- Instituto de Recursos Naturales y Agrobiología de Sevilla (IRNAS-CSIC), Seville, Spain
A PAHs-contaminated industrial soil was analyzed using PCR amplification of the gene 16S ribosomal RNA for the detection and identification of different isolated bacterial strains potentially capable of degrading PAHs. Novel degrader strains were isolated and identified as Achromobacter xylosoxidans 2BC8 and Stenotrophomonas maltophilia JR62, which were able to degrade PYR in solution, achieving a mineralization rate of about 1% day–1. A. xylosoxidans was also able to mineralize PYR in slurry systems using three selected soils, and the total extent of mineralization (once a plateau was reached) increased 4.5, 21, and 57.5% for soils LT, TM and CR, respectively, regarding the mineralization observed in the absence of the bacterial degrader. Soil TM contaminated with PYR was aged for 80 days and total extent of mineralization was reduced (from 46 to 35% after 180 days), and the acclimation period increased (from 49 to 79 days). Hydroxypropyl-ß-cyclodextrin (HPBCD) was used as a bioavailability enhancer of PYR in this aged soil, provoking a significant decrease in the acclimation period (from 79 to 54 days) due to an increase in PYR bioavailable fraction just from the beginning of the assay. However, a similar global extension of mineralization was obtained. A. xylosoxidans was then added together with HPBCD to this aged TM soil contaminated with PYR, and the total extent of mineralization decreased to 25% after 180 days, possibly due to the competitive effect of endogenous microbiota and the higher concentration of PYR in the soil solution provoked by the addition of HPBCD, which could have a toxic effect on the A. xylosoxidans strain.
Introduction
Organic pollutants released by the increasing number of industries is the direct cause of environmental impacts that have detrimental effects on living beings (Paul et al., 2005). Polycyclic aromatic hydrocarbons (PAHs) are pervasive hydrophobic organic compounds, which consist of two or more aromatic rings. Under natural environmental conditions, PAHs are not easily dissipated. Persistence increases with an increase in the molecular weight (Agrawal and Shahi, 2017). Low bioavailability and high persistence are important properties that make PAHs a cause for concern (Badr et al., 2004; Zhao et al., 2017), along with the potential toxic, mutagenic and carcinogenic effects of these compounds. PAHs are listed as priority pollutants by the United States Environmental Protection Agency (U. S. Environmental Protection Agency, 2008).
Bioremediation is considered as an useful and eco-friendly technology for cleaning up polluted environments using living organisms (Isaac et al., 2017). In natural soil bioremediation, the existing native microflora already present in the polluted soil is used to degrade the target contaminants (bioattenuation). Microorganisms that can degrade organic pollutants have been isolated with the aim of using their metabolic potential for the remediation of polluted soils (Paul et al., 2005). Especially, hydrocarbons have been part of the biosphere for millions of years, and also, a diverse group of eukaryotes has evolved to degrade them. From this group, most of the cultures are fungi, but there are also examples from several algal phyla, and there are reports that some protozoa can degrade hydrocarbons (Prince, 2018). Spini et al. (2018) evaluated the evolution of bacterial and fungal communities enriched from polluted soil by culture independent and dependent methods, concluding that the strains isolated reflected the microbial composition of the enriched consortia. The use of pure degrader strains is a recommended way to seek metabolic pathways or to evaluate the effect of different environmental conditions on PAHs biodegradation. A disadvantage of this approach is that it does not take into account the possibility that organic pollutant biodegradation can be the result of a synergic process between different microbial strains (Molina et al., 2009).
Bioaugmentation is defined as a technique for improvement of the degradative capacity of contaminated areas by the introduction of specific competent strains or consortia of microorganisms (Mrozik and Piotrowska-Seget, 2010). Bioaugmentation has been proven successful in the cleaning up of sites contaminated with aromatic compounds but still faces many environmental problems (Kauppi et al., 2011). One of the most difficult issues is survival of strains introduced to soil. It has been observed that the number of exogenous microorganisms has decreased shortly after soil inoculation. Many studies have shown that both abiotic and biotic factors influence the effectiveness of bioaugmentation (Cycon et al., 2017). This contaminant removal technique should be applied when the natural attenuation and biostimulation have failed (Mrozik and Piotrowska-Seget, 2010). In fact, bioaugmentation seems to be effective for the removal of PAH compounds at contaminated sites (Wu et al., 2016) or for the remediation of pesticides and their residues from soil (Cycon et al., 2017). In terms of efficiency and economy, this strategy for treating contaminated sites gives better results than chemical and/or physical methods (Isaac et al., 2017).
Numerous microorganisms have been isolated that are capable to degrade PAHs, but less are able to biodegrade chemical structures with four or more aromatic rings (Bastiaens et al., 2000). It is essential to be sure that these microorganisms do not produce more toxic metabolites than the pollutant parent compound during PAH degradation. Stenotrophomonas maltophilia strain VUN 10,003 was isolated by enrichment technique and evaluated for four and five PAHs aromatic rings in a basal liquid medium for fluorene (FLU) degradation and co-metabolization of other PAHs (Juhasz et al., 2000). Nzila et al. (2018) isolated a novel strain capable of metabolizing the four fused aromatic rings, identified as Achormobacter xylosoxidans PY4, which was able to utilize PYR in solution as the sole source of carbon, degrading more than 50% of the PYR in solution.
The low bioavailability of PAHs in soil affects their biodegradability, as they are considered hydrophobic compounds, and therefore, they sorb strongly to soil organic matter (Cerniglia, 1993). Cyclodextrins (CDs) are compounds capable of increasing water solubility of hydrophobic compounds. Organic compounds of the appropriate shape and size can form inclusion complexes with the low-polarity cavity of CDs, including PAHs (Morillo et al., 2012; Sánchez-Trujillo et al., 2013), pesticides and nitroaromatic compounds, provoking an increase of the bioavailability of these pollutants for degradation (Villaverde et al., 2013; Morillo et al., 2014; Morillo and Villaverde, 2017). Over organic solvents and/or non-ionic surfactants, CDs present advantages, such as improved desorption, non-toxicity, biodegradability and no sorption to the soil particles. For these reasons, the use of CDs have emerged as a useful tool for contaminants removal from soil systems (Villaverde et al., 2018a). Biocompounds produced by fermentative processes appeared as an economic and sustainable alternative to many synthetic molecules (Lopes et al., 2019). Thereby, biosurfactants have become a promising substitute due to their synthesis potential by a wide variety of microorganisms. However, despite their benefits, biosurfactants are not widely used because of the high production costs. Hence, cost-effective substrates, optimized cultivation conditions, and mutant lineage development are imperative to make these biomolecules an economically competitive product to propose a widespread replacement of synthetic surfactants. The data currently available indicate that the cost of production for biosurfactants is between 1 and 60 USD/kg, depending on the degree of purity and product specifications required by the desired application (Morillo and Villaverde, 2017). The feasibility of CDs use concerning the price of bulk material is frequently argued for not selecting them as remediation technology. Gruiz et al. (2009) compared the use of cyclodextrin (RAMEB) as bioavalability enhancer for in situ bioremediation with various realistic alternative technologies [monitored natural attenuation, excavation and disposal on landfills, on-site bioventing, soil flushing with water (pump-and-treat)]. They demonstrated that the cost-efficiency was similar or even lower than in the others technologies, proving their efficiency and competitiveness. The estimated cost of this bioremediation technology using CDs was $220/ton, and the duration of the treatment was only 1.5 years (for monitored natural attenuation the cost was $218/ton, but the duration was 15 years). One of the strengths is that CDs technologies are less time consuming than other alternative ones (40–70% lower than the other treatments), and it compensates the price of the bulk material.
The present work studied the isolation and characterization of PAHs degrading bacterial strains from a real contaminated soil (industrial site) with the objective of testing their capacity to be used as a soil bioremediation tool. Therefore, the objective of this study was to prove an effective bioremediation tool based on the inoculation of potential PAHs bacterial degraders previously isolated from soil, coupled with the use of hydroxypropil-β-cyclodextrin (HPBCD), to increase the PAHs bioavailability in order to achieve an improved bioremediation after PYR has been aged in soil.
Materials and Methods
Materials
Powdered PAHs (fluorene, FLU, phenanthrene, PHE and pyrene, PYR) were purchased from Sigma-Aldrich (Madrid, Spain). HPBCD was supplied by Cyclolab (Budapest, Hungary). A PAHs contaminated soil from an industrial site was used to obtain potential PAHs microbial degraders (Sánchez-Trujillo et al., 2013). Radiolabeled [ring-14C]-PYR was purchased from the Institute of Isotopes, Budapest, Hungary (specific activity 36 mCi mmol–1, chemical purity 99.9% and radiochemical purity 100%). There were three different soils (LT, CR, and TM) employed to carry out the biodegradation experiments. They were taken from the superficial horizon (0–20 cm). The soil samples were air-dried for 24 h, stones and plant materials removed, sieved through 2 mm and stored at 20°C. The soils were analyzed for particle size distribution, organic matter, pH and total carbonate content (Table 1).
Methods
Isolation and Identification of Bacteria From the Contaminated Soil by 16SrRNA Sequence Analysis
The strains employed in this study were isolated from an industrial contaminated soil, which was sampled from an area located at Llaneras (Oviedo, Northern Spain) previously described by Sánchez-Trujillo et al. (2013). The isolates were obtained from both the original contaminated soil and from PHE enriched cultures. The soil (0.3 g) was mixed with 15 mL of sterilized water, and the mixture was shaken for 1 h; after that, 100 μL of the mixture were added in Petri dishes with two different culture media, Tryptone Soya Agar (TSA) and mineral salt basal agar (MSB), and incubated at 28°C for 48 h. Morphologically different bacterial colonies were isolated following standard microbiological protocols and stored in MicrobankTM cryovials (2 mL microtubes containing 20 porous spheres of 3 mm diameter) and kept at −80°C (Villaverde et al., 2018b).
PHE enriched cultures were performed with 0.3 g of soil in 15 mL of sterilized water supplemented with PHE (1000 mg L–1) and incubated at 30°C for 30 days. Every 2 weeks, 100 μL of the culture was transferred to another flask containing MSB medium plus PHE and incubated again. At the end of the enrichment procedure, 100 μL of this suspension were inoculated in Petri dishes with MSB and incubated at 28°C for 48 h. There were 28 morphologically different bacterial colonies isolated following standard microbiological protocols, stored in Microbank cryovials and kept at −80°C.
DNA from all the isolated bacteria was extracted using an Extraction Kit (Jet quick, PCR TE, Genomed). The 16S ribosomal RNA gene (16S rRNA) was used for bacteria identification. The 16S rRNA gene was amplified by PCR using the primers 616F (5′−AGA GTT TGA TCC TGG CTC AG) and 1510R (5′−GGC TAC CTT GTT ACG ACT T). There were PCR reactions performed in 50 μL volumes, containing 1–2 μL of template DNA, 5 μL of 10 × PCR buffer Biotaq (Bioline, United States), 1.5 μL of 50 mM MgCl2 (Bioline), 1 μL of 10 mM deoxyribonucleoside triphosphate mixture (dNTPs) (Invitrogen, Carlsbad, CA, United States), 0.5 μL of 50 μM of each primer and 0.25 μL of Taq DNA polymerase enzyme (Bioline, United States), made up to 50 μL with nuclease−free water (Sigma–Aldrich, United States). PCR thermal conditions were as follow: 94°C for 120 s; 35 cycles of 94°C for 20 s, 55°C for 45 s, 72°C for 120 s and a final extension cycle at 72°C for 10 min. The amplified DNA fragment was purified using a purification kit Jetquick PCR Spin kit (Genomed, Löhne, Germany).
Taxonomic identification was done by comparing to NCBI database (National Centre for Biotechnology Information) using the BLASTN algorithm. The sequences were deposited in the NCBI GenBank database with accession numbers JF815694, GQ423064, JF262928 – JF262935, JQ895558, and JQ895559 (Table 2).
Characterisation of Phenanthrene Enriched Cultures by Molecular Analysis
In order to obtain information of phenanthrene enriched cultures, a 16S rRNA gene library was constructed with the TOPO TA Cloning kit (Invitrogen), as described in Gonzalez-Pimentel et al. (2018). The sequences were deposited in the NCBI GenBank database with accession numbers JF262936–JF262945 and JF262952, indicating that 82% of the 16SrRNA sequences analyzed belonged to the class Betaproteobacteria (Table 3). Similar results were observed by Martin et al. (2012) when the microbial community from a soil from a nearby road, which was spiked with PHE, was studied.
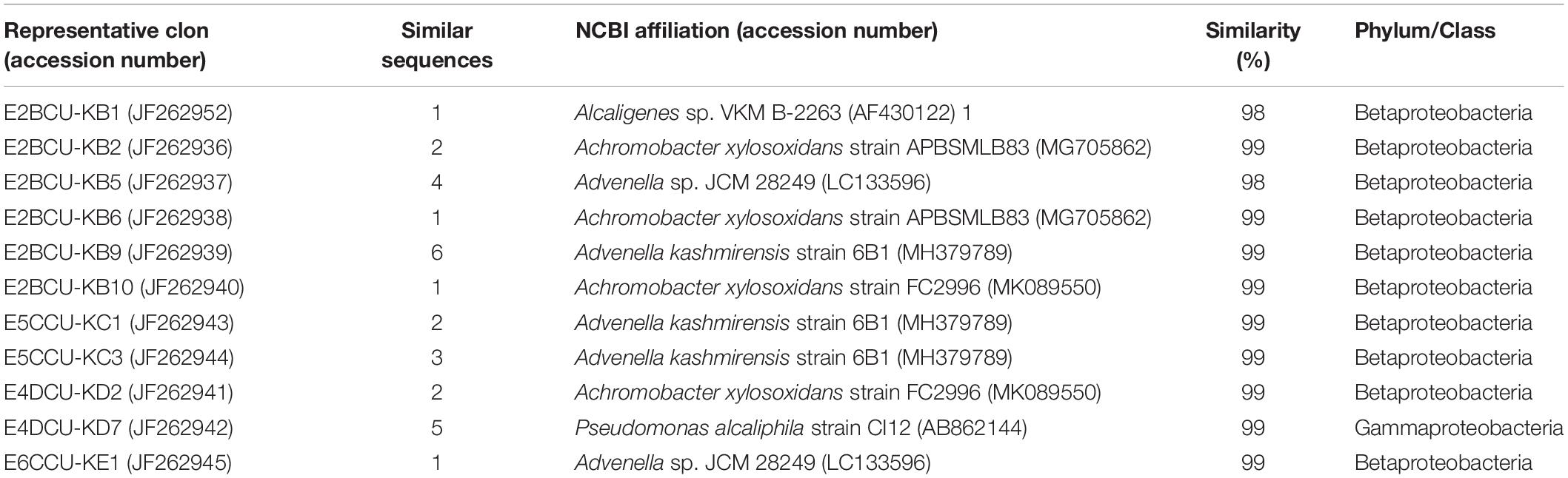
Table 3. Phylogenetic affiliations of the 16S rRNA sequences obtained from PHE enrichment cultures of the contaminated soil.
Isolation and Identification of Bacteria From the Contaminated Soil by 16SrRNA Sequence Analysis
Phylogenetic trees based on the 16SrRNA genes were constructed using the maximum-likelihood (Felsenstein, 1981), neighbor-joining (Saitou and Nei, 1987) and maximum-parsimony algorithms in MEGA7 (Kumar et al., 2016). The tree robustness was evaluated using a boot-strap analysis of 1,000 resamplings (Felsenstein, 1985).
Inoculum Preparation
There were six bacterial strains (Table 2) chosen to carry out the biodegradation of PYR and FLU, which were cultivated and subsequently stored in cryovials (Microbank). Cryovials were thawed and the selected bacteria were grown in Luria-Bertani (LB) medium. The bacteria were harvested just after starting stationary phase and washed twice in a sterile MSM solution. The final density of each strain added was 108 CFU mL–1.
Biodegradation Experiments in Solution
Both FLU and PYR biodegradation in solution, three replicates, were performed in 20 mL glass vials flasks (autoclave Auster-G, P-Selecta with one cycle at 120°C, inlet pressure of 103 kPa, for 20 min) with 15 mL of MSB (g L–1): Na2HPO.H2O (8 g); KH2PO4 (3 g); NH4Cl (1 g); NaCl (0.5 g); MgSO4 1M (1 mL), spiked with 4 mg L–1 of PYR or FLU as the only source of C and energy. Vials were inoculated when was required with 300 μL of bacterial culture (108 CFU mL–1). Glass vials were kept at a temperature of 20°C in a laboratory oven for 37 days. Throughout this period samples were taken at different times to observe the degradation of the PAHs.
Mineralization Assays in Solution and in Soil Suspensions
Mineralization of 14C-labeled PYR in solution and in suspension of three soils of different characteristics (both in triplicate) was measured through the evolution of 14CO2 produced (Villaverde et al., 2012). Mineralization assays were performed in respirometers. The mineralization assays in solution were carried out in modified 250 mL Erlenmeyers as respirometers with 100 mL of MSB, containing 14C-ring-labeled and unlabeled PYR to obtain a final concentration of 10 mg L–1 in solution. In soil suspension assays, 1 mL of a 1,000 mg L–1 PYR stock solution in methanol, which also contained 14C labeled PYR, was added to 2.5 g of soil (25% of the total soil used). The solvent was allowed to evaporate for 16 h. The remaining 75% soil was added and mixed to avoid damage to indigenous soil microorganisms, obtaining a final concentration of 100 mg kg–1 and a radioactivity of approximately 900 Bq per flask. After that, 100 mL of MSB was added (Reid et al., 1998), and 1 mL of a micronutrient solution (NS) containing trace elements (CaSO4⋅2H2O, ZnSO4⋅7H2O, Al2(SO4)3⋅16H2O, NiCl2⋅ 6H2O, CoCl2 ⋅ 2H2O, KBr, KCl, MnCl2⋅ 4H2O, SnCl2⋅2H2O, and FeSO4⋅7H2O) was also added in both mineralization experiments (Fenlon et al., 2011). Parallel experiments were carried out, inoculating when required with 1 mL of a suspension of A. xylosoxidans 2BC8 containing 108 CFU mL–1. The flasks were closed with Teflon-lined stoppers and incubated at 20 ± 1°C under shaking. Production of 14CO2 was measured as radioactivity appearing in the alkali trap of the biometer flasks, which contained 1 mL of 0.5M NaOH. Periodically, the solution was removed from the trap and replaced with fresh alkali. The NaOH solution was mixed with 5 mL of a liquid scintillation cocktail (Ready safe from PerkinElmer, Inc., United States), and the mixture was kept in darkness for about 24 h for dissipation of chemiluminescence. Radioactivity was measured with a liquid scintillation counter (Beckman Instruments Inc., Fullerton, California, model LS5000TD).
The same mineralization experiments were carried out but using only the soil TM which was incubated for 80 days after spiking with unlabeled and 14C labeled PYR. No biostimulation or bioaugmentation were added to the soil during this time. Before carrying out mineralization experiments, PYR which could be degraded in soil during this period was measured. An exhaustive extraction of PYR was carried out in the soil samples before and after aging, but less than 5% was lost. After this period, 100 mL of MSB, 1 mL of NS solution containing trace elements and inoculation with AX 2BC8 when required were added and the mineralization procedure was the same as previously described. The percentages PYR degraded along the mineralization were referred to its amount in soil at the beginning of this process.
Mineralization experiments in soil suspension of TM soil aged with PYR were also performed in the presence of HPBCD (20 times the PYR added to soil), with the aim of increasing its bioavailability. The flasks were inoculated, when required, with the specific bacterium A. xylosoxidans 2BC8 (1 mL with an initial inoculum density of 108 CFU mL–1) (Villaverde et al., 2013) and incubated at 20 ± 1°C.
Model of Mineralization Kinetics
Mineralization data were fitted to a first-order equation of the following form (Guerin and Boyd, 1992):
Ct = C0 e–kt, and DT50 = ln 2/k
A non-linear regression analysis (Sigmaplot v. 8.0) was used to estimate the kinetic parameter DT50.
Enumeration of Bacterial Strain Degraders in Soil
Enumeration of viable bacteria potentially PYR degraders for each soil (CR, LT, and TM) were performed (three replicates). In a 250 mL flask, 10 g of soil were spiked until reaching a PYR concentration of 10 mg kg–1, then 100 mL of TSB 0.05× medium was added. The systems were closed and shaken at 23°C for 30 h. There was 100 μL of the solution applied on agar plates prepared from a TSB 0.05× medium, and CFUs were counted after 48 h.
Statistical Analysis
Following blank-correction, statistical analysis of the results was performed in SigmaStat for windows (Version 2.03, SPSS Inc.). Significant effects on the mineralization of 14C-PYR in soil slurry (three different studied soils) and in inoculated and non-inoculated solution were compared statistically using a General Linear Model (ANOVA) (three replicates) (Tukey test, P < 0.05).
Results and Discussion
Isolation and Characterization of Potential PAHs Degraders Present in the Contaminated Soil
There were 28 strains isolated from the original contaminated soil and from PHE enrichment cultures (Table 2). These strains were differentiated by their morphology, as indicated in previous section. 16S rRNA gene sequences of all twenty-eight bacteria showed a match of 99% to those from bacteria in the NCBI GenBank database. There were 19 strains (68%) belonging to the class Gammaproteobacteria (Pseudomonas sp., Stenotrophomonas sp., and Acinetobacter sp.), four strains belonging to the class Betaproteobacteria (Ahromobacter sp., Advenella sp.), another four strains belonging to the phylum Actinobacteria (Microbacterium sp., Cellulomonas sp.) and only one strain belonging to the Bacteroidetes (Olivibacter sp.), concluding that 23 isolates belong to the phylum Proteobacteria (Delgado-Baquerizo et al., 2018). This result is in accordance with those observed by Labbé et al. (2007) and Cebrón et al. (2009) in hydrocarbon contaminated soils, which observed that the majority of microorganisms belonged to Gammaproteobacteria and Betaproteobacteria.
Figure 1 shows Maximum Likelihood tree based on 16S rRNA gene showing the relationships between the FLU and PYR degrading strains (2BC8 and JR62) and other isolated bacteria from the enrichment culture.
