- 1Departamento de Biología, División de Ciencias Naturales y Exactas, Campus Guanajuato, Universidad de Guanajuato, Guanajuato, Mexico
- 2Department of Microbiology, University of Szeged, Szeged, Hungary
- 3Laboratorio de Glicobiología Humana y Diagnóstico Molecular, Universidad Autónoma del Estado de Morelos, Cuernavaca, Mexico
- 4MTA-SZTE “Lendület” Mycobiome Research Group, University of Szeged, Szeged, Hungary
Mannans are components of the fungal wall attached to proteins via N- or O-linkages. In Candida albicans, Och1 is an α1,6-mannosyltransferase that adds the first mannose unit to the N-linked mannan outer chain; whereas Pmr1 is an ion pump that imports Mn2+ into the Golgi lumen. This cation is the cofactor of Golgi-resident mannosyltransferases, and thus Pmr1 is involved in the synthesis of both N- and O-linked mannans. Since we currently have limited information about the genetic network behind the Candida tropicalis protein mannosylation machinery, we disrupted OCH1 and PMR1 in this organism. The C. tropicalis pmr1Δ and och1Δ mutants showed increased doubling times, aberrant colony and cellular morphology, reduction in the wall mannan content, and increased susceptibility to wall perturbing agents. These changes were accompanied by increased exposure of both β1,3-glucan and chitin at the wall surface of both mutant strains. Our results showed that O-linked mannans are dispensable for cytokine production by human mononuclear cells, but N-linked mannans and β1,3-glucan are key ligands to trigger cytokine production in a co-stimulatory pathway involving dectin-1 and mannose receptor. Moreover, we found that the N-linked mannan core found on the surface of C. tropicalis och1Δ null mutant was capable of inducing cytokine production; and that a mannan-independent pathway for IL-10 production is present in the C. tropicalis-mononuclear cell interaction. Both mutant strains showed virulence attenuation in the Galleria mellonella and the mouse model of systemic candidiasis. Therefore, mannans are relevant for cell wall composition and organization, and for the C. tropicalis-host interaction.
Introduction
Candidiasis, a superficial or deep-seated infection, is caused by members of the Candida genus, and thus far Candida albicans is the most frequent species isolated from infected tissues. In most of the cases, superficial candidiasis is a benign disease with low morbidity rates; however, systemic candidiasis is a life-threatening condition that is associated with significant rates of morbidity and mortality (Brown et al., 2012). Candida tropicalis is among the causative agents of candidiasis that are usually isolated from lesions. This species is commonly found in tropical countries and causes 33–48% of systemic candidiasis (Kothavade et al., 2010; Wang et al., 2016). It is a regular causative agent of candidiasis in neutropenic patients and in recent years has shown increased resistance to antifungal drugs, in particular to fluconazole (Kothavade et al., 2010; Zuza-Alves et al., 2017).
The secreted macromolecules, the capsule, and the cell wall are the fungal components that participate in the early stages of the host-fungus interaction and are key players in the establishment of an immune response against the fungal pathogen. The cell wall of C. albicans has been thoroughly characterized and significant amount of information is already available about its role during the interaction with components of the immune system (Díaz-Jiménez et al., 2012; Gow and Hube, 2012; Hall and Gow, 2013; Hall et al., 2013; West et al., 2013; Estrada-Mata et al., 2015; Netea et al., 2015; Erwig and Gow, 2016; Navarro-Arias et al., 2016; Perez-Garcia et al., 2016; Hernández-Chávez et al., 2017; Garcia-Carnero et al., 2018). The C. albicans cell wall is composed of chitin, β1,3- and β1,6-glucans that are regarded as structural polysaccharides, localized closer to the plasma membrane, and covered by an outer layer composed of N- and O-linked mannoproteins (Klis et al., 2001). Thus far, we have evidence indicating that most of the cell wall components are engaged with innate immune receptors that signaling involved in cytokine production and phagocytosis (Martinez-Alvarez et al., 2014). The N-linked mannans are recognized by mannose receptor, DC-SIGN, Mincle, dectin-2, and dectin-3 (Netea et al., 2006; Cambi et al., 2008; Wells et al., 2008; Saijo et al., 2010; Zhu et al., 2013); the O-linked mannans interact with TLR4 (Netea et al., 2006), β1,3-glucan with dectin-1 and TLR2 (Brown and Gordon, 2001; Netea et al., 2006), and chitin with the mannose receptor, TLR9, and NOD2 (Wagener et al., 2014).
Since genomic analyses have demonstrated that C. albicans and C. tropicalis are closely related species (Butler et al., 2009), it is assumed the cell wall of both organisms should be similar. So far, it has been reported the presence of chitin, β1,6- and β1,3-glucans, and N-linked mannans that contain lateral chains composed of α1,2- and β1,2-mannose units (Kobayashi et al., 1994; Bizerra et al., 2011; Mesa-Arango et al., 2016; Navarro-Arias et al., 2019). Moreover, the proportion of polysaccharides composing the cell wall is similar for both C. albicans and C. tropicalis (Navarro-Arias et al., 2019). The N-linked mannan outer chain is also modified with phosphomannan, a β1,2-oligomannoside attached to the glycan by a mannosyl-phosphate residue (Kobayashi et al., 1994), which is more abundant in the C. tropicalis cell wall than in C. albicans (Navarro-Arias et al., 2019). In quantitative terms, C. tropicalis has a similar amount of cell wall protein than C. albicans, but higher wall porosity, suggesting shorter mannan branches (Navarro-Arias et al., 2019). Despite the structure has not been described, the C. tropicalis cell wall contains O-linked mannans, which are as abundant as those found in C. albicans (Navarro-Arias et al., 2019). Even though the cell wall structure of C. tropicalis is similar to that described for C. albicans, these subtle differences may lead to differential recognition of both pathogens by components of the immune system. C. tropicalis induces higher levels of pro- and anti-inflammatory cytokines than C. albicans when interacting with human peripheral blood mononuclear cells (PBMCs) (Navarro-Arias et al., 2019), with a strong dependence on dectin-1 engagement with its ligand to induce cytokine production (Duan et al., 2018; Navarro-Arias et al., 2019). In addition, C. tropicalis is more readily phagocytosed by human monocyte-derived macrophages, than C. albicans cells, in a phosphomannan-dependent mechanism (Hernandez-Chavez et al., 2018; Navarro-Arias et al., 2019). When C. tropicalis and C. albicans interact with dendritic cells, only the former is capable of inducing the formation of some fungipods (Neumann and Jacobson, 2010). In contrast with our current knowledge in the C. albicans-host interaction, the protection against the systemic disease caused by C. tropicalis does not require IL-17 signaling but the CARD9-dependent production of TNF-α that enhances the antifungal ability of neutrophils (Whibley et al., 2015).
Besides the importance of the immune cell-Candida interaction, mannans are key players in maintaining the cell wall integrity, cellular and colonial morphology, as well as in determining biofilm formation and virulence (Bates et al., 2005, 2006, 2013; Munro et al., 2005; Prill et al., 2005; Mora-Montes et al., 2007, 2010; Hall et al., 2013; West et al., 2013; Estrada-Mata et al., 2015; Navarro-Arias et al., 2016, 2017; Perez-Garcia et al., 2016).
The Golgi-resident P-type ATPase (EC: 7.2.2.10), Pmr1, is an ion pump that imports the mannosyltransferase cofactor Mn2+ into the Golgi lumen, allowing proper modification of both N- and O-linked mannans by Golgi-resident mannosyltransferases (Bates et al., 2005). In Botrytis cinerea, C. albicans and C. guilliermondii, disruption of PMR1 affected the cell wall composition and proper elongation of both N- and O-linked mannans (Bates et al., 2005; Plaza et al., 2015; Navarro-Arias et al., 2016) and thus, the Candida null mutants stimulated poor cytokine production by human PBMCs and dendritic cells, reduced uptake by macrophages, and showed virulence attenuation (Netea et al., 2006; Cambi et al., 2008; McKenzie et al., 2010; Navarro-Arias et al., 2016).
The OCH1 encodes a Golgi-resident α1,6-mannosyl- transferase (EC: 2.4.1.232) that primes the elaboration of the N-linked mannan outer chain (Martinez-Duncker et al., 2014). Loss of this gene in both C. albicans and C. parapsilosis increased the sensitivity to cell wall perturbing agents, affected the cell wall composition, the ability to stimulate cytokine production by human PMBCs and dendritic cells, and the uptake by macrophages (Bates et al., 2006; Netea et al., 2006; Cambi et al., 2008; McKenzie et al., 2010; Perez-Garcia et al., 2016). Similar to the pmr1Δ null mutants, the och1Δ mutants showed virulence attenuation, but only defects in the structure of N-linked mannans, with normal O-linked mannans decorating the cell surface (Bates et al., 2006; Perez-Garcia et al., 2016). Besides the genetic approach to assess the relevance of mannans in the fungal biology, the chemical removal of O-linked mannans by β-elimination or the enzymatic trimming of N-linked mannans with endoglycosidase H (endo H) have positively impacted in our current knowledge about the versatile functions of mannans in the biology of Candida spp. and other fungal species (Hamada et al., 1981; Hazen and Glee, 1994; Mormeneo et al., 1994; Goins and Cutler, 2000; Spreghini et al., 2003; Navarro-Arias et al., 2016, 2017, 2019; Perez-Garcia et al., 2016; Martinez-Alvarez et al., 2017; Lozoya-Perez et al., 2019).
Here, to assess the relevance of mannans in the biology of C. tropicalis, we generated single null mutants in the genes OCH1 and PMR1 and conducted the phenotypical characterization with an emphasis on the cell wall composition and status of the protein glycosylation pathways. In addition, the ability to stimulate cytokine production by human PBMCs, and the virulence in both mouse and Galleria mellonella models were evaluated.
Results
Identification and Disruption of Candida tropicalis PMR1 and OCH1
The C. tropicalis PMR1 and OCH1 sequences were identified following a standard BLAST analysis at the NCBI website1, using the protein sequences of C. albicans Pmr1 (GenBank accession code XP_720380) and Och1 (GenBank accession code AOW28617) as a query. The putative ortholog of C. albicans Pmr1 was the product encoded by the locus EER31186 (GenBank accession code EER31186). The open reading frame (ORF) spans 2760 bp with no putative introns identified and is predicted to encode a polypeptide of 919 amino acids, with 88 and 95% identity and similarity to C. albicans Pmr1, respectively. The putative protein is predicted to fold in nine transmembrane domains, to contain the canonical motif 352DKTGTLT that includes the aspartic acid involved in the phosphorylation of P-type ATPases (Lutsenko and Kaplan, 1995; Bates et al., 2005), the type A and C cation transport domains, and a putative dehalogenase domain; all these traits already identified in the C. albicans Pmr1 (Bates et al., 2005). For the case of OCH1, the putative ortholog of C. albicans Och1 was the gene product of the locus EER33436 (GenBank accession code EER33436), whose ORF spans 1131 bp with no intron predicted. The encoded protein spans 376 amino acids, with 72 and 85% identity and similarity to C. albicans Och1, respectively, is predicted to be a type-II transmembrane protein belonging to the glycosyltransferase family 32, and contains the sequence 180DXD, a common signature sequence of proteins that use divalent cations, such as Mn2+ as a cofactor during the transference of monosaccharides from a nucleotide-activated sugar to oligo and polysaccharides (Bates et al., 2006). To test these hypotheses generated by bioinformatic means, we next complemented C. albicans pmr1Δ and och1Δ null mutants (Bates et al., 2005, 2006) with the corresponding C. tropicalis ORFs under the control of the strong C. albicans ACT1 promoter (Barelle et al., 2004). As previously reported (Bates et al., 2005, 2006), the C. albicans pmr1Δ and och1Δ null mutants showed defects in the cell wall composition, with low mannan and phosphomannan content and increased glucan levels (Table 1). Chitin content was only modified in the och1Δ null mutant but not in pmr1Δ cells (Table 1). Moreover, in line with the reduction in mannan content, both mutant strains showed increased cell wall porosity (Table 1). Expression of C. tropicalis PMR1 or OCH1 in the corresponding C. albicans null mutant restored this phenotype to levels comparable to those found in the wild-type (WT) control strain (Table 1). Collectively, these data suggest that C. tropicalis PMR1 and OCH1 are the functional orthologs of C. albicans PMR1 and OCH1, respectively.
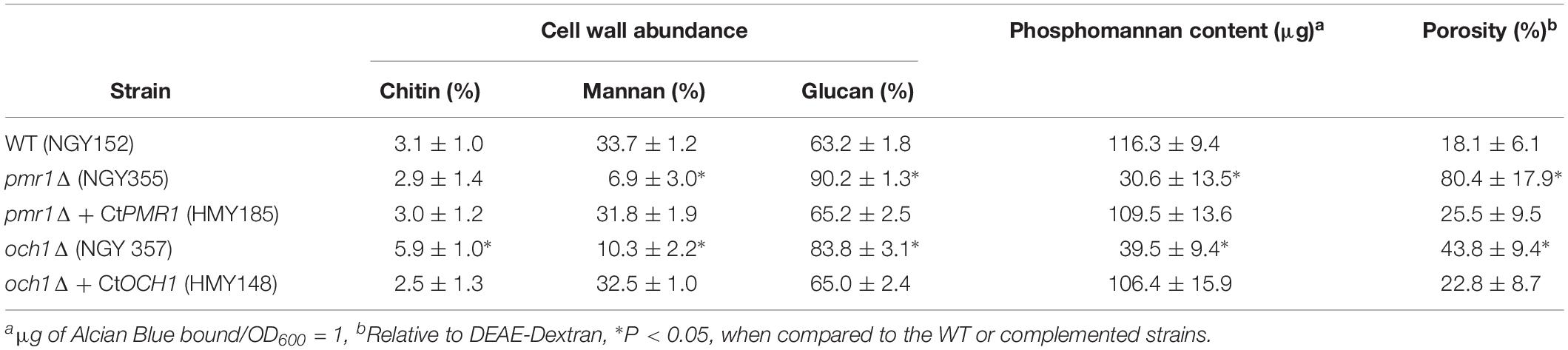
Table 1. Complementation of Candida albicans pmr1Δ and och1Δ null mutants with the functional orthologs of Candida tropicalis.
Next, to generate a C. tropicalis strain lacking either PMR1 or OCH1 the SATI flipper methodology was used to disrupt both alleles of these two genes. The disruption cassettes were constructed in the pSFS2 plasmid (Reuß et al., 2004) and contained 1500 bp regions upstream and downstream the targeted ORF for homologous recombination. For both genes, disruption of the first allele was confirmed by PCR, cells underwent marker recycling, used in the second round of transformation with the same disruption cassette, and again transformation marker recycling, generating the pmr1Δ and the och1Δ null mutants (Table 2). To generate reintegrant control strains, either PMR1 or OCH1 ORF plus regulatory regions were cloned into pSFS2 and this construction used to transform the pmr1Δ or och1Δ null mutant, respectively. The integration of constructions into the targeted loci was confirmed by PCR (data not shown).
Both the pmr1Δ and och1Δ null mutants showed defects in the cell morphology, forming cell aggregates, which were more prominent in the former (Figure 1). The reintegration of one allele of the disrupted gene reverted the phenotype of the null mutant strains, with a cell morphology similar to that displayed by the WT control strain (Figure 1). In addition, both mutant strains showed defects in the colony morphology when grown on a solid culture medium, forming colonies with irregular borders and a wrinkled surface (Figure 1). Again, the reintegrant control strains showed a phenotype similar to the WT strain (Figure 1). These changes were accompanied by changes in the doubling time at 28°C, with the null mutant strains tending to grow slower than the WT and reintegrant control strains (doubling time of the WT control strain 1.49 ± 0.19 h, pmr1Δ strain 2.25 ± 0.24 h, pmr1Δ + PMR1 strain 1.47 ± 0.25 h, och1Δ strain 2.22 ± 0.27 h, and och1Δ + OCH1 strain 1.52 ± 0.12 h, p < 0.05 when compared the null mutant strain with the WT strain or the corresponding reintegrant strain), and both the pmr1Δ and och1Δ null mutants, but not the WT or reintegrant control strains, were unable to grow at 42°C (data not shown).
