- 1Department of Soil Microbiology and Symbiotic Systems, Estación Experimental del Zaidín, Consejo Superior de Investigaciones Científicas, Granada, Spain
- 2Laboratory of Stable Isotopes Biogeochemistry, Instituto Andaluz de Ciencias de la Tierra, Consejo Superior de Investigaciones Científicas, Granada, Spain
- 3School of Biological Sciences, University of East Anglia, Norwich, United Kingdom
Legume-rhizobia symbiotic associations have beneficial effects on food security and nutrition, health and climate change. Hypoxia induced by flooding produces nitric oxide (NO) in nodules from soybean plants cultivated in nitrate-containing soils. As NO is a strong inhibitor of nitrogenase expression and activity, this negatively impacts symbiotic nitrogen fixation in soybean and limits crop production. In Bradyrhizobium diazoefficiens, denitrification is the main process involved in NO formation by soybean flooded nodules. In addition to denitrification, nitrate assimilation is another source of NO in free-living B. diazoefficiens cells and a single domain hemoglobin (Bjgb) has been shown to have a role in NO detoxification during nitrate-dependent growth. However, the involvement of Bjgb in protecting nitrogenase against NO in soybean nodules remains unclear. In this work, we have investigated the effect of inoculation of soybean plants with a bjgb mutant on biological nitrogen fixation. By analyzing the proportion of N in shoots derived from N2-fixation using the 15N isotope dilution technique, we found that plants inoculated with the bjgb mutant strain had higher tolerance to flooding than those inoculated with the parental strain. Similarly, reduction of nitrogenase activity and nifH expression by flooding was less pronounced in bjgb than in WT nodules. These beneficial effects are probably due to the reduction of NO accumulation in bjgb flooded nodules compared to the wild-type nodules. This decrease is caused by an induction of expression and activity of the denitrifying NO reductase enzyme in bjgb bacteroids. As bjgb deficiency promotes NO-tolerance, the negative effect of NO on nitrogenase is partially prevented and thus demonstrates that inoculation of soybean plants with the B. diazoefficiens bjgb mutant confers protection of symbiotic nitrogen fixation during flooding.
Introduction
Legumes have the unique ability to establish a dinitrogen (N2)-fixing symbiotic association with soil bacteria collectively termed rhizobia. Consequently, inoculation of legumes with rhizobia can substantially reduce the dependency on synthetic nitrogen fertilizers. This has several advantages, including mitigating greenhouse gas (GHG) emissions as well as protecting ground water from N-oxyanion contamination while improving soil fertility. In this context, a better understanding of the microorganisms associated with legume crops would contribute to improve food security and to reduce climate change. Following invasion of the root plant cells through a signaling exchange between bacteria and plant, rhizobia stop dividing and undergo differentiation into nitrogen-fixing bacteroids. In the bacteroid, nitrogenase is the key enzyme that reduces atmospheric N2 into biologically useful forms in a process called “Biological N Fixation” (reviewed by Sprent, 2009; Udvardi and Poole, 2013; Poole et al., 2018). Several studies have reported that nitrogenase is inhibited by nitric oxide (NO) (Kato et al., 2010; Sanchez et al., 2010). This highly reactive gas also contributes to the ozone layer depletion and has multiples roles in diverse physiological processes in living organisms. At low concentrations (nmolar levels) NO acts as signaling molecule, while at higher concentrations (μmolar levels) it is a pathological or toxic agent (Toledo and Augusto, 2012). In plants, NO is involved in many processes essential for growth and development. This signaling molecule is also implicated in the response of plants to many abiotic stresses (hypoxia, salinity, heavy metals, among others) (Corpas and Barroso, 2015; Domingos et al., 2015). During plant-pathogen interactions, NO also plays a key role in the hypersensitive response during plant defense (Thalineau et al., 2016). Interestingly, NO is also formed during the legume-rhizobia symbiotic interactions. In the root nodules, both the bacterial and plant partners are involved in NO production (reviewed by Hichri et al., 2015; Berger et al., 2018). From the plant perspective, NO is formed by the plant nitrate reductase (NR), nitrite (NO2–): NO reductase activity associated to the mitochondrial electron transport chain (ETC), and the NO synthase (NOS)-like activity (see Figure 3). In addition to plant sources, bacteroidal denitrification and particularly the periplasmic nitrate and nitrite reductases (Nap, and NirK, respectively) have been reported to be also involved in NO formation in root nodules (Sanchez et al., 2010; Horchani et al., 2011) (see Figure 3).
In the legume-rhizobia symbiosis, NO has an essential role during the establishment of the symbiotic interaction favoring nodule formation and development (Boscari et al., 2013). However, in mature nitrogen-fixing nodules, NO negatively affects nitrogenase expression and activity (Kato et al., 2010; Sanchez et al., 2010). This effect is more significant in response to hypoxia caused by flooding where an induction of NO formation occurs (Sanchez et al., 2010). Given the evidence for NO production in root nodules, the presence of NO-detoxification systems is crucial for maintaining a low steady-state intracellular NO concentration to support an efficient symbiosis. In this context, non-symbiotic and symbiotic plant hemoglobins have been reported to be involved in NO detoxification in nodules (Berger et al., 2018). From the bacterial perspective, several systems have been proposed to detoxify NO anaerobically such as the nitric oxide reductase (Nor) from denitrifiers, cytochrome c respiratory nitrite reductase (NrfA) from nitrate-ammonifying bacteria, or flavorubredoxin (NorVW), among others (reviewed by Stern and Zhu, 2014; Torres et al., 2016). Here, NO is detoxified by reduction to either ammonia or nitrous oxide (N2O). Bacterial hemoglobins (Hbs) are also a large family of important and well-characterized proteins for aerobic NO detoxification in bacteria. There are three main classes of Hbs: flavohemoglobins (fHb), single domain hemoglobins (sdHb), and truncated hemoglobins (tHb) (for a review see Poole, 2005; Stern and Zhu, 2014; Gell, 2018). FHbs, such as Hmp from Escherichia coli, consist of three domains: an N-terminal domain with a heme b-type cofactor, a central FAD binding domain and a C-terminal NADP-binding domain (Poole, 2005). In the presence of oxygen, reduced heme from Hmp catalyzes the reaction between NO and O2 producing nitrate by either NO denitrosylase mechanism or O2 dioxygenase process (Gardner et al., 1998; Hausladen et al., 2001; Gardner, 2005). Under anaerobiosis, Hmp has reductase activity and it is capable to reduce NO to N2O (Kim et al., 1999). The sdHbs, such as Cgb from Campylobacter jejuni, also have a role in NO detoxification. They contain the hemoglobin domain, but they do not possess the oxidoreductase domain and the FAD-containing domain (for a review see Tinajero-Trejo et al., 2013). TrHbs, such as HbN from Mycobacterium tuberculosis, lacks the oxidoreductase domain and the FAD-containing domain and its hemoglobin domain is 20–40 residues shorter than classical sdHbs (Poole, 2005). In the legume root nodules, the denitrifying Nor rhizobial enzyme has been shown to be involved in NO removal (Sanchez et al., 2010; Blanquet et al., 2015). In addition to Nor, a combined role for Ensifer meliloti fHb (Hmp), and NnrS1 and NnrS2 proteins in NO degradation has been reported in Medicago truncatula nodules (Cam et al., 2012; Meilhoc et al., 2013; Blanquet et al., 2015).
Bradyrhizobium diazoefficiens is a Gram-negative soil bacterium that fixes N2 during symbiotic interaction with soybean plants (Glycine max). This bacterium is also able to denitrify under free-living conditions or inside the root nodules (reviewed by Bedmar et al., 2005, 2013; Torres et al., 2016). In B. diazoefficiens, denitrification reactions are catalyzed by four enzymes that reduce nitrate (Nap), nitrite (NirK), nitric oxide (Nor), and nitrous oxide (Nos), respectively. These enzymes are encoded by napEDABC (Delgado et al., 2003), nirK (Velasco et al., 2001), norCBQD (Mesa et al., 2002), and nosRZDYFLX (Velasco et al., 2004) genes. The denitrification process by B. diazoefficiens bacteroids is proposed to be the main driver for NO formation in soybean nodules in response to flooding conditions (Sanchez et al., 2010). In addition to denitrification, a coordinated nitrate assimilation and NO detoxification system, encoded by the narK-bjgb-flp-nasC operon, is also involved in NO homeostasis within free-living cells. This cluster codes for a putative single domain hemoglobin (Bjgb), the assimilatory nitrate reductase (NasC), a nitrate/nitrite transporter (NarK) and a FAD-dependent NAD(P)H oxidoreductase (Flp). Bjgb mitigates the NO produced by NasC as by-product of nitrate/nitrite assimilation (Cabrera et al., 2016). Thus, a role for Bjgb in protecting B. diazoefficiens free-living cells from nitrosative stress has been proposed (Cabrera et al., 2016). However, the function of B. diazoefficiens Bjgb in soybean nodules remains unclear. The aim of this research is to analyze the role of Bjgb from B. diazoefficiens in the response of symbiotic nitrogen fixation to flooding as well as in the NO homeostasis in soybean nodules.
Materials and Methods
Bacterial Strains and Growth Conditions
Bradyrhizobium diazoefficiens USDA 110 (WT) (United States Department of Agriculture, Beltsville, MD, United States), and a bjgb deletion mutant (named 4001 strain), which was previously constructed by Cabrera et al. (2016), were used in this study. B. diazoefficiens strains were grown under aerobic conditions at 30°C in peptone-salts-yeast extract (PSY) medium added with 0.1% (w/v) L-arabinose (Regensburger and Hennecke, 1983). For inocula preparation, cells were collected by centrifugation at 8000 g for 10 min, washed twice and cultured aerobically for 48 h at 30°C in Bergersen minimal medium (Bergersen, 1977) where glycerol was substituted by 10 mM succinate as carbon source and L-glutamate was replaced by 10 mM KNO3 as sole N-source. Chloramphenicol was added to the cultures at 20 μg ml–1.
Plant Growth Conditions
Soybean (G. max L. Merr., cv. Williams) seeds were surface-sterilized with ethanol for 5 min, immersed in H2O2 (30%, v/v) for 15 min, and finally washed with sterile distilled water. Then, seeds were germinated in 1% agar petri-dishes (8–9 seeds each) and incubated in darkness at 30°C for 72 h. Seedlings were sowed in autoclaved Leonard jars which contained vermiculite (Trung and Yoshida, 1983). Two soybean plants per jar were inoculated at sowing with 1 ml of a single bacterial strain (approx. 108 cells ml–1) and overlaid with autoclaved perlite. Plants were transferred to a plant growth chamber for 35 days (16–8 h day/night cycle, day/night temperatures of 26–22°C and photosynthesis photon flux density of 128–148 μmol photons m–2 s–1). Plants were cultivated using a mineral solution (Rigaud and Puppo, 1975) with or without 4 mM KNO3. Treatment of plants with 4 mM KNO3 does not inhibit nodule formation or nitrogenase activity as previously reported by Mesa et al. (2004). After growth for 28 days, a set of plants were kept under flooding for 7 days by immersing them to 1 cm above substrate level applying mineral solution as described previously (Sanchez et al., 2010). For determination of the plant N content acquired from biological N2 fixation, plants were watered with 4 mM 15N-labeled KNO3 (Potassium nitrate-15N; 5 atom% 15N; Cat. #CS01-185_272; Campro Scientific GmbH). Nodules were collected from 35-day-old plants. Plant physiological parameters were determined per plant: nodule number per plant (NNP), nodule dry weight per plant (NDWP), and plant dry weight (PDW).
Total Nitrogen and Nitrogen Derived From Biological N2 Fixation
These analyses were carried out as previously described (Sanchez et al., 2011b). Plants were oven-dried and then weighed and grounded in an IKA A 11 basic analytical mill (Rose Scientific Ltd., Alberta, Canada). For total nitrogen (TN) and 15N enrichment (δ15N), subsamples of 3 mg were analyzed with an elemental analyzer (EA1500 NC, Carlo Erba, Milan, Italy) combined to isotope-ratio mass spectrometer (Delta Plus XL, ThermoQuest, Bremen, Germany). The general precision of analyses for δ15N was ± 0.1‰. The stable composition was shown as δ15N values per mil: δ15N (‰) = (Rsample/Rstandard − 1) × 1000, where R = 15N/14N. Commercial N2 was the internal standard for the nitrogen isotopic analyses. δ15N data for all samples were standardized against internationally accepted reference materials (IAEA N1, δ15N = +0.4‰, IAEA N2, δ15N = +20.3‰ and USGS32 δ15N = +174.5 vs. AIR). The proportion of N derived from the atmosphere (%Ndfa) was calculated by following the formula: %Nfda = 100 × [1 − (A/B)], where A = Atom% 15N excess in inoculated plants, B = Atom% 15N excess in uninoculated plants. Atom% 15N excess = atom% 15N in labeled treatment – atom% 15N in non-labeled treatment. Atom% 15N was calculated as: δ15N (‰) × 100. To calculate the atom% 15N excess, a set of plants was grown only under N2-fixing conditions to obtain the atom% 15N of the non-labeled treatment. The fixed-nitrogen content (FN) was calculated as: FN = (%Ndfa × TN)/100.
Acetylene Reduction Activity
Acetylene reduction activity (ARA) was analyzed with fresh detached nodules from plants provided with mineral solution containing 4 mM KNO3. About 20 nodules per replica were placed in 20-ml headspace vials (SUPELCO®) containing 100 μl mineral solution added with 4 mM KNO3. Tubes were sealed, filled with 1 ml of pure acetylene and incubated at 30°C. After incubation for 2 and 4 h, gas samples (0.5 ml) were extracted from the tubes for ethylene analysis. A Hewlett-Packard model 5890 gas chromatograph (Agilent Technologies, S.L., Madrid) with a flame ionization detector and a molecular sieve 5A (60–80 mesh) column (180 cm × 0.32 cm) (Agilent Technologies, S.L.) was used. N2 at 60 ml min–1 was used as carrier gas. Temperatures of oven, injector, and detector were 60, 90, and 110°C, respectively. Ethylene concentration in each sample was calculated from standards of 2% (v/v) ethylene. Acetylene reduction rate was calculated by measuring the increase of ethylene (C2H4) production inside the vials headspace determined in the lineal range (0, 2, and 4 h of incubation) (Supplementary Figure S1) and calculated as: Δ C2H4 nmol (4–2 h)/Δ time (4–2 h). Results presented in Figure 1A were expressed as: [nmol C2H4 min–1 (g NFW) –1] where NFW = nodule fresh weight.
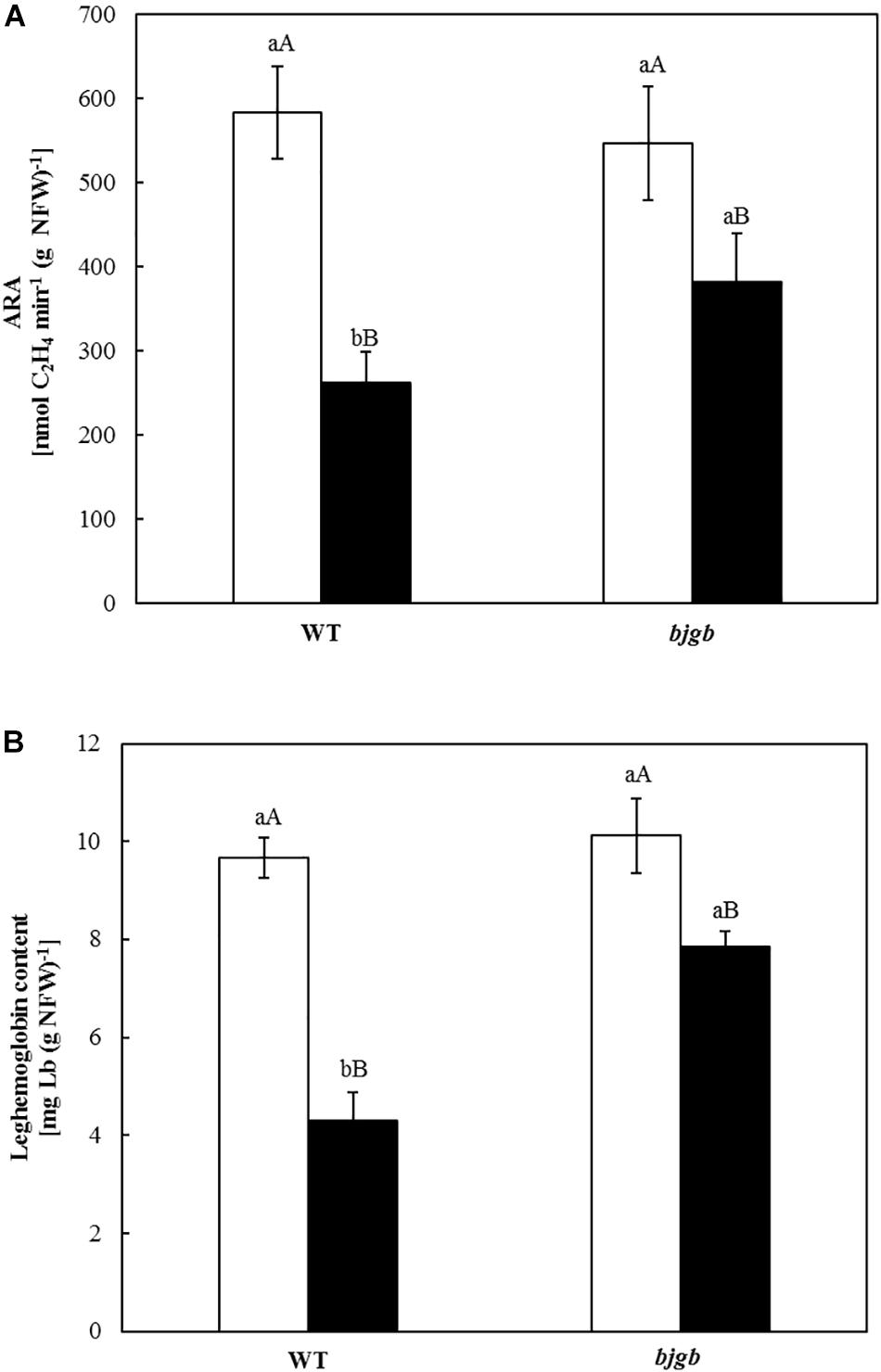
Figure 1. Acetylene reduction activity (ARA) (A) and Leghemoglobin (Lb) content of soybean nodules (B). Plants were inoculated with B. diazoefficiens USDA 110 (WT) or 4001 (bjgb mutant) strains. Nodules were isolated from non-flooded plants (white bars) or plants subjected to flooding conditions for 7 days (black bars). Data are means with standard deviations from two independent experiments assayed by using six replicates. In individual graphs, bars within the same treatment marked with the same lower-case letter, and bars within the same strain followed by the same capital letter, are not significantly different as determined by the ANOVA test at P ≤ 0.05.
Leghemoglobin Content
Leghemoglobin (Lb) was quantified in nodules from plants provided with mineral solution containing 4 mM KNO3 following the protocol described by Sanchez et al. (2010). Fluorimetric detection of Lb was analyzed in a Shimadzu (Shimadzu Scientific Instruments, Kyoto, Japan) spectrophotofluorometer with a mercury-xenon lamp and a RF-549 red-sensitive photomultiplier. The excitation wavelength was 405 nm and the emission monochromator adjustment was 650 nm. Heme protein concentration was proportional to the difference in fluorescence between heated and unheated samples and therefore it was indicative of the leghemoglobin concentration in the samples. Lb content in each sample was calculated using bovine hemoglobin as standard. Lb content was expressed as: [mg Lb (g NFW) –1].
Transcript Levels Determination
Nodules were detached from roots, frozen in liquid nitrogen and stored at −80°C until further use. For RNA isolation, nodules were distributed in 2-ml Eppendorf tubes and were disrupted and homogenized by using a TissueLyser (Mixer Mill MM 301; Retsch GmbH, Haan, Germany) (three times for 50 s at 30 Hertz) with a tungsten carbide bead (3 mm; Qiagen). Then, RNA was isolated by following the hot phenol-extraction protocol described elsewhere (Babst et al., 1996). RNA integrity was confirmed by agarose gel electrophoresis. Precipitated RNA was treated with DNaseI amplification grade (Invitrogen). Next, RNA samples were cleaned up with RNeasy Mini spin columns (Qiagen). Genomic DNA contamination was checked by PCR using fixN4_For and fixN4_Rev primers (Supplementary Table S1). Then, 2 μg of total RNA was reverse transcribed to cDNA employing random hexamers and SuperScript II reverse transcriptase (Invitrogen) following the supplier’s instructions. For quantitative reverse transcriptase-polymerase chain reaction (qRT-PCR) analyses, an iQTM5 Optical System (Bio-Rad, Foster City, CA, United States) was used. Each PCR reaction (with a total volume of 19 μl) was performed using 9.5 μl iQTM SYBR Green Supermix (Bio-Rad), 2 μM (final concentration) of individual primers (Supplementary Table S1) and proper dilutions of different cDNA samples. The PCR program included an initial denaturation and Taq activation step of 5 min at 95°C, followed by 40 cycles of 15 s at 95°C, 45 s at 60°C, and 45 s at 72°C. PCR reactions were performed in triplicate. The formation of specific PCR products was checked by melting curve analyses. Expression of the gene encoding the 16S rRNA was used as reference for normalization (primers 16S_qRT_For and 16S_qRT _Rev; Supplementary Table S1). Relative changes were calculated by using the Pfaffl method (Pfaffl, 2001). Expression of narK in WT flooded nodules was determined relative to that observed in WT non-flooded nodules. For nifH and norC expression, values were calculated relative to the levels of nifH or norC expression in WT non-flooded nodules. The expression levels given as fold-change for both the WT and bjgb mutant were compared with those from WT under non-flooding conditions.
Detection of NO in Nodules
Nitric oxide was detected in fresh detached nodules (about five nodules) from plants provided with mineral solution added with 4 mM KNO3. For NO scavenger treatment, nodules were incubated for 1 h in the dark at 30°C in a solution containing 2-[4-carboxyphenyl]-4,4,5,5-tetramethylimidazoline-1-oxyl-3-oxide (c-PTIO) (Sigma-Aldrich) at a final concentration of 3 mM. Then, NO was detected by dipping the nodules in 7 μM of 4,5-diaminofluorescein diacetate (DAF-2 DA) solution (Genaxxon bioscience) for 2 h in the dark at 30°C, while control nodules were incubated in distilled water under the same conditions. The relative fluorescence units of the DAF-2 DA solution were determined using a fluorometer (MD-5020, Photon Technology International, Birmingham, NJ, United States) with 495 nm excitation and 515 nm emission wavelength (2 nm band width). Nodular NO accumulation was expressed as relative fluorescence units per nodule dry weight (NDW): [RFU (mg NDW)–1].
Nitrous Oxide Production by Nodules
N2O was measured in fresh detached nodules from plants provided with mineral solution supplemented with 4 mM KNO3. Essentially, detached nodules from roots were placed in 20-ml headspace vials (SUPELCO®) (about 20 nodules) provided with 100 μl (for nodules incubated under non-flooding conditions) or 5 ml (to maintain nodules under flooding treatment) of mineral solution with 4 mM KNO3. Acetylene 10% (v/v) was injected into each tube in order to inhibit nitrous oxide reductase (Yoshinari and Knowles, 1976). Samples were incubated for 6 h at 30°C before measuring N2O production. Then, gaseous aliquots (1 ml) were taken from the headspace using a gas-tight syringe and they were manually injected into an HP 4890D gas chromatograph with an electron capture detector and a Porapak Q 80/100 MESH (8 ft) packed column. N2 at 28 ml min–1 was the carrier gas. The injector, column and detector temperatures were 125, 60, and 375°C, respectively. Concentrations of N2O in the samples were determined employing 2% (v/v) N2O as standard. Total N2O concentration was calculated considering N2O in headspace and dissolved N2O using Bunsen water solubility coefficient (54.4% at 25°C). For each replicate, N2O flux was recorded after 4 and 6 h of incubation, which was the lineal range of N2O emission. The N2O emission flux was calculated as: Δ N2O molar concentration (6–4 h)/Δ time increase (6–4 h). Results were expressed as: [nmol N2O h–1 (g NDW) –1].
Statistical Analyses
The total number of replicates for each assay is shown in each figure and table. For each parameter, descriptive statistical analyses (media and standard deviation) were calculated and data were checked for normal distribution and homoscedasticity. The analyses of variance (ANOVA) within treatments were performed using the post hoc Tukey–Kramer test (P ≤ 0.05). For inferential statistical analyses, GNU-PSPP open-source software v0.9.01 was used.
Results
Loss of B. diazoefficiens Bjgb Confers Tolerance of Soybean Symbiotic Nitrogen Fixation to Flooding
After 35 days of growth, NNP, NDWP, and PDW were analyzed (Table 1). As previously reported (Mesa et al., 2004), the presence of nitrate in the nutrient solution did not inhibit NNP or NDWP in plants inoculated with either the WT or the bjgb mutant (Table 1). Flooding treatment did not significantly alter NNP in plants grown either in the presence or in the absence of nitrate, independently of the strain used as inoculum (Table 1). On the contrary, flooding provoked a significant inhibition of NDWP of plants cultivated with or without nitrate. Interestingly, those plants inoculated with the bjgb mutant and grown with nitrate showed higher NDWP in response to flooding (about 30%) than plants that were inoculated with the WT (Table 1). As expected, the presence of nitrate increased PDW compared to non-nitrate treated plants. As shown in Table 1, in the absence of nitrate, flooding did not significantly affect PDW in plants inoculated with any of the strains. However, in nitrate-treated plants, flooding provoked a negative effect on PDW that was only significant (about 28%) in those plants inoculated with the WT strain but not in those inoculated with the bjgb mutant (Table 1). As control of symbiotic nitrogen fixation, a set of uninoculated plants were included in the analyses. After 35 days growth, nodulation did not take place in uninoculated plants and very low levels of PDW were observed in those plants grown without nitrate (Table 1). In plants that were only nitrate dependent, flooding decreased PDW about 36% (Table 1).
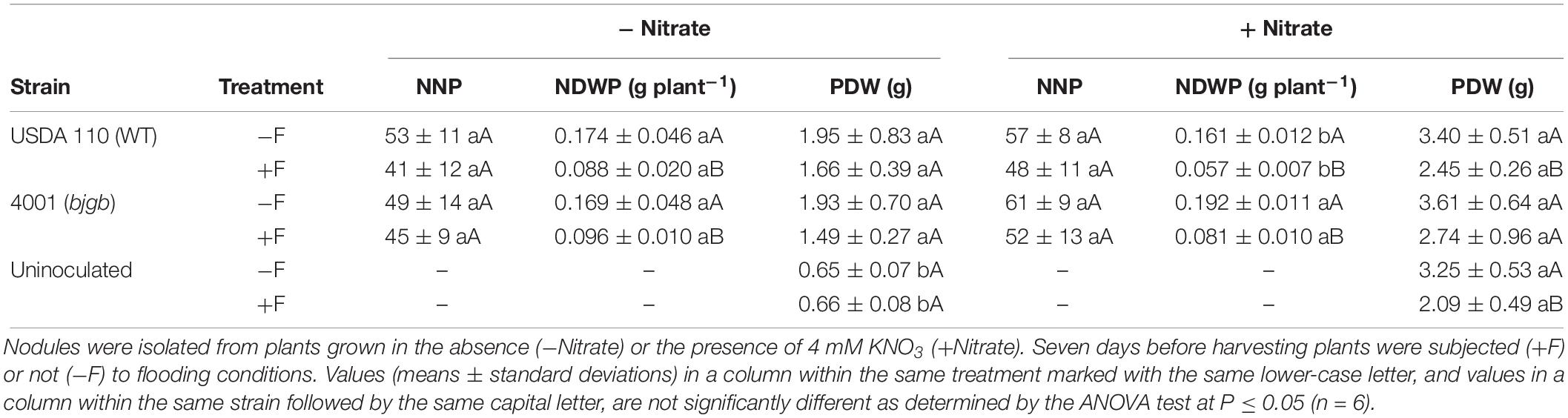
Table 1. Nodule number per plant (NNP), nodule dry weight per plant (NDWP), and plant dry weight (PDW) of plants inoculated with B. diazoefficiens USDA 110 (WT) or 4001 (bjgb mutant) strains.
As is indicated in Table 2, flooding stress provoked a significant decrease in the TN content of shoots from inoculated plants grown with 4 mM KNO3 (Table 2). Nevertheless, plants inoculated with bjgb showed an increase of about 12% in TN compared to plants where the WT was used as inoculum (Table 2). In uninoculated plants cultured with nitrate, flooding did not affect TN (Table 2). In order to differentiate between N acquired from N2-fixation or nitrate assimilation, we used the 15N isotope dilution technique that allowed us to determine the proportion of N derived from the atmosphere (Ndfa%) in plants that were cultivated with 4 mM 15N-labeled KNO3. Here, flooding decreased %Ndfa of plants, being this reduction significantly higher in plants inoculated with the WT compared to those inoculated with the bjgb mutant (55% vs. 30%). Consequently, the content of fixed nitrogen (FN) of flooded plants inoculated with bjgb was about 47% higher than that of flooded plants inoculated with the WT (Table 2). These results clearly indicate that inoculation of the plants with the bjgb mutant confers tolerance of symbiotic nitrogen fixation to flooding. By using the 15N isotope dilution technique, we also calculated the % of 15N atom in excess (atom% 15N excess) of the shoots. As shown in Table 2, 15N excess in uninoculated plants was significantly higher compared to inoculated plants and no effect of flooding in 15N excess of uninoculated plants was perceived. However, in plants inoculated either with the WT or bjgb mutant, flooding increased the % of 15N excess. These results indicate that soybean nitrogen fixation is more sensitive to flooding than nitrate assimilation supporting previous findings (Bacanamwo and Purcell, 1999; Sanchez et al., 2011b).
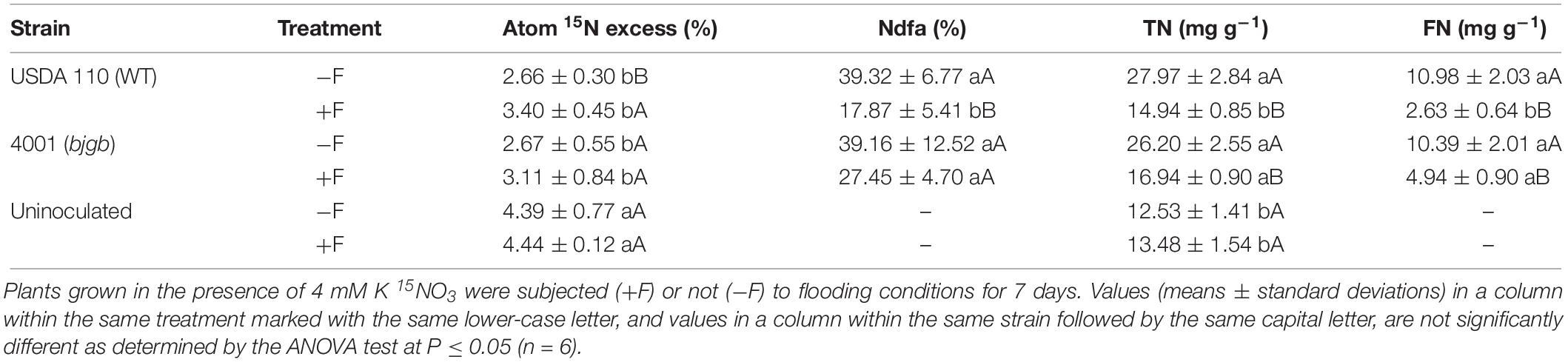
Table 2. Atom 15N excess, proportion of nitrogen derived from the atmosphere (%Ndfa), total nitrogen content (TN) and fixed-nitrogen content (FN) of shoot tissue of uninoculated plants or plants inoculated with B. diazoefficiens USDA 110 (WT) or 4001 (bjgb mutant) strains.
In order to confirm the flooding effect on N2 fixation, nitrogenase activity was measured by determining ARA in nodules incubated for 2 and 4 h (Supplementary Figure S1). Values for C2H4 produced in nmol per min and g NFW (Figure 1A) showed a decrease in ARA (about 55%) for WT nodules isolated from plants submitted to flooding compared to non-flooded nodules. However, nitrogenase activity only decreased about 30% in bjgb nodules from flooded plants compared to non-flooded bjgb nodules (Figure 1A). Functionality of the nodules was also estimated by measuring the Lb content (Figure 1B). Consistent with ARA determinations, flooding provoked a smaller decrease of Lb content in nodules produced by the bjgb mutant (about 22%) compared to that observed in WT nodules (about 55%).
Next, we tested the effect of deleting bjgb on the expression of the nifH gene which is responsible for the synthesis of the Fe-protein from nitrogenase complex (Table 3). Transcript levels for nifH were examined in WT or bjgb nodules by qRT-PCR. As observed in Table 3, nifH expression was not significantly affected in the nodules induced by the bjgb mutant (−1.31 fold-change) compared to WT nodules, both collected from non-flooded plants. Flooding decreased nifH expression by ∼16-fold in WT nodules compared to that observed in WT non-flooded nodules. However, nifH mRNA levels decreased by ∼10-fold in bjgb flooded nodules compared to those observed in WT non-flooded nodules.
In order to ascribe the effect of bjgb inoculation to the presence of Bjgb in the nodules, we checked the expression of the bjgb gene by analyzing transcript levels of narK, the lead gene of the narK-bjgb-flp-nasC transcriptional unit (Cabrera et al., 2016) (Table 3). As shown in Table 3, narK expression increased ∼11-fold in flooded nodules compared to non-flooded nodules collected from plants inoculated with the WT strain.
Loss of B. diazoefficiens Bjgb Reduces NO Levels in Soybean Nodules in Response to Flooding
The contribution of B. diazoefficiens Bjgb in NO homeostasis in nodules was investigated by analyzing the capacity to accumulate NO as well as to produce N2O, the product of the nitric oxide reductase (Nor). To perform these experiments, we used nodules from plants grown under the conditions that induced NO and N2O accumulation, as previously reported (Sanchez et al., 2010; Tortosa et al., 2015). Thus, nodules were collected from soybean plants inoculated with B. diazoefficiens USDA 110 (WT) or 4001 (bjgb mutant) strains, cultivated with 4 mM nitrate and submitted or not to flooding conditions for 7 days before harvesting. Free NO was detected by using the DAF-2DA specific fluorescent probe. As shown in Figure 2A, very low levels of NO were observed in WT and bjgb nodules of non-flooded plants. However, flooding significantly induced NO formation in WT nodules confirming previous results (Sanchez et al., 2010). This induction was also observed in nodules from plants inoculated with the bjgb mutant that accumulated approximately 2-fold less NO than WT nodules in response to flooding conditions (Figure 2A). In order to prove that the increase of fluorescence perceived in flooded nodules was caused by NO, WT, and bjgb flooded nodules were incubated with a NO scavenger (c-PTIO). After incubation of the nodules with c-PTIO, nodular NO accumulation was significantly reduced (Figure 2A), indicating that the fluorescence signal was mostly due to NO production.
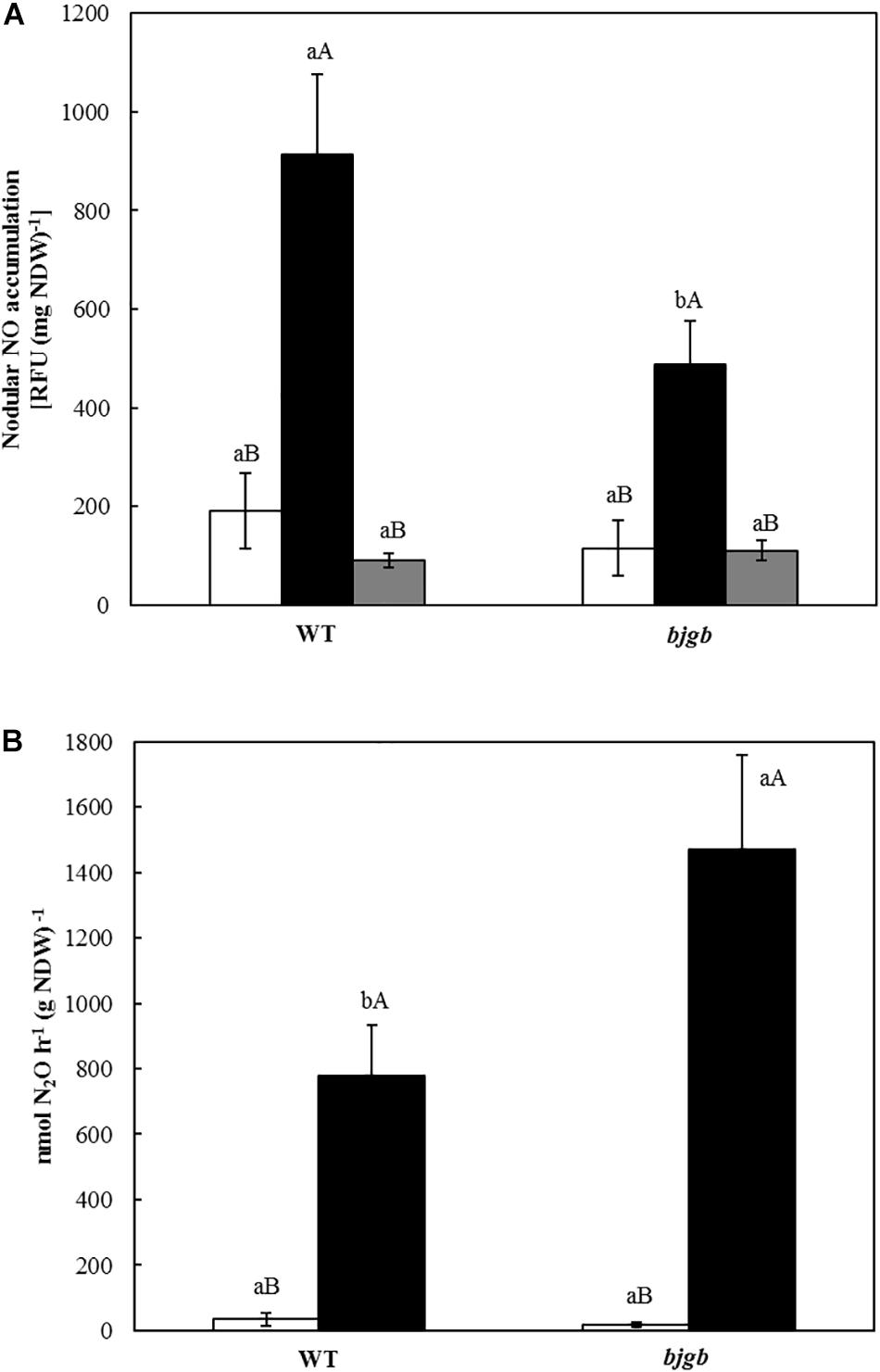
Figure 2. Nitric oxide (NO) detection (A) and N2O production (B) by soybean nodules. Plants were inoculated with B. diazoefficiens USDA 110 (WT) or 4001 (bjgb mutant) strains. Nodules were isolated from non-flooded plants (white bars) or plants subjected to flooding conditions for 7 days (black bars). In A, flooded nodules were incubated with 3 mM c-PTIO (gray bars). Data are means with standard deviations from two independent experiments assayed by using six replicates. In individual graphs, bars within the same treatment marked with the same lower-case letter, and bars within the same strain followed by the same capital letter, are not significantly different as determined by the ANOVA test at P ≤ 0.05.
We also analyzed Nor activity by measuring N2O production by soybean nodules using gas chromatography. While non-flooded nodules produced by WT or bjgb strains showed basal levels of N2O, flooding conditions induced N2O production in B. diazoefficiens WT nodules, consistent with previous observations (Tortosa et al., 2015). Interestingly, nodules produced by the bjgb mutant accumulated about twofold more N2O than WT nodules in response to flooding (Figure 2B). The lower NO levels as well as higher N2O production capacity observed in the nodules from the bjgb mutant compared to WT levels (Figures 2A,B), suggest that Nor activity that reduces NO to N2O is induced in bjgb bacteroids. In order to establish if the differences of Nor activity observed between WT and bjgb bacteroids could be explained by changes in nor gene expression, norC transcripts were measured by performing qRT-PCR analyses. RNA was isolated from nodules harvested from soybean plants inoculated with B. diazoefficiens WT or bjgb mutant strains. In nodules from plants that were not subjected to flooding, bjgb mutation did not significantly affect norC expression compared to wild-type (+1.12 fold-change). Flooding provoked a notable increase of norC expression in either WT or bjgb flooded-nodules compared to WT non-flooded nodules (+67.53 and +89.16 fold-change, respectively). Interestingly, the induction of norC expression by flooding was about 33% higher in bjgb nodules than in WT nodules (Table 3).
Discussion
Several studies have reported that NO production in soybean nodules is induced by nitrate and hypoxia that is promoted by flooding conditions (Meakin et al., 2007; Sanchez et al., 2010). This molecule is a potent inhibitor of nitrogenase activity and expression (Kato et al., 2010; Sanchez et al., 2010). In nodules, NO can also bind Lb contributing to the formation of nitrosyl-leghemoglobin (LbNO) complexes that have a major role in detoxifying reactive nitrogen species (Sanchez et al., 2010). It is also well established that the denitrification pathway in B. diazoefficiens is the major source of NO and N2O in soybean nodules (Sanchez et al., 2010; Tortosa et al., 2015). Previous experiments revealed that in soybean plants inoculated with a B. diazoefficiens napA null strain, where denitrification is inhibited, basal levels of NO and N2O were still detected in nodules (Sanchez et al., 2010; Tortosa et al., 2015). These observations suggested that, in addition to denitrification, other mechanisms give rise to NO and N2O in nodules. The recently identified hemoglobin Bjgb is one such system which has been reported to be involved in NO detoxification under free living conditions (Cabrera et al., 2011, 2016; Sanchez et al., 2011a), however, its function inside the nodules is unknown. In this work, the role of Bjgb in symbiotic nitrogen fixation and NO homeostasis in the B. diazoefficiens–G. max symbiosis has been investigated by inoculating plants with a bjgb mutant. In order to induce NO formation in the nodules, soybean plants were grown in the presence of KNO3 (4 mM) and 7 days before harvesting they were subjected to flooding, as previously reported (Mesa et al., 2004; Meakin et al., 2007; Sanchez et al., 2010). This nitrate concentration was selected based in a previous work (Mesa et al., 2004) where G. max L. Merr., cv. Williams inoculated with B. diazoefficiens USDA 110 was grown in the presence of 0, 1, 2, 3, 4, 5, and 6 mM KNO3. Mesa et al. (2004) found that 4 mM KNO3 resulted in the induction of bacteroidal denitrification by measuring N2O formation in nodules, and this concentration did not inhibit either nodule formation or nitrogenase activity. Since the effect of flooding on NNP was similar independently of the strain used for inoculation, the increase in NDWP observed in plants where bjgb was used as inoculum is probably due to a higher individual nodule weight. This observation suggests that bjgb mutant has a beneficial effect on nodule development and growth rather than on nodule number formation.
The impact of flooding on nitrogen fixation was further investigated by calculating the amount of FN in shoots employing the 15N isotope dilution method. Consistent with previous observations (Sanchez et al., 2011b), this approach was determinant to demonstrate the negative effect of flooding on nitrogen fixation in plants grown in the presence of nitrate. The decrease of FN provoked by flooding was significantly less pronounced in plants that were inoculated with the bjgb mutant, indicating that the loss of Bjgb confers tolerance of N2 fixation to flooding. These findings were confirmed by analysis of nitrogenase activity (ARA) and levels of leghemoglobin (estimation of nodule functionality). As reported previously, flooding significantly inhibited ARA and Lb levels of WT nodules (Sanchez et al., 2010). However, the negative effect of flooding on ARA and Lb levels was smaller in nodules from plants inoculated with the bjgb mutant compared to WT nodules.
In contrast to our findings, inoculation of M. truncatula plants with an E. meliloti strain lacking the flavohemoglobin (Hmp) strongly inhibited ARA and provoked nodule senescence (Cam et al., 2012), as compared to those inoculated with the WT. The authors attributed this effect to an increase in the NO levels observed in the nodules produced by the hmp mutant which negatively affected nitrogen fixation and increased root nodule senescence. Contrary to the higher NO levels observed in M. truncatula nodules induced by the E. meliloti hmp mutant compared to those produced by the WT (Cam et al., 2012), our results showed that NO formation was significantly lower in bjgb than in WT nodules in response to flooding. The apparent differences observed regarding the involvement of the E. meliloti hmp and B. diazoefficiens bgjb mutants in N2-fixation and nodule NO formation could be due to the different plant growth conditions used by Cam et al. (2012) and in this work. While Cam et al. cultured M. truncatula in nitrogen−free medium, in the present work soybean plants were grown with nitrate and subjected to flooding for 7 days. In B. diazoefficiens, denitrification accounts for about 90% of NO present in flooded soybean nodules (Sanchez et al., 2010), while in M. truncatula nodules, E. meliloti produces only ∼35% of NO detected (Cam et al., 2012). The contradictory observations found between the roles of E. meliloti Hmp and B. diazoefficiens Bjgb might be also due to metabolic differences in their nitrate-reducing pathways. While B. diazoefficiens is a complete denitrifier which grows anoxically by nitrate respiration, E. meliloti is unable to respire nitrate under anoxic conditions (reviewed by Torres et al., 2016). Furthermore, it might also be possible that the nature of nodule-type supports different mechanisms of dealing with the nitrosative stress, considering that indeterminate nodules (M. truncatula) are characterized by a persistent meristem and a continuous growth, while determinate nodules (G. max) are characterized by a not persistent meristem and a limited growth potential.
The decreased levels of NO produced by bjgb nodules could explain the tolerance of nitrogen fixation to flooding observed in plants inoculated with the bjgb mutant. In B. diazoefficiens there are two main processes involved in NO formation, which are denitrification and nitrate assimilation. Under low oxygen nitrate-dependent free-living conditions, pathways for both respiratory denitrification and nitrate assimilation are active to promote bacterial survival. In fact, both respiratory (Nap) and assimilatory (NasC) nitrate reductases contribute similarly to the total activity. Under these conditions, a bjgb mutant showed substantial growth inhibition compared to WT cells suggesting a NO detoxifying role for Bjgb (Cabrera et al., 2016). However, under symbiotic conditions, where growth is not needed, the contribution of assimilatory nitrate reduction in the bacteroids is only ∼10% (Sanchez et al., 2010). Consequently, it may be possible that low NO concentrations arising from nitrate assimilation in the bacteroids cytoplasm does not present toxicity in nodules. These observations may also explain that, in contrast to the reported role in NO detoxification during nitrate-dependent anaerobic growth, Bjgb would not be directly involved in NO detoxification inside nodules and thus may instead act as an NO-buffer.
The assimilatory nitrate reductase (NasC) is encoded by the narK-bjgb-flp-nasC operon that also contains the gene encoding the sdHb (Bjgb). Cabrera et al. (2016) reported that NO produced by NasC in the cytoplasm acts as signal molecule which activates expression of the denitrifying nor genes. In this context, it has been recently demonstrated that NO is the signaling molecule that induces nor genes in B. diazoefficiens (Bueno et al., 2017). Under free-living conditions, expression of the respiratory Nor was significantly up-regulated in a bjgb mutant relative to WT, probably due to increased intracellular NO levels that arise during assimilatory nitrate reduction (Cabrera et al., 2016). In soybean nodules, NO produced by the periplasmic denitrifying enzyme NirK is the main source of NO, and Nor the principal NO removal protein (Sanchez et al., 2010). It might be possible that, as it has been demonstrated in free-living cells (Cabrera et al., 2016), NO produced in the cytoplasm from nitrate assimilation is increased in bacteroids induced by the bjgb mutant. Then, this molecule would act in the cytoplasm as a signal that induces nor genes expression. In fact, induction of norC expression by flooding was greater in the bjgb nodules than in WT nodules. Furthermore, analysis of Nor activity showed a higher N2O formation capacity in bjgb nodules compared to those produced by the parental strain.
It has been previously reported that soybean plants inoculated with a B. diazoefficiens nirK mutant, whose nodules do not produce NO from denitrification, were more tolerant to flooding than plants inoculated with the WT strain (Sanchez et al., 2011b). Similarly, results obtained in this work suggest that inoculation with the bjgb mutant partially diminished the negative effect of flooding on N2 fixation observed in WT-inoculated plants. This advantage is probably due to the increased capacity of bjgb deficient nodules to induce expression of nor genes, whereby the gene product removes the NO produced by denitrification. The decreased levels of NO observed in bjgb nodules compared to WT nodules in response to flooding lead us to suggest that B. diazoefficiens Bjgb, instead of functioning as a direct NO-detoxifying protein in the nodules, it would contribute indirectly by modulating cytoplasmic NO levels, the signaling molecule required for induction of the denitrifying nitric oxide reductase enzyme, which is the major protein involved in NO removal in soybean nodules (see Figure 3). In conclusion, this work reveals a strategy for nitrogenase protection and consequently for efficient symbiotic nitrogen fixation that requires the modulation of NO levels in root nodules by the microsymbiont. Therefore, using rhizobia strains that modulate NO levels in nodules is an important practice that would enhance legume production and promote sustainable agriculture. From this perspective, the contribution of bjgb mutation is positive for nitrogenase protection. However, elevated N2O production resulting from increased NO reduction results in release of a potent and stable GHG that has a negative environmental impact and may contribute to climate change.
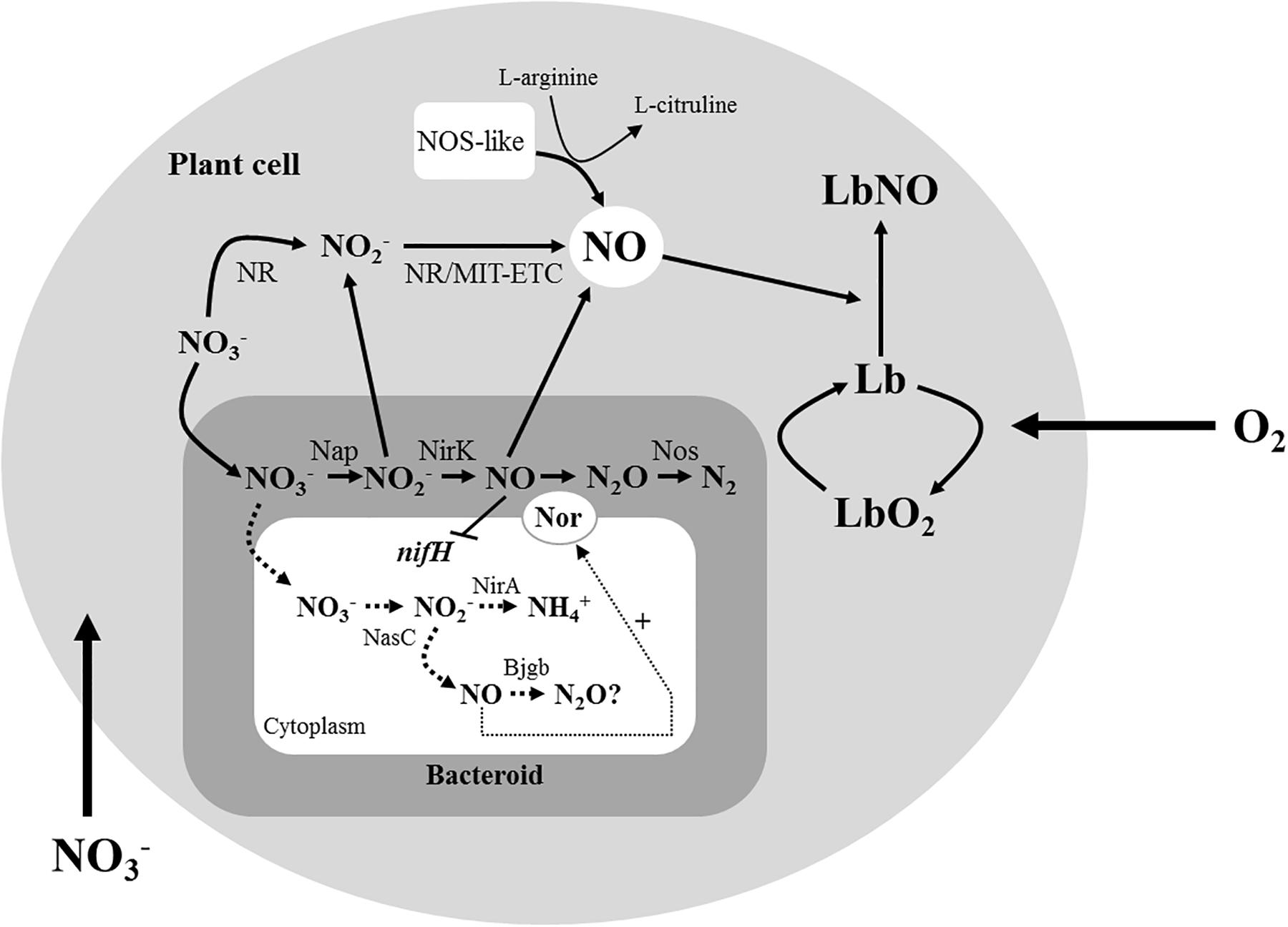
Figure 3. Proposed role of Bjgb in soybean nodules, alongside well-characterized sources of NO in nodules. The large gray circle represents the plant cell, and the small gray square represents the bacteroid where the periplasm is shown in gray and the cytoplasm is shown in white. Adapted from Torres et al. (2016). In addition to the reported plant sources of NO in legume nodules, denitrification in the bacteroids also contributes to the formation of this molecule. During this process, NO2– and NO produced by Nap and NirK, respectively, can bind Lb producing LbNO complexes. It has also been reported that NO is an inhibitor of nifH expression. Results from this work suggest that in addition to denitrification, assimilatory nitrate reduction by NasC might be another source of NO in nodules. NO produced in the bacteroid cytoplasm by this system would act as a signal molecule to activate nor genes. The ability of Bjgb to bind NO in vitro or to reduce NO to N2O in vivo (indicated with a question mark) is under investigation.
Data Availability Statement
All datasets generated for this study are included in the article/Supplementary Material.
Author Contributions
AS, AG, and MD conceived and designed the study, analyzed the results, and wrote the manuscript. AS, GT, AH-G, and AD performed the experiments. DR and EB critically revised the manuscript. All authors read and approved the final version of the manuscript.
Funding
This work was supported by the European Regional Development Fund (ERDF) co-financed grants from Ministerio de Economia y Competitividad, Spain [Grant Numbers: AGL2013-45087-R and AGL2017-85676R (MD)]; the Junta de Andalucia [Grant Number: PE2012-AGR1968 (EB)]; the Biotechnology and Biological Sciences Research Council [Grant Number: BB/M00256X/1 (AG)]; and the Royal Society International Exchanges Program, United Kingdom [Grant Number: IE140222 (AG and MD)]. AS was financed by a FPU contract from Ministerio de Educación, Cultura y Deporte (FPU14/04527).
Conflict of Interest
The authors declare that the research was conducted in the absence of any commercial or financial relationships that could be construed as a potential conflict of interest.
Acknowledgments
We thank the analysis service from Estación Experimental del Zaidín (Consejo Superior de Investigaciones Científicas, Granada, Spain) for performing the total nitrogen analysis. We also thank María Romero from Estación Experimental del Zaidín (Consejo Superior de Investigaciones Científicas, Granada, Spain) for helping in the fluorimetric detection of NO.
Supplementary Material
The Supplementary Material for this article can be found online at: https://www.frontiersin.org/articles/10.3389/fmicb.2019.02915/full#supplementary-material
FIGURE S1 | Ethylene emission by soybean nodules. Plants were inoculated with B. diazoefficiens USDA 110 (WT) (squares) or 4001 (bjgb mutant) (circles) strains. Nodules were isolated from non-flooded plants (white symbols) or plants subjected to flooding conditions for 7 days (black symbols). Data are means with standard deviations from two independent experiments assayed by using six replicates.
TABLE S1 | Primers used for qRT-PCR analyses.
Abbreviations
ARA, acetylene reduction activity; Bjgb, Bradyrhizobium diazoefficiens hemoglobin; fHb, flavohemoglobin; Flp, flavoprotein; FN, fixed nitrogen; Hbs, hemoglobins; Lb, leghemoglobin; N2O, nitrous oxide; Nap, periplasmic respiratory nitrate redutase; NarK, nitrate/nitrite transporter; NasC, assimilatory nitrate reductase; Ndfa, nitrogen derived from the atmosphere; NDW, nodule dried weight; NDWP, nodule dry weight per plant; NFW, nodule fresh weight; NifH, Fe-protein of the nitrogenase complex; NirK, copper-dependent respiratory nitrite reductase; nmol, nanomol; NNP, nodule number per plant; NO, nitric oxide; Nor, nitric oxide reductase; PDW, plant dry weight; TN, total nitrogen; WT, wild-type.
Footnotes
References
Babst, M., Hennecke, H., and Fischer, H. M. (1996). Two different mechanisms are involved in the heat-shock regulation of chaperonin gene expression in Bradyrhizobium japonicum. Mol. Microbiol. 19, 827–839. doi: 10.1046/j.1365-2958.1996.438968.x
Bacanamwo, M., and Purcell, L. C. (1999). Soybean dry matter and N accumulation responses to flooding stress, N sources and hypoxia. J. Exp. Bot. 50, 689–696. doi: 10.1093/jexbot/50.334.689
Bedmar, E. J., Bueno, E., Correa, D., Torres, M. J., Delgado, M. J., and Mesa, S. (2013). “Ecology of denitrification in soils and plant-associated bacteria,” in Beneficial Plant-Microbial Interactions: Ecology and Applications, eds M. B. R. González and J. González-López, (Florida: CRC Press), 164–182.
Bedmar, E. J., Robles, E. F., and Delgado, M. J. (2005). The complete denitrification pathway of the symbiotic, nitrogen-fixing bacterium Bradyrhizobium japonicum. Biochem. Soc. Trans. 33, 141–144. doi: 10.1042/BST0330141
Berger, A., Brouquisse, R., Pathak, P. K., Hichri, I., Singh, I., Bhatia, S., et al. (2018). Pathways of nitric oxide metabolism and operation of phytoglobins in legume nodules: missing links and future directions. Plant Cell Environ. 41, 2057–2068. doi: 10.1111/pce.13151
Bergersen, F. J. (1977). “A treatise on dinitrogen fixation,” in Biology: Section III, eds R. W. Hardy and W. Silver, (New York, NY: John Wiley & Sons), 519–556.
Blanquet, P., Silva, L., Catrice, O., Bruand, C., Carvalho, H., and Meilhoc, E. (2015). Sinorhizobium meliloti Controls nitric oxide-mediated post-translational modification of a Medicago truncatula nodule protein. Mol. Plant Microbe Interact. 28, 1353–1363. doi: 10.1094/MPMI-05-15-0118-R
Boscari, A., Del Giudice, J., Ferrarini, A., Venturini, L., Zaffini, A. L., Delledonne, M., et al. (2013). Expression dynamics of the Medicago truncatula transcriptome during the symbiotic interaction with Sinorhizobium meliloti: which role for nitric oxide? Plant Physiol. 161, 425–439. doi: 10.1104/pp.112.208538
Bueno, E., Robles, E. F., Torres, M. J., Krell, T., Bedmar, E. J., Delgado, M. J., et al. (2017). Disparate response to microoxia and nitrogen oxides of the Bradyrhizobium japonicum napEDABC, nirK and norCBQD denitrification genes. Nitr. Oxide 68, 137–149. doi: 10.1016/j.niox.2017.02.002
Cabrera, J. J., Salas, A., Torres, M. J., Bedmar, E. J., Richardson, D. J., Gates, A. J., et al. (2016). An integrated biochemical system for nitrate assimilation and nitric oxide detoxification in Bradyrhizobium japonicum. Biochem. J. 473, 297–309. doi: 10.1042/BJ20150880
Cabrera, J. J., Sanchez, C., Gates, A. J., Bedmar, E. J., Mesa, S., Richardson, D. J., et al. (2011). The nitric oxide response in plant-associated endosymbiotic bacteria. Biochem. Soc. Trans. 39, 1880–1885. doi: 10.1042/BST20110732
Cam, Y., Pierre, O., Boncompagni, E., Herouart, D., Meilhoc, E., and Bruand, C. (2012). Nitric oxide (NO): a key player in the senescence of Medicago truncatula root nodules. N. Phytol. 196, 548–560. doi: 10.1111/j.1469-8137.2012.04282.x
Corpas, F. J., and Barroso, J. B. (2015). Nitric oxide from a “green” perspective. Nitr. Oxide 45, 15–19. doi: 10.1016/j.niox.2015.01.007
Delgado, M. J., Bonnard, N., Tresierra-Ayala, A., Bedmar, E. J., and Muller, P. (2003). The Bradyrhizobium japonicum napEDABC genes encoding the periplasmic nitrate reductase are essential for nitrate respiration. Microbiology 149, 3395–3403. doi: 10.1099/mic.0.26620-0
Domingos, P., Prado, A. M., Wong, A., Gehring, C., and Feijo, J. A. (2015). Nitric oxide: a multitasked signaling gas in plants. Mol. Plant 8, 506–520. doi: 10.1016/j.molp.2014.12.010
Gardner, P. R. (2005). Nitric oxide dioxygenase function and mechanism of flavohemoglobin, hemoglobin, myoglobin and their associated reductases. J. Inorg. Biochem. 99, 247–266. doi: 10.1016/j.jinorgbio.2004.10.003
Gardner, P. R., Gardner, A. M., Martin, L. A., and Salzman, A. L. (1998). Nitric oxide dioxygenase: an enzymic function for flavohemoglobin. Proc. Natl. Acad. Sci. U.S.A. 95, 10378–10383. doi: 10.3410/f.718030775.793480279
Gell, D. A. (2018). Structure and function of hemoglobins. Blood Cells Mol. Dis. 70, 13–42. doi: 10.1016/j.bcmd.2017.10.006
Hausladen, A., Gow, A., and Stamler, J. S. (2001). Flavohemoglobin denitrosylase catalyzes the reaction of a nitroxyl equivalent with molecular oxygen. Proc. Natl. Acad. Sci. U.S.A. 98, 10108–10112. doi: 10.1073/pnas.181199698
Hichri, I., Boscari, A., Castella, C., Rovere, M., Puppo, A., and Brouquisse, R. (2015). Nitric oxide: a multifaceted regulator of the nitrogen-fixing symbiosis. J. Exp. Bot. 66, 2877–2887. doi: 10.1093/jxb/erv051
Horchani, F., Prevot, M., Boscari, A., Evangelisti, E., Meilhoc, E., Bruand, C., et al. (2011). Both plant and bacterial nitrate reductases contribute to nitric oxide production in Medicago truncatula nitrogen-fixing nodules. Plant Physiol. 155, 1023–1036. doi: 10.1104/pp.110.166140
Kato, K., Kanahama, K., and Kanayama, Y. (2010). Involvement of nitric oxide in the inhibition of nitrogenase activity by nitrate in Lotus root nodules. J. Plant Physiol. 167, 238–241. doi: 10.1016/j.jplph.2009.08.006
Kim, S. O., Orii, Y., Lloyd, D., Hughes, M. N., and Poole, R. K. (1999). Anoxic function for the Escherichia coli flavohemoglobin (Hmp): reversible binding of nitric oxide and reduction to nitrous oxide. FEBS Lett. 445, 389–394. doi: 10.1016/S0014-5793(99)00157-X
Meakin, G. E., Bueno, E., Jepson, B., Bedmar, E. J., Richardson, D. J., and Delgado, M. J. (2007). The contribution of bacteroidal nitrate and nitrite reduction to the formation of nitrosylleghemoglobin complexes in soybean root nodules. Microbiology 153, 411–419. doi: 10.1099/mic.0.2006/000059-0
Meilhoc, E., Blanquet, P., Cam, Y., and Bruand, C. (2013). Control of NO level in rhizobium-legume root nodules: not only a plant globin story. Plant Signal. Behav. 8:e25923. doi: 10.4161/psb.25923
Mesa, S., Alche, J. D., Bedmar, E., and Delgado, M. J. (2004). Expression of nir, nor and nos denitrification genes from Bradyrhizobium japonicum in soybean root nodules. Physiol. Plant. 120, 205–211. doi: 10.1111/j.0031-9317.2004.0211.x
Mesa, S., Velasco, L., Manzanera, M. E., Delgado, M. J., and Bedmar, E. J. (2002). Characterization of the norCBQD genes, encoding nitric oxide reductase, in the nitrogen fixing bacterium Bradyrhizobium japonicum. Microbiology 148, 3553–3560. doi: 10.1099/00221287-148-11-3553
Pfaffl, M. W. (2001). A new mathematical model for relative quantification in real-time RT-PCR. Nucleic Acids Res. 29:e45. doi: 10.1093/nar/29.9.e45
Poole, P., Ramachandran, V., and Terpolilli, J. (2018). Rhizobia: from saprophytes to endosymbionts. Nat. Rev. Microbiol. 16, 291–303. doi: 10.1038/nrmicro.2017.171
Poole, R. K. (2005). Nitric oxide and nitrosative stress tolerance in bacteria. Biochem. Soc. Trans. 33, 176–180. doi: 10.1042/BST0330176
Regensburger, B., and Hennecke, H. (1983). RNA polymerase from Rhizobium japonicum. Arch. Microbiol. 135, 103–109. doi: 10.1007/BF00408017
Rigaud, J., and Puppo, A. (1975). Indole-3-acetic acid catabolism by soybean bacteroids. J. Gen. Microbiol. 88, 223–228. doi: 10.1099/00221287-88-2-223
Sanchez, C., Cabrera, J. J., Gates, A. J., Bedmar, E. J., Richardson, D. J., and Delgado, M. J. (2011a). Nitric oxide detoxification in the rhizobia-legume symbiosis. Biochem. Soc. Trans. 39, 184–188. doi: 10.1042/BST0390184
Sanchez, C., Tortosa, G., Granados, A., Delgado, A., Bedmar, E. J., and Delgado, M. J. (2011b). Involvement of Bradyrhizobium japonicum denitrification in symbiotic nitrogen fixation by soybean plants subjected to flooding. Soil Biol. Biochem. 43, 212–217. doi: 10.1016/j.soilbio.2010.09.020
Sanchez, C., Gates, A. J., Meakin, G. E., Uchiumi, T., Girard, L., Richardson, D. J., et al. (2010). Production of nitric oxide and nitrosylleghemoglobin complexes in soybean nodules in response to flooding. Mol. Plant Microbe Interact. 23, 702–711. doi: 10.1094/MPMI-23-5-0702
Sprent, J. I. (2009). An interdisciplinary look at legumes and their bacterial symbionts: some thoughts from Big Sky. New Phytol. 184, 15–17. doi: 10.1111/j.1469-8137.2009.03006.x
Stern, A. M., and Zhu, J. (2014). An introduction to nitric oxide sensing and response in bacteria. Adv. Appl. Microbiol. 87, 187–220. doi: 10.1016/B978-0-12-800261-2.00005-0
Thalineau, E., Truong, H. N., Berger, A., Fournier, C., Boscari, A., Wendehenne, D., et al. (2016). Cross-regulation between N metabolism and nitric oxide (NO) signaling during plant immunity. Front. Plant Sci. 7:472. doi: 10.3389/fpls.2016.00472
Tinajero-Trejo, M., Vreugdenhil, A., Sedelnikova, S. E., Davidge, K. S., and Poole, R. K. (2013). Nitric oxide reactivities of the two globins of the foodborne pathogen Campylobacter jejuni: roles in protection from nitrosative stress and analysis of potential reductants. Nitr. Oxide 34, 65–75. doi: 10.1016/j.niox.2013.06.002
Toledo, J. C. Jr., and Augusto, O. (2012). Connecting the chemical and biological properties of nitric oxide. Chem. Res. Toxicol. 25, 975–989. doi: 10.1021/tx300042g
Torres, M. J., Simon, J., Rowley, G., Bedmar, E. J., Richardson, D. J., Gates, A. J., et al. (2016). Nitrous oxide metabolism in nitrate-reducing bacteria: physiology and regulatory mechanisms. Adv. Microb. Physiol. 68, 353–432. doi: 10.1016/bs.ampbs.2016.02.007
Tortosa, G., Hidalgo, A., Salas, A., Bedmar, E. J., Mesa, S., and Delgado, M. J. (2015). Nitrate and flooding induce N2O emissions from soybean nodules. Symbiosis 67, 125–133. doi: 10.1007/s13199-015-0341-3
Trung, B. C., and Yoshida, S. (1983). Improvement of Leonard jar assembly for screening of effective rhizobium. Soil Sci. Plant Nutr. 29, 97–100. doi: 10.1080/00380768.1983.10432410
Udvardi, M., and Poole, P. S. (2013). Transport and metabolism in legume-rhizobia symbioses. Annu. Rev. Plant Biol. 64, 781–805. doi: 10.1146/annurev-arplant-050312-120235
Velasco, L., Mesa, S., Delgado, M. J., and Bedmar, E. J. (2001). Characterization of the nirK gene encoding the respiratory, Cu-containing nitrite reductase of Bradyrhizobium japonicum. Biochim. Biophys. Acta 1521, 130–134. doi: 10.1016/S0167-4781(01)00279-2
Velasco, L., Mesa, S., Xu, C. A., Delgado, M. J., and Bedmar, E. J. (2004). Molecular characterization of nosRZDFYLX genes coding for denitrifying nitrous oxide reductase of Bradyrhizobium japonicum. Antonie Van Leeuwenhoek 85, 229–235. doi: 10.1023/B:ANTO.0000020156.42470.db
Keywords: bacterial hemoglobins, nitrogen fixation, nitrogenase, nitric oxide, 15N isotope, soybean nodules
Citation: Salas A, Tortosa G, Hidalgo-García A, Delgado A, Bedmar EJ, Richardson DJ, Gates AJ and Delgado MJ (2020) The Hemoglobin Bjgb From Bradyrhizobium diazoefficiens Controls NO Homeostasis in Soybean Nodules to Protect Symbiotic Nitrogen Fixation. Front. Microbiol. 10:2915. doi: 10.3389/fmicb.2019.02915
Received: 02 September 2019; Accepted: 03 December 2019;
Published: 10 January 2020.
Edited by:
Aymé Spor, INRA UMR 1347 Agroécologie, FranceReviewed by:
Mika Nomura, Kagawa University, JapanToshiki Uchiumi, Kagoshima University, Japan
Anibal Roberto Lodeiro, CONICET Institute of Biotechnology and Molecular Biology (IBBM), Argentina
Copyright © 2020 Salas, Tortosa, Hidalgo-García, Delgado, Bedmar, Richardson, Gates and Delgado. This is an open-access article distributed under the terms of the Creative Commons Attribution License (CC BY). The use, distribution or reproduction in other forums is permitted, provided the original author(s) and the copyright owner(s) are credited and that the original publication in this journal is cited, in accordance with accepted academic practice. No use, distribution or reproduction is permitted which does not comply with these terms.
*Correspondence: Andrew J. Gates, YS5nYXRlc0B1ZWEuYWMudWs=; María J. Delgado, bWRlbGdhZG9AZWV6LmNzaWMuZXM=