, even in the Cy-immunosuppressed mice. However, microscopically, lymphoplasmacytic enteritis was observed in association with E. intestinalis spores.
The adaptive immune response is very crucial for containing microsporidiosis. For example, T cells-deficient mice (athymic/nude or SCID) are incapable of successfully killing the pathogen and die due to encephalitozoonosis as opposed to immunocompetent mice (Gannon, 1980; Moretto et al., 2012). Similarly, IFN-γ–/– mice lacking major proinflammatory cytokines showed hepatomegaly, colicystitis, splenomegaly, intestinal enlargement, and ascites three to four weeks post infection with E. intestinalis. Moreover, these mice could live only up to 6 weeks post infection (El Fakhry et al., 2001). Therefore, physical or genetic deletion of immune cells or cytokines represents a situation that does not correspond to clinical observations observed in individuals immunosuppressed by drugs. Since immunosuppressive drugs act on different cells of the immune system, leading to a diversity of immunocompromised states, we used Cy to mimic the physiological immunosuppression situations.
We observed that Cy-infected mice showed a higher number of spores, reflecting the increased susceptibility to pathogen upon treatment with Cy, as previously reported by our group in experimental E. cuniculi infection in mice (Lallo and Hirschfeld, 2012). Unexpectedly, the present results showed a decrease in fungal burden and histopathological lesions in immunosuppressed mice at 21 and 28 dpi, indicating successful elimination of pathogen. Cy treatment is lymphotoxic and rapidly decreases the T and B cell populations (Brodsky, 2010). However, a study reported it to cause an extensive mobilization of immune cells from the bone marrow and other lymphoid organs (Sistigu et al., 2011). In the present study, we observed an increase in CD4+ T and CD8+ T cells population in the IM of Cy-treated mice. These results may be associated to two possible explanations: (i) the displacement of immune cells from nearby immune sites such as Peyer’s patches, lymph nodes and peritoneum favored the assembly of an immune response in the gut capable of controlling infection, however, in this case, it should be considered that such reduction could be linked to the suppressive effect of Cy on these immune sites, or (ii) low action on the immune population of the intestinal mucosa, especially T cells, data that may be linked to a possible immunomodulatory activity of Cy, which should be further explored.
Another observation that point toward resolution of infection is the increased presence of mitosis in the intestinal crypts of the small intestine associated with enteritis in all infected animals, indicating tissue remodeling in the intestine under both pathological and physiological conditions (Fuss et al., 2004). Since enterocytes serve as the first physical barrier against pathogens in intestine, maintenance of a strong epithelial barrier is crucial for normal physiology and evasion of infections. Moreover, epithelial cells secrete important chemoattractants that are involved in cell chemotaxis (Goodman and Pizarro, 2013). The IELs constitute a sub-population of T lymphocytes in the IM that has been implicated in both intestinal homeostasis and inflammation, as observed in intestinal toxoplasmosis. Another important category of immune cells is dendritic cells that reside in the lamina propria beneath the IM and act as antigen presenting cells. They play a crucial role in containing toxoplasmosis. Lamina propria also comprises CD4+ T, CD8+ T, and B lymphocytes; B cells are known for producing secretory IgA that is responsible for the exclusion of environmental antigens (Cohen and Denkers, 2015).
There are reports stating an effective immune response against microsporidia to involve majorly CD8+ T cells (Khan et al., 1999; Braunfuchsová et al., 2001). Interestingly, other studies report CD8+ T cells and not CD4+ T cells to be indispensable in successfully eliminating pathogens in intraperitoneal infection by E. cuniculi (Moretto et al., 2000; Braunfuchsová et al., 2001). In fact, the dichotomic role of CD4+ T cells has been shown to be associated with the infection route. It has been shown that the oral infection with E. cuniculi stimulates a synergistic effect of CD8+ and CD4+ T cells (Moretto et al., 2004). The present study also reported an increased number of CD4+ T cells in the intestines of infected mice, contributing to successful E. intestinalis elimination. We believe this increase in CD4+ T cells to be attributed to the immunomodulatory effect of Cy in the intestinal mucosa.
Evidence from literature reports CD4+ and CD8+ T cells, associated with IFN-γ and IL-12 cytokines, to be important players in eliminating E. intestinalis infection (Salát et al., 2004, 2006). The present results reinforce this hypothesis; as evident by a significant increase in CD8+ T cells in all infected groups. A subsequent decrease in CD8+ T cell population was associated with a decrease in spore numbers and histopathological lesions. At 7 and 14 dpi, the increased production of TNF-α, IFN-γ, and IL-10 cytokines was noticed in the ileum of infected mice, together with an increase in IL-2 and IL-4 in Cy-infected mice. The results suggest that besides IFN-γ (Salát et al., 2004, 2006), other cytokines, including anti-inflammatory cytokines, play a crucial role in mediating an intestinal immune response against E. intestinalis, for the resolution of enteritis and pathogen killing.
The IELs comprise a heterogeneous population, predominantly composed of CD8+ T (CD8αα and CD8αβ) cells and a few CD4+ T lymphocytes and known to play an important role in oral infections. Upon infection, an expansion in the number of these cells causes increased production of IFN-γ, cytolytic properties of which inhibit intestinal E. cuniculi proliferation and dissemination (Moretto et al., 2004). In fact, our results showed an increased CD8+ T IELs in intestinal E. intestinalis infection. However, CD8+ T cells decreased in the peritoneal cavity, suggesting their migration to the site of pathogen proliferation. This finding is in corroboration to previous studies that have already shown cells in the peritoneal cavity to migrate to other sites (Almeida et al., 2001; Palos et al., 2012).
Our group has previously shown B-1 cells to be an important player in the generation of the immune response against E. cuniculi infection (Da Costa et al., 2017; Langanke dos Santos et al., 2018). B-1 cells are important in generating adaptive immune response and antibody production, which is independent of T cells (Choi and Baumgarth, 2008). However, B-1 cells are also dependent on T cells for generating an effective immune response, such as cell-mediated hypersensitivity (Szczepanik et al., 2003; De Lorenzo et al., 2007) and rejection of aloenxerts (Nogueira-Martins and Mariano, 2010). Moreover, adoptive transfer of B-1 cells activates T cells that produce IFN-γ (Margry et al., 2013). Here, we showed a decrease in B-1 cell frequency in infected animals as compared to controls of both intestine and peritoneal cavity. We hypothesized that B-1 cells from the peritoneal cavity differentiated into B-1 cell-derived phagocyte (B-1 CDP) in infected mice, which, in turn, promoted phagocytosis of E. intestinalis spores. In vitro studies showed that B-1 cells may also differentiate into mononuclear phagocytes, which upon attachment to a substrate, acquire a myeloid phenotype (Almeida et al., 2001). Moreover, Propionibacterium acnes infection of B-1 cells of myeloid lineage induced only differentiation into phagocytes (Mussalem et al., 2012), however, the role of B-1 cells in the intestines warrants further understanding of microsporidia infection.
We also found a decrease in the NKT/NK cells in the intestine and mesenteric lymph nodes in all infected mice. NKT cells are known to play a protective role in Toxoplasma gondii oral infection although these cells are susceptible to direct invasion by the parasite (Persson et al., 2009). In fact, NKT cells develop a hypermotility phenotype in vivo during T. gondii oral infection, suggesting manipulation of motility of immune cells by T. gondii, which assist in the spread of the causative organism (Seipel et al., 2010; Harker et al., 2013), as already shown in macrophages (Seipel et al., 2010). E. cuniculi uses macrophages as a Trojan horse (Mathews et al., 2009), however, whether this phenomenon occurs with NKT/NK cells in microsporidiosis remains largely unknown.
While an effective immune response against microsporidia is predominantly driven by T cells, dendritic cells have also been shown to play an important and critical role in stimulating these cells. Dendritic cells of the IM act as antigen-presenting cells and are responsible for priming T naive cells in effectors and memory cells upon infection (Steimnman and Hemmi, 2006.). The secretion of cytokines and chemokines from infected enterocytes results in migration of these cells from PP to the mucosa (Goodman and Pizarro, 2013). On the other hand, a study reported in vitro inhibition of dendritic cell differentiation by E. intestinalis (Bernal et al., 2016). On the same lines, we, in the present study, demonstrate a reduction in dendritic cells in PP of infected animals at 7 and 14 dpi, which was associated with a higher number of fungal spores and histopathological lesions, suggesting the migration of these cells to the site of infection.
In the gastrointestinal tract, dendritic cells play an important role in suppression of colitis development, inducing the traffic of T regulatory cells in the intestine, and inducing IgA secretion from B cells in the intestine (Goodman and Pizarro, 2013). These are also involved in inducing oral tolerance and preventing inflammatory response mediated by gut microbiota and ingested antigens (Goodman and Pizarro, 2013). The breach in this function may decrease the number of T regulatory cells and increase the number of cells producing Th1 and Th17 cytokines (Goodman and Pizarro, 2013). A lower frequency of dendritic cells observed in the present study is related to a higher parasite burden, however, this is yet to be clarified.
It has been previously shown that microsporidia spores infect new cells mostly by injection of sporoplasm into the host cells via the polar tubule (Leitch et al., 2005). However, different cell lineages are capable of phagocytosing microsporidia spores (Couzinet et al., 2000; Franzen et al., 2005), suggesting endocytosis to be an important mechanism in intestinal microsporidiosis. In order to evade this protective mechanism, microorganisms have developed complex systems to subvert endocytosis by host cells, allowing invasion of even those cells that generally do not phagocytose (Cossart and Sansonetti, 2004). Previous studies have suggested microsporidia to induce invaginations of the cell membrane of host cells close to the polar tubule in a process similar to endocytosis, causing injection of sporoplasm into the host cell. The results of TEM showed that a phenomenon similar to phagocytosis occur more frequently in E. intestinalis infection.
The results of this study showed that the increase in the CD8+ and CD4+ T cell population in the intestinal mucosa of Cy immunosuppressed mice and orally infected with E. intestinalis, in association with the presence of pro-inflammatory cytokines, controlled the infection by the opportunistic fungus, although reduction of cellular populations at immune sites has been observed, confirming a state of immunosuppression, reinforcing that the selective effect of Cy should be better understood.
Data Availability Statement
The raw data supporting the conclusions of this manuscript will be made available by the authors, without undue reservation, to any qualified researcher.
Ethics Statement
The animals were manipulated in accordance with the Brazilian legislation that is regulated by the National Council for the Control of Animal Experimentation (CONCEA) and by the Ethical Principles in Animal Research formulated by the Brazilian Society of Science in Laboratory Animals. The whole experimental protocol was also approved by the Paulista University Ethics Committee on Use of Animals (CEUA – protocol number 313/15), SP, Brazil.
Author Contributions
ML, AA-S, and EP conceived and designed the experiments. MM, CM, PR, DS-M, and JX performed the experiments and contributed to immunological and histopathological analysis tools. ML, AA-S, and EP analyzed the data and wrote the manuscript. All authors approved the final version to be published.
Funding
This work was supported by the Fundação de Amparo à Ciência do Estado de São Paulo–Fapesp and Coordenação de Aperfeiçoamento de Pessoal de Nível Superior - Capes (project numbers: 2015/25948-2 and 2012/51727-5).
Conflict of Interest
The authors declare that the research was conducted in the absence of any commercial or financial relationships that could be construed as a potential conflict of interest.
Acknowledgments
We thank Magna Maltauro Soares (Instituto Butantan) for histopathological processing.
Supplementary Material
The Supplementary Material for this article can be found online at: https://www.frontiersin.org/articles/10.3389/fmicb.2019.02205/full#supplementary-material
FIGURE S1 | T cells population in the intestinal mucosa of mice infected with E. intestinalis and treated or not with Cy for 7, 14, 21, and 28 dpi. Percentage of lymphocytes (A) CD8 T (CD19– CD4–CD8+), and (B) NK/NKT cells (CD19– CD4+ NK1.1+). ANOVA test with Tukey’s post-test showed $p < 0.05, when compared to the same group at different dpi.
FIGURE S2 | B and T cells into the peritoneal cavity of mice infected with E. intestinalis and treated or not with Cy for 7, 14, 21 and 28 dpi. (A) B-1 (CD23– CD19+), (B) B-2 (CD23+ CD19+), (C) T CD4 (CD19– CD8– CD4+), and (D) T CD8 (CD19– CD4– CD8+) cells numbers. ANOVA test with Tukey’s post-test showing ∗p < 0.05,∗∗p < 0.01, ∗∗∗p < 0.001, and ∗∗∗∗p < 0.0001.