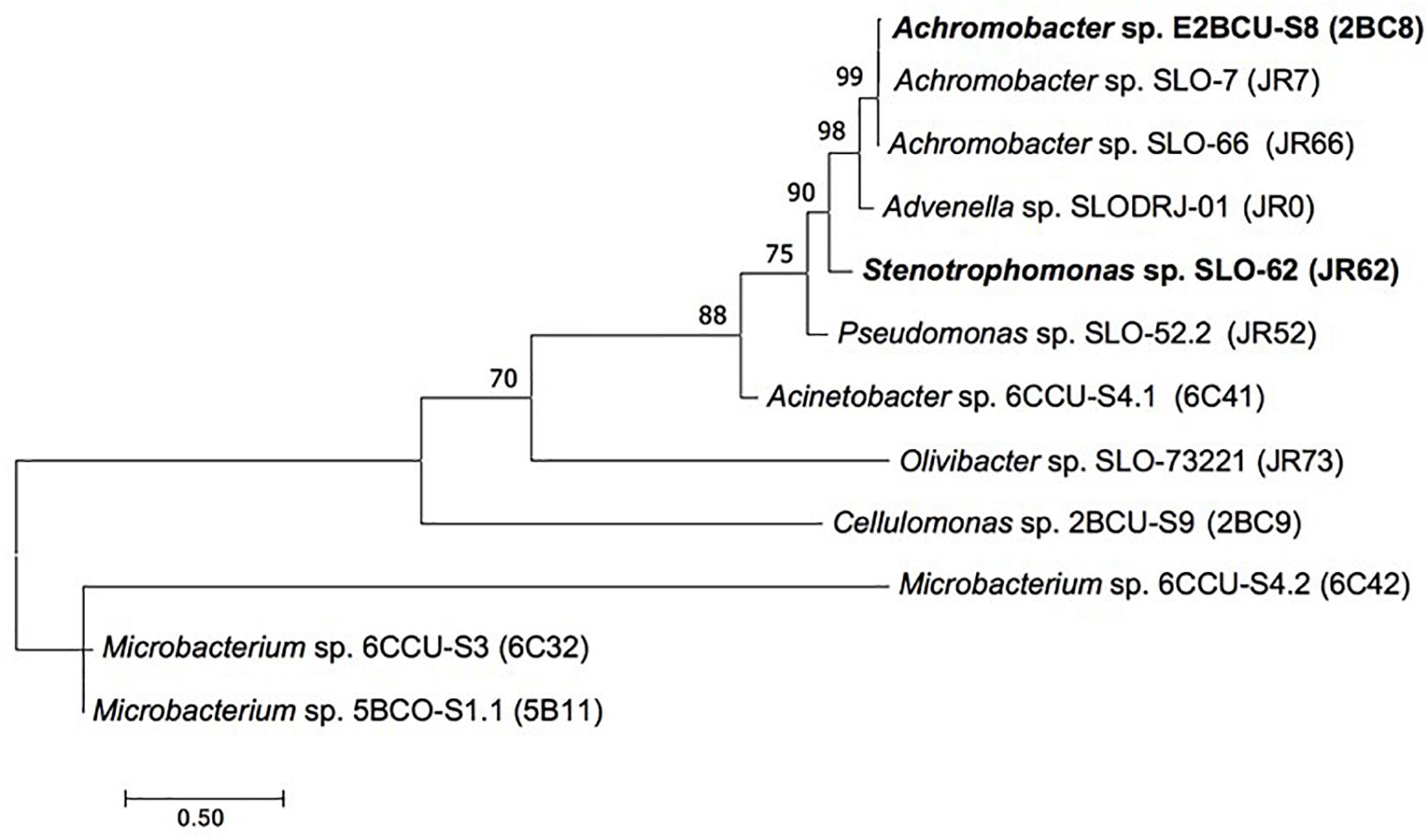
Figure 1. Maximum Likelihood tree based on 16S rRNA gene showing the relationships between the strains Achromobacter xylosoxidans 2BC8 and Stenotrophomonas maltophilia JR62 and other isolated bacteria obtained from the original soil or from the enrichment culture. Bootstrap values (>70%) are expressed as percentages of 1000 replicates. Same branches representation was recovered by the neighbour-joining and maximum-parsimony algorithms. The scale bar indicates 0.50 substitutions per nucleotide position in 16S rRNA gene sequences tree.
The studied soil showed a high level of contamination of Σ16 PAHs 1068 ± 101 mg kg–1 (Sánchez-Trujillo et al., 2013). This soil has been contaminated for more than 20 years, and hence, these strains have been exposed to this contamination over a long time, which indicates that these microorganisms have been capable of adapting their metabolism in the presence of the PAHs, using them as carbon source (Serrano et al., 2009). Haleyur et al. (2018) identified different bacterial strains previously isolated from aged weathered soils in an Australian former gasworks site, showing the catabolic potential of indigenous bacteria for PAHs-degradation.
Pseudomonas has been the most frequently found in the studied strains. The identified genus has been previously described as a potential PAHs degrader in literature, isolated from sites affected by significant levels of contamination by hydrocarbons (Haritash and Kaushik, 2009; Ghosal et al., 2016). Zhao et al. (2009) determined that there are a wide variety of bacterial strains capable of degrading PAHs, in particular low weight PAHs, isolated from contaminated soils. Among the identified, Acinetobacter sp., Pseudomonas sp., and Stenotrophomonas sp. prevailed. The genus Acinetobacter sp. has been reported in literature as a PAHs degrader (Yu et al., 2005; Janbandhu and Fulekar, 2011; Kafilzadeh et al., 2011). Kostka et al. (2011) isolated 24 bacterial strains belonging to 14 different genera, including Acinetobacter, from beach sand affected by oil discharge.
Zhao et al. (2011) tested the capacity to degrade hydrocarbons of a bacterial consortium obtained from a contaminated soil in an oil reservoir, where Microbacterium sp. was present. Schippers et al. (2005) isolated three oil degrading bacterial strains, which were identified as three new strains of the genus Microbacterium sp.
The role of different strains belonging to the genus Cellulomonas sp. in bioremediation of polluted soils by hydrocarbons has been also reported. Al-Awadhi et al. (2007) identified different bacterial strains of Cellulomonas sp. capable of degrading both aliphatic and aromatic hydrocarbons. Brito et al. (2006) carried out a microbial characterization of the PAHs degrading bacterial communities isolated from the mangrove sediments and they identified a Cellulomonas sp. as PYR degrader.
The genus Olivibacter sp. was identified and characterized for first time during the process of production of olive oil (Ntougias et al., 2007). Different species of the genus Olivibacter can be found in contaminated soils, wastes and cave environments, which can be able to degrade complex and toxic chemicals (Ntougias et al., 2015). Szabó et al. (2011) isolated a novel strain capable of degrading hydrocarbons, Olivibacter oleidegradans, from a clean-up facility (biofilter) in a hydrocarbon contaminated site.
The ability of Stenotrophomonas sp. to degrade PAHs and other hydrocarbons has been demonstrated (Tiwari et al., 2016; Nowak and Mrozik, 2018) and in particular, S. maltophilia strains (Chowdhury et al., 2017; Kumari et al., 2018). The strain S. maltophilia AJH1 was able to degrade low and high molecular weight PAHs (up to 95 and 80%, respectively) in acidophilic medium at pH 2 (Arulazhagan et al., 2017). Zafra et al. (2014) selected a degrading microbial consortium isolated from crude oil contaminated soils (50 fungal and bacteria isolates), highly tolerant to three-, four-, and five-ring PAHs, and S. maltophilia B14 grew employing PAHs as the sole carbon source and presented a high tolerance to PAHs up to 6 g L–1. Juhasz et al. (2000) proved the ability of S. maltophilia strain VUN 10,003 to degrade PYR, FLU, benz[a] anthracene, benzol[a] pyrene, dibenz[a,h]anthracene and coronene.
In relation to Achromobacter sp., Janbandhu and Fulekar (2011) reported, for the first time, that the novel bacterium Achromobacter insolitus MHF ENV IV degraded PHE; Ma et al. (2015) observed the degradation of fluoranthene by A. xylosoxidans DN002 isolated from a petroleum-contaminated soil. Dave et al. (2014) observed an enhanced biodegradation of total polycyclic aromatic hydrocarbons (TPAHs) when using A. xylosoxidans, isolated from crude oil polluted marine sites. Gunasekera et al. (2018) isolated a Achromobacter spanius strain which was present in the hydrocarbon-degrading bacterial community in a desert soil sample obtained under a fuel bladder.
FLU and PYR Biodegradation in Solution
A gen bank was created with the genes that codified by 16S RNA amplified by polymerase chain reaction (PCR) of the isolated 28 strains (Table 2). These strains were cultivated, although it was not possible to obtain enough microbial biomass of Olivibacter soli JR73 to be used in biodegradation processes. The most representative potential PYR degraders strains found in the studied soil were selected to perform FLU and PYR biodegradation assays in solution: Advenella sp. JRO and Achromobacter sp. 2BC8 (Betaproteobacteria); Stenotrophomonas sp. JR62 (SM) and Acinetobacter sp. 6C41 (Gammaproteobacteria) and Microbacterium sp. 6C32 and Cellulomonas sp. 2BC9 (Actinobacteria). Pseudomonas spp. (Betaproteobacteria) was not selected since it is a very known PAH degrader (Sopeña et al., 2013). Only two of the six strains selected showed a significant biodegradation capacity for FLU and PYR (Supplementary Figure S1). With A. xylosoxidans 2BC8 inoculum, FLU reached a biodegradation of about 45%, while when S. maltophilia JR62 was inoculated, a complete biodegradation of FLU was observed. The percentage of PYR biodegraded by A. xylosoxidans 2BC8 reached about 40% during the experimental period, while with S. maltophilia JR62, the PYR biodegraded was 32%.
The selected strains were identified as A. xylosoxidans 2BC8 and S. maltophilia JR62, both showing an identity of 99% with accession numbers CP014028.2 and GU385870.1, respectively. Other authors have also reported PAHs degradation using A. xylosoxidans and S. maltophilia, as it has been mentioned before, but they are quite uncommon strains for PAHs degradation. Castro-Gutiérrez et al. (2012) isolated sixteen PAH-degrading strains with the ability to grow on naphthalene (NAP), PHE, FLU, and PYR. Most of the isolates belonged to the genus Pseudomonas, although Comamonas, Sphingomonas, Stenotrophomonas and Delftia were also found. Tiwari et al. (2010) studied a bacterium characterized as A. xylosoxidans, isolated from an oil refinery effluent sludge and capable of aerobic degradation of PYR. Ghevariya et al. (2011) isolated a multiple PAH degrading halotolerant A. xylosoxidans, which was isolated from crude oil polluted saline site and exhibited 86% chrysene degradation. As far as authors know, the scarce studies carried out using A. xylosoxidans as a degrader of PAHs have arisen after 2010.
PYR Mineralization in Solution
PYR is considered one of the priority organic contaminants due to its toxicity and persistence. One of the strategies followed to improve organic pollutant biodegradation consists of using isolated microbial consortia due to their capacity for synergistic metabolism, avoiding potential toxic effects of the metabolites formed. However, the use of a single mineralizing microorganism, from a scientific point of view, is more interesting in order to control the different factors that influence in the effectiveness of bioaugmentation treatment and to determine the pollutant biodegradation metabolic pathway. For these reasons, isolation of a single degrader strain can be considered a first step to optimize an effective bioaugmentation tool for PAHs bioremediation. The two strains isolated and identified in this work (A. xylosoxidans 2BC8 and S. maltophilia JR62) were also proved for PYR mineralization. Figure 2 shows PYR mineralization curves obtained after their inoculation. Authors have concluded that inoculum density at a level of 106–1010 UFC g–1 of soil is suitable for the efficient degradation of organic contaminants by the microorganisms inoculated (Alexander, 2000). However, some authors observed an initial lag-phase in an organic pollutant biodegradation profile after inoculation of microorganisms at an even higher level (Cycoń et al., 2014).
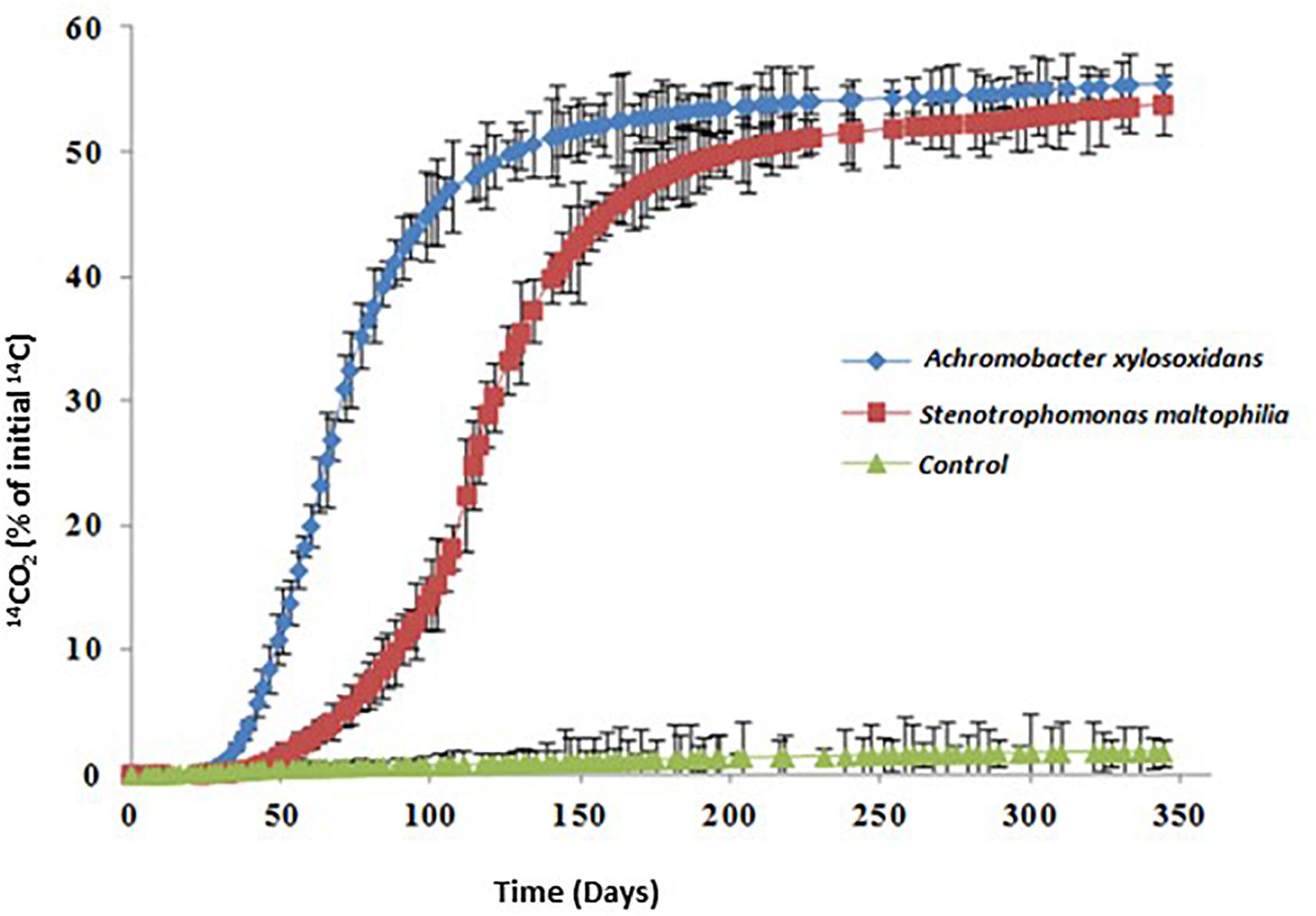
Figure 2. PYR mineralization curves in solution in the presence of Achromobacter xylosoxidans 2BC8 and Stenotrophomonas maltophilia JR62.
The tested strains were capable of mineralizing PYR (Table 4). A. xylosoxidans 2BC8 was able to adapt to use PYR as carbon source quicker than S. maltophilia JR62 (40 and 69 days of acclimation period, respectively). Analysis of variance when the only factor study was soil indicated that there were only significant differences in the acclimation period and DT50.

Table 4. Parameters obtained from PYR mineralization in solution after inoculation with Achromobacter xylosoxidans 2BC8 and Stenotrophomonas maltophilia JR62.
The mineralization rate was similar for both strains, 1.00 and 1.08% days–1. Significant differences between the values reached for the PYR extent of mineralization were not found (55.5 and 53.8%, respectively). By contrast, A. xylosoxidans was able to mineralize 50% of PYR in solution faster than S. maltophilia JR62 (DT50 118 and 200 days, respectively).
Numerous microorganisms capable of degrading low and medium weight PAHs have been isolated. It is important that these microorganisms do not form toxic metabolites during the PAH degradation, and for this reason, it is important to determine their pollutant mineralization capacity (Guo et al., 2017; Isaac et al., 2017; Aziz et al., 2018), and therefore, soil toxicity evolution during the biodegradation process should be also analyzed (Molina et al., 2009).
From the two selected strains, A. xylosoxidans 2BC8 was chosen for the subsequent soil mineralization studies due to its higher capacity to adapt to the presence of PYR in the medium (lower acclimation period) and DT50, and also because there are very few published works which have demonstrated the effectivity of Achromobacter sp. as PAH degrader, as commented previously.
PYR Mineralization in Contaminated Soils
With the aim of determining the natural attenuation capacity of soils non-exposed previously to PAHs contamination, three soils with different properties (Table 1) were selected and spiked with PYR (10 mg kg–1). Soil mineralization curves corresponding to the soils named as CR, LT, and TM are shown in Figure 3. For all the studied soils significant differences in acclimation period of the endogenous microbiota were observed between them (Table 5). When the factors soil and inoculation were separately taken into account, the statistical analysis indicated that they have effect over all the studied variables. Interaction of both factors (soil and inoculation) indicated that the effect of the inoculum depended of the type of soil (Supplementary Table S1). However, although the extent of mineralization was similar for the soils LT and TM, the PYR mineralization rate was the highest in the case of soil LT. Regarding DT50 values obtained in Table 5, this value was not reached for CR and LT soils during the assay time and in the case of TM soil the calculated value was 258 days.
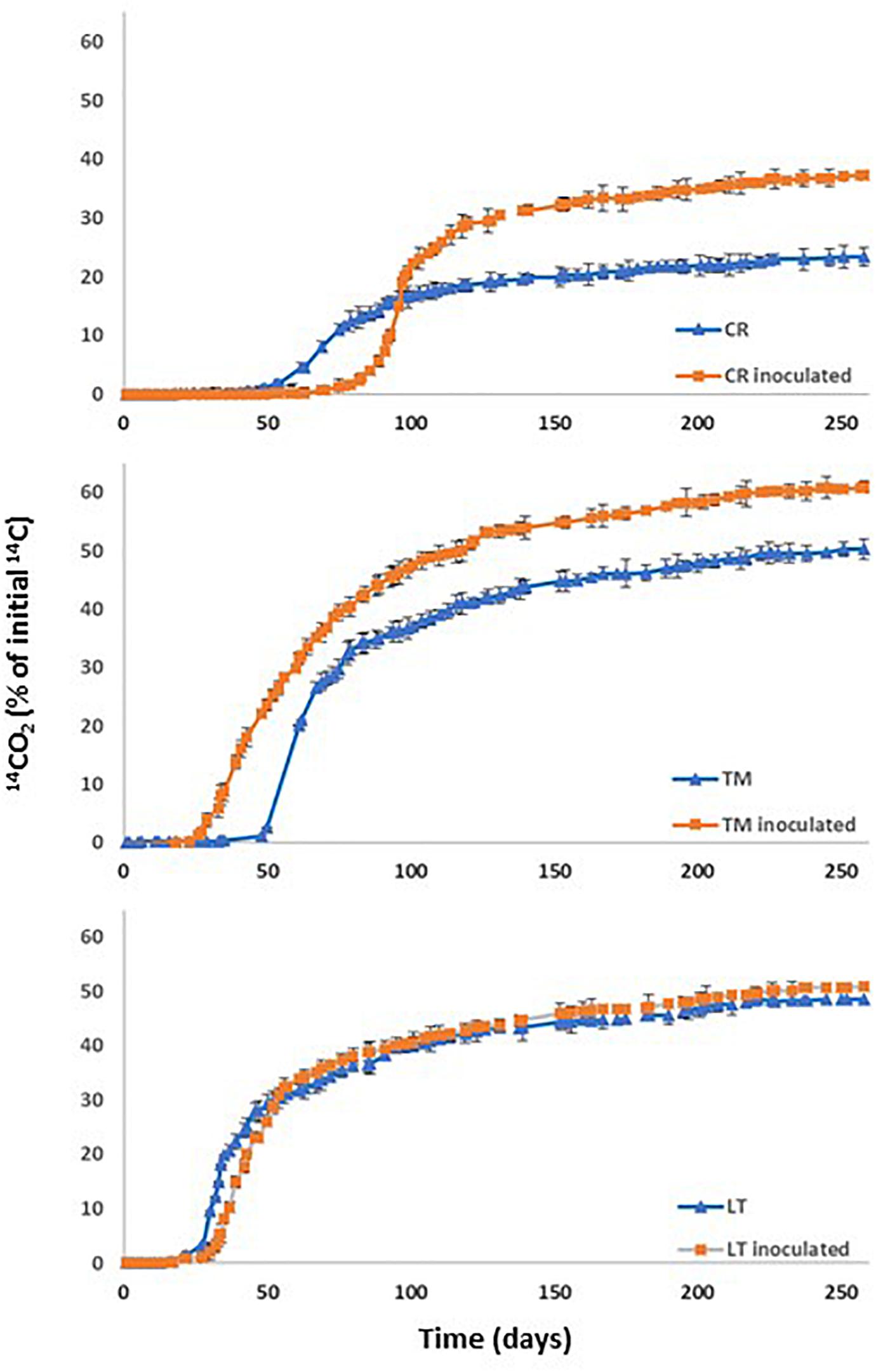
Figure 3. PYR mineralization curves in non-sterilised soil CR, LT, TM without inoculation and after inoculation with Achromobacter xylosoxidans 2BC8.
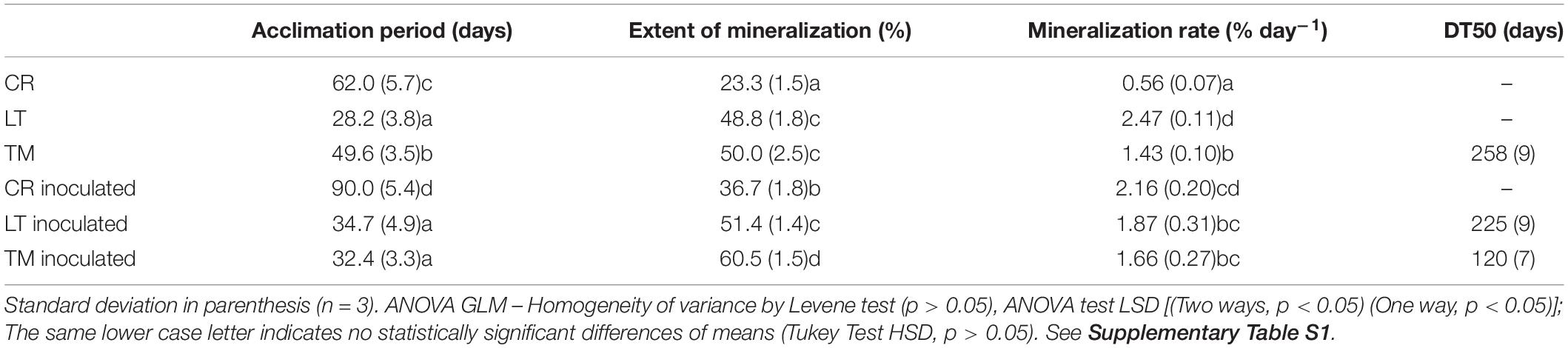
Table 5. Parameters obtained from PYR mineralization in soils before and after their inoculation with Achromobacter xylosoxidans 2BC8 (AX 2BC8).
Soil natural attenuation assessments determined for the three investigated soils were correlated with the enumerated soil PYR microbial degraders in each soil (CFU g–1 of soil). The soils, LT and TM, which showed a similar extent of PYR mineralization, presented 8 × 107 and 2 × 107 CFU g–1, respectively, of potential PYR bacterial degraders. CR soil showed the longest acclimation period (62 days) and the lowest extent of mineralization (23.3%) and mineralization rate (0.56% days–1), which is clearly correlated with the lowest concentration of soil PYR potential degraders (8 × 106 CFU g–1). Towell et al. (2011) investigated the microbial degradation of 14C-labeled hexadecane, octacosane, PHE and PYR and observed that soils with the highest concentration of microbial PYR degraders showed the highest mineralization rates. Wu et al. (2016) concluded that the populations of the total petroleum hydrocarbons (TPH) degraders in soil were positively correlated to TPH biodegradation efficiency during bioremediation. Simarro et al. (2013) assessed the effectiveness of different in situ bioremediation treatments (bioaugmentation, biostimulation, bioaugmentation and biostimulation, and natural attenuation) on creosote polluted soil, and results showed that creosote decreased significantly in all treatments, and no significant differences were found between treatments. However, some specific polycyclic aromatic hydrocarbons (PAH) were degraded to a greater extent by biostimulation.
Bioaugmentation of the contaminated soil with the strain A. xylosoxidans 2BC8 was performed with the aim of showing its potential for PYR biodegradation. PYR mineralization curves after the inoculation of CR, LT and TM soils are also shown in Figure 3 and mineralization results in Table 5. In all cases, the global extent of PYR mineralization was improved with bioaugmentation regarding when the soils were not inoculated. Inoculation in soils CR and TM led to an important increase of the global extent of mineralization, regarding that obtained with the autochthonous soil microbiota (57.5 and 21% of increase, respectively). In LT, soil inoculation had no significant effect in the global extent of mineralization, increasing only about 4.5%. The fact that LT soil had the highest autochthonous PYR bacterial degraders (8 × 107 UFC g–1) could result in a higher competition with the A. xylosoxidans 2BC8 inoculated, resulting in a similar PYR biodegradation profile. Among the biotic factors affecting bioaugmentation, the most important seem to be the interactions between autochthonous and inoculated microorganisms, such as predation and the competition for nutrients and niches. However, it is thought that the most important factor influencing the success of bioaugmentation is the ability of inoculants to survive in the contaminated environment (Cycon et al., 2017). On the contrary, soil CR had the lowest concentration in specific PYR degraders (8 × 106 g–1), which makes the effect of A. xylosoxidans 2BC8 inoculation more notorious. Villaverde et al. (2017) observed a competitive effect between the inoculum and soil endogenous microbiota when a diuron degrading microbial consortium was inoculated in six different soils. Cycon et al. (2017) indicated that it has been observed that when lower inoculum densities (<104 CFU g–1 of soil) were used, only a small part of the introduced bacteria survived the initial competition and participated in pesticide degradation. In our case, the final density of each strain added was 107 CFU g–1 of soil, what would secure an increase in the period of effectiveness of the inoculate. Maddelaa et al. (2016) conducted a study a field-level to determine total petroleum hydrocarbon (TPH)-degrading potential of two bacterial strains, Bacillus thuringiensis B3 and B. cereus B6, and two fungi, Geomyces pannorum HR and Geomyces sp. strain HV, all soil isolates obtained from an oil field located in north-east region of Ecuador. Crude oil-treated soil samples contained in wooden boxes received a mixture of all the four microorganisms and were incubated for 90 days in an open low-land area of Amazon rainforest and the bacteria mixed inoculum density used was 0.1 × 106 cfu g–1.
Aging Effect on Soil PYR Mineralization
Soil TM was selected for this study due to its high content in organic matter and clay fraction. It was spiked with PYR (10 mg kg–1) and aged for 80 days. In Figure 4A, the aging effect on PYR mineralization in the soil is shown. An increase in the acclimation period from 49 days for TM soil to 79 days for aged TM soil (TME), a decrease in mineralization rate (from 1.43 to 1.20% d–1), and the total extension of mineralization (from 46 to 35%) was observed (Table 6). Aging of organic chemicals in soils has been described by several mechanisms involved, including non-desorbed fraction of the chemical molecules within some components of soil organic matter (Brusseau et al., 1994) and organic molecules can diffuse slowly into organic matter soil matrix and be entrapped within small pores (Villaverde et al., 2009; Cheng et al., 2012; Ran et al., 2013). The aging effect is described as the trapping of organic pollutants, reducing their bioaccessibility. It is also possible that strong bonds may form between organic pollutants and soil organic matter (Kong et al., 2013). Rubio-Bellido et al. (2018) studied the aging of diuron contaminated soil for 100 days and observed a drastic decrease of diuron desorbed and mineralized fraction.
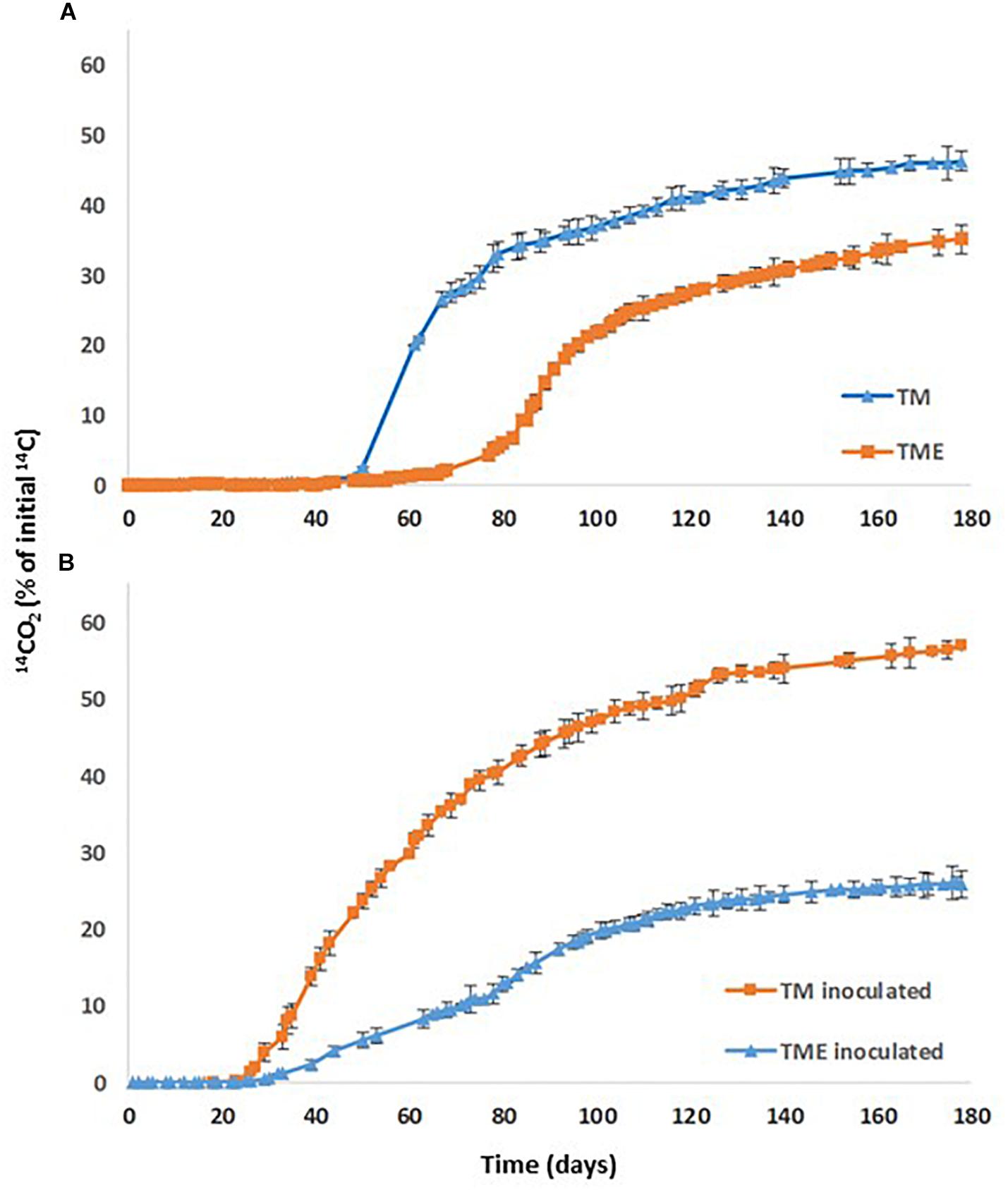
Figure 4. PYR mineralization curves in: (A) TM soil (TM) and TM soil after aging (TME); (B) TM soil inoculated with Achromobacter xylosoxidans 2BC8 (TM inoculated) and TM soil inoculated after aging (TME inoculated).
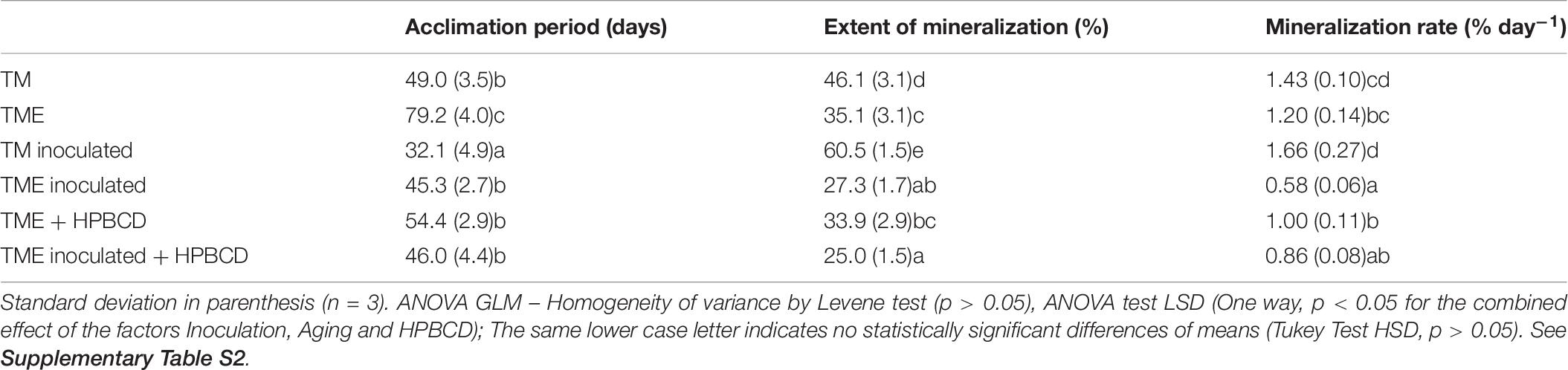
Table 6. Parameters obtained from PYR mineralization in TM soil (TM), TM soil after aging (TME), TM soil inoculated with AX 2BC8 (TM inoculated), TM soil inoculated after aging (TME inoculated), TM soil treated with HPBCD after aging (TME + HPBCD), and TM soil inoculated and treated with HPBCD after aging (TME inoculated + HPBCD).
When PYR is aged in the studied soil and after 80 days it is inoculated with A. xylosoxidans 2BC8 (Figure 4B), the acclimation period was significantly reduced from 79 to 45 days (Table 6 and Figure 5A), but this was not translated into an enhancement of the total extent of PYR mineralization results, unlike when PYR is not aged in the soil. The presence in the soil TM of specific PYR degraders and the increasing of the contact time between PYR molecules and microbial degraders will contribute to a catabolic induction, producing specific enzymes for PYR degradation and giving rise to a reduced acclimation period due to this previous activation (Fenlon et al., 2011). However, the lower mineralization observed after inoculation with A. xylosoxidans 2BC8 of the aging soil TME seems to indicate the strong microbial competence established between the endogenous soil flora capable of degrading PYR, already activated due to the aging, and the inoculated A. xylosoxidans 2BC8 strain. A similar competence was observed by Villaverde et al. (2017) in the bioremediation of diuron contaminated soils by a novel degrading microbial consortium.
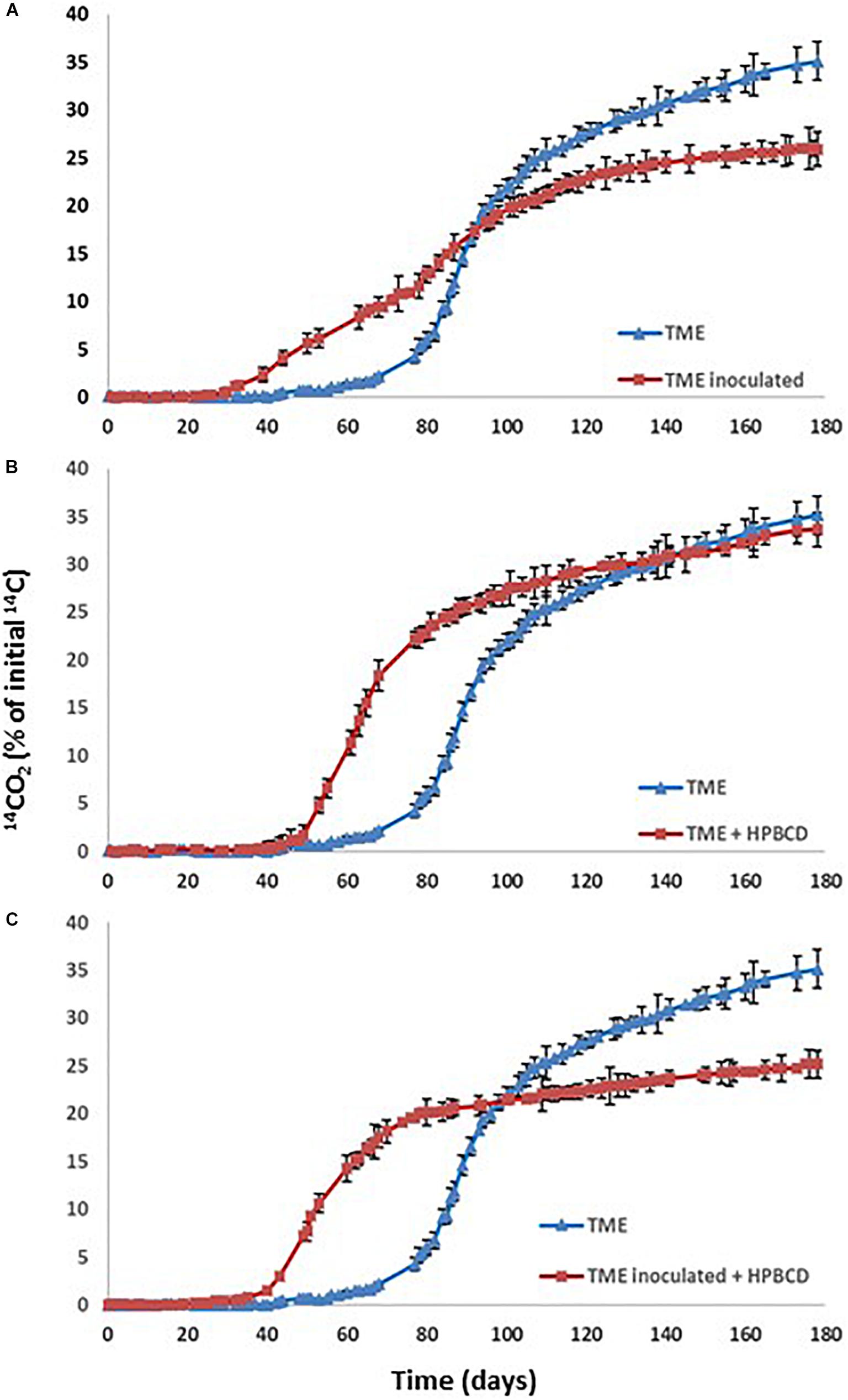
Figure 5. PYR mineralization curves in aged TM soil (TME): (A) Effect of the inoculation with Achromobacter xylosoxidans 2BC8; (B) Effect of HPBCD addition; (C) Effect of HPBCD addition combined with Achromobacter xylosoxidans 2BC8.
Effect of HPBCD Application on PYR Mineralization in Soil
Bioremediation of contaminated soil has been improved with the use of CDs due to their ability to increase the water solubility of hydrophobic organic compounds, facilitating their mobilization (Morillo and Villaverde, 2017). A way of increasing the biodegradable fraction of POPs (persistent organic pollutants) in soils is this movement of the contaminant molecules from the soil into soil solution (Morillo et al., 2014; Sánchez-Trujillo et al., 2014; Madrid et al., 2016, 2019; Simpanen et al., 2016; Guo et al., 2017). CDs have been proposed as an alternative to synthetic surfactants for the removal of hydrophobic contaminants from soils (Fenyvesi et al., 2009). Synthetic surfactants have the disadvantage of being toxic to the resident microbial population. Moreover, surfactants use to form high-viscosity emulsions difficult to remove from soil due to their low water-solubility, and sometimes the adsorption of surfactants onto soils is high. The advantage of using CDs as extractants is their low environmental impact. CDs have been approved as non-toxic compounds that do not harm resident microbial populations. In addition, due to their glucose-based composition, CDs are considered biodegradable, although some CDs are resistant for at least a few months (Fenyvesi et al., 2005).
The tested CD in this work (HPBCD) was proved for use as a carbon source by A. xylosoxidans 2BC8 because if this strain was capable of metabolizing HPBCD, it would be highly likely that it would be employed as a carbon source instead of PYR. The studied strain grew in a similar way in the absence (control) and the presence of HPBCD, indicating that the selected strain does not use HPBCD as a carbon source or energy. Therefore, when A. xylosoxidans 2BC8 in the PYR mineralization assays in soil is inoculated, the strain will not prefer to biodegrade HPBCD.
In PYR mineralization assays, HPBCD was added to the TM soil after PYR aging for 80 days, with the aim of simulating a more realistic scenario of soil contamination and PYR bioaccessibility evaluation. The PYR mineralization profile in the presence of HPBCD is shown in Figure 5B and data from the different kinetic profiles in Table 6. CDs are able to increase the hydrosolubility of different PAHs, as observed in previous studies through the formation of inclusion complexes (Morillo et al., 2012). HPBCD addition (Figure 5B) provoked a significant decrease of 25 days in the acclimation period (from 79 days for TME to 54 days for TME + HPBCD) (Table 6) in relation to the mineralization in the absence of HPBCD, although a similar global extension of mineralization and mineralization rate was determined (33.9 and 1.00% day–1, respectively). The explanation for this reduction is the higher bioaccessibility of PYR after HPBCD application due to an increase of its bioavailable fraction just from the beginning of the assay (Reid et al., 2000), reaching a similar global extension of mineralization corresponding to the global PYR bioavailable fraction in soil (Rubio-Bellido et al., 2016). These results also reveal that the increase of PYR bioavailability due to HPBCD addition has no toxic effects on the indigenous microbiota.
Figure 5C shows the PYR mineralization profiles in the aged soil when A. xylosoxidans 2BC8 inoculation and HPBCD were jointly applied. The extent of PYR mineralization was reduced to 25% (Table 6). The statistical analysis indicated that the effect of inoculation depended on the presence or absence of HPBCD (Supplementary Table S2). In this case, besides the competitive effect of endogenous microbiota with A. xylosoxidans 2BC8 strain previously mentioned, the application of HPBCD increased PYR bioavailability, and hence its concentration in the soil suspension, what would give rise to a toxic effect on the exogenous inoculated bacterium. Stroud et al. (2009) observed that the amendment of HPBCD to phenanthrene and hexadecane contaminated soil did not enhance biodegradation after 25 days of aging because CD interfered with microbial degradation, which resulted in a lower biodegradation extent.
Conclusion
From the 28 strains isolated from the original contaminated soil and from the enrichment cultures, two of them, A. xylosoxidans 2BC8 and S. maltophilia JR62 (uncommon bacterial strains capable of degrading PAHs) demonstrated their capacity to degrade fluorene and pyrene in solution. Both strains were also capable of mineralizing pyrene in solution, but A. xylosoxidans 2BC8 was faster and was selected to carry out bioaugmentation of three pyrene contaminated soils. The global extent of mineralization was improved in all cases in the bioaugmented soils, but the increment was less pronounced as the number of autochthonous pyrene bacterial degraders in the soil was greater. Bioaugmentation with A. xylosoxidans 2BC8 in aged pyrene contaminated TM soil increased the acclimation period, and decreased the total extent of mineralization, in comparison to the non-inoculated PYR aged soil, indicating a stronger microbial competence between the indigenous soil flora specific degrader of PYR, activated due to the aging, and the inoculated exogenous strain. The same competitive effect between indigenous and exogenous flora was also observed when HPBCD was added as extractant to increase pyrene bioavailability. All these results indicate that the effect of the addition of exogenous bacterial strains for the remediation of contaminated soils will depend on the specific microbiological characteristics of the soil and the positive or negative impact of the new strains on it. And the same occurs with the use of extractants such as HPBCD, since the increase of the contaminant bioavailability could have negative toxic effects on both the indigenous and the exogenous microbiota.
Data Availability Statement
All datasets generated for this study are included in the article/Supplementary Material.
Author Contributions
JV, LL, and EM designed research. JV, LL, EM, and AL-M performed research. JV, LL, EM and JG-P analyzed data. JV, LL, EM, JG-P and AL-M wrote the manuscript. All authors read and approved the final manuscript version.
Funding
This work was supported by the Spanish Ministry of Economy and Competitiveness under the research project CTM2O13-42599-R (AEI/FEDER, UE) and Junta de Andalucía Proyect RNM 894. AL-M acknowledges the Spanish Ministry of Education. Culture and Sports for her FPU fellowship (FPU15/03740).
Conflict of Interest
The authors declare that the research was conducted in the absence of any commercial or financial relationships that could be construed as a potential conflict of interest.
Supplementary Material
The Supplementary Material for this article can be found online at: https://www.frontiersin.org/articles/10.3389/fmicb.2019.02588/full#supplementary-material
References
Agrawal, N., and Shahi, S. K. (2017). Degradation of polycyclic aromatic hydrocarbon (pyrene) using novel fungal strain Coriolopsis byrsina strain APC5. Inter. Biodeter. Biodegr. 122, 69–81. doi: 10.1016/j.ibiod.2017.04.024
Al-Awadhi, H., Sulaiman, R. H. D., Mahmoud, H. M., and Radwan, S. S. (2007). Alkaliphilic and halophilic hydrocarbon-utilizing bacteria from Kuwaiti coasts of the Arabian Gulf. Appl. Microbiol. Biotechnol. 77, 183–186. doi: 10.1007/s00253-007-1127-1
Alexander, M. (2000). Aging, bioavailability, and overestimation of risk from environmental pollutants. Environ. Sci. Technol. 34, 4259–4265.
Arulazhagan, P., Al-Shekri, K., Huda, Q., Godon, J. J., Basahi, J. M., and Jeyakumar, D. (2017). Biodegradation of polycyclic aromatic hydrocarbons by an acidophilic Stenotrophomonas maltophilia strain AJH1 isolated from a mineral mining site in Saudi Arabia. Extremophiles 21, 163–174. doi: 10.1007/s00792-016-0892-0
Aziz, A., Agamuthu, P., Alaribe, F. O., and Fauziah, S. H. (2018). Biodegradation of benzo[a]pyrene by bacterial consortium isolated from mangrove sediment. Environ. Technol. 39, 527–535. doi: 10.1080/09593330.2017.1305455
Badr, T., Hanna, K., and De Brauer, C. (2004). Enhanced solubilization and removal of naphthalene and phenanthrene by cyclodextrins from two contaminated soils. J. Hazard. Mater. 112, 215–223. doi: 10.1016/j.jhazmat.2004.04.017
Bastiaens, L., Springael, D., Wattiau, P., Verachtert, H., Harms, H., DeWachter, R., et al. (2000). Isolation of adherent polycyclic aromatic hydrocarbon (PAH) -degrading bacteria using PAH-sorbing carriers. App. Environ. Microbiol. 66, 1834–1843. doi: 10.1128/AEM.66.5.1834-1843.2000
Brito, S., Guyoneaud, R., and Goñiurriza, M. (2006). Characterization of hydrocarbonoclastic bacterial communities from mangrove sediments in Guanabara Bay. Braz. Res. Microbiol. 157, 752–762. doi: 10.1016/j.resmic.2006.03.005
Brusseau, M. L., Wang, X. J., and Hu, Q. H. (1994). Enhanced transport of low polarity organic compounds through soil by cyclodextrin. Environ. Sci. Technol. 28, 952–956. doi: 10.1021/es00054a030
Castro-Gutiérrez, V. M., Rodríguez-Rodríguez, C. E., and Vargas-Azofeifa, I. (2012). Hydrocarbon degrading microflora in a tropical fuel-contaminated aquifer: assessing the feasibility of PAH bioremediation. Int. J. Environ. Res. 6, 345–352. doi: 10.22059/ijer.2011.500
Cebrón, A., Beguiristain, T., Faure, P., Norini, M. P., Masfaraud, J. F., and Leyval, C. (2009). Influence of vegetation on the situ bacterial community and polyclyclic hydrocarbon (PAH) degraders in aged PAH-contaminated or thermal-desorption-treated soil. App. Environ. Microbiol. 75, 6322–6330. doi: 10.1128/AEM.02862-08
Cerniglia, C. E. (1993). Biodegradation of polycyclic aromatic hydrocarbons. Curr. Opin. Biotechnol. 4, 331–338. doi: 10.1007/BF00129093
Cheng, H., Hu, E., and Hu, Y. (2012). Impact of mineral micropores on transport and fate of organic contaminants: a review. J. Contam. Hydrol. 12, 80–90. doi: 10.1016/j.jconhyd.2011.09.008
Chowdhury, R., Dhar, K., Ahamed, F., Alam, S., and Uddin, M. (2017). Biodegradation of anthracene and phenanthrene by bacteria isolated from oil-contaminated soil of Bangladesh. Chem. Ecol. 33, 843–855.