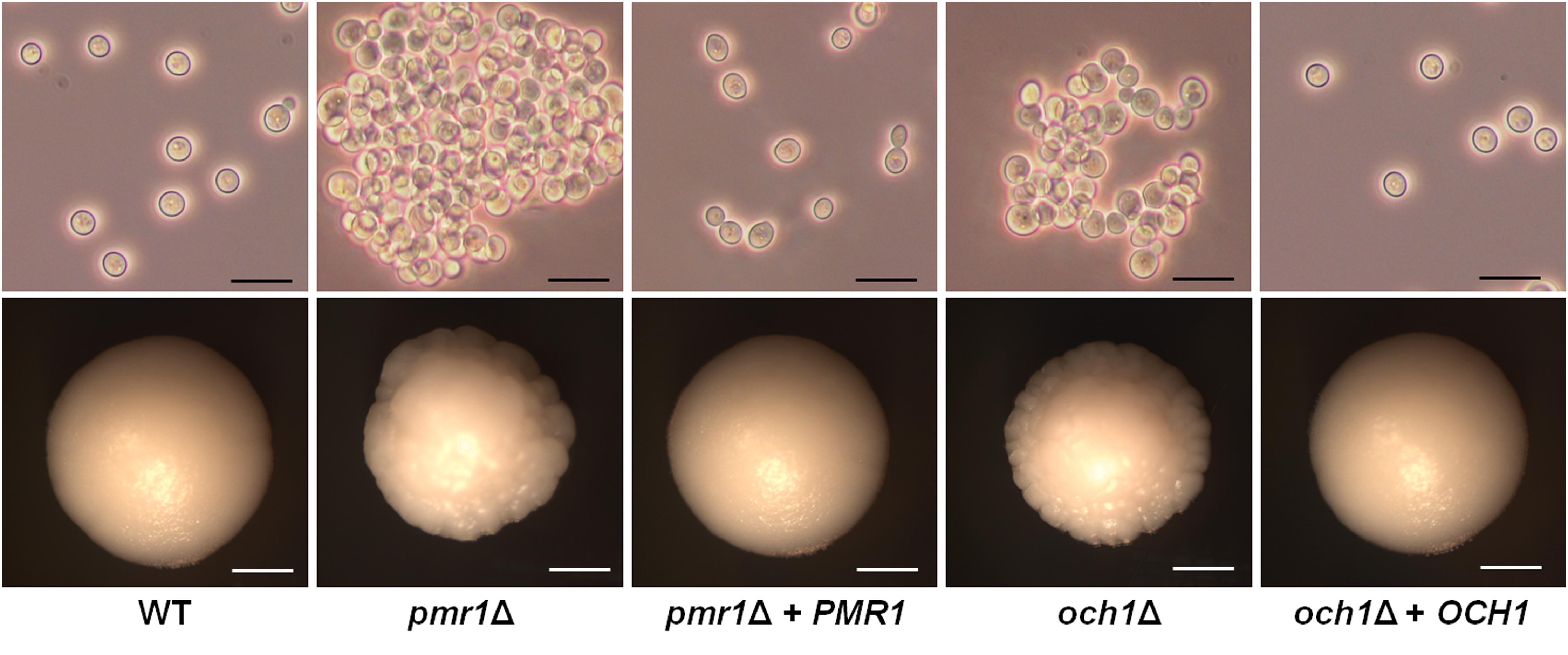
Figure 1. Cell and colony morphology of Candida tropicalis pmr1Δ and och1Δ null mutants. The upper panels show the cell morphology after growth at 28°C for 15 h in YPD medium, demonstrating cell aggregates in both null mutant strains. Scale bar, 10 μm. The lower panels show colony morphology after 3 days of growth at 28°C on YPD plates. Both null mutants formed colonies with irregular borders and a wrinkled surface. Scale bar, 1 mm. Strains used are MYA-3404 (WT), HMY207 (pmr1Δ), HMY208 (pmr1Δ + PMR1), HMY181 (och1Δ), and HMY205 (och1Δ + OCH1).
The Candida tropicalis pmr1Δ and och1Δ Null Mutants Have Defects in the Cell Wall and Protein Glycosylation Pathways
To assess the role of either PMR1 or OCH1 in the C. tropicalis wall composition, cell walls were isolated, cleaned, acid-hydrolyzed, and analyzed by High-Performance Anion-Exchange Chromatography with Pulsed Amperometric Detection (HPAEC-PAD). The cell wall composition of the WT control strain was similar to that recently reported for C. tropicalis, with a large amount of glucan, followed by mannan, and a small proportion of chitin (Hernandez-Chavez et al., 2018; Table 3). The pmr1Δ null mutant showed about 10-fold more chitin content than the WT control strain, a significant 5-fold reduction in the mannan levels, and no changes in the glucan content (Table 3). The och1Δ null mutant showed a 3-fold reduction in the mannan content, no changes in the chitin levels and a significant 1.3-fold increment in the glucan content (Table 3). Interestingly, the changes in the content of these three cell wall components were different when both null mutants were compared (Table 3). In both cases, the reintegrant control strains restored the content of these wall components to levels similar to those found in the WT control strain (Table 3). The phosphomannan content and the porosity to DEAE-dextran are wall parameters that have been correlated with defects in the protein glycosylation pathways (Mora-Montes et al., 2007, 2010; Cheng et al., 2011; Navarro-Arias et al., 2016, 2017; Perez-Garcia et al., 2016; Lozoya-Perez et al., 2019). The phosphomannan content was reduced in both null mutants, and this was accompanied by an increment in the cell wall porosity (Table 3). Again, both reintegrant strains showed a phenotype similar to the WT strain (Table 3). To further explore defects in the mannosylation pathways in either the pmr1Δ or the och1Δ null mutant, cells were subjected to treatment with endo H or β-elimination to remove N-linked or O-linked mannans, respectively (Navarro-Arias et al., 2016, 2019; Lozoya-Perez et al., 2019). The N-linked and O-linked mannan content in the WT control strain was similar to that recently reported (Navarro-Arias et al., 2019), with most of the cell wall mannan bound to proteins by N-linkages (Figure 2). The pmr1Δ null mutant showed a low level of both mannans, whereas the och1Δ null mutant had a reduction of N-linked mannans and an increment in the O-linked mannan content (Figure 2). The mannan content was different when both null mutant strains were compared (Figure 2). The reintegrant control strains displayed a phenotype similar to the WT strain, confirming that the defects found in the mannosylation pathways are associated with the disruption of the genes under analysis (Figure 2). To further confirm the decreased content of cell wall mannan in both mutants, we labeled these oligosaccharides with fluorescein isothiocyanate-concanavalin A conjugate (Con A-FITC) and inspected the cells under fluorescent microscopy. The lectin strongly bound to the mannan of the WT cells, but a weak interaction of this with the cell wall of either the pmr1Δ or the och1Δ null mutant was observed, confirming of findings of decreased mannan content in these mutants (Figure 3 and Supplementary Figure S1). The reintegrant control strains showed a similar ability to bind Con A-FITC than the WT cells (Figure 3 and Supplementary Figure S1). Since heat inactivation of cells artificially exposes β1,3-glucan and chitin at the cell wall surface (Gow et al., 2007; Mora-Montes et al., 2011; Estrada-Mata et al., 2015; Navarro-Arias et al., 2016, 2017, 2019; Perez-Garcia et al., 2016; Hernandez-Chavez et al., 2018); we next repeated the experiment but using heat-killed (HK) cells for fluorescent labeling. This cell treatment did not have an impact on the ability of Con A-FITC to bind the cell wall (Figure 3 and Supplementary Figure S1). When these experiments were performed with β-eliminated cells we observed a small reduction in the ability of the lectin to bind cell walls, but this was not significant (P > 0.05; Figure 3). When endo H-treated cells were used for interaction with Con A-FITC there was a significant reduction in the binding of the lectin by both the live and HK WT and reintegrant control cells (Figure 3). In the case of both mutants, the treatment with endo H decreased the binding of Con A-FITC, but this was not significant (Figure 3).
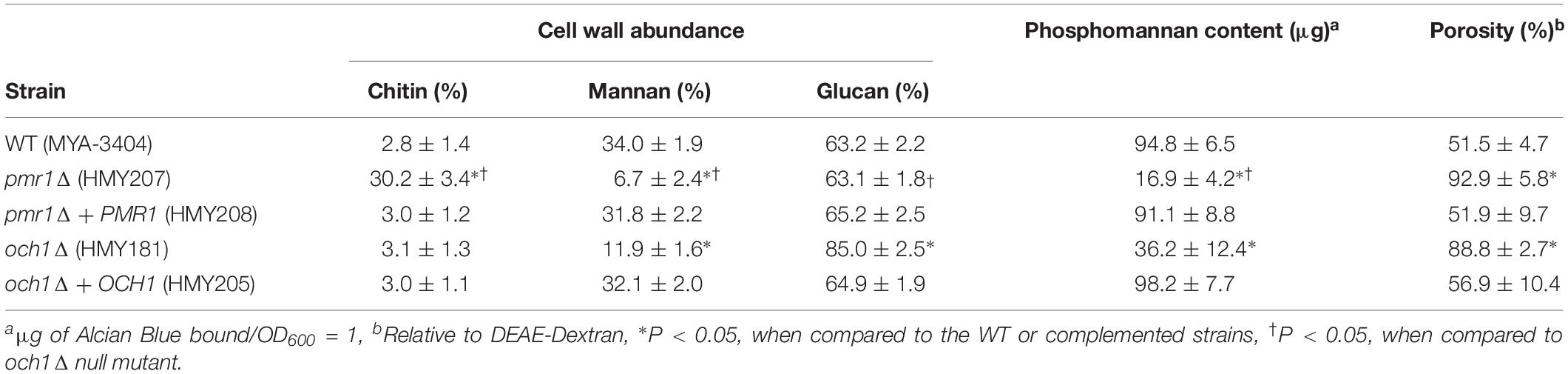
Table 3. Cell wall composition of Candida tropicalis WT, pmr1Δ, och1Δ, and reintegrant control strains.
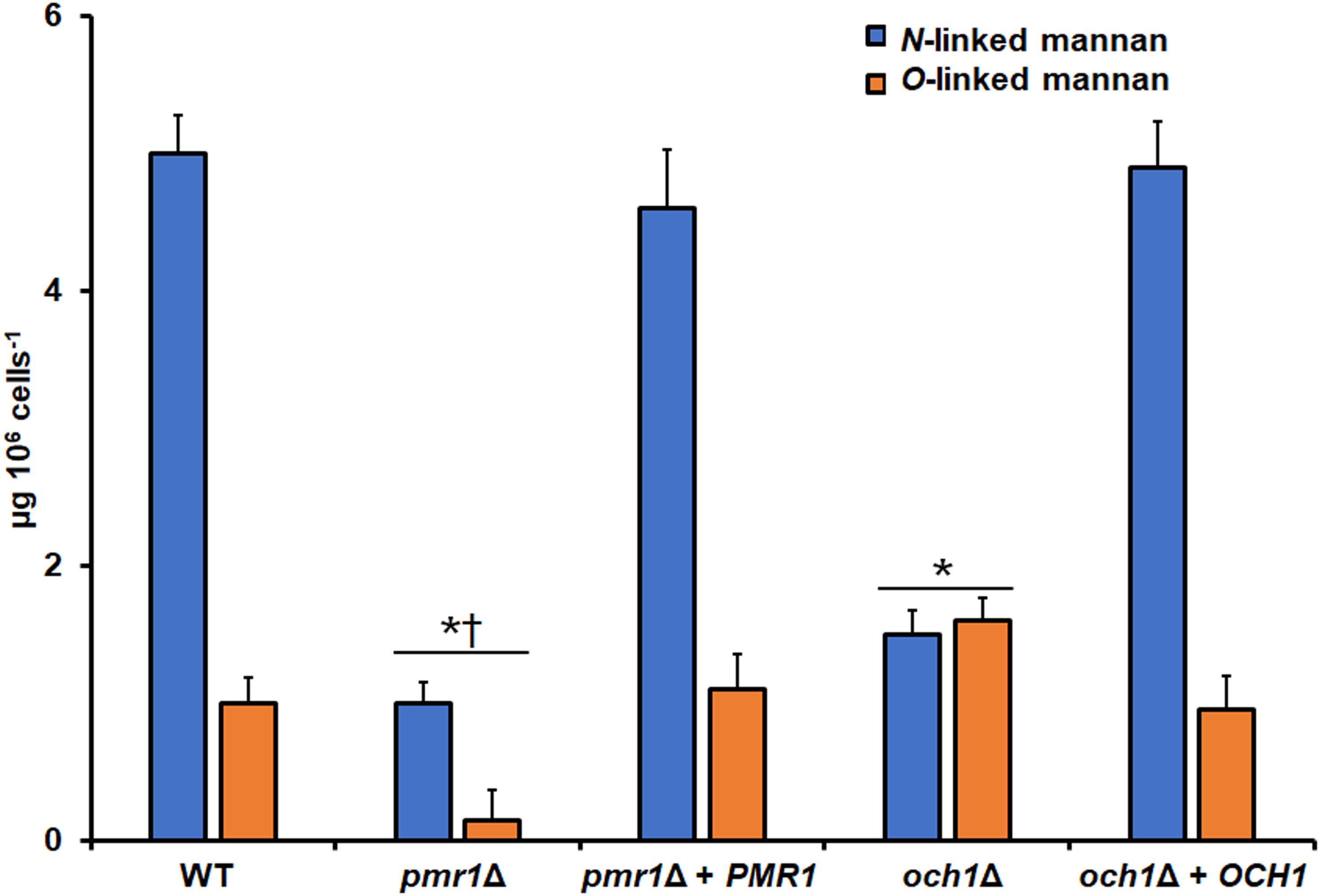
Figure 2. Cell wall mannan content in the Candida tropicalis pmr1Δ and och1Δ null mutants. Cells were treated with endoglycosidase H or β-elimination to remove N-linked mannans or O-linked mannans, respectively, the trimmed oligosaccharides were concentrated and the carbohydrate content was quantified as described in methods. Data are means ± SD of three independent experiments performed in duplicates. Strains used are MYA-3404 (WT), HMY207 (pmr1Δ), HMY208 (pmr1Δ + PMR1), HMY181 (och1Δ), and HMY205 (och1Δ + OCH1). ∗P < 0.05, when compared with either the WT or reintegrant control cells. †P < 0.05, when compared with the och1Δ null mutant.
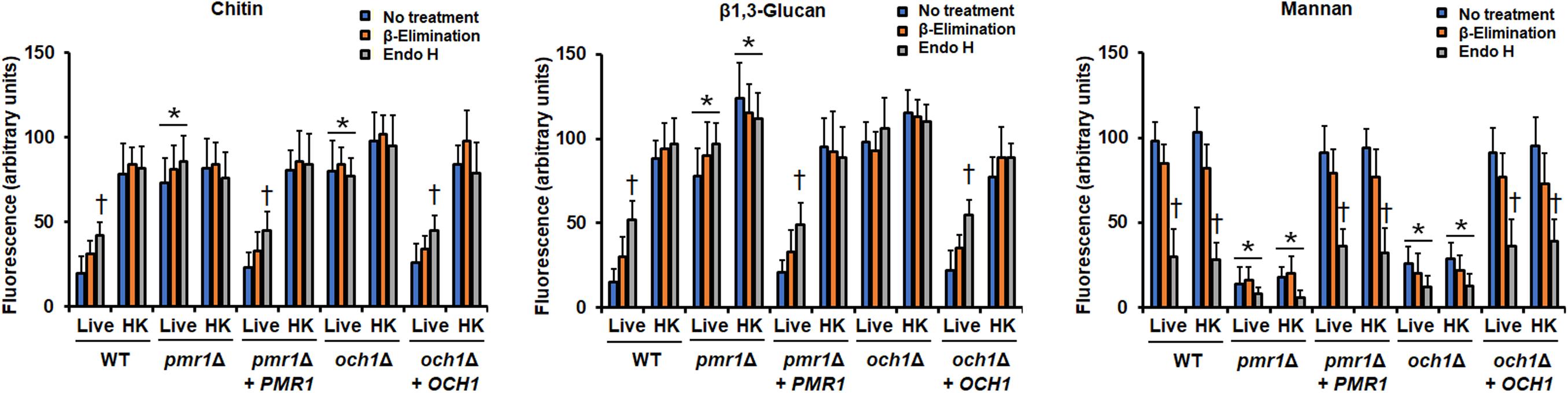
Figure 3. Fluorescent labeling of cell wall chitin, β1,3-glucan, and mannan in the Candida tropicalis pmr1Δ and och1Δ null mutants. Live or heat-killed (HK) yeast cells were labeled with either fluorescein isothiocyanate-wheat germ agglutinin conjugate that binds chitin, IgG Fc-Dectin-1 chimera that binds β1,3-glucan, or fluorescein isothiocyanate-concanavalin A conjugate that binds mannan, as described in the methods, inspected under fluorescence microscopy, and the fluorescence associated to 300 individual cells recorded. In addition, cells were previously β-eliminated or treated with endoglycosidase H (endo H) before labeling with lectins. ∗P < 0.05, when compared with live and HK cells from the WT strain. †P < 0.05, when compared with no treated cells. Strains used are MYA-3404 (WT), HMY207 (pmr1Δ), HMY208 (pmr1Δ + PMR1), HMY181 (och1Δ), and HMY205 (och1Δ + OCH1).
Next, we analyzed the organization of the structural polysaccharides chitin and β1,3-glucan within the cell wall, using fluorescence labeling with fluorescein isothiocyanate-wheat germ agglutinin conjugate (WGA-FITC) and an IgG Fc-Dectin-1 chimera, respectively (Graham et al., 2006; Mora-Montes et al., 2011; Marakalala et al., 2013; Estrada-Mata et al., 2015; Navarro-Arias et al., 2016, 2017, 2019; Hernandez-Chavez et al., 2018). Both lectins barely bound to chitin and β1,3-glucan localized in the cell wall of the WT and the re-integrant control strains (Figure 3 and Supplementary Figures S2, S3). Cells from either the pmr1Δ or the och1Δ null mutant were significantly more labeled than the WT or reintegrant control cells with both lectins, suggesting exposure of chitin and β1,3-glucan at the cell wall surface (Figure 3 and Supplementary Figures S2, S3). The HK WT and reintegrant control cells showed an increased ability to bind both lectins (Figure 3 and Supplementary Figures S2, S3), suggesting that the modest interaction between the lectins and live cells was due to inaccessibility of lectins to polysaccharides, i.e., these are in the inner part of the cell wall. The chitin labeling in both the pmr1Δ and och1Δ null mutants was similar to that observed in live cells, and β1,3-glucan labeling was increased upon heat inactivation (Figure 3). As in the case of mannan labeling, the β-elimination treatment did not affect the chitin or β1,3-glucan labeling (Figure 3). Upon incubation with endo H, the lectin binding to both polysaccharides from live and HK null mutant strains was similar to the untreated cells, but an increased binding to both chitin and β1,3-glucan was observed in the walls of live WT and reintegrant control cells (Figure 3). Collectively these data confirm that both polysaccharides are exposed at the cell surface of the pmr1Δ and och1Δ null mutants. In addition, they are in line with the increment in glucan content quantified by HPAEC-PAD.
It has been previously reported that Candida mutants in either the PMR1 or the OCH1 genes have increased susceptibilities to cell wall perturbing agents (Bates et al., 2005, 2006; Navarro-Arias et al., 2016; Perez-Garcia et al., 2016). Therefore, we analyzed the effect of this kind of compounds on the growth of the C. tropicalis pmr1Δ and och1Δ null mutants. Congo red, a compound that disturbs the glucan within the cell wall (Kopecka and Gabriel, 1992), had a modest effect on the growth of the WT and reintegrant control strains but both the pmr1Δ and och1Δ null mutants showed increased susceptibility to this stressor (Figure 4). The WT and reintegrant control strains showed a dose-dependent increased susceptibility to both Calcofluor white and hygromycin B, and this effect was more prominent in the presence of the latter (Figure 4). Calcofluor white binds to chitin; while increased susceptibility to hygromycin B has been reported in yeast cells with defects in the protein glycosylation pathways (Dean, 1995; Mora-Montes et al., 2011). Again, the pmr1Δ and och1Δ null mutants had increased susceptibility to these perturbing agents (Figure 4). Interestingly, when compared to the ability of both mutants to grow in presence of these three cell wall perturbing agents, the och1Δ null mutant was more affected than the strain were PMR1 was disrupted (P < 0.05 in all cases; Figure 4). Altogether, these data indicate that loss of either PMR1 or OCH1 affects the C. tropicalis protein glycosylation and the cell wall composition, organization, and fitness.
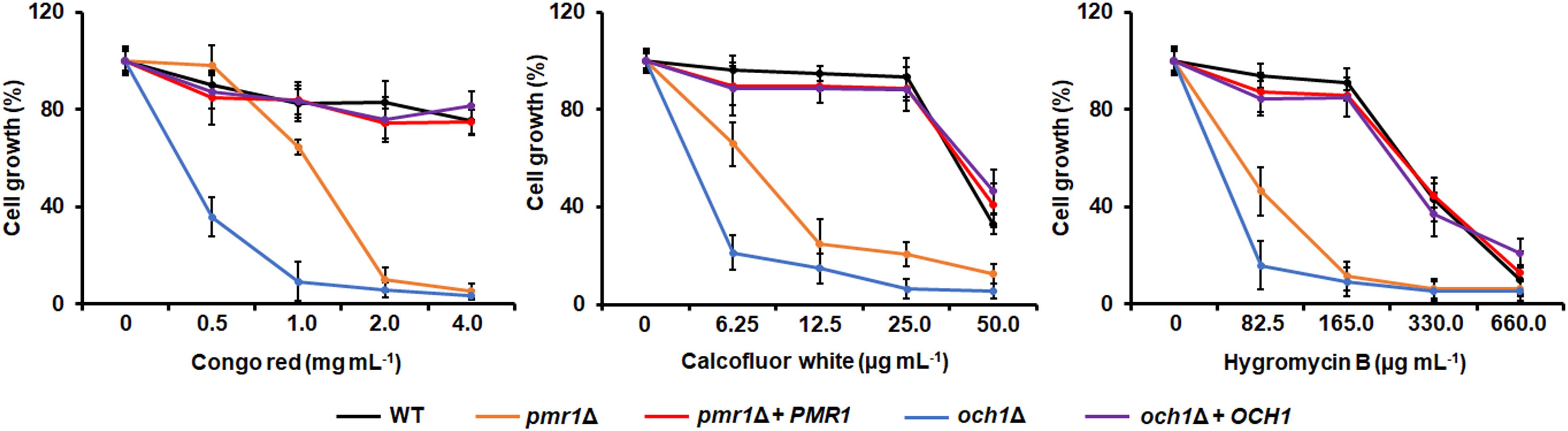
Figure 4. Susceptibility to cell wall perturbing agents in the Candida tropicalis pmr1Δ and och1Δ null mutants. Yeast cells were incubated in YPD broth supplemented with different concentrations of Congo red, Calcofluor white, or Hygromycin B, and cell growth was determined after incubation for 24 h at 30°C. For normalization, growth results are shown as a percentage of those obtained with the same strain grown in the absence of any perturbing agent. Data are means ± SD of three independent experiments performed in duplicates. Both null mutant strains showed increased susceptibility to the three cell wall perturbing agents analyzed, and were significantly different to either the WT or the reintegrant control strain (P < 0.01 when compared by two-way ANOVA). Moreover, the increased susceptibility of the null mutants was different when compared to each other (P < 0.05 when compared by two-way ANOVA). Strains used are MYA-3404 (WT), HMY207 (pmr1Δ), HMY208 (pmr1Δ + PMR1), HMY181 (och1Δ), and HMY205 (och1Δ + OCH1).
Removal of Cell Wall Mannans Affects the Ability of Candida tropicalis to Stimulate Cytokine Production by Human PBMCs
Next, we assessed the importance of cell wall mannan on the C. tropicalis-host interaction, analyzing the ability of this fungal species to stimulate cytokine production by human PBMCs. We first compared the cytokine profile induced by the WT strain treated with endo H or β-elimination, to remove N-linked or O-linked mannans, respectively (Navarro-Arias et al., 2016; Perez-Garcia et al., 2016). Moreover, since in other Candida species β1,3-glucan is one of the major fungal ligands to stimulate cytokine production and is normally buried behind the mannan layer, and therefore inaccessible to engage with dectin-1 (Gow et al., 2007; Estrada-Mata et al., 2015; Navarro-Arias et al., 2016, 2017, 2019; Perez-Garcia et al., 2016), we also compared the ability of live and HK cells to stimulate cytokine production. Live cells stimulated low levels of TNFα, IL-1β, IL-6, and IL-10, but PMBCs interacting with HK cells produced a significantly higher level of these cytokines (Figure 5). The experimental setting where PBMCs were pre-incubated with laminarin and challenged with HK cells stimulated cytokine levels similar to those with the live cells (Figure 5). Thus, the high cytokine levels induced by HK cells were associated with the engagement of dectin-1 with its ligand, as reported for other fungal cells (Estrada-Mata et al., 2015; Navarro-Arias et al., 2016, 2017, 2019; Perez-Garcia et al., 2016). Removal of O-linked mannans via β-elimination did not affect the TNFα, IL-1β, or IL-6 profiles, but live cells with no O-linked mannans on the surface tended to simulate higher IL-10 levels than untreated cells (Figure 5). The trimming of N-linked mannans with endo H only affected the ability of live cells to stimulate IL-10, which was higher, when compared with the levels stimulated with untreated cells (Figure 5). The endo H-treated HK cells were not able to stimulate a strong production of TNFα, IL-1β, or IL-6; while the levels of IL-10 were not affected when compared with those stimulated with the untreated HK cells (Figure 5). It is possible to hypothesize that treatment with endo-H could expose cell wall proteins to the extracellular compartment, promoting detachment of these macromolecules from the wall, accounting for the changes in the ability to stimulate cytokine production. However, these is unlikely, as the wall protein content of cells was not affected upon treatment with endo-H (132.4 ± 21.4, 144.1 ± 17.2, 122.9 ± 13.9, and 136.8 ± 15.6 μg of protein mg of cell wall–1 for live, live + endo H-, HK, HK + endo H-treated cells, respectively).
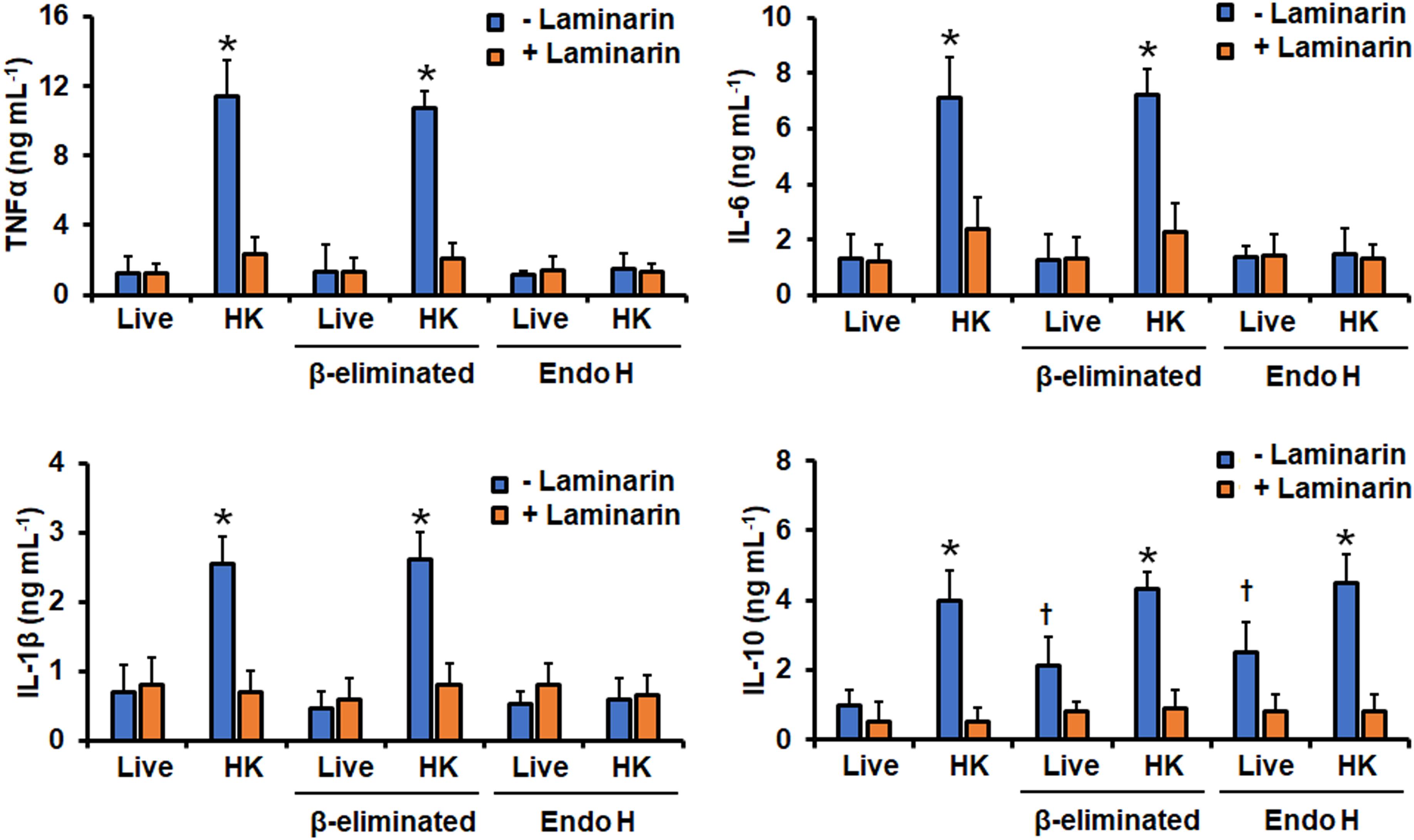
Figure 5. Cytokine production by human PBMCs stimulated with Candida tropicalis. Yeast cells and human PBMCs were co-incubated for 24 h, the supernatant saved and used to quantify the levels of secreted TNFα, IL-6, IL-1β, and IL-10. Results (means ± SD) were obtained using samples from seven donors, each assayed in duplicate wells, and the wild type strain MYA-3404. ∗P < 0.05, when compared with live cells under the same treatment; †P < 0.05, when compared with live cells with no treatment. HK, heat-inactivated cells; Endo-H, endoglycosidase H.