References
Allaire, J. M., Crowley, S. M., Law, H. T., Chang, S., Ko, H., and Vallance, B. A. (2018). The intestinal epithelium: central coordinator of mucosal immunity. Trends Immunol. 39, 677–696. doi: 10.1016/j.it.2018.04.002
Almeida, S. R., Aroeira, L. S., Frymuller, E., Dias, M. A., Bogsan, C. S., Lopes, J. D., et al. (2001). Mouse B-1 cell-derived mononuclear phagocyte, a novel cellular component of acute non-specific inflammatory exudate. Int. Immunol. 13, 1193–1201. doi: 10.1093/intimm/13.9.1193
Bednarska, M., Jankowska, I., Pawelas, A., Piwczynìska, K., Bajer, A., Wolska-Kusìnierz, B., et al. (2018). Prevalence of Cryptosporidium, Blastocystis, and other opportunistic infections in patients with primary and acquired immunodeficiency. Parasitol. Res. 117, 2869–2879. doi: 10.1007/s00436-018-5976-6
Bernal, J. A., Andrés, M., and Palmero, M. F. (2016). Bruton’s tyrosine kinase inhibitors could induce rheumatoid arthritis-like manifestations: a comment on the article by Nyhoff et al. Arthritis Rheumatol. 16:475. doi: 10.1002/art.39956
Braunfuchsová, P., Salát, J., and Kopecký, J. (2001). CD8+ T-lymphocytes protect SCID mice against Encephalitozoon cuniculi infection. Int. J. Parasitol. 15, 681–686. doi: 10.1016/s0020-7519(01)00134-5
Brodsky, R. A. (2010). High-dose cyclophosphamide for autoimmunity and alloimmunity. Immunol. Res. 47, 79–84. doi: 10.1007/s12026-009-8149-y
Chabchoub, N., Abdelmalek, R., Mellouli, F., Kanoun, F., Thellier, M., Bouratbine, A., et al. (2009). Genetic identification of intestinal microsporidia species in immunocompromised patients in Tunisia. Am. J. Trop. Med. Hyg. 80, 24–27. doi: 10.4269/ajtmh.2009.80.24
Choi, Y. S., and Baumgarth, N. (2008). Dual role for B-1a cells in immunity to influenza virus infection. J. Exp. Med. 205, 3053–3064. doi: 10.1084/jem.20080979
Cohen, S. B., and Denkers, E. Y. (2015). The gut mucosal immune response to Toxoplasma gondii. Parasite Immunol. 37, 108–117. doi: 10.1111/pim.12164
Colvin, O. M. (1999). An overview of cyclophosphamide development and clinical applications. Curr. Pharm. Des. 5, 555–560.
Cossart, P., and Sansonetti, P. J. (2004). Bacterial invasion: the paradigms of entero invasive pathogens. Science 304, 242–248. doi: 10.1126/science.1090124
Couzinet, S., Cejas, E., Schittny, J., Deplazes, P., Weber, R., and Zimmerli, S. (2000). Phagocytic up take of Encephalitozoon cuniculi by non professional phagocytes. Infect. Immun. 68, 6939–6945. doi: 10.1128/iai.68.12.6939-6945.2000
Da Costa, L. F., Alvares-Saraiva, A. M., Dell’Armelina Rocha, P. R., Spadacci-Morena, D. D., Perez, E. C., Mariano, M., et al. (2017). B-1 cell decreases susceptibility to encephalitozoonosis in mice. Immunobiol 222, 218–227. doi: 10.1016/j.imbio.2016.09.018
De Lorenzo, B., Brito, R. R., Godoy, L. C., Lopes, J. D., and Mariano, M. (2007). Tolerogenic property of B-1b cells in a model of allergic reaction. Immunol. Lett. 114, 110–118. doi: 10.1016/j.imlet.2007.09.013
Dezern, A. E., Styler, M. J., Drachman, D. B., Hummers, L. K., Jones, R. J., and Brodsky, R. A. (2013). Repeated treatment with high dose cyclophosphamide for severe autoimmune diseases. Am. J. Blood Res. 3, 84–90.
