- 1UCIBIO-REQUIMTE, Departamento de Ciências da Vida, Faculdade de Ciências e Tecnologia, Universidade Nova de Lisboa, Caparica, Portugal
- 2Instituto de Tecnologia Química e Biológica António Xavier, Universidade Nova de Lisboa, Oeiras, Portugal
- 3Centro de Investigação Interdisciplinar Egas Moniz (CiiEM), Instituto Universitário Egas Moniz, Caparica, Portugal
The yeasts belonging to the Wickerhamiella and Starmerella genera (W/S clade) share a distinctive evolutionary history marked by loss and subsequent reinstatement of alcoholic fermentation mediated by horizontal gene transfer events. Species in this clade also share unusual features of metabolism, namely the preference for fructose over glucose as carbon source, a rare trait known as fructophily. Here we show that fructose may be the preferred sugar in W/S-clade species because, unlike glucose, it can be converted directly to mannitol in a reaction with impact on redox balance. According to our results, mannitol is excreted to the growth medium in appreciable amounts along with other fermentation products such as glycerol and ethanol but unlike the latter metabolites mannitol production increases with temperature. We used comparative genomics to find genes involved in mannitol metabolism and established the mannitol biosynthesis pathway in W/S-clade species Starmerella bombicola using molecular genetics tools. Surprisingly, mannitol production seems to be so important that St. bombicola (and other W/S-clade species) deploys a novel pathway to mediate the conversion of glucose to fructose, thereby allowing cells to produce mannitol even when glucose is the sole carbon source. Using targeted mutations and 13C-labeled glucose followed by NMR analysis of end-products, we showed that the novel mannitol biosynthesis pathway involves fructose-6-phosphate as an intermediate, implying a key role for a yet unknown fructose-6-P phosphatase. We hypothesize that mannitol production contributed to mitigate the negative effects on redox balance of the ancient loss of alcoholic fermentation in the W/S clade. Presently, mannitol also seems to play a role in stress protection.
Introduction
Fungi are excellent models in which to explore the evolution of metabolism, as shown by multiple studies focused chiefly on secondary metabolite production by filamentous fungi (Spatafora and Bushley, 2015; Wisecaver and Rokas, 2015; Theobald et al., 2018; Keller, 2019). Ascomycetous yeasts (Saccharomycotina) produce very few secondary metabolites and are mainly known for their ability to convert sugars into ethanol, a trait of wide biotechnological relevance. However, central carbon and nitrogen metabolisms are in fact quite diverse in yeasts, accounting for the utilization of very different carbon and nitrogen sources and different regulation of energy production processes (Kurtzman et al., 2011). Comparative genomics of a large and ever-increasing number of yeast genomes is beginning to shed light on the evolution of metabolic traits in this sub-phylum, thus far revealing a general trend of loss of metabolic capabilities that implies a common ancestor metabolically more complex than most extant species (Shen et al., 2018). These losses most often resulted in narrowing spectra of nutrient assimilation, but we reported recently that even a central metabolic feature like alcoholic fermentation, which usually plays a crucial role in the metabolism of yeasts, either as the main pathway for energy production or as a means to circumvent oxygen shortage, was lost in a lineage within the Saccharomycotina currently comprising approximately 100 species belonging to the genera Wickerhamiella and Starmerella (referred to as the W/S clade) (Gonçalves et al., 2018). An ancestor of this clade seems to have lost the genes required for alcoholic fermentation, encoding pyruvate decarboxylase and alcohol dehydrogenase, which are also absent in at least one extant species, Candida (Wickerhamiella) galacta (Gonçalves et al., 2018). Reinstatement of alcoholic fermentation was subsequently made possible through horizontal acquisition of a bacterial alcohol dehydrogenase, and co-option of a new decarboxylase to accept pyruvate as a substrate (Gonçalves et al., 2018). Alcohol dehydrogenase genes were among the more than 500 bacterial genes detected in W/S-clade genomes. Although this remains to be empirically demonstrated, the unusually high number of horizontally acquired genes in the W/S clade suggests that other aspects of metabolism were also impacted during evolution of the lineage (Gonçalves et al., 2018; Shen et al., 2018; Kominek et al., 2019).
One additional unusual trait in common between W/S-clade species is their preference for fructose over glucose when both carbon sources are present at high concentrations, a trait named fructophily. The preference for fructose relies on a highly unusual specific fructose transporter, Ffz1 (Pina et al., 2004; Leandro et al., 2011, 2014), present only in fructophilic yeasts, which does not belong to the sugar transporter family and was horizontally acquired from filamentous fungi by a W/S-clade ancestor (Gonçalves et al., 2016). Ffz1, like fructophily, is present in most W/S-clade species examined and was shown to be indispensable for preferred fructose metabolism in at least one species in this clade (Gonçalves et al., 2018). However, while Ffz1 ensures that cells can take up large amounts of fructose in a selective manner, it remained unclear why the preferential utilization of fructose was beneficial for W/S-clade yeasts. Most species included in the Wickerhamiella and Starmerella genera have an ecological association with the floral niche, being often isolated from flowers or insects that visit flowers (Lachance et al., 2001; Canto et al., 2017; de Vega et al., 2017). In floral nectars, overall sugar concentrations are very high and fructose is usually roughly as abundant as glucose, sucrose being frequently the main sugar present (Canto et al., 2017; de Vega et al., 2017). Why, in this kind of environment, fructose assimilation should be more advantageous than that of other equally available sugars remained unknown. However, the metabolism of fructophilic bacteria that are also associated with the floral niche (Endo et al., 2009, 2010; Neveling et al., 2012) provides a useful clue. In fructophilic bacteria, the loss of alcoholic fermentation appeared to be linked to acquisition of fructophily because fructose can function both as carbon source and as an electron acceptor (in a reaction that converts fructose to mannitol), ensuring redox balance in the absence of alcoholic fermentation (Maeno et al., 2016, 2019). Interestingly, some fructophilic yeasts from the W/S clade are also known to produce large amounts of mannitol (Savergave et al., 2013), suggesting a possible link between mannitol metabolism and the evolutionary history of alcoholic fermentation in this lineage. On the other hand, mannitol often plays stress protective roles, namely as compatible solute (Chaturvedi et al., 1996; Solomon et al., 2007; Wyatt et al., 2014; Zahid and Deppenmeier, 2016), and W/S-clade yeasts are known to be osmotolerant (Rosa and Lachance, 1998).
To shed light on the role played by mannitol in the metabolism of W/S-clade yeasts, we used comparative genomics and metabolic characterization of mutants constructed in the genetically amenable W/S-clade species Starmerella bombicola.
Our findings suggest that mannitol metabolism was important to the fulfillment of redox balance in the absence of alcoholic fermentation, because direct conversion of fructose into mannitol regenerates NADP+. In support of this, we uncovered a novel biochemical pathway promoting the conversion of glucose to fructose. This pathway, which to our knowledge has not been reported before, enables mannitol production even when glucose is the only sugar available. Hence, we unraveled further the biochemical basis for fructophily in these yeasts and present a model for the evolution of metabolism in the W/S clade in which fructophily is linked to mannitol production and possibly to the ancient loss of alcoholic fermentation in this lineage.
Materials and Methods
Yeast Strains and Culture Conditions
St. bombicola PYCC 5882, Candida magnoliae PYCC 2903, Starmerella bacillaris PYCC 3044, St. bacillaris PYCC 6282 and Wickerhamiella domercqiae PYCC 3067 were obtained from the Portuguese Yeast Culture Collection (PYCC). Wickerhamiella versatilis JCM 5958 was obtained from the Division of Genomic Technologies, RIKEN Center for Life Science Technologies, Japan. All strains were maintained in YPD medium [1% (w/v) yeast extract, 2% (w/v) peptone and 2% (w/v) glucose)].
Assessment of Mannitol Production in W/S-Clade Species
W/S-clade species (St. bombicola, St. bacillaris, C. magnoliae, W. domercqiae and W. versatilis) were cultivated in rich medium [YP, 1% (w/v) yeast extract and 2% (w/v) peptone] supplemented with 10% (w/v) glucose or 10% (w/v) fructose at 30°C until late exponential phase (when most of the sugar was consumed). Extracellular concentrations of fructose, glucose and other extracellular metabolites including mannitol were determined by HPLC using a carbohydrate analysis column (300 mm × 7.8 mm, Aminex HPX-87P, Biorad) and a differential refractometer (LKB 2142). The column was kept at 80°C and H2O was used as the mobile phase at 0.6 mL min–1. The results were treated and integrated using the software Chromeleon v6.8 (Dionex).
Identification of Genes Involved in Mannitol Biosynthesis in W/S Genomes and Construction of Phylogenies
Genes possibly involved in mannitol biosynthesis, namely mannitol dehydrogenase (MtDH), mannitol-1P dehydrogenase (M1PDH) and mannitol-1P phosphatase (MPP), were searched in W/S-clade genomes by tBLASTx, using as query MtDH from Yarrowia lipolytica (YlSDR, accession number: Q6CEE9.1), M1PDH from Aspergillus fumigatus (KEY81154.1) and MPP from Saccharina japonica (AIC75600.1). An E-value cutoff of e–10 was considered for the tBLASTx search. The best hits were used as queries for BLASTp against the NCBI nr database to identify the closest related protein sequences in other yeasts (Saccharomycotina). No hits were obtained for genes other than MtDH and for that reason a detailed Maximum Likelihood (ML) phylogeny was constructed for the protein encoded by this gene. To that end, the top 750 hits BLASTp hits using the Mtdh-like protein from St. bombicola in UniprotKB (reference proteomes database) were selected to construct a ML phylogeny. Mtdh sequences from other W/S-clade species were retrieved by tBLASTx searches in local genome databases. The resulting protein sequences were aligned using an iterative refinement method (L-INS-i) in MAFFT v.6.956 (Katoh and Standley, 2014) and poorly aligned regions were removed with the trimAl (Capella-Gutierrez et al., 2009) software using the ‘gappyout’ option. An ML phylogeny was constructed with IQ-TREE v 1.4.3 (Nguyen et al., 2015) using the ultra-fast bootstrap method and the LG + I + G model of substitution (found as the best-fitting model). Accession numbers for W/S-clade genomes are the following: GCA_003033765.1 (St. bacillaris PYCC 3044), GCA_001005415.1 (Candida apicola NRRL Y-50540), GCA_003033435.1 (St. bombicola PYCC 5882), GCA_003033705.1 (C. magnoliae PYCC 2903), GCA_001600375.1 (W. domercqiae PYCC 3067), GCA_001005415.1 (W. versatilis JCM 5958) and NW_020193984.1 (Wickerhamiella sorbophila DS02). Complete phylogeny and alignment files can be accessed in figshare (10.6084/m9.figshare.9959693).
Construction of Starmerella bombicola Deletion Mutants
Construction of deletion mutants was performed as described in Gonçalves et al. (2018). For mtdh1Δ and mtdh2Δ, the coding sequence (CDS) with ∼1 kb upstream and downstream were amplified from genomic DNA using the primer pairs listed in Supplementary Table S1. For the double mutant (mtdh1Δmtdh2Δ), the same strategy was used as the two genes are located next to each other in the genome separated by a small intergenic region (∼1 kb). The three disruption cassettes were subsequently used to transform St. bombicola PYCC 5882 as previously described in Gonçalves et al. (2018). Two different transformants from each gene disruption transformation were subsequently used for the phenotypic assays.