In similar experiments, the ability of the pmr1Δ and och1Δ null mutant to stimulate cytokine production by human PBMCs was also assessed. Live cells of either the pmr1Δ or och1Δ null mutant stimulated higher TNFα, IL-6, IL-1β, and IL-10 when compared with the WT live cells (Figure 6). For the three pro-inflammatory cytokines quantified, the stimulated levels using HK mutant cells were similar to those observed in the system with live cells and not sufficient enough to be comparable to those stimulated with the WT HK cells (Figure 6). For IL-10, the cytokine level stimulated with HK cells was comparable with that observed with live mutant cells, but slightly higher, and therefore statistically similar to the levels stimulated with the WT HK cells (P = 0.627 when WT and och1Δ null mutant cells are compared; P = 0.295 when WT and pmr1Δ null mutant cells are compared). Both live and HK cells of the reintegrant control strains for OCH1 and PMR1 showed an ability to stimulate cytokine production similar to WT control cells (Figure 6). It is worthy of note that it was expected that the cytokine profile stimulated with endo-H-treated WT cells was similar to that observed with cells lacking OCH1 but results from Figures 5, 6 clearly showed this was not the case. Since Och1 is a protein localized within the Golgi complex, it is expected that the N-linked mannan core is properly assembled and transferred to glycoproteins, as demonstrated in C. albicans (Bates et al., 2006). Therefore, the cell surface of an och1Δ null mutant strain will display the N-linked mannan core on the wall surface, which contrasts with the surface of endo-H-treated cells where all the N-linked mannan, including the oligosaccharide core, is absent. Thus, we next compared the ability to stimulate cytokine production of the null mutants subjected to endo H treatment. For both live and HK cells treated with endo H, the levels of the proinflammatory cytokines TNFα, IL-6, and IL-1β were similar to those produced in the system where live untreated cells were included (Figure 6). For IL-10 stimulation, the och1Δ null mutant did not show any difference in the ability to stimulate this cytokine when compared to the system where endo H was not included (Figure 6). In the case of the pmr1Δ null mutant, live cells treated with endo H stimulated significantly higher levels of this cytokine, when compared to the system that not included the glycosidase (Figure 6). The reintegrant control strains for both genes showed a similar ability to stimulate cytokine production to that observed with the WT control cells (Figure 6). To further explore the differences between endo-H-treated WT cells and the och1Δ null mutant we performed interaction experiments in the presence of the N-linked mannan core Man9GlcNAc2 (M9) (Mora-Montes et al., 2004) and measured the cytokine production. Human PBMCs pre-incubated with M9 and then challenged with live WT cells stimulated similar cytokine levels than cells without the preincubation step; however, in similar experiments using HK cells the amount of TNFα, IL-6 and IL-1β was significantly diminished, when compared with the system without M9 (Figure 7 and data not shown). For the case of IL-10, pre-incubation with M9 did not affect the cytokine levels stimulated by either live or HK cells (Figure 7). In the case of both live and HK och1Δ null mutant cells, the levels of TNFα, IL-6, and IL-1β were significantly reduced upon pre-incubation with M9 (Figure 7 and data not shown). As the WT cells, the levels of IL-10 stimulated with either live and HK och1Δ null mutant cells were not affected by pre-incubation with M9 (Figure 7). The reintegrant control strain stimulated similar cytokine levels to those observed with the WT strain (Figure 7). The pmr1Δ null mutant showed a similar ability to stimulate cytokine production than the och1Δ null strain (data not shown). This antagonistic ability of M9 during cytokine production suggested the involvement of mannose receptor (MR) in the PBMC-yeast interaction, as this pattern recognition receptor senses branched oligosaccharides with alpha glycosidic bonds, as M9 (Netea et al., 2006). Therefore, we explored the contribution of this receptor in the C. tropicalis recognition. Human PBMCs preincubated with anti-MR antibodies were challenged with either WT or mutant cells. The anti-MR antibodies did not affect the ability to produce IL-10 but negatively affected the TNFα, IL-6, and IL-1β levels stimulated with HK WT cells (Figure 7 and data not shown). For the case of the och1Δ null mutant, the presence of anti-MR antibodies within the system had little impact on the production of IL-10 but reduced the production of TNFα, IL-6, and IL-1β stimulated with live cells (Figure 7 and data not shown). Although the pro-inflammatory cytokine levels were reduced in the presence of anti-MR and HK cells of the och1Δ null mutant, this was not statistically significant (Figure 7 and data not shown). The control system with an isotype-matched antibody or with the reintegrant control strain shown cytokine levels similar to those stimulated with the WT cells.
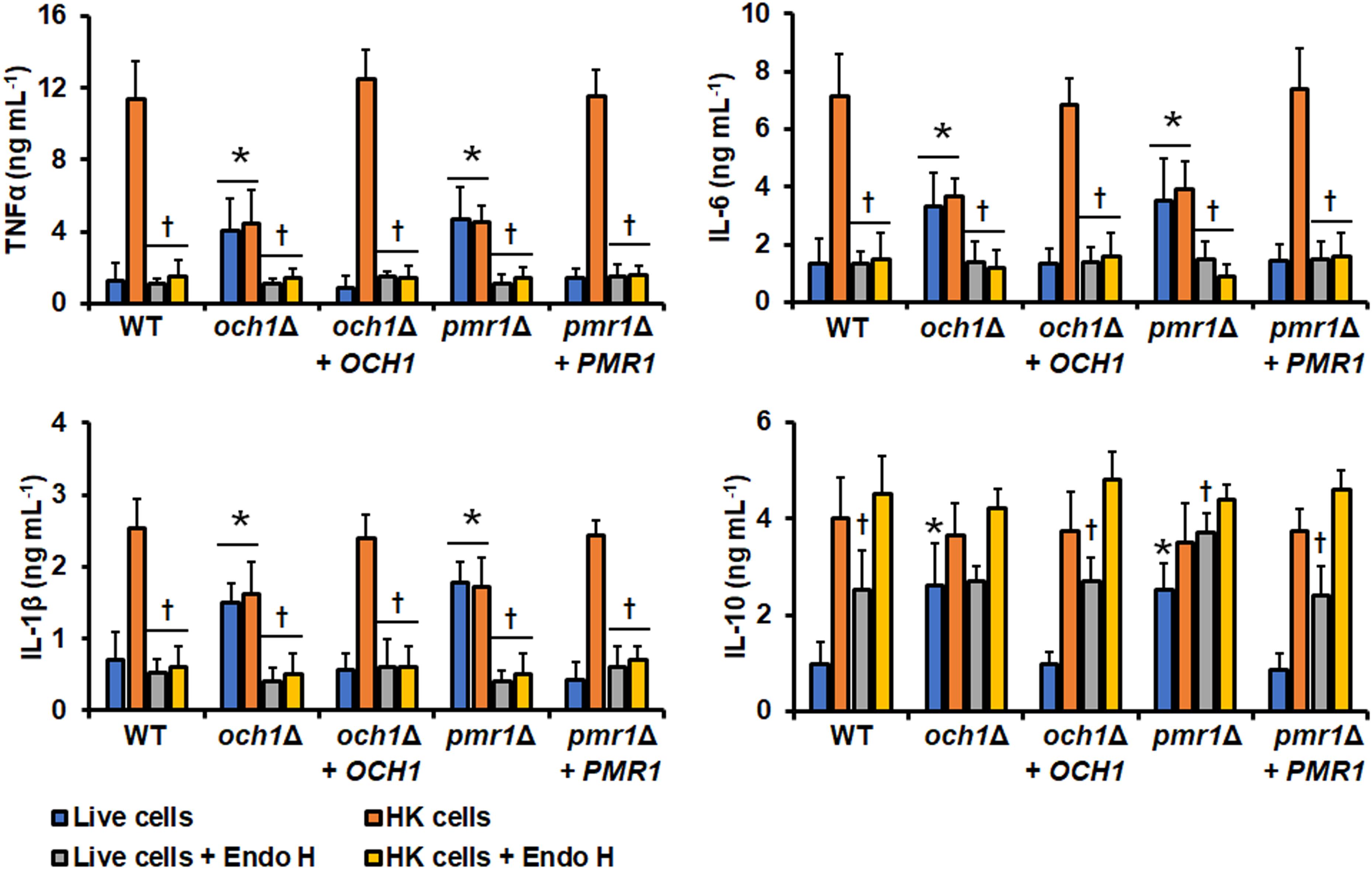
Figure 6. Cytokine production by human PBMCs stimulated with Candida tropicalis och1Δ and pmr1Δ null mutants. Yeast cells and human PBMC were co-incubated for 24 h, the supernatant saved and used to quantify the levels of secreted TNFα, Il-6, IL-1β, and IL-10. Results (means ± SD) were obtained using samples from seven donors, each assayed in duplicate wells. ∗P < 0.05, when compared with WT control cells under the same treatment; †P < 0.05, when compared with cells without treatment with endo H. HK, heat-inactivated cells; Endo-H, endoglycosidase H. Strains used are MYA-3404 (WT), HMY207 (pmr1Δ), HMY208 (pmr1Δ + PMR1), HMY181 (och1Δ), and HMY205 (och1Δ + OCH1).
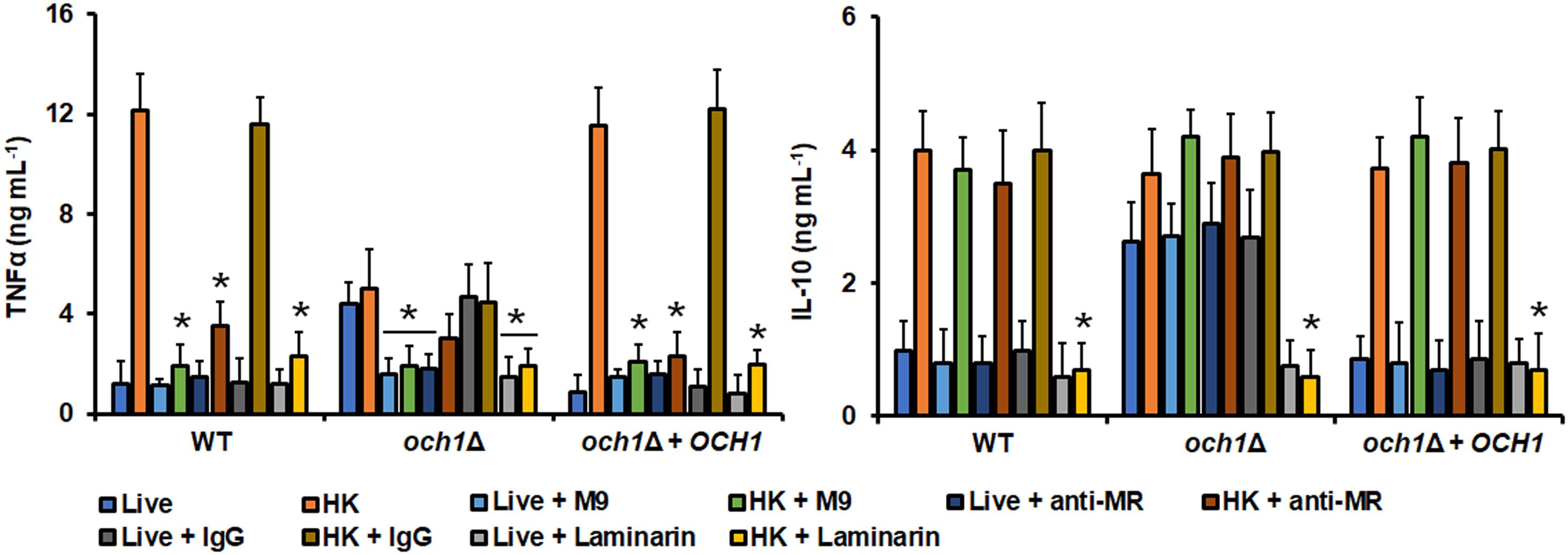
Figure 7. Cytokine production by human PBMCs preincubated with M9, anti-MR, or laminarin and stimulated with the Candida tropicalis och1Δ null mutant. human PBMCs were pre-incubated with M9, anti-MR, or laminarin as described in the Materials and Methods section and then stimulated with either live or heat-killed (HK) yeast cells for 24 h. The supernatant of the interactions was saved and used to quantify cytokine production by ELISA. In the experiments using anti-MR antibodies, control assays with PBMCs pre-incubated with an irrelevant IgG were included as a control. Results (means ± SD) were obtained using samples from seven donors, each assayed in duplicate wells. ∗P < 0.05, when compared with the corresponding live or HK untreated cells. M9, Man9GlcNAc2; MR, mannose receptor. Strains used are MYA-3404 (WT), HMY181 (och1Δ), and HMY205 (och1Δ + OCH1).
Finally, we assessed the contribution of dectin-1 during the interaction with och1Δ null cells. As in the case of the WT strain, the TNFα, IL-6, IL-1β, and IL-10 levels stimulated by either live or HK mutant cells was significantly reduced (Figure 7 and data not shown). Similarly, the cytokine production stimulated by live or HK pmr1Δ cells was negatively affected by pre-incubation with laminarin (data not shown), stressing the importance of dectin-1 engagement with β1,3-glucan during the C. tropicalis-human PBMC interaction.
PMR1 and OCH1 Are Required for Candida tropicalis Virulence in Both Galleria mellonella and the Mouse Model of Systemic Candidiasis
To assess the relevance of protein mannosylation in the C. tropicalis virulence, we first infected larvae of G. mellonella and evaluated the survival rate of these animals. The animals infected with WT and the reintegrant control strains showed similar survival curves, with the total of the animal population dead after 8 days of infection when injected with the WT strain (Figure 8). For the case of the reintegrant strains, the total of the animal population succumbed after 10 days post-infection, and this difference was not statistically significant (Figure 8; P = 0.19 and 0.27, when the curve associated to WT cells is compared with either pmr1Δ + PMR1 or och1Δ + OCH1, respectively). The survival curves of animals infected with either the pmr1Δ or the och1Δ null mutant were similar and showed virulence attenuation, with 50% of the animal population surviving at the end of the observation period (Figure 8). The fungal burdens in the inoculated animals indicated a defect in the null mutant strains to colonize the host tissues, as this was lower than that associated to the WT or the reintegrant control strains (1.3 × 107 ± 0.6 × 107 cells mL–1, 0.7 × 107 ± 0.2 × 107, 1.1 × 107 ± 0.4 × 107cells mL–1, 0.6 × 107 ± 0.4 × 107, 1.0 × 107 ± 0.6 × 107cells mL–1 for WT, pmr1Δ, pmr1Δ + PMR1, och1Δ, och1Δ + OCH1, respectively. P < 0.05 when compared to the null mutant strains with the WT control strain).
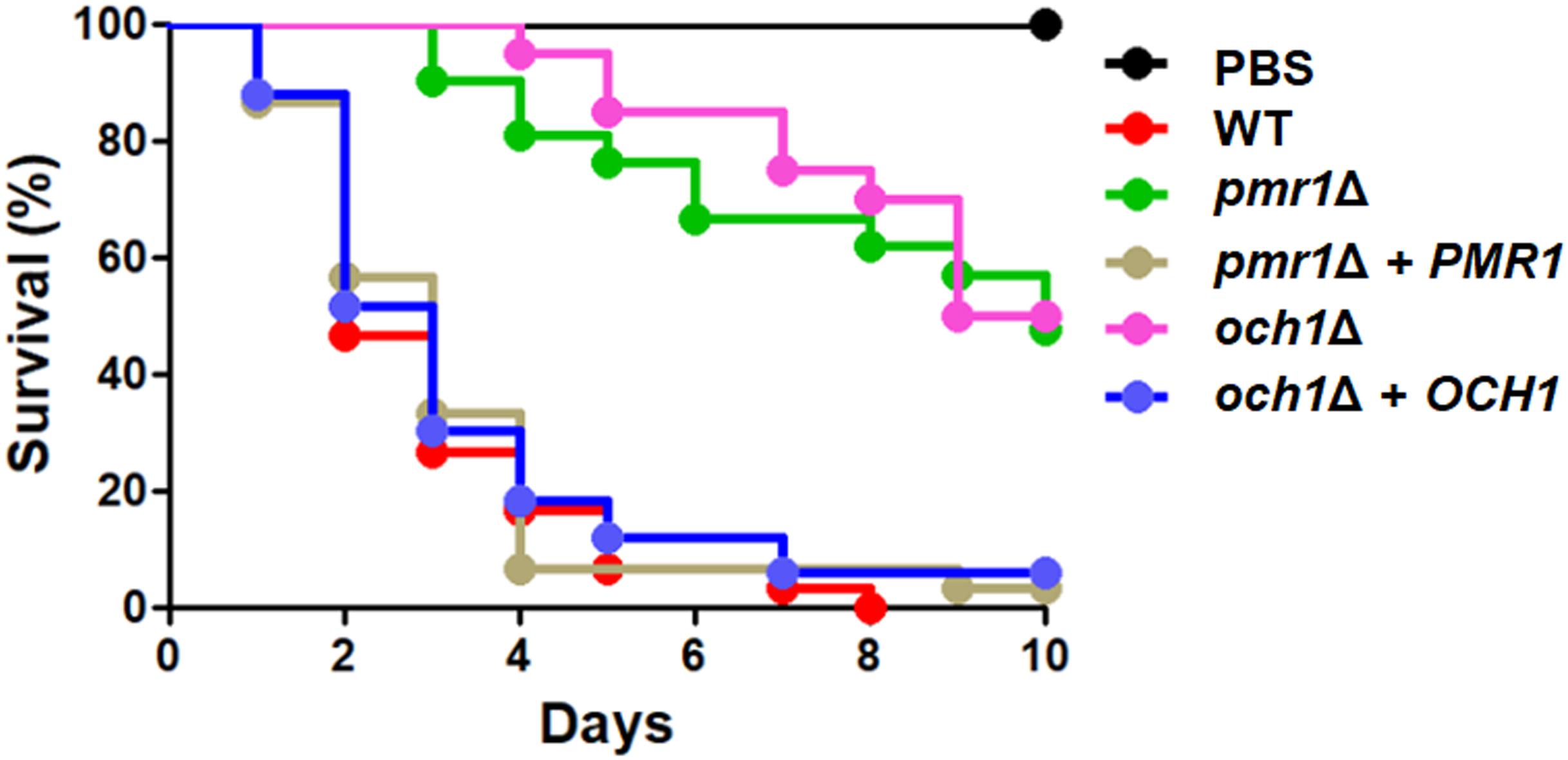
Figure 8. Mortality curve of Galleria mellonella larvae infected with either the Candida tropicalis pmr1Δ or och1Δ null mutant strains. Each larva was infected with 2 × 107 yeast cells and survival was monitored daily. Experiments were performed three times, with a total of 30 larvae per group (10 larvae for each experiment). PBS, control group injected only with PBS. Strains used are MYA-3404 (WT), HMY207 (pmr1Δ), HMY208 (pmr1Δ + PMR1), HMY181 (och1Δ), and HMY205 (och1Δ + OCH1). The null mutant strains showed a significant difference in the ability to kill larvae when compared to the parental or reintegrant control strains (P < 0.05 in all cases).
