- 1Department of Infectious Diseases, Robert Koch-Institute, Wernigerode, Germany
- 2SLK-Kliniken Heilbronn, Institute for Infection Prevention and Clinical Hygiene, Heilbronn, Germany
- 3German National Reference Centre for Multidrug-Resistant Gram-Negative Bacteria, Department of Medical Microbiology, Ruhr-University Bochum, Bochum, Germany
One of the most demanding challenges in infection control is the worldwide dissemination of multidrug-resistant (MDR) bacteria in clinical settings. Especially the increasing prevalence of carbapenemase producing Gram-negative pathogens poses an urgent threat to public health, as these enzymes confer resistance to almost all β-lactam antibiotics including carbapenems. In this study, we report a prolonged nosocomial outbreak of various NDM-1-producing Enterobacterales species due to clonal spread and cross-species exchange of plasmids and possibly transposons. Between July 2015 and September 2017, a total of 51 carbapenemase-positive isolates were collected from 38 patients and three environmental sources in a single German hospital. Combining molecular typing methods and whole genome sequencing, the metallo-β-lactamase gene blaNDM–1 was found to be present in 35 isolates of which seven additionally carried the carbapenemase gene blaKPC–2. Core genome MLST (cgMLST) revealed different clusters of closely related isolates of Escherichia coli, Klebsiella pneumoniae, Citrobacter freundii, Morganella morganii or Enterobacter cloacae indicating clonal spread. The detailed reconstruction of the plasmid sequences revealed that in all outbreak-associated isolates blaNDM–1 was located on similar composite transposons, which were also very similar to Tn125 previously described for Acinetobacter baumannii. In contrast to Tn125, these structures were flanked by IS26 elements, which could facilitate horizontal gene transfer. Moreover, the identical plasmid was found to be shared by E. coli and M. morganii isolates. Our results highlight the importance of detailed genome-based analyses for complex nosocomial outbreaks, allowing the identification of causal genetic determinants and providing insights into potential mechanisms involved in the dissemination of antibiotic resistances between different bacterial species.
Introduction
The rapid spread of antimicrobial resistance (AMR) in nosocomial pathogens poses an urgent threat to patients and public health worldwide. In particular, the increasing incidence of multidrug-resistant (MDR) Gram-negative bacteria that are resistant to fluoroquinolones, third-generation cephalosporins, and carbapenems is of major concern, as these MDR pathogens dramatically limit therapeutic options (Pfeifer et al., 2010; Ruppe et al., 2015; Vasoo et al., 2015). For this reason, bacterial pathogens such as Acinetobacter baumanii, Pseudomonas aeruginosa, and various Enterobacterales species have been identified by the World Health Organization as first priority for research and development of new antibiotics (Tacconelli and Magrini, 2016).
Resistance to third-generation cephalosporins in enterobacterial species is primarily mediated by the acquisition of plasmid-encoded β-lactamases that are characterized by an expansion of the substrate spectrum and are thus referred to as extended-spectrum β-lactamases (ESBL) (Paterson and Bonomo, 2005; Pfeifer et al., 2010). Due to the rapid spread and worldwide dissemination of ESBL-producing Enterobacterales and other third-generation cephalosporin-resistant Gram-negative bacteria, carbapenems and other antibiotics of last resort have been increasingly used since the early 1990s (Hawkey and Livermore, 2012). The administration of carbapenems in antimicrobial chemotherapy supported the selection of specific β-lactamases (carbapenemases) that can hydrolyze virtually all β-lactams, including carbapenems, and often resist prominent β-lactam/β-lactamase inhibitor combinations (Queenan and Bush, 2007; Rodriguez-Bano et al., 2018).
In 2009, a new Ambler class B broad-spectrum β-lactamase, the New Delhi metallo-β-lactamase-1 (NDM-1), was described in a Klebsiella pneumoniae isolate from a Swedish patient who was previously hospitalized in India (Yong et al., 2009). This enzyme hydrolyzes penicillins, third-generation cephalosporins and carbapenems but not the monobactam aztreonam (Nordmann et al., 2011). Since then, 16 NDM variants have been described in various enterobacterial species from different geographical locations; most of them are encoded by plasmids of distinct Inc types such as IncA/C, IncF, and IncL/M (Khong et al., 2016; Wailan et al., 2016). These plasmids usually co-harbor large collections of genetic AMR determinants, thereby mediating a MDR phenotype (Pfeifer et al., 2010; Nordmann et al., 2011; Bush, 2016). Therapeutics of last resort against MDR and NDM-1-producing Gram-negative bacteria are colistin, tigecycline, and fosfomycin; none of which can be considered ideal in terms of overall efficacy, resistance selection and/or side effects (Nordmann et al., 2011).
Since its first description, NDM-1 has been recorded worldwide in a great diversity of clinical Enterobacterales species and has often been associated with a travel history to endemic regions like India, Pakistan, and the Balkan states (Nordmann et al., 2011; Dortet et al., 2014). The potential of blaNDM–1 to rapidly disseminate between related species of Enterobacterales is facilitated by the high genetic mobility of blaNDM–1 and an association of this resistance determinant with promiscuous plasmids that have a broad host range (Nordmann et al., 2011; Curran and Otter, 2014). As a result of effective horizontal gene transfer (HGT), identical mobile genetic elements (MGEs) occur in different bacteria. However, identical MGEs shared by different bacteria are not necessarily proof of HGT, as they may originate from different sources. The reliable reconstruction of transmission chains is therefore essential to the analysis of multi-species outbreaks, although often difficult and laborious (Pirs et al., 2019). Outbreaks of NDM-1-producing Enterobacterales have been reported, as described for the United Kingdom (Fairley et al., 2019), Greece (Politi et al., 2019), Poland (Baraniak et al., 2016), Slovenia (Pirs et al., 2019), Belgium (Heinrichs et al., 2019), Netherlands (Bosch et al., 2017), and most recently Italy (ECDC, 2019). In Europe and more particular in Germany, the prevalence of invasive Enterobacterales with resistance to carbapenems is still low, and NDM-1-producing isolates have only been observed occasionally (Becker et al., 2018b). However, data based on the voluntary submission of MDR isolates to the National Reference Centre for Multidrug-resistant Gram-negative Bacteria (NRC) in Bochum, Germany, indicate an increasing number of carbapenemase-producing isolates in Germany over the past years (Robert Koch-Institute, 2018). From 2012 to 2017, the number of NDM-1-producing Enterobacterales isolates received from hospitals across Germany rose from 40 to 199. Focusing on all carbapenemase-positive Enterobacterales, a total of 1594 isolates were registered in 2017, including 752 Klebsiella pneumoniae, 328 Escherichia coli and 199 Enterobacter cloacae. The most commonly detected carbapenemase was OXA-48 (n = 529), followed by VIM-1 (n = 299), NDM-1 (n = 199), and KPC-2 (n = 148). In the present study, molecular typing methods and whole genome sequencing were combined to trace the prolonged nosocomial outbreak of different NDM-1-producing Enterobacterales species back to clonal spread and cross-species exchange of plasmids and possibly transposons.
Materials and Methods
Clinical Case Definition
In a German hospital with 18 wards and approx. 1,000 beds, several cases of Gram-negative, carbapenem-resistant bacteria producing NDM-1 and/or KPC-2 (K. pneumoniae carbapenemase 2) were detected by PCR in 2015. Two of the first three isolates were obtained from patients with travel history. A clinical case definition based on the successful molecular detection of NDM-1 in Enterobacterales was adopted to track a possible spread of these pathogens.
Bacterial Isolates
As part of the primary diagnostics, species identification and antibiotic susceptibility testing were performed by the hospital’s routine laboratory using the MicroScan WalkAway plus system (Beckman Coulter, CA, United States). Analyses were performed and interpreted according to EUCAST1. In case of ambiguous results, carbapenemase detection was conducted using a commercial cassette PCR system considering genes encoding for KPC, NDM, VIM, IMP-1, and OXA-48. Isolates were sent to the NRC to confirm the diagnosis and carbapenemase type. Finally, a total of 51 carbapenemase-positive Enterobacterales isolates collected between July 2015 and October 2017 were submitted to the Robert Koch-Institute (RKI) for detailed molecular analyses and strain typing (Supplementary Table 1).
Susceptibility Testing
At the RKI, antimicrobial susceptibility testing was repeated by the use of broth microdilution (BMD) according DIN58940 and EUCAST guidelines v9.0 (see text footnote 1). In total, nine antibiotics were tested, including ampicillin, cefotaxime, ceftazidime, cefoxitin, meropenem, gentamicin, amikacin, ciprofloxacin, sulfamethoxazole/trimethoprim, and colistin. Additionally, automated susceptibility testing was performed for piperacillin/tazovactam, aztreonam, cefepime, imipenem, fosfomycin, and tigecycline using the automated test system VITEK® 2 with card AST-N248 (bioMérieux, Nuertingen, Germany).
Molecular Typing
PCR-based detection of resistance genes
The presence of various β-lactamase genes (blaNDM–like, blaVIM–like, blaIMP–like, blaOXA–48–like, blaKPC–like, blaCTX–M–1–2–9group, blaTEM–like, blaSHV–like, blaCMY–like, blaDHA–like, blaOXA–1–2–9–10group, blaPER–like, blaGES–like, and blaVEB–like) was tested by PCR and subsequent Sanger sequencing using previously described primers and protocols (Turton et al., 2006; Grobner et al., 2009; Pfeifer et al., 2011). Additionally, a PCR screening for genes contributing to resistance to fluoroquinolones and aminoglycosides [aac(6′)Ib-like, qnrA/B/S-like, armA, rmtB] was performed as described elsewhere (Park et al., 2006; Doi and Arakawa, 2007; Pfeifer et al., 2009). For PCR-based screening of qnrC and qnrD genes the following primers were used: qnrC_fwd 5′-atttccaaggggcaaactg-3′and qnrC_rev 5′-aactgctccaaaagctgctc-3′(amplification product 400 bp), qnrD_fwd 5′-ttgtgatttttcaggggttg-3′ and qnrD_rev 5′- cctgctctccatccaacttc-3′ (amplification product 521 bp).
XbaI-macrorestriction and pulsed field gel electrophoresis
Bacterial strain typing was performed by XbaI-macrorestriction followed by PFGE. Fragment patterns were interpreted according to the criteria of Tenover et al. (1995).
Conjugation experiments
Transfer of β-lactam resistance was tested by broth mating assays with E. coli J53 Azir as the recipient (Clowes and Rowley, 1954). Selection of transconjugants was performed on Mueller–Hinton agar plates that contained sodium azide (200 mg/L) and ampicillin (100 mg/L). Transconjugants were tested for presence of transferred β-lactamase genes and plasmid-mediated quinolone resistance (PMQR) genes by PCR screening. Finally, the plasmid DNA of transconjugants was isolated with the QIAGEN Plasmid Mini Kit according to manufacturer’s instructions (QIAGEN, Hilden, Germany). PCR-based replicon typing (PBRT) was performed using a commercial kit (PBRT KIT, Diatheva, Italy).
Hybridization experiments
Plasmid DNA of donor strains and/or transconjugants was isolated using the QIAGEN Plasmid Mini Kit according to manufacturer’s instruction (QIAGEN, Hilden, Germany). Southern hybridization using digoxigenin-dUTP-labeled probes and signal detection using CDP-Star were performed following the manufacturer’s guidelines to localize blaNDM–1 (Roche Diagnostics Ltd., West Sussex, United Kingdom).
S1 nuclease restriction
Plasmid linearization and separation were performed by S1 nuclease restriction of whole genomic DNA (donor strains and transconjugants) combined with PFGE as previously described (Barton et al., 1995).
Whole Genome Sequencing and Quality Control
Brain Heart Infusion (BHI) broth was used for the cultivation of bacteria. DNA was extracted from an overnight culture using the DNeasy Blood & Tissue Kit (Qiagen, Hilden, Germany). For quantification of nucleic acids, the Qubit dsDNA HS Assay Kit (Thermo Fisher Scientific, Karlsruhe, Germany) was used in line with the manufacturer’s instructions. Sequencing libraries were generated with the Nextera XT DNA Library Preparation Kit (Illumina, San Diego, CA, United States) and paired-end sequencing was performed using a MiSeq instrument with the 2 × 300 MiSeq v3 reagent kit (Illumina, San Diego, CA, United States). Additionally, different isolates were selected for long-read sequencing via PacBio® Single Molecule Real Time (SMRT) sequencing (isolate 24-16) or Oxford Nanopore Technology (ONT; isolates 20-16, 22-16, 132-16 and 460-16). Isolation of DNA for SMRT and ONT sequencing was performed by using the MagAttract High Molecular Weight DNA Kit (Qiagen, Hilden, Germany) and the Genomic-tip 100/G Kit (Qiagen, Hilden, Germany), respectively, according to the manufacturer’s instructions. ONT sequencing was carried out at the RKI, while SMRT sequencing was conducted by GATC Biotech (Konstanz, Germany). Quality of raw sequence data was checked using FastQC v0.11.5 (Andrews et al., 2012). Additionally, Kraken v0.10.6 was used to verify the taxonomic read classification (Wood and Salzberg, 2014). For reference-based read alignments, raw reads were trimmed using Trimmomatic v0.36 (default parameters) (Bolger et al., 2014).
De novo Assembly
If available, a de novo hybrid assembly was performed based on both long (SMRT/ONT) and short (NGS) reads using Unicycler v0.4.7 with conservative parameters (conservative mode) (Wick et al., 2017). Illumina-only read sets were de novo assembled using SPAdes v2.1.3 with default parameters but activated careful mode (mismatch correction) (Bankevich et al., 2012).
Phylogenetic Analysis
Multilocus sequence typing (MLST) and core genome MLST (cgMLST) were performed using de novo assembled contigs (see section “De novo assembly”) and Ridom SeqSphere+ v6.0.0 (Ridom; Münster, Germany). The following cgMLST templates were used for E. coli and K. pneumoniae, respectively: E. coli cgMLST2 and K. pneumoniae sensu lato cgMLST3. For Citrobacter freundii and E. cloacae, an ad hoc cgMLST was established according to Ridom SeqSphere+ guidelines4. For this purpose, C. freundii CAV1321 (GenBank: CP011612.1), and Enterobacter hormaechei 20710 (GenBank: CP030076.1) were selected as seed genomes. Minimum spanning trees were inferred by ignoring pairwise missing values. Since no stable task templates are available for Morganella morganii, phylogenetic relationships were reconstructed on the level of single nucleotide polymorphisms (SNPs). A reference sequence was selected by using refRank (Becker et al., 2018a) with a set of 11 complete M. morganii genome sequences available at RefSeq (Park et al., 2006). Subsequently, trimmed paired-end reads were aligned to Morganella morganii subsp. morganii KT (NC_020418) using our in-house pipeline batchMap5 as previously described (Halbedel et al., 2018). Alignment of consensus sequences were reduced to variant positions using SNPFilter v3.2.3 with default parameters (Becker et al., 2018a) and then used to calculate distance matrices and minimum spanning trees (Geneious v11.1.5).
Resistance Gene and Plasmid Replicon Identification
De novo assembled contigs were used for both resistance gene and plasmid replicon type identification. For the detection of acquired AMR genes, the ResFinder tool v3.2 was used (Zankari et al., 2012). In silico replicon typing was performed with the PlasmidFinder v2.0 (Carattoli et al., 2014). Both tools are available at http://www.genomicepidemiology.org/.
Plasmid and Transposon Reconstruction
For the reconstruction of outbreak related MGEs, different strategies have been used (Figure 1). Briefly, de novo assembled contigs were screened for blaNMD–1, blaKPC–2, and replication sequences using BLAST (Camacho et al., 2009). In order to identify defective assemblies, trimmed Illumina reads were aligned to the selected contigs (Geneious v11.1.5 Mapper with default parameters and fine tuning including up to five iterations) and manually searched for unaligned regions. Unaligned regions were checked using Sanger sequencing (see below) and corrected if necessary (pKP39-T3). In two cases (pEC405-T3, pPS-T1), the detected resistance gene and replication sequences (associated by Southern hybridization and PBRT as described before) were located on separate contigs. These contigs were annotated using a customized database of relevant sequence motifs such as repeats and transposases (Geneious v11.1.5). Corresponding elements (e.g., IS26 IRR and IRL) located at contig ends were used to merge respective contigs. In several cases (pCF104-T3, pPS-T1, pKP15-T2, pEC744-T5), contigs had to be extended by iterative alignment of trimmed Illumina reads to detect matching elements at their ends (Geneious v11.1.5 Mapper). Merging sites and contig orientation were validated using PCR (see below). To form a circular sequence, (merged) contigs were checked for identical sequence ends. When indicated by complementary motifs (e.g., IS4321 IRL and IRR), the respective element was added (pKP39-T3, pKP39-T4, pPS-T1, pEC6332-T3). Ring closing sites were validated using Sanger sequencing and/or PCR (see below). To check its integrity, both trimmed Illumina reads and de novo assembled (corrected) contigs were aligned to the final sequence (Supplementary Data Sheet 2). Samtools v1.9 (Li et al., 2009) were used to check for proper read pairing (fixmate) and to remove potential PCR duplicates (rmdup). After this, reads were aligned using Bowtie v2.3.5 with stringent parameter settings (–end-to-end –very-sensitive –qc-filter –gbar 30 –no-1 mm-upfront -R 10 –score-min L,0,-0.2) to prevent misleading alignments of very similar but still different reads of repetitive elements from other locations/replicons. Contigs were aligned using Mummer3/Nucmer v3.23 (Kurtz et al., 2004). Sequencing depth was assessed using samtools v1.9 (depth). After the reconstruction, final sequences were used as a reference for isolates that should have the same plasmid based on results from Southern hybridization and PBRT. Respective sets of Illumina reads and de novo assembled contigs were aligned using Geneious (Geneious v11.1.5 Mapper with default parameters and fine tuning including up to five iterations). Unaligned regions indicating structural variations (insertions) were corrected based on results from sequence homology searches using BLAST resulting in plasmid variants (pEC405-T3, pEC6332-T7, pCF104-T3). A detailed description of the reconstruction of each plasmid reported in this study can be found in Supplementary Data Sheet 1.
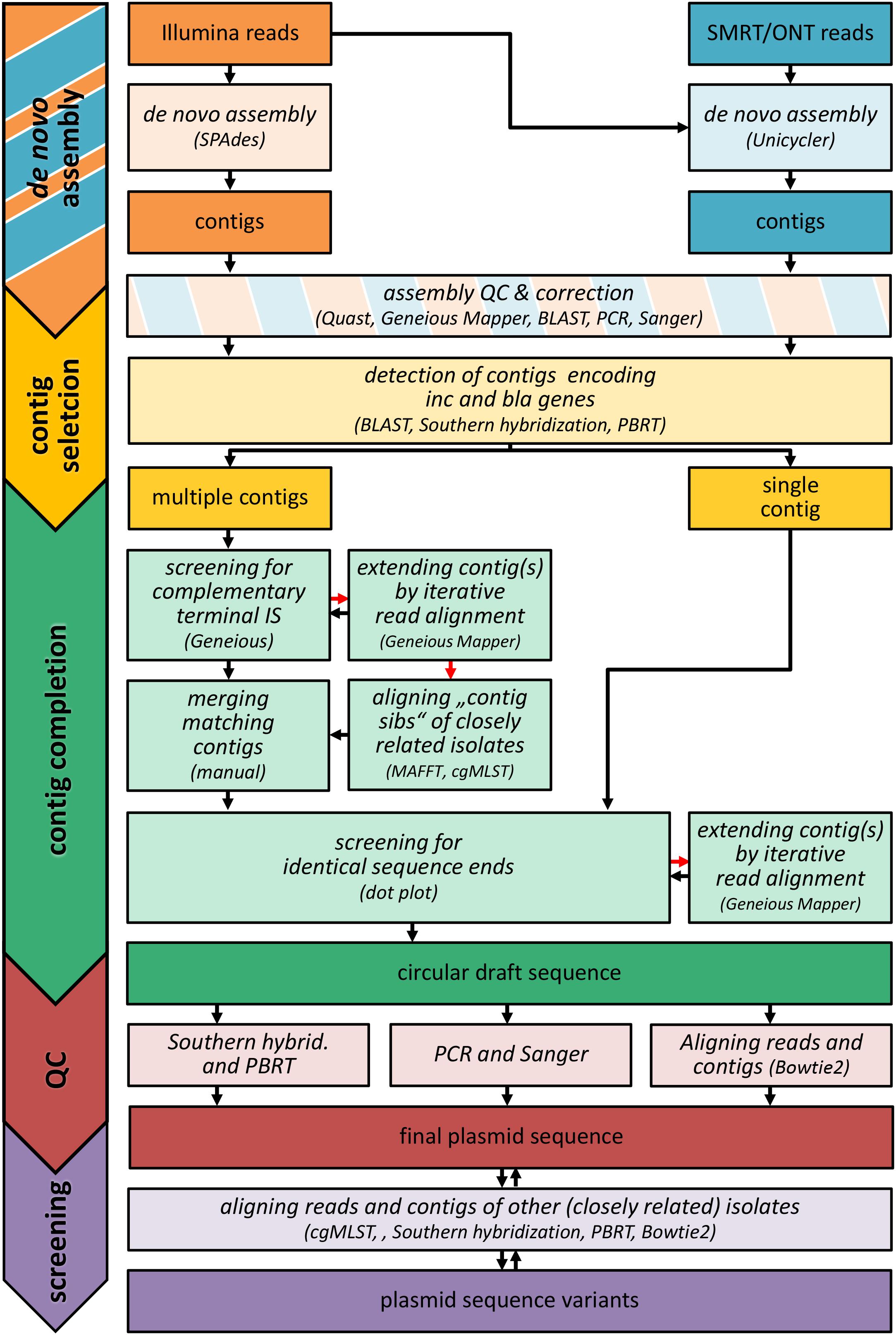
Figure 1. Workflow of plasmid reconstruction. The graphic illustrates the different strategies used for the reconstruction of outbreak-related replicon sequences in this study. The different analyses tasks are shown in italics (bright colored boxes). The respective input and output (reads, contigs, draft and final sequences) is highlighted by boxes with strong colors. If a method could not successfully applied, alternative methods were used (indicated by red arrows). For a detailed description of the reconstruction process, see Supplementary Data Sheet 1. SMRT: PacBio® Single Molecule Real Time (SMRT) sequencing; ONT, Oxford Nanopore Technology; Inc, replication sequences; bla, β-lactamase gene; IS, insertion sequence; QC, quality control; PBRT, PCR-based replication typing; contig sib(ling)s, orthologous contigs; cgMLST, core genome MLST.
Verification of Plasmid Reconstruction
DNA for Sanger sequencing and PCR-based validation (Thermocycler Biometra Tadvanced; Analytic Jena AG, Jena, Germany) of ring closing sites was extracted with the DNeasy Blood & Tissue Kit (Qiagen, Hilden, Germany). Primers were obtained from Integrated DNA Technologies (Leuven, Belgium) (Supplementary Table 2). Sanger sequencing was performed with the BigDye® v3.1 Cycle Sequencing Kit (Thermo Fisher Scientific, Waltham, MA, United States) according to the manufacturer’s instructions. For capillary gel electrophoresis the Applied Biosystems® 3500 series (Thermo Fisher Scientific, Waltham, MA, United States) was used.
Results
Epidemiological Setting
In July 2015, a carbapenem-resistant C. freundii was detected during a routine screening in a German hospital with a capacity of approximately 1,000 beds. The hospital includes 18 hospital wards that cover almost all medical disciplines. As confirmed by the NRC, carbapenem resistance was mediated by the metallo-β-lactamase NDM-1 (gene blaNDM–1). By an established prospective search including both admission and weekly screenings, 51 carbapenem-resistant Enterobacterales producing NDM-1 and/or KPC-2 were detected. These were collected from 38 patients and three environmental sources (rinse water samples) across seven different wards (I-VII) between July 2015 and October 2017. Patients were either colonized (n = 31; 36 isolates), infected (n = 5; 7 isolates) or both (n = 2; 5 isolates). Most patients were primarily hospitalized at the hematologic oncology ward I (Supplementary Table 1). The majority of isolates originated from rectal swabs (n = 41). Further isolates were collected from catheter tips (n = 2), intraabdominal sources (n = 2), urine culture (n = 1), wound (n = 1), and respiratory tract (n = 1) (Supplementary Table 1). Containment and finally cessation of transmission was achieved by extensive staff training and the introduction of substantial organizational, structural and infection control measures. No new cases were observed since the last involved patient (n = 45) had been discharged in August 2018 (Figure 2).
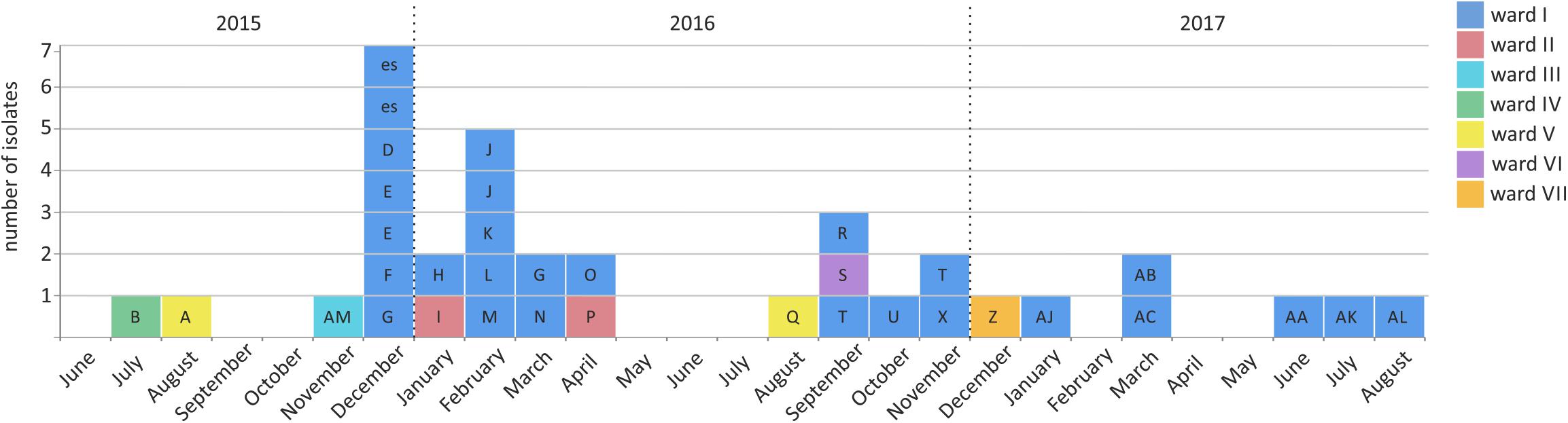
Figure 2. Emergence of NDM-1-producing Enterobacterales between July 2015 and October 2017 in a German hospital. In total, 35 NDM-1 producing Enterobacterales isolates have been collected from 29 patients (indicated by capital box letters) and two environmental samples (labeled as “es”) across different wards (indicated by box colors).
Microbiological Investigation and Molecular Characterization
The blaNDM–1 gene was detected in 35 isolates from 29 patients and two environmental sources (Figure 2, Supplementary Table 1). NDM-1-positive isolates were identified as C. freundii (n = 3), E. cloacae (n = 2), E. coli (n = 18), K. pneumoniae (n = 8), M. morganii (n = 3) and Providencia stuartii (n = 1). Among these, seven isolates additionally encoded KPC-2. Moreover, we identified 16 blaNDM–1 negative but blaKPC–2 positive isolates from 13 patients and one environmental source, that revealed K. pneumoniae (n = 15) and E. coli (n = 1) species (Supplementary Table 1). All isolates exhibited resistance to ampicillin, cefoxitin, cefotaxime, ceftazidime, piperacillin/tazobactam, imipenem and meropenem. Resistance to ciprofloxacin and sulfamethoxazole/trimethoprim was detected in 98% of all isolates, and several K. pneumoniae were additionally resistant to tigecycline (see Supplementary Table 1 for more details). Resistance to colistin was only measured in intrinsically resistant isolates (M. morganii and P. stuartii). Besides blaNDM–1 and blaKPC–2 additional β-lactamase genes were detected by PCR screening, and these were additionally confirmed by WGS analyses. These included blaTEM–1 (n = 36), blaOXA–1 (n = 32), blaCTX–M–15 (n = 29), blaOXA–9 (n = 15), blaSHV–28 (n = 12), blaCMY–4 (n = 9), blaSHV–1 (n = 7), blaSHV–11 (n = 4), blaCTX–M–1 (n = 2), blaCMY–108 (n = 1), blaOXA–10 (n = 1), blaOXA–2 (n = 1), blaACC–4 (n = 1), blaTEM–116 (n = 1) and blaACT–7 (n = 1) (Supplementary Table 1). PFGE analyses revealed different NDM and/or KPC-producing strains of E. coli (n = 4), K. pneumoniae (n = 4), C. freundii (n = 2), M. morganii (n = 1), E. cloacae (n = 1), and P. stuartii (n = 1) (Supplementary Table 1). Conjugation assays combined with S1-nuclease PFGE, PBRT and hybridization experiments showed an association of blaNDM–1 with plasmids of different sizes and Inc types (Supplementary Table 1).
WGS-Based Phylogenetic Analyses
cgMLST and SNP-based analyses revealed different clusters of genetically highly related isolates, indicating a clonal spread. The 19 E. coli were assigned to three clusters and one singleton that were named according to the respective sequence types (STs) (Figure 3A). The three clusters comprised nine ST744 isolates, seven ST6332 isolates, and two ST405 isolates, respectively, which was consistent with PFGE results (Supplementary Table 1). Within each cluster, isolates did not show more than eight allele differences in the cgMLST (Figure 3A). Three different clusters and one singleton have also been detected for the 22 K. pneumoniae isolates hereinafter referred to as ST307 (n = 11), ST39 (n = 7), ST37 (n = 3), and ST15 (n = 1) (Figure 3B). Less than eleven different alleles were detected between K. pneumoniae isolates of the same cluster (Figure 3B). For three C. freundii isolates, the WGS-derived MLST revealed two new STs (ST104 and ST105) of which the two ST104 isolates showed differences in seven alleles only (Figure 3C). Likewise, both ST231 E. cloacae isolates were closely related with differences in only four alleles (Figure 3D). Since a cgMLST scheme was not yet available for M. morganii, we used a SNP-based analysis to investigate the genetic relationship between those isolates. By this, a maximal difference of six SNPs was found (data not shown).
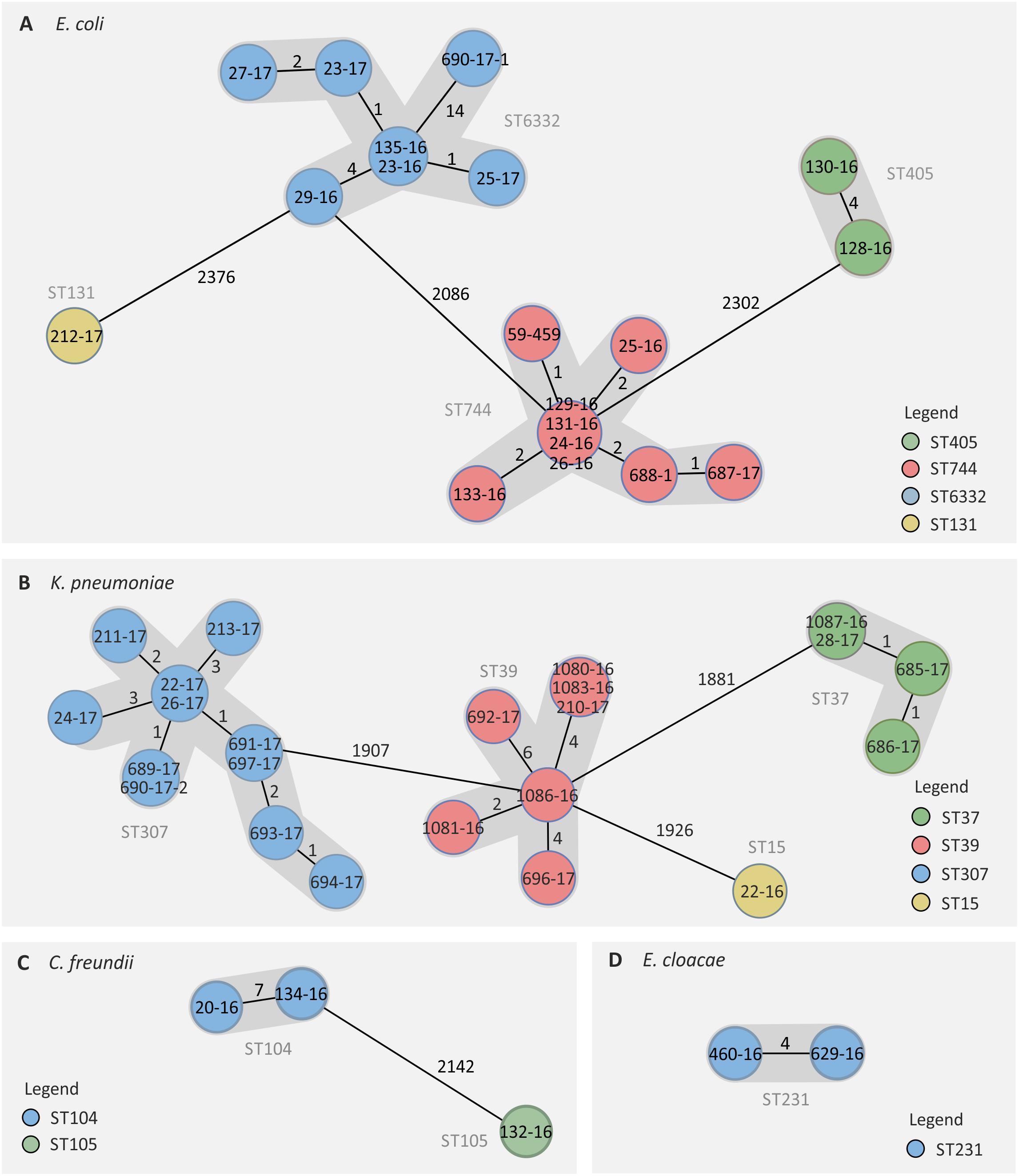
Figure 3. Genetically related clusters of NDM-1-positive Enterobacterales based on cgMLST. For E. coli (A), K. pneumoniae (B), C. freundii (C), and E. cloacae (D) isolates, genetic distances were calculated based on the allelic profiles of 2513, 2159, 4156, and 2385 target genes, respectively (pairwise ignored missing values). The number of different alleles between isolates is shown next to black lines. Please note: the distances displayed are not proportional to the number of differing targets. Isolate names are shown within color-coded circles representing MLST-based sequence types.
Plasmid and Transposon Analyses
To assess whether blaNDM–1 associated MGEs in the different Enterobacterales isolates were genetically related or not, WGS data were used to reconstruct the involved genetic elements. For this purpose, data from short- and long-read sequencing were utilized in combination with results of conjugation assays, S1-PFGE-based plasmid size determination and Southern hybridization, thus allowing the complete reconstruction of eleven blaNDM–1 carrying plasmids present in 34 isolates (Table 1 and Supplementary Image 1). Only for one isolate of this study (132-16 C. freundii-ST105), complete reconstruction of the blaNDM–1 associated replicon (pCF105-T8) failed. A detailed description of the sequence reconstruction processes is given in Supplementary Data Sheet 1. The majority of reconstructed blaNDM–1 carrying plasmids belonged to incompatibility group IncA/C2 (n = 8) followed by IncN (n = 2) and IncFII/FIB (n = 1) (Table 1). Plasmids pCF104-T3 (IncA/C2) and pKP15-T2 (IncFII/FIB) originated from isolates of patients with a travel history and showed a unique genetic organization (Figure 4). They were not considered to be involved in local transmission events and therefore not addressed in further details.
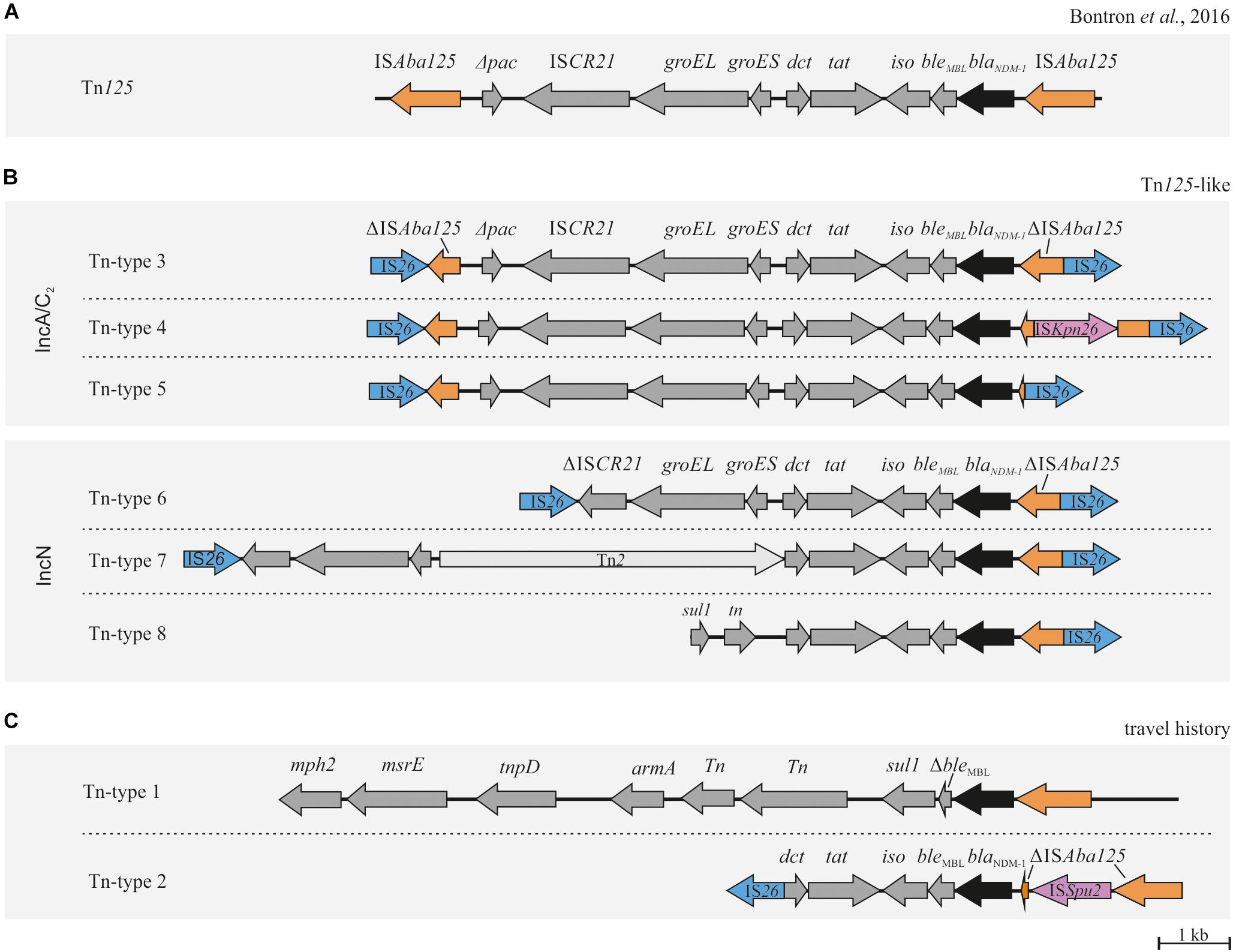
Figure 4. Genetic organizations of blaNDM–1 associated Tn125 composite transposons. (A) Structure of the genuine Tn125 composite transposon originally described by Bontron et al. (2016). (B) Organization of Tn125-like structures in plasmids potentially involved in horizontal transmission. The complete reconstruction of pCF105-T8 failed (Tn-type 8). (C) blaNDM–1 associated structures from isolates of patients with travel history demonstrating a different Tn structure. ORFs and respective orientations are represented by arrows. IS26 elements are indicated in blue, ISAba125 and ΔISAba125 in orange, blaNDM–1 in black and other ORFs in dark gray.
Interestingly, seven of the eight IncA/C2 plasmids (pCF104-T3, pEC744-T5, pEC405-T3, pECl-T3, pEC6332-T3, pKP39-T4, and pKP39-T3) shared a highly conserved backbone structure (Supplementary Image 1). However, they varied in distinct regions containing deletions, additional genes or MGEs and/or multi-replicon/hybrid plasmid elements. These regions were located upstream the antibiotic resistance island ARI-A that mainly affected genes coding for conjugative transfer proteins (Supplementary Image 1). Within ARI-A, we found the blaNDM–1 gene located on a previously described Tn125 transposon structure (Bontron et al., 2016). This structure, however, was consistently flanked by two IS26 elements which were integrated into the adjacent ISAba125 elements of Tn125 (Figure 4). As a consequence, the genuine composite transposon structure of Tn125 is turned into an IS26-dependent composite transposon structure. Owing to this variation, we will refer to it as “Tn125-like.” In the seven IncA/C2 plasmids three different Tn125-like transposon types (Tn-types) had been identified that showed only minor structural variations (Tn-types 3–5) (Table 1 and Figure 4).
In the E. cloacae isolate 629-16, we found the IncA/C2 plasmid pECl-T3 not only present as plasmid but additionally integrated into the bacterial chromosome (i460-16; potentially flanked by two IS26 elements). This observation was confirmed by hybridization results (data not shown). The second E. cloacae isolate, 460-16, possessed only the chromosomal variant i460-16 flanked by two IS26 elements as confirmed by ONT.
The K. pneumoniae isolates 1080-16, 1081-16, 1083-16, 1086-16, 210-17, 692-17, and 696-17 did not only possess the IncA/C2 plasmids pKP39-T4 or pKP39-T3 but also a blaKPC–2 carrying plasmid designated as pKPC-2. This plasmid (pKPC-2) was found in all NDM-1-positive ST39 K. pneumoniae isolates and was also found in other K. pneumoniae isolates (ST37 and ST307) (Supplementary Image 2).
In accordance to results from conjugation and hybridization experiments, blaNDM–1 has also been found to be located on the IncN plasmid pEC6332-T6 in several isolates (Table 1). In pEC6332-T6, the above described IS26 flanked composite transposon has been integrated upstream of Hsp20 and a putative transposase (Supplementary Image 1). The involved transposon structure (Tn-type 6) showed only minor deletions compared to the Tn-type 3 found in IncA/C2 plasmids (Figure 4). Interestingly, pEC6332-T6 could be detected in both E. coli (n = 6) and M. morganii (n = 3) isolates (Table 1) suggesting interspecies transmission. A minor structural variation of pEC6332-T6 (named pEC6332-T7) was present in four E. coli isolates (Table 1). This variant is defined by an insertion of Tn2 in the Tn125-like transposon structure of pEC6332-T6, thereby forming a new transposon and plasmid structure (Tn-type 7) (Figure 4).
Discussion
Since carbapenems belong to the most important last resort antibiotics for the treatment of infections caused by MDR Gram-negative bacteria, the emergence of bacteria producing carbapenem hydrolysing enzymes is of particular interest. KPC-2 producing strains, especially K. pneumoniae, have been frequently reported in Europe; whereas the number of reports on NDM-1 producers is much smaller (ECDC, 2019; Fairley et al., 2019; Hernandez-Garcia et al., 2019). Hospital-associated infections caused by NDM-1-producing Enterobacterales have recently been described in Spain, England, and Italy (ECDC, 2019; Fairley et al., 2019; Hernandez-Garcia et al., 2019). In Germany, screening for “carbapenem-resistant Enterobacterales and A. baumanii including NDM-1-producing bacteria” is currently only recommended for patients who were previously admitted to hospitals in “high-risk countries” such as India, Pakistan and the Balkan states (KRINKO, 2012). Colonization and infections with carbapenem-resistant Enterobacterales and A. baumanii have been notifiable since 2016 in Germany. For about half of the notified cases accompanying microbiological data are available (2017: n = 1488/2633; 2018: 2061/3794). NDM-1 accounted for 13% (n = 56) in 2017 and 4% (n = 23) in 2018 of all Klebsiella spp. isolates. Moreover, 9% (n = 17) of E. coli isolates in 2017 and 5% (n = 15) in 2018 were positively tested for blaNDM–1. Considering a substantial underreporting of the number of NDM-1 cases, we could still assume and extrapolate that during the time of the present study 2015–2017, blaNDM–1 was a rare carbapenemase type in Germany. Therefore, the spatial and temporal clustering of NDM-1-positive isolates in a single hospital – as described in this study – strongly pointed to possible transmissions across different bacterial species. To verify this, we complemented and combined results from traditional typing methods such as PFGE and MLST with WGS data from NGS and ultra-long sequencing technologies. This combination made it possible to track and resolve different (potential) transmission routes (Figure 5).
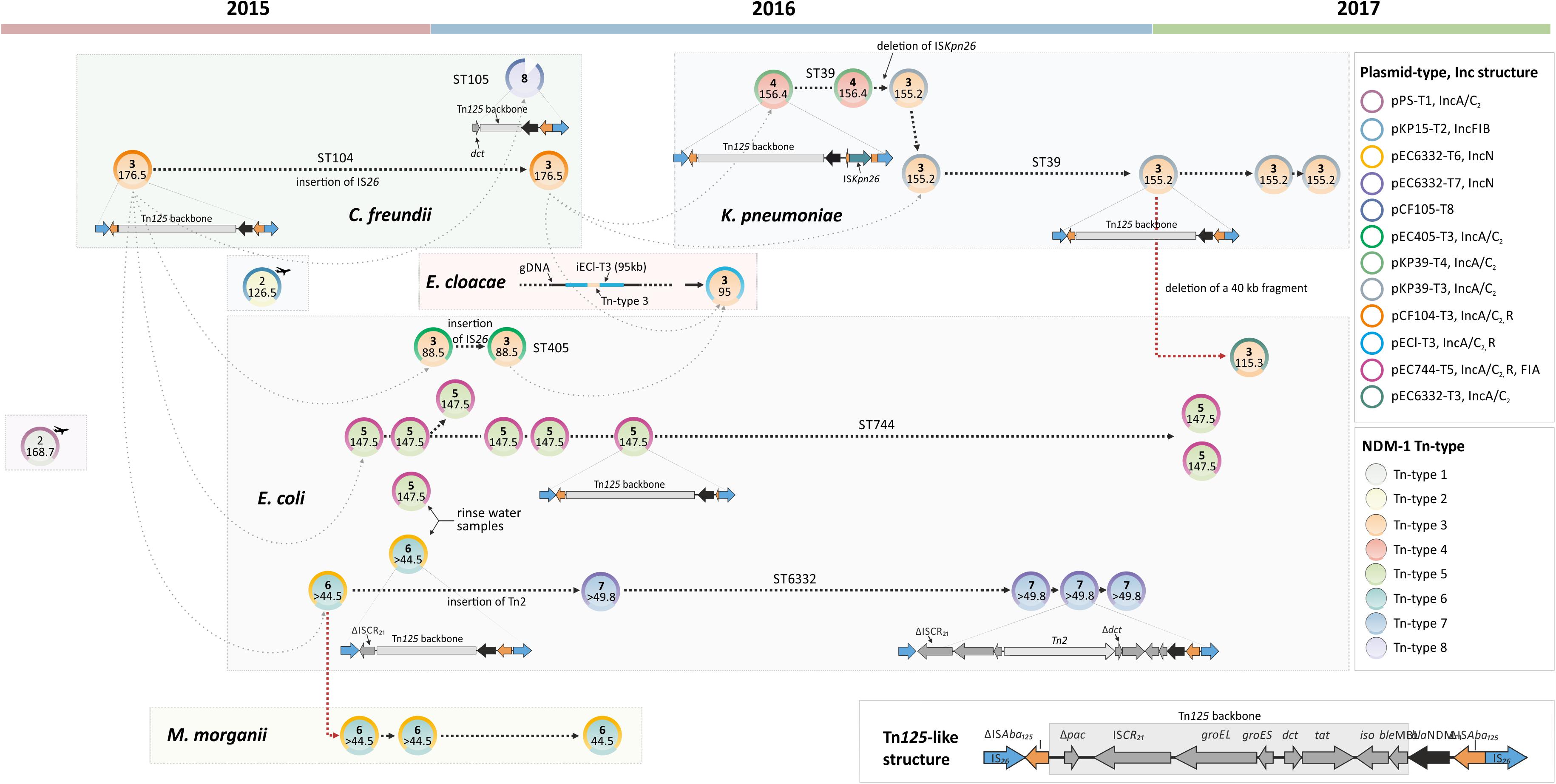
Figure 5. Scheme of NDM-1-encoding plasmids and potential intra-/interspecies transmission pathways between Enterobacterales isolates in a German hospital from 2015 to 2017. The illustrated plasmids are horizontally aligned to date of isolation of the respective strain. Plasmid- and Tn-types are represented by color-coded circles and fillings, respectively (see legend on right side). Upper numbers (bold) within plasmid rings highlight Tn-types, while the numbers below show the plasmid size [kb]. The reconstructed transposon structures are schematically illustrated beneath the corresponding plasmids. Directed dotted lines indicate possible vertical (black) and horizontal (red) transmission routes, while gray-shaded lines hypothesize possible transfers of the respective blaNDM–1 carrying transposon. At the bottom, the Tn125-like structure is shown in detail (Tn-type 3). Two blaNDM–1 carrying plasmids belong to isolates collected from patients with a travel history and, thus not linked to the outbreak (indicated by airplane symbols).
Both PFGE and cgMLST analyses identified consistent clusters of genetically highly related isolates (e.g., E. coli ST744, ST6332 or K. pneumoniae ST307, Figure 3) suggesting a clonal spread of these strains (most likely by person-to-person transmission) and, thus, the vertical transmission of NDM-1. Isolates belonging to the largest clonal clusters formed by E. coli ST744 (n = 9) and ST6332 (n = 7) were detected almost within the entire screening period. Clonal spread of NDM-1-positive species have been described in various parts of the world mainly caused by K. pneumoniae and E. cloacae (Jin et al., 2015; Mahida et al., 2017; Bocanegra-Ibarias et al., 2019; Fairley et al., 2019; Monteiro et al., 2019). Nosocomial outbreaks caused by K. pneumoniae ST39, ST37, and ST307 have also been reported worldwide (Belbel et al., 2014; Zhu et al., 2016; Bocanegra-Ibarias et al., 2019). Moreover, the here detected K. pneumoniae ST37 and ST307 in combination with KPC-2 production were classified as “high-risk clones” (Yan et al., 2015; Villa et al., 2017).
Isolates of different NDM-1-producing species (E. coli and M. morganii) collected from different patients shared the identical plasmid (pEC6332-T6) suggesting horizontal gene spread as the most probable route of transmission. Several studies already reported a horizontal transfer of blaNDM–1 and/or other plasmid encoded resistance determinants between different enterobacterial species linked to nosocomial outbreaks (Borgia et al., 2012; Bosch et al., 2017; Schweizer et al., 2019). However, using traditional molecular typing methods we were able to locate blaNDM–1 on plasmids of various sizes and Inc types (Supplementary Table 1 and Figure 5). The limited resolution of techniques used beforehand hardly allowed a reliable reconstruction of possible transmission events via smaller MGEs such as transposons. It makes whole genome sequencing including long read sequencing technologies indispensable to resolve such scenarios adequately (Salipante et al., 2015). In the present study, however, molecular typing information proved to be extremely helpful, if not essential, for the reconstruction of replicon sequences (see Supplementary Data Sheet 1).
In this study, blaNDM–1 was mainly associated with IncA/C2 plasmids. These broad host range plasmids have been associated with transcontinental spread of blaNDM–1 in Asia, North America, Africa and Europe and have frequently been described to carry various resistance determinants including β-lactamase genes like blaNDM–1 and blaCMY–2 (Fernandez-Alarcon et al., 2011; Harmer and Hall, 2015; Pietsch et al., 2018; Rozwandowicz et al., 2018).
Interestingly, in 2017 an outbreak of NDM-1-producing K. pneumoniae was described in a Dutch hospital with an inter-species transfer of a blaNDM–1 carrying IncA/C2 plasmid (Bosch et al., 2017). The sequence of this plasmid shared significant sequence similarities with the plasmids pKP39-T3 and pKP39-T4 described in the present study (data not shown). This similarity may be attributed to the fact that IncA/C2 plasmids usually possess a conserved backbone structure including characteristic core regions such as the conjugative transfer region (tra) and genes for plasmid maintenance (Harmer and Hall, 2015). In line with these findings, we found a conserved backbone structure for all IncA/C2 plasmids analyzed in this study (Supplementary Image 1). However, several structural variations were found in close proximity to the antibiotic resistance island ARI-A such as the acquisition of additional genetic elements and/or the loss of tra genes in pCF104-T3, pEC744-T5, pEC405-T3, pECl-T3, and pEC6332-T3 (Supplementary Image 1). Broth mate conjugation assays were not successful for the most IncA/C2 plasmids of this study (see Supplementary Table 1). Fernandez-Alarcon et al. (2011) previously described rearrangements in tra regions of several plasmids carrying ISEcp1-blaCMY–2 which might correlate with transmission deficiency. Due to their high sequence variability it is unlikely that all IncA/C2 plasmids originated from a common source.
A more detailed analysis of the plasmids of the present study showed that blaNDM–1 is located in a conserved sequence within the ARI-A of IncA/C2 and various sites of IncN plasmids, respectively (Figure 4 and Supplementary Image 1). This structure has been previously described as Tn125 (Bontron et al., 2016). In 2016, Bontron et al. (2016) demonstrated that the Tn125 composite transposon can efficiently transpose within A. baumanii which most likely serves as an intermediate reservoir for the blaNDM–1 gene. The genetic organization of Tn125 is defined by terminal ISAba125 elements that have been shown to be functional for transposition (Bontron et al., 2016). It is assumed that the high prevalence of ISAba125 in Acinetobacter spp. genomes indicates frequent transposition events, the presence of HGT, a replicative transposition mechanism, and the ability of ISAba125 to expand within genomes (Bontron et al., 2016). In the present study we found this genuine Tn125 structure slightly changed by the integration of IS26 into each ISAba125 element (Figure 4). The blaNDM–1 gene described in almost all isolates in our study was found to be located within Tn125-like structures supporting the theory of a common origin of blaNDM–1 (Figure 4). In 2015, a very similar transposon associated with blaNDM–4 was identified in E. coli isolates from a transmission event in a Chinese hospital (Zhang et al., 2018). Similar to our findings IS26 inserted into ISAba125 upstream of blaNDM–4, however, its orientation was not reverse to blaNDM–4 and a duplication of IS26 was present in ISAba125. Moreover, an IS26 element (in reverse orientation to blaNDM–4) was found instead of a downstream ISAba125. The authors hypothesized that the two flanking IS26 have the potential to form a mobile composite transposon. Supporting this, IS26 elements are known to be crucial for the acquisition and dissemination of resistance genes and have also been reported to be involved in transposon building (Argudin et al., 2017; Montana et al., 2017). Thus, IS26 might be responsible for the transposition of composite transposons between different plasmids. Likewise we hypothesize that IS26 plays a key role in the mobilization of the identified blaNDM–1-IS26 composite transposon structures. We found only minor structural variations of the IS26-flanked Tn125 composite transposon among our blaNDM–1 carrying IncA/C and IncN plasmids (IncA/C2 and IncN) that might indicate that these transposons were exchanged across different plasmids. Based on the detected variations mainly occurred in regions of IS26 in ISAba125 we defined six different Tn-types (Figure 4 and Supplementary Image 1). Based on these variations we hypothesized a certain transposon flux with Tn-type 3 as a driving force.
Conclusion
In conclusion, we depicted different transmissions of NDM-1-encoding MGEs within isolates of various bacterial species present at the same time in a single hospital. A clonal spread of different NDM-1-producing Enterobacterales strains was clearly supported by cgMLST analyses for E. coli, K. pneumoniae, C. freundii, and E. cloacae. Furthermore, there are clear indications of inter-species transmission of IncN plasmids carrying blaNDM–1, and potential transmission of mobilized Tn125-like transposons with blaNDM–1 into different plasmids or into the chromosome. Reliable reconstruction of these complex transmission routes was only possible by a combination of traditional plasmid typing methods and hierarchical bioinformatics analyses using NGS and SMRT/ONT data. An assumption or molecular evidence of a clonal spread of resistant strains is essential to initiate infection control and prevention measures. On the other hand, multispecies outbreaks often go undetected. The situation described in this study only became clear through the comparatively high prevalence of a rare carbapenemase gene. We therefore assume a certain underestimation of “plasmid- and/or transposon-driven outbreaks” in general.
Data Availability Statement
Raw reads datasets generated for this study can be found in the European Nucleotide Archive (https://www.ebi.ac.uk/ena; Study accession number: PRJEB34353). Plasmid assemblies can be found in GenBank under the following accessions: MN657241 (pCF104a-T3), MN657242 (pEC405a-T3), MN657243 (pEC744-T5), MN657244 (pEC6332-T3), MN657245 (pEC6332-T6), MN657246 (pEC6332-T7), MN657247 (pECl-T3), MN657248 (pKP15-T2), MN657249 (pKP39-T3), MN657250 (pKP39-T4), MN657251 (pKPC-2), and MN657252 (pPS-T1).
Author Contributions
GW, SG, YP, and SF conceptualized and designed the study. MM and DL collected the isolates and metadata, and primary diagnostics and strain characterization. MK, NP, YP, and SG were involved in confirmatory diagnostics and strain characterization. AF was involved in WGS sequencing. RW, MP, AF, SF contributed to the data curation and analyses. RW, MP, SF, YP, and GW wrote the manuscript. All authors edited and reviewed the manuscript.
Funding
This study was supported by a grant no. 643476 to the project COMPARE under the European Union’s Horizon 2020 Research and Innovation Program. The analyses received additional support by the Robert Koch-Institute’s internal funding for establishing genome-based pathogen surveillance and outbreak analyses (Molecular Surveillance Grant 2017).
Conflict of Interest
The authors declare that the research was conducted in the absence of any commercial or financial relationships that could be construed as a potential conflict of interest.
Acknowledgments
We are thankful for excellent technical assistance by Christine Günther, Sibylle Mueller-Bertling, and Kirstin Ganske. We thank Dr. Jennifer Bender for fruitful discussions of WGS results. We also thank Drs. Annicka Reuss and Sebastian Haller for providing detailed data of NDM-1 cases in Germany as extracted from the mandatory notification system for carbapenemase-producing E. coli and Klebsiella spp. in Germany for this report.
Supplementary Material
The Supplementary Material for this article can be found online at: https://www.frontiersin.org/articles/10.3389/fmicb.2019.02817/full#supplementary-material
Footnotes
- ^ www.eucast.org
- ^ https://enterobase.warwick.ac.uk/species/index/ecoli
- ^ www.ridom.de/seqsphere/u/Task_Template_Sphere.html
- ^ www.ridom.de/seqsphere/u/Tutorial_for_Ad_hoc_cgMLST_Scheme.html
- ^ https://gitlab.com/s.fuchs/batchMap
References
Andrews, S., Krueger, F., Segonds-Pichon, F., Biggins, L., Krueger, C., and Wingett, S. (2012). FastQC: A Quality Control Tool for High Throughput Sequence Data. Cambridge: Babraham institude.
Argudin, M. A., Deplano, A., Meghraoui, A., Dodemont, M., Heinrichs, A., Denis, O., et al. (2017). Bacteria from animals as a pool of antimicrobial resistance genes. Antibiotics 6:E12. doi: 10.3390/antibiotics6020012
Bankevich, A., Nurk, S., Antipov, D., Gurevich, A. A., Dvorkin, M., Kulikov, A. S., et al. (2012). SPAdes: a new genome assembly algorithm and its applications to single-cell sequencing. J. Comput. Biol. 19, 455–477. doi: 10.1089/cmb.2012.0021
Baraniak, A., Izdebski, R., Fiett, J., Gawryszewska, I., Bojarska, K., Herda, M., et al. (2016). NDM-producing Enterobacteriaceae in Poland, 2012-14: inter-regional outbreak of Klebsiella pneumoniae ST11 and sporadic cases. J. Antimicrob. Chemother. 71, 85–91. doi: 10.1093/jac/dkv282
Barton, B. M., Harding, G. P., and Zuccarelli, A. J. (1995). A general method for detecting and sizing large plasmids. Anal. Biochem. 226, 235–240. doi: 10.1006/abio.1995.1220
Becker, L., Fuchs, S., Pfeifer, Y., Semmler, T., Eckmanns, T., Korr, G., et al. (2018a). Whole genome sequence analysis of CTX-M-15 producing Klebsiella isolates allowed dissecting a polyclonal outbreak scenario. Front. Microbiol. 9:322. doi: 10.3389/fmicb.2018.00322
Becker, L., Kaase, M., Pfeifer, Y., Fuchs, S., Reuss, A., Von Laer, A., et al. (2018b). Genome-based analysis of carbapenemase-producing Klebsiella pneumoniae isolates from German hospital patients, 2008-2014. Antimicrob. Resist. Infect. Control 7:62. doi: 10.1186/s13756-018-0352-y
Belbel, Z., Chettibi, H., Dekhil, M., Ladjama, A., Nedjai, S., and Rolain, J. M. (2014). Outbreak of an armA methyltransferase-producing ST39 Klebsiella pneumoniae clone in a pediatric Algerian Hospital. Microb. Drug Resist. 20, 310–315. doi: 10.1089/mdr.2013.0193
Bocanegra-Ibarias, P., Garza-Gonzalez, E., Padilla-Orozco, M., Mendoza-Olazaran, S., Perez-Alba, E., Flores-Trevino, S., et al. (2019). The successful containment of a hospital outbreak caused by NDM-1-producing Klebsiella pneumoniae ST307 using active surveillance. PLoS One 14:e0209609. doi: 10.1371/journal.pone.0209609
Bolger, A. M., Lohse, M., and Usadel, B. (2014). Trimmomatic: a flexible trimmer for illumina sequence data. Bioinformatics 30, 2114–2120. doi: 10.1093/bioinformatics/btu170
Bontron, S., Nordmann, P., and Poirel, L. (2016). Transposition of Tn125 encoding the NDM-1 carbapenemase in Acinetobacter baumannii. Antimicrob. Agents Chemother. 60, 7245–7251.
Borgia, S., Lastovetska, O., Richardson, D., Eshaghi, A., Xiong, J., Chung, C., et al. (2012). Outbreak of carbapenem-resistant Enterobacteriaceae containing blaNDM-1. Ontario, Canada. Clin. Infect. Dis. 55, e109–e117. doi: 10.1093/cid/cis737
Bosch, T., Lutgens, S. P. M., Hermans, M. H. A., Wever, P. C., Schneeberger, P. M., Renders, N. H. M., et al. (2017). Outbreak of NDM-1-producing Klebsiella pneumoniae in a Dutch Hospital, with interspecies transfer of the resistance plasmid and unexpected occurrence in unrelated health care centers. J. Clin. Microbiol. 55, 2380–2390. doi: 10.1128/JCM.00535-17
Bush, K. (2016). Overcoming beta-lactam resistance in gram-negative pathogens. Future Med. Chem. 8, 921–924. doi: 10.4155/fmc-2016-0076
Camacho, C., Coulouris, G., Avagyan, V., Ma, N., Papadopoulos, J., Bealer, K., et al. (2009). BLAST+: architecture and applications. BMC Bioinformatics 10:421. doi: 10.1186/1471-2105-10-421
Carattoli, A., Zankari, E., Garcia-Fernandez, A., Voldby Larsen, M., Lund, O., Villa, L., et al. (2014). In silico detection and typing of plasmids using plasmidfinder and plasmid multilocus sequence typing. Antimicrob. Agents Chemother. 58, 3895–3903. doi: 10.1128/AAC.02412-14
Clowes, R. C., and Rowley, D. (1954). Some observations on linkage effects in genetic recombination in Escherichia coli K-12. J. Gen. Microbiol. 11, 250–260. doi: 10.1099/00221287-11-2-250
Curran, E. T., and Otter, J. A. (2014). Outbreak Column 15: carbapenemase-producing Enterobacteriaceae. J. Infect. Prev. 15, 193–198. doi: 10.1177/1757177414546707
Doi, Y., and Arakawa, Y. (2007). 16S ribosomal RNA methylation: emerging resistance mechanism against aminoglycosides. Clin. Infect. Dis. 45, 88–94. doi: 10.1086/518605
Dortet, L., Poirel, L., and Nordmann, P. (2014). Worldwide dissemination of the NDM-type carbapenemases in gram-negative bacteria. Biomed. Res. Int. 2014:249856. doi: 10.1155/2014/249856
ECDC (2019). Regional Outbreak of New Delhi Metallo-Beta-Lactamase Producing Carbapenem-Resistant Enterobacteriaceae. Italy, 2018–2019. Solna Municipality: ECDC.
Fairley, D., Protaschik, Y., Finn, R., Turton, J. F., Doumith, M., Elbaz, W., et al. (2019). Investigation of a hospital Enterobacter cloacae NDM-1 outbreak using whole genome sequencing. Access Microbiol. 1. doi: 10.1099/acmi.ac2019.po0391
Fernandez-Alarcon, C., Singer, R. S., and Johnson, T. J. (2011). Comparative genomics of multidrug resistance-encoding IncA/C plasmids from commensal and pathogenic Escherichia coli from multiple animal sources. PLoS One 6:e23415. doi: 10.1371/journal.pone.0023415
Grobner, S., Linke, D., Schutz, W., Fladerer, C., Madlung, J., Autenrieth, I. B., et al. (2009). Emergence of carbapenem-non-susceptible extended-spectrum beta-lactamase-producing Klebsiella pneumoniae isolates at the university hospital of Tubingen, Germany. J. Med. Microbiol. 58, 912–922. doi: 10.1099/jmm.0.005850-0
Halbedel, S., Prager, R., Fuchs, S., Trost, E., Werner, G., and Flieger, A. (2018). Whole-genome sequencing of recent listeria monocytogenes isolates from Germany reveals population structure and disease clusters. J. Clin. Microbiol. 56:e119-118. doi: 10.1128/JCM.00119-18
Harmer, C. J., and Hall, R. M. (2015). The A to Z of A/C plasmids. Plasmid 80, 63–82. doi: 10.1016/j.plasmid.2015.04.003
Hawkey, P. M., and Livermore, D. M. (2012). Carbapenem antibiotics for serious infections. BMJ 344:e3236. doi: 10.1136/bmj.e3236
Heinrichs, A., Argudin, M. A., De Mendonca, R., Deplano, A., Roisin, S., Dodemont, M., et al. (2019). An outpatient clinic as a potential site of transmission for an outbreak of New Delhi metallo-beta-lactamase-producing Klebsiella pneumoniae sequence type 716: a study using whole-genome sequencing. Clin. Infect. Dis. 68, 993–1000. doi: 10.1093/cid/ciy581
Hernandez-Garcia, M., Perez-Viso, B., Leon-Sampedro, R., Navarro-San Francisco, C., Lopez-Fresnena, N., Diaz-Agero, C., et al. (2019). Outbreak of NDM-1+CTX-M-15+DHA-1-producing Klebsiella pneumoniae high-risk clone in Spain owing to an undetectable colonised patient from Pakistan. Int. J. Antimicrob. Agents 54, 233–239. doi: 10.1016/j.ijantimicag.2019.05.021
Jin, Y., Shao, C., Li, J., Fan, H., Bai, Y., and Wang, Y. (2015). Outbreak of multidrug resistant NDM-1-producing Klebsiella pneumoniae from a neonatal unit in shandong province, China. PLoS One 10:e0119571. doi: 10.1371/journal.pone.0119571
Khong, W. X., Xia, E., Marimuthu, K., Xu, W., Teo, Y. Y., Tan, E. L., et al. (2016). Local transmission and global dissemination of New Delhi Metallo-Beta-Lactamase (NDM): a whole genome analysis. BMC Genomics 17:452. doi: 10.1186/s12864-016-2740-0
KRINKO (2012). Hygienemaßnahmen bei infektionen oder besiedlung mit multiresistenten gramnegativen stäbchen. Bundesgesundheitsblatt 55, 1311–1354. doi: 10.1007/s00103-012-1549-5
Kurtz, S., Phillippy, A., Delcher, A. L., Smoot, M., Shumway, M., Antonescu, C., et al. (2004). Versatile and open software for comparing large genomes. Genome Biol. 5:R12.
Li, H., Handsaker, B., Wysoker, A., Fennell, T., Ruan, J., Homer, N., et al. (2009). The sequence alignment/map format and SAMtools. Bioinformatics 25, 2078–2079. doi: 10.1093/bioinformatics/btp352
Mahida, N., Clarke, M., White, G., Vaughan, N., and Boswell, T. (2017). Outbreak of Enterobacter cloacae with New Delhi Metallo-beta-lactamase (NDM)-1: challenges in epidemiological investigation and environmental decontamination. J. Hosp. Infect. 97, 64–65. doi: 10.1016/j.jhin.2017.05.016
Montana, S., Almuzara, M., Pennini, M., Pennini, A. D. C., Vay, C., and Ramirez, M. S. (2017). ISCR2 and IS26: two insertion sequences highly dispersed among Acinetobacter spp. Clin. Strains J. Bacteriol. Mycol. 4, 33–36.
Monteiro, J., Inoue, F. M., Lobo, A. P. T., Ibanes, A. S., Tufik, S., and Kiffer, C. R. V. (2019). A major monoclonal hospital outbreak of NDM-1-producing Klebsiella pneumoniae ST340 and the first report of ST2570 in Brazil. Infect. Control Hosp. Epidemiol. 40, 492–494. doi: 10.1017/ice.2018.333
Nordmann, P., Poirel, L., Walsh, T. R., and Livermore, D. M. (2011). The emerging NDM carbapenemases. Trends Microbiol. 19, 588–595. doi: 10.1016/j.tim.2011.09.005
Park, C. H., Robicsek, A., Jacoby, G. A., Sahm, D., and Hooper, D. C. (2006). Prevalence in the United States of aac(6’)-Ib-cr encoding a ciprofloxacin-modifying enzyme. Antimicrob. Agents Chemother. 50, 3953–3955. doi: 10.1128/aac.00915-06
Paterson, D. L., and Bonomo, R. A. (2005). Extended-spectrum beta-lactamases: a clinical update. Clin. Microbiol. Rev. 18, 657–686. doi: 10.1128/cmr.18.4.657-686.2005
Pfeifer, Y., Cullik, A., and Witte, W. (2010). Resistance to cephalosporins and carbapenems in gram-negative bacterial pathogens. Int. J. Med. Microbiol. 300, 371–379. doi: 10.1016/j.ijmm.2010.04.005
Pfeifer, Y., Matten, J., and Rabsch, W. (2009). Salmonella enterica serovar Typhi with CTX-M beta-lactamase, Germany. Emerg. Infect. Dis. 15, 1533–1535. doi: 10.3201/eid1509.090567
Pfeifer, Y., Wilharm, G., Zander, E., Wichelhaus, T. A., Gottig, S., Hunfeld, K. P., et al. (2011). Molecular characterization of blaNDM-1 in an Acinetobacter baumannii strain isolated in Germany in 2007. J. Antimicrob. Chemother. 66, 1998–2001. doi: 10.1093/jac/dkr256
Pietsch, M., Irrgang, A., Roschanski, N., Brenner Michael, G., Hamprecht, A., Rieber, H., et al. (2018). Whole genome analyses of CMY-2-producing Escherichia coli isolates from humans, animals and food in Germany. BMC Genomics 19:601. doi: 10.1186/s12864-018-4976-3
Pirs, M., Cerar Kisek, T., Krizan Hergouth, V., Seme, K., Mueller Premru, M., Jeverica, S., et al. (2019). Successful control of the first OXA-48 and/or NDM carbapenemase-producing Klebsiella pneumoniae outbreak in Slovenia 2014-2016. J. Hosp. Infect. 101, 142–149. doi: 10.1016/j.jhin.2018.10.022
Politi, L., Gartzonika, K., Spanakis, N., Zarkotou, O., Poulou, A., Skoura, L., et al. (2019). Emergence of NDM-1-producing Klebsiella pneumoniae in Greece: evidence of a widespread clonal outbreak. J. Antimicrob. Chemother. 74, 2197–2202. doi: 10.1093/jac/dkz176
Queenan, A. M., and Bush, K. (2007). Carbapenemases: the versatile beta-lactamases. Clin. Microbiol. Rev. 20, 440–458. doi: 10.1128/cmr.00001-07
Rodriguez-Bano, J., Gutierrez-Gutierrez, B., Machuca, I., and Pascual, A. (2018). Treatment of infections caused by extended-spectrum-beta- lactamase-, AmpC-, and carbapenemase-producing Enterobacteriaceae. Clin. Microbiol. Rev. 31:e79-17. doi: 10.1128/CMR.00079-17
Rozwandowicz, M., Brouwer, M. S. M., Fischer, J., Wagenaar, J. A., Gonzalez-Zorn, B., Guerra, B., et al. (2018). Plasmids carrying antimicrobial resistance genes in Enterobacteriaceae. J. Antimicrob. Chemother. 73, 1121–1137. doi: 10.1093/jac/dkx488
Ruppe, E., Woerther, P. L., and Barbier, F. (2015). Mechanisms of antimicrobial resistance in gram-negative Bacilli. Ann. Intensiv. Care 5:61. doi: 10.1186/s13613-015-0061-0
Salipante, S. J., Sengupta, D. J., Cummings, L. A., Land, T. A., Hoogestraat, D. R., and Cookson, B. T. (2015). Application of whole-genome sequencing for bacterial strain typing in molecular epidemiology. J. Clin. Microbiol. 53, 1072–1079. doi: 10.1128/JCM.03385-14
Schweizer, C., Bischoff, P., Bender, J., Kola, A., Gastmeier, P., Hummel, M., et al. (2019). Plasmid-mediated transmission of KPC-2 carbapenemase in Enterobacteriaceae in critically Ill patients. Front. Microbiol. 10:276. doi: 10.3389/fmicb.2019.00276
Tacconelli, E., and Magrini, N. (2016). Global Priority List of Antibiotic-Resistant Bacteria to Guide Research, Discovery, and Development of New Antibiotics. Geneva: World Health Organization.
Tenover, F. C., Arbeit, R. D., Goering, R. V., Mickelsen, P. A., Murray, B. E., Persing, D. H., et al. (1995). Interpreting chromosomal DNA restriction patterns produced by pulsed-field gel electrophoresis: criteria for bacterial strain typing. J. Clin. Microbiol. 33, 2233–2239.
Turton, J. F., Ward, M. E., Woodford, N., Kaufmann, M. E., Pike, R., Livermore, D. M., et al. (2006). The role of ISAba1 in expression of OXA carbapenemase genes in Acinetobacter baumannii. FEMS Microbiol. Lett. 258, 72–77. doi: 10.1111/j.1574-6968.2006.00195.x
Vasoo, S., Barreto, J. N., and Tosh, P. K. (2015). Emerging issues in gram-negative bacterial resistance: an update for the practicing clinician. Mayo Clin. Proc. 90, 395–403. doi: 10.1016/j.mayocp.2014.12.002
Villa, L., Feudi, C., Fortini, D., Brisse, S., Passet, V., Bonura, C., et al. (2017). Diversity, virulence, and antimicrobial resistance of the KPC-producing Klebsiella pneumoniae ST307 clone. Microb. Genom. 3:e000110. doi: 10.1099/mgen.0.000110
Wailan, A. M., Sidjabat, H. E., Yam, W. K., Alikhan, N. F., Petty, N. K., Sartor, A. L., et al. (2016). Mechanisms Involved in Acquisition of blaNDM genes by IncA/C2 and IncFIIY plasmids. Antimicrob. Agents Chemother. 60, 4082–4088. doi: 10.1128/AAC.00368-16
Wick, R. R., Judd, L. M., Gorrie, C. L., and Holt, K. E. (2017). Unicycler: resolving bacterial genome assemblies from short and long sequencing reads. PLoS Comput. Biol. 13:e1005595. doi: 10.1371/journal.pcbi.1005595
Wood, D. E., and Salzberg, S. L. (2014). Kraken: ultrafast metagenomic sequence classification using exact alignments. Genome Biol. 15:R46. doi: 10.1186/gb-2014-15-3-r46
Yan, J. J., Wang, M. C., Zheng, P. X., Tsai, L. H., and Wu, J. J. (2015). Associations of the major international high-risk resistant clones and virulent clones with specific ompK36 allele groups in Klebsiella pneumoniae in Taiwan. New Microbes. New Infect. 5, 1–4. doi: 10.1016/j.nmni.2015.01.002
Yong, D., Toleman, M. A., Giske, C. G., Cho, H. S., Sundman, K., Lee, K., et al. (2009). Characterization of a new metallo-beta-lactamase gene, bla(NDM-1), and a novel erythromycin esterase gene carried on a unique genetic structure in Klebsiella pneumoniae sequence type 14 from India. Antimicrob. Agents Chemother. 53, 5046–5054. doi: 10.1128/AAC.00774-09
Zankari, E., Hasman, H., Cosentino, S., Vestergaard, M., Rasmussen, S., Lund, O., et al. (2012). Identification of acquired antimicrobial resistance genes. J. Antimicrob. Chemother. 67, 2640–2644. doi: 10.1093/jac/dks261
Zhang, X., Feng, Y., Zhou, W., Mcnally, A., and Zong, Z. (2018). Cryptic transmission of ST405 Escherichia coli carrying bla NDM-4 in hospital. Sci. Rep. 8:390. doi: 10.1038/s41598-017-18910-w
Keywords: antibiotic resistance, carbapenemase, horizontal gene transfer, whole genome sequencing, NDM-1, KPC-2, IS26
Citation: Weber RE, Pietsch M, Frühauf A, Pfeifer Y, Martin M, Luft D, Gatermann S, Pfennigwerth N, Kaase M, Werner G and Fuchs S (2019) IS26-Mediated Transfer of blaNDM–1 as the Main Route of Resistance Transmission During a Polyclonal, Multispecies Outbreak in a German Hospital. Front. Microbiol. 10:2817. doi: 10.3389/fmicb.2019.02817
Received: 11 September 2019; Accepted: 20 November 2019;
Published: 17 December 2019.
Edited by:
Costas C. Papagiannitsis, University of Thessaly, GreeceReviewed by:
Iris Spiliopoulou, University of Patras, GreeceAlberto Antonelli, University of Florence, Italy
Copyright © 2019 Weber, Pietsch, Frühauf, Pfeifer, Martin, Luft, Gatermann, Pfennigwerth, Kaase, Werner and Fuchs. This is an open-access article distributed under the terms of the Creative Commons Attribution License (CC BY). The use, distribution or reproduction in other forums is permitted, provided the original author(s) and the copyright owner(s) are credited and that the original publication in this journal is cited, in accordance with accepted academic practice. No use, distribution or reproduction is permitted which does not comply with these terms.
*Correspondence: Stephan Fuchs, ZnVjaHNzQHJraS5kZQ==
†These authors have contributed equally to this work
‡Present address: Martin Kaase, Institute of Infection Control and Infectious Diseases, University of Göttingen, Göttingen, Germany