Cycon, M., Mrozik, A., and Piotrowska-Seget, Z. (2017). Bioaugmentation as a strategy for the remediation of pesticide-polluted soil: a review. Chemosphere 172, 52–71. doi: 10.1016/j.chemosphere.2016.12.129
Cycoń, M., Zmijowska, A., and Piotrowska-Seget, Z. (2014). Enhancement of deltamethrin degradation by soil bioaugmentation with two different strains of Serratia marcescens. Int. J. Environ. Sci. Technol. 11, 1305–1316. doi: 10.1007/s13762-013-0322-0
Dave, B. P., Ghevariya, C. M., Bhatt, J. K., Dudhagara, D. R., and Rajpara, R. K. (2014). Enhanced biodegradation of total polycyclic aromatic hydrocarbons (TPAHs) by marine halotolerant Achromobacter xylosoxidans using Triton X-100 and β-cyclodextrin. A microcosm approach. Mar. Pollut. Bull. 79, 123–129. doi: 10.1016/j.marpolbul.2013.12.027
Delgado-Baquerizo, M., Oliverio, A. M., Brewer, T. E., Benavent-González, A., Eldridge, D. J., Bardgett, R. D., et al. (2018). A global atlas of the dominant bacteria found in soil. Science 359, 320–325. doi: 10.1126/science.aap9516
Felsenstein, J. (1981). Evolutionary trees from DNA sequences: a maximum likelihood approach. J. Mol. Evol. 17, 368–376.
Felsenstein, J. (1985). Confidence limits on phylogenies: an approach using the bootstrap. Evolution 39, 783–791. doi: 10.1111/j.1558-5646.1985.tb00420.x
Fenlon, K. A., Andreou, K., Jones, K. C., and Semple, K. T. (2011). The extractability and mineralisation of cypermethrin aged in four UK soils. Chemosphere 82, 187–192. doi: 10.1016/j.chemosphere.2010.10.025
Fenyvesi, E., Gruiz, K., Verstichel, S., DeWilde, B., Leitgib, L., and Csabai, K. (2005). Biodegradation of cyclodextrins in soil. Chemosphere 60, 1001–1008.
Fenyvesi, É, Molnár, M., Leitgib, L., and Gruiz, K. (2009). Cyclodextrin-enhanced soil remediation technologies. Land Contam. Reclam. 17, 585–597.
Ghevariya, C. M., Bhatt, J. K., and Dave, B. P. (2011). Enhanced chrysene degradation by halotolerant Achromobacter xylosoxidans using response surface methodology. Bioresour. Technol. 102, 9668–9674. doi: 10.1016/j.biortech.2011.07.069
Ghosal, D., Ghosh, S., Dutta, T. K., and Ahn, Y. (2016). Current state of knowledge in microbial degradation of polycyclic aromatic hydrocarbons (PAHs): a review. Front. Microbiol. 7:1369. doi: 10.3389/fmicb.2016.01369
Gonzalez-Pimentel, J. L., Miller, A. Z., Jurado, V., Laiz, L., Pereira, M. F. C., and Saiz-Jimenez, C. (2018). Yellow coloured mats from lava tubes of La Palma (Canary Islands, Spain) are dominated by metabolically active Actinobacteria. Sci. Rep. 8:1944. doi: 10.1038/s41598-018-20393-2
Gruiz, K., Molnár, M., and Fenyvesi, É. (2009). Verification tool for in situ soil remediation. Land Cont. Reclam. 17, 661–684. doi: 10.2462/09670513.982
Guerin, W. F., and Boyd, S. A. (1992). Differential bioavailability of soil sorbed Naphthalene to two bacterial species. Am. Soc. Microbiol. 58, 1142–1152.
Gunasekera, T. S., Radwan, O., Bowen, L. L., Brown, L. M., and Ruiz, O. N. (2018). Draft genome sequence of Achromobacter spanius strain 6, a soil bacterium isolated from a hydrocarbon degrading microcosm. Microbiol. Resour. Announc. 7:e1124-18. doi: 10.1128/MRA.01124-18
Guo, G., Tian, F., Ding, K., Wang, L., Liu, T., and Yang, F. (2017). Effect of a bacterial consortium on the degradation of polycyclic aromatic hydrocarbons and bacterial community composition in Chinese soils. Int. Biodeterior. Biodegradation 123, 56–62. doi: 10.1016/j.ibiod.2017.04.022
Haleyur, N., Shahsavari, E., Taha, M., Khudur, L. S., Koshlaf, E., Osborn, A. M., et al. (2018). Assessing the degradation efficacy of native PAH-degrading bacteria from aged, weathered soils in an Australian former gasworks site. Geoderma 321, 110–117. doi: 10.1016/j.geoderma.2018.02.004
Haritash, A. K., and Kaushik, C. P. (2009). Biodegradation aspects of polycyclic aromatic hydrocarbons (PAHs): a review. J. Hazard. Mater. 169, 1–15. doi: 10.1016/j.jhazmat.2009.03.137
Isaac, P., Alessandrello, M. J., Macedo, A. J., Estévez, M. C., and Ferrero, M. A. (2017). Pre-exposition to polycyclic aromatic hydrocarbons (PAHs) enhance biofilm formation and hydrocarbon removal by native multi-species consortium. J. Environ. Chem. Eng. 5, 1372–1378. doi: 10.1016/j.jece.2017.02.031
Janbandhu, A., and Fulekar, M. H. (2011). Biodegradation of phenanthrene using adapted microbial consortium isolated from petrochemical contaminated environment. J. Hazard. Mater. 187, 333–340. doi: 10.1016/j.jhazmat.2011.01.034
Juhasz, A. L., Stanley, G. A., and Britz, M. L. (2000). Microbial degradation and detoxification of high molecular weight polycyclic aromatic hydrocarbons by Stenotrophomonas maltophilia strain VUN 10,003. Lett. Appl. Microbiol. 30, 396–401. doi: 10.1046/j.1472-765x.2000.00733.x
Kafilzadeh, F., Sahragard, P., Jamali, H., and Tahery, Y. (2011). Isolation and identification of hydrocarbons degrading bacteria in soil around Shiraz Refinery. Afr. J. Microbiol. Res. 5, 3084–3089. doi: 10.5897/AJMR11.195
Kauppi, S., Sinkkonen, A., and Romantschuk, M. (2011). Enhancing bioremediation of diesel-fuel-contaminated soil in a boreal climate: comparison of biostimulation and bioaugmentation. Inter. Biodeterior. Biodegradation 65, 359–368. doi: 10.1016/j.ibiod.2010.10.011
Kong, D., Xia, Q., Liu, G., Huang, Q., and Lu, J. (2013). Covalent bonding of chloroanilines to humic constituents: pathways, kinetics, and stability. Environ. Pollut. 180, 48–54. doi: 10.1016/j.envpol.2013.05.018
Kostka, J. E., Prakash, O., Overholt, W. A., Green, S. J., Freyer, G., Canion, A., et al. (2011). Hydrocarbon-degrading bacteria and the bacterial community response in Gulf of Mexico beach sands impacted by the deepwater horizon oil spill. Appl. Environ. Microbiol. 77, 7962–7974. doi: 10.1128/AEM.05402-11
Kumar, S., Stecher, G., and Tamura, K. (2016). MEGA7: molecular Evolutionary Genetics Analysis version 7.0 for bigger datasets. Mol. Biol. Evol. 33, 1870–1874. doi: 10.1093/molbev/msw054
Kumari, S., Regar, R. K., and Manickam, N. (2018). Improved polycyclic aromatic hydrocarbon degradation in a crude oil by individual and a consortium of bacteria. Bioresour. Technol. 254, 174–179. doi: 10.1016/j.biortech.2018.01.075
Labbé, D., Margesin, R., Schinner, F., Whyte, L. G., and Greer, W. (2007). Comparative phylogenetic analysis of microbial communities in pristina and hydrocarbon-contaminated Alpine soils. FEMS Microbiol. Ecol. 59, 446–475. doi: 10.1111/j.1574-6941
Lopes, P. R. M., Montagnolli, R. N., Cruz, J. M., Claro, E. M. T., and Bidoia, E. D. (2019). “Biosurfactants in improving bioremediation effectiveness in environmental contamination by hydrocarbons,” in Microbial Action on Hydrocarbons, Chap, eds V. Kumar, M. Kumar, and R. Prasad, (Singapore: Springer), 21–34.
Ma, Y. L., Lu, W., Wan, L. L., and Luo, N. (2015). Elucidation of fluoranthene degradative characteristics in a newly isolated Achromobacter xylosoxidans DN002. Appl. Biochem. Biotechnol. 175, 1294–1305. doi: 10.1007/s12010-014-1347-7
Maddelaa, N. R., Scalvenzic, L., and Venkateswarlud, K. (2016). Microbial degradation of total petroleum hydrocarbons in crude oil: a field-scale study at the low-land rainforest of Ecuador. Environ. Technol. 38, 2543–2550. doi: 10.1080/09593330.2016.1270356
Madrid, F., Rubio-Bellido, M., Villaverde, J., Peña, A., and Morillo, E. (2019). Natural and assisted dissipation of polycyclic aromatic hydrocarbons in a long-term co-contaminated soil with creosote and potentially toxic elements. Sci. Total Environ. 660, 705–714. doi: 10.1016/j.scitotenv.2018.12.376
Madrid, F., Rubio-Bellido, M., Villaverde, J., Tejada, M., and Morillo, E. (2016). Natural attenuation of fluorene and pyrene in contaminated soils and assisted with hydroxypropyl-β-cyclodextrin. Effect of co-contamination. Sci. Total Environ. 571, 42–49. doi: 10.1016/j.scitotenv.2016.07.110
Martin, F., Torelli, S., Le Paslier, D., Barbance, A., Martin-Laurent, F., Bru, D., et al. (2012). Betaproteobacteria dominance and diversity shifts in the bacterial community of a PAH-contaminated soil exposed to phenanthrene. Environ. Pollut. 162, 345–353. doi: 10.1016/j.envpol.2011.11.032
Molina, M. C., González, N., Fernando Bautista, L., Sanz, R., Simarro, R., Sánchez, I., et al. (2009). Isolation and genetic identification of PAH degrading bacteria from a microbial consortium. Biodegradation 20, 789–800. doi: 10.1007/s10532-009-9267-x
Morillo, E., Sánchez-Trujillo, M. A., Moyano, J. R., Villaverde, J., Gómez-Pantoja, M. E., and Pérez-Martínez, J. I. (2012). Enhanced solubilisation of six PAHs by three synthetic cyclodextrins for remediation applications: molecular modelling of the inclusion complexes. PLoS One 7:e44137. doi: 10.1371/journal.pone.0044137
Morillo, E., Sánchez-Trujillo, M. A., Villaverde, J., Madrid, F., and Undabeytia, T. (2014). Effect of contact time and the use of hydroxypropyl-β-cyclodextrin in the removal of fluorene and fluoranthene from contaminated soils. Sci. Total. Environ. 496, 144–154. doi: 10.1016/j.scitotenv.2014.07.027
Morillo, E., and Villaverde, J. (2017). Advanced technologies for the remediation of pesticide-contaminated soils. Sci. Total Environ. 586, 576–597. doi: 10.1016/j.scitotenv.2017.02.020
Mrozik, A., and Piotrowska-Seget, Z. (2010). Bioaugmentation as a strategy for cleaning up of soils contaminated with aromatic compounds. Microbiol. Res. 165, 363–375. doi: 10.1016/j.micres.2009.08.001
Nowak, A., and Mrozik, A. (2018). Degradation of 4-chl orophenol and microbial diversity in soil inoculated with single Pseudomonas sp. CF600 and Stenotrophomonas maltophilia KB2. J. Environ. Manag. 215, 216–229. doi: 10.1016/j.jenvman.2018.03.052
Ntougias, S., Fasseas, C., and Zervakis, G. I. (2007). Olivibacter sitiensis gen. nov., sp. nov., isolated from alkaline olive-oil mill wastes in the region of Sitia. Crete. Int. J. Syst. Evol. Microbiol. 57, 398–404. doi: 10.1099/ijs.0.64561-0
Ntougias, S., Lapidus, A., Han, J., Mavromatis, K., Pati, A., Chen, A., et al. (2015). High quality draft genome sequence of Olivibacter sitiensis type strain (AW-6T), a diphenol degrader with genes involved in the catechol pathway. Stand. Genomic Sci. 9, 783–793. doi: 10.4056/sigs.5088950
Nzila, A., Ramirez, C. O., Musa, M. M., Sankara, S., Basheer, C., and Li, Q. X. (2018). Pyrene biodegradation and proteomic analysis in Achromobacter xylosoxidans PY4 strain. Int. Biodeterior. Biodegradation 130, 40–47. doi: 10.1016/j.ibiod.2018.03.014
Paul, D., Pandey, G., Pandey, J., and Jain, R. K. (2005). Accessing microbial diversity for bioremediation and environmental restoration. Trends Biotechnol. 23, 135–142. doi: 10.1016/j.tibtech.2005.01.001
Prince, R. C. (2018). “Eukaryotic hydrocarbon degraders,” in Taxonomy, Genomics and Ecophysiology of Hydrocarbon-Degrading Microbes, Handbook of Hydrocarbon and Lipid Microbiology, ed. T. J. McGenity (Switzerland: Springer International Publishing AG, part of Springer Nature 2018). doi: 10.1007/978-3-319-60053-6_16-1
Ran, Y., Yang, Y., Xing, B., Pignatello, J. J., Kwon, S., Su, W., et al. (2013). Evidence of microspore filling for sorption of nonpolar organic contaminants by condensed organic matter. J. Environ, Qual. 42, 806–814. doi: 10.2134/jet2012.0286
Reid, B. J., Jones, K. C., and Semple, K. T. (2000). Bioavailability of persistent organic pollutants in soils and sediments a perspective on mechanisms, consequences and assessment. Environ. Pollut. 108, 103–112. doi: 10.1016/S0269-7491(99)00206-7
Reid, B. J., Northcott, G. L., Jones, K. C., and Semple, K. T. (1998). Evaluation of spiking procedures for the introduction of poorly water soluble contaminants into soil. Environ. Sci. Technol. 32, 3224–3227. doi: 10.1021/es9800941
Rubio-Bellido, M., Morillo, E., and Villaverde, J. (2016). Effect of addition of HPBCD on diuron adsorption–desorption, transport and mineralization in soils with different properties. Geoderma 265, 196–203. doi: 10.1016/j.geoderma.2015.11.022
Rubio-Bellido, M., Morillo, E., and Villaverde, J. (2018). Assessment of soil diuron bioavailability to plants and microorganisms through non-exhaustive chemical extractions of the herbicide. Geoderma 312, 130–138. doi: 10.1016/j.geoderma.2017.09.031
Saitou, N., and Nei, M. (1987). The neighbor-joining method: a new method for reconstructing phylogenetic trees. Mol. Biol. Evol. 4, 406–425.
Sánchez-Trujillo, M. A., Lacorte, S., Villaverde, J., Barata, C., and Morillo, E. (2014). Decontamination of polycyclic aromatic hydrocarbons and nonylphenol from sewage sludge using hydroxypropyl-β-cyclodextrin and evaluation of the toxicity of leachates. Environ. Sci. Pollut. Res. 21, 507–517. doi: 10.1007/s11356-013-1930-4
Sánchez-Trujillo, M. A., Morillo, E., Villaverde, J., and Lacorte, S. (2013). Comparative effects of several cyclodextrins on the extraction of PAHs from an aged contaminated soil. Environ. Pollut. 178, 52–58. doi: 10.1016/j.envpol.2013.02.029
Schippers, A., Bosecker, K., Spröer, C., and Schumann, P. (2005). Microbacterium oleivorans sp. nov. and Microbacterium hydrocarbonoxydans sp. nov., novel crude-oil-degrading gram-positive bacteria. Int. J. Syst. Evol. Microbiol. 55, 655–660. doi: 10.1099/ijs.0.63305-0
Serrano, A., Tejada, M., Gallego, M., and Gonzalez, J. L. (2009). Evaluation of soil biological activity after a diesel fuel spill. Sci. Total Environ. 407, 4056–4061. doi: 10.1016/j.scitotenv.2009.03.017
Simarro, R., González, N., Bautista, L. F., and Molina, M. C. (2013). Assessment of the efficiency of in situ bioremediation techniquesin a creosote polluted soil: change in bacterial community. J. Hazard. Mater. 262, 158–167. doi: 10.1016/j.jhazmat.2013.08.025
Simpanen, S., Mäkelä, R., Mikola, J., Silvennoinen, J., and Romsntdchuk, M. (2016). Biorremediation of creosote contaminated soil in both laboratory and field scale: Investigating the ability of methyl-ß-cyclodextrin to enhance biostimulation. Int. Biodeterior. Biodegradation 106, 117–126. doi: 10.1016/j.ibiod.2015.10.013
Sopeña, F., Laiz, L., Morillo, E., Sanchez-Trujillo, M. A., Villaverde, J., Jurado, V., et al. (2013). Phenanthrene Biodegradation by Pseudomonas xanthomarina Isolated from an Aged Contaminated Soil. Clean Air Soil Water. 41, 1–6. doi: 10.1002/clen.201300247
Spini, G., Spina, F., Poli, A., Blieux, A.-L., Regnier, T., Gramellini, C., et al. (2018). Molecular and microbiological insights on the enrichment procedures for the isolation of petroleum degrading bacteria and fungi. Front. Microbiol. 9:2543. doi: 10.3389/fmicb.2018.02543
Stroud, J. L., Tzima, M., Paton, G. I., and Semple, K. T. (2009). Influence of hydroxypropyl-β-cyclodextrin on the biodegradation of14C-phenanthrene and 14C-hexadecane in soil. Environ. Pollut. 157, 2678–2683. doi: 10.1016/j.envpol.2009.05.009
Szabó, I., Szoboszlay, S., Kriszt, B., Háhn, J., Harkai, P., Baka, E., et al. (2011). Olivibacter oleidegradans sp. nov., a hydrocarbon degrading bacterium isolated from a biofilter cleanup facility on a hydrocarbon-contaminated site. Int. J. Syst. Evol. Microbiol. 61, 2861–2865. doi: 10.1099/ijs.0.026641-0
Tiwari, B., Manickam, N., Kumari, S., and Tiwari, A. (2016). Biodegradation and dissolution of polyaromatic hydrocarbons by Stenotrophomonas sp. Biores. Technol. 216, 1102–1105. doi: 10.1016/j.biortech.2016.06.047
Tiwari, J. N., Reddy, M. M. K., Patel, D. K., Jain, S. K., Murthy, R. C., and Manickam, N. (2010). Isolation of pyrene degrading Achromobacter xylooxidans and characterization of metabolic product. World J. Microbiol. Biotechnol. 26, 1727–1733. doi: 10.1007/s11274-010-0350-6
Towell, M. G., Bellarby, J., Paton, G. I., Coulon, F., Pollard, S. J. T., and Semple, K. T. (2011). Mineralisation of target hydrocarbons in three contaminated soils from former refinery facilities. Environ. Pollut. 159, 515–523. doi: 10.1016/j.envpol.2010.10.015
U. S. Environmental Protection Agency. (2008). EPA’S Report On the Environment (ROE). Washington, DC: U.S. Environmental Protection Agency.
Villaverde, J., Posada-Baquero, R., Rubio-Bellido, M., Laiz, L., Saiz-Jimenez, C., Sanchez-Trujillo, M. A., et al. (2012). Enhanced mineralization of diuron using a cyclodextrin-based bioremediation technology. J. Agric. Food Chem. 60, 9941–9947. doi: 10.1021/jf3021909
Villaverde, J., Rubio-Bellido, M., Lara-Moreno, A., Merchán, F., and Morillo, E. (2018a). Combined use of microbial consortia isolated from different agricultural soils and cyclodextrin as a bioremediation technique for herbicide contaminated soils. Chemosphere 193, 118–125. doi: 10.1016/j.chemosphere.2017.10.172
Villaverde, J., Rubio-Bellido, M., Merchán, F., and Morillo, E. (2018b). Bioremediation of diuron contaminated soils by a novel degrading microbial consortium. J. Environ. Manage. 188, 379–386. doi: 10.1016/j.jenvman.2016.12.020
Villaverde, J., Rubio-Bellido, M., Merchán, F., and Morillo, E. (2017). Bioremediation of diuron contaminated soils by a novel degrading microbial consortium. J. Environ. Manage. 188, 379–386. doi: 10.1016/j.jenvman.2016.12.020
Villaverde, J., Rubio-Bellido, M., Posada-Baquero, R., Madrid, F., and Morillo, E. (2013). Hydroxypropyl-β-cyclodextrin-based extraction for diuron bioaccessibility in an artificially contaminated soil. Int. J Environ. Anal. Chem. 93, 1620–1627. doi: 10.1080/03067319.2013.814120
Villaverde, J., Van Beinum, W., Beulke, S., and Brown, C. D. (2009). The kinetics of sorption by retarded diffusion into soil aggregate pores. Environ. Sci. Technol. 43, 8227–8232. doi: 10.1021/es9015052
Wu, M., Dick, W. A., Li, W., Wang, X., Yang, Q., Wang, T., et al. (2016). Bioaugmentation and biostimulation of hydrocarbon degradation and the microbial community in a petroleum-contaminated soil. Int. Biodeterior. Biodegradation 107, 158–164. doi: 10.1016/j.ibiod.2015.11.019
Yu, S. H., Ke, L., Wong, Y. S., and Tam, N. F. Y. (2005). Degradation of polycyclic aromatic hydrocarbons by a bacterial consortium enriched from mangrove sediments. Environ. Int. 31, 149–154. doi: 10.1016/j.envint.2004.09.008
Zafra, G., Absalón, ÁE., Cuevas, M. D. C., and Cortés-Espinosa, D. V. (2014). Isolation and selection of a highly tolerant microbial consortium with potential for PAH biodegradation from heavy crude oil-contaminated soils. Water Air Soil Pollut. 225:1826.
Zhao, D., Liu, C., Liu, L., Zhang, Y., Liu, Q., and Wu, W. M. (2011). Selection of functional consortium for crude oil-contaminated soil remediation. Int. Biodeterior. Biodegradation 65, 1244–1248. doi: 10.1016/j.ibiod.2011.07.008
Zhao, H. P., Wu, Q. S., Wang, L., Zhao, X. T., and Gao, H. W. (2009). Degradation of phenanthrene by bacterial strain isolated from soil in oil refinery fields in Shanghai China. J. Hazard. Mater. 164, 863–869. doi: 10.1016/j.jhazmat.2008.08.098
Zhao, Q., Yue, S., Bilal, M., Hu, H., Wang, W., and Zhang, X. (2017). Comparative genomic analysis of 26 Sphingomonas and Sphingobium strains: dissemination of bioremediation capabilities, biodegradation potential and horizontal gene transfer. Sci. Total Environ. 609, 1238–1247. doi: 10.1016/j.scitotenv.2017.07.249
Keywords: soil, bioaugmentation, cyclodextrin, Stenotrophomonas maltophilia, Achromobacter xylosoxidans, PAHs
Citation: Villaverde J, Láiz L, Lara-Moreno A, González-Pimentel JL and Morillo E (2019) Bioaugmentation of PAH-Contaminated Soils With Novel Specific Degrader Strains Isolated From a Contaminated Industrial Site. Effect of Hydroxypropyl-β-Cyclodextrin as PAH Bioavailability Enhancer. Front. Microbiol. 10:2588. doi: 10.3389/fmicb.2019.02588
Received: 15 February 2019; Accepted: 25 October 2019;
Published: 14 November 2019.
Edited by:
Mariusz Cycoń, Medical University of Silesia, PolandReviewed by:
Ying Teng, Institute of Soil Science (CAS), ChinaXinying Zhang, Shanghai University, China
Natalia Gonzalez Benitez, Rey Juan Carlos University, Spain
Copyright © 2019 Villaverde, Láiz, Lara-Moreno, González-Pimentel and Morillo. This is an open-access article distributed under the terms of the Creative Commons Attribution License (CC BY). The use, distribution or reproduction in other forums is permitted, provided the original author(s) and the copyright owner(s) are credited and that the original publication in this journal is cited, in accordance with accepted academic practice. No use, distribution or reproduction is permitted which does not comply with these terms.
*Correspondence: Esmeralda Morillo, bW9yaWxsb0Bpcm5hc2UuY3NpYy5lcw==