For the construction of mtdh1Δsor1Δ, the SOR1 gene was searched in the genome of St. bombicola PYCC 5882 using Y. lipolytica sorbitol dehydrogenase as query (XM_503864.1). The putative SOR1 gene was deleted in the mtdh1:HYG background using a zeocin resistance cassette (consisting in the ble gene from Staphylococcus aureus under control of the GPD promoter from St. bombicola and the CYC terminator from Saccharomyces cerevisiae). Transformants were selected in YPD plates (pH = 6.8) supplemented with 650 μg/mL zeocin (Invivogen, San Diego, CA, United States). For the construction of the hxk1Δglk1Δ mutant, HXK1 and GLK1 from S. cerevisiae (YCL040W and YFR053C) were used to search for the respective orthologs in St. bombicola PYCC 5882 genome. A similar strategy as aforementioned was employed, starting with the construction of the glk1:ZEO mutant followed by disruption of the HXK1 in the glk1:ZEO background using a hygromycin resistance cassette.
Enzymatic Assays
For the preparation of cell-free extracts, the cells were collected by centrifugation (3,000 × g for 5 min), washed twice with cold 100 mM Tris-HCl buffer (pH = 7.5) and disrupted with glass beads in 500 μL of Lysis Buffer (0.1 M triethanolamine hydrochloride, 2 mM MgCl2, 1 mM DTT and 1 μM PMSF) with six cycles of 60 s vortex-ice. Cell debris were removed by centrifugation at 4°C, 16,000× g for 20 min and the cleared raw extracts were stored at −20°C. Protein was quantified using the QUBIT fluorimeter following the manufacturer’s guidelines. The mannitol dehydrogenase (Mtdh) assay was performed in both the reductive and the oxidative directions at 25°C. For the oxidative reaction, 500 μL of reaction mixture was used containing 50 mM Tris-HCl (pH = 8.5), 1 mM of NADH or NADPH and 25 μL of protein extract (200–500 ng/μL of total protein). The reaction was started by the addition of fructose to a final concentration of 200 mM and oxidation of NADH or NADPH was monitored by the decrease in absorbance at 340 nm. For the reductive reaction, 50 mM of Tris-HCl (pH = 10) buffer and 1 mM of NAD+ or NADP+ were used instead. The reaction was started by adding mannitol to a final concentration of 50 mM and reduction of NAD+ or NADP+ was monitored by the increase in absorbance at 340 nm.
For the hexokinase assay (used as a control for the quality of the extracts when testing for the lack of Mtdh activity in the St. bombicola mtdh deletion mutants), a reaction mixture was prepared containing 50 mM Tris buffer (pH = 7.5), 10 mM MgCl2, 1 mM ATP, 1 mM NADP+, 0.2 U of glucose-6-P dehydrogenase (Sigma Aldrich, St. Louis, MO, United States) and 1 U phosphoglucose isomerase (Sigma Aldrich, St. Louis, MO, United States). Enzymatic assays were performed at 25°C in a total volume of 500 μL and 25 μL of cell-free extract (200–500 ng/μL of total protein) was used. The reaction was started by the addition of fructose to a final concentration of 100 mM and reduction of NADP+ was monitored spectrophotometrically by the increase in absorbance at 340 nm for 2 min.
For the detection of NADP+-dependent glycerol dehydrogenase activity a reaction mixture containing 50 mM Tris-HCl (pH = 8.5) buffer, 1 mM NADP+ and 25 μL of cell-free extract was prepared. The reaction was started by the addition of 100 mM of glycerol, and NADPH formation was monitored spectrophotometrically for 2 min.
For the detection of glucose isomerase activity, a protocol based on Sayyed et al. (2010) was used. Cells of both the wild type St. bombicola and the mtdh1Δmtdh2Δ mutant were cultivated for 24 or 76 h in YP supplemented with 20% (w/v) of glucose and cell-free extracts were obtained as described above. Glucose isomerase activity was measured in a 2.4 mL reaction mixture containing 20–50 μg of total protein, 0.5 mL 50 mM of KPi (pH = 7.2), 2 mM CaCl2, 20 mM glucose, 10 mM MgSO4.7H2O. Different temperatures (25, 30, and 60°C) and reaction times (1, 3, and 24 h) were tested. The eventual formation of fructose was assessed by HPLC.
For the detection of fructose-6-phosphate phosphatase activity, the mtdh1Δmtdh2Δ mutant was grown in YP medium supplemented with 20% (w/v) glucose for 24 h at 25°C. Cell-free extracts were obtained as previously described. A solution containing 20–50 μg of total protein was incubated at 25°C in 100 mM of Tris Buffer (pH = 7.5) for 3 min. The reaction was started by the addition of fructose-6-phosphate to a final concentration of 50 mM or glucose-6-phosphate (used as a control) and left to proceed for 25 min. Two controls were also included, one in which no substrate was added and another one in which no extract was added. 300 μL samples were taken at different time points (0, 5, 10, and 20 min) and were immediately subjected to a procedure for the detection of inorganic phosphate based on Ames (1966). Briefly, 700 μL of a reagent mix (consisting in six parts of a solution of 0.42% (w/v) ammonium molybdate prepared in 1 N H2SO4 and one part of a solution of 10% (w/v) ascorbic acid prepared in milli-Q water) was added to each of the samples. The reaction was left to proceed for 1 h at 37°C. Absorbance at 820 nm was then measured.
Growth Assays and Sugar Consumption
For growth assays, St. bombicola wild type and MtDH deletion mutants were pre-grown overnight in 100 mL flasks containing 20 mL of culture medium at the temperature of choice. This inoculum was diluted to OD640 = 0.2 in 30 mL of YP (1% (w/v) yeast extract and 2% (w/v) peptone) supplemented with the different carbon sources tested in 250 mL flasks. Growth was monitored for 96–150 h.
To assess sugar consumption and metabolite production, wild type, mtdh1Δ and mtdh1Δmtdh2Δ were grown as described before in YP supplemented with 10% (w/v) glucose and 10% (w/v) fructose at 30°C. Samples of 1 mL were collected from culture supernatants, centrifuged at 16,000 x g for 1 min and analyzed by HPLC as described previously. Data was visualized using GraphPad Prism version 6 for Windows, GraphPad Software, San Diego, CA, United States, www.graphpad.com.
Quantification of Extracellular and Intracellular Metabolites
Starmerella bombicola wild type was cultivated for 72 h in 30 mL of YP medium supplemented with 10% (w/v) fructose and 10% (w/v) glucose (20FG medium) at 20, 25, and 30°C at 180 r.p.m. After this period, cells were harvest by centrifugation (4°C, 10 min, 8,000 × g) and 1 mL of supernatant was collected to quantify extracellular metabolites by HPLC as described previously.
Cell-free extracts for intracellular metabolite analysis were obtained as described for the enzymatic assays. The samples were filtered using a nylon filter with a pore diameter of 0.2 μm and subsequently eluted with 0.6 M NaOH at 0.4 mL/min at 25°C in a CarboPac MA1 column and the peaks were identified with a pulsed amperometric detector.
To determine the total dry weight 2 mL of culture broth were centrifuged for 5 min at 9,000 × g. Pellets were washed twice and resuspended in distilled water. The suspension was filtered onto a nitrocellulose filter with a pore diameter of 0.45 μm and was dried at 80°C until a constant weight was attained.
To estimate the intracellular concentration of mannitol per cell (mM), the total amount of mannitol in the cell-free extracts was determined and divided by the number of cells used to produce the extract. We estimate that the volume of one cell of St. bombicola would be ∼4 × 10–10 mL [half the volume of S. cerevisiae, Zakhartsev and Reuss (2018)]. Statistical significance was tested using a one-way ANOVA using the Bonferroni’s correction for multiple testing, implemented in GraphPad Prism v6.
13C-NMR Analysis of 13C-Labeled Glucose Metabolism by Resting Cells
Cells were grown in YP medium supplemented with 20% (w/v) glucose (YP20G) for 24 h at 30°C. Cells were harvested, washed twice with 50 mM KPi buffer (pH = 7) and kept on ice. The cells were resuspended in 5 mL of KPi buffer (50 mM, pH = 7), to a final OD640∼50. The cell suspension was incubated at 30°C and approximately 50 mM of [1-13C]glucose or [2-13C]glucose was provided at time point zero; aliquots of 750 μL were withdrawn at different time points (0, 15, 30, 45, 60, and 120 min). The collected samples were centrifuged, and the supernatant solutions stored at −20°C until further analysis. NMR samples were prepared by adding 75 μL of deuterated water containing trimethylsilylpropanoic acid (TSP, final concentration of 0.44 mM) to 475 μL of each supernatant solution. All spectra were acquired at 30°C in a Bruker Avance II+ 500 spectrometer, equipped with a 5 mm TCI H/C/N Prodigy Cryoprobe. For each sample the following spectra were acquired: one-dimensional 1H-NMR (noesygppr1d, 64 free induction decays, with 64 k complex points, spectral window of 20 ppm, 5 s relaxation delay and 10 ms mixing time); 13C-NMR with inverse gated proton decoupling (zgig, 1k free induction decays, with 64 k complex points, spectral window of 248.5 ppm and 60 s relaxation delay). Whenever needed for spectral assignment, extra spectra were acquired: quantitative 1H-NMR spectra (zgprde, 64 free induction decays, with 64 k complex points, spectral window of 20 ppm, 4 s relaxation delay and 60 s extra relaxation delay) and two-dimensional 13C-1H HSQC spectra (hsqcetgpsisp2 pulse sequence, 256 points in F1 and 2048 points in F2; 32 free induction decays; relaxation delay of 1.5 s; sweep width of 25153.695 Hz in F1 and 8012.820 Hz in F2). Spectra acquisition and processing was performed using TopSpin 3.2. The concentration of β-[2-13C]glucose and [2-13C]ethanol was determined in the fully relaxed 1H-NMR spectra using the TSP signal as an internal concentration standard; this information was used to determine 13C concentrations from 13C-NMR spectra. Assignment of spectra was performed resorting to the HMDB1 and BMRB2 databases.
Analysis of Metabolic Fluxes
The essential features of the model required for flux analysis were determined by the metabolites which were observed (shown in bold in Figure 3B) and the standard routes into the TCA cycle. The appearance of significant amounts of fructose with reversed label (e.g., [6-13C]fructose from [1-13C]glucose) required the inclusion of a reverse flux from the triose pool to fructose. Numerical values were obtained by assuming that the fructose concentration reached an approximate steady state after 30 min, i.e., that the fluxes into and out of the fructose pool are then equal. The ratio of original and reversed label is then given by (2 × fIN + fR)/fR, where fR is the back-flux and fIN is the inward flux of labeled glucose, obtained from its approximately linear consumption.
The flux through the pentose phosphate pathway (PPP) was obtained from the concentrations of [1-13C]fructose and [1,3-13C]fructose observed in experiments starting with [2-13C]glucose. In the first cycle of PPP, three molecules of [2-13C]glucose will produce one of [1-13C]fructose-6-P and one of [1,3-13C]fructose-6-P. The mole fractions of fructose with carbon 1, 2 or 5 labeled (m1, m2 and m5) are approximately constant, and reflects its origin either directly from [2-13C]glucose (with back-flux) or via PPP. The flux into PPP is then 1.5 m1fIN/(1.5 m1 + m2 + m5), allowing for the production of two fructose molecules from three of glucose in PPP. Consumption of [1,3-13C]fructose in a second PPP cycle produced a small amount of [2,3-13C]fructose which was not used in the estimation of fluxes. A small but significant amount of [3-13C]fructose was also generated but its origin remains obscure.
Results
Identification of Putative Mannitol Biosynthetic Genes in W/S-Clade Species
Mannitol production in appreciable amounts, an unusual feature in yeasts, was previously detected in at least one W/S-clade species, C. magnoliae (Baek et al., 2003; Lee et al., 2003). To find out whether mannitol production is a widespread trait in the W/S clade, we quantified mannitol in the culture supernatants of representatives of five W/S-clade species, namely C. magnoliae, St. bacillaris, St. bombicola, W. domercqiae and W. versatilis, cultivated in rich medium containing either 10% glucose or 10% fructose as carbon and energy source. We found that all species but one produced variable amounts of mannitol in addition to ethanol and glycerol, and that more mannitol was produced from fructose than from glucose (Figure 1A). In St. bacillaris neither of the two strains tested (PYCC 3044 and PYCC 6282) produced mannitol; in this species glycerol, erythritol and ethanol were produced instead.
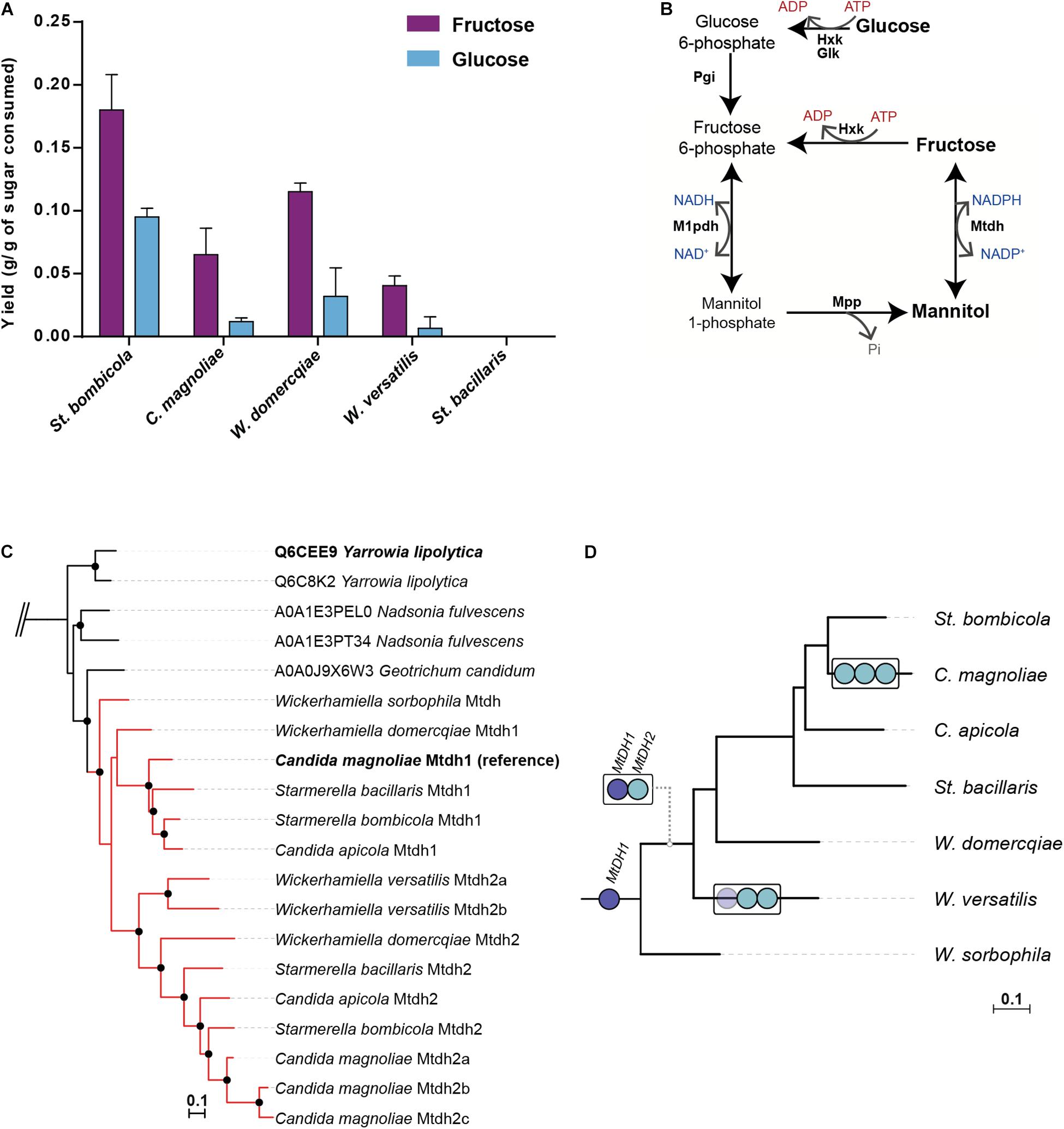
Figure 1. Mannitol production and phylogenetic analyses of mannitol-related genes in W/S-clade species. (A) Mannitol yields determined after 72–150 h of growth on YP medium supplemented with 10% (w/v) glucose or 10% (w/v) fructose at 30°C in several W/S-clade species (Starmerella bombicola, Candida magnoliae, Starmerella bacillaris, Wickerhamiella domercqiae and Wickerhamiella versatilis). Assays were performed in duplicate and the error bars represent the standard deviation. (B) Mannitol biosynthetic pathways in Fungi as described by Hult et al. (1980). Glk – Glucokinase, Hxk – Hexokinase, Pgi – Phosphoglucose isomerase, Mtdh – mannitol dehydrogenase, Mpp – mannitol-1-phosphate phosphatase, M1pdh – mannitol-1-phosphate dehydrogenase. (C) Pruned Maximum Likelihood (ML) phylogeny of Mtdh-like proteins using the top 750 hits (UniprotKB database) to St. bombicola Mtdh1. Branches with support higher than 95% are indicated by black dots. W/S-clade proteins are shown in red. Complete phylogeny can be accessed in Figshare 10.6084/m9.figshare.9959693. (D) Schematic representation of the phylogenetic relationships between W/S-clade species based on Gonçalves et al. (2018). MtDH genes are represented by blue circles (dark blue- MtDH1, light blue- MtDH2). The duplication events are represented by white boxes in the respective branches in which they were assumed to have occurred. In W. versatilis, MtDH1 seems to have been lost (faded dark blue circle).
In fungi, mannitol can be produced from fructose through two distinct pathways. The first, usually found in filamentous fungi (Solomon et al., 2007), involves the NADH dependent conversion of fructose-6-phosphate (fructose-6-P) to mannitol-1-phosphate (mannitol-1P), followed by dephosphorylation to yield mannitol. The second, previously shown to operate in the oleaginous yeast Y. lipolytica (Napora et al., 2013), involves the direct conversion of fructose to mannitol through an NADPH dependent mannitol dehydrogenase (Figure 1B). The two pathways may coexist, in which case they form a cycle that may interconvert NAD(H) and NADP(H) (Hult et al., 1980). The fact that W/S-clade species produce mannitol when glucose is the only carbon and energy source, suggests that the pathway involving the conversion of fructose-6-P to mannitol-1P is operating in these yeasts, since the direct conversion pathway is specific for fructose. This specificity was demonstrated for the purified mannitol dehydrogenase from W/S-clade species C. magnoliae (Lee et al., 2003).
To obtain a first insight into the pathway(s) involved in mannitol production in the W/S clade, the genomes of six W/S-clade species were surveyed for the presence of genes likely to encode components of the mannitol biosynthetic pathways. Hence, tBLASTx searches were performed using available fungal protein sequences for the various components of the mannitol biosynthetic pathways as queries. The results (Supplementary Table S2) showed that genes encoding mannitol-1P dehydrogenase and mannitol-1P phosphatase seem to be absent in all genomes examined, leading to the unanticipated conclusion that in the W/S clade biosynthesis of mannitol from glucose most probably does not proceed through the pathway involving these two enzymes. On the contrary, homologs of the Y. lipolytica mannitol dehydrogenase (YlSDR) gene responsible for the direct conversion of fructose to mannitol (Napora et al., 2013; Dulermo et al., 2015) were readily found in all genomes examined. Among the genes identified was the previously characterized MtDH1 from C. magnoliae (Lee et al., 2003). In St. bombicola, we also found next to MtDH1 gene (Supplementary Figure S1), a second, very similar gene (encoding a protein with ∼63% similarity to Mtdh1) that we named MtDH2.
To shed light on the evolutionary origin of the Mtdh-like proteins identified in W/S-clade genomes, a ML phylogeny was constructed including all the Mtdh proteins identified in W/S-clade genomes as well as the 750 best BLASTp hits in UniprotKB (reference proteomes database) obtained using St. bombicola Mtdh1 as a bait (Figure 1C). This phylogeny suggests that Mtdh1 and Mtdh2 are derived from a duplication event that took place in a common ancestor of the W/S clade (Figure 1D). Each examined species possesses a single gene of each type, with the exception of C. magnoliae, which possesses three MtDH2-like genes and W. versatilis that lacks the MtDH1 gene. Wickerhamiella sorbophila (formerly Candida infanticola) does not exhibit the duplication (Figures 1C,D).
Direct Conversion of Fructose to Mannitol in W/S-Clade Yeasts
Comparative genomic analyses showed in the previous section that W/S-clade genomes encoded two (or more) putative paralogous mannitol dehydrogenases, which potentially catalyze the interconversion between fructose and mannitol. To consubstantiate this finding and demonstrate that mannitol is produced directly from fructose in W/S-clade species, we assayed Mtdh activity in cell-free extracts of the species under study. In line with the absence of mannitol in culture supernatants, no Mtdh activity was detected in St. bacillaris irrespective of the cofactor (NADH or NADPH) used, although the MtDH1 and MtDH2 genes are also present in this species (Figure 1C and Supplementary Figure S1). For all other W/S-clade species, NADPH-dependent Mtdh activity was readily detected (Supplementary Figure S2), while no activity could be measured when NADH was supplied as a cofactor. St. bacillaris does not produce mannitol but rather glycerol and erythritol. Interestingly, in St. bacillaris glycerol synthesis involves NADP+ recycling since it requires NADPH as cofactor (Supplementary Figure S2B) while in the other W/S-clade species this cofactor was apparently not used.
Genetic Dissection of Mannitol Production in St. bombicola
In order to find out which of the two MtDH genes, if any, was responsible for mannitol production in W/S-clade yeasts, single and double MtDH deletion mutants were constructed in the genetically amenable species St. bombicola, which encodes one Mtdh1 and one Mtdh2. As shown in Figure 2, in the mtdh1Δ and mtdh1Δmtdh2Δ mutants no mannitol was detected in culture supernatants (Figure 2A) and no mannitol dehydrogenase activity could be measured in cell free extracts of mtdh1Δ (Figure 2C), suggesting that Mtdh1 is the main enzyme responsible for mannitol synthesis in this yeast. This agrees with the fact that mannitol biosynthesis seems to be unaffected in the mtdh2Δ mutant (Figure 2A).