We next used a murine model of non-lethal disseminated candidiasis as previously reported (Ifrim et al., 2014; Navarro-Arias et al., 2016; Perez-Garcia et al., 2016), to evaluate the virulence in this in vivo setting. After 3 days of infection, BALB/c mice infected with the pmr1Δ null mutant had lower fungal burdens in the spleen, kidneys, brain, and liver, when compared to either the WT control cells or the reintegrant control strain (Figure 9). Similarly, the fungal burden of animals infected with the och1Δ null mutant strain was lower to that observed in animals infected with the WT strain, but interestingly, the och1Δ + OCH1 reintegrant control strain behaved like the null mutant strain, and fewer colony-forming units were recovered from the spleen, kidneys, brain, and liver of infected animals (Figure 10). Collectively, these results indicate that PMR1 and OCH1 have a significant role in the interaction of C. tropicalis with either G. mellonella or the mouse.
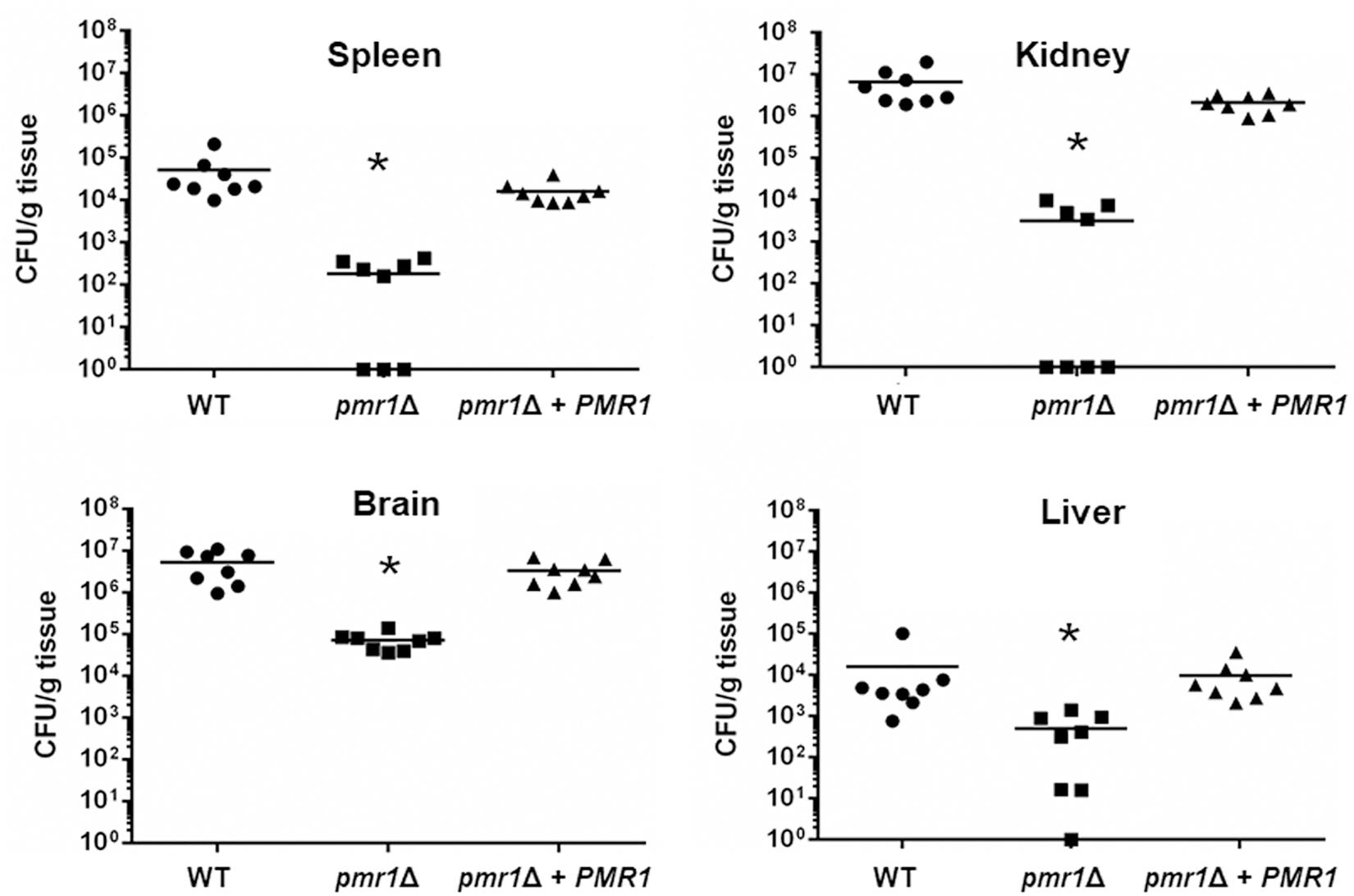
Figure 9. The Candida tropicalis pmr1Δ null mutant has decreased virulence in a mouse model of non-lethal systemic candidiasis. Wild-type Balb/c mice were infected with 1 × 106 yeast cells and the fungal burdens in the spleen, kidneys, brain, and liver were determined after 3 days of infection. Data are expressed as colony forming units (CFU) g–1 tissue (mean). Results are pooled data from two separate experiments with eight mice per group. ∗P < 0.05 when compared to animals infected with the WT control strain. Strains used are MYA-3404 (WT, closed circle), HMY207 (pmr1Δ, closed square), and HMY208 (pmr1Δ + PMR1, closed triangle).
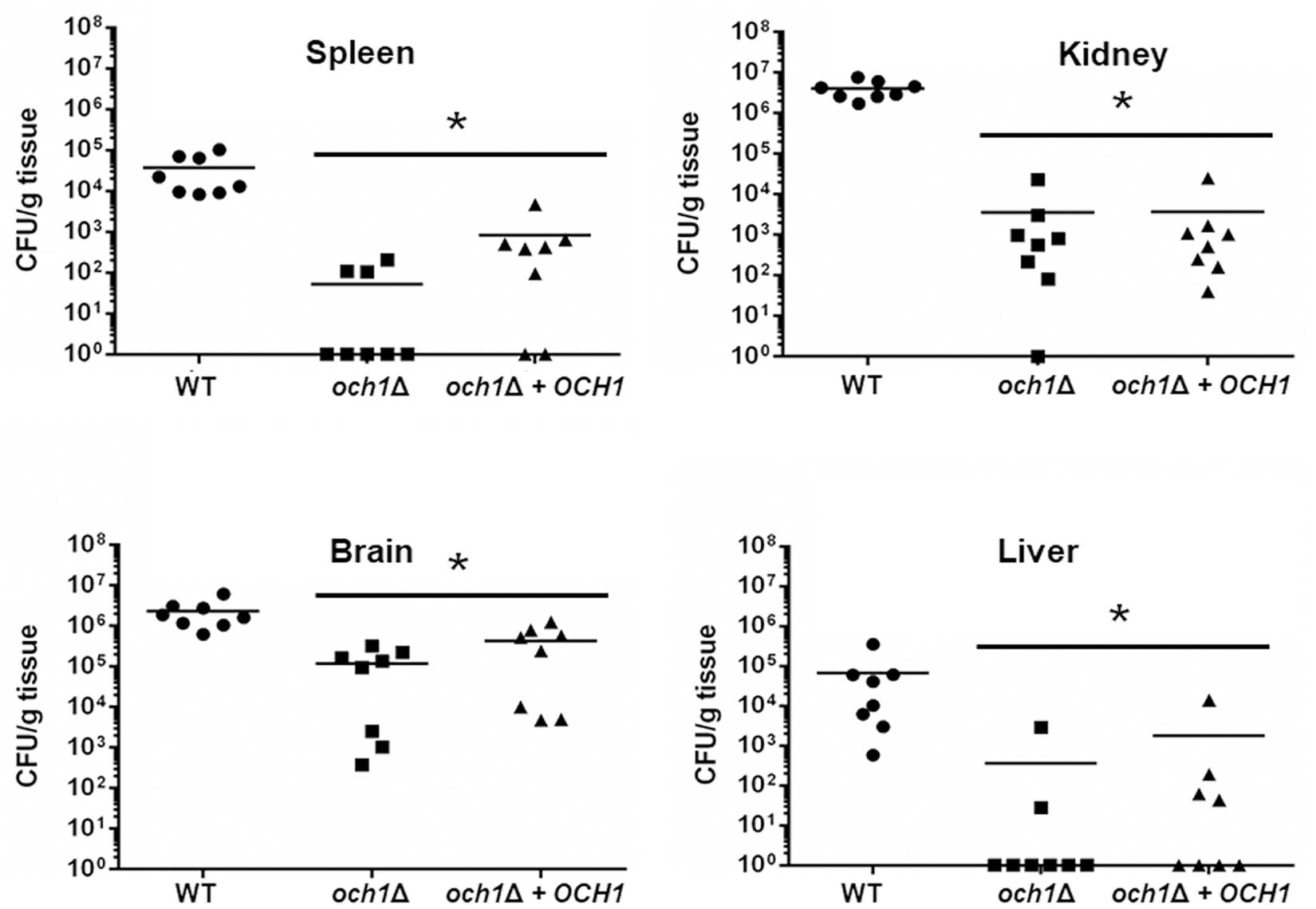
Figure 10. The Candida tropicalis och1Δ null mutant has decreased virulence in a mouse model of non-lethal systemic candidiasis. Same legend as Figure 9, but strains used are MYA-3404 (WT), HMY207 (pmr1Δ), HMY208 (pmr1Δ + PMR1). MYA-3404 (WT, closed circle), HMY181 (och1Δ, closed square), and HMY205 (och1Δ + OCH1, closed triangle). ∗P < 0.05, when compared to animals infected with the WT control strain.
Discussion
So far, the knowledge we have about the genetic machinery behind protein glycosylation and cell wall assembly in C. tropicalis is limited, likely because of the close genetic relationship with C. albicans, a thoroughly studied model of fungal cell wall synthesis. Previously, we have genetically addressed the relevance of protein phosphomannosylation during the C. tropicalis-host interaction and found that the role of this wall component in the interaction with macrophages is different from that described in C. albicans (Hernandez-Chavez et al., 2018). This report represents the first genetic approach to address the relevance of C. tropicalis N- and O-linked mannan for cell wall composition, organization, and interaction with the host.
The O-linked mannosylation pathway includes the Pmt and Mnt transferases that belong to gene families composed each of team of five members with functional redundancy (Mora-Montes et al., 2009; Martinez-Duncker et al., 2014). This has represented a challenge to assess the contribution of each of the family members to both O-linked mannan elaboration and the study of the host-fungus interaction (Munro et al., 2005; Prill et al., 2005; Mora-Montes et al., 2010). Therefore, we chose to disrupt PMR1 to get indirect insights on the contribution of O-linked mannans to the C. tropicalis-host interaction.
The bioinformatics analysis and the heterologous complementation in C. albicans strongly suggest that the ORFs we have analyzed are indeed the functional orthologs of C. albicans PMR1 and OCH1. The C. albicans genome also contains HOC1, a gene encoding for a mannosyltransferase that is part of the M-Pol II complex, involved in the elongation of the α1,6-backbone of the N-linked mannan outer chain (Martinez-Duncker et al., 2014). Both OCH1 and HOC1 encode products with significant similarity in the primary sequences but they are unable to complement each other and have signature sequences that differentiate them from each other (Lambou et al., 2010; Lozoya-Perez et al., 2019; see Supplementary Figure S4). Bioinformatics analysis of the C. tropicalis genome could identify the putative gene encoding Hoc1, whose primary structure is closer to C. albicans Hoc1 than Och1. Moreover, a sequence analysis differentiated Och1 proteins from Hoc1 proteins, being grouped in different clades (Supplementary Figure S4). Therefore, it is unlikely that we have identified the functional ortholog of HOC1 instead of OCH1. In addition, if this C. tropicalis ORF was the true ortholog of HOC1, it would be difficult to explain the phenotype restoration of the C. albicans och1Δ null mutant expressing C. tropicalis HOC1, as the M-Pol II complex works after Och1 has added the first mannose residue to the N-linked mannan outer chain backbone (Martinez-Duncker et al., 2014).
Both the C. tropicalis pmr1Δ and och1Δ null mutants displayed phenotypical traits characteristic of yeast mutants with defects in the protein mannosylation pathways: increased doubling rates, abnormal cell and colony morphology, decreased mannan content, changes in the cell wall composition and organization, and fitness to resist the action of wall perturbing agents (Bates et al., 2005, 2006, 2013; Munro et al., 2005; Mora-Montes et al., 2007, 2010; Hall et al., 2013; Navarro-Arias et al., 2016; Perez-Garcia et al., 2016). In Candida guilliermondii, loss of PMR1 affected the cellular growth, changing from a radial growth that maintains the yeast cell shape, to a unilateral one, establishing the growth of pseudohyphae (Navarro-Arias et al., 2016). Our results are in line with those found in C. albicans and Saccharomyces cerevisiae, where cells grow as yeast cells upon PMR1 disruption (Antebi and Fink, 1992; Bates et al., 2005), stressing that C. guilliermondii has different mechanisms to mobilize calcium within the cell to those found in C. albicans or C. tropicalis.
Interestingly, the changes in the composition and organization of structural polysaccharides were not similar in the mutant strains under study, but comparable to those reported in C. albicans (Bates et al., 2005, 2006). One discrepancy between C. albicans and C. tropicalis though is the chitin content in the och1Δ null mutant strains, as its abundance in the former species was doubled but not affect in the C. tropicalis strain (Bates et al., 2006). Since all the components of the core of the cell wall integrity pathway (the master regulator to control the wall composition and for triggering compensatory mechanisms when this structure is affected) are present within the C. tropicalis genome (Butler et al., 2009), it is likely this controls the cell wall remodeling upon aggression by stressors or genetic modifications that affect the cell wall structure (Dichtl et al., 2016). It has been described that the repertoire of receptors that activate this signaling pathway and its ability to engage with ligands is species-specific (Dichtl et al., 2016), which may offer an explanation to the differential effect of OCH1 loss on the chitin content in both C. albicans and C. tropicalis. In both cases, the decreased amount of mannan content may account for the exposure of this wall polymer on the surface.
It was anticipated that the och1Δ null mutant had defects only in the N-linked mannan synthesis; while the pmr1Δ mutant in both N- and O-linked mannan synthesis, a fact that was confirmed in our study. Therefore, it is feasible to hypothesize that O-linked mannans have a significant role in the signaling pathways that control the cell wall integrity pathway (Levin, 2005). Despite the contribution of N-linked mannans in the activation of this pathway has been largely documented, the detailed mechanisms behind the contribution of O-linked mannans to this signaling pathway are poorly understood (Levin, 2005).