Didier, E. S. (2005). Microsporidiosis: an emerging and opportunistic infection in human and animals. Acta Trop. 94, 61–76. doi: 10.1016/j.actatropica.2005.01.010
Didier, E. S., and Weiss, L. M. (2011). Microsporidiosis: not just in AIDS patients. Curr. Opin. Infect. Dis. 24, 490–495. doi: 10.1097/QCO.0b013e32834aa152
El Fakhry, Y., Achbarou, A., Franetich, J. F., Desportes-Livage, I., and Mazier, D. (2001). Dissemination of Encephalitozoon intestinalis, a causative agent of human microsporidiosis, in IFN-gamma receptor knockout mice. Parasite Immunol. 23, 19–25. doi: 10.1046/j.1365-3024.2001.00351.x
Emadi, A. L., Jones, R. J., and Brodsky, R. A. (2009). Cyclophosphamide and cancer: golden anniversary. Nat. Rev. Clin. Oncol. 6, 638–647. doi: 10.1038/nrclinonc.2009.146
Field, A. S., and Milner, D. A. (2015). Intestinal microsporidiosis. Clin. Lab. Med. 35, 445–459. doi: 10.1016/j.cll.2015.02.011
Franzen, C., Muller, A., Hartmann, P., and Salzberger, B. (2005). Cell invasion and intracellular fate of Encephalitozoon cuniculi (Microsporidia). Parasitol 130, 285–292. doi: 10.1017/s003118200400633x
Fuss, I. J., Heller, F., Boirivant, M., Leon, F., Yoshida, M., Fichtner-Feigi, S., et al. (2004). Nonclassical CD1d-restricted NK T cells that produce IL-13 characterize an atypical Th2 response in ulcerative colitis. J. Clin. Invest. 15, 1490–1497. doi: 10.1172/JCI19836
Gannon, J. (1980). The course of infection of Encephalitozoon cuniculi in immunodeficient and immunocompetent mice. Lab. Anim. 14, 189–192. doi: 10.1258/002367780780937652
Ghoyounchi, R., Mahami-Oskouei, M., Rezamand, A., Spotin, A., Aminisani, N., Nami, S., et al. (2019). Molecular phylodiagnosis of Enterocytozoon bieneusi and Encephalitozoon intestinalis in children with cancer: microsporidia in malignancies as an emerging opportunistic infection. Acta Parasitol. 64, 103–111. doi: 10.2478/s11686-018-00012-w
Goodman, W. A., and Pizarro, T. T. (2013). Regulatory cell populations in the intestinal mucosa. Curr. Opin. Gastroenterol. 29, 614–620. doi: 10.1097/MOG.0b013e328365d30f
Guy-Grand, D., Griscelli, C., and Vassali, P. (1978). The mouse gut T lymphocyte, a novel type of T cell: nature, origin, and traffic in mice in normal and graft-versus-host conditions. J. Exp. Med. 148, 1661–1677. doi: 10.1084/jem.148.6.1661
Hamamci, B., Çetinkaya, Ü, Berk, V., and Kaynar, L. (2015). Prevalence of Encephalitozoon intestinalis and Enterocytozoon bieneusi in cancer patients under chemotherapy. Mikrobiyol. Bul. 49, 105–113. doi: 10.5578/mb.8787
Han, B., and Weiss, L. M. (2017). Microsporidia: obligate intracellular pathogens within the fungal kingdom. Microbiol. Spectr. 5, 1–26. doi: 10.1128/microbiolspec.FUNK-0018-2016
Harker, K. S., Ueno, N., Wang, T., Bonhomme, C., Liu, W., and Lodoen, M. B. (2013). Toxoplasma gondii modulates the dynamics of human monocyte adhesion to vascular endothelium under fluidic shear stress. J. Leukoc. Biol. 93, 789–800. doi: 10.1189/jlb.1012517
Hinney, B., Sak, B., Joachim, A., and Kváč, M. (2016). More than a rabbit’s tale e Encephalitozoon spp. in wild mammals and birds. Int. J. Parasitol. 5, 76–87. doi: 10.1016/j.cll.2015.02.011
Khan, I. A., Schwartzmn, J. D., Kasper, L. H., and Moretto, M. (1999). CD8+CTLS are essencial for protective against Encephalitozoon cuniculi infection. J. Immunol. 162, 6086–6091.