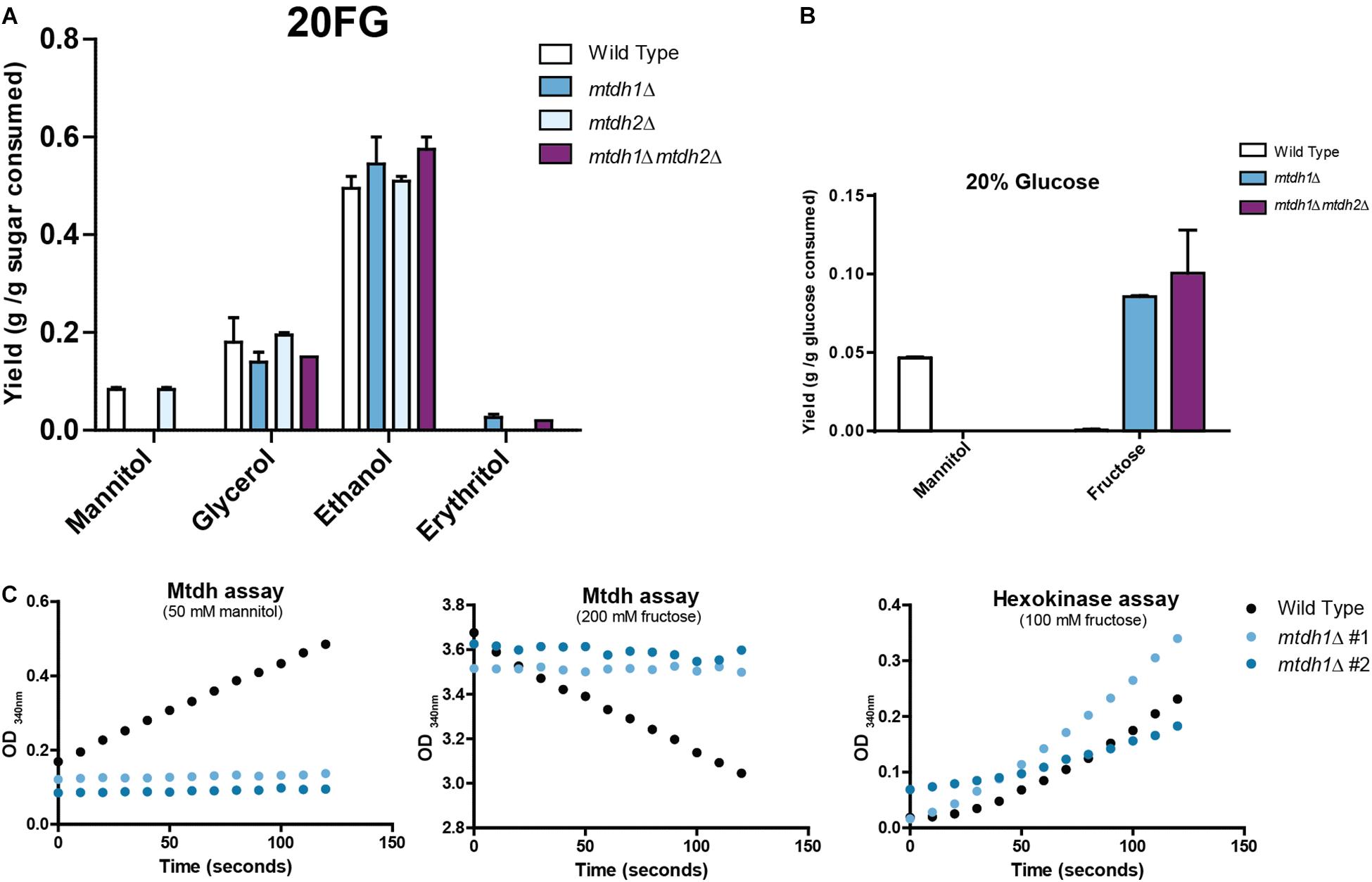
Figure 2. Metabolite production and mannitol dehydrogenase (Mtdh) activities in St. bombicola wild type and deletion mutants. (A) Metabolite production in St. bombicola wild type and deletion mutants (mtdh1Δ, mtdh2Δ, mtdh1Δmtdh2Δ) cultivated in YP supplemented with 10% (w/v) glucose and 10% (w/v) fructose (20FG) at 30°C for 76 h. (B) Mannitol and fructose production in St. bombicola wild type, mtdh1Δ, mtdh1Δmtdh2Δ cultivated in YP medium supplemented with 20% (w/v) glucose at 30°C for 76 h. (C) Mtdh activities in cell-free extracts of wild type St. bombicola (black dotted line) and mtdh1Δ (blue lines represent two biological replicates). The hexokinase assay served as a control for the quality of the extracts.
Notably, mannitol production from glucose is also hampered in the mutants lacking MtDH1 (Figure 2B). This result can only be explained by the existence of a yet unknown pathway mediating conversion of glucose to mannitol. Our genetic evidence suggests that such a pathway must include the reaction catalyzed by Mtdh1. Corroborating this, we found considerable amounts of fructose (∼10 g/L; Figure 2B) in the culture supernatants of mtdh1Δ and mtdh1Δmtdh2Δ mutants cultivated on glucose. Therefore, we conclude that fructose is an intermediate in a novel pathway responsible for the biosynthesis of mannitol from glucose in St. bombicola and that fructose accumulates and is excreted when the fructose —> mannitol conversion is genetically prevented.
Conversion of Glucose to Fructose in W/S-Clade Yeasts
To examine whether the observed conversion of glucose to fructose was likely to be occurring as well in wild type St. bombicola, we examined the culture supernatants of the wild type strain for the presence of fructose. Although in much lower amounts than in the mtdh1Δ mutant (∼0.24 g/L in the wild type vs. ∼10 g/L in the mutant), fructose could also be detected in the supernatants of the wild type strain cultivated on 200 g/L glucose. The same was observed for wild type W. versatilis (1 g/L fructose detected by HPLC after ∼200 h of growth at 30°C), another W/S-clade species that produces mannitol (Figure 1A), showing that the glucose to fructose conversion was not an artifact of the St. bombicola mutant. Instead, this reaction seems to be part of a new pathway likely shared by W/S-clade yeasts that produce mannitol from glucose.
Conversion of glucose to fructose has not been described in yeasts so far but many bacteria and a few fungal species conduct this direct conversion using a glucose isomerase, an enzyme of exceptional biotechnological interest (Bhosale et al., 1996). Examples in fungi are found in species belonging to the genera Piromyces (Lee et al., 2017), Aspergillus (Sayyed et al., 2010) and Penicillium (Kathiresan and Manivannan, 2006). We searched for the presence of a glucose isomerase in the St. bombicola genome and the genomes of other W/S-clade species using fungal and bacterial glucose isomerases as queries in tBLASTx searches (AJ249909.1 and FJ858195.1, respectively), but no candidate genes could be found (e-value >1e–3, Supplementary Figure S3). Importantly, we also failed to detect glucose to fructose conversion in cell free extracts.
Since no evidence could be found for the presence of a gene encoding a glucose isomerase, we searched for other known biochemical routes that could potentially be used by St. bombicola to produce fructose from glucose. A two-step conversion can be envisioned involving an aldose reductase to convert glucose to sorbitol and then a sorbitol dehydrogenase to convert sorbitol to fructose (Supplementary Figure S3A), a pathway that has been previously described (Muntz and Carroll, 1960). A candidate gene for a sorbitol dehydrogenase could be readily retrieved from the genome of St. bombicola by tBLASTx using a sorbitol dehydrogenase sequence from Y. lipolytica (YALI0E12463, XM_503864.1) while the results for the aldose reductase (XM_502540.1) were not clear (Supplementary Figure S3B). Nevertheless, disruption of the sorbitol dehydrogenase (SOR1) gene in St. bombicola was performed and we observed that this mutant was unable to grow on sorbitol as carbon source confirming the presumed function of the St. bombicola Sor1 protein. However, deletion of SOR1 in the mtdh1Δ mutant background (mtdh1Δsor1Δ) did not affect glucose conversion to fructose, since similar levels of fructose were measured in the supernatants of the mtdh1Δ and mtdh1Δsor1Δ mutants (∼10 g/L and ∼8 g/L, respectively) leading to the conclusion that this pathway is not involved in the conversion. Finally, we considered the possibility that fructose might be generated by dephosphorylation of the glycolytic intermediate fructose-6-phosphate (fructose-6P). Very few references are found in the literature to fructose-6P phosphatases in fungi but the activity has been studied in connection with mannitol metabolism in mushrooms (Kulkarni, 1990). Since genes that can be used as baits in tBLASTx searches are unavailable, we decided to use 13C-labeled glucose and NMR analysis of end-products to obtain definite evidence for the occurrence of this novel metabolic pathway.
A Fructose-6-Phosphate Phosphatase Is the Key to Mannitol Production From Glucose
To trace the metabolic fate of glucose in the mtdh1Δmtdh2Δ mutant, where considerable accumulation of fructose in the growth medium was observed, we first established that resting cells of this mutant, pre-grown on glucose and resuspended in phosphate buffer, produced ∼3 g/L of fructose when given a glucose pulse (∼50 mM). We subsequently performed similar experiments but using pulses of 13C-glucose labeled on C2 ([2-13C]glucose) or labeled on C1 ([1-13C]glucose) to allow for tracing the metabolic fate of glucose by NMR. The label from [2-13C]glucose was retrieved mainly in fructose and ethanol (Figure 3A and Supplementary Table S3); minor amounts of [2-13C]pyruvate, [1-13C]acetate, [2-13C]glycerol and [2-13C]dihydroxyacetone were also detected (Supplementary Table S3). If a direct conversion of glucose into fructose would occur, [1-13C]glucose and [2-13C]glucose would yield exclusively [1-13C]fructose and [2-13C]fructose, respectively. However, the fructose pool contained a substantial fraction (∼10%) of [6-13C]fructose derived from [1-13C]glucose and [5-13C]fructose from [2-13C]glucose (Figure 3A and Supplementary Table S3). This label diversion implies the occurrence of a reversed flux from the triose phosphate pool toward fructose-1,6-bisphosphate through aldolase (Supplementary Figure S4) and has been amply illustrated in early in vivo 13C-NMR studies of central metabolism in Lactococcus lactis (Neves et al., 1999). This reversed flux is especially apparent when there is a metabolic constraint such as that created by a deficiency in activities that are key for the fulfillment of the redox balance (Neves et al., 2000). In the case of St. bombicola the observed label diversion unequivocally shows that there is fructose-6P phosphatase activity in addition to the gluconeogenic enzyme, fructose-bisphosphatase; unfortunately, we were unable to detect such activity in crude cell-free extracts of the mtdh1Δmtdh2Δ using a standard methodology for detection of inorganic phosphate (Ames, 1966).
