- 1Laboratorio de Referencia de E. coli (LREC), Departamento de Microbiología y Parasitología, Facultad de Veterinaria, Universidad de Santiago de Compostela, Lugo, Spain
- 2Instituto de Investigación Sanitaria de Santiago de Compostela (IDIS), Santiago, Spain
- 3Plataforma de Genómica y Bioinformática, Centro de Investigación Biomédica de La Rioja (CBIR), Logroño, Spain
Porcine Escherichia coli ST131 isolates are scarcely documented. Here, whole genome sequencing and core genome (CG) and plasmidome analysis of seven isolates collected from diarrheic piglets and four from pork meat were performed. All of the 11 ST131 isolates belonged to serotype O25b:H4 and clade B and showed fimH22 allele or mutational derivatives. The 11 porcine isolates possessed virulence traits that classified the isolates as avian pathogenic, uropathogenic, and extraintestinal pathogenic E. coli–like (APEC-, UPEC-, and ExPEC-like) and constituted virotype D. The CG was performed for all porcine isolates in addition to 73 ST131 reference isolates from different origins. Within clade B, the CG showed nine subclusters, allowing us to describe five new subclades (B6, B6-like, B7, B8, and B9). There was an association between subclade B6, PST43, virotype D2, and food origin, whereas subclade B7 included PST9 isolates with virotype D5 from diarrheic piglets (p = 0.007). The distance between human and porcine isolates from subclades B6 and B7 had an average of 20 and 15 SNP/Mb, respectively. [F2:A-:B1]-IncF, ColE1-like, and IncX plasmids were the most prevalent. Besides, IncF plasmids harbored a ColV region frequent among APEC isolates. Antimicrobial resistance genes conferring resistance to penicillin, tetracycline, quinolones, and colistin were the most common. The mcr-1.1 gene was detected in 5 of 11 porcine isolates, integrated into the chromosome of one isolate and into plasmids in the remainder isolates (two MOBH11/IncHI2-ST4, one MOBP3/IncX4, and one MOBF12/IncF [F2:A-:B1] supposedly cointegrated with an IncHI2). The surrounding environments of the mcr-1 cassette showed variability. However, there were conserved structures within the same plasmid family. In conclusion, CG analysis defined five new subclades. The ST131 porcine isolates belonged to new subclades B6 and B7. Moreover, porcine and clinical human isolates were strongly related. The 11 porcine ST131 isolates harbored a wide variety of plasmids, virulence, and resistance genes. Furthermore, epidemic plasmids IncX4 and IncHI2 are responsible for the acquisition of mcr-1.1 gene. We hypothesize that the APEC-IncF plasmid acquired the mcr-1.1 gene via cointegrating an IncHI2 plasmid, which is worrying due to combination of virulence and resistance attributes in a single mobile genetic element.
Introduction
Escherichia coli exists as part of the commensal microbiota in the mammalian digestive tract, as well as a zoonotic pathogen responsible for intestinal and extraintestinal infections in both humans and animals (Poirel et al., 2018). Treatment of multidrug-resistant (MDR) E. coli infections has become a serious clinical issue, especially with the emergence of high-risk clones, such as clone ST131 (Nicolas-Chanoine et al., 2008, 2014).
ST131 clone is one of the most prevalent extraintestinal pathogenic E. coli clones (ExPEC). Although it remains unclear why this clone is prevailing over the others, antimicrobial resistance and virulence factors are suspected contributors (Dobrindt, 2005; Stoesser et al., 2016b). Even though ST131 clone is prevalent in human samples, it remains less frequent from animals and especially rare from porcine source (Reid et al., 2019). However, it has already been identified in many animal species, including in Antarctic pinnipeds (Mora et al., 2018). The majority of animal isolates belong to clade B (associated with fimH22), whereas in those that cause extraintestinal infections in humans predominate clade C (associated with fimH30) and especially those belonging to subclades C1-nM27, C1-M27, and C2 (H30Rx). This could be the reason of the underrepresentation of ST131 clade B isolates in the literature and sequence databases (Reid et al., 2019).
Worryingly, resistance to last-resort antibiotics such as carbapenems and polymyxins has already been reported in the pandemic E. coli clone ST131 (Trobos et al., 2009; Schink et al., 2013; Hasman et al., 2015; Ewers et al., 2016; Kuo et al., 2016; Sonnevend et al., 2016; de Toro et al., 2017; Ortiz de la Tabla et al., 2017; García-Meniño et al., 2018; Liu H. et al., 2018; Ellaby et al., 2019; Hojabri et al., 2019; Reid et al., 2019). Colistin (polymyxin E) is associated with nephrotoxicity and neurotoxicity. However, it has been widely used in veterinary medicine. Livestock, and particularly porcine farming, has been singled out as reservoir for colistin resistance (García-Meniño et al., 2019) and foodborne pathogens (FBPs) that could have devastating health and economic consequences (Sekse et al., 2017).
Intrinsic resistance to colistin has been related with two chromosomally encoded systems, the PhoPQ component and the pmrCAB operon (Olaitan et al., 2014; Poirel et al., 2018). However, the newly discovered plasmid-borne mobile colistin resistance (mcr) gene is responsible for a transferable mechanism of resistance (Liu Y.-Y. et al., 2016). Since the description of the mcr-1 gene by Liu Y.-Y. et al. (2016), several amino acid variants have been described, encoded in mcr-2 to mcr-9 genes (AbuOun et al., 2017; Borowiak et al., 2017; Carattoli et al., 2017; Yin et al., 2017; Wang et al., 2018; Yang et al., 2018; Carroll et al., 2019). Besides, several subvariants for some of them have been reported, that is, 13 nucleotide and protein variants in the mcr-1 family (designated mcr-1.1 to mcr-1.13) (Partridge et al., 2018).
Colistin plasmid-borne resistance has widely spread geographically (Kempf et al., 2016). It has been described in numerous genera of Enterobacteriaceae including Escherichia, Moraxella, Klebsiella, Salmonella, Enterobacter, Cronobacter, Shigella, Kluyvera, Citrobacter, and Raoultella (Campos et al., 2016; Liu B.-T. et al., 2016; Liu Y.-Y. et al., 2016; Olaitan et al., 2016; Pham Thanh et al., 2016; Stoesser et al., 2016a; Zeng et al., 2016; Zhao and Zong, 2016; AbuOun et al., 2017; Li et al., 2017c; Luo et al., 2017). Although mcr genes have been found in a large diversity of clones, Matamoros et al. (2017) and García-Meniño et al. (2019) established the ST10 and ST155 E. coli clones as potential reservoirs of the mcr-1 gene.
The mcr gene has been detected in many plasmid types, including IncI2, IncHI2, IncP, IncX4, IncY, IncF, and ColE10-like ones (Madec and Haenni, 2018) from different origins (Sun et al., 2018). Nevertheless, Matamoros et al. (2017) and García-Meniño et al. (2019) found that the majority of the mcr-carrier plasmids belonged mainly to four plasmid incompatibility groups: IncX4, IncI2, IncHI2, and ColE10-like. In contrast, it remains rare to find colistin resistance genes chromosomally encoded, in accordance to Li et al. (2018) studies, where the prevalence of chromosomal mcr carrier isolates was estimated in 4% of the analyzed isolates.
Broadly, the mcr-1 cassette is described as an approximately 2,600 base pair (bp) fragment containing the mcr-1 gene followed by a phosphoesterase (Poirel et al., 2016). It has been proposed that the ISApl1 insertion sequence (IS) mediates the transmission of mcr-1 by forming circular intermediates, which can translocate (Tegetmeyer et al., 2008; Chandler and Siguier, 2013; Snesrud et al., 2016; Zurfluh et al., 2016a, b; Li et al., 2017a).
Genomic tools allow a compressive characterization of FBPs and the identification of clonal groups of bacteria that represent public health hazards (Kovac et al., 2017). However, epidemiological surveillance of epidemic plasmids related to antimicrobial resistances or/and virulence genes spreading in the bacterial population is still complicated because of the intrinsic plasticity of plasmids (Orlek et al., 2017).
In this study, we have performed the whole genome sequencing (WGS) analysis of 11 ST131 E. coli isolates from porcine samples. Our objectives were (I) perform a core genome (CG) analysis to establish the phylogenetic relationship of our isolates within clade B of ST131 E. coli isolates, (II) to determine and describe the genetic location of the resistance and virulence genes, (III) to investigate the role of mobile genetic elements (MGEs) in the dissemination of those genes, and (IV) to explore the genetic environment of the mcr-1 gene. As far as we know, this study would be the first one in which the whole genome of an extended collection of ST131 clade B isolates from porcine origin, collected from diseased animals and food, has been performed.
Materials and Methods
Epidemiological Background of the E. coli Collection
In the present study, we performed WGS analysis of a collection of 11 resistant E. coli O25b:H4-B2-ST131 clade B isolates, seven from piglets with diarrhea and four from pork meat. The isolates belong to extensive epidemiological studies accomplished in Spain. The seven isolates from diarrheic piglets were isolated during period 2006–2016 (García-Meniño et al., 2018) and the four from pork meat during the years 2011 and 2012 (Herrera Estévez, 2015).
Antimicrobial Susceptibility Testing
Antimicrobial susceptibility was determined by minimal inhibitory concentrations by using the MicroScan WalkAway® -automated system (BECKMAN COULTER, Inc., Brea, CA, United States) according to the manufacturer’s instructions. The antibiotics tested were ticarcillin, aztreonam, ceftazidime, cefepime, ampicillin–sulbactam, piperacillin–tazobactam, imipenem, meropenem, amikacin, gentamicin, tobramycin, levofloxacin, ciprofloxacin, trimethoprim–sulfamethoxazole, fosfomycin, colistin, minocycline, and tigecycline. Additionally, resistance to ampicillin, cefotaxime, chloramphenicol, and nalidixic acid was determined by disk diffusion assays (Becton Dickinson, Sparks, MD, United States). All results were interpreted according to the CLSI guidelines (Wayne, 2017). Multidrug resistance status was attributed to those isolates resistant to at least one agent of three or more different antimicrobial categories, including resistance to β-lactamase inhibitors (Magiorakos et al., 2012).
Serotyping, Phylogenetic Grouping, Multilocus Sequence Typing, CH Typing, and Virulence Genotyping
ST131 isolates were characterized with regard to O:H serotypes, phylogenetic groups, clonotypes (fumC and fimH genes), sequence type by multilocus sequence typing (MLST) (according to the Achtman scheme and by the Pasteur Institute scheme), and 34 extraintestinal virulence-associated genes encoding adhesins (fimH, fimAvMT78, papAH, papC, papEF, papGII, papGIII, sfa/focDE, afa/draBC, yfcV), toxins (sat, cnf1, hlyA, hlyF, cdtB, tsh, vat), siderophores-iron uptake (iucD, iutA, iroN, fyuA, chuA), capsule (kpsM II, kpsM II-K2, kpsM II-K5, neuC-K1, kpsM III), and miscellaneous [cvaC, iss, traT, ibeA, malX (PAI), usp, ompT] as described previously (Clermont et al., 2013; Dahbi et al., 2014; Mamani et al., 2019). Based on the definitions given by Johnson et al. (2008, 2015) and Spurbeck et al. (2012), the isolates that genetically satisfied the following criteria (I) positive for ≥2 of 5 markers, including papAH and/or papC, sfa/focDE, afa/draBC, kpsM II, and iutA; (II) positive for three or more of four markers, including chuA, fyuA, vat, and yfcV; and (III) positive for ≥4 of 5 markers, including hlyF, iutA, iroN, iss, and ompT, were presumptively designated as ExPEC, uropathogenic E. coli (UPEC), and avian pathogenic E. coli (APEC)–like isolates, respectively, taking into account that their site of isolation (meat and feces) and animal species differ from the original definitions. The virotypes (A–F) of the ST131 isolates were established according to the scheme described by Dahbi et al. (2014). Primers used in this study for polymerase chain reaction (PCR) amplification of virulence genes are indicated in Supplementary Table S1.
WGS, Assembly, and Primary Analysis
Total DNA was extracted with the QIAmp DNA Mini Kit (Qiagen GmbH, Qiagen Strasse 1, Hilden, Germany). Libraries were prepared by using the TruSeq DNA PCR-Free protocol (Illumina, San Diego, California, United States) at the Genomics and Bioinformatics Core Facility (Centre for Biomedical Research of La Rioja). Paired-end 100-bp reads on fragments of 550-bp insert size were sequenced in an Illumina HiSeq 1500. Genomes were reconstructed by using PLACNETw (Vielva et al., 2017). Identification of Open Reading Frames (ORFs) and genome annotation of the assembled genetic elements was performed by using Prokka (Seemann, 2014). Genomes were in silico typed by the following databases: SerotypeFinder (Joensen et al., 2015), MLSTtyper (Larsen et al., 2012), and CHtyper (Camacho et al., 2009). Three different databases were used for the identification of antibiotic resistance genes: ResFinder (Zankari et al., 2012), CARD Resistance Gene Identifier (McArthur et al., 2013), and ARG-ANNOT (Antibiotic Resistance Gene-ANNOTation) (Gupta et al., 2014). Besides, the PointFinder database (Zankari et al., 2017) was used to determine point mutations. The VirulenceFinder (Ren et al., 2017) and the VFDB (Chen et al., 2005) databases were used to explore virulence factors.
The genetic environment of the mcr detected genes was manually revisited and compared to previously reported ones with CLC Sequence Viewer (version 8.9; Qiagen) and EasyFig tools (Sullivan et al., 2011).
CG and Phylogenetic Analysis
For phylogenetic analysis, we used the 11 genomes of the porcine E. coli sequenced in this study plus 73 full-genomes references from the ST131 clone retrieved from the Enterobase1 and the NCBI Bioproject Database.2 The CG was defined as the collection of genes present at least once in all the ST131 genomes analyzed, with more than 90% similarity and 90% coverage, as defined by Lanza et al. (2014). All 84 genomes used in this study and their accession number are available on Supplementary Table S2. The analysis included genomes representative of all clades and subclades described by Ben Zakour et al. (2016) and Matamoros et al. (2017).
Plasmid Analysis
Plasmid reconstruction from WGS data was performed by PLACNETw method (Vielva et al., 2017). In PLACNETw representation, most plasmids can be recognized by their replication initiation proteins (RIPs) and/or RELaxase proteins (REL), both of them considered as plasmid markers. Incompatibility groups (Inc) and pMLST subtypes were in silico determined with PlasmidFinder and pMLST (Carattoli et al., 2014), respectively, via the CGE online services.3 Besides, reconstructed plasmids were subtyped according to the exact relaxase family subtype by phylogenetic comparison with previously defined relaxases subfamilies (Alvarado et al., 2012). Reconstructed plasmids and references belonging to the same Inc groups were compared by using BRIG (Alikhan et al., 2011) and EasyFig tools (Sullivan et al., 2011). All reference plasmids used in this study were recovered from NCBI database4 (Supplementary Table S3).
Statistics
Normality test was performed (Shapiro–Wilk test). A non-parametric Mann–Whitney–Wilcoxon test on paired data was conducted. A significance level of 0.05 was used for all tests. All statistical analyses were carried out with XLSTAT software.5
Results
Pasteur Sequence Types and fimH Alleles
All of the 11 ST131 isolates belonged to serotype O25b:H4 and the phylogenetic group B2. The seven isolates from piglets with diarrhea showed the Pasteur sequence type (PST) 9, whereas the four isolates from pork meat showed PST43 (Table 1).
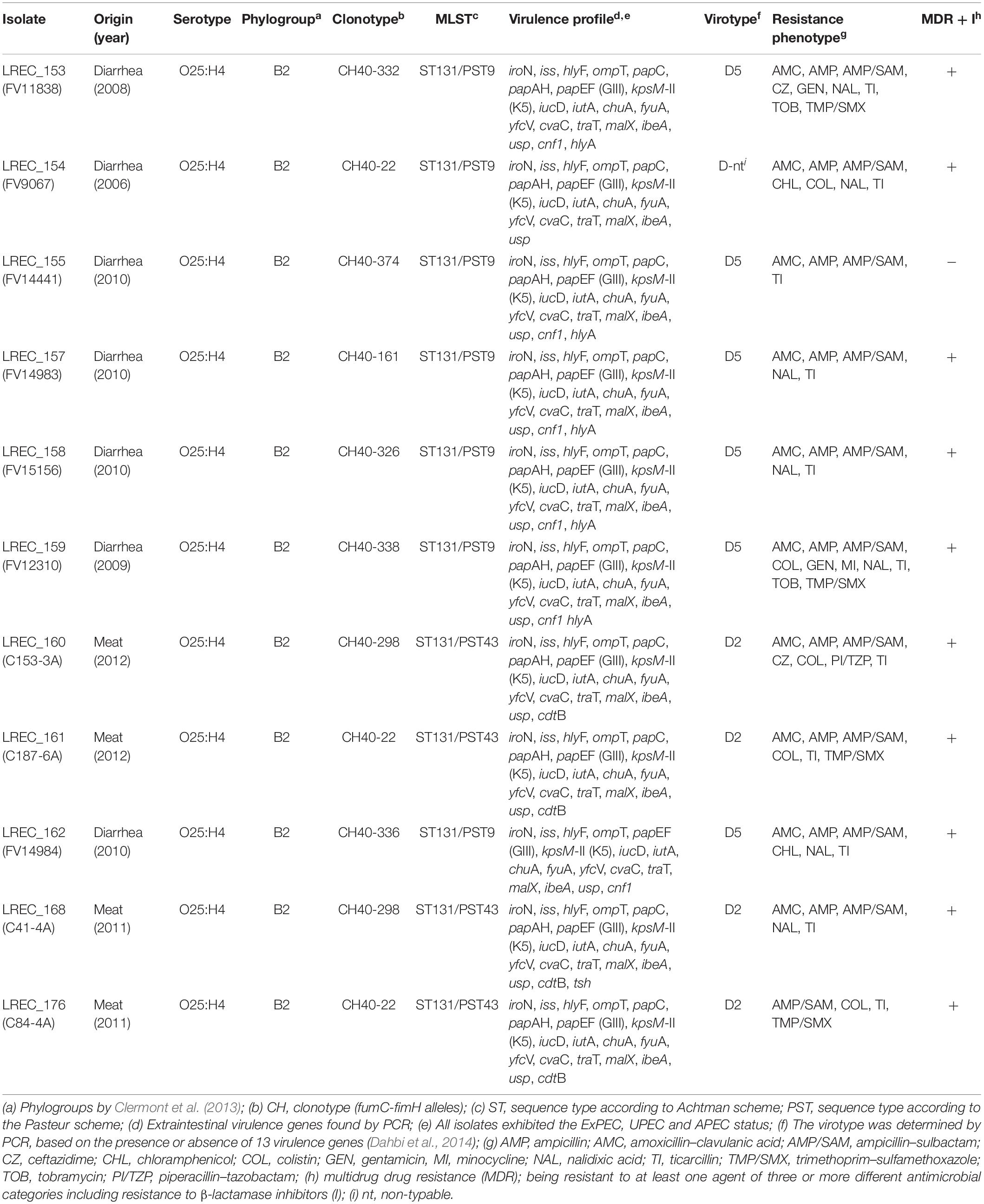
Table 1. Features, molecular typing, virulence, and resistance profiles of ST131 Escherichia coli isolates from porcine origin.
Also all of the 11 ST131 isolates belonged to clade B and showed the fimH22 allele or mutational derivatives of the fimH22 allele (fimH161, fimH298, fimH326, fimH332, fimH336, fimH338, and fimH374), resulting in clonotypes CH40-22 (3 isolates), CH40-161 (1), CH40-298 (2), CH40-326 (1), CH40-332 (1), CH40-336 (1), CH40-338 (1), and CH40-374 (1).
Virotypes
The 11 ST131 isolates harbored between 17 and 20 virulence gene markers screened by PCR. All isolates harbored the following genes: iroN, iss, hlyF, ompT, papEF, papG III, kpsM-II-K5, iucD, iutA, chuA, fyuA, yfcV, cvaC, traT, malX, ibeA, and usp. However, some genes were variables: papC and papAH (10 isolates), cnf1 (six isolates), hlyA (five isolates), and cdtB (four isolates). All isolates belonged to virotype D (Table 1). Six of seven isolates recovered from piglets with diarrhea with PST9 showed the virotype D5 (ibeA, papG III, cnf1, hlyA, and kpsM-II-K5), whereas the four PST43 isolates from meat showed the virotype D2 (ibeA, papG III, cdtB, and kpsM-II-K5). All the 11 porcine ST131 isolates exhibited a virulence profile that satisfied the APEC, UPEC, and ExPEC status and because of their origin were classified as APEC, UPEC, and ExPEC like isolates (Table 1 and Supplementary Table S1).
Resistance Phenotype
The resistance profile of the 11 ST131 porcine isolates was determined, showing that all but one exhibited an MDR phenotype. Amoxicillin/clavulanic acid resistance was present in 10 isolates, quinolone resistance in seven, colistin resistance in five isolates (with mcr-1 gene), cotrimoxazole resistance in four isolates, chloramphenicol resistance in two isolates, aminoglycoside resistance in two isolates, and tetracycline resistance in one isolate (Table 1).
Whole Genome Sequencing
The draft genomes of the 11 ST131 porcine isolates yielded 32 to 78 contigs larger than 1 Kb, with assembly sizes ranging from 4.940 to 5.451 Mb (Supplementary Table S4). The WGS data were in complete agreement with our previous characterization performed with conventional methods.
CG and Phylogenetic Analysis
A total of 84 E. coli genomes were taken from different clades and subclades of ST131 in order to calculate the ST131 CG. The main objectives were in regard to insights of ST131 lineage and the relationship between isolates of different origins, with special attention to clade B of ST131 lineage. Because of that, the CG analysis was dominated by clade B (n = 49, 58%), and the remainder isolates belonged to clade C (n = 26, 31%), clade A (n = 8, 10%), and clade I (n = 1, 1%). The final collection represented sequences from human (n = 51, 61%), porcine (n = 19, 23%), avian (n = 8, 10%), and wild animals (Antarctic pinnipeds) (n = 6, 7%) sources.
The CG encompasses 2.98 Mb (length = 2,983,333 ± 7,338 pb), which comprised 3,100 total CDS. The single nucleotide polymorphisms (SNPs) analysis of the CG performed within ST131 lineage revealed a well-defined clade structure, similar to those previously described, including the three defined clades: A, B, and C. In total, 14,838 SNPs were present in the alignment. Furthermore, there was a distance of 7,742 to 8,565 SNPs between isolates from clade A and clade C; 6,508 to 8,675 between isolates from clade A and clade B; and 2,214 to 4,280 between isolates from clade B and clade C. The distance within clades varies greatly: being 36 to 1,782 SNPs in clade A, 8 to 2,051 SNPs in clade B, and 1 to 723 SNPs in clade C (Figure 1 and Supplementary Table S2).
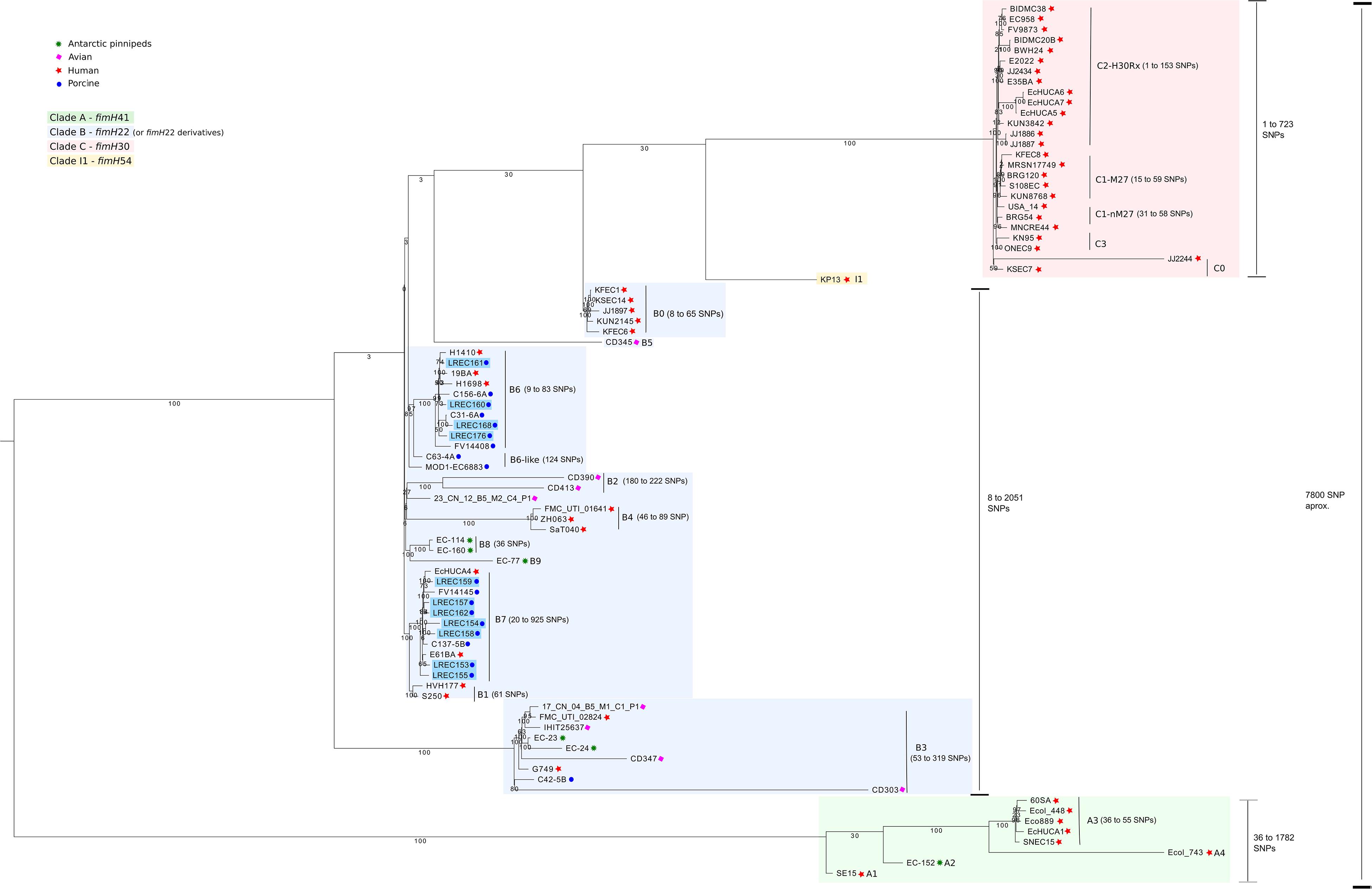
Figure 1. Phylogenetic analysis by core genome of the Escherichia coli sequenced in this study (n = 11) plus other references (n = 73) from ST131 clone.
All isolates of porcine origin analyzed in this study were grouped in clade B. As shown in Figure 1, clade B was characterized by the presence of a mixture of human, livestock, and food origin genomes and showed the greatest intragroup diversity. We identified 11 clusters or subclades (B0, B1, B2, B3, B4, B5, B6, B6-like, B7, B8, and B9), including three subclades (B3, B6, and B7) with isolates of both human and animal origin. In this study, we described five new subclades, named B6, B6-like, B7, B8, and B9. There was a distance of 167 to 232 SNPs between isolates from B6 and B6-like and 172 to 304 SNPs between isolates from B6 and B7. Further, B7 showed 119 to 145 SNPs distance with B1. Those subclades appeared as the closer within each other in clade B (Supplementary Table S2). The 11 ST131 porcine isolates from this study were grouped in the new subclades B6 (LREC_160/PST43, LREC_161/PST43, LREC_168/PST43, LREC_176/PST43 from pork meat and virotype D2) and B7 (LREC_153/PST9, LREC_154/PST9, LREC_155/PST9, LREC_157/PST9, LREC_158/PST9, LREC_159/PST9, LREC_162/PST9 from piglets with diarrhea and virotype D5). The new subclade B6-like includes two isolates from porcine origin. Otherwise, new subclades B8 and B9 only include ST131 isolates from Antarctic pinnipeds.
Notably, there were three (H1410, 19BA, H1698) isolates from human origin in subclade B6 and two (EcHUCA4, E61BA) in subclade B7. The distance between human isolates and porcine isolates from subclades B6 and B7 had an average of 60 SNPs and of 46 SNPs (i.e., 20 and 15 SNP/Mb), respectively.
Plasmidome Analysis
The 11 ST131 porcine isolates showed high heterogeneity in their plasmid content, harboring between two and seven different plasmids (Table 2 and Supplementary Figure S1). We find members of eight of the 17 main MOB plasmid groups found in γ−proteobacteria, ordered by prevalence: MOBF12 (n = 13), MOBP51 (n = 8), MOBP3 (n = 3), MOBV2 (n = 2), MOBH11 (n = 2), MOBP12 (n = 2), MOBP131 (n = 1), and MOBQ12 (n = 1). Finally, we described six plasmids (16%) in which any MOB protein was found (non-mobilizable-infective plasmids). Twenty-two plasmids were considered large (>33 kb) and presumptively conjugative, and sixteen were small multicopy plasmids (<7 kb).
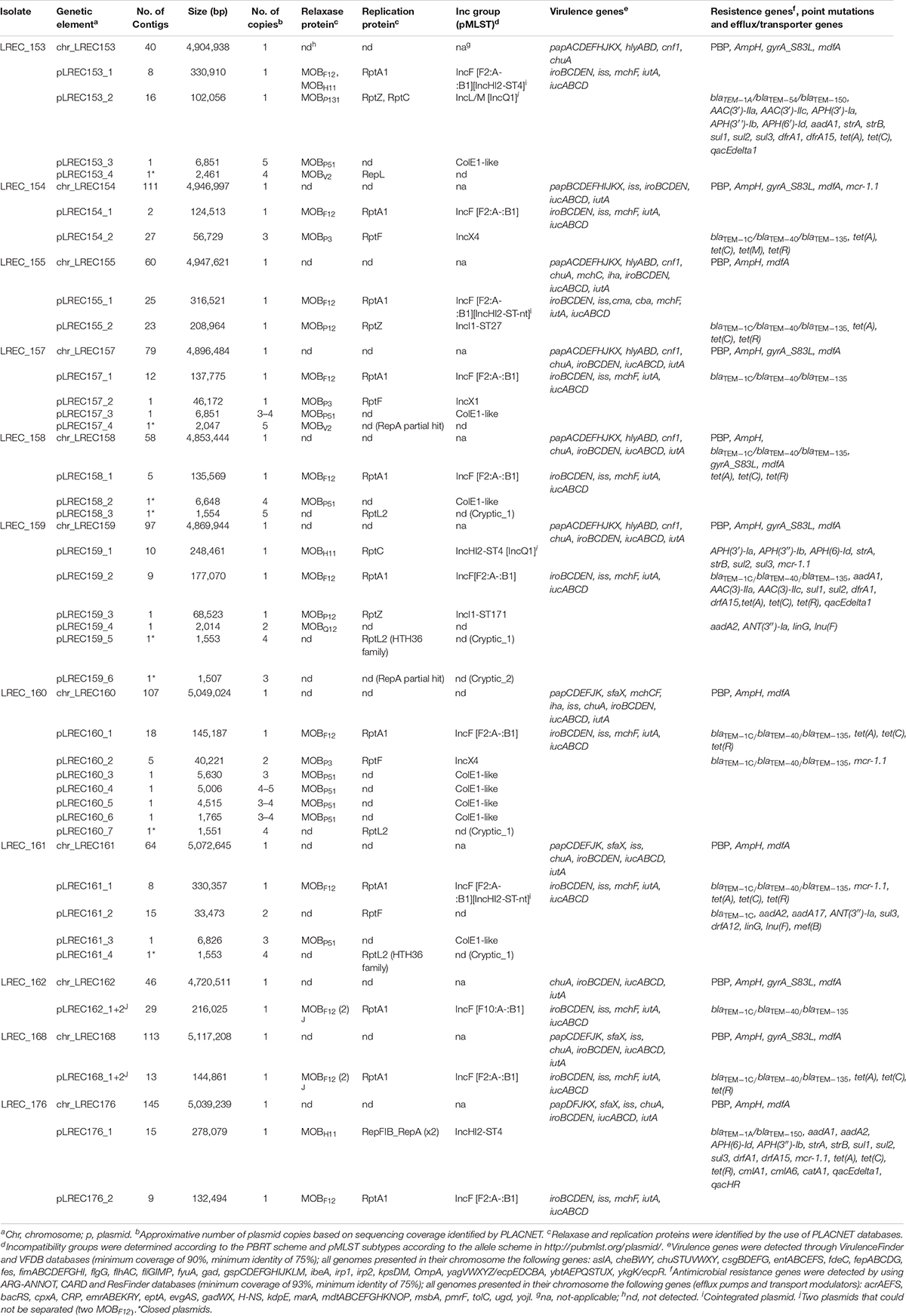
Table 2. Plasmid content of the ST131 Escherichia coli isolates from porcine origin and location of resistance and virulence genes.
In summary, 38 plasmids were described in the 11 genomes analyzed. The plasmids belonged to the following relaxase families (MOB) and incompatibility groups (Inc): MOBP51/ColE1-like (n = 8), MOBF12/IncF [F2:A-:B1] (n = 8), MOBF12/IncF [F2:A-:B1] plus and presumptively cointegrated IncHI2-ST4/ST-nt (not typable) (n = 3), MOBF12/IncF [F10:A-:B1] (n = 2), MOBP12/IncI1-ST27/ST171 (n = 2), MOBP3/IncX4 (n = 2), MOBV2/nd (not detectable) (n = 2), MOBH11/IncHI2-ST4 (n = 1), MOBH11/IncHI2-ST4 plus and presumptively cointegrated IncQ1 (n = 1), MOBP131/IncL/M plus and presumptively cointegrated IncQ1 (n = 1), MOBP3/IncX1 (n = 1), and MOBQ12/nd (n = 1). We also localized six plasmids that could not be affiliated with any categories.
All 13 MOBF12/IncF plasmids were carriers of virulence genes. The genes iroBCDEN, iss, mchF, iutA, and iucABCD were constant (Table 2). All the MOBF12/IncF plasmids found in our study were structurally compared by BRIG software, using the pJIE186_2 (NC_020271) plasmid as reference (Supplementary Figure S2). The plasmids analyzed showed extensive sequence similarity over at least 100 kb, which includes not only backbone genes. The comparison emphasizes that all of them share an 80-kb conserved region comprising the virulence genes described previously as well as cvaABC, sitABC, ompT, and hlyF.
The eight MOBP51/ColE1-like plasmids identified in this study exhibited a wide size range (1.7–6.8 kb) and were analyzed using the ColE1 plasmid (J01566.1) as reference (Supplementary Figure S3). The plasmids pLREC153_3, pLREC157_3, pLREC158_2, and pLREC161_3 (all of them >6.8 Kb) showed the highest homology to the reference plasmid. All of them carried the colicin E1 cea and immunity (imm) genes and the entry exclusion (exc1-exc2) system genes. On the other hand, plasmids from isolate LREC_160 were different among them and with the others, exhibiting a wide size range (1.8–5.6 Kb), and only pLREC160_4 carried the colicin E1 gene.
Three MOBP3/IncX plasmids were present among our isolates. We found pLREC157_2 that belong to the IncX1 subcluster and had a similar backbone than p2ESCUM (NC_011739.1) but codes for a completely different RIP (Supplementary Figure S4), whereas pLREC154_2 and pLREC160_2 belong to the IncX4 subcluster and were analyzed using pSH696_34 (JX258654.1) as a reference (Supplementary Figure S5). Because pLREC160_2 carried the mcr.1-1 gene, some previously reported IncX4 reference plasmids harboring the mcr gene were added. Compared to pSH696_34, plasmid pLREC160_2 and the other mcr carrier plasmids (pESTMCR and pICBEC7Pmcr) lacked two important backbone genes: the conjugative coupling protein gene (traG) and the replication protein encoding region (rep).
Structural comparison of the two MOBP12/IncI1-ST171 plasmids and the single MOBP131/IncL/M plasmid found within our isolates was also performed using pEK204 (EU935740) and pEC743-OXA48 (CP015071.1) as references, respectively. Both showed a high coverage of homology with the reference (Supplementary Figures S6, S7).
We also described five small (1.5 kb) multicopy plasmids in which any relaxase protein was found, categorized as cryptic. Those cryptic no-MOB plasmids were analyzed by using pEC10D (NC_017650.1) as reference (Supplementary Figure S8). BRIG comparison showed that four plasmids were highly similar to pEC10D and codes for the same replication protein (cryptic 1, from the HTH36 superfamily replication proteins), whereas pLREC159_6 codes for a different replication protein and was called cryptic 2 (Table 2).
Finally, we analyzed the MOBV2 plasmids found in the isolates LREC_153 and LREC_157. A BLAST research was performed to identify a reference plasmid, being pEC0674 (MF684783.1) as the closest match. Two MOBV2-like–related plasmids were also included for structural comparison (Supplementary Figure S9). Although no significant similarity was found between these latter two references and the pEC0674, the pLREC153_4 showed similarity with the replication protein, a hypothetical one and the Rec protein from the reference plasmid, whereas the pLREC157_4 only showed similarity to the latter two proteins.
Antimicrobial Resistance Genes
Many antimicrobial resistance genes (ARGs) were found in the 11 ST131 porcine isolates (Table 2), including acquired resistance genes, point mutations, and efflux/transporter genes.
Besides constitutive genes (PBP and AmpH, which were present in all isolates), ARGs conferring resistance to penicillin (variants of blaTEM gene, accounting: blaTEM–1C, n = 10; blaTEM–40, n = 10; blaTEM–135, n = 10; blaTEM–150, n = 2; blaTEM–1A, n = 2; blaTEM–54, n = 1), tetracycline (variants of tet gene, accounting: tetA, n = 9; tetC, n = 9; tetR, n = 8; tetM, n = 1), quinolones (gyrA_S83L, n = 7), and colistin (mcr-1.1, n = 5) were the most common. In addition, we described genes conferring resistance to sulfonamides (variants of sul gene, accounting: sul2, n = 4; sul3, n = 4; sul1, n = 3), trimethoprim (variants of drf gene, accounting: dfrA1, n = 3; dfrA15, n = 3; dfrA12, n = 1), aminoglycosides (different ACCs, APHs, and ANTs genes, accounting: APH-3″-Ib, n = 3; APH-6′-Id, n = 3; aadA1, n = 3; ANT-3″-Ia, n = 3; strA, n = 3; strB, n = 3; APH-3′-Ia, n = 2; aadA2, n = 2; AAC-3′-IIa, n = 2; AAC-3′-IIc, n = 2; aadA17, n = 1), phenicols (variants of cmlA gene, catA1, and mdfA gene, accounting: mdfA, n = 11; cmlA1, n = 1; cmlA6, n = 1; catA1, n = 1), and to ammonium quaternary biocide (qacEdelta1, n = 3; qacHR, n = 1) (Table 2).
The phenotypic resistance profile in the studied genomes was due to genes carried by plasmids, except for quinolone resistance, which in our study is mediated by a Ser83Leu point mutation found in the chromosomal gyrA gene. In the case of LREC_158 isolate, it exhibited the blaTEM genes (−1c, −40, and −135) variants set into a chromosomal assembled contig. However, these genes were located into IncL/M, IncX4, IncI1, IncF, and IncHI2 in the other analyzed isolates (Table 2).
As shown in Table 1, all but one isolate exhibited an MDR profile. Large conjugative MDR plasmids were identified in four of the 10 isolates. These were typed as MOBH11/IncHI2-ST4 (pLREC159_1 and pLREC176_1), MOBF12/IncF (pLREC159_2, pLREC161_1), and MOBP131/IncL/M (pLREC153_2) and a non-typable one (pLREC161_2). Most common antibiotic resistance profiles encoded by these plasmids are ampicillin/penicillin, aminoglycosides, tetracyclines, sulfonamides, cotrimoxazole, and polymyxins. Finally, we described some drug efflux genes in pLREC159_4 and pLREC161_2 (Table 2).
Class 1 integrons were present in pLREC153_2 (IntI-attI [-sul2 + aph-(3″)-Ib + aph-(6)-Id + dfrA1 + aadA1]-qacE1-sul1), pLREC159_2 (IntI-attI [dfrA1 + aadA1]-qacE1-sul1), and pLREC176_1 (IntI-attI [dfrA1 + aadA1]-qacE1-sul1). No class 2 or 3 integrons were detected in our genomes.
Colistin Resistance Vehicles and Genetic Environment
Colistin resistance by mcr gene was found in five of the analyzed isolates. Therefore, the mcr-carrying plasmids were investigated in greater depth. The mcr-1.1 gene was located in two MOBH11/IncHI2-ST4, a MOBP3/IncX4 and a MOBF12/IncF [F2:A-:B1], which was theoretically cointegrated with an IncHI2-ST-nt plasmid. Besides, the LREC_154 isolate showed the mcr-1.1 in a chromosomal location (Table 2). In this study, any point mutation in the chromosomally encoded genes pmrA or pmrB was found.
The complete sequence of pLREC160_2 (MOBP3/IncX4) was 40,221 bp in size and contains 69 predicted ORFs. A structural comparison against other reference IncX4 plasmids is shown in Figure 2, where pICBEC7Pmcr (34,992 pb, NZ_CP017246.1) was used as the internal reference. The structure of the mcr-1 carriers IncX4 plasmid remains stable if we exclude one ORF encoding for an IS91 family transposase present in pLREC160_2 and pICBEC7Pmcr. The BRIG comparison showed that the mcr-1–negative plasmid pJIE143 differs from pICBEC7Pmcr in a region of approximately 6 kb (encoding for parA, mcr, and pap2 genes). All the analyzed plasmids showed a typical IncX4 backbone, including the RIP gene (pir), conjugal transfer protein genes (tra) plus taxABC, and pilX operons (Supplementary Figures S10–S13).
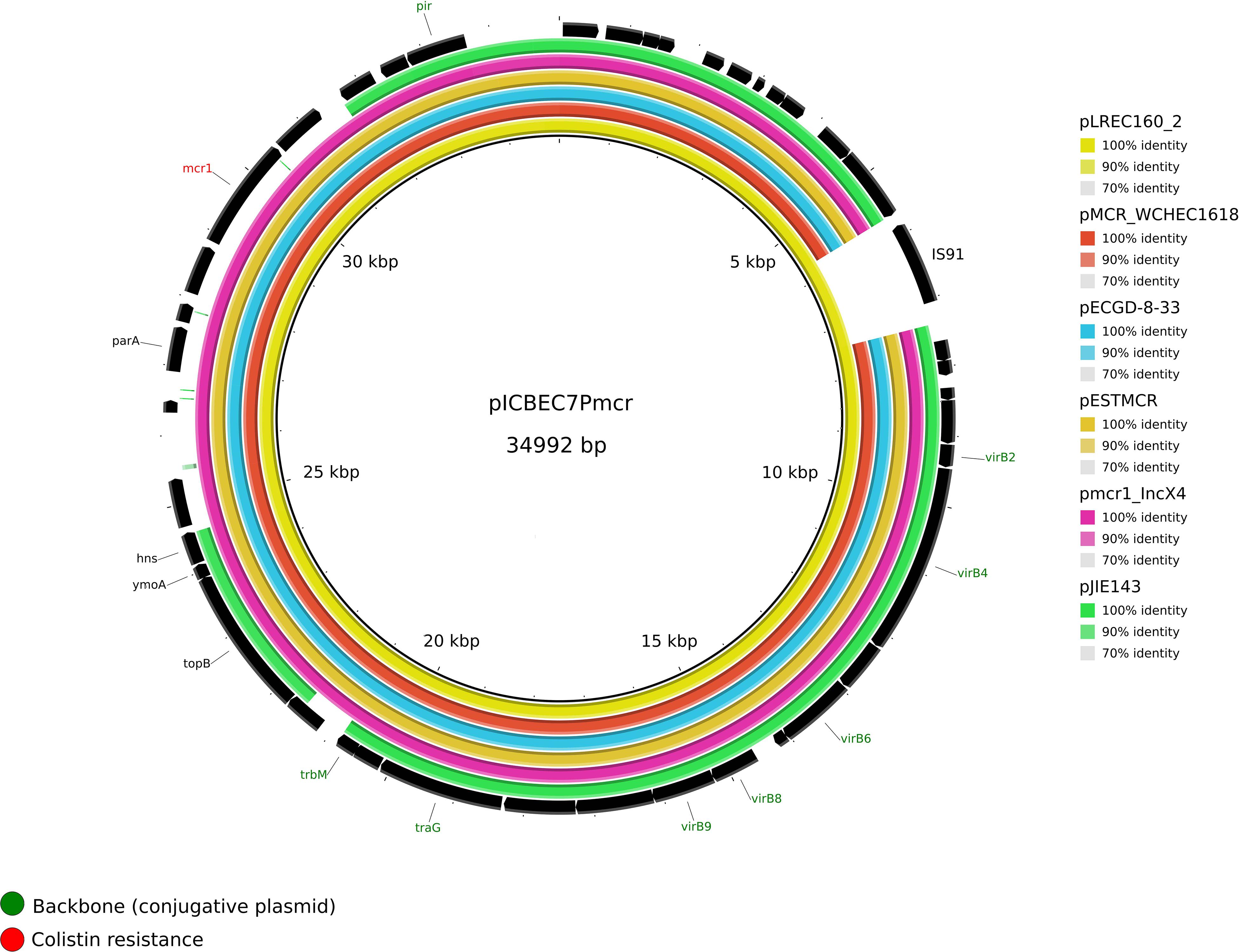
Figure 2. Structural comparison between mcr-1 plasmids and IncX4 references. The alignment includes five references and the one IncX4 mcr-1–bearing plasmid found in our study. The plasmid pICBEC7mcr (IncX4) was used as a reference to match with the other plasmids with [pMCR_WCHEC1618, pECGD-8-33, pESTMCR, and pmcr1_IncX4] and without the mcr-1 gene [pIJE143]. The outer circle with black arrows denotes the annotation of reference sequence pICBEC7mcr. The image was generated using BRIG (default parameters with 90/70 as upper/lower threshold).
Plasmid pLREC161_1 was 330,357 bp in size and contains 616 predicted ORFs. This MOBF12 plasmid belongs to the IncF [F2:A-:B1] incompatibility group and was theoretically cointegrated with an IncHI2 plasmid. A structural comparison with plasmid pMR0516mcr [F18:A-:B1] (KX276657.1) as an internal reference (225,069 bp) showed 100% identity in more than 70% of query coverage from BRIG (Figure 3). Genes involved in the conjugative transfer (tra, trb, and pili operons) in the toxin-antitoxin system (vagC) and the inhibition of SOS response (psiAB) were present. Most of the differences were observed in the resistance module. It is worthy of note that pLREC161_1 and pMR0516mcr plasmids contained APEC virulence genes and that the surrounding region of the mcr gene includes heavy metal resistance genes to tellurium (ter genes). Moreover, in Supplementary Figure S14, where pKP81_BE [F2:A-:B] (KU994859.1) was used as the reference, we observed 100% of identity in more than 78% of query coverage from BRIG with pLREC161_1. Interestingly, plasmid R478 (IncHI2 reference plasmid, U62007.2) also shared with pKP81_BE some backbone genes and the tellurium resistance module like pMR0516mcr (Supplementary Figure S14). A wide part of the backbone in pLREC161_1 (approximately 44 kb) was common with the widely studied IncF plasmid from E. coli-K12, used as non-mcr plasmid reference (from coord. 55 to 99 kb). The ancestral IncF plasmid of ST131 (pECSF1) was also included in BRIG comparisons (Supplementary Figure S15).
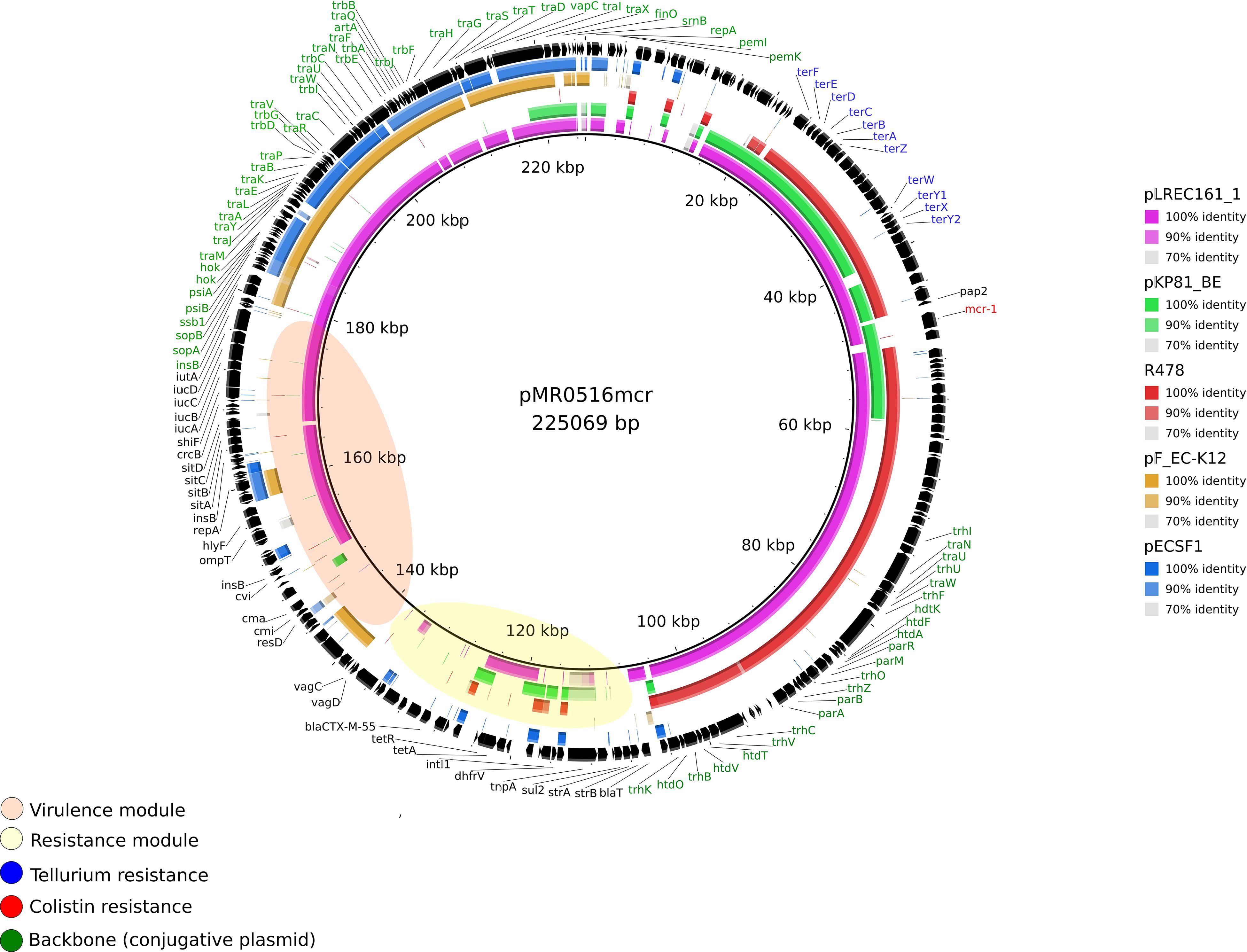
Figure 3. Structural comparison between mcr-1 plasmids, IncF, and IncHI2 references. The alignment includes four references and the one IncF mcr-1–bearing plasmid found in our study. pMR0516mcr (IncF) was used as a reference to match with the other plasmids with [pKP81_BE (IncF)] and without mcr-1 gene [IncF plasmid from Escherichia coli K12, pECSF1 (IncF) and R478 (IncHI2)]. The outer circle with black arrows denotes the annotation of pMR0516mcr. The image was generated using BRIG (default parameters with 90/70 as upper/lower threshold).
There were two MOBH11/IncHI2-ST4 mcr-1–bearing plasmids: (I) pLREC159_1, which was 248,461 bp in size and contains 420 predicted ORFs, and (II) pLREC176_1, which was 278,079 bp in size and contains 523 predicted ORFs. Both showed 100% of identity over more than 75% of query coverage in BRIG comparison with pHNSHP45-2 (KU341381.1) (Figure 4). The backbone was almost identical; nevertheless, the resistance module was distinct between them. The result of this comparative analysis showed that a wide range of resistance genes could be found in the MDR module of IncHI2 plasmids. Moreover, all the IncHI2 plasmids analyzed presented a tellurium resistance module close to the mcr-1 cassette. The similarities between IncHI2 plasmids were high, even though their origin and parental isolate characteristics differ.
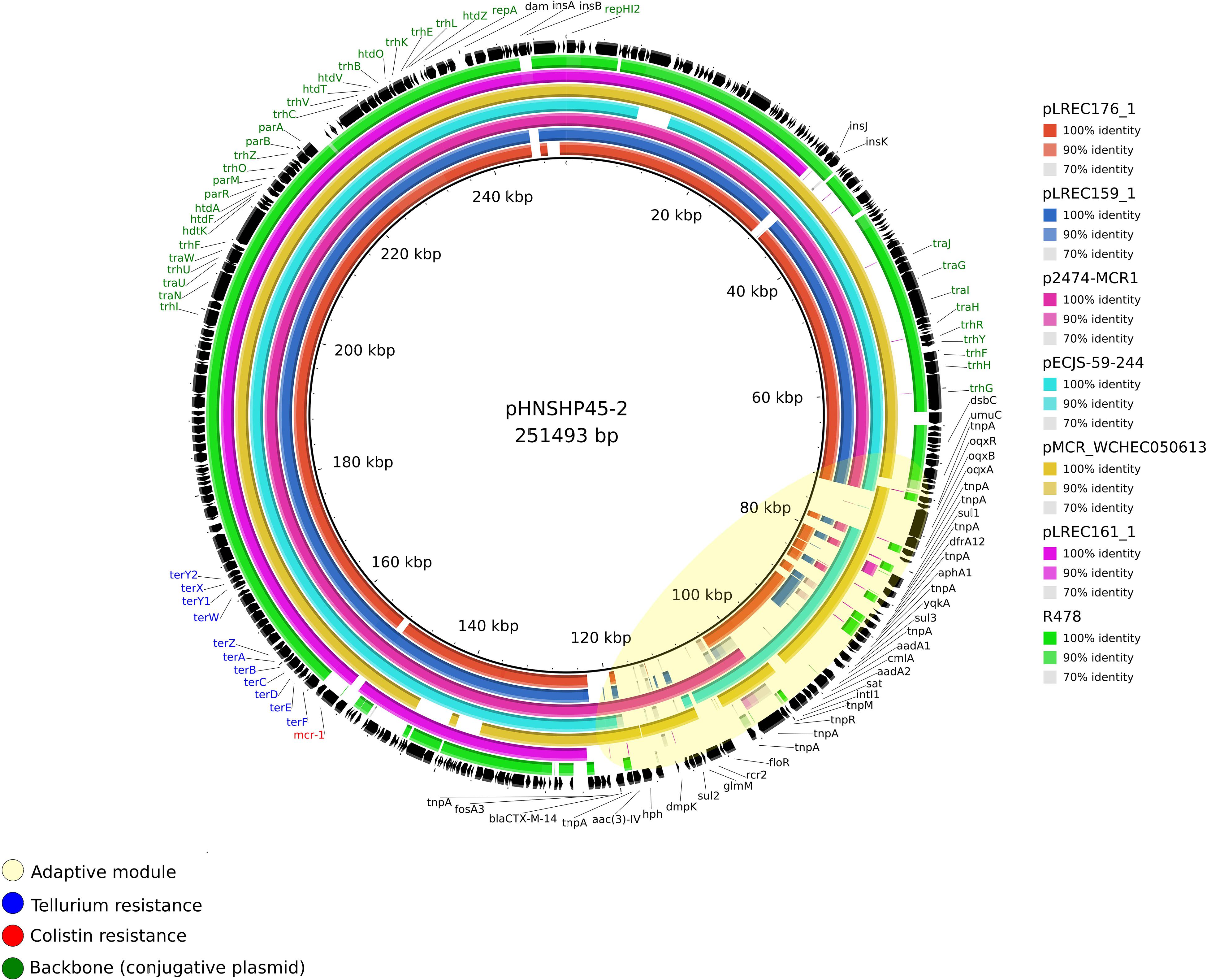
Figure 4. Structural comparison between mcr-1 plasmids and IncHI2 references. The alignment includes four references and the mcr-1–bearing plasmids from our study pLREC176_1 and pLREC159_1, which are IncHI2 and pLREC161_1, which is an IncF plasmid with a presumptively IncHI2 cointegrated. The plasmid pHNSHP45-2 (IncHI2) was used as a reference to match with the other plasmids with [p2474-MCR1 (IncHI2), pECJS-59-244 (IncHI2), and pMCR_WCHEC050613 (IncHI2)] and without the mcr-1 gene [R478 (IncHI2)]. The outer circle with black arrows denotes the annotation of reference sequence pHNSHP45-2. The image was generated using BRIG (default parameters with 90/70 as upper/lower threshold).
Afterward, a comparison between all our mcr-1–bearing plasmids was performed with pHNSHP45-2 (MOBH11/IncHI2) as a reference, and as expected, almost none homologous region was found between plasmids from different families (Supplementary Figure S16). Nevertheless, pLREC161_1 (MOBF12/IncF [F2:A-:B1] plus IncHI2-ST-nt) plasmid shared a part of his backbone with the reference one, including the module encoding for tellurium resistance and the mcr gene, suggesting that the mcr-1 cassette was introduced into pLREC161_1 through the cointegrated IncHI2 plasmid.
We had also made a sequence alignment to analyze the surrounding region of our mcr-bearing contigs and compare their structure against plasmids references (Figure 5). Regarding plasmid families, all the IncX4 plasmids were identical in terms of the genetic arrangement in the vicinity of the mcr-1 gene, whereas the insertion site of mcr-1 gene in IncHI2 plasmid was variable. Interestingly, the mcr region in plasmids pMCR_WCHEC050613 (IncHI2) and plasmid pMR0516mcr (IncF) shared 100% of identity. It is also worth to note that the IncF mcr harboring plasmid pKP81_BE carried the tellurite resistance module surrounding the mcr gene like the IncHI2 plasmids.
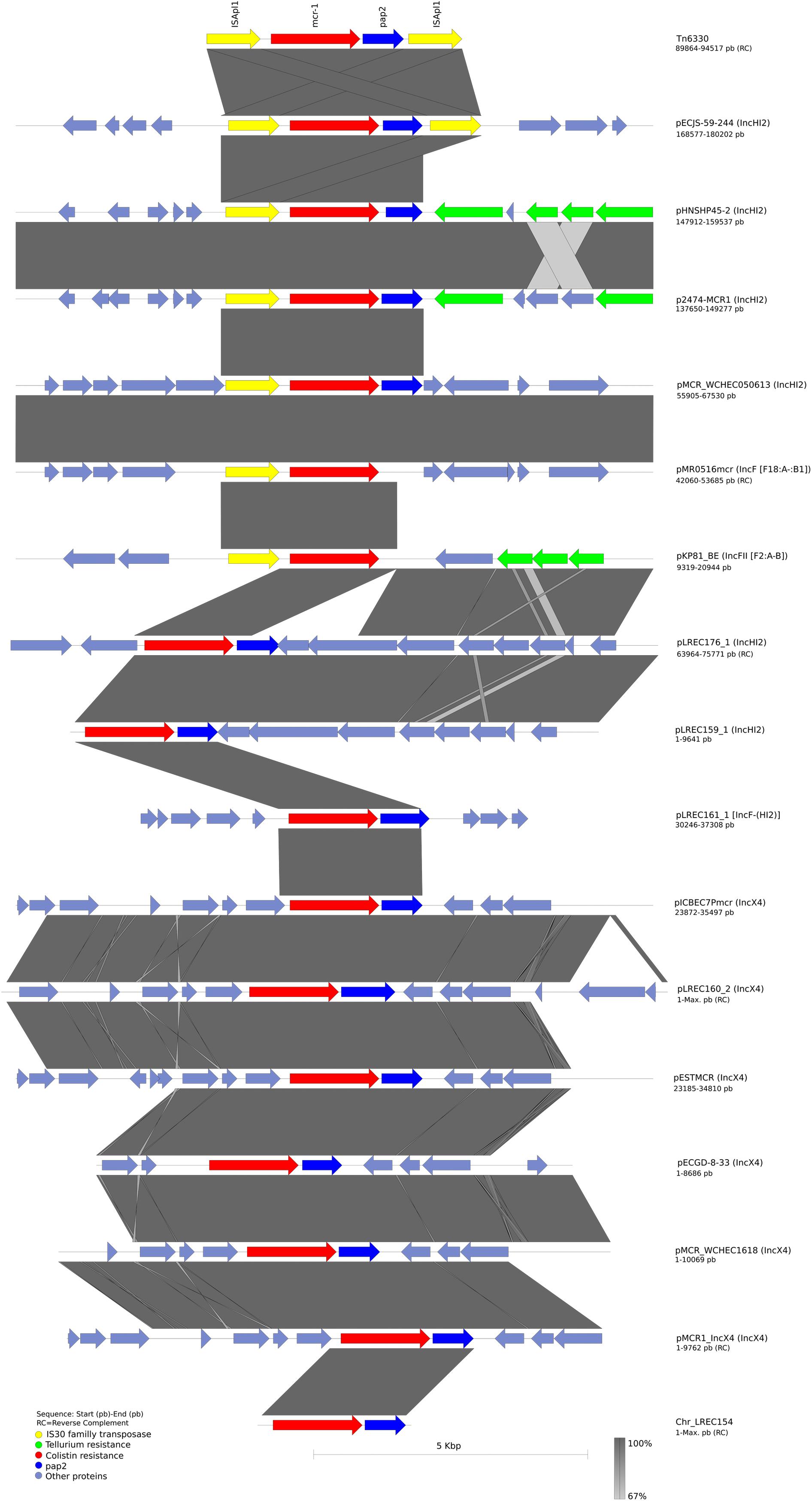
Figure 5. Comparison of the homologous region of the composite transposon Tn6330 (CP029493.1) containing mcr-1 gene with our sequences and other frequently used reference sequences analyzed in this article. Open arrows represent coding sequences (green for tellurium resistance, yellow for ISApl1, red for mcr-1, and blue for pap2) and indicate direction of transcription. The arrow size is proportional to the gene length. The shadow parallelograms denote genetic regions that exhibit sequence homology among different segments. Light shadow denotes regions with a lower level of sequence identity (99%) by BLAST. The image was generated using EasyFig (default parameters).
Finally, the genetic arrangement of the mcr-1 cassette was investigated (Supplementary Figure S17). All sequences share a 2,542-bp DNA segment, containing mcr-1.1 and a putative ORF, encoding a Pap2 superfamily protein. However, some variability in the last 3′ end of the pap2 gene was observed.
The genome reconstruction of LREC_154 showed that mcr gene was inserted into the chromosome. However, the mcr harboring contig included only mcr and pap2 genes. It was flanked at both ends by a partial ISApl1 sequence of 99 bp upstream and 405 pb downstream the mcr-1 cassette. In pLREC159_1, the mcr harboring contig was disrupted after a segment of 87 bp of DNA homologous to ISApl1 upstream the mcr gene. Probably the presence of the IS (repeated element) has interfered with the de novo reconstruction of the sequenced genome by the assembly of Illumina short reads. In addition, we identified the IS26 and an IS1294 surrounding the mcr-1 cassette in pLREC160_2.
Plasmids pLREC159_1 and pLREC176_1 identified as IncHI2-ST4 had identical genetic environments downstream the mcr-1 cassette.
Statistical Analysis
The variables (cdtB, cnf1, hlyA, virotypes, PST, and subclades) did not follow a normal distribution (Shapiro–Wilk test: p < 0.0001). We performed the Mann–Whitney–Wilcoxon test to assess significative differences in the distribution of features (virotype D5| subclade B7| PST9| cnf1| hlyA vs. virotype D2| subclade B6| PST43| cdtB variables; p = 0.007) between ST131 isolates from piglets with diarrhea and pork meat.
Discussion
As far as we know, there are only six reports on ST131 from swine: (I) Trobos et al. (2009) studied 68 sulfonamide-resistant E. coli from different hosts and identified the first swine ST131 isolate obtained from pork meat in Denmark in 2003; (II) Schink et al. (2013) detected a CTX-M-1 ST131 isolate recovered from a porcine gastrointestinal tract infection among 495 ESBL-producing E. coli analyzed in Germany during the years 2006–2007; (III) Herrera Estévez (2015) detected 18 ST131 isolates from 200 pork meat samples obtained in Spain during the years 2011 and 2012; (IV) Liu X. et al. (2018) collected an isolate from a diarrheal pig among 44 ESBL-producing E. coli from Northwest China (2015–2017); (V) García-Meniño et al. (2018) identified 18 ST131 isolates recovered from the 1823 piglets with diarrhea sampled in Spain between the years 2009 and 2017; and (VI) Reid et al. (2019) reported two highly related ST131 E. coli isolates, the first from a healthy pig and the second from a human urinary tract infection, obtained in New South Wales (Australia) in 2007 and 2009, respectively.
In the present study, we performed WGS analysis of a collection of 11 representative ST131 isolates obtained from piglets with diarrhea and pork meat in Spain. All of the 11 ST131 isolates belonged to serotype O25b:H4 and clade B and presented a high number of virulence genes typical of E. coli that cause extraintestinal infections. In addition, the 11 porcine isolates harbored traits that genetically satisfied the criteria for designation as ExPEC, UPEC, and APEC status being designated as APEC-, UPEC-, and ExPEC-like isolates because of their site of isolation. Although seven of the 11 ST131 isolates were isolated from piglets with diarrhea, none of them were positive for virulence genes of intestinal pathogenic E. coli (Mora et al., 2011).
In Supplementary Figure S18, based on the results published by Matsumura et al. (2017) and Ben Zakour et al. (2016), we had represented the already known ST131 lineages plus the new lineages found in this study. These sublineages were designated according to their fimH allele (type 1 fimbriae adhesin gene), phylogenetic clade (A, B, B0, I1, C0, C, C1, C2, and C3), and resistance profile. Briefly, B-H22 clade, a fluoroquinolone (FQ)– and cephalosporin-susceptible ancestor, evolved in C-H30R (where “R” indicates resistance to FQ) clade that was the predecessor of two sister subclades, C1-H30R and C2-H30Rx (often carrier of the blaCTX–M–15 enzyme). Later, two additional ST131 sublineages referred to C1-M27 (positive for the blaCTX–M–27 enzyme) and C1-nM27 (non-ESBL or positive for the blaCTX–M–14 enzyme) have been described. The ST131 clonal group accounted for 490 (16%) of 2,995 isolates obtained from extraintestinal clinical samples collected in five Spanish hospitals during the period 2005 to 2012 (Dahbi et al., 2014). The most common lineages in this clinical collection were the C2-H30Rx (61.6%), B (17%), and C1-nM27 (16%). Recently, Mamani et al. (2019) also described the subclade C2-H30Rx as the most prevalent (85%) among 41 ST131 ESBL-producing E. coli causing bacteremia in a Spanish hospital over a 12-year period (2000–2011). In contrast, almost all Spanish ST131 isolates obtained from animal origin belonged to clade B. In our laboratory, we have tested a collection of 98 ST131 isolates from animals (57 from avian, 36 porcine, and five from Antarctic pinnipeds), and all but one from pinnipeds belonged to clade B. Although clade B isolates share numerous virulence genes, virotypes vary depending on the origin of the isolates. Thus, all avian isolates have virotype D4 (ibeA, neuC-K1), whereas swine isolates have virotypes D2 (ibeA, papG III, cdtB, kpsM-II-K5) and D5 (ibeA, papG III, cnf1, hlyA, kpsM-II-K5). Among the 45 clade B isolates causing bacteremia in humans in Spain, the most frequent virotype was D2 (n = 19), followed by virotypes D3 (n = 12), D1 (n = 8), D4 (n = 6), and D5 (n = 6) (Blanco et al. unpublished data).
The CG SNP-based phylogenetic tree of 84 human and animal ST131 isolates analyzed in this study showed (Figure 1) that they are mostly clustered into three well-defined clades (A, B, and C), and similarly to Petty et al. (2014) study, we have observed that clade A is the most divergent from clades B and C. Our results also agree with those published by other authors (Ben Zakour et al., 2016; Matsumura et al., 2017; Liu C. M. et al., 2018; Decano and Downing, 2019) regarding that clade B isolates are displayed into multiple subclades. We identified the six subclades (B0, B1, B2, B3, B4, and B5) described by Ben Zakour et al. (2016) and five new ones (B6, B6-like, B7, B8, and B9). Notably, the 11 ST131 porcine isolates from this study were grouped in the new subclades B6 (four isolates from pork meat and virotype D2) and B7 (seven isolates from piglets with diarrhea and virotype D5). The new subclade B6-like also includes two isolates of porcine origin, whereas the new subclades B8 and B9 included only ST131 isolates from Antarctic pinnipeds. In contrast, avian isolates of virotype D4 were distributed in the subclades B2, B3, and B5.
The newly described B6 and B7 subclades were primarily associated with porcine isolates, but also include some isolates causing bacteremia and other extraintestinal infections in humans. Therefore, it is remarkable how those human isolates were closely related (46–60 SNPs average distance) with porcine isolates. Those results suggest the ecological overlap of human and animal isolates. Recently, Reid et al. (2019) compared the genomes of two ST131 clade B fimH22 isolates obtained in Australia, the first one isolated in 2007 from a healthy piglet and the second one obtained in 2009 from a human urinary tract infection. This study showed that the two isolates were highly related, separated by only 20 core SNPs (Reid et al., 2019). Thus, the analysis of the phylogeny confirmed E. coli ST131 as pathogen capable of frequent interspecies movements.
The plasmidome of E. coli ST131 clade B from porcine origin had shown variability and some interesting differences concerning the actual published data. IncF plasmids are a common feature in all ST131 isolates (Lanza et al., 2014), having at least one per isolate in this study. Specifically, F1:A2:B20 and F2:A1:B- plasmids are more commonly associated with the C1-H30R clade and C2-H30Rx, respectively (Johnson et al., 2016). In contrast, F2:A-:B1 plasmids were dominant in the porcine clade B isolates used in this study. This type of plasmid was absent in 35 non-ST131 E. coli porcine isolates from colibacillosis (García-Meniño et al., 2019), suggesting that IncF plasmids vary greatly within the different lineages. Reid et al. (2019) reported 23 F-types among 282 isolates from a mixture of origins belonging to the clade B of ST131, and the F2:A-:B1 IncF subtype was one of the commonest (32%).
In accordance with previous studies, plasmids carrying virulence genes belong to the IncF family (Lanza et al., 2014; Nicolas-Chanoine et al., 2017). All the IncF plasmids found in this study conserved the ColV region, frequently detected among avian pathogenic E. coli (APEC) (Liu C. M. et al., 2018). This region comprises the iss (increased serum survival) gene, the iroBCDEN gene cluster (encoding the salmochelin siderophore system present in Salmonella enterica spp. and some ExPEC isolates), the iucABCD operon (encoding the enzymes of the biosynthetic pathway for aerobactin), iutA (outer membrane receptor of ferri-aerobactin complexes) gene, cvaB and cvaC (production of colicin) genes, sitC (Salmonella iron transporter C), and an ompT-hlyF-mig14 cassette (outer membrane related genes). Some APECs cause human infection (Liu C. M. et al., 2018); nevertheless, ColV plamids were absent in specific human clades, so they may not be a necessary feature to cause human infection (Reid et al., 2019). It is currently unknown which advantages could the IncF plasmids confer to the bacterial cell apart from virulence and resistance to antibiotics. However, Johnson et al. (2016) has hypothesized that they must play multiple roles in the host success because ST131 clone has adapted to harbor these plasmids at lower fitness cost and has stabilized them through mutational events. We assume that the presence of both, resistance and virulence genes, on the same MGE is worrying for public health, as shown in our study in IncF plasmids such as pLREC161_1 and pLREC 159_2. For instance, the evolutionary analysis performed by Ben Zakour et al. (2016) proved that ST131 emergence was adapted by isolates that had acquired first a subset of virulence genes followed by the gain of antibiotic resistances.
ColE1-like plasmids were the second more prevalent in our collection and the replication protein could not be determined; these results are in accordance with the findings of Lanza et al. (2014). Furthermore, ColE plasmids have been reported as mcr-4 and mcr-5 carriers (Borowiak et al., 2017; Carattoli et al., 2017; García-Meniño et al., 2019). The ColE1-like plasmids from this study did not carry resistance genes. However, they might be an important adaptive weapon. Moreover, we described one MOBP131 and two MOBV2 plasmids, which are not frequently documented and therefore absent among the description given by Lanza et al. (2014) over ST131 plasmidome, although another MOBP131 plasmid has been recently found from a blaOXA–48 E. coli ST131 isolate collected from a patient with urinary infection (Stoesser et al., 2016c), and MOBV2 plasmids have been described in other STs, such as ST69 from a human urine sample and ST93 from chicken meat samples (de Been et al., 2014). Besides, MOBV2 plasmids pLREC153_4 and pLREC157_4 showed structural homology (around coordinates 3.7–7 kb in Supplementary Figure S9) with the pEC0674 plasmid (6 kb), an mcr-5 carrier plasmid belonging from an ST29 E. coli isolate recovered from porcine feces in Germany (Hammerl et al., 2018). Finally, and also barely documented, were the cryptic plasmids found in our study. Similar small no-MOB plasmids such as pLREC158_3, pLREC159_5, pLREC159_6, pLREC160_7, and pLREC161_4 have been reported among ST131 isolates. The role of these plasmids is still unknown, but researchers have suggested that they could be providing useful mobilization tools for ST131 adaptation (Lanza et al., 2014).
Antimicrobial resistance genes conferring resistance to penicillin/ampicillin, tetracycline, quinolones, and colistin were the most common determinants. García-Meniño et al. (2019) described chromosomal mutation (PmrB V161G or PmrA S39I) in addition to the plasmid mechanism (mcr) in 16 (46%) of non-ST131 colistin-resistant porcine E. coli isolates. Here, any complementary chromosomal mechanism related with system PmrAB was found. Nonetheless, some drug efflux genes were found in plasmids. Members of the lnu (previously lin) gene family (mediate lincosamide resistance) (Achard et al., 2005; Roberts, 2008) and the mef(B) gene, which coexists with the aadA and sul3 resistance genes (pLREC161_2). This combination has previously been described in plasmids from porcine E. coli isolates and mediates macrolide resistance (Liu et al., 2009).
The recent analysis of 35 colistin-resistant non-ST131 E. coli isolates from porcine colibacillosis showed that 18 isolates (51%) were carriers of the mcr-1.1 gene variant. Those isolates were all MDR, and the 50% belonged to Clonal Complex 10 (6 ST10, 1 ST5786, and 2 ST7323). However, the remaining isolates belonged to different linages with single representants (ST1, ST29, ST42, ST93, ST100, ST118, ST156, ST398, and ST4247). The mcr-1.1 gene was located in plasmids IncHI2 (33%) and IncX4 (22%), but it was also found chromosomally encoded in four isolates (García-Meniño et al., 2019).
Currently, the mcr-1 cassette was found in a large diversity of plasmids and clonal lineages of E. coli. The broad adaptation of colistin resistance all over the world could be due to the ease with which the mcr-1 gene integrates into various regions (Hadjadj et al., 2017). There is an association with successful plasmid families (Matamoros et al., 2017; Zhong et al., 2018). On the other hand, any association with clonal lineages of E. coli is described (Li et al., 2018). However, the description of mcr-1–positive E. coli ST131 isolates is exceptionally worrying. Because ST131 has once been responsible for the worldwide appearance, ESBL resistances due to the acquisition of specific IncF epidemic plasmids harboring blaCTX–M–15 gene (Nicolas-Chanoine et al., 2008, 2014; Mathers et al., 2015) and therefore major therapeutic failure could come due to the association of mcr-1 gene with other broad-spectrum resistance mechanisms, such as ESBLs and/or carbapenemases (Du et al., 2016; Haenni et al., 2016; Li et al., 2016; Olaitan et al., 2016; Skov and Monnet, 2016). Further, clade B of ST131 has been signaled out as a potential foodborne uropathogen and as a stronger early biofilm producer than clade C of ST131 (Nicolas-Chanoine et al., 2017; Liu C. M. et al., 2018; Flament-Simon et al., 2019).
In this study, the genetic context of mcr-1 gene was diverse. Recent studies propose that the transposon Tn6330, flanked at both ends by ISApl1, has led the mobilization of mcr-1 gene. Therefore, four structures of mobile elements carrying mcr-1 have been described: (I) the composite transposon Tn6330 (ISApl1–mcr-1–orf–ISApl1 structure); (II) a single ISApl1 upstream (ISApl1–mcr-1–orf structure); (III) a single ISApl1 downstream (mcr-1–orf–ISApl1 structure); and (IV) and sequences lacking ISApl1 altogether (mcr-1–orf structure), although a few other truncated or interrupted versions have been reported (Pham Thanh et al., 2016; Snesrud et al., 2016). In the study carried by Snesrud et al. (2018), prevalence rates of 11% for structure I, 22% for structure II, 1% for structure III, and 66% for structure IV (with no associated IS) have been described. Besides, the upstream ISApl1 element was observed in 78% of IncHI2 plasmids (Matamoros et al., 2017), whereas the mcr-1–orf structure has been described as dominant in small mcr-1–IncX4 plasmids (Li et al., 2017b; Sun et al., 2017). These results are in line with our findings. The five ST131 colistin-resistant isolates from this study presented the mcr-1.1 allele (KP347127.1) and showed the mcr-1–orf structure. However, pLREC159_1 (IncHI2) presumptively harbored the ISApl1 upstream, whereas any copy of ISApl1 was present around the mcr-1 gene in pLREC160_2 (IncX4). Nevertheless, pap2 gene and ISs IS26 and IS1924 were present in pLREC160_2. Zhong et al. (2018) suggested that for IncX4 plasmids IS26/26 structures could be involved in mcr-1 gene mobilization. The BRIG comparison showed that the mcr-1–harboring IncX4 plasmids were genetically homologous between each other as previously described (Sun et al., 2017), suggesting that mobilization of the mcr-1 cassette was quite stable within this type of plasmids. Furthermore, pLREC159_1 and pLREC176_1 were almost identical in structure around the mcr-1 gene and were related to the presence of tellurium resistance genes, as well as for pLREC161_1. This heavy metal resistance lends HI2 plasmids to success in wastewater environments (Reid et al., 2019).
Moreover, a highly relevant finding of the present study was the appearance of chromosomally encoded colistin resistance. We found a presumptive ISApl1–mcr-1–orf–ISApl1-like structure. This genetic arrangement was described as the most prevalent by Li et al. (2018) in bacterial chromosomes. It has been proposed that the lack of one or both of the ISApl1-elements implies stabilization of the mcr-1 gene (non-transposable) (Snesrud et al., 2016, 2018). Thus, chromosomally encoded colistin resistance might mean a more stable inheritance in ST131 porcine lineage. Nevertheless, it also implies less mobilization among isolates.
Some studies have proved that stopping using colistin in positive mcr-1 carriers porcine farms has resulted in the disappearance of the resistance (Randall et al., 2018). However, because of the MDR profile of our ST131 isolates, this might not be that easy, due to coselection by other antimicrobials and host fitness adaptation. The fitness effects of carrying the mcr-1 gene were evaluated by Wu et al. (2018). They proved that IncI2 and IncX4 mcr-1–positive plasmids conferred fitness advantage for its host. In contrast, IncHI2 plasmids imposed a slight fitness cost and competitive disadvantage.
To sum up, it seems that epidemic plasmid types IncX4 and IncHI2, which have been reported as the more prevalent carriers of mcr-1 gene, are responsible for the acquisition of the colistin resistance in the high-risk clone ST131 of E. coli in our isolates from porcine origin. Worryingly, the mcr-1 gene has also integrated an IncF plasmid from ST131. The clinical significance of this category of plasmids is therefore highlighted because they are responsible for the epidemic dissemination of ESBL resistance worldwide (Johnson et al., 2016). We hypothesize that IncF plasmids such as pLREC161_1 had acquired the mcr gene via cointegrating an IncHI2 mcr-1–harboring plasmid. The mcr-carrying IncF plasmid pMR0516mcr that has been described by McGann et al. (2016) also shared 89 kb with pHNSHP45-2 (mcr-1–carrying IncHI2 plasmid), and possibly the same phenomenon had occurred.
The impact that humans have on the environment and other species is evident. A multidisciplinary point of view, taking into account the environment, animals, and human for the surveillance of health, is given by the concept of “One Health” (Destoumieux-Garzón et al., 2018). This philosophy fits perfectly the actual global situation. Measures to reinforce prevention and proper management for overall antimicrobials might be the right strategy to fight against antibiotic resistance.
Further studies to understand why some plasmids evolve to become genetically stable are necessary to be able to anticipate the evolutionary dynamic of high risk clones.
As a limitation, it is worth mentioning that short reads obtained by WGS do not always allow a perfect genome reconstruction due to the high amount of repeated sequences in the bacterial genomes. Long read sequencing technologies will probably bring new perspectives in future researches.
Conclusion
Clade B of ST131 showed a huge genetic diversity, and five new subclades were defined (B6, B6-like, B7, B8, and B9). The majority of ST131 porcine isolates belong to new subclades B6 and B7. An association between the phylogeny, the virotype, and the origin of porcine isolates was established. Some porcine and human clinical isolates were highly related. Most porcine ST131 isolates are MDR (91%) and carry many ARGs. Colistin resistance was introduced through MGEs and had been able to stabilize chromosomally. IncX4 and IncHI2 epidemic mcr-1–harboring plasmids are responsible for acquired colistin resistance encoded by mcr-1.1 gene. The surrounding environment of the mcr-1 cassette is variable but within the same family of plasmids, insertion, and stabilization had common structures. The plasmidome of ST131 clade B is distinct from other clades within ST131 clone and contains an impressive variety of different plasmids. Characteristically ST131 porcine isolates have APEC [F2:A-:B1]–IncF plasmids. ColE1-like plasmids and IncX plasmids are also frequently observed. This behavior could be related to the clonal success due to its ability to easily rearrange DNA by horizontal gene transfer.
Data Availability Statement
The datasets of the 11 LREC genomes for this study can be found in the NCBI sequence databases as part of BioProject SUB5714329. With accession codes SAMN11936814 to SAMN11937940. Accession in NCBI is PRJNA546088.
Author Contributions
S-CF-S, MT, AM, VG, IG-M, DD-J, and AH undertook the laboratory work. MT and JB conceived the concept for the manuscript and designed the experiments. All authors provided the critical input and contributed to the writing of the manuscript and approved the final version.
Funding
This study was supported by projects: PI16/01477 from Plan Estatal de I+D+I 2013–2016, Instituto de Salud Carlos III (ISCIII), Subdirección General de Evaluación y Fomento de la Investigación and Fondo Europeo de Desarrollo Regional (FEDER); AGL2016-79343-R from the Spanish Agencia Estatal de Investigación (AEI) from the Spanish Ministerio de Economía y Competitividad (MINECO) and FEDER; and ED431C2017/57 from the Consellería de Cultura, Educación e Ordenación Universitaria of Xunta de Galicia and FEDER.
Conflict of Interest
The authors declare that the research was conducted in the absence of any commercial or financial relationships that could be construed as a potential conflict of interest.
Acknowledgments
S-CF-S acknowledges the FPU programme for her grant (FPU15/02644) from the Secretaría General de Universidades, Spanish Ministerio de Educación, Cultura y Deporte. IG-M and VG acknowledge the Consellería de Cultura, Educación e Ordenación Universitaria, Xunta de Galicia for his predoctoral grant (ED481A-2015/149) and her postdoctoral grant (ED481B2018/018), respectively. AM acknowledges the Ministerio de Educación, Cultura y Deporte (Spain) for the mobility grant PRX16/00023 for teachers and researchers from the Programa Estatal de Promoción del Talento y su Empleabilidad, Plan Estatal de Investigación Científica y Técnica y de Innovación 2013–2016. She also expresses her gratitude to Dr. Mark Achtman and his group for their invaluable help with Enterobase during the stay at the Microbiology and Infection Unit, Warwick Medical School, University of Warwick, Coventry, United Kingdom.
Supplementary Material
The Supplementary Material for this article can be found online at: https://www.frontiersin.org/articles/10.3389/fmicb.2020.00387/full#supplementary-material
Footnotes
- ^ http://enterobase.warwick.ac.uk/species/index/ecoli
- ^ https://www.ncbi.nlm.nih.gov/bioproject/
- ^ http://genomicepidemiology.org/
- ^ https://www.ncbi.nlm.nih.gov/pubmed
- ^ https://www.xlstat.com
References
AbuOun, M., Stubberfield, E. J., Duggett, N. A., Kirchner, M., Dormer, L., Nunez-Garcia, J., et al. (2017). mcr-1 and mcr-2 variant genes identified in Moraxella species isolated from pigs in Great Britain from 2014 to 2015. J. Antimicrob. Chemother. 72, 2745–2749. doi: 10.1093/jac/dkx286
Achard, A., Villers, C., Pichereau, V., and Leclercq, R. (2005). New lnu(C) gene conferring resistance to lincomycin by nucleotidylation in Streptococcus agalactiae UCN36. Antimicrob. Agents Chemother. 49, 2716–2719. doi: 10.1128/AAC.49.7.2716-2719.2005
Alikhan, N.-F., Petty, N. K., Ben Zakour, N. L., and Beatson, S. A. (2011). BLAST ring image generator (BRIG): simple prokaryote genome comparisons. BMC Genomics 12:402. doi: 10.1186/1471-2164-12-402
Alvarado, A., Garcillán-Barcia, M. P., and de la Cruz, F. (2012). A degenerate primer MOB typing (DPMT) method to classify gamma-proteobacterial plasmids in clinical and environmental settings. PLoS One 7:e40438. doi: 10.1371/journal.pone.0040438
Ben Zakour, N. L., Alsheikh-Hussain, A. S., Ashcroft, M. M., Khanh Nhu, N. T., Roberts, L. W., Stanton-Cook, M., et al. (2016). Sequential acquisition of virulence and fluoroquinolone resistance has shaped the evolution of Escherichia coli ST131. mBio 7:e00347-16. doi: 10.1128/mBio.00347-16
Borowiak, M., Fischer, J., Hammerl, J. A., Hendriksen, R. S., Szabo, I., and Malorny, B. (2017). Identification of a novel transposon-associated phosphoethanolamine transferase gene, mcr-5, conferring colistin resistance in d-tartrate fermenting Salmonella enterica subsp. enterica serovar Paratyphi B. J. Antimicrob. Chemother. 72, 3317–3324. doi: 10.1093/jac/dkx327
Camacho, C., Coulouris, G., Avagyan, V., Ma, N., Papadopoulos, J., Bealer, K., et al. (2009). BLAST+: architecture and applications. BMC Bioinformatics 10:421. doi: 10.1186/1471-2105-10-421
Campos, J., Cristino, L., Peixe, L., and Antunes, P. (2016). MCR-1 in multidrug-resistant and copper-tolerant clinically relevant Salmonella 1,4,[5],12:i:- and S. Rissen clones in Portugal, 2011 to 2015. Eurosurveillance 21:30270. doi: 10.2807/1560-7917.ES.2016.21.26.30270
Carattoli, A., Villa, L., Feudi, C., Curcio, L., Orsini, S., Luppi, A., et al. (2017). Novel plasmid-mediated colistin resistance mcr-4 gene in Salmonella and Escherichia coli, Italy 2013, Spain and Belgium, 2015 to 2016. Eurosurveillance 22:30589. doi: 10.2807/1560-7917.ES.2017.22.31.30589
Carattoli, A., Zankari, E., García-Fernández, A., Voldby Larsen, M., Lund, O., Villa, L., et al. (2014). In silico detection and typing of plasmids using plasmidfinder and plasmid multilocus sequence typing. Antimicrob. Agents Chemother. 58, 3895–3903. doi: 10.1128/AAC.02412-14
Carroll, L. M., Gaballa, A., Guldimann, C., Sullivan, G., Henderson, L. O., and Wiedmann, M. (2019). Identification of novel mobilized colistin resistance gene mcr-9 in a multidrug-resistant, colistin-susceptible Salmonella enterica serotype typhimurium isolate. mBio 10:e00853-19. doi: 10.1128/mBio.00853-19
Chandler, M., and Siguier, P. (2013). “Insertion sequences,” in Brenner’s Encyclopedia of Genetics, 2nd Edn., eds S. Maloy and K. Hughes (Amsterdam: Elsevier), 86–94. doi: 10.1016/B978-0-12-374984-0.00799-3
Chen, L., Yang, J., Yu, J., Yao, Z., Sun, L., Shen, Y., et al. (2005). VFDB: a reference database for bacterial virulence factors. Nucleic Acids Res. 33, D325–D328. doi: 10.1093/nar/gki008
Clermont, O., Christenson, J. K., Denamur, E., and Gordon, D. M. (2013). The Clermont Escherichia coli phylo-typing method revisited: improvement of specificity and detection of new phylo-groups. Environ. Microbiol. Rep. 5, 58–65. doi: 10.1111/1758-2229.12019
Dahbi, G., Mora, A., Mamani, R., López, C., Alonso, M. P., Marzoa, J., et al. (2014). Molecular epidemiology and virulence of Escherichia coli O16: H5-ST131: comparison with H30 and H30-Rx subclones of O25b: H4-ST131. Int. J. Med. Microbiol. 304, 1247–1257. doi: 10.1016/j.ijmm.2014.10.002
de Been, M., Lanza, V. F., de Toro, M., Scharringa, J., Dohmen, W., Du, Y., et al. (2014). Dissemination of cephalosporin resistance genes between Escherichia coli strains from farm animals and humans by specific plasmid lineages. PLoS Genet. 10:e1004776. doi: 10.1371/journal.pgen.1004776
de Toro, M., Fernández, J., García, V., Mora, A., Blanco, J., de la Cruz, F., et al. (2017). Whole genome sequencing, molecular typing and in vivo virulence of OXA-48-producing Escherichia coli isolates including ST131 H30-Rx, H22 and H41 subclones. Sci. Rep. 7:12103. doi: 10.1038/s41598-017-12015-0
Decano, A. G., and Downing, T. (2019). An Escherichia coli ST131 pangenome atlas reveals population structure and evolution across 4,071 isolates. Sci. Rep. 9:17394. doi: 10.1038/s41598-019-54004-5
Destoumieux-Garzón, D., Mavingui, P., Boetsch, G., Boissier, J., Darriet, F., Duboz, P., et al. (2018). The one health concept: 10 years old and a long road ahead. Front. Vet. Sci. 5:14. doi: 10.3389/fvets.2018.00014
Dobrindt, U. (2005). (Patho-)Genomics of Escherichia coli. Int. J. Med. Microbiol. 295, 357–371. doi: 10.1016/j.ijmm.2005.07.009
Du, H., Chen, L., Tang, Y. W., and Kreiswirth, B. N. (2016). Emergence of the mcr-1 colistin resistance gene in carbapenem-resistant Enterobacteriaceae. Lancet Infect. Dis. 16, 287–288. doi: 10.1016/S1473-3099(16)00056-6
Ellaby, N., Doumith, M., Hopkins, K. L., Woodford, N., and Ellington, M. J. (2019). Emergence of diversity in carbapenemase-producing Escherichia coli ST131, England, January 2014 to June 2016. Eurosurveillance 24:1800627. doi: 10.2807/1560-7917.ES.2019.24.37.1800627
Ewers, C., Göttig, S., Bülte, M., Fiedler, S., Tietgen, M., Leidner, U., et al. (2016). Genome sequence of avian Escherichia coli strain IHIT25637, an extraintestinal pathogenic E. coli strain of ST131 encoding colistin resistance determinant MCR-1. Genome Announc. 4:e00863-16. doi: 10.1128/genomeA.00863-16
Flament-Simon, S.-C., Duprilot, M., Mayer, N., García, V., Alonso, M. P., Blanco, J., et al. (2019). Association between kinetics of early biofilm formation and clonal lineage in Escherichia coli. Front. Microbiol. 10:1183. doi: 10.3389/fmicb.2019.01183
García-Meniño, I., Díaz-Jiménez, D., García, V., de Toro, M., Flament-Simon, S. C., Blanco, J., et al. (2019). Genomic characterization of prevalent mcr-1, mcr-4, and mcr-5 Escherichia coli within swine enteric colibacillosis in Spain. Front. Microbiol. 10:2469. doi: 10.3389/fmicb.2019.02469
García-Meniño, I., García, V., Mora, A., Díaz-Jiménez, D., Flament-Simon, S. C., Alonso, M. P., et al. (2018). Swine enteric colibacillosis in Spain: pathogenic potential of mcr-1 ST10 and ST131 E. coli Isolates. Front. Microbiol. 9:2659. doi: 10.3389/fmicb.2018.02659
Gupta, S. K., Padmanabhan, B. R., Diene, S. M., Lopez-Rojas, R., Kempf, M., Landraud, L., et al. (2014). ARG-annot, a new bioinformatic tool to discover antibiotic resistance genes in bacterial genomes. Antimicrob. Agents Chemother. 58, 212–220. doi: 10.1128/AAC.01310-13
Hadjadj, L., Riziki, T., Zhu, Y., Li, J., Diene, S., and Rolain, J.-M. (2017). Study of mcr-1 gene-mediated colistin resistance in Enterobacteriaceae isolated from humans and animals in different countries. Genes (Basel) 8:394. doi: 10.3390/genes8120394
Haenni, M., Poirel, L., Kieffer, N., Châtre, P., Saras, E., Métayer, V., et al. (2016). Co-occurrence of extended spectrum β lactamase and MCR-1 encoding genes on plasmids. Lancet Infect. Dis. 16, 281–282. doi: 10.1016/S1473-3099(16)00007-4
Hammerl, J. A., Borowiak, M., Schmoger, S., Shamoun, D., Grobbel, M., Malorny, B., et al. (2018). mcr-5 and a novel mcr-5.2 variant in Escherichia coli isolates from food and food-producing animals, Germany, 2010 to 2017. J. Antimicrob. Chemother. 73, 1433–1435. doi: 10.1093/jac/dky020
Hasman, H., Hammerum, A. M., Hansen, F., Hendriksen, R. S., Olesen, B., Agersø, Y., et al. (2015). Detection of mcr-1 encoding plasmid-mediated colistin-resistant Escherichia coli isolates from human bloodstream infection and imported chicken meat, Denmark 2015. Eurosurveillance 20:30085. doi: 10.2807/1560-7917.ES.2015.20.49.30085
Herrera Estévez, A. (2015). El Papel de los Alimentos en la Transmisión de Escherichia coli Potencialmente Patógenas Para el Hombre: Prevalencia y Caracterización de Cepas Diarreagénicas y Productoras de β−Lactamasas de Espectro Extendido. Available online at: http://hdl.handle.net/10347/13687 (accessed November 16, 2015).
Hojabri, Z., Darabi, N., Arab, M., Saffari, F., and Pajand, O. (2019). Clonal diversity, virulence genes content and subclone status of Escherichia coli sequence type 131: comparative analysis of E. coli ST131 and non-ST131 isolates from Iran. BMC Microbiol. 19:117. doi: 10.1186/s12866-019-1493-8
Joensen, K. G., Tetzschner, A. M. M. M., Iguchi, A., Aarestrup, F. M., and Scheutz, F. (2015). Rapid and easy in silico serotyping of Escherichia coli isolates by use of whole-genome sequencing data. J. Clin. Microbiol. 53, 2410–2426. doi: 10.1128/JCM.00008-15
Johnson, J. R., Porter, S., Johnston, B., Kuskowski, M. A., Spurbeck, R. R., Mobley, H. L. T., et al. (2015). Host characteristics and bacterial traits predict experimental virulence for Escherichia coli bloodstream isolates from patients with urosepsis. Open Forum Infect. Dis. 2:ofv083. doi: 10.1093/ofid/ofv083
Johnson, T. J., Danzeisen, J. L., Youmans, B., Case, K., Llop, K., Munoz-Aguayo, J., et al. (2016). Separate F-type plasmids have shaped the evolution of the H 30 subclone of Escherichia coli sequence type 131. mSphere 1:e121-16. doi: 10.1128/msphere.00121-16
Johnson, T. J., Wannemuehler, Y., Doetkott, C., Johnson, S. J., Rosenberger, S. C., and Nolan, L. K. (2008). Identification of minimal predictors of avian pathogenic Escherichia coli virulence for use as a rapid diagnostic tool. J. Clin. Microbiol. 46, 3987–3996. doi: 10.1128/JCM.00816-08
Kempf, I., Jouy, E., and Chauvin, C. (2016). Colistin use and colistin resistance in bacteria from animals. Int. J. Antimicrob. Agents 48, 598–606. doi: 10.1016/j.ijantimicag.2016.09.016
Kovac, J., Bakker, H., den Carroll, L. M., and Wiedmann, M. (2017). Precision food safety: a systems approach to food safety facilitated by genomics tools. TrAC Trends Anal. Chem. 96, 52–61. doi: 10.1016/j.trac.2017.06.001
Kuo, S.-C., Huang, W.-C., Wang, H.-Y., Shiau, Y.-R., Cheng, M.-F., and Lauderdale, T.-L. (2016). Colistin resistance gene mcr -1 in Escherichia coli isolates from humans and retail meats, Taiwan. J. Antimicrob. Chemother. 71, 2327–2329. doi: 10.1093/jac/dkw122
Lanza, V. F., de Toro, M., Garcillán-Barcia, M. P., Mora, A., Blanco, J., Coque, T. M., et al. (2014). Plasmid flux in Escherichia coli ST131 sublineages, analyzed by plasmid constellation network (PLACNET), a new method for plasmid reconstruction from whole genome sequences. PLoS Genet. 10:e1004766. doi: 10.1371/journal.pgen.1004766
Larsen, M. V., Cosentino, S., Rasmussen, S., Friis, C., Hasman, H., Marvig, R. L., et al. (2012). Multilocus sequence typing of total-genome-sequenced bacteria. J. Clin. Microbiol. 50, 1355–1361. doi: 10.1128/JCM.06094-11
Li, A., Yang, Y., Miao, M., Chavda, K. D., Mediavilla, J. R., Xie, X., et al. (2016). Complete sequences of mcr-1 -harboring plasmids from extended-spectrum-β-lactamase- and carbapenemase-producing Enterobacteriaceae. Antimicrob. Agents Chemother. 60, 4351–4354. doi: 10.1128/AAC.00550-16
Li, R., Xie, M., Lv, J., Wai-Chi Chan, E., and Chen, S. (2017a). Complete genetic analysis of plasmids carrying mcr-1 and other resistance genes in an Escherichia coli isolate of animal origin. J. Antimicrob. Chemother. 72, 696–699. doi: 10.1093/jac/dkw509
Li, R., Xie, M., Zhang, J., Yang, Z., Liu, L., Liu, X., et al. (2017b). Genetic characterization of mcr-1 -bearing plasmids to depict molecular mechanisms underlying dissemination of the colistin resistance determinant. J. Antimicrob. Chemother. 72, 393–401. doi: 10.1093/jac/dkw411
Li, R., Yu, H., Xie, M., Chen, K., Dong, N., Lin, D., et al. (2018). Genetic basis of chromosomally-encoded mcr-1 gene. Int. J. Antimicrob. Agents 51, 578–585. doi: 10.1016/j.ijantimicag.2017.11.015
Li, X. P., Fang, L. X., Jiang, P., Pan, D., Xia, J., Liao, X. P., et al. (2017c). Emergence of the colistin resistance gene mcr-1 in Citrobacter freundii. Int. J. Antimicrob. Agents 49, 786–787. doi: 10.1016/j.ijantimicag.2017.04.004
Liu, B.-T., Song, F.-J., Zou, M., Hao, Z.-H., and Shan, H. (2016). Emergence of colistin resistance gene mcr-1 in Cronobacter sakazakii producing NDM-9 and Escherichia coli from the same animal. Antimicrob. Agents Chemother. 61:AAC.01444-16. doi: 10.1128/AAC.01444-16
Liu, C. M., Stegger, M., Aziz, M., Johnson, T. J., Waits, K., Nordstrom, L., et al. (2018). Escherichia coli ST131- H 22 as a foodborne uropathogen. MBio 9:e00470-18. doi: 10.1128/mBio.00470-18
Liu, H., Zhou, H., Li, Q., Peng, Q., Zhao, Q., Wang, J., et al. (2018). Molecular characteristics of extended-spectrum β-lactamase-producing Escherichia coli isolated from the rivers and lakes in Northwest China. BMC Microbiol. 18:125. doi: 10.1186/s12866-018-1270-0
Liu, J., Keelan, P., Bennett, P. M., and Enne, V. I. (2009). Characterization of a novel macrolide efflux gene, mef(B), found linked to sul3 in porcine Escherichia coli. J. Antimicrob. Chemother. 63, 423–426. doi: 10.1093/jac/dkn523
Liu, X., Liu, H., Wang, L., Peng, Q., Li, Y., Zhou, H., et al. (2018). Molecular characterization of extended-spectrum β-lactamase-producing multidrug resistant Escherichia coli from swine in Northwest China. Front. Microbiol. 9:1756. doi: 10.3389/fmicb.2018.01756
Liu, Y.-Y., Wang, Y., Walsh, T. R., Yi, L.-X., Zhang, R., Spencer, J., et al. (2016). Emergence of plasmid-mediated colistin resistance mechanism MCR-1 in animals and human beings in China: a microbiological and molecular biological study. Lancet Infect. Dis. 16, 161–168. doi: 10.1016/S1473-3099(15)00424-7
Luo, J., Yao, X., Lv, L., Doi, Y., Huang, X., Huang, S., et al. (2017). Emergence of mcr-1 in Raoultella ornithinolytica and Escherichia coli isolates from retail vegetables in China. Antimicrob. Agents Chemother. 61, e01139-17. doi: 10.1128/AAC.01139-17
Madec, J. Y., and Haenni, M. (2018). Antimicrobial resistance plasmid reservoir in food and food-producing animals. Plasmid 99, 72–81. doi: 10.1016/j.plasmid.2018.09.001
Magiorakos, A. P., Srinivasan, A., Carey, R. B., Carmeli, Y., Falagas, M. E., Giske, C. G., et al. (2012). Multidrug-resistant, extensively drug-resistant and pandrug-resistant bacteria: an international expert proposal for interim standard definitions for acquired resistance. Clin. Microbiol. Infect. 18, 268–281. doi: 10.1111/j.1469-0691.2011.03570.x
Mamani, R., Flament-Simon, S. C., García, V., Mora, A., Alonso, M. P., López, C., et al. (2019). Sequence types, clonotypes, serotypes, and virotypes of extended-spectrum β-lactamase-producing Escherichia coli causing bacteraemia in a Spanish Hospital over a 12-year period (2000 to 2011). Front. Microbiol. 10:1530. doi: 10.3389/fmicb.2019.01530
Matamoros, S., van Hattem, J. M., Arcilla, M. S., Willemse, N., Melles, D. C., Penders, J., et al. (2017). Global phylogenetic analysis of Escherichia coli and plasmids carrying the mcr-1 gene indicates bacterial diversity but plasmid restriction. Sci. Rep. 7:15364. doi: 10.1038/s41598-017-15539-7
Mathers, A. J., Peirano, G., and Pitout, J. D. D. (2015). The role of epidemic resistance plasmids and international high-risk clones in the spread of multidrug-resistant Enterobacteriaceae. Clin. Microbiol. Rev. 28, 565–591. doi: 10.1128/CMR.00116-14
Matsumura, Y., Pitout, J. D. D., Peirano, G., DeVinney, R., