- 1Shemyakin-Ovchinnikov Institute of Bioorganic Chemistry, Russian Academy of Sciences, Moscow, Russia
- 2Department of Biology, Lomonosov Moscow State University, Moscow, Russia
- 3Zelinsky Institute of Organic Chemistry, Russian Academy of Sciences, Moscow, Russia
- 4Research Center “PhytoEngineering” Ltd., Rogachevo, Moscow, Russia
- 5Winogradsky Institute of Microbiology, Federal Research Center “Fundamentals of Biotechnology”, Russian Academy of Sciences, Moscow, Russia
Soft rot caused by numerous species of Pectobacterium and Dickeya is a serious threat to the world production of potatoes. The application of bacteriophages to combat bacterial infections in medicine, agriculture, and the food industry requires the selection of comprehensively studied lytic phages and the knowledge of their infection mechanism for more rational composition of therapeutic cocktails. We present the study of two bacteriophages, infective for the Pectobacterium brasiliense strain F152. Podoviridae PP99 is a representative of the genus Zindervirus, and Myoviridae PP101 belongs to the still unclassified genomic group. The structure of O-polysaccharide of F152 was established by sugar analysis and 1D and 2D NMR spectroscopy:
→ 4)-α-D-Manp6Ac-(1→ 2)-α-D-Manp-(1→ 3)-β-D-Galp-(1→
The recombinant tail spike protein of phage PP99, gp55, was shown to deacetylate the side chain talose residue of bacterial O-polysaccharide, thus providing the selective attachment of the phage to the cell surface. Both phages demonstrate lytic behavior, thus being prospective for therapeutic purposes.
Introduction
Pectobacterium brasiliense (Pbr) is a member of the soft rot Pectobacteriaceae (SRP) (Adeolu et al., 2016). The representatives of Pectobacterium and Dickeya genera comprising this family are important pathogens of many crops and ornamental plants worldwide (Ma et al., 2007). Pbr was first identified as a strain group within Pectobacterium carotovorum, causing severe black leg disease of potatoes in Brazil (Duarte et al., 2004). This group of strains shared some physiological properties with P. atrosepticum (Pat), and was able to grow in a broader range of environmental temperatures. Since then, similar strains were identified as causative agents of black leg and soft rot of potatoes in many countries, including Russia (Malko et al., 2019; Voronina et al., 2019b). Following the taxonomic reassessment of phytopathogenic pectobacteria, Pbr was rated as a subspecies of the P. carotovorum (Nabhan et al., 2012), and was recently elevated to the species rank (Portier et al., 2019).
One of the currently applied promising strategies for plant and crop preservations is treating seeds and harvested tubers with bacteriophage preparations. This approach is friendly to the environment and offers a good protection degree against plant bacterial pathogens (Frampton et al., 2012; Svircev et al., 2018). One of the pathogen groups that were studied in this context are pectolytic bacteria Pectobacterium and Dickeya, where good results of phage application have been reported. Most trials in phage control were applied either to the most widespread or most virulent pathogens–P. carotovorum (Lim et al., 2013; Muturi et al., 2019), P. atrosepticum (Carstens et al., 2019) and Dickeya solani (Adriaenssens et al., 2012; Czajkowski et al., 2017). Most phages suitable for the control of these phytopathogens are members of subfamilies Autographivirinae, Aglimvirinae, and lytic phages belonging to the less characterized genera. Usually, such phages are stable and infective in a broad pH range and ionic strength, at temperatures up to 50°C, resistant to chloroform, but are rapidly inactivated by UV irradiation (Gupta et al., 1995; Czajkowski et al., 2015, 2017). Up to now, no bacteriophages specifically infectious to Pbr are reported, to our knowledge. Some phages isolated against Pectobacterium carotovorum subsp. carotovorum have been shown to infect several strains of Pbr (Lim et al., 2015, 2017; Kim et al., 2019). Information on the factors explaining the host range of particular SRP phages is also limited. Present study is focused on an investigation of Pbr–specific bacteriophages isolated in Russia, and the details of their interaction with the bacterial host on molecular level.
Materials and Methods
Bacterial Strain
Pectobacterium brasiliense strain F152 (PB29) was isolated in 2014 in a Moscow region from a potato tuber with soft rot symptoms. The strain was analyzed using commercial Biolog GN2 Microplate System (Biolog, Inc.) and API 20E system (bioMérieux). The Biolog GN2 Microplate System and the API 20E system were used in parallel twice, independently. The bacteria were incubated on LB agar (10 g of tryptone, 5 g of yeast extract, and 10 g of NaCl in 1 l, pH 7.2) at 28°C for 24 h prior to analysis. The optical density of the suspension was adjusted as recommended by the manufacturer, and the further procedure was held according to the system instructions. Control for repeatability was held by second round of testing.
Isolation and Purification of Phages PP99 and PP101
Bacteriophage PP99 and PP101 were isolated in May, 2015, from the same sample of sewage water in a warehouse containing potatoes (Moscow region, Russia). Phages were propagated on a Pbr strain F152 (PB29) in LB at 28°C using a published protocol (Clokie and Kropinski, 2009). The lysate was treated with chloroform, centrifuged to clear the cell debris (8,000 g, 20 min), sterilized by filtering through a 0.22 μm pore size membrane filter (Millipore), and treated with DNase I (0.5 mg/mL, 60 min). Phage particles were pelleted in the ultracentrifuge (100,000 g, 60 min, 4°C, Beckman Type 45 rotor) and further purified by CsCl step gradient (0.5–1.7 g/ml density range) ultracentrifugation (22,000 g, 120 min, 4°C, Beckman SW28 rotor). The resulting suspensions of PP99 and PP101 were dialyzed overnight against suspension medium SM (10 mM Tris HCl, pH 7.4, 10 mM MgSO4) to remove CsCl. Purified phages were stored at 4°C in SM buffer as a transparent liquid showing no signs of aggregation.
Electron Microscopy
Purified phage particles were applied to nitrocellulose/carbon film-coated TEM grids and stained with a 1% uranyl acetate aqueous solution (Ackermann, 2009). The specimens were visualized in a Zeiss Libra 120 electron microscope at 100 kV accelerating voltage. The dimensions of phage virions were averaged from the reads of at least 20 particles.
Host Range and General Characterization of Phages PP99 and PP101
The host range of phages was tested by standard plaque formation assay using phage serial dilutions and by direct spotting phage suspensions (106 pfu/ml) onto a bacterial lawn. Bacterial strains listed in Table S1 were cultivated on LB agar at 28°C. In phage adsorption experiments, the host strains were grown to an OD600 ~0.4 and infected with individual phages at a multiplicity of infection of 0.1, or a mixture of PP99 and PP101 at MOI 0.05 each. Every 1 min after infection, 100 μl aliquots of phage-host mixture were taken and transferred into an 800 μl LB medium supplied with 50 μl of chloroform. After bacterial lysis the mixtures were centrifuged and the supernatant was assayed to determine the amount of non-adsorbed or reversibly bound phages. Phage stability was studied by incubating a 107 pfu/ml phage suspension at different temperatures or in a range of buffer solutions (20 mM Tris HCl/20 mM Na citrate/20 mM Na phosphate), adjusted with NaOH to pH range 4–9. One-step-growth assays were performed according to Adriaenssens et al. (2012). To assay a lytic activity of phages an exponentially growing culture of host bacteria (106 cfu/ml) was mixed with PP99 or PP101 (MOI of 0.1). The mixture was then incubated with shaking at 28°C. Every 10 min, aliquots were taken, chilled, centrifuged, and the appropriate dilutions of the supernatant containing unbound phages were spread on LB agar plates and incubated overnight at 28°C. The next day, colonies were counted. All experiments were performed independently 3–4 times, and the results were averaged.
Genome Sequencing and Annotation
Bacterial and phage DNA was phenol extracted and fragmented with a Bioruptor Sonicator (Diagenode). Paired-end libraries were constructed using a Nebnext Ultra DNA library prep kit (New England Biolabs) and sequenced on the Illumina MiSeq™ platform (Illumina), using paired 150 bp reads. After filtering with a CLC Genomics Workbench 8.5 (Qiagen), overlapping paired-end library reads were merged with the SeqPrep tool (https://github.com/jstjohn/SeqPrep). Reads from bacteriophages PP99 and PP101 were assembled on a CLC Genomic workbench v. 7.5; reads from a F152 (PB29) bacterial strain were assembled using SPADES 3.6.1 (Bankevich et al., 2012).
The bacterial genome was annotated with the online RAST automated pipeline (http://rast.nmpdr.org/) (Aziz et al., 2008). Phage genomes were annotated by predicting and validating open reading frames (ORFs) using Prodigal 2.6.1 (Hyatt et al., 2010), GeneMarkS 4.3 (Besemer et al., 2001), and Glimmer 3.02 (Delcher et al., 1999). Found ORFs were manually curated to ensure fidelity. Functions were assigned to ORFs using a BLAST search on NCBI databases (http://blast.ncbi.nlm.nih.gov), InterProScan (Mitchell et al., 2015), HHpred (https://toolkit.tuebingen.mpg.de/#/tools/hhpred) (Söding et al., 2005), using databases PDB, SCOP, Pfam, NCBI_CONSERVED. tRNA coding regions were identified with tRNAscan-SE (Schattner et al., 2005) and ARAGORN (Laslett and Canback, 2004). Putative phage promoters were predicted by PHIRE (Lavigne et al., 2004) and phiSITE (Klucar et al., 2009). Resulting genomes were visualized in Geneious Prime, version 2019.2.1 (https://www.geneious.com).
Genome Comparison and Taxonomy
Bacterial and phage reference genomes were downloaded from NCBI Genbank (ftp://ftp.ncbi.nlm.nih.gov/genbank). The phylogenetic tree of Pectobacterium brasiliense was generated by means of a UBCG pipeline, using 92 core genes (Na et al., 2018). To conduct bootstrap analysis phylogeny, we have aligned concatenated sequences of 92 core genes made by UBCG with MAFFT (FFT-NS-x1000, 200 PAM/k = 2), and constructed bootstrap trees with an RAxML program (Stamatakis, 2014) (GTR Gamma I DNA substitution model). The robustness of the trees was assessed by fast bootstrapping (1000).
Genes of phage DNA polymerase, a major capsid protein, RNA polymerase and a terminase large subunit were extracted from downloaded Genbank annotated genomes. Gene products in genomes annotated as “hypothetical protein CDS” were considered as known genes if their pairwise identity with known homologous was more than 50%. Phylograms were generated based on the amino acid sequences of proteins and their concatenated alignments, using Geneious Prime and applying Clustal Omega for sequence alignment with auto settings. Trees were constructed using the maximum likelihood (ML) method with an RAxML program (Stamatakis, 2014) with a GAMMA I BLOSUM62 protein model and the robustness of the trees was assessed by fast bootstrapping (1000). Average nucleotide identity (ANI) was computed using Jspecies (Richter and Rossello-Mora, 2009) (blast algorithm ANIb, 500 bp fragment length for phages and 1020 bp for bacteria). Distance matrix was computed using an Enveomics server (http://enve-omics.ce.gatech.edu) (Konstantinidis and Tiedje, 2005). Digital DNA-DNA hybridization (dDDH) was estimated using a GGDC calculator (https://ggdc.dsmz.de/ggdc.php) (Meier-Kolthoff et al., 2013).