As reported in C. albicans (Netea et al., 2006; Gow et al., 2007), C. tropicalis O-linked mannans were dispensable for stimulation of cytokine production, being N-linked mannans and β1,3-glucan the major stimuli. In the case of IL-10 stimulation, we found that mannans were not required for this cytokine production, in fact, removal of these cell wall components had a positive effect on the IL-10 levels. It has been reported that different to other cytokines, engagement of dectin-1 with β1,3-glucan is enough to signaling the production of IL-10, representing a mannan-independent pathway for stimulation of this anti-inflammatory cytokine (Reid et al., 2009). Our results confirm this mannan-independent mechanism of IL-10 production also occurs in the C. tropicalis-PBMC interaction. The activation of this signaling pathway is the most likely explanation to the fact that the och1Δ and the pmr1Δ null mutants were capable of inducing more IL-10 production that the control strains in the live form, as most of the β1,3-glucan was exposed on the cell surface of the mutants. This increment in the amount of superficial β1,3-glucan is also the likely explanation of the increased ability of the live mutant strains to stimulate higher levels of proinflammatory cytokines, stressing once again that the major fungal player in cytokine production is β1,3-glucan. Alternatively, it is possible to speculate that removal of mannans by disruption of either OCH1 or PMR1 increments the exposure of cell wall phospholipomannan on the cell surface, accounting for the increased ability to stimulate cytokine production. The presence of phospholipomannan has been described in the cell wall of both C. albicans and C. tropicalis (Cantelli et al., 1995). In the former, it has been demonstrated that stimulates pro-inflammatory cytokines via engagement with TLR2 and galectin-3 (Jouault et al., 2003, 2006). Further experiments are required to assess the contribution of phospholipomannan in the ability of the och1Δ and pmr1Δ mutants to stimulate cytokine production. Nonetheless, our results clearly suggest that like in other Candida species C. tropicalis mannans are masking β1,3-glucan, precluding interaction with dectin-1 (Wheeler and Fink, 2006; Gow et al., 2007; Navarro-Arias et al., 2016; Perez-Garcia et al., 2016). It is worthy of note that despite the null mutant cells have more β1,3-glucan exposed at the cell surface, this did not lead to a higher stimulation of pro-inflammatory cytokines, even after heat inactivation of cells. Similar observations have been reported for C. albicans, C. parapsilosis, and C. guilliermondii null mutants with defects in mannan elaboration and higher levels of β1,3-glucan at the cell wall (Netea et al., 2006; Mora-Montes et al., 2007, 2010; Navarro-Arias et al., 2016; Perez-Garcia et al., 2016). Our results showed that MR plays a role as important as that described for dectin-1 in the stimulation of the proinflammatory cytokines analyzed in this work, and our observations point out that this receptor engages with N-linked mannans, as reported in C. albicans (Netea et al., 2006). Therefore, it is tempting to speculate that even though signaling via dectin-1 is important for cytokine production, the interaction of this lectin with its ligand should be part of a co-stimulation network where other receptors, or at least MR, are involved in cytokine production. In line with this hypothesis, it has been reported the synergistic interaction between dectin-1 with TLR-2, TLR-4, TLR-5, TLR-7 or TLR-9 during cytokine stimulation (Reid et al., 2009).
It is worthy of mention that despite the fact that β-elimination can remove O-linked mannans from the wall, this alkali treatment could affect protein structures and can be released to the medium (Mormeneo et al., 1994), potentially affecting the outcome of the immune cell-fungus interaction. However, no pattern-recognition receptor on the surface of immune cells has been described to engage with the polypeptide backbone of a protein cell wall (Netea et al., 2008).
Another interesting observation is the fact that the C. albicans och1Δ null mutant barely stimulates cytokine production (Bates et al., 2006), whereas here, loss of OCH1 had a mild effect on the ability of C. tropicalis to stimulate cytokine production, highlighting a subtle but different relevance of N-linked mannans in cytokine stimulation by these species. We found that the presence of the N-linked mannan core on the C. tropicalis surface is likely to account for this observation and that this oligosaccharide has the ability to block the stimulation of cytokines most likely via MR. It was reported that in C. albicans the N-linked mannan core is further modified in the och1Δ null mutant with two to seven α1,2-mannose units (Bates et al., 2006), which contrast with the glycans isolated from S. cerevisiae och1Δ null mutant that added only one mannose unit to the N-linked mannan core (Bates et al., 2006). It is tempting to speculate that in C. tropicalis this N-linked mannan core is elongated with more mannose residues than in C. albicans, which accounts for its ability to stimulate higher cytokine levels to that observed with C. albicans. If this was true, the number of mannose units modifying the N-linked mannan core and the kind of glycosidic linkages involved remain to be addressed.
Both null mutant strains showed decreased virulence in both the G. mellonella and murine models of systemic candidiasis, similar to other Candida species where mutants in either OCH1 or PMR1 have been generated (Bates et al., 2005; Navarro-Arias et al., 2016; Perez-Garcia et al., 2016). However, in the mouse model, the reintegrant control strain for OCH1 failed to colonize as the WT control strain. Since in all the phenotypical analysis this strain behaved like the WT control strain, it is unlikely this could be related to the generation of the strains. Haploinsufficiency has been described in C. albicans (Chaillot et al., 2017), and a significant reduction of mannosyltransferase activity has been reported for the heterozygous strain in OCH1 (Bates et al., 2006). Therefore, we hypothesize that in C. tropicalis the gene could be haploinsufficient and the enzyme activity provided by one OCH1 copy could be enough to display a normal phenotype under the conditions tested but insufficient to adapt to the mouse milieu. Nevertheless, our results clearly showed virulence attenuation in the mouse model upon OCH1 disruption. Since both mutant strains showed defects in the doubling time and wall fitness, it is likely the whole-cell fitness is compromised by disruption of any of the genes under study, which is likely to account to the defects of the null mutants to interact with either G. mellonella or the mice, in other words, the virulence defect is most likely due to inability to properly adapt to the in vivo milieu than the loss of virulence factors.
In conclusion, we report the identification and disruption of C. tropicalis PMR1 and OCH1. These genes contribute to protein mannosylation in C. tropicalis and are relevant for fungal virulence, cell wall composition, assembly, and fitness. In addition, N- and O-linked mannans have differential roles for cytokine stimulation during the C. tropicalis-human PBMC interaction.
Materials and Methods
Strains and Growth Conditions
Organisms used in this study are listed in Table 2. Unless otherwise indicated, cells were maintained and propagated at 28°C in YPD medium [2% (w/v) bacteriological peptone, 1% (w/v) yeast extract, 2% (w/v) glucose]. The medium was supplemented with 2% (w/v) agar when the solid plates were required. During gene disruption, the SAT1 marker was recycled by growing cells at 28°C in liquid YEP [2% (w/v) bacteriological peptone and 1% (w/v) yeast extract]. To prepare cells for interaction with the host and cell-wall analysis, the strains were grown at 30°C in 500 mL flasks containing 100 mL of fresh medium with shaking at 200 rpm until reaching the logarithmic growth phase. Cells were inactivated by incubating at 56°C for 60 min. The confirmation of the presence of non-viable cells was performed on YPD plates incubated for 4 days at 28°C. For chemical remotion of O-linked mannans, cells were β-eliminated in 10 mL of NaOH 0.1 N and incubated at room temperature during 18 h, as previously described (Diaz-Jimenez et al., 2012). Cell suspensions were neutralized with HCl 0.1 N, and pelleted at 2000 × g, for 5 min. The supernatant was saved for O-linked mannans analysis (see below). The enzymatic N-linked mannan removal was performed with 25 U endo H (New England Biolabs) as reported (Mora-Montes et al., 2012). In both cases, cells were washed twice with sterile phosphate-buffered saline (PBS) and viability was confirmed by quantifying the colony-forming units before and after the treatment. The loss of viability after β-elimination and endo H treatment was 1.8 ± 0.9% and 3.3 ± 4.1%, respectively). To disperse cell aggregates, 2 units mL–1 chitinase (Sigma) was added to medium when required (Bates et al., 2006; Perez-Garcia et al., 2016).
Heterologous Complementation in Candida albicans
The PMR1 ORF was amplified by PCR using the primer pair 5′-AAGCTTATGAGTGATAACCCTTATGAACTA-3′ and 5′-GCTAGCTTATACTCCATATGTATAATTATTAGTATAAATAG T-3′ (underlined sequences correspond to restriction sites for HindIII and NheI, respectively); while OCH1 ORF was amplified with the primer pair 5′-AAGCTTATG CGTCTGAAGGATATCA-3′ and 5′- GCTAGCTTAATCTTCC ATTTCTGGCAT (underlined sequences correspond to restriction sites for HindIII y NheI, respectively). The amplicons were cloned into pCR®2.1-TOPO® (Invitrogen), and subcloned into the HindIII and NheI sites of pACT1 (Barelle et al., 2004), generating pACT1-CtPMR1 and pACT1-CtOCH1. A C. albicans pmr1Δ null mutant (Bates et al., 2005) was transformed with StuI-digested pACT1-CtPMR1, generating strain HMY185; whereas a C. albicans och1Δ null mutant (Bates et al., 2006) was transformed with StuI-digested pACT1-CtOCH1, generating the strain HMY148. Since the OCH1 ORF contains an internal recognition site for HindIII, after cloning into pCR®2.1-TOPO® (Invitrogen), the codon 450AAA453 was modified to 450AAG453 by site-directed mutagenesis using the Phusion Site-Directed Mutagenesis Kit (Thermo) and the primer pair 5′- CCTGATGTTTCAAGGCTTATAAAATTATGCCAAAATC-3′ and 5′- GGCATAATTTTATAAGCTTTGAAACATCAGGAA TTTC-3′. The mutation was confirmed by DNA sequencing, before cloning into pACT1.
Construction of Null Mutant and Reintegrant Control Strains
PMR1 and OCH1 were deleted using the SAT1 flipper method, as previously described (Hernandez-Chavez et al., 2018). For both genes, the 1500 bp upstream and the 1500 bp downstream regions of the ORF were amplified by PCR and cloned into the ApaI-XhoI and NotI-SacI sites of pSFS2 (Reuß et al., 2004), respectively, generating the corresponding disruption cassettes. The primer pairs used for the generation of the disruption cassettes were: for the PMR1 upstream region 5′-GGGCCCAGTTGATTGAAAAATTTTGGCCAGG-3′ and 5′- CTCGAGAGGGAGTCTGTTGAAGGAGTG; for the PMR1 downstream region 5′-GCGGCCGCACTTCCAGAAG TGTGGTATGTGT-3′ and 5′- GAGCTCACTACGCATACCT TCCAACCA-3′; for the OCH1 upstream region 5′-GGGCCCACACAACCGTCTCTATCAGG-3′ and 5′-CTCGAG TGTTAACTTCTGAATTGGTCTATGA-3′; and for the OCH1 downstream region 5′- GCGGCCGCTCTAATGTTCACAA TTAAAACTTGTATT-3′ and GAGCTCGATTTGTTAATTAA AGAAGATGGTGT-3′. The disruption cassettes were excised from the vector by digesting with ApaI and SacI and used for cell transformation of strain ATCC® MYA-3404 (referred to as WT control strain). The selection of transformed cells was performed in YPD plates added with 200 μg mL–1 nourseothricin (Goldbio). The integration of the disruption cassettes in the targeted locus was analyzed by PCR with primer pairs aligning inside and outside of the recombinations regions. Cells were grown for 2 days at 28°C in YEP broth added with 2% (w/v) maltose to recycle the SAT1 marker. The loss of SAT1 was confirmed by plating cells in YPD supplemented with 10 μg mL–1 nourseothricin and PCR. The second allele of PMR1 and OCH1 was disrupted in the second round of transformation with the same cassettes and recycling of the SAT1 marker.
To reintegrate PMR1 or OCH1 into the C. tropicalis null mutant generated, the ORF plus 1000 bp upstream and 500 bp downstream was amplified by PCR using the following primer pairs: 5′- GGGCCCCCAATGTTATTACGTCCTGGTGG-3′ and 5′- CTCGAGGAGGGAGAAAAGGGGAGGATAC-3′ for PMR1, and 5′- GGGCCCGGTAATGCTTCACCATCATCAACT-3′ and 5′- CTCGAGATCCACGGAAAGAACCGCAA-3′ for OCH1. Either the 2789 bp fragment corresponding to OCH1 or PMR1, a 4517 bp amplicon, were cloned into the ApaI-XhoI sites of pSFS2, and then a 700 bp fragment downstream of the disrupted locus was cloned into the NotI-SacI sites of the same vector. These downstream fragments were generated by PCR using the primer pairs 5′-GCGGCCGCATTGCTTCATTTGGAGGTGTT G-3′ and 5′-GAGCTCCTACGCATACCTTCCAACCA-3′ for PMR1; and 5′-GCGGCCGCCCTGGAGGCAAGTGCTTTTCA-3′ and 5′-GAGCTCACGGATTTGTATTTGTCAAGAGCCA-3′ for OCH1. These reintegration constructions were linearized with ApaI and SacI and used to transform the null mutant strains. Upon recycling of the SAT1 marker, confirmation of the reintegration of either PMR1 or OCH1 in one of the native loci was confirmed by PCR.
Cell Wall Analysis
For the analysis of the composition, grown-cells in YPD were disrupted in a Precellys homogenizer (Bertin), the homogenate was centrifuged, the pellet saved, thoroughly washed with deionized water, and the cell walls were cleansed and acid-hydrolyzed as described previously (Mora-Montes et al., 2007). The acid-hydrolyzed samples were analyzed by HPAEC-PAD in a carbohydrate analyzer system (Thermo), using the columns and separation conditions previously reported (Plaine et al., 2008). The cell wall protein content was measured upon alkali hydrolysis of the cell wall and the Bradford method, as previously described (Mora-Montes et al., 2007).
The cell wall porosity was determined by the relative porosity of polycations, as reported earlier (De Nobel et al., 1990). Cells were grown in YPD broth at 28°C until reaching the mid-log phase, washed twice with PBS, and aliquots containing 1 × 108 cells were suspended in either 10 mM Tris–HCl, pH 7.4 (buffer A), buffer A plus 30 μg/mL poly-L-lysine (Mw 30–70 kDa, Sigma) or buffer A plus 30 μg/mL DEAE-dextran (MW 500 kDa, Sigma), and incubated for 30 min at 30°C with constant shaking at 200 rpm. Cell suspensions were pelleted by centrifuging, the supernatants saved, further centrifuged, and used to measure the absorbance at 260 nm. The cell wall porosity was calculated as described previously, using the porosity to poly-L-lysine for data normalization (De Nobel et al., 1990). To quantify the cell wall phosphomannan, the cellular ability to bind Alcian blue was analyzed as described (Hobson et al., 2004).
Localization of mannan, chitin, and β1,3-glucan within the cell wall was analyzed by fluorescent microscopy using 1 mg mL–1 Con A-FITC (Sigma), 1 μg mL–1 WGA-FITC (Sigma) and 5 μg/mL IgG Fc-Dectin-1 chimera conjugated with anti-Fc IgG-FITC for mannan, chitin, and β1,3-glucan staining, respectively (Graham et al., 2006; Mora-Montes et al., 2011; Marakalala et al., 2013). Cells were examined by fluorescence microscopy using a Zeiss Axioscope-40 microscope and an Axiocam MRc camera. The fluorescence was quantified from the pictures acquired with Adobe PhotoshopTM CS6 using the formula: [(total of green pixels-background green pixels) × 100]/total pixels. The experiment was performed three independent times, with a total of 300 cells analyzed per strain.
Quantification of Cell Wall N-Linked and O-Linked Mannans
Cells grown at the mid-log phase in YPD were incubated overnight at 37°C with 25 U endo H (New England Biolabs) to trim cell wall N-linked mannans (Mora-Montes et al., 2012). Cells were also β-eliminated with 0.1 N NaOH, as described (Diaz-Jimenez et al., 2012). Then, cells were pelleted, and the supernatant used to determine the mannose content with the phenol-sulfuric-acid method (Dubois et al., 1956). In both cases, mannan release was confirmed by HPAEC-PAD as reported (Perez-Garcia et al., 2016).
Analysis of Susceptibility to Cell Wall Perturbing Agents
Cell growth in the presence of cell wall perturbing agents was performed as previously described (Navarro-Arias et al., 2016). Briefly, overnight grown cells in YPD were washed with deionized water, separated using a syringe with a 32-gauge needle and suspended at an OD600nm = 1.0. Then, fresh YPD broth was inoculated with the fungal cells at an OD600nm = 0.01, and aliquots containing 95 μL were placed into 96-well plates. The cell wall perturbing agents, in a final volume of 5 μL, were added to each well. Mock wells contained 5 μL of the vehicle only and were used to normalize the results. The final OD600nm for each well was quantified after 24 h incubation at 30°C. Calcofluor white and Congo red were from Sigma; whereas hygromycin B from GoldBio. Growth data were normalized as a percentage of those generated with the mock interactions.
Ethics Statement
The use of human PMBCs was approved by the Ethics Committee from Universidad de Guanajuato (permission number 17082011), and peripheral blood samples were withdrawn from healthy adult volunteers after information of the study was disclosed and a written consent form signed. The Ethics Committee at the University of Szeged approved the experimentation with mice (permission number XVI./3646/2016).
Cytokine Production by Human PBMCs
Upon peripheral blood was collected in tubes containing EDTA as anticlotting, PBMCs were isolated by differential centrifugation using Histopaque-1077 (Sigma), as reported (Endres et al., 1988). To stimulate cytokine production, 100 μL containing 5 × 105 PBMCs in RPMI 1640 Dutch modification (added with 2 mM glutamine, 0.1 mM pyruvate and 0.05 mg mL–1 gentamycin; all reagents from Sigma) were plated onto round-bottom 96-well microplates, and then 100 μL containing 1 × 105 fungal cells freshly harvested or treated were added to each well. The plates were incubated for 24 h at 37°C with 5% (v/v) CO2. In some experiments, PBMCs were pre-incubated for 1 h at 37°C with 200 μg mL–1 laminarin (Sigma), 10 μg mL–1 M9 (Man9GlcNAc2; Sigma), 10 μg mL–1 anti-MR (Invitrogen, Cat. No. Mab-Hmr), or 10 μg mL–1 IgG1 antibodies (Santa Cruz Biotechnology) before interaction with fungal cells. All these reagents were LPS free, as tested with the Limulus amebocyte lysate (Sigma, data not shown). Nonetheless, interactions were conducted in the presence of 5 μg mL–1 polymyxin B (Sigma) (Navarro-Arias et al., 2016). The plates were centrifuged for 10 min at 3000 × g at 4°C, and the supernatants were collected and stored at −20°C until used.
The concentrations of TNFα, IL-6 and IL-10 were quantified with the ABTS ELISA Development kits from Preprotech; while IL-1β levels were measured with a DuoSet ELISA Development kit (R&D Systems).
Infection of G. mellonella Larvae
The G. mellonella infection was performed as previously reported (Perez-Garcia et al., 2016). Briefly, 2 × 107 yeast cells contained in 10 μL PBS were passaged through a syringe with a 32-gauge needle, the last left pro-leg of the larva sanitized and fungal cells directly injected into the hemolymph, using a Hamilton syringe and a 26-gauge needle. Upon infection, the animals were kept at 37°C and survival was monitored daily. Animal death was defined by extense melanization and irritability absence. Each experimental group contained 30 larvae. Mock infections with animals injected with PBS were included as a control. The content of colony-forming units was determined by incubating serial dilutions of the hemolymph on YPD plates for 28°C for 72 h.
Infection of Mice and Analysis of the Fungal Burden
A non-lethal experimental model of disseminated candidiasis was performed as reported (Ifrim et al., 2014; Perez-Garcia et al., 2016). Animal groups containing 8 female Balb/c WT mice (older than 12 weeks old and 20 to 25 g of weight) were injected via the lateral tail vein with 100 μL of sterile PBS containing 1 × 106 C. tropicalis yeast cells, previously passaged through a syringe with a 29-gauge needle. As a control group, eight mice were injected with 100 μL of sterile PBS. Animals having access to sterile water and a normal diet ad libitum were monitored daily and showed no signs of infection such as weight loss, lethargy, ruffled fur or rapid shallow breathing during 3 days following the intravenous injection. At this point, animals were euthanatized, and the liver, brain, kidneys, and spleen were removed, weighed, and separately homogenized with a tissue grinder. The fungal burden was determined by incubating serial dilutions on YPD agar plates for 28°C for 48 h.
Statistical Analysis
Statistical analysis was performed using GraphPad Prism 6 software. Growth data in the presence of cell wall perturbing agents were analyzed by two-way ANOVA. Cytokine stimulation using human PMBCs was performed in duplicate with seven healthy donors, whereas the rest of the in vitro experiments were performed at least thrice in duplicate. Data represent the cumulative results of all experiments performed. The Mann–Whitney U test or unpaired t-test was used to establish statistical significance (see figure legends for details), with a significance level set at P < 0.05. Experiments with G. mellonella were performed three times, with a total of 30 larvae per group (10 larvae for each experiment). Results were analyzed using the Log–rank test and arranged in survival curves using Kaplan-Meier charts. The statistical significance was set at P < 0.05.
Data Availability Statement
The datasets generated for this study are available on request to the corresponding author.
Ethics Statement
The studies involving human participants were reviewed and approved by the Ethics Committee from Universidad de Guanajuato. The patients/participants provided their written informed consent to participate in this study. The animal study was reviewed and approved by the Ethics Committee at the University of Szeged.
Author Contributions
MH-C, AG, IM-D, and HM-M designed and conceived the study. MH-C, DC-G, ÁN, NL-P, JM-Á, RS-M, and NH performed the experiments. MH-C, DC-G, NL-P, JM-Á, RS-M, NH, AG, IM-D, and HM-M analyzed the data. AG and HM-M wrote the manuscript. All authors approved the final version of the manuscript.
Funding
This work was supported by the Consejo Nacional de Ciencia y Tecnología (ref. FC 2015-02-834), Universidad de Guanajuato (ref. CIIC 087/2019), and Red Temática Glicociencia en Salud (CONACYT-México). AG and ÁN were supported by the FIKP program (TUDFO/4738-1/2019 ITM) of the Ministry of Human Capacities, and grants NKFIH K 123952 and GINOP-2.3.2.-15-2016-00015. The funding sources that supported this work did not have any involvement in the design, acquisition, and analysis of data, and writing of the manuscript.
Conflict of Interest
The authors declare that the research was conducted in the absence of any commercial or financial relationships that could be construed as a potential conflict of interest.
Acknowledgments
We thank Professor Gordon Brown (University of Aberdeen) for the donation of the IgG Fc-Dectin-1 chimera. We also thank Luz A. López-Ramírez (Universidad de Guanajuato) for technical assistance.
Supplementary Material
The Supplementary Material for this article can be found online at: https://www.frontiersin.org/articles/10.3389/fmicb.2019.02743/full#supplementary-material
Footnotes
References
Antebi, A., and Fink, G. R. (1992). The yeast Ca-2+-ATPase homolog, Pmr1, is required for normal Golgi function and localizes in a novel golgi-like distribution. Mol. Biol. Cell 3, 633–654. doi: 10.1091/mbc.3.6.633
Barelle, C. J., Manson, C. L., MacCallum, D. M., Odds, F. C., Gow, N. A., and Brown, A. J. (2004). GFP as a quantitative reporter of gene regulation in Candida albicans. Yeast 21, 333–340. doi: 10.1002/yea.1099
Bates, S., Hall, R. A., Cheetham, J., Netea, M. G., MacCallum, D. M., Brown, A. J., et al. (2013). Role of the Candida albicans MNN1 gene family in cell wall structure and virulence. BMC Res. Notes 6:294. doi: 10.1186/1756-0500-6-294
Bates, S., Hughes, H. B., Munro, C. A., Thomas, W. P., MacCallum, D. M., Bertram, G., et al. (2006). Outer chain N-glycans are required for cell wall integrity and virulence of Candida albicans. J. Biol. Chem. 281, 90–98. doi: 10.1074/jbc.m510360200
Bates, S., MacCallum, D. M., Bertram, G., Munro, C. A., Hughes, H. B., Buurman, E. T., et al. (2005). Candida albicans Pmr1p, a secretory pathway P-type Ca2+/Mn2+-ATPase, is required for glycosylation and virulence. J. Biol. Chem. 280, 23408–23415. doi: 10.1074/jbc.m502162200
Bizerra, F. C., Melo, A. S. A., Katchburian, E., Freymüller, E., Straus, A. H., Takahashi, H. K., et al. (2011). Changes in cell wall synthesis and ultrastructure during paradoxical growth effect of caspofungin on four different Candida species. Antimicrob. Agents Chemother. 55, 302–310. doi: 10.1128/AAC.00633-10
Brand, A., MacCallum, D. M., Brown, A. J., Gow, N. A., and Odds, F. C. (2004). Ectopic expression of URA3 can influence the virulence phenotypes and proteome of Candida albicans but can be overcome by targeted reintegration of URA3 at the RPS10 locus. Eukaryot. Cell 3, 900–909.
Brown, G. D., Denning, D. W., Gow, N. A., Levitz, S. M., Netea, M. G., and White, T. C. (2012). Hidden killers: human fungal infections. Sci. Transl. Med. 4:165rv113. doi: 10.1126/scitranslmed.3004404
Brown, G. D., and Gordon, S. (2001). Immune recognition. A new receptor for beta-glucans. Nature 413, 36–37.
Butler, G., Rasmussen, M. D., Lin, M. F., Santos, M. A. S., Sakthikumar, S., Munro, C. A., et al. (2009). Evolution of pathogenicity and sexual reproduction in eight Candida genomes. Nature 459, 657–662. doi: 10.1038/nature08064
Cambi, A., Netea, M. G., Mora-Montes, H. M., Gow, N. A., Hato, S. V., Lowman, D. W., et al. (2008). Dendritic cell interaction with Candida albicans critically depends on N-linked mannan. J. Biol. Chem. 283, 20590–20599. doi: 10.1074/jbc.M709334200
Cantelli, C., Trinel, P. A., Bernigaud, A., Jouault, T., Polonelli, L., and Poulain, D. (1995). Mapping of β-1,2-linked oligomannosidic epitopes among glycoconjugates of Candida species. Microbiology 141, 2693–2697. doi: 10.1099/13500872-141-10-2693
Chaillot, J., Cook, M. A., Corbeil, J., and Sellam, A. (2017). Genome-wide Screen for haploinsufficient cell size genes in the opportunistic yeast Candida albicans. G3 7, 355–360. doi: 10.1534/g3.116.037986
Cheng, S. C., van de Veerdonk, F. L., Lenardon, M., Stoffels, M., Plantinga, T., Smeekens, S., et al. (2011). The dectin-1/inflammasome pathway is responsible for the induction of protective T-helper 17 responses that discriminate between yeasts and hyphae of Candida albicans. J. Leukoc. Biol. 90, 357–366. doi: 10.1189/jlb.1210702
De Nobel, J. G., Klis, F. M., Munnik, T., Priem, J., and Van Den Ende, H. (1990). An assay of relative cell wall porosity in Saccharomyces cerevisiae, Kluyveromyces lactis and Schizosaccharomyces pombe. Yeast 6, 483–490. doi: 10.1002/yea.320060605
Dean, N. (1995). Yeast glycosylation mutants are sensitive to aminoglycosides. Proc. Natl. Acad. Sci. U.S.A. 92, 1287–1291. doi: 10.1073/pnas.92.5.1287
Diaz-Jimenez, D. F., Mora-Montes, H. M., Hernandez-Cervantes, A., Luna-Arias, J. P., Gow, N. A., and Flores-Carreon, A. (2012). Biochemical characterization of recombinant Candida albicans mannosyltransferases Mnt1, Mnt2 and Mnt5 reveals new functions in O- and N-mannan biosynthesis. Biochem. Biophys. Res. Commun. 419, 77–82. doi: 10.1016/j.bbrc.2012.01.131
Díaz-Jiménez, D. F., Pérez-García, L. A., Martínez-Álvarez, J. A., and Mora-Montes, H. M. (2012). Role of the fungal cell wall in pathogenesis and antifungal resistance. Curr. Fungal. Infect. Rep. 6, 275–282. doi: 10.1007/s12281-012-0109-7
Dichtl, K., Samantaray, S., and Wagener, J. (2016). Cell wall integrity signalling in human pathogenic fungi. Cell Microbiol. 18, 1228–1238. doi: 10.1111/cmi.12612
Duan, Z., Chen, Q., Zeng, R., Du, L., Liu, C., Chen, X., et al. (2018). Candida tropicalis induces pro-inflammatory cytokine production, NF-κB and MAPKs pathways regulation, and dectin-1 activation. Can. J. Microbiol. 64, 937–944. doi: 10.1139/cjm-2017-0559
Dubois, M., Gilles, K. A., Hamilton, J. K., Rebers, P. A., and Smith, F. (1956). Colorimetric method for determination of sugars and related substances. Anal. Chem. 28, 350–356. doi: 10.1021/ac60111a017
Endres, S., Ghorbani, R., Lonnemann, G., van der Meer, J. W., and Dinarello, C. A. (1988). Measurement of immunoreactive interleukin-1 beta from human mononuclear cells: optimization of recovery, intrasubject consistency, and comparison with interleukin-1 alpha and tumor necrosis factor. Clin. Immunol. Immunopathol. 49, 424–438. doi: 10.1016/0090-1229(88)90130-4
Erwig, L. P., and Gow, N. A. R. (2016). Interactions of fungal pathogens with phagocytes. Nat. Rev. Micro. 14, 163–176. doi: 10.1038/nrmicro.2015.21
Estrada-Mata, E., Navarro-Arias, M. J., Perez-Garcia, L. A., Mellado-Mojica, E., Lopez, M. G., Csonka, K., et al. (2015). Members of the Candida parapsilosis complex and Candida albicans are differentially recognized by human peripheral blood mononuclear cells. Front. Microbiol. 6:1527. doi: 10.3389/fmicb.2015.01527
Garcia-Carnero, L. C., Perez-Garcia, L. A., Martinez-Alvarez, J. A., Reyes-Martinez, J. E., and Mora-Montes, H. M. (2018). Current trends to control fungal pathogens: exploiting our knowledge in the host-pathogen interaction. Infect. Drug Resist. 11, 903–913. doi: 10.2147/IDR.S170337
Goins, T. L., and Cutler, J. E. (2000). Relative abundance of oligosaccharides in Candida species as determined by fluorophore-assisted carbohydrate electrophoresis. J. Clin. Microbiol. 38, 2862–2869.
Gow, N. A. R., and Hube, B. (2012). Importance of the Candida albicans cell wall during commensalism and infection. Curr. Opin. Microbiol. 15, 406–412. doi: 10.1016/j.mib.2012.04.005
Gow, N. A. R., Netea, M. G., Munro, C. A., Ferwerda, G., Bates, S., Mora-Montes, H. M., et al. (2007). Immune recognition of Candida albicans beta-glucan by dectin-1. J. Infect. Dis. 196, 1565–1571.
Graham, L. M., Tsoni, S. V., Willment, J. A., Williams, D. L., Taylor, P. R., Gordon, S., et al. (2006). Soluble Dectin-1 as a tool to detect beta-glucans. J. Immunol. Methods 314, 164–169. doi: 10.1016/j.jim.2006.05.013
Hall, R. A., Bates, S., Lenardon, M. D., Maccallum, D. M., Wagener, J., Lowman, D. W., et al. (2013). The Mnn2 mannosyltransferase family modulates mannoprotein fibril length, immune recognition and virulence of Candida albicans. PLoS Pathog. 9:e1003276. doi: 10.1371/journal.ppat.1003276
Hall, R. A., and Gow, N. A. (2013). Mannosylation in Candida albicans: role in cell wall function and immune recognition. Mol. Microbiol. 90, 1147–1161. doi: 10.1111/mmi.12426
Hamada, T., Nakajima, T., Izaki, K., and Matsuda, K. (1981). Comparison of the mannan structure from the cell-wall mutant Candida sp. M-7002 and its wild type. I. Characterization of the proteo-mannan from the mutant and the wild-type cells. Eur. J. Biochem. 119, 365–371. doi: 10.1111/j.1432-1033.1981.tb05617.x
Hazen, K. C., and Glee, P. M. (1994). Hydrophobic cell wall protein glycosylation by the pathogenic fungus Candida albicans. Can. J. Microbiol. 40, 266–272. doi: 10.1139/m94-043
Hernández-Chávez, M., Pérez-García, L., Niño-Vega, G., and Mora-Montes, H. (2017). Fungal strategies to evade the host immune recognition. J. Fungi. 3, 51. doi: 10.3390/jof3040051
Hernandez-Chavez, M. J., Franco, B., Clavijo-Giraldo, D. M., Hernandez, N. V., Estrada-Mata, E., and Mora-Montes, H. M. (2018). Role of protein phosphomannosylation in the Candida tropicalis-macrophage interaction. FEMS Yeast Res. 18:foy053. doi: 10.1093/femsyr/foy053
Hobson, R. P., Munro, C. A., Bates, S., MacCallum, D. M., Cutler, J. E., Heinsbroek, S. E., et al. (2004). Loss of cell wall mannosylphosphate in Candida albicans does not influence macrophage recognition. J. Biol. Chem. 279, 39628–39635. doi: 10.1074/jbc.m405003200
Ifrim, D. C., Bain, J. M., Reid, D. M., Oosting, M., Verschueren, I., Gow, N. A. R., et al. (2014). Role of dectin-2 for host defense against systemic infection with Candida glabrata. Infect. Immun. 82, 1064–1073. doi: 10.1128/IAI.01189-13
Jouault, T., El Abed-El Behi, M., Martínez-Esparza, M., Breuilh, L., Trinel, P.-A., Chamaillard, M., et al. (2006). Specific recognition of Candida albicans by macrophages requires galectin-3 to discriminate Saccharomyces cerevisiae and needs association with TLR2 for signaling. J. Immunol. 177, 4679–4687. doi: 10.4049/jimmunol.177.7.4679
Jouault, T., Ibata-Ombetta, S., Takeuchi, O., Trinel, P.-A., Sacchetti, P., Lefebvre, P., et al. (2003). Candida albicans phospholipomannan is sensed through Toll-Like receptors. J. Infect. Dis. 188, 165–172.