Lallo, M. A., and Hirschfeld, M. M. P. (2012). Encephalitozoonosis in pharmacologically immunosuppressed mice. Exp. Parasitol. 131, 339–343. doi: 10.1016/j.exppara.2012.04.019
Langanke dos Santos, D., Alvares-Saraiva, A. M., Xavier, J. G., Spadacci-Morena, D. D., Peres, G. B., Dell’Armelina Rocha, P. R., et al. (2018). B-1 cells upregulate CD8 T lymphocytes and increase proinflammatory cytokines serum levels in oral encephalitozoonosis. Microbes Infect. 20, 196–204. doi: 10.1016/j.micinf.2017.11.004
Leitch, G. J., Ward, T. L., Shaw, A. P., and Newman, G. (2005). Apical spore phagocytosis is not a significant route of infection of differentiated enterocytes by Encephalitozoon intestinalis. Infect. Immun. 73, 7697–7704. doi: 10.1128/IAI.73.11.7697-7704.2005
Mangan, N. E., and Fung, K. Y. (2012). Type I interferons in regulation of mucosal immunity. Immunol. Cell Biol. 90, 510–519. doi: 10.1038/icb.2012.13
Margry, B., Wieland, W. H., van Kooten, P. J., van Eden, W., and Broere, F. (2013). Peritoneal cavity B-1a cells promote peripheral CD4+ T-cell activation. Eur. J. Immunol. 43, 2317–2326. doi: 10.1002/eji.201343418
Mathews, A., Hotard, A., and Hale-Donze, H. (2009). Innate immune responses to Encephalitozoon species infections. Microbes Infect. 11, 905–911. doi: 10.1016/j.micinf.2009.06.004
Moretto, M., Casciotti, L., Durell, B., and Khan, I. A. (2000). Lack of CD4+ T cells does not affect induction of CD8+ T-cell immunity against Encephalitozoon cuniculi infection. Infect. Immun. 68, 6223–6232. doi: 10.1128/iai.68.11.6223-6232.2000
Moretto, M., Khan, I. A., and Weiss, L. M. (2012). Gastrointestinal cell mediated immunity and the microsporídia. PLoS Pathogen 8:e1002775. doi: 10.1371/journal.ppat.1002775
Moretto, M., Weiss, L. M., and Khan, I. A. (2004). Induction of a rapid and strong antigen-specific intraepithelial lymphocyte response during oral Encephalitozoon cuniculi infection. J. Immunol. 172, 4402–4409. doi: 10.4049/jimmunol.172.7.4402
Mussalem, J. S., Squaiella-Baptistão, C. C., Teixeira, D., Yendo, T. M., Thies, F. G., Popi, A. F., et al. (2012). Adjuvant effect of killed Propionibacterium acnes on mouse peritoneal B-1 lymphocytes and their early phagocyte differentiation. PLoS One 7:e33955. doi: 10.1371/journal.pone.0033955
Nogueira-Martins, M. F., and Mariano, M. (2010). B-1 cell participation in T-cell-mediated alloimmune response. Immunobiol 215, 264–274. doi: 10.1016/j.imbio.2009.05.007
Palos, M. C., Azevedo, M. C., Thies, F. G., Osugui, L., Alveres, A. M., Laurindo, M. F., et al. (2012). Different inflammatory stimuli in the footpad of mice influence the kinetics of resident peritoneal cells. Inflamm. Res. 61, 1187–1194. doi: 10.1007/s00011-012-0514-y
Persson, C. M., Lambert, H., Vutova, P. P., Dellacasa-Lindberg, I., Nederby, J., Yagita, H., et al. (2009). Transmission of Toxoplasma gondii from infected dendritic cells to natural killer cells. Infect. Immun. 77, 970–976. doi: 10.1128/IAI.00833-08
Salát, J., Horká, H., Sak, B., and Kopecký, J. (2006). Pure CD4+ T-lymphocytes fail to protect perorally infected SCID mice from lethal microsporidiosis caused by Encephalitozoon cuniculi. Parasitol. Res. 99, 682–686. doi: 10.1007/s00436-006-0208-x
Salát, J., Sak, B., Le, T., and Kopecký, J. (2004). Susceptibility of IFN- gamma or IL-12 knock-out and SCID mice to infection with two microsporidian species, Encephalitozoon cuniculi and E. intestinalis. Folia Parasitol. 51, 275–282. doi: 10.14411/fp.2004.033