Tail Spike Protein Cloning, Expression, and Purification
ORF55 of phage PP99 (42,368–44,020 bp range) was PCR-amplified using primers 5′-TATTTCCAGGGCAGCGGATCCGGTTATAGTACAAAGCCAAAAAGA (forward) and 5′-GCTCGAGTGCGGCCGCAAGCTTACAGGTTTGCTGTTACAGAATA (reverse) with generated BamHI and HindIII cloning sites, respectively. The amplified ORF was cloned to vector pTSL (Taylor et al., 2016), using a NEBuilder HiFi DNA Assembly kit (New England Biolabs). Clones carrying the insert were screened by PCR using the same primers, endonuclease hydrolysis, and verified by Sanger sequencing. Protein synthesis was performed in E. coli B834(DE3) inducing the expression with 1 mM IPTG at 16°C overnight. Cells were centrifuged at 4,000 g, resuspended in a 20 mM Tris-HCl (pH 8.0), 200 mM NaCl buffer, lysed by ultrasonic treatment (Virsonic, VirTis) and the debris and unbroken cells were removed by centrifugation at 13,000 g. The protein product (PP99 gp55) was purified on a Ni-NTA Sepharose column (GE Healthcare, 5 mL) by 0–200 mM imidazole step gradient in 20 mM TrisHCl (pH 8.0), 200 mM NaCl. The resulting eluate containing the purified protein was dialyzed against 20 mM TrisHCl (pH 8.0) to remove imidazole, and 6× His-tag was removed by TEV protease (12 h at 20°C incubation). The target protein was finally purified on a 5 mL SourceQ 15 (GE Healthcare) using a linear gradient of 0–600 mM NaCl in 20 mM TrisHCl (pH 8.0). Protein concentration was determined spectrophotometrically at 280 nm, using a calculated molar extinction coefficient of 56,435 M−1 cm−1. The PP99 gp55 oligomeric state was assessed by gel-filtration on a calibrated Superdex 200 10 × 300 column (GE Healthcare).
Isolation and O-Deacetylation of the O-Polysaccharides
Bacterial lipopolysaccharide (LPS) was isolated in a yield of 8.4% from bacterial cells by the phenol-water method (Westphal and Jann, 1965) and purified by precipitation of nucleic acids and proteins with aq 50% CCl3CO2H as described (Zych et al., 2001). Then the LPS sample (84 mg) was degraded with aq 2% HOAc for 1.5 h at 100°C. Lipids were removed by centrifugation (13,000 g, 20 min), and the supernatant was applied to the gel-filtration column 70 × 3.0 cm, Sephadex G-50 Superfine (GE Healthcare), using 0.05 M pyridinium acetate pH 4.5 as eluent and monitoring with a differential refractometer (Knauer). A high-molecular mass polysaccharide was obtained in a yield of ~25% of the lipopolysaccharide weight.
Total O-Deacetylation was performed by treatment of an O-polysaccharide sample (21 mg), with 12% aq ammonia (0.6 mL) at 37°C for 16 h. After ammonia evaporation the remaining solution was lyophilized, and an O-deacetylated polysaccharide (DPS) sample was obtained in a yield of 46% of the O-polysaccharide weight.
The effect of the phage PP99 tail spike protein was studied by an addition of a 300 μg aliquote of PP99 gp55 to the O-polysaccharide sample (20 mg), and incubation for 2 h at room temperature. The product was isolated by gel-permeation chromatography, as described above.
Sugar Analysis
Hydrolysis of the O-polysaccharide was performed with 2 M CF3CO2H (120°C, 2 h), and the monosaccharides were analyzed by GLC as the alditol acetates (Sawabdekeb et al., 1965) on a Maestro (Agilent 7820) chromatograph (Interlab) equipped with an HP-5ms column (0.32 mm × 30 m), using a temperature program of 160°C (1 min) to 290°C at 7°C min−1.
NMR Spectroscopy
Samples were deuterium-exchanged by freeze-drying from 99.9% D2O. NMR spectra were recorded for solutions in 99.95% D2O at 30°C on a Bruker Avance II 600 MHz spectrometer with a 5-mm broadband inverse probe head. Sodium 3-(trimethylsilyl) propanoate-2,2,3,3-d4 (δH 0, δC −1.6) was used as an internal reference for calibration. Two-dimensional NMR spectra were obtained using standard Bruker software, and a Bruker TopSpin 2.1 program was used to acquire and process the NMR data. A spin-lock time of 60 ms and a mixing time of 200 ms were used in two-dimensional TOCSY and ROESY experiments, respectively. A two-dimensional 1H, 13C HMBC experiment was performed with a 60-ms delay for evolution of long-range couplings in order to optimize the spectrum for coupling constant JH,C 8 Hz.
Results
Properties and Genomics of Strain F152 (PB29)
Pectobacterium brasiliense strain F152 (PB29) was isolated in 2014, and initially was attributed as P. c. subsp. carotovorum. Further complete genome sequencing identified this strain as a Pbr. Variability in physiology, plant host range, virulence, and genomics of strains representing P. carotovorum have promoted the first rough distribution of this genus to separate the subspecies carotovorum, brasiliense and odoriferum (Nabhan et al., 2012). Later, a number of further separations of P. carotovorum subsp. carotovorum were offered, forming new species, polaris (Dees et al., 2017), maceratum/versatile (Shirshikov et al., 2018; Portier et al., 2019), peruviense (Waleron et al., 2018), and aquaticum (Pédron et al., 2019), based on genomic analysis of available MLST markers, draft and complete genomes in databases. Recently, a substantial redistribution of pectobacterial taxonomy was processed, elevating a number of P. carotovorum subspecies to the species level, including Pbr (Portier et al., 2019). Strain F152 follows the general biochemical and physiological properties typical for Pbr species (Nabhan et al., 2012; Portier et al., 2019). Bacterial cells of strain F152 are gram-negative, facultative anaerobes, negative for oxidase, urease, indol production, and gelatin liquefaction. They were negative for acid production from D-arabitol, dulicitol, and sorbitol, and were unable to utilize malonate and citrate. Cells are catalase positive, produce acid from lactose, rhamnose, and trehalose, resistant to 6% NaCl and grow at both 28 and 37°C. Strain F152 induces a hypersensitive reaction in tobacco leaves and causes severe black leg symptoms on green plants and soft rot symptoms on potato tuber disks compared to the characterized strains of P. polaris, P. aquaticum, P. versatile, and P. atrosepticum in a temperature range from 20 to 28°C. The same properties were observed for other Pbr strains previously isolated in Russia, F126 and F157 (Voronina et al., 2019b). However, the genome sequences of Pbr strains deposited to the NCBI GenBank demonstrate pronounced diversity, forming several branches of the phylogenomic clade (Zhang et al., 2016; Li et al., 2018). Phylogenetic analysis using concatenated sequences of 92 core genes resulted in a similar tree (Figure 1), where the genomes of F126 (RRYQ00000000), F152 (PJDM00000000), and F157 (PJDL00000000) are located in a branch diverged from the Pbr type strain LMG 21371 (=PBR1692). Measurements of ANI and dDDH also demonstrate a genetic difference between two clades of Pbr (Figure 1, Figure S1). Therefore, the properties of strain F152, including phage susceptibility, cannot be directly extrapolated to all strains representing Pbr.
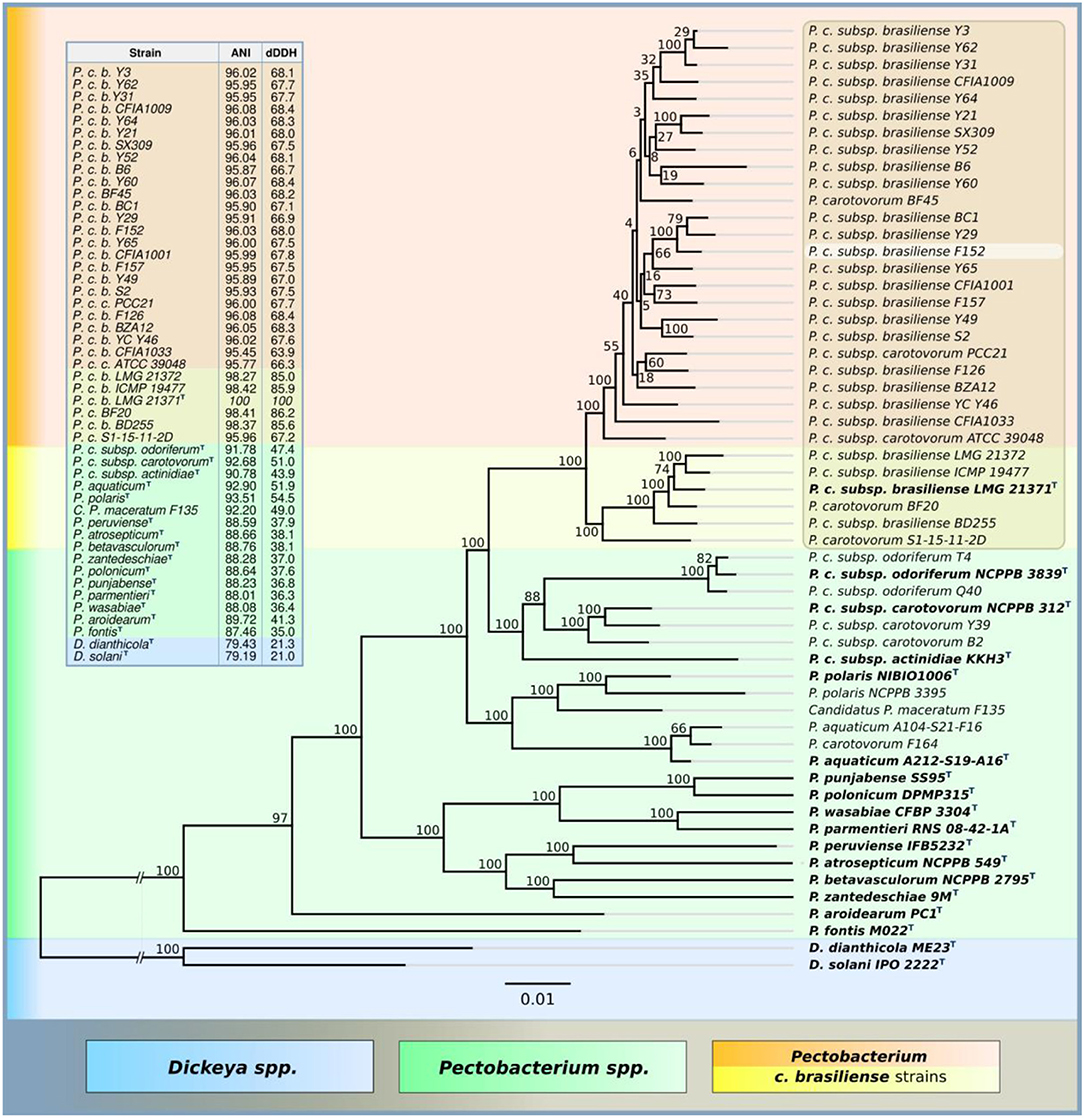
Figure 1. Phylogenetic tree of nucleotide sequences of UBCG proteins of Pectobacterium spp. NCBI genomes (RAxML,GAMMA I BLOSSUM62 protein model, with 1,000 bootstrap replicates), ANI and dDDH are compared to P. brasiliense LMG 21371 type strain.
Phage PP99 and PP101—Biology, Host Range, Morphology
Bacteriophages PP99 and PP101 were isolated using Pbr strain F152 previously found in the same location as a host. Both phages form small (1–3 mm in diameter) plaques, normally indistinguishable from each other. Host ranges of PP99 and PP101 are nearly identical to each other. Both phages infect all strains of Pbr isolated in Central European Russia in 2000–2015, as well as nine of 26 Pectobacterium strains available in our collection (Table S1). Besides Pbr, all Pectobacterium strains susceptible to PP99 and PP101 belong to the newly formed species P. versatile (Pve) (Portier et al., 2019). The only strain that is infected by PP101 but is resistant to PP99 is F100, attributed as Pve by 16S RNA gene sequencing and genomic fingerprinting. Other tested strains belonging to P. atrosepticum, P. polaris, P. parmentieri, P. carotovorum, P. aquaticum, some strains of P. versatile, and all strains of genus Dickeya, were resistant to phages PP99 and PP101.
The morphology of bacteriophages PP99 and PP101 was assessed using transmission electron microscopy. Phage PP101 belongs to the family Myoviridae in the order Caudovirales, morphotype A1 (Figure 2B). The tail length is ~132 ± 5 nm, and the head diameter is ~62 ± 3 nm. PP99 shows typical Podoviridae morphology (morphotype C1) with an icosahedric capsid of ~54 ± 3 nm in diameter and a short (~10 nm) tail (Figure 2A). According to the proposed unified phage naming (Kropinski et al., 2009), the phages should be referred to as vB_PbrP_PP99 and vB_PbrM_PP101, respectively.
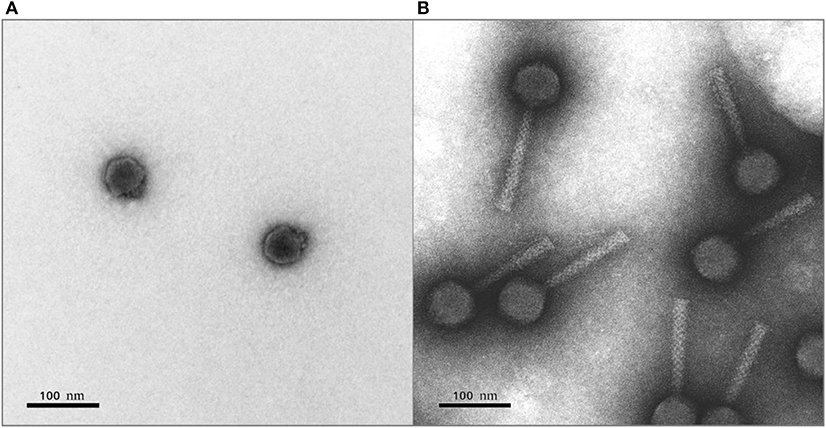
Figure 2. Transmission electron micrograph of bacteriophages PP99 (A) and PP101 (B). Staining with 1% uranyl acetate. The scale bar is 100 nm.
Both phages demonstrate biological properties close to each other and other studied pectobacterial phages, retaining infectional activity at temperatures below 50°C, and in the pH range 3–8. With respect to their isolation host, Pbr strain F152, phages PP99 and PP101 demonstrate similar infection kinetics adsorbing to bacteria in 4–6 min, with a latent period of 30–35 min and a burst size of 100–150 progeny phages/cell at 28°C (Figure 3). The combined action of PP99 and PP101 did not change the overall shape of the single-step growth curve (Figure 3) in short-term observations. Within a 2 h interval, the concentration of unadsorbed or progeny phages never changed substantially compared to a single-action mode of PP99 or PP101. This indicates an absence of synergistic or antagonistic character of two phages (Schmerer et al., 2014). It may mean that phages PP99 and PP101 independently use the same receptor molecule on the bacterial surface for adsorption, and none of them shows dominant affinity to this receptor; additionally, both phages can overcome the protective systems of Pbr F152 equally effectively.
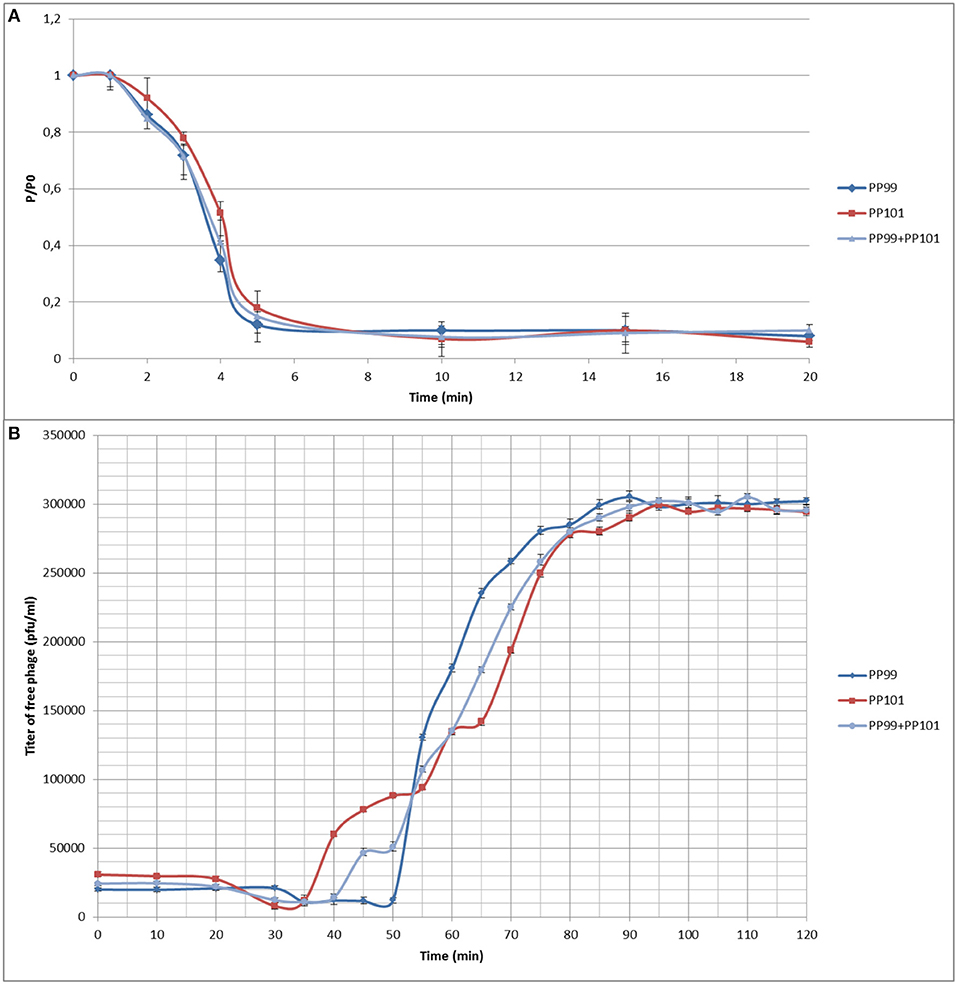
Figure 3. (A) Adsorption of phages on the surface of host strain F152: PP99 (blue) and PP101 (red) in MOI = 0.1 separately, and a mixture of PP99 and PP101 (gray) in MOI = 0.05 each. P/Po—ratio of titers of unadsorbed phages to the phage amount initially added; (B) One-step growth curves of phages PP99 (blue) and PP101 (red) in MOI = 0.1 separately, and a mixture of PP99 and PP101 (gray) in MOI = 0.05 each.
Bacteriophage PP99 Genome Features
The genome of bacteriophage PP99 (read in 1006× coverage) consists of 46,609 bp with a GC content of 45.54%. The only bacteriophage with noticeable homology in the genome sequence is the Escherichia phage ECBP5 (KJ749827), genetically assessed as a mosaic composition between various Autographivirinae phages (Lee et al., 2015; Figure 4A). Phylogenetic trees generated using concatenated alignments of amino acid sequences of DNA polymerase, a major capsid protein and a terminase large subunit show the relationship between PP99 and Salmonella phage SP6 (NCBI accession number AY370673) (Dobbins et al., 2004), Pectobacterium phage PP1 (NC_019542) (Lee et al., 2012), Vibrio phage φA318 (KF322026) (Liu et al., 2014), and a number of other Podoviruses infecting a broad range of bacteria (Figures S2, S3). Thus, PP99 can be assigned as a member of the genus SP6virus. This genus is currently named Zindervirus, after Norman D. Zinder (1928–2012), who discovered the phage-driven gene transfer. A total of 56 unidirectional ORFs and no tRNA genes were predicted. The functions of 26 ORFs can be predicted as members of transcription/translation, DNA replication/modification and nucleotide metabolism, phage morphogenesis, and host lysis modules. We were able to predict 10 phage-specific promotors (Figure 4A). Twenty-five ORFs were characterized as hypothetical proteins (Table S2). Only three small ORFs can be considered as unique to the PP99 genome. No genes responsible for lysogeny and toxin production were identified. The overall genome layout of PP99 follows the general features of SP6-like phages, including the presence of a pronounced set of early genes preceding the gene-encoding phage RNA polymerase, several apparently non-coding regions, and a holin/muramidase lysis module. Many SP6-like phages, including PP99, share a unified two-component structure of a tail spike (Gebhart et al., 2017; Tu et al., 2017). The gene encoding an adaptor protein providing an attachment of the spike to the tail is separated from the gene encoding the spike in the genome. The C-terminal part of the tail spike protein encoded by PP99 (ORF 55), optimized for an attachment to the surface of the Pbr host, differs from those of other SP6-like phages. The highest (though moderate) sequence similarity is observed with the tail spike protein of phage PP1 infecting a similar pectobacterial host. Sequence analysis of PP99 ORF55 using HHPred predicts a structure resembling (E-value 3.3 e−19) the tail spike of Escherichia phage G7C (Podovididae, Gamaleyavirus) with the proposed polysaccharide-modifying enzyme activity (Prokhorov et al., 2017). However, according to the genome analysis, tail spikes of PP99 are not branched as in G7C, so we suggest that PP99 gp55 interacts with the single receptor of Pbr.
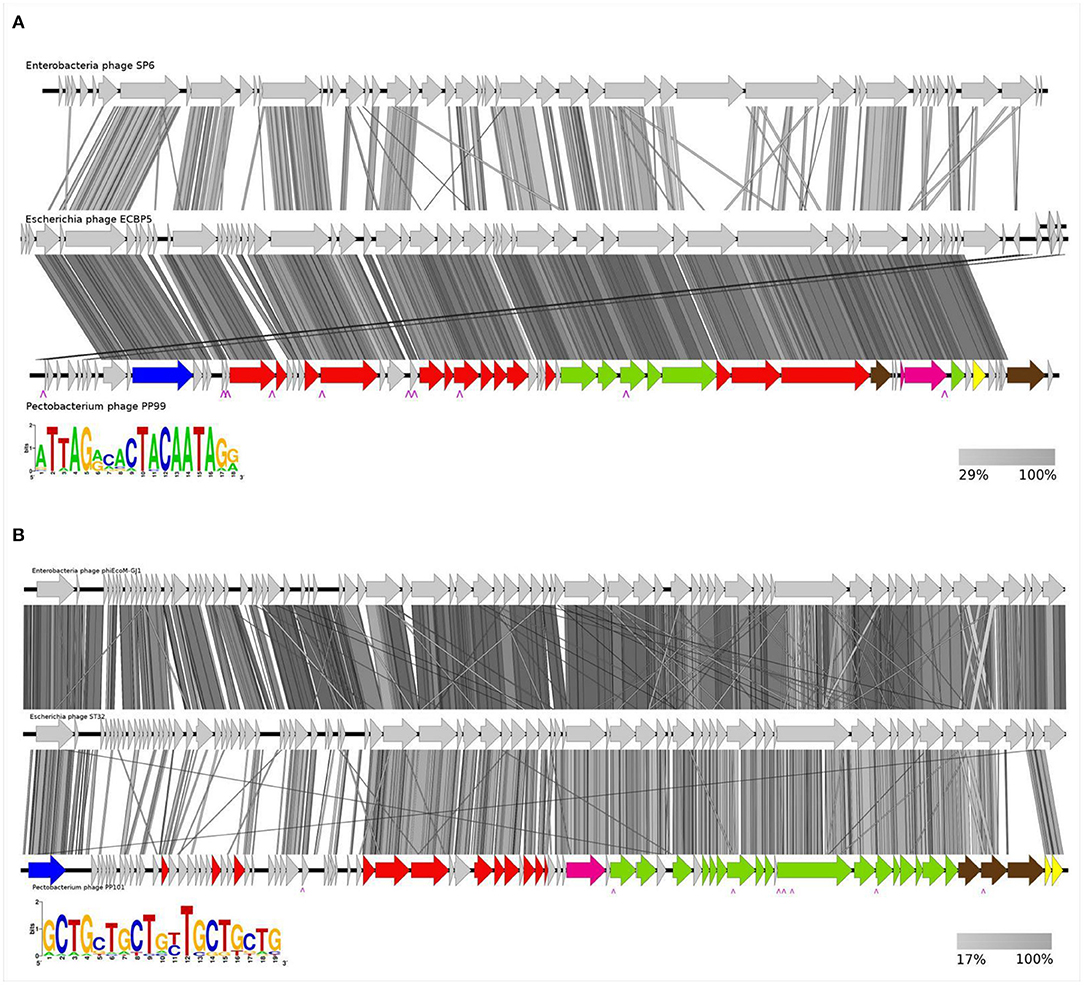
Figure 4. Genome map of bacteriophages. (A) PP99, and comparison with phages ECBP5 and SP6; (B) PP101, and comparison with PM1 and ST32. Arrows indicate predicted open reading frames presumably responsible for replication and metabolism (red), assembly and packaging (green). ORFs of discussed functions are marked blue (RNA polymerase), magenda (terminase), brown (tail spikes and fibers), and yellow (host lysis). Hypothetical proteins are indicated by tan arrows. The positions of predicted phage promotors are marked. The amino acid sequence similarities between the phages are indicated by gray shading (right insert). The left insert indicates the consensus sequence of the phage promoter.
Bacteriophage PP101 Genome Features
Phage PP101 (vB_PbrM-PP101) contains a dsDNA genome (read in 1,458× coverage) of 53,333 bp, with a GC content of 44.94%. We were unable to verify the exact location and size of terminal repeats in the PP101 genome experimentally. However, according to the annotations of similar phages, we consider it as terminally redundant linear with the headful DNA-packing mechanism (Born et al., 2011). Comparative analysis of complete genomes shows noticeable identity (above 80%) of PP101 with Pectobacterium carotovorum phage PM1 (NCBI accession number NC_023865) (Lim et al., 2014), Escherichia phages ST32 (MF044458) (Liu et al., 2018), phiEcoM-GJ1 (NC_010106) (Jamalludeen et al., 2008), Erwinia phages vB_EamM-Y2 (NC_019504) (Born et al., 2011), and Faunus (MH191398) (Table S3). This group of phages forms a separate phylogenetic clade not yet assigned as a taxonomic genus (Figure 4B, Figures S2, S3). Compared to these phages, the genome of phage PP101 has the same unidirectional transcription orientation, and a similar number of identified ORFs organized in functional clusters (Figure 4B). The functions of 36 ORFs could be predicted. Only six ORFs (07, 18, 20, 26, 35, 36), encoding small hypothetical proteins, are unique for PP101. The comprehensive genomic comparison of this group of lytic phages (with the emphasis to Escherichia phages) has been presented previously (Liu et al., 2018). We would like to outline a few features of this group of Enterobacterial phages, including PP101, that are essential for the realization of the inflectional cycle. First, all phages of this group possess the early gene encoding a single-subunit RNA polymerase, similar to T7-like Autographivirinae Podoviruses, and a similar arrangement of transcription-related ORFs. Therefore, we were able to hypothesize the existence of eight consensus promotor sequences throughout the genome that regulates the transcription of gene cascades and key structural genes. The sequence and the proposed positions of phage RNA-polymerase-specific promotors are shown in Figure 4B, and this arrangement provides effective multiplication of the phage in a broad range of conditions and host strains. Second, all the phages of this group contain the two-gene lysis module “holin-SAR endolysin” (ORF 80 and 81 in case of PP101) (Table S3). The third feature is the complex composition of the adsorption apparatus. Three proteins are predicted to form tail fibers of PP101, and these proteins have orthologs in other phages of the group (Table 1). We suggest the presence of the multicomponent tail fibers resembling those of phage T4 (Hyman and van Raaij, 2018) in PP101. PP101 ORF 77 and ORF78 have a high degree of identity with corresponding ORFs of PM1/ST32/phiEcoM-GJ1/vB_EamM-Y2/Faunus, presumably forming the proximal part of the fiber and the knee-knob. A high-resolution EM image of the Erwinia phage vB_EamM-Y2 (Born et al., 2011) reveals bicomponent fibers with a globular junction. This observation supports our hypothesis about the structure of PP101 tail fibers involving distal and proximal fibrous parts, and the globular intermediate. HHPred analysis of PP101gp78 shows a number of structural homologs with a β-structural domain responsible for carbohydrate-binding activity (5HON, E-value 9.1e-10; 4XJW, 3.9e-9). Being a part of the fiber, this protein may provide irreversible binding to the carbohydrates of the cell surface. The third putative tail fiber component, ORF79, has no homology with any protein of the above phages, except for PM1 infecting P. carotovorum. However, genes encoding putative prophage proteins with distantly close (30–40% identity) sequences can be found in the genomes of Pectobacterium spp. Therefore, we could propose that the receptor recognized by a similar protein is conservative in Pectobacteria, and it was used for host attachment by various phages in an evolutionally long period.
Composition of F152 O-Polysaccharide
Sugar analysis of F152 O-polysaccharide (OPS) revealed 6-deoxytalose (6dTalp), mannose, and galactose in the ratios 1–2.2: 1.3 (GLC detector response), respectively. The 1H NMR and 13C NMR (Figure 5, middle) spectra showed significant structural heterogeneity of the OPS due to non-stoichiometric O-acetylation (there were multiple signals for O-acetyl groups at δH 2.10–2.23 and δC 21.5–21.8). The OPS was O-deacetylated with aqueous ammonia to give a regular polymer (DPS). Its 13C NMR spectrum (Figure 5, top) contained, inter alia, signals for four anomeric carbons at δ 95.9–103.7, one CH3-C group at δ 16.6 (C-6 of 6dTalp), and three HOCH2-C groups at δ 61.6–62.9 (C-6 of Man and Gal). Accordingly, the 1H NMR spectrum of the DPS displayed signals for four anomeric protons at δ 4.44–5.27 and one CH3-C group at δ 1.26 (3H, d, J5, 6 6.3, H-6 of 6dTal). These data demonstrated that DPS has a tetrasaccharide repeating unit, containing two residues of D-Man and one residue each of D-Gal and L-6dTal.
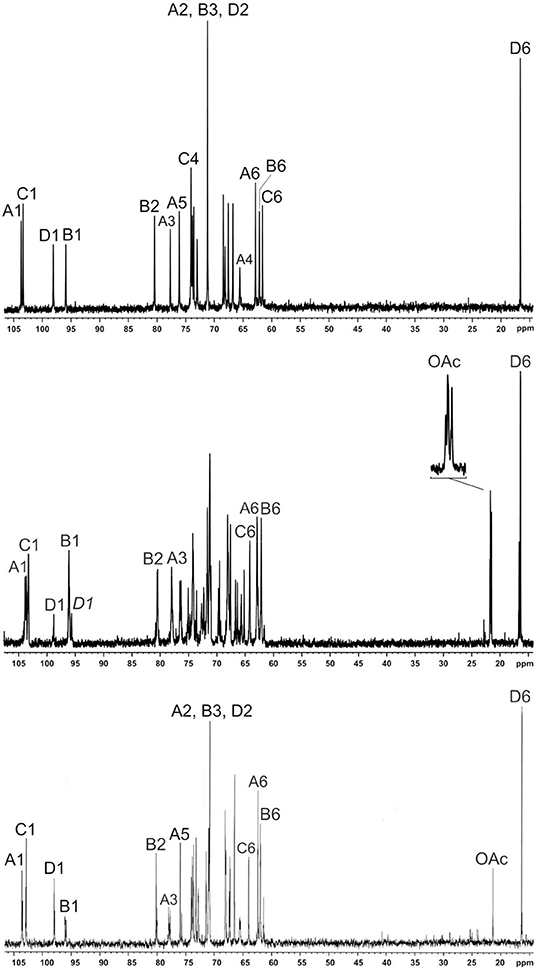
Figure 5. 13C NMR spectra of F152 O-polysaccharide (middle), O-deacetylated polysaccharide (top), and polysaccharide modified by phage PP99gp55 tail spike protein (bottom). The region for the CO groups is not shown. Numbers refer to carbons in sugar residue denoted by letters, as indicated in Figure 7. D1 and D1 indicate the 6dTal residues that do and do not include the 2-O-acetyl group, respectively.
The D configuration of Man and Gal and the L configuration 6dTalp were established by known regularities in glycosylation effects on 13C NMR chemical shifts (Shashkov et al., 1988), combined with calculation of the specific optical rotation of the DPS by Klyne's rule (αcalc = 18.9°, αexp = 15.6°).
Linkage and Sequence Analyses
The 1H NMR and 13C NMR spectra of the DPS were assigned (Table S4) using one-dimensional 1H,1H TOCSY, two-dimensional 1H,1H COSY, TOCSY, ROESY, 1H,13C HSQC, HMBC, HSQC-NOESY, and HSQC-TOCSY experiments. The configurations of the glycosidic linkages were established by 13C NMR chemical shifts of C-5, compared with published data of the corresponding α- and β-pyranosides (Lipkind et al., 1988; Knirel et al., 1992). The β configuration of the Gal residue (unit A) was confirmed by a relatively large coupling constant J1, 2 7.3 Hz and H-1/H-3 and H-1/H-5 correlations in the 1H,1H ROESY spectrum of the DPS. The α configuration of the Man and 6dTal residues (units B–D) was confirmed by H-1/H-2 correlations in the 1H,1H ROESY spectrum, with no H-1/H-3 and H-1/H-5 correlations.
The two-dimensional 1H,1H ROESY spectrum showed inter-residue correlations between the following anomeric protons and protons at the linkage carbons: Gal A H-1/Man B H-4, Man C H-1/Man B H-2, Man B H-1/Gal A H-3, and 6dTal D H-1/Man C H-3 at δ 4.44/3.94, 5.08/4.04, 5.27/3.75, and 5.07/4.02, respectively. These findings were supported by the 1H,13C HMBC and HSQC-NOESY experiments.
The glycosylation pattern of the monosaccharides was confirmed by low-field positions of the linkage carbons C-3 of unit A, C-2 of unit B, and C-3 and C-4 of unit C at δ 77.7, 80.4, 73.1, and 74.1 in the 13C NMR spectrum of the DPS, as compared with their positions at δ 74.1, 72.0, 71.5, and 68.2 in the corresponding non-substituted monosaccharides (Lipkind et al., 1988). The 1H and 13C NMR chemical shifts of 6dTalp were essentially identical to those for the O-polysaccharide of Aeromonas hydrophila O:34, in which this monosaccharide terminated a side chain (Knirel et al., 2002).
Based on the data obtained, we conclude that the DPS has the structure shown in Figure 6, middle.
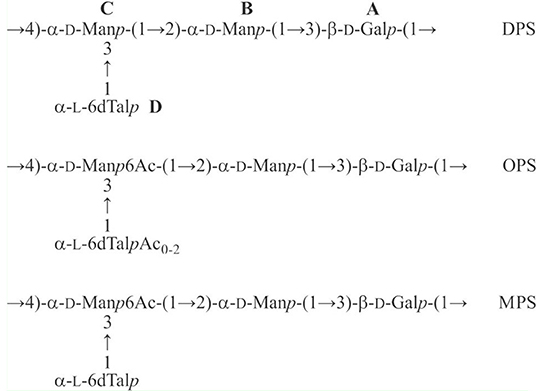
Figure 6. Structures of the O-polysaccharide (OPS), O-deacetylated polysaccharide (DPS), and modified polysaccharide (MPS) of Pectobacterium brasiliense strain F152.
O-Acetylation Pattern of the O-Polysaccharide
A comparison of the 1D and 2D NMR spectra of the initial OPS and DPS showed that the signals for units A and B have almost the same 1H and 13C NMR chemical shifts in both samples, and hence, they are not O-acetylated in the OPS. In the 1H, 13C HSQC spectrum of the OPS, a major part of the H-6/C-6 cross-peak of D-Man C shifted downfield to δH/δC 4.35, 4.53/64.2 from its position at δH/δC 3.81, 3.96/61.6 in the spectrum of DPS. Accordingly, the signal for C-5 of D-Man C shifted upfield from δ 73.1 in the DPS to δ 71.9 in the OPS (a β-effect of O-acetylation). As judged by the ratio of integral intensities of the signals for the O-acetylated and non-acetylated forms of Man C, the degree of O-acetylation of this sugar residue at position 6 is ~75%.
The 6dTal residue showed multiple signals in the NMR spectra of the OPS, due to the presence of various O-acetylated forms. Particularly, in the 2D 1H, 1H COSY spectrum, there were seven H-5/H-6 cross-peaks for 6dTalp, which formed two series, 1 and 2 (Figure 7). Such an O-acetylation pattern is similar to that reported for the O-polysaccharide of Aeromonas hydrophila O:34 (Knirel et al., 2002). Series 1 of four cross-peaks contained the H-5/H-6 cross-peak for the non-acetylated form at δ 4.61/1.25 (in Figure 5, this cross-peak is indicated by an arrow; compare with the 6dTalp H-5/H-6 cross-peak at δ 4.62/1.26 in the COSY spectrum of the DPS). As the H-5 and H-6 chemical shift are influenced mostly by an acetyl group at O-4, the three other peaks of Series 1 were assigned to the O-acetylated forms that do not include the 4-O-acetyl group, namely to the 2-O-acetylated, 3-O-acetylated, and 2,3-di-O-acetylated forms (Knirel et al., 2002). Correspondingly, the three cross-peaks of series 2 were assigned to the 4-O-acetylated, 2,4-di-O-acetylated and 3,4-di-O-acetylated forms of 6dTal. Therefore, the O-polysaccharide of Pbr F152 has the structure shown in Figure 6, top.
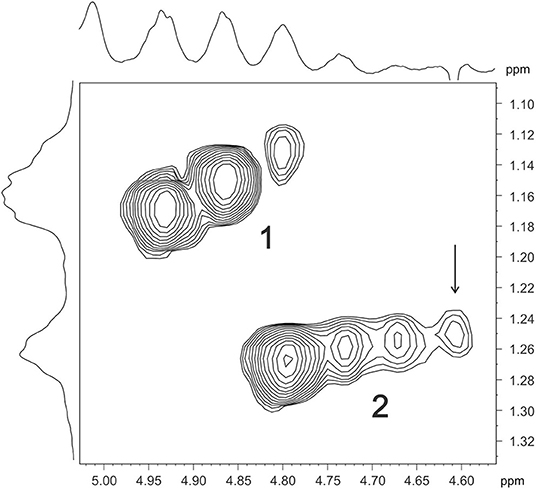
Figure 7. Part of a 600-MHz two-dimensional COSY spectrum of F152 O-polysaccharide, displaying 6dTal H-5/H-6 correlations. Two series of cross-peaks (1 and 2) were assigned to the O-acetylated forms of 6dTal that do and do not include the 4-O-acetyl group, respectively. Arrow points to the H-5/H-6 cross-peak of a non-acetylated 6dTal residue.
Properties of Recombinant PP99 Tail Spike Protein Gp55, and Modification of the O-Polysaccharide
Highly copious moieties such as cell surface polysaccharides, including the O-antigens of lipopolysaccharides (LPS), are good candidates for receptor interaction with phages (Steinbacher et al., 1996). Tail spikes contain processive enzymatic domains (Leiman and Molineux, 2008) that depolymerize (Barbirz et al., 2008) or deacetylate (Prokhorov et al., 2017) OPS to allow a phage to attach to the cell surface. Recombinant tail spike proteins of phages were shown to feature mostly uniform trimeric β-helical architecture (Fokine and Rossmann, 2014). The enzymatically active domains located either on the surface of the resulting trimeric prism, or within the secondary structure elements such as loops protruding from the prism (Leiman and Molineux, 2008). Recombinant PP99 gp55 tail spike protein forms trimeric complexes spontaneously (Figure S4), and is easily purified to homogeneous state. The protein tends to aggregate at high concentrations, so the structural research is hindered. In a dilute solution (<2 mg/mL), PP99 gp55 is stable for a few weeks at 4°C. Treatment of the O-polysaccharide with a PP99 tail spike protein resulted in a modified polysaccharide (MPS), which was studied by NMR spectroscopy, as described above for the initial polysaccharide (for the 13C NMR spectrum of the MPS, see Figure 5, bottom). The H-6/C-6 and H-5/C-5 cross-peaks of the 6-O-acetylated D-Man C residue in the 1H,13C HSQC spectrum of the MPS were found at the same positions as in the OPS. In contrast, signals of the variously O-acetylated L-6dTal D residues were absent from the MPS, and the cross-peaks of α-L-6dTal were at the same positions as in the DPS. Therefore, the MPS has the structure shown in Figure 6, bottom.
Discussion
Soft rot and black leg caused by Pectobacterium and Dickeya spp. are responsible for considerable damage to the potatoes, both during the vegetation season and post-harvest storage (Pérombelon, 2002). No effective control methods currently exist to combat these diseases. Considering the limitations in using pesticides and antibacterial compounds, especially post-harvest, there exists a noticeable demand for new safe and environmentally friendly alternative approaches to pathogen control. The use of bacteriophages to combat plant pathogenic bacteria is one of such promising methods. Phages naturally occur in the environment and are easily biodegradable, which make them suitable for organic agriculture (Frampton et al., 2012; Buttimer et al., 2017; Svircev et al., 2018). Successful applications of phages to protect potatoes from SRP are reported in simulated conditions (Czajkowski et al., 2017; Carstens et al., 2019) and field trials (Adriaenssens et al., 2012; Voronina et al., 2019a). However, these experiments employed a limited number of phages and/or model strains of pathogen initially known to be susceptible to the phage used. An implication of phage control to real horticulture needs to consider many additional factors, including the diversity of pathogens (Svircev et al., 2018).
In this paper we investigate two different phages, isolated using Pectobacterium brasiliense as a major host. Pbr has been shown to play an important role in potato soft rot pathogenesis in many countries worldwide in recent years. However, phages specifically infecting this bacterium are understudied, as are the details of their interaction with the host. Both studied phages, PP99 (vB_PbrP_PP99) and PP101 (vB_PbrM_PP101), are lytic phages suitable for phage control. These phages demonstrate different morphology, and can be phylogenetically assigned. PP99 belongs to the genus Zindervirus of the Autographivirinae subfamily, and PP101 is a member of a clade of unclassified Myoviridae phages infecting Enterobacteria (Jamalludeen et al., 2008; Lim et al., 2014; Liu et al., 2018) that can be potentially assigned as a new genus of bacteriophages (Table 1, Figure 8). It is worth noting that despite the morphologic difference of PP99 and PP101, their genomes share some features common for “T7-like phages”: unidirectional assignment of ORFs, clear distribution of ORFs to separate gene cascades, early position of the gene encoding the single-subunit RNA polymerase, and a similar arrangement of promotors presumably specific to this polymerase. Some of such features were noticed earlier for phages similar to PP101 (Jamalludeen et al., 2008; Liu et al., 2018). Some evolutionary relationship between phages PP99 and PP101 may be traced based on the phylogeny of DNA polymerase, the terminase large subunit, and, to a lesser extent, major capsid protein genes (Figure 8, Figures S2, S3).
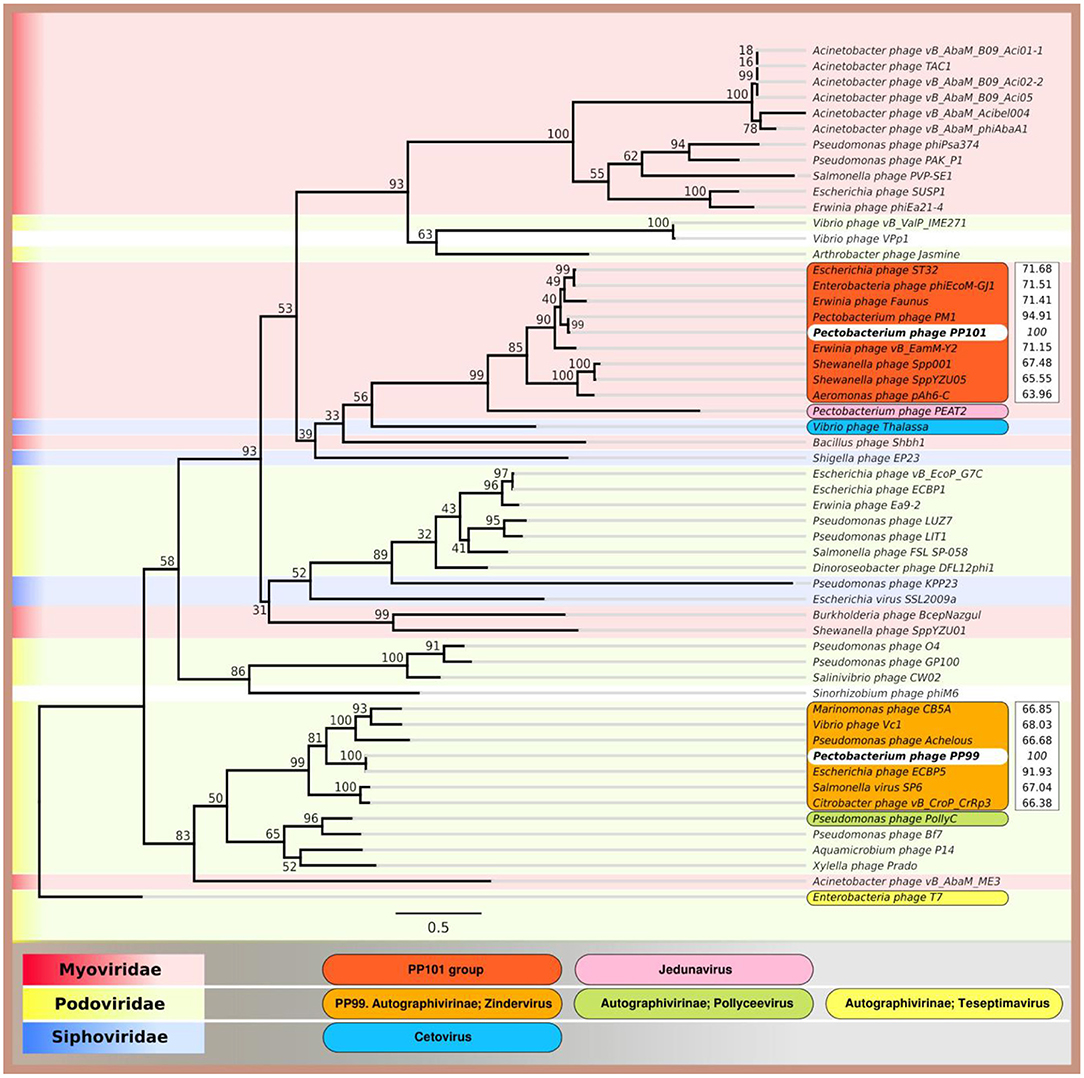
Figure 8. Phylogenetic tree of amino acid sequences of the major capsid proteins of Pectobacterium brasiliense phage PP99, Pectobacterium brasiliense phage PP101, and 53 phages using RAxML (GAMMA I BLOSSUM62 protein model with 1,000 bootstrap replicates), and ANI compared to PP99 and PP101 calculated with Jspecies.
Phages PP99 and PP101 demonstrate similar infection parameters with respect to their type host, Pbr strain F152 (PB29). Host range of tailed phages is largely defined by interaction of their baseplate structures (tail fibers and tail spikes) with molecules on the bacterial surface (Fokine and Rossmann, 2014), as earlier papers suggest. Many bacteriophages use tail spike/fiber proteins for primary contact with bacterial surface polysaccharides. A recognition mechanism involving lipopolysaccharides (LPS) and capsule polysaccharides was suggested as common for SPR phages (Evans et al., 2010) and was experimentally shown for Dickeya phage PP35 (Kabanova et al., 2019) and Pectobacterium carotovorum subsp. carotovorum phage POP72 (Kim et al., 2019). Therefore, our further investigation was directed to reveal the molecular details of phage-host interaction. We have determined the structure of O-polysaccharide of F152, which has no direct analogs in the bacterial world, especially in its acetylation pattern, with ~75% repeating units of D-mannose residues in the main chain O-acetylated at position 6, and ~90% side-chain 6-deoxy-L-talose residues is randomly mono- or di-O-acetylated at any position (Figure 6). This unique composition of F152 O-polysaccharide requires very specific arrangement of amino acid residues on the surface of the proteins of phage recognition apparatus to provide selective contact with the bacterial host. The adsorption system of phage PP101 involves three putative tail fiber proteins (ORF 77–79). This composition is conserved in all phages similar to PP101 (Table 1), and the product of ORF79 is believed to be responsible for the contact with the receptor. However, we were unable to obtain functionally active PP101gp79, therefore this hypothesis still requires experimental proof.
Unlike tail fibers, the structural architecture of phage tail spike proteins performing the contact with the receptor and often bearing the enzyme domain degrading or modifying bacterial polysaccharide is relatively unified (Leiman et al., 2007; Leiman and Molineux, 2008). The genome of phage PP99 contains the gene encoding such tail spike proteins. The recombinant product of this gene, PP99gp55, forms a trimeric biologically active protein that deacetylates the carbohydrate chain of Pbr OPS. The removal of the acetyl group occurs at the side chain of 6-deoxytalose residue, but the acetylated mannose residue of the main chain remains unchanged. We could suggest that the removal of the acetyl provides the spatial flexibility of sugar chains sufficient for the penetration of the tail spike protein to the cell surface. Similar dependence of phage specificity upon the modification pattern of bacterial OPS was previously observed for Escherichia phages (Bertozzi Silva et al., 2016; Letarov and Kulikov, 2017).
The knowledge of phage adsorption mechanism and cell receptors used in the course of phage infection is beneficial for the construction of phage cocktails to combat bacterial infections in medicine, agriculture, and the food industry. The use of phages employing different cell receptors of the same bacterial host usually expands an efficacy of antibacterial action, and reduces the possibility of the evolution of phage-resistant mutants. In the studied case, Pectobacterium brasiliense phages PP99 and PP101 provide no advantage for combined use, but either of them can be potentially included in phage preparations to control the soft rot of potatoes.
Data Availability Statement
The complete genome sequences of phages PP99 and PP101 have been deposited in the NCBI database under the GenBank accession numbers KY250034 and KY087898, correspondingly. Draft genome assembly of strain F152 (PB29) and related information can be found in the NCBI database under the GenBank Accession number PJDM00000000.1.
Author Contributions
MS, AI, and KAM designed the experiment. AL, MS, AMS, EB, AK, EO, SS, and ASS planned and performed experiments. AL, PE, KKM, ST, AI, YK, and KAM performed data analysis. AL, AI, and KAM wrote the manuscript.
Funding
This research was supported by a Russian Science foundation grant #16-16-00073.
Conflict of Interest
EB, AK, and AI are employed by Research Center “PhytoEngineering” Ltd.
The remaining authors declare that the research was conducted in the absence of any commercial or financial relationships that could be construed as a potential conflict of interest.
Supplementary Material
The Supplementary Material for this article can be found online at: https://www.frontiersin.org/articles/10.3389/fmicb.2019.03147/full#supplementary-material
References
Ackermann, H. W. (2009). Basic phage electron microscopy. Methods Mol. Biol. 501, 113–126. doi: 10.1007/978-1-60327-164-6_12
Adeolu, M., Alnajar, S., Naushad, S., and Gupta, R. S. (2016). Genome-based phylogeny and taxonomy of the ‘Enterobacteriales': proposal for Enterobacterales ord. nov. divided into the families Enterobacteriaceae, Erwiniaceae fam. nov., Pectobacteriaceae fam. nov., Yersiniaceae fam. nov., Hafniaceae fam. nov., Morganellaceae fam. nov., and Budviciaceae fam. nov. Int. J. Syst. Evol. Microbiol. 66, 5575–5599. doi: 10.1099/ijsem.0.001485
Adriaenssens, E. M., van Vaerenbergh, J., Vandenheuvel, D., Dunon, V., Ceyssens, P. J., de Proft, M., et al. (2012). T4-related bacteriophage LIMEstone isolates for the control of soft rot on potato caused by “Dickeya solani.” PLoS ONE 7:e33227. doi: 10.1371/journal.pone.0033227
Aziz, R. K., Bartels, D., Best, A. A., DeJongh, M., Disz, T., Edwards, R. A., et al. (2008). The RAST Server: rapid annotations using subsystems technology. BMC Genomics 9:75. doi: 10.1186/1471-2164-9-75
Bankevich, A., Nurk, S., Antipov, D., Gurevich, A. A., Dvorkin, M., Kulikov, A. S., et al. (2012). SPAdes: a new genome assembly algorithm and its applications to single-cell sequencing. J. Comput. Biol. 19, 455–477. doi: 10.1089/cmb.2012.0021
Barbirz, S., Müller, J. J., Uetrecht, C., Clark, A. J., Heinemann, U., and Seckler, R. (2008). Crystal structure of Escherichia coli phage HK620 tailspike: podoviral tailspike endoglycosidase modules are evolutionarily related. Mol. Microbiol. 69, 303–316. doi: 10.1111/j.1365-2958.2008.06311.x
Bertozzi Silva, J., Storms, Z., and Sauvageau, D. (2016). Host receptors for bacteriophage adsorption. FEMS Microbiol. Lett. 363:fmw002. doi: 10.1093/femsle/fnw002
Besemer, J., Lomsadze, A., and Borodovsky, M. (2001). GeneMarkS: a self-training method for prediction of gene starts in microbial genomes. Implications for finding sequence motifs in regulatory regions. Nucleic Acids Res. 29, 2607–2618. doi: 10.1093/nar/29.12.2607
Born, Y., Fieseler, L., Marazzi, J., Lurz, R., Duffy, B., and Loessner, M. J. (2011). Novel virulent and broad-host-range Erwinia amylovora bacteriophages reveal a high degree of mosaicism and a relationship to Enterobacteriaceae phages. Appl. Environ. Microbiol. 77, 5945–5954. doi: 10.1128/AEM.03022-10
Buttimer, C., McAuliffe, O., Ross, R. P., Hill, C., O'Mahony, J., and Coffey, A. (2017). Bacteriophages and bacterial plant diseases. Front. Microbiol. 8:34. doi: 10.3389/fmicb.2017.00034
Carstens, A. B., Djurhuus, A. M., Kot, W., and Hansen, L. H. (2019). A novel six-phage cocktail reduces Pectobacterium atrosepticum soft rot infection in potato tubers under simulated storage conditions. FEMS Microbiol. Lett. 366:fnz101. doi: 10.1093/femsle/fnz101
Clokie, M. R. J., and Kropinski, A. M. (2009). Bacteriophages: Methods and Protocols Volume 1: Isolation, Characterization, and Interactions, Vol. 502. New York, NY: Humana Press.