Klis, F. M., de Groot, P., and Hellingwerf, K. (2001). Molecular organization of the cell wall of Candida albicans. Med. Mycol. 39(Suppl. 1), 1–8. doi: 10.1080/744118876
Kobayashi, H., Matsuda, K., Ikeda, T., Suzuki, M., Takahashi, S., Suzuki, A., et al. (1994). Structures of cell wall mannans of pathogenic Candida tropicalis IFO 0199 and IFO 1647 yeast strains. Infect. Immun. 62, 615–622.
Kopecka, M., and Gabriel, M. (1992). The influence of congo red on the cell wall and (1—-3)-beta-D-glucan microfibril biogenesis in Saccharomyces cerevisiae. Arch. Microbiol. 158, 115–126. doi: 10.1007/bf00245214
Kothavade, R. J., Kura, M. M., Valand, A. G., and Panthaki, M. H. (2010). Candida tropicalis: its prevalence, pathogenicity and increasing resistance to fluconazole. J. Med. Microbiol. 59, 873–880. doi: 10.1099/jmm.0.013227-0
Lambou, K., Perkhofer, S., Fontaine, T., and Latge, J. P. (2010). Comparative functional analysis of the OCH1 mannosyltransferase families in Aspergillus fumigatus and Saccharomyces cerevisiae. Yeast 27, 625–636. doi: 10.1002/yea.1798
Levin, D. E. (2005). Cell wall integrity signaling in Saccharomyces cerevisiae. Microbiol. Mol. Biol. Rev. 69, 262–291. doi: 10.1128/mmbr.69.2.262-291.2005
Lozoya-Perez, N. E., Casas-Flores, S., de Almeida, J. R. F., Martinez-Alvarez, J. A., Lopez-Ramirez, L. A., Jannuzzi, G. P., et al. (2019). Silencing of OCH1 unveils the role of Sporothrix schenckii N-linked glycans during the host-fungus interaction. Infect. Drug Resist. 12, 67–85. doi: 10.2147/IDR.S185037
Lutsenko, S., and Kaplan, J. H. (1995). Organization of P-type ATPases: significance of structural diversity. Biochemistry 34, 15607–15613. doi: 10.1021/bi00048a001
Marakalala, M. J., Vautier, S., Potrykus, J., Walker, L. A., Shepardson, K. M., Hopke, A., et al. (2013). Differential adaptation of Candida albicans in vivo modulates immune recognition by dectin-1. PLoS Pathog. 9:e1003315. doi: 10.1371/journal.ppat.1003315
Martinez-Alvarez, J. A., Perez-Garcia, L. A., Flores-Carreon, A., and Mora-Montes, H. M. (2014). The immune response against Candida spp. and Sporothrix schenckii. Rev. Iberoam. Micol. 31, 62–66. doi: 10.1016/j.riam.2013.09.015
Martinez-Alvarez, J. A., Perez-Garcia, L. A., Mellado-Mojica, E., Lopez, M. G., Martinez-Duncker, I., Lopes-Bezerra, L. M., et al. (2017). Sporothrix schenckii sensu stricto and Sporothrix brasiliensis are differentially recognized by human peripheral blood mononuclear cells. Front. Microbiol. 8:843. doi: 10.3389/fmicb.2017.00843
Martinez-Duncker, I., Diaz-Jimenez, D. F., and Mora-Montes, H. M. (2014). Comparative analysis of protein glycosylation pathways in humans and the fungal pathogen Candida albicans. Int. J. Microbiol. 2014:267497. doi: 10.1155/2014/267497
McKenzie, C. G. J., Koser, U., Lewis, L. E., Bain, J. M., Mora-Montes, H. M., Barker, R. N., et al. (2010). Contribution of Candida albicans cell wall components to recognition by and escape from murine macrophages. Infect. Immun. 78, 1650–1658. doi: 10.1128/IAI.00001-10
Mesa-Arango, A. C., Rueda, C., Román, E., Quintin, J., Terrón, M. C., Luque, D., et al. (2016). Cell wall changes in amphotericin B-resistant strains from Candida tropicalis and relationship with the immune responses elicited by the host. Antimicrob. Agents Chemother. 60, 2326–2335. doi: 10.1128/AAC.02681-15
Mora-Montes, H. M., Bates, S., Netea, M. G., Castillo, L., Brand, A., Buurman, E. T., et al. (2010). A multifunctional mannosyltransferase family in Candida albicans determines cell wall mannan structure and host-fungus interactions. J. Biol. Chem. 285, 12087–12095. doi: 10.1074/jbc.M109.081513
Mora-Montes, H. M., Bates, S., Netea, M. G., Diaz-Jimenez, D. F., Lopez-Romero, E., Zinker, S., et al. (2007). Endoplasmic reticulum alpha-glycosidases of Candida albicans are required for N glycosylation, cell wall integrity, and normal host-fungus interaction. Eukaryot. Cell 6, 2184–2193. doi: 10.1128/ec.00350-07
Mora-Montes, H. M., Lopez-Romero, E., Zinker, S., Ponce-Noyola, P., and Flores-Carreon, A. (2004). Hydrolysis of Man9GlcNAc2 and Man8GlcNAc2 oligosaccharides by a purified alpha-mannosidase from Candida albicans. Glycobiology 14, 593–598. doi: 10.1093/glycob/cwh091
Mora-Montes, H. M., McKenzie, C., Bain, J. M., Lewis, L. E., Erwig, L. P., and Gow, N. A. (2012). Interactions between macrophages and cell wall oligosaccharides of Candida albicans. Methods Mol. Biol. 845, 247–260. doi: 10.1007/978-1-61779-539-8_16
Mora-Montes, H. M., Netea, M. G., Ferwerda, G., Lenardon, M. D., Brown, G. D., Mistry, A. R., et al. (2011). Recognition and blocking of innate immunity cells by Candida albicans chitin. Infect. Immun. 79, 1961–1970. doi: 10.1128/IAI.01282-10
Mora-Montes, H. M., Ponce-Noyola, P., Villagómez-Castro, J. C., Gow, N. A. R., Flores-Carreón, A., and López-Romero, E. (2009). Protein glycosylation in Candida. Future Microbiol. 4, 1167–1183. doi: 10.2217/fmb.09.88
Mormeneo, S., Marcilla, A., Iranzo, M., and Sentandreu, R. (1994). Structural mannoproteins released by beta-elimination from Candida albicans cell walls. FEMS Microbiol. Lett. 123, 131–136. doi: 10.1016/0378-1097(94)90285-2
Munro, C. A., Bates, S., Buurman, E. T., Hughes, H. B., Maccallum, D. M., Bertram, G., et al. (2005). Mnt1p and Mnt2p of Candida albicans are partially redundant alpha-1,2-mannosyltransferases that participate in O-linked mannosylation and are required for adhesion and virulence. J. Biol. Chem. 280, 1051–1060. doi: 10.1074/jbc.m411413200
Navarro-Arias, M. J., Defosse, T. A., Dementhon, K., Csonka, K., Mellado-Mojica, E., Dias Valerio, A., et al. (2016). Disruption of protein mannosylation affects Candida guilliermondii cell wall, immune sensing, and virulence. Fron.t Microbiol. 7:1951.
Navarro-Arias, M. J., Dementhon, K., Defosse, T. A., Foureau, E., Courdavault, V., Clastre, M., et al. (2017). Group X hybrid histidine kinase Chk1 is dispensable for stress adaptation, host-pathogen interactions and virulence in the opportunistic yeast Candida guilliermondii. Res. Microbiol. 168, 644–654. doi: 10.1016/j.resmic.2017.04.009
Navarro-Arias, M. J., Hernandez-Chavez, M. J., Garcia-Carnero, L. C., Amezcua-Hernandez, D. G., Lozoya-Perez, N. E., Estrada-Mata, E., et al. (2019). Differential recognition of Candida tropicalis, Candida guilliermondii, Candida krusei, and Candida auris by human innate immune cells. Infect. Drug Resist. 12, 783–794. doi: 10.2147/IDR.S197531
Netea, M. G., Brown, G. D., Kullberg, B. J., and Gow, N. A. (2008). An integrated model of the recognition of Candida albicans by the innate immune system. Nat. Rev. Microbiol. 6, 67–78. doi: 10.1038/nrmicro1815
Netea, M. G., Gow, N. A., Munro, C. A., Bates, S., Collins, C., Ferwerda, G., et al. (2006). Immune sensing of Candida albicans requires cooperative recognition of mannans and glucans by lectin and Toll-like receptors. J. Clin. Invest. 116, 1642–1650. doi: 10.1172/jci27114
Netea, M. G., Joosten, L. A., van der Meer, J. W., Kullberg, B. J., and van de Veerdonk, F. L. (2015). Immune defence against Candida fungal infections. Nat. Rev. Immunol. 15, 630–642. doi: 10.1038/nri3897
Neumann, A. K., and Jacobson, K. (2010). A novel pseudopodial component of the dendritic cell anti-fungal response: the fungipod. PLoS Pathog. 6:e1000760. doi: 10.1371/journal.ppat.1000760
Perez-Garcia, L. A., Csonka, K., Flores-Carreon, A., Estrada-Mata, E., Mellado-Mojica, E., Nemeth, T., et al. (2016). Role of protein glycosylation in Candida parapsilosis cell wall integrity and host interaction. Front. Microbiol. 7:306. doi: 10.3389/fmicb.2016.00306
Plaine, A., Walker, L., Da Costa, G., Mora-Montes, H. M., McKinnon, A., Gow, N. A., et al. (2008). Functional analysis of Candida albicans GPI-anchored proteins: roles in cell wall integrity and caspofungin sensitivity. Fungal Genet. Biol. 45, 1404–1414. doi: 10.1016/j.fgb.2008.08.003
Plaza, V., Lagues, Y., Carvajal, M., Perez-Garcia, L. A., Mora-Montes, H. M., Canessa, P., et al. (2015). bcpmr1 encodes a P-type Ca(2+)/Mn(2+)-ATPase mediating cell-wall integrity and virulence in the phytopathogen Botrytis cinerea. Fungal Genet. Biol. 76, 36–46. doi: 10.1016/j.fgb.2015.01.012
Prill, S. K., Klinkert, B., Timpel, C., Gale, C. A., Schroppel, K., and Ernst, J. F. (2005). PMT family of Candida albicans: five protein mannosyltransferase isoforms affect growth, morphogenesis and antifungal resistance. Mol. Microbiol. 55, 546–560. doi: 10.1111/j.1365-2958.2004.04401.x
Reid, D. M., Gow, N. A. R., and Brown, G. D. (2009). Pattern recognition: recent insights from Dectin-1. Curr. Opin. Immunol. 21, 30–37. doi: 10.1016/j.coi.2009.01.003
Reuß, O., Vik, Å, Kolter, R., and Morschhäuser, J. (2004). The SAT1 flipper, an optimized tool for gene disruption in Candida albicans. Gene 341, 119–127. doi: 10.1016/j.gene.2004.06.021
Saijo, S., Ikeda, S., Yamabe, K., Kakuta, S., Ishigame, H., Akitsu, A., et al. (2010). Dectin-2 recognition of alpha-mannans and induction of Th17 cell differentiation is essential for host defense against Candida albicans. Immunity 32, 681–691. doi: 10.1016/j.immuni.2010.05.001
Spreghini, E., Davis, D. A., Subaran, R., Kim, M., and Mitchell, A. P. (2003). Roles of Candida albicans Dfg5p and Dcw1p cell surface proteins in growth and hypha formation. Eukaryot. Cell 2, 746–755. doi: 10.1128/ec.2.4.746-755.2003
Wagener, J., Malireddi, R. K., Lenardon, M. D., Koberle, M., Vautier, S., MacCallum, D. M., et al. (2014). Fungal chitin dampens inflammation through IL-10 induction mediated by NOD2 and TLR9 activation. PLoS Pathog. 10:e1004050. doi: 10.1371/journal.ppat.1004050
Wang, H., Xu, Y. C., and Hsueh, P. R. (2016). Epidemiology of candidemia and antifungal susceptibility in invasive Candida species in the Asia-Pacific region. Future Microbiol. 11, 1461–1477. doi: 10.2217/fmb-2016-0099
Wells, C. A., Salvage-Jones, J. A., Li, X., Hitchens, K., Butcher, S., Murray, R. Z., et al. (2008). The macrophage-inducible C-type lectin, mincle, is an essential component of the innate immune response to Candida albicans. J. Immunol. 180, 7404–7413. doi: 10.4049/jimmunol.180.11.7404
West, L., Lowman, D. W., Mora-Montes, H. M., Grubb, S., Murdoch, C., Thornhill, M. H., et al. (2013). Differential virulence of Candida glabrata glycosylation mutants. J. Biol. Chem. 288, 22006–22018. doi: 10.1074/jbc.M113.478743
Wheeler, R. T., and Fink, G. R. (2006). A drug-sensitive genetic network masks fungi from the immune system. PLoS Pathog. 2:e35. doi: 10.1371/journal.ppat.0020035
Whibley, N., Jaycox, J. R., Reid, D., Garg, A. V., Taylor, J. A., Clancy, C. J., et al. (2015). Delinking CARD9 and IL-17: CARD9 protects against Candida tropicalis infection through a TNF-α-dependent, IL-17-independent mechanism. J. Immunol. 195, 3781–3792. doi: 10.4049/jimmunol.1500870
Zhu, L. L., Zhao, X. Q., Jiang, C., You, Y., Chen, X. P., Jiang, Y. Y., et al. (2013). C-type lectin receptors Dectin-3 and Dectin-2 form a heterodimeric pattern-recognition receptor for host defense against fungal infection. Immunity 39, 324–334. doi: 10.1016/j.immuni.2013.05.017
Keywords: cell wall, innate immunity, host-fungus interplay, virulence, protein glycosylation
Citation: Hernández-Chávez MJ, Clavijo-Giraldo DM, Novák Á, Lozoya-Pérez NE, Martínez-Álvarez JA, Salinas-Marín R, Hernández NV, Martínez-Duncker I, Gácser A and Mora-Montes HM (2019) Role of Protein Mannosylation in the Candida tropicalis-Host Interaction. Front. Microbiol. 10:2743. doi: 10.3389/fmicb.2019.02743
Received: 26 August 2019; Accepted: 11 November 2019;
Published: 28 November 2019.
Edited by:
Agostinho Carvalho, University of Minho, PortugalReviewed by:
Rebecca Anne Hall, University of Birmingham, United KingdomChantal Fradin, Université de Lille, France
Copyright © 2019 Hernández-Chávez, Clavijo-Giraldo, Novák, Lozoya-Pérez, Martínez-Álvarez, Salinas-Marín, Hernández, Martínez-Duncker, Gácser and Mora-Montes. This is an open-access article distributed under the terms of the Creative Commons Attribution License (CC BY). The use, distribution or reproduction in other forums is permitted, provided the original author(s) and the copyright owner(s) are credited and that the original publication in this journal is cited, in accordance with accepted academic practice. No use, distribution or reproduction is permitted which does not comply with these terms.
*Correspondence: Attila Gácser, Z2Fjc2VyYUBiaW8udS1zemVnZWQuaHU=; Héctor M. Mora-Montes, aG1vcmFAdWd0by5teA==