Schiavoni, G., Sistigu, A., Valentini, M., Mattei, F., XSestili, P., Spadaro, F., et al. (2011). Cyclophosphamide synergizes with type I interferons through systemic dendritic cell reactivation and induction of immunogenic tumor apoptosis. Cancer Res. 71, 768–778. doi: 10.1158/0008-5472.CAN-10-2788
Seipel, D., Oliveira, B. C., Resende, T. L., Schuindt, S. H., Pimentel, P. M., Kanashiro, M. M., et al. (2010). Toxoplasma gondii infection positively modulates the macrophages migratory molecular complex by increasing matrix metalloproteinases, CD44 and alpha v beta 3 integrin. Vet. Parasitol. 169, 312–319. doi: 10.1016/j.vetpar.2009.12.042
Sevko, A., Sade-Feldman, M., Kanterman, J., Michels, T., Falk, C. S., Umnsky, L., et al. (2013). Cyclophosphamide promotes chronic inflammation-dependent immunosuppression and prevents antitumor response in melanoma. J. Invest. Dermatol. 133, 1610–1619. doi: 10.1038/jid.2012.444
Sistigu, A., Viaud, S., Chaput, N., Bracci, L., Proietti, E., and Zitvogel, L. (2011). Immunomodulatory effects of cyclophosphamide and implementations for vaccine design. Semin. Immunopathol. 33, 369–383. doi: 10.1007/s00281-011-0245-0
Steimnman, R. M., and Hemmi, H. (2006). Dendritic cells: translating innate to adaptive immunity. Curr. Top. Microbiol. Immunol. 311, 17–58. doi: 10.1007/3-540-32636-7_2
Szczepanik, M., Akahira-Azuma, M., Bryniarski, K., Tsuji, R. F., Kawikova, I., Ptak, W., et al. (2003). B-1 B cells mediate required early T cell recruitment to elicit protein-induced delayed-type hypersensitivity. J. Immunol. 171, 6625–6635.
Viaud, S., Flament, C., Zoubir, M., Pautier, P., LeCesne, A., Ribrag, V., et al. (2011). Cyclophosphamide induces differentiation of Th17 cells in cancer patients. Cancer Res. 71, 661–665. doi: 10.1158/0008-5472.CAN-10-1259
Wang, Z. D., Liu, Q., Liu, H. H., Li, S., Zhang, L., Zhao, Z. Y., et al. (2018). Prevalence of Cryptosporidium, microsporidia and Isospora infection in HIV-infected people: a global systematic review and meta-analysis. Parasit. Vectors. 11:28. doi: 10.1186/s13071-017-2558-x
World Health Organization (2013). WHO Model List of Essential Medicine. Available at: http://www.who.int/medicines/publications/essentialmedicines/18th_EML.pdf (accessed May 14, 2017).
Keywords: Encephalitozoon intestinalis, microsporidia, mucosal immunity, intestinal inflammation, cyclophosphamide
Citation: Moura MLC, Alvares-Saraiva AM, Pérez EC, Xavier JG, Spadacci-Morena DD, Moysés CRS, Rocha PRDA and Lallo MA (2019) Cyclophosphamide Treatment Mimics Sub-Lethal Infections With Encephalitozoon intestinalis in Immunocompromised Individuals. Front. Microbiol. 10:2205. doi: 10.3389/fmicb.2019.02205
Received: 15 July 2019; Accepted: 09 September 2019;
Published: 25 September 2019.
Edited by:
Wanderley De Souza, Federal University of Rio de Janeiro, BrazilReviewed by:
Louis Weiss, Albert Einstein College of Medicine, United StatesAndreas Kupz, James Cook University, Australia
Copyright © 2019 Moura, Alvares-Saraiva, Pérez, Xavier, Spadacci-Morena, Moysés, Rocha and Lallo. This is an open-access article distributed under the terms of the Creative Commons Attribution License (CC BY). The use, distribution or reproduction in other forums is permitted, provided the original author(s) and the copyright owner(s) are credited and that the original publication in this journal is cited, in accordance with accepted academic practice. No use, distribution or reproduction is permitted which does not comply with these terms.
*Correspondence: Maria Anete Lallo, bWFyaWEubGFsbG9AZG9jZW50ZS51bmlwLmJy; YW5ldGVsYWxsb0Bob3RtYWlsLmNvbQ==