Czajkowski, R., Ozymko, Z., de Jager, V., Siwinska, J., Smolarska, A., Ossowicki, A., et al. (2015). Genomic, proteomic and morphological characterization of two novel broad host lytic bacteriophages ΦPD10.3 and ΦPD23.1 infecting pectinolytic Pectobacterium spp. and Dickeya spp. PLoS ONE 10:e0119812. doi: 10.1371/journal.pone.0119812
Czajkowski, R., Smolarska, A., and Ozymko, Z. (2017). The viability of lytic bacteriophage ΦD5 in potato-associated environments and its effect on Dickeya solani in potato (Solanum tuberosum L.) plants. PLoS ONE 12:e0183200. doi: 10.1371/journal.pone.0183200
Dees, M. W., Lysøe, E., Rossmann, S., Perminow, J., and Brurberg, M. B. (2017). Pectobacterium polaris sp. nov., isolated from potato (Solanum tuberosum). Int. J. Syst. Evol. Microbiol. 67, 5222–5229. doi: 10.1099/ijsem.0.002448
Delcher, A. L., Harmon, D., Kasif, S., White, O., and Salzberg, S. L. (1999). Improved microbial gene identification with GLIMMER. Nucleic Acids Res. 27, 4636–4641. doi: 10.1093/nar/27.23.4636
Dobbins, A. T., George, M., Basham, D. A., Ford, M. E., Houtz, J. M., Pedulla, M. L., et al. (2004). Complete genomic sequence of the virulent salmonella bacteriophage SP6. J. Bacteriol. 186, 1933–1944. doi: 10.1128/jb.186.7.1933-1944.2004
Duarte, V., De Boer, S. H., Ward, L. J., and De Oliveira, A. M. R. (2004). Characterization of atypical Erwinia carotovora strains causing blackleg of potato in Brazil. J. Appl. Microbiol. 96, 535–545. doi: 10.1111/j.1365-2672.2004.02173.x
Evans, T. J., Ind, A., Komitopoulou, E., and Salmond, G. P. C. (2010). Phage-selected lipopolysaccharide mutants of Pectobacterium atrosepticum exhibit different impacts on virulence. J. Appl. Microbiol. 109, 505–514. doi: 10.1111/j.1365-2672.2010.04669.x
Fokine, A., and Rossmann, M. G. (2014). Molecular architecture of tailed double-stranded DNA phages. Bacteriophage 4:e28281. doi: 10.4161/bact.28281
Frampton, R. A., Pitman, A. R., and Fineran, P. C. (2012). Advances in bacteriophage-mediated control of plant pathogens. Int. J. Microbiol. 2012, 326452. doi: 10.1155/2012/326452
Gebhart, D., Williams, S. R., and Scholl, D. (2017). Bacteriophage SP6 encodes a second tailspike protein that recognizes Salmonella enterica serogroups C2 and C3. Virology. 507, 233–236. doi: 10.1016/j.virol.2017.02.025
Gupta, K., Lee, Y., and Yin, J. (1995). Extremo-phage: in vitro selection of tolerance to a hostile environment. J. Mol. Evol. 41, 113–114. doi: 10.1007/BF00170661
Hyatt, D., Chen, G. L., Locascio, P. F., Land, M. L., Larimer, F. W., and Hauser, L. J. (2010). Prodigal: prokaryotic gene recognition and translation initiation site identification. BMC Bioinformatics 11:119. doi: 10.1186/1471-2105-11-119
Hyman, P., and van Raaij, M. (2018). Bacteriophage T4 long tail fiber domains. Biophys. Rev. 10, 463–471. doi: 10.1007/s12551-017-0348-5
Jamalludeen, N., Kropinski, A. M., Johnson, R. P., Lingohr, E., Harel, J., and Gyles, C. L. (2008). Complete genomic sequence of bacteriophage phiEcoM-GJ1, a novel phage that has myovirus morphology and a podovirus-like RNA polymerase. Appl. Environ. Microbiol. 74, 516–525. doi: 10.1128/AEM.00990-07
Kabanova, A. P., Shneider, M. M., Korzhenkov, A. A., Bugaeva, E. N., Miroshnikov, K. K., Zdorovenko, E. L., et al. (2019). Host specificity of the Dickeya bacteriophage PP35 is directed by a tail spike interaction with bacterial O-antigen, enabling the infection of alternative non-pathogenic bacterial host. Front. Microbiol. 9:3288. doi: 10.3389/fmicb.2018.03288
Kim, H., Kim, M., Bai, J., Lim, J. A., Heu, S., and Ryu, S. (2019). Colanic acid is a novel phage receptor of Pectobacterium carotovorum subsp. carotovorum Phage POP72. Front. Microbiol. 10:143. doi: 10.3389/fmicb.2019.00143
Klucar, L., Stano, M., and Hajduk, M. (2009). PhiSITE: database of gene regulation in bacteriophages. Nucleic Acids Res. 38, D366–D370. doi: 10.1093/nar/gkp911
Knirel, Y. A., Paramonov, N. A., Shashkov, A. S., Kochetkov, N. K., Yarullin, R. G., Farber, S. M., et al. (1992). Structure of the polysaccharide chains of Pseudomonas pseudomallei lipopolysaccharides. Carbohydr. Res. 233, 185–193. doi: 10.1016/S0008-6215(00)90930-3
Knirel, Y. A., Shashkov, A. S., Senchenkova, S. N., Merino, S., and Tomás, J. M. (2002). Structure of the O-polysaccharide of Aeromonas hydrophila O:34; a case of random O-acetylation of 6-deoxy-L-talose. Carbohydr. Res. 337, 1381–1386. doi: 10.1016/s0008-6215(02)00136-2
Konstantinidis, K. T., and Tiedje, J. M. (2005). Genomic insights that advance the species definition for prokaryotes. Proc. Natl. Acad. Sci. U.S.A. 102, 2567–2572. doi: 10.1073/pnas.0409727102
Kropinski, A. M., Prangishvili, D., and Lavigne, R. (2009). Position paper: the creation of a rational scheme for the nomenclature of viruses of Bacteria and Archaea. Environ. Microbiol. 11, 2775–2777. doi: 10.1111/j.1462-2920.2009.01970.x
Laslett, D., and Canback, B. (2004). ARAGORN, a program to detect tRNA genes and tmRNA genes in nucleotide sequences. Nucleic Acids Res. 32, 11–16. doi: 10.1093/nar/gkh152
Lavigne, R., Sun, W. D., and Volckaert, G. (2004). PHIRE, a deterministic approach to reveal regulatory elements in bacteriophage genomes. Bioinformatics 20, 629–635. doi: 10.1093/bioinformatics/btg456
Lee, J. H., Shin, H., Ji, S., Malhotra, S., Kumar, M., Ryu, S., et al. (2012). Complete genome sequence of phytopathogenic Pectobacterium carotovorum subsp. carotovorum bacteriophage PP1. J. Virol. 86, 8899–8900. doi: 10.1128/JVI.01283-12
Lee, J. S., Jang, H. B., Kim, K. S., Kim, T. H., Im, S. P., Kim, S. W., et al. (2015). Complete genomic and lysis-cassette characterization of the novel phage, KBNP1315, which infects Avian Pathogenic Escherichia coli (APEC). PLoS ONE 10:e0142504. doi: 10.1371/journal.pone.0142504
Leiman, P. G., Battisti, A. J., Bowman, V. D., Stummeyer, K., Mühlenhoff, M., Gerardy-Schahn, R., et al. (2007). The structures of bacteriophages K1E and K1-5 explain processive degradation of polysaccharide capsules and evolution of new host specificities. J. Mol. Biol. 371, 836–849. doi: 10.1016/j.jmb.2007.05.083
Leiman, P. G., and Molineux, I. J. (2008). Evolution of a new enzyme activity from the same motif fold. Mol. Microbiol. 69, 287–290. doi: 10.1111/j.1365-2958.2008.06241.x
Letarov, A. V., and Kulikov, E. E. (2017). Adsorption of bacteriophages on bacterial cells. Biochemistry 82, 1632–1658. doi: 10.1134/S0006297917130053
Li, X., Ma, Y., Liang, S., Tian, Y., Yin, S., Xie, S., et al. (2018). Comparative genomics of 84 Pectobacterium genomes reveals the variations related to a pathogenic lifestyle. BMC Genomics 19:889. doi: 10.1186/s12864-018-5269-6
Lim, J.-A., Heu, S., Park, J., and Roh, E. (2017). Genomic characterization of bacteriophage vB_PcaP_PP2 infecting Pectobacterium carotovorum subsp. carotovorum, a new member of a proposed genus in the subfamily Autographivirinae. Arch. Virol. 162, 2441–2444. doi: 10.1007/s00705-017-3349-6
Lim, J.-A., Lee, D. H., and Heu, S. (2015). Isolation and genomic characterization of the T4-like bacteriophage PM2 infecting Pectobacterium carotovorum subsp. carotovorum. Plant Pathol. J. 31, 83–89. doi: 10.5423/PPJ.NT.09.2014.0099
Lim, J.-A., Shin, H., Lee, D. H., Han, S.-W., Lee, J.-H., Ryu, S., et al. (2014). Complete genome sequence of the Pectobacterium carotovorum subsp. carotovorum virulent bacteriophage PM1. Arch. Virol. 159, 2185–2187. doi: 10.1007/s00705-014-2005-7
Lim, J. A., Jee, S., Lee, D. H., Roh, E., Jung, K., Oh, C., et al. (2013). Biocontrol of Pectobacterium carotovorum subsp. carotovorum using bacteriophage PP1. J. Microbiol. Biotechnol. 23, 1147–1153. doi: 10.4014/jmb.1304.04001
Lipkind, G. M., Shashkov, A. S., Knirel, Y. A., Vinogradov, E. V., and Kochetkov, N. K. (1988). A computer-assisted structural analysis of regular polysaccharides on the basis of 13C-n.m.r. data. Carbohydr. Res. 175, 59–75. doi: 10.1016/0008-6215(88)80156-3
Liu, H., Geagea, H., Rousseau, G., Labrie, S., Tremblay, D., Liu, X., et al. (2018). Characterization of the Escherichia coli virulent myophage ST32. Viruses 10:E616. doi: 10.3390/v10110616
Liu, W., Lin, Y. R., Lu, M. W., Sung, P. J., Wang, W. H., and Lin, C. S. (2014). Genome sequences characterizing five mutations in RNA polymerase and major capsid of phages ϕA318 and ϕAs51 of Vibrio alginolyticus with different burst efficiencies. BMC Genomics 15:505. doi: 10.1186/1471-2164-15-505
Ma, B., Hibbing, M. E., Kim, H.-S., Reedy, R. M., Yedidia, I., Breuer, J. J., et al. (2007). Host range and molecular phylogenies of the soft rot enterobacterial genera Pectobacterium and Dickeya. Phytopathology 97, 1150–1163. doi: 10.1094/PHYTO-97-9-1150