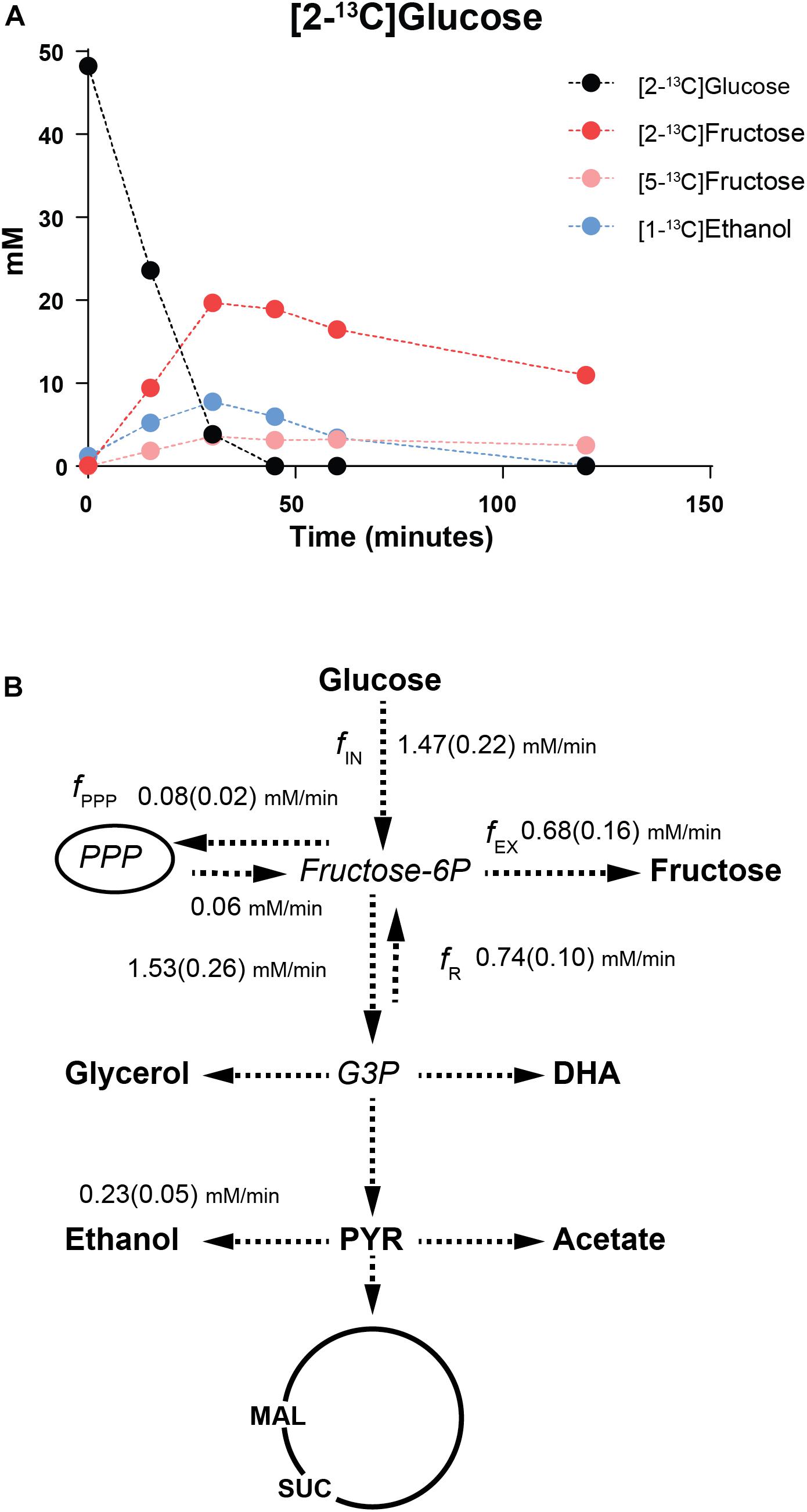
Figure 3. 13C-NMR analyses of [2-13C]glucose metabolism in St. bombicola mtdh1Δmtdh2Δ mutant. (A) Time course of 13C-labeled glucose consumption and product formation by resting cell suspensions of the St. bombicola mtdh1Δmtdh2Δ mutant. End products were quantified by 13C-NMR in supernatants after a pulse (at time zero) of [2-13C]glucose to a final concentration of approximately 50 mM. A comprehensive list of labeled end-product concentrations for this and similar assays is shown in Supplementary Table S3. (B) Metabolic fluxes deduced from experiments with 13C-labeled glucose. Observed metabolites are shown in bold (DHA: dihydroxyacetone; PYR: pyruvate; MAL: malate; SUC: succinate) and inferred intermediates in italic (G3P: glyceraldehyde-3-phosphate). PPP represents the reactions of the pentose phosphate pathway. Each conversion, shown by dashed lines, may involve multiple steps. Standard deviations are shown in brackets.
While these results show that a phosphatase is implicated in the conversion of glucose to fructose in St. bombicola, they do not exclude the involvement of an isomerase (see Supplementary Figure S4). To investigate this, we constructed a St. bombicola mutant in which the hexokinase (HXK1) and glucokinase (GLK1) genes were disrupted, completely precluding hexose phosphorylation. We envisaged that in this mutant, glucose to fructose conversion could still take place only if an isomerase was involved, but not if a fructose-6P phosphatase was required (Supplementary Figure S5A). When the hxk1Δglk1Δ mutant was grown in 10 g/L glycerol and 10 g/L fructose, mannitol was normally produced yielding ∼10 g/L (Supplementary Figure S5B). On the other hand, when the mutant was grown in a mixture of glycerol and glucose no mannitol or fructose production and no glucose consumption were observed (Supplementary Figure S5B), indicating that the inability to produce fructose-6P impedes mannitol formation from glucose and that the direct conversion of glucose to fructose, if present, is negligible and undetectable using this methodology. Altogether, our results led us to postulate that in St. bombicola glucose is converted into fructose via the intermediate metabolites glucose-6P and fructose-6P and the action of a yet unknown fructose-6P phosphatase.
On the basis of the 13C-labeling patterns observed on the fructose pool the relevant carbon fluxes around the fructose-6P node were estimated (Figure 3B; for details on flux analysis see section “Materials and Methods”). Glucose was consumed at a rate of 1.47 ± 0.22 mM/min while fructose was produced at a rate of 0.68 ± 0.16 mM/min. The flux from fructose-6P toward the Pentose Phosphate Pathway (fPPP) was small, only 0.08 mM/min. On the other hand, the reversed flux from the triose phosphate pool to fructose-6P was about half (48%) of the forward glycolytic flux. It is worth noting that there is a substantial flux from fructose-6P to fructose, in fact amounting to 86% of the net flux from fructose-6P to glycolysis.
Mannitol Production Contributes to Fructophily
As shown in the previous sections, mannitol production seems to be a key reaction in the metabolism of W/S-clade yeasts, which is best illustrated by the diversion of fructose-6P to mannitol production during growth on glucose. Mannitol production from glucose demands ATP expenditure but this is not the case when fructose is the substrate (Figure 1B). This is in line with the higher mannitol yield obtained from fructose when compared to the mannitol yield when glucose is used as the sole carbon source (Figure 1A) and could explain why fructose is preferred over glucose in these yeasts. Hence, we set out to investigate whether deletion of the MtDH1 gene affected fructophily, by cultivating the mutant under the conditions where fructophily is usually observed (YP medium supplemented with 10% glucose + 10% fructose; henceforth referred to as 20FG). The results, shown in Figure 4A revealed an effect of the MtDH1 gene deletion on the consumption rates of both fructose and glucose, decreasing the first and increasing the latter, which results in attenuation of the preference for fructose. In the culture supernatants of the two mutants that fail to produce mannitol (mtdh1Δ and mtdh1Δ mtdh2Δ), small amounts of erythritol were detected, while ethanol and glycerol yields on sugar remained virtually unaltered (Figure 2A). Since erythritol production regenerates NADP+, this result suggests that abolition of mannitol synthesis causes a redox imbalance at least partly compensated by an increase in erythritol production.
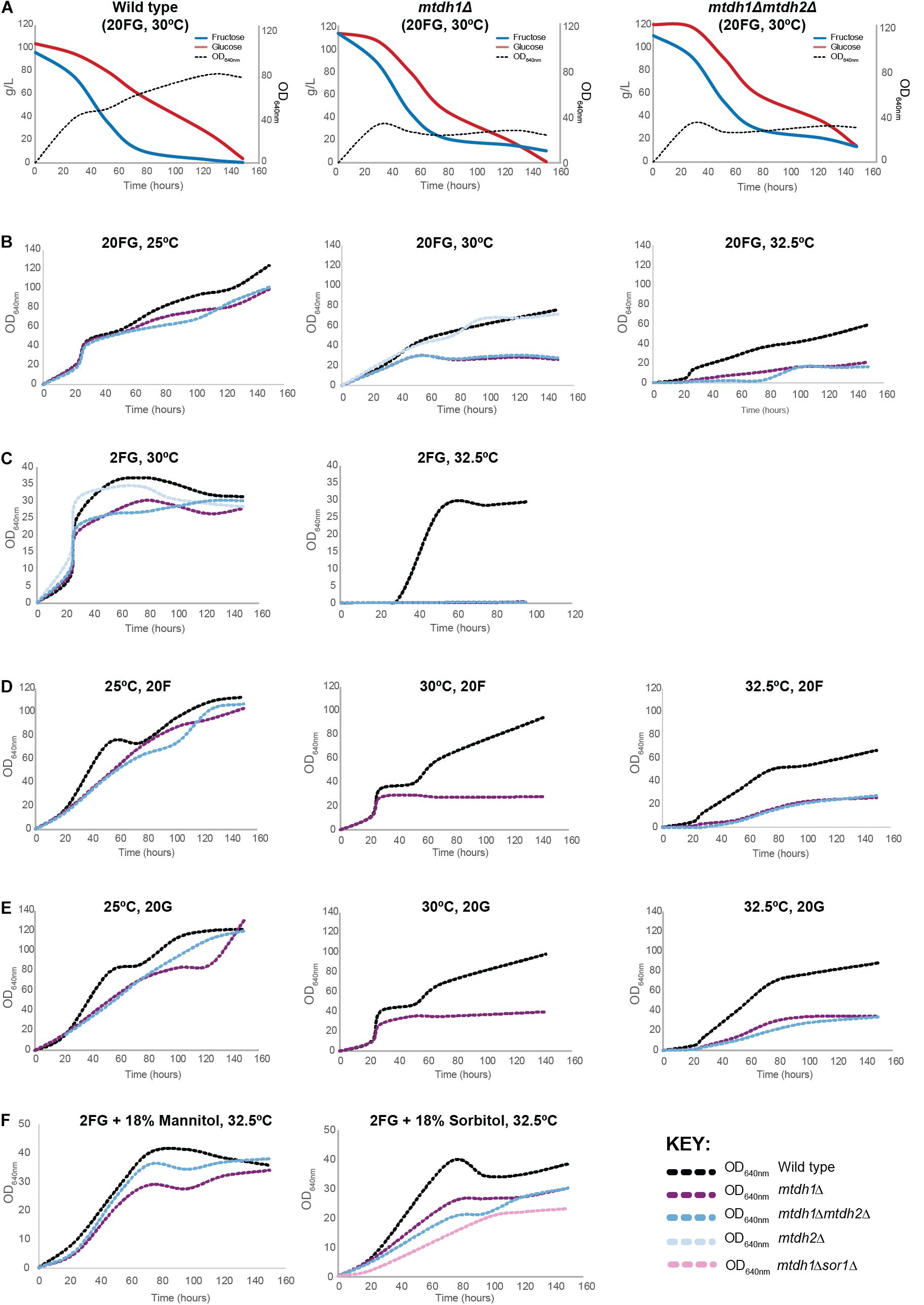
Figure 4. Effect of MtDH deletion on fructophily and on biomass yield at different growth temperatures. (A) Sugar consumption profiles of St. bombicola wild type, mtdh1Δ and mtdh1Δmtdh2Δ mutants. Strains were cultivated in YP supplemented with 10% (w/v) glucose and 10% (w/v) fructose (20FG) for 150 h at 30°C. Experiments were performed with two biological replicates. (B) Growth of wild type, mtdh1Δ, mtdh2Δ and mtdh1Δmtdh2Δ in YP supplemented with 10% (w/v) glucose and 10% (w/v) fructose (20FG) for 150 h at different temperatures: 25, 30, and 32.5°C. (C) Growth of St. bombicola wild type, mtdh1Δ and mtdh1Δmtdh2Δ in YP supplemented with 1% (w/v) glucose and 1% (w/v) fructose (2FG) at 30 and 32.5°C. (D,E) Growth of St. bombicola wild type, mtdh1Δ and mtdh1Δmtdh2Δ in YP supplemented with 20% (w/v) fructose (20F) (D) or 20% (w/v) glucose (20G) (E) for 150 h at 25, 30, and 32.5°C. (F) Growth of St. bombicola wild type, mtdh1Δ, mtdh1Δmtdh2Δ in (2FG) supplemented with 18% (w/v) mannitol (left) or 18% (w/v) sorbitol (right) at 32.5°C. St. bombicola mtdh1Δsor1Δ was only grown in 2FG supplemented with 18% (w/v) sorbitol. Raw data can be found in Supplementary Data S1.
A Possible Role for Mannitol in Thermal Protection
Loss of the ability to synthesize mannitol was accompanied by a drop in the biomass yield on sugar, when compared with the wild type (Figure 4A). We first hypothesized that this decrease in biomass yield in the mutants incapable of producing mannitol could be due to a role of mannitol as a compatible solute, required to cope with osmotic stress in the high sugar concentration medium. In line with this possibility, we observed that the mtdh1Δ and mtdh1Δ mtdh2Δ mutants grew as well as the wt in medium containing 2% sugar (1% glucose + 1% fructose; henceforth referred to as 2FG), attaining similar cell densities (Figure 4C). However, when we grew the mutants in low sugar concentrations (2FG) but at 32.5°C instead of the previous 30°C, the mtdh1Δ and mtdh1Δmtdh2Δ mutants did not grow (Figure 4C), suggesting that mannitol might rather play a role in adaptation to thermal stress.
To assess the possibility that mannitol might function as a thermal protector in St. bombicola, we revisited the performance of the single and double mtdh mutants in high sugar medium (20FG), at three different temperatures. The results, shown in Figure 4B demonstrate that also in 20FG medium, the growth defect is more pronounced at 32.5°C than at 30°C, although in this medium the mutants are still capable of growing at 32.5°C. Since at 25°C, only a mild effect of the inability to synthesize mannitol on growth was observed (Figure 4B) irrespective of the osmolarity of the growth medium it seems unlikely that mannitol plays a major role in osmoprotection. The effects produced by the increase in temperature were not specific for one of the sugars in particular (Figures 4D,E).
Next, we examined whether mannitol production increased with temperature in the wild type, which we anticipated should occur if it has a thermo-protective role. Relative internal (in cell-free extracts) and external mannitol concentrations were measured at different temperatures. An increase in extracellular mannitol concentration was readily observed with increasing temperatures (Supplementary Figure S6A), which does not happen for other fermentation products like ethanol and glycerol (Supplementary Figure S6B). We estimated intracellular mannitol concentrations per cell to vary between 5 and 40 mM, which might be sufficient to fulfill a role in thermal protection, but we did not see a clear increase in intracellular concentrations (Supplementary Figures S6C,D). Nevertheless, this represents a small fraction (approximately 1–5%) of the total amount of mannitol produced.
Starmerella bombicola does not grow well on mannitol, requiring up to 1 week of lag phase, but since it does eventually grow, this means that it possesses a transport system that enables it to take up mannitol. We reasoned that if mannitol itself, and not (only) the mannitol producing reaction was important for growth of the mtdh1Δ mutants at high temperatures, we might be able to ameliorate the growth defect by adding mannitol to the culture medium. In fact, when we added 18% (w/v) mannitol to the 2FG medium in which the mutants fail to grow at 32.5°C, the MtDH1 mutants were able to achieve near wild type cell densities, albeit somewhat slower (Figure 4F). To find out whether this might also hold true for other polyols, we performed the same experiment using sorbitol, with similar results (Figure 4F). Moreover, when we used a mutant rendered unable to use sorbitol as carbon and energy source because of the deletion of a gene encoding an essential enzyme of sorbitol metabolism (SOR1) and lacking also MtDH1, growth was still observed (Figure 4F), showing that growth rescue is not dependent on sorbitol metabolization.
Discussion
We showed recently that fructophily arose in yeasts in the W/S clade, a lineage that comprises yeast species sharing a recent common ancestor that lost the ability to conduct alcoholic fermentation. This central metabolic pathway was later reacquired by incorporation of an alcohol dehydrogenase of bacterial origin (Gonçalves et al., 2018). We also showed previously that the molecular basis for fructophily was established in a W/S-clade ancestor through the horizontal acquisition of a high capacity specific fructose transporter, Ffz1, from filamentous fungi (Gonçalves et al., 2016). Hence, two events with impact on metabolism, both unique in the evolutionary history of yeasts, took place in the same lineage and as far as can be currently judged, at not too distant a moment in evolution from each other. One pressing question arising from this observation is whether the two events might be somehow related. In order to explore this possibility, we sought to gather additional information on the fate of fructose in fructophilic yeasts, once it is taken up by the Ffz1 transporter. Here we showed that a significant amount of fructose is converted to mannitol in most W/S-clade yeasts tested.
Role of the Mannitol Biosynthetic Pathway in the W/S-Clade
Contrary to what was suggested previously for the yeast Y. lipolytica, our evidence does not support a role for mannitol as transient carbon storage (Dulermo et al., 2015). The fact that most of the mannitol produced is excreted to the growth medium and not reutilized, and that the mannitol biosynthesis reaction is associated with cofactor recycling, rather suggests that mannitol is mainly a fermentation product and that its production has a role in redox balance. Consistent with this, we found that the pathway for mannitol production consists in a direct conversion of fructose to mannitol with NADP+ regeneration, mediated by an NADPH-dependent mannitol dehydrogenase and that all W/S-clade genomes examined lacked genes encoding the enzymes involved in the alternative pathway that converts fructose-6P to mannitol-1P. In St. bombicola, we obtained genetic evidence for the involvement of a mannitol dehydrogenase only, since elimination of MtDH1 obliterates detectable mannitol production, notably also when glucose is the carbon source. Most importantly, we demonstrated that in spite of the absence of the mannitol-1P dehydrogenase pathway, glucose is also efficiently converted to mannitol through a novel pathway involving a fructose-6P phosphatase but not a glucose isomerase.
The role of a second gene, presumably paralogous to MtDH1 could not be established with certainty. This gene, named MtDH2 is phylogenetically closely related to MtDH1 but its deletion has no measurable impact on the amount of mannitol produced. However, it seems likely that MtDH2 has a role in mannitol metabolism in at least some W/S-clade species because W. versatilis lacks the MtDH1 type of gene, possessing only several copies of the MtDH2 type, but still produces mannitol. On the other hand, St. bacillaris has intact MtDH1 and MtDH2 genes but did not produce mannitol under the conditions tested, rather directing an important part of the carbon flux to glycerol. Therefore, either mannitol production occurs in this species under conditions distinct from those presently tested or these genes acquired a different function in St. bacillaris.
The fact that the production of the main polyol (either mannitol or glycerol) seems to be associated in W/S-clade species with NADP+ recycling suggests that their production plays a role in dealing with excess NADPH, which may result from the Pentose Phosphate Pathway (PPP) carrying a higher relative flux than for example in S. cerevisiae, where it seems to be finely tuned to the needs of NADPH for biosynthesis (Frick and Wittmann, 2005). In the oleaginous yeast Y. lipolytica, which is more closely related to the W/S clade, this pathway was shown to be overactive, providing also the NADPH required for lipid production (Wasylenko et al., 2015). The fact that erythritol was present in measurable amounts in the culture supernatant of the mtdh mutants but not of the wt, suggests a compensation as is normally observed in glycerol synthesis when ethanol production is impaired in yeasts (Drewke et al., 1990; Gonçalves et al., 2018).
Characterization of St. bombicola mutants unable to produce mannitol also suggested a role for mannitol as thermo protector, which we showed could be taken over by a similar compound as sorbitol. The growth rescue by external polyols is only partial, probably reflecting that redox balance is not optimal under these conditions. The effect of temperature on the growth defect phenotype of the mtdh mutants as well as the increase in mannitol production at higher temperatures consubstantiate the role of mannitol as thermo protector. Similar substances, like trehalose have been suggested to fulfill a thermo-protective role in S. cerevisiae by stabilizing the membrane when present on both sides (Magalhães et al., 2018). The fact that the mtdh1Δ mutants are capable of some growth at 32.5°C in 20FG medium but not in 2FG medium could suggest that the sugars, which are structurally similar to sugar alcohols, might contribute to ameliorate the thermo sensitive phenotype when present at higher concentrations (Corry, 1976; Henle et al., 1985).
A New Pathway for the Conversion of Glucose Into Fructose Ensures Mannitol Biosynthesis in the Absence of Fructose
Taken together our findings point to mannitol playing an important role in central carbon metabolism in St. bombicola and also a possible role in stress protection (Wyatt et al., 2013), suggesting that fructose is preferred by this and other W/S-clade yeasts because it can be converted directly to mannitol. Our most striking finding that greatly emphasizes the importance of mannitol production for this yeast is the fact that it features a new pathway to convert glucose to fructose capable of carrying a considerable flux, therefore being able to operate the mannitol dehydrogenase reaction efficiently also when glucose is the only sugar available. In all documented cases so far in fungi, mannitol production from glucose relied on the mannitol-1P pathway (Solomon et al., 2007). As far as we could investigate taking advantage of the available W/S-clade genomes, the new pathway here reported is probably widespread in the W/S clade, a lineage that comprises presently close to 10% of all known yeast species, because all species examined are capable of producing mannitol from glucose and the only pathway for mannitol production seems to involve the fructose-specific Mtdh.
A Possible Link Between Mannitol Metabolism and Evolution of Alcoholic Fermentation
We propose a model (Figure 5) in which the advantage of preferential utilization of fructose would have arisen first in a background of lack of, or inefficient alcoholic fermentation, similarly to what is currently observed in fructophilic bacteria (Maeno et al., 2016, 2019). The advantage of fructose under these circumstances would be likewise to provide a means to alleviate redox imbalance by facilitating cofactor recycling through mannitol synthesis, which concomitantly oxidizes NADPH. Although alcoholic fermentation in yeasts in general oxidizes only NADH, extant W/S-clade species have alcohol dehydrogenases that operate using both NADH and NADPH as cofactors (Gonçalves et al., 2018). The likelihood of the proposed model depends on whether fructose reduction to mannitol was capable of minimizing redox balance problems resulting from loss of alcoholic fermentation. This would require either that the cofactors involved in both processes were the same in the W/S ancestor that lost alcoholic fermentation or that mechanisms existed for the equilibration of phosphorylated and unphosphorylated nicotinamide adenine cofactors. Such mechanisms have not been identified in extant yeast species (Bruinenberg et al., 1983). In this model, mannitol may have acquired later a role in stress protection in addition to its role in redox balancing. Evidence for the ancestral importance of mannitol synthesis, in addition to the evolutionary novelty of the pathway mediating glucose to fructose conversion, resides in the duplication of the mannitol dehydrogenase gene, which occurred in the W/S lineage. After efficient alcoholic fermentation was again in place, through acquisition of a bacterial gene and recruitment of the Aro10 decarboxylase for alcoholic fermentation (Gonçalves et al., 2018), the contribution of mannitol biosynthesis to redox balance may have become less important, possibly allowing one of the paralogous genes, MtDH2, to acquire a different main function. In conclusion, the surprisingly high flux through the novel pathway involving a fructose-6P phosphatase further underscores the link between fructophily and mannitol synthesis, since it ensures a supply of fructose even when it is not available in the environment, apparently solely for the purpose of mannitol production.
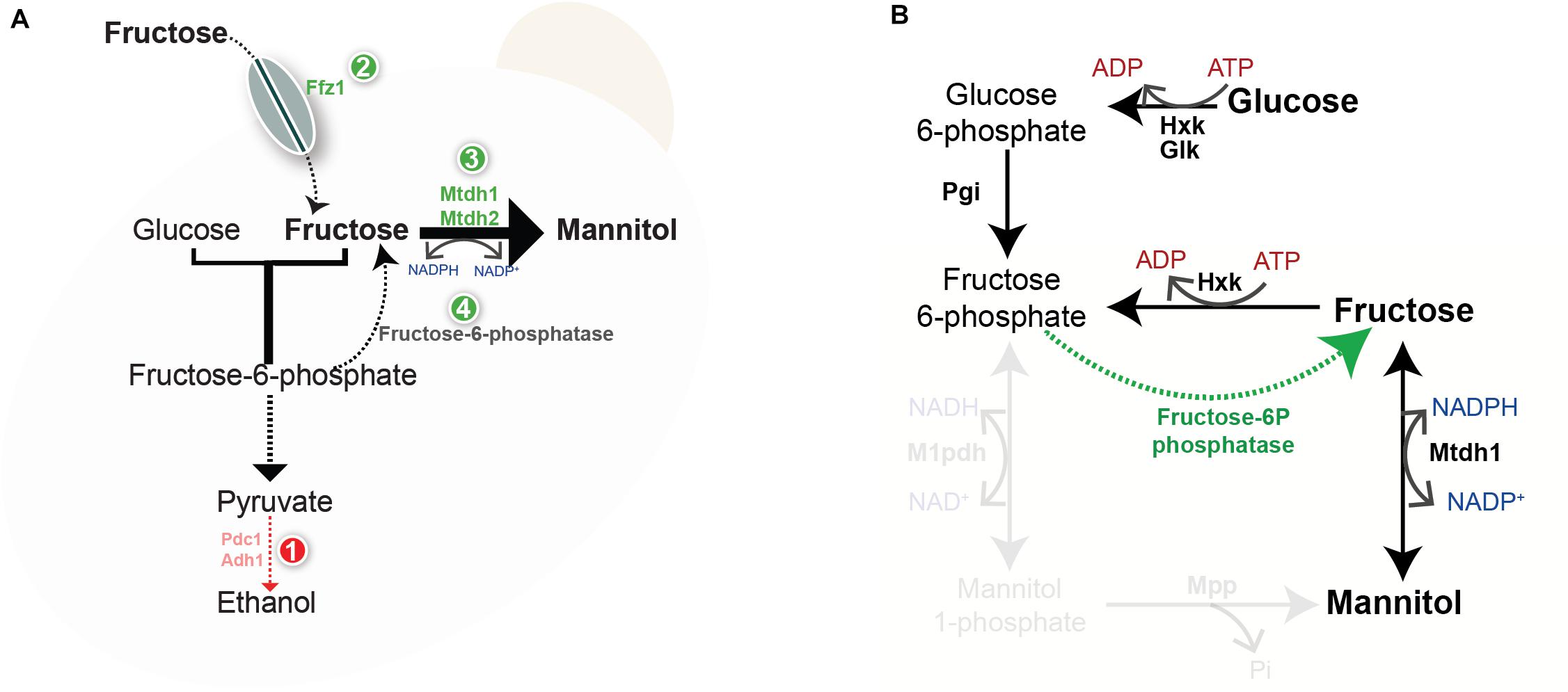
Figure 5. Working model for the evolution of mannitol metabolism. (A) Lack of or inefficient alcoholic fermentation (1) causes redox constraints that can be partly alleviated by oxidized cofactor recycling by the mannitol dehydrogenase reaction. Acquisition of Ffz1 (2) and duplication of the MtDH gene (3) may have supported an increase in the flux toward mannitol production. In order to harvest the advantages of mannitol production also when glucose is the sole carbon and energy source a novel pathway (4) emerged converting fructose-6-P to fructose. (B) Novel pathway mediating the production of mannitol from glucose in W/S-clade yeasts; Hxk- Hexokinase, Glk- Glucokinase; Pgi- Phosphoglucose isomerase, Mtdh1- Mannitol dehydrogenase. Reactions involving M1pdh and Mpp are faded to indicate that they are absent in W/S-clade species.
Data Availability Statement
Publicly available datasets were analyzed in this study. This data can be found here: Bioproject PRJNA416493.
Author Contributions
PG, HS, CG, MS-O, and LG designed the research. CG, CF, LG, and ML performed the experiments and analyzed the data. DT built the metabolic model and calculated the fluxes. PG wrote the manuscript with the assistance from HS, CG, and MS-O. DT, ML, and LG proofread the manuscript. PG and HS were responsible for funding acquisition. All authors read and approved the final manuscript.
Conflict of Interest
The authors declare that the research was conducted in the absence of any commercial or financial relationships that could be construed as a potential conflict of interest.
Funding
This work was supported by the Applied Molecular Biosciences Unit – UCIBIO which is financed by national funds from FCT/MCTES (UID/Multi/04378/2019), by grant FructYEAST – LISBOA-01-0145-FEDER-029529/PTDC/BIA-MIC/29529/2017 from FCT/MCTES, Portugal (https://www.fct.pt/) and by project LISBOA-01-0145-FEDER-007660 (Microbiologia Molecular, Estrutural e Celular) funded by FEDER through COMPETE2020 – POCI and by national funds through FCT. The NMR data was acquired at CERMAX (Centro de Ressonância Magnética António Xavier) ITQB-NOVA, Oeiras, Portugal with equipment funded by FCT, project AAC 01/SAICT/2016. CG, LG, and ML were recipients of grants (SFRH/BD/89489/2012, SFRH/BPD/111100/2015, and SFRH/BPD/102803/2014, respectively) from FCT, Portugal.
Acknowledgments
The authors would like to acknowledge PYCC for providing the strains used for this work and members of the Yeast Genomics Lab for helpful discussions.
Supplementary Material
The Supplementary Material for this article can be found online at: https://www.frontiersin.org/articles/10.3389/fmicb.2019.02510/full#supplementary-material
Footnotes
References
Ames, B. N. (1966). Assay of inorganic phosphate, total phosphate and phosphatases. Methods Enzymol. 8, 115–118.
Baek, H., Song, K. H., Park, S. M., Kim, S. Y., and Hyun, H. H. (2003). Role of glucose in the bioconversion of fructose into mannitol by Candida magnoliae. Biotechnol. Lett. 25, 761–765.
Bhosale, S. H., Rao, M. B., and Deshpande, V. V. (1996). Molecular and industrial aspects of glucose isomerase. Microbiol. Rev. 60, 280–300.
Bruinenberg, P. M., van Dijken, J. P., and Scheffers, W. A. (1983). An enzymic analysis of NADPH production and consumption in Candida utilis. J. Gen. Microbiol. 129, 965–971. doi: 10.1099/00221287-129-4-965
Canto, A., Herrera, C. M., and Rodriguez, R. (2017). Nectar-living yeasts of a tropical host plant community: diversity and effects on community-wide floral nectar traits. PeerJ 5:e3517. doi: 10.7717/peerj.3517
Capella-Gutierrez, S., Silla-Martinez, J. M., and Gabaldon, T. (2009). trimAl: a tool for automated alignment trimming in large-scale phylogenetic analyses. Bioinformatics 25, 1972–1973. doi: 10.1093/bioinformatics/btp348
Chaturvedi, V., Flynn, T., Niehaus, W. G., and Wong, B. (1996). Stress tolerance and pathogenic potential of a mannitol mutant of Cryptococcus neoformans. Microbiology 142, 937–943. doi: 10.1099/00221287-142-4-937
Corry, J. E. (1976). The effect of sugars and polyols on the heat resistance and morphology of osmophilic yeasts. J. Appl. Bacteriol. 40, 269–276.
de Vega, C., Albaladejo, R. G., Guzman, B., Steenhuisen, S. L., Johnson, S. D., Herrera, C. M., et al. (2017). Flowers as a reservoir of yeast diversity: description of Wickerhamiella nectarea f.a. sp. nov., and Wickerhamiella natalensis f.a. sp. nov. from South African flowers and pollinators, and transfer of related Candida species to the genus wickerhamiella as new combinations. FEMS Yeast Res. 17:fox054. doi: 10.1093/femsyr/fox054
Drewke, C., Thielen, J., and Ciriacy, M. (1990). Ethanol formation in adh0 mutants reveals the existence of a novel acetaldehyde-reducing activity in Saccharomyces cerevisiae. J. Bacteriol. 172, 3909–3917. doi: 10.1128/jb.172.7.3909-3917.1990
Dulermo, T., Lazar, Z., Dulermo, R., Rakicka, M., Haddouche, R., and Nicaud, J. M. (2015). Analysis of ATP-citrate lyase and malic enzyme mutants of Yarrowia lipolytica points out the importance of mannitol metabolism in fatty acid synthesis. Biochim. Biophys. Acta 1851, 1107–1117. doi: 10.1016/j.bbalip.2015.04.007
Endo, A., Futagawa-Endo, Y., and Dicks, L. M. (2009). Isolation and characterization of fructophilic lactic acid bacteria from fructose-rich niches. Syst. Appl. Microbiol. 32, 593–600. doi: 10.1016/j.syapm.2009.08.002
Endo, A., Futagawa-Endo, Y., Sakamoto, M., Kitahara, M., and Dicks, L. M. (2010). Lactobacillus florum sp. nov., a fructophilic species isolated from flowers. Int. J. Syst. Evol. Microbiol. 60(Pt 10), 2478–2482. doi: 10.1099/ijs.0.019067-0
Frick, O., and Wittmann, C. (2005). Characterization of the metabolic shift between oxidative and fermentative growth in Saccharomyces cerevisiae by comparative 13C flux analysis. Microb. Cell. Fact. 4:30. doi: 10.1186/1475-2859-4-30
Gonçalves, C., Coelho, M. A., Salema-Oom, M., and Gonçalves, P. (2016). Stepwise functional evolution in a fungal sugar transporter family. Mol. Biol. Evol. 33, 352–366. doi: 10.1093/molbev/msv220
Gonçalves, C., Wisecaver, J. H., Kominek, J., Oom, M. S., Leandro, M. J., Shen, X. X., et al. (2018). Evidence for loss and reacquisition of alcoholic fermentation in a fructophilic yeast lineage. eLife 7:e33034. doi: 10.7554/eLife.33034
Henle, K. J., Monson, T. P., Nagle, W. A., and Moss, A. J. (1985). Heat protection by sugars and sugar analogues. Int. J. Hyperthermia 1, 371–382.
Hult, K., Veide, A., and Gatenbeck, S. (1980). The distribution of the NADPH regenerating mannitol cycle among fungal species. Arch. Microbiol. 128, 253–255.
Kathiresan, K., and Manivannan, S. (2006). Glucose isomerase production by Penicillium fellutanum isolated from mangrove sediment. Trends Appl. Sci. Res. 1, 524–528.
Katoh, K., and Standley, D. M. (2014). MAFFT: iterative refinement and additional methods. Methods Mol. Biol. 1079, 131–146. doi: 10.1007/978-1-62703-646-7_8
Keller, N. P. (2019). Fungal secondary metabolism: regulation, function and drug discovery. Nat. Rev. Microbiol. 17, 167–180. doi: 10.1038/s41579-018-0121-1
Kominek, J., Doering, D. T., Opulente, D. A., Shen, X. X., Zhou, X., DeVirgilio, J., et al. (2019). Eukaryotic acquisition of a bacterial operon. Cell 176, 1356.e10–1366.e10. doi: 10.1016/j.cell.2019.01.034
Kulkarni, R. K. (1990). Mannitol metabolism in Lentinus edodes, the shiitake mushroom. Appl. Environ. Microbiol. 56, 250–253.
Kurtzman, C. P., Fell, J. W., and Boekhout, T. (2011). The Yeasts: A Taxonomic Study, 5th Edn. Amsterdam: Elsevier, 1–2354.
Lachance, M. A., Starmer, W. T., Rosa, C. A., Bowles, J. M., Barker, J. S., and Janzen, D. H. (2001). Biogeography of the yeasts of ephemeral flowers and their insects. FEMS Yeast Res. 1, 1–8. doi: 10.1111/j.1567-1364.2001.tb00007.x
Leandro, M. J., Cabral, S., Prista, C., Loureiro-Dias, M. C., and Sychrova, H. (2014). The high-capacity specific fructose facilitator ZrFfz1 is essential for the fructophilic behavior of Zygosaccharomyces rouxii CBS 732T. Eukaryot. Cell 13, 1371–1379. doi: 10.1128/ec.00137-14
Leandro, M. J., Sychrova, H., Prista, C., and Loureiro-Dias, M. C. (2011). The osmotolerant fructophilic yeast Zygosaccharomyces rouxii employs two plasma-membrane fructose uptake systems belonging to a new family of yeast sugar transporters. Microbiology 157(Pt 2), 601–608. doi: 10.1099/mic.0.044446-0
Lee, J. K., Koo, B. S., Kim, S. Y., and Hyun, H. H. (2003). Purification and characterization of a novel mannitol dehydrogenase from a newly isolated strain of Candida magnoliae. Appl. Environ. Microbiol. 69, 4438–4447. doi: 10.1128/aem.69.8.4438-4447.2003
Lee, M., Rozeboom, H. J., de Waal, P. P., de Jong, R. M., Dudek, H. M., and Janssen, D. B. (2017). Metal dependence of the xylose isomerase from Piromyces sp. E2 explored by activity profiling and protein crystallography. Biochemistry 56, 5991–6005. doi: 10.1021/acs.biochem.7b00777
Maeno, S., Kajikawa, A., Dicks, L., and Endo, A. (2019). Introduction of bifunctional alcohol/acetaldehyde dehydrogenase gene (adhE) in Fructobacillus fructosus settled its fructophilic characteristics. Res. Microbiol. 170, 35–42. doi: 10.1016/j.resmic.2018.09.004
Maeno, S., Tanizawa, Y., Kanesaki, Y., Kubota, E., Kumar, H., Dicks, L., et al. (2016). Genomic characterization of a fructophilic bee symbiont Lactobacillus kunkeei reveals its niche-specific adaptation. Syst. Appl. Microbiol. 39, 516–526. doi: 10.1016/j.syapm.2016.09.006
Magalhães, R. S. S., Popova, B., Braus, G. H., Outeiro, T. F., and Eleutherio, E. C. A. (2018). The trehalose protective mechanism during thermal stress in Saccharomyces cerevisiae: the roles of Ath1 and Agt1. FEMS Yeast Res. 18:foy066. doi: 10.1093/femsyr/foy066
Muntz, J. A., and Carroll, R. E. (1960). A method for converting glucose to fructose. J. Biol. Chem. 235, 1258–1260.
Napora, K., Wrodnigg, T. M., Kosmus, P., Thonhofer, M., Robins, K., and Winkler, M. (2013). Yarrowia lipolytica dehydrogenase/reductase: an enzyme tolerant for lipophilic compounds and carbohydrate substrates. Bioorg. Med. Chem. Lett. 23, 3393–3395. doi: 10.1016/j.bmcl.2013.03.064
Neveling, D. P., Endo, A., and Dicks, L. M. (2012). Fructophilic Lactobacillus kunkeei and Lactobacillus brevis isolated from fresh flowers, bees and bee-hives. Curr. Microbiol. 65, 507–515. doi: 10.1007/s00284-012-0186-4
Neves, A. R., Ramos, A., Nunes, M. C., Kleerebezem, M., Hugenholtz, J., de Vos, W. M., et al. (1999). In vivo nuclear magnetic resonance studies of glycolytic kinetics in Lactococcus lactis. Biotechnol. Bioeng. 64, 200–212.
Neves, A. R., Ramos, A., Shearman, C., Gasson, M. J., Almeida, J. S., and Santos, H. (2000). Metabolic characterization of Lactococcus lactis deficient in lactate dehydrogenase using in vivo 13C-NMR. Eur. J. Biochem. 267, 3859–3868.
Nguyen, L. T., Schmidt, H. A., von Haeseler, A., and Minh, B. Q. (2015). IQ-TREE: a fast and effective stochastic algorithm for estimating maximum-likelihood phylogenies. Mol. Biol. Evol. 32, 268–274. doi: 10.1093/molbev/msu300
Pina, C., Goncalves, P., Prista, C., and Loureiro-Dias, M. C. (2004). Ffz1, a new transporter specific for fructose from Zygosaccharomyces bailii. Microbiology 150(Pt 7), 2429–2433. doi: 10.1099/mic.0.26979-0
Rosa, C. A., and Lachance, M. A. (1998). The yeast genus Starmerella gen. nov. and starmerella bombicola sp. nov., the teleomorph of Candida bombicola (Spencer, Gorin & Tullock) meyer & yarrow. Int. J. Syst. Bacteriol. 48(Pt 4), 1413–1417. doi: 10.1099/00207713-48-4-1413
Savergave, L. S., Gadre, R. V., Vaidya, B. K., and Jogdand, V. V. (2013). Two-stage fermentation process for enhanced mannitol production using Candida magnoliae mutant R9. Bioprocess Biosyst. Eng. 36, 193–203. doi: 10.1007/s00449-012-0775-4
Sayyed, R. Z., Shimpi, G. B., and Chincholkar, S. B. (2010). Constitutive production of extracellular glucose isomerase by an osmophillic Aspergillus sp. under submerged conditions. J. Food Sci. Technol. 47, 496–500. doi: 10.1007/s13197-010-0084-3
Shen, X. X., Opulente, D. A., Kominek, J., Zhou, X., Steenwyk, J. L., Buh, K. V., et al. (2018). Tempo and mode of genome evolution in the budding yeast subphylum. Cell 175, 1533.e20–1545.e20. doi: 10.1016/j.cell.2018.10.023
Solomon, P. S., Waters, O. D., and Oliver, R. P. (2007). Decoding the mannitol enigma in filamentous fungi. Trends Microbiol. 15, 257–262. doi: 10.1016/j.tim.2007.04.002
Spatafora, J. W., and Bushley, K. E. (2015). Phylogenomics and evolution of secondary metabolism in plant-associated fungi. Curr. Opin. Plant Biol. 26, 37–44. doi: 10.1016/j.pbi.2015.05.030
Theobald, S., Vesth, T. C., Rendsvig, J. K., Nielsen, K. F., Riley, R., de Abreu, L. M., et al. (2018). Uncovering secondary metabolite evolution and biosynthesis using gene cluster networks and genetic dereplication. Sci. Rep. 8:17957. doi: 10.1038/s41598-018-36561-3
Wasylenko, T. M., Ahn, W. S., and Stephanopoulos, G. (2015). The oxidative pentose phosphate pathway is the primary source of NADPH for lipid overproduction from glucose in Yarrowia lipolytica. Metab. Eng. 30, 27–39. doi: 10.1016/j.ymben.2015.02.007
Wisecaver, J. H., and Rokas, A. (2015). Fungal metabolic gene clusters-caravans traveling across genomes and environments. Front. Microbiol. 6:161. doi: 10.3389/fmicb.2015.00161
Wyatt, T. T., van Leeuwen, M. R., Wosten, H. A., and Dijksterhuis, J. (2014). Mannitol is essential for the development of stress-resistant ascospores in Neosartorya fischeri (Aspergillus fischeri). Fungal Genet. Biol. 64, 11–24. doi: 10.1016/j.fgb.2013.12.010
Wyatt, T. T., Wosten, H. A., and Dijksterhuis, J. (2013). Fungal spores for dispersion in space and time. Adv. Appl. Microbiol. 85, 43–91. doi: 10.1016/B978-0-12-407672-3.00002-2
Zahid, N., and Deppenmeier, U. (2016). Role of mannitol dehydrogenases in osmoprotection of Gluconobacter oxydans. Appl. Microbiol. Biotechnol. 100, 9967–9978.
Keywords: alcoholic fermentation, yeast metabolism, mannitol metabolism, Starmerella, fructophily
Citation: Gonçalves C, Ferreira C, Gonçalves LG, Turner DL, Leandro MJ, Salema-Oom M, Santos H and Gonçalves P (2019) A New Pathway for Mannitol Metabolism in Yeasts Suggests a Link to the Evolution of Alcoholic Fermentation. Front. Microbiol. 10:2510. doi: 10.3389/fmicb.2019.02510
Received: 21 August 2019; Accepted: 18 October 2019;
Published: 01 November 2019.
Edited by:
Amparo Querol, Spanish National Research Council (CSIC), SpainReviewed by:
Raul Raya, CONICET Centro de Referencia para Lactobacilos (CERELA), ArgentinaElvira Maria Hebert, National Scientific and Technical Research Council (CONICET), Argentina
Copyright © 2019 Gonçalves, Ferreira, Gonçalves, Turner, Leandro, Salema-Oom, Santos and Gonçalves. This is an open-access article distributed under the terms of the Creative Commons Attribution License (CC BY). The use, distribution or reproduction in other forums is permitted, provided the original author(s) and the copyright owner(s) are credited and that the original publication in this journal is cited, in accordance with accepted academic practice. No use, distribution or reproduction is permitted which does not comply with these terms.
*Correspondence: Paula Gonçalves, cG16QGZjdC51bmwucHQ=
†These authors have contributed equally to this work