Malko, A., Frantsuzov, P., Nikitin, M., Statsyuk, N., Dzhavakhiya, V., Golikov, A., et al. (2019). Potato pathogens in Russia's regions: an instrumental survey with the use of real-time PCR/RT-PCR in matrix format. Pathogens 8:E18. doi: 10.3390/pathogens8010018
Meier-Kolthoff, J. P., Auch, A. F., Klenk, H.-P. P., and Göker, M. (2013). Genome sequence-based species delimitation with confidence intervals and improved distance functions. BMC Bioinformatics 14:60. doi: 10.1186/1471-2105-14-60
Mitchell, A., Chang, H. Y., Daugherty, L., Fraser, M., Hunter, S., Lopez, R., et al. (2015). The InterPro protein families database: the classification resource after 15 years. Nucleic Acids Res. 43, D213–221. doi: 10.1093/nar/gku1243
Muturi, P., Yu, J., Maina, A. N., Kariuki, S., Mwaura, F. B., and Wei, H. (2019). Bacteriophages isolated in China for the control of Pectobacterium carotovorum causing potato soft rot in Kenya. Virol. Sin. 34, 287–294. doi: 10.1007/s12250-019-00091-7
Na, S. I., Kim, Y. O., Yoon, S. H., Ha, S-m, Baek, I., and Chun, J. (2018). UBCG: Up-to-date bacterial core gene set and pipeline for phylogenomic tree reconstruction. J. Microbiol. 56, 280–285. doi: 10.1007/s12275-018-8014-6
Nabhan, S., De Boer, S. H., Maiss, E., and Wydra, K. (2012). Taxonomic relatedness between Pectobacterium carotovorum subsp. carotovorum, Pectobacterium carotovorum subsp. Odoriferum and Pectobacterium carotovorum subsp. brasiliense subsp. nov. J. Appl. Microbiol. 113, 904–913. doi: 10.1111/j.1365-2672.2012.05383.x
Pédron, J., Bertrand, C., Taghouti, G., Portier, P., and Barny, M.-A. (2019). Pectobacterium aquaticum sp. nov., isolated from waterways. Int. J. Syst. Evol. Microbiol. 69, 745–751. doi: 10.1099/ijsem.0.003229
Pérombelon, M. C. M. (2002). Potato diseases caused by soft rot erwinias: an overview of pathogenesis. Plant Pathol. 51, 1–12. doi: 10.1046/j.0032-0862.2001.Short~title.doc.x
Portier, P., Pédron, J., Taghouti, G., Fischer-Le Saux, M., Caullireau, E., Bertrand, C., et al. (2019). Elevation of Pectobacterium carotovorum subsp. odoriferum to species level as Pectobacterium odoriferum sp. nov., proposal of Pectobacterium brasiliense sp. nov. and Pectobacterium actinidiae sp. nov., emended description of Pectobacterium carotovorum and description of Pectobacterium versatile sp. nov., isolated from streams and symptoms on diverse plants. Int. J. Syst. Evol. Microbiol. 69, 3207–3216. doi: 10.1099/ijsem.0.003611
Prokhorov, N. S., Riccio, C., Zdorovenko, E. L., Shneider, M. M., Browning, C., Knirel, Y. A., et al. (2017). Function of bacteriophage G7C esterase tailspike in host cell adsorption. Mol. Microbiol. 105, 385–398. doi: 10.1111/mmi.13710
Richter, M., and Rossello-Mora, R. (2009). Shifting the genomic gold standard for the prokaryotic species definition. Proc. Natl. Acad. Sci. U.S.A. 106, 19126–19131. doi: 10.1073/pnas.0906412106
Sawabdekeb, J. S., Sloneker, J. H., and Jeanes, A. (1965). Quantitative determination of monosaccharides as their alditol acetates by gas liquid chromatography. Anal. Chem. 37, 1602–1604. doi: 10.1021/ac60231a048
Schattner, P., Brooks, A. N., and Lowe, T. M. (2005). The tRNAscan-SE, snoscan and snoGPS web servers for the detection of tRNAs and snoRNAs. Nucleic Acids Res. 33, W686–689. doi: 10.1093/nar/gki366
Schmerer, M., Molineux, I. J., and Bull, J. J. (2014). Synergy as a rationale for phage therapy using phage cocktails. PeerJ 2:e590. doi: 10.7717/peerj.590
Shashkov, A. S., Lipkind, G. M., Knirel, Y. A., and Kochetkov, N. K. (1988). Stereochemical factors determining the effects of glycosylation on the 13C chemical shifts in carbohydrates. Magn. Reson. Chem. 26, 735–747. doi: 10.1002/mrc.1260260904
Shirshikov, F. V., Korzhenkov, A. A., Miroshnikov, K. K., Kabanova, A. P., Barannik, A. P., Ignatov, A. N., et al. (2018). Draft genome sequences of new genomospecies “Candidatus Pectobacterium maceratum” strains, which cause soft rot in plants. Genome Announc. 6:e00260-18. doi: 10.1128/genomeA.00260-18
Söding, J., Biegert, A., and Lupas, A. N. (2005). The HHpred interactive server for protein homology detection and structure prediction. Nucleic Acids Res. 33, W244–248. doi: 10.1093/nar/gki408
Stamatakis, A. (2014). RAxML version 8: A tool for phylogenetic analysis and post-analysis of large phylogenies. Bioinformatics 30, 1312–1313. doi: 10.1093/bioinformatics/btu033
Steinbacher, S., Baxa, U., Miller, S., Weintraub, A., Seckler, R., and Huber, R. (1996). Crystal structure of phage P22 tailspike protein complexed with Salmonella sp. O-antigen receptors. Proc. Natl. Acad. Sci U.S.A. 93, 10584–10588. doi: 10.1073/pnas.93.20.10584
Svircev, A., Roach, D., and Castle, A. (2018). Framing the future with bacteriophages in agriculture. Viruses 10:E218. doi: 10.3390/v10050218
Taylor, N. M. I., Prokhorov, N. S., Guerrero-Ferreira, R. C., Shneider, M. M., Browning, C., Goldie, K. N., et al. (2016). Structure of the T4 baseplate and its function in triggering sheath contraction. Nature 533, 346–352. doi: 10.1038/nature17971
Tu, J., Park, T., Morado, D. R., Hughes, K. T., Molineux, I. J., and Liu, J. (2017). Dual host specificity of phage SP6 is facilitated by tailspike rotation. Virology 507, 206–215. doi: 10.1016/j.virol.2017.04.017
Voronina, M. V., Bugaeva, E. N., Vasiliev, D. N., Kabanova, A. P., Barannik, A. P., Shneider, M. M., et al. (2019a). Characterization of Pectobacterium carotovorum subsp. carotovorum bacteriophage PP16 prospective for biocontrol of potato soft rot. Microbiology. 13, 458–469. doi: 10.1134/S0026261719040118
Voronina, M. V., Kabanova, A. P., Shneider, M. M., Korzhenkov, A. A., Toschakov, S. V., Miroshnikov, K. K., et al. (2019b). First report of Pectobacterium carotovorum subsp. brasiliense causing blackleg and stem rot disease of potato in Russia. Plant Dis. 103, 364–364. doi: 10.1094/PDIS-03-18-0456-PDN
Waleron, M., Misztak, A., Waleron, M., Franczuk, M., Wielgomas, B., and Waleron, K. (2018). Transfer of Pectobacterium carotovorum subsp. carotovorum strains isolated from potatoes grown at high altitudes to Pectobacterium peruviense sp. nov. Syst. Appl. Microbiol. 41, 85–93. doi: 10.1016/j.syapm.2017.11.005
Westphal, O., and Jann, K. (1965). Bacterial lipopolysaccharides extraction with phenol-water and further applications of procedure. Methods Carbohydr. Chem. 5, 83–91.
Zhang, Y., Fan, Q., and Loria, R. (2016). A re-evaluation of the taxonomy of phytopathogenic genera Dickeya and Pectobacterium using whole-genome sequencing data. Syst. Appl. Microbiol. 39, 252–259. doi: 10.1016/j.syapm.2016.04.001
Zych, K., Toukach, F. V., Arbatsky, N. P., Kołodziejska, K., Senchenkova, S. N., Shashkov, A. S., et al. (2001). Structure of the O-specific polysaccharide of proteus mirabilis D52 and typing of this strain to Proteus serogroup O33. Eur. J. Biochem. 268, 4346–4351. doi: 10.1046/j.1432-1327.2001.02356.x
Keywords: bacteriophage, Pectobacterium brasiliense, lipopolysaccharide, O-specific polysaccharide, 6-Deoxy-l-talose, random sugar O-acetylation, tail spike, deacetylase
Citation: Lukianova AA, Shneider MM, Evseev PV, Shpirt AM, Bugaeva EN, Kabanova AP, Obraztsova EA, Miroshnikov KK, Senchenkova SN, Shashkov AS, Toschakov SV, Knirel YA, Ignatov AN and Miroshnikov KA (2020) Morphologically Different Pectobacterium brasiliense Bacteriophages PP99 and PP101: Deacetylation of O-Polysaccharide by the Tail Spike Protein of Phage PP99 Accompanies the Infection. Front. Microbiol. 10:3147. doi: 10.3389/fmicb.2019.03147
Received: 30 September 2019; Accepted: 29 December 2019;
Published: 23 January 2020.
Edited by:
Robert Czajkowski, University of Gdansk, PolandReviewed by:
Grazyna Majkowska-Skrobek, University of Wrocław, PolandJens Andre Hammerl, Federal Institute for Risk Assessment (BfR), Germany
Shuguang Lu, Third Military Medical University, China
Copyright © 2020 Lukianova, Shneider, Evseev, Shpirt, Bugaeva, Kabanova, Obraztsova, Miroshnikov, Senchenkova, Shashkov, Toschakov, Knirel, Ignatov and Miroshnikov. This is an open-access article distributed under the terms of the Creative Commons Attribution License (CC BY). The use, distribution or reproduction in other forums is permitted, provided the original author(s) and the copyright owner(s) are credited and that the original publication in this journal is cited, in accordance with accepted academic practice. No use, distribution or reproduction is permitted which does not comply with these terms.
*Correspondence: Konstantin A. Miroshnikov, a21pQGliY2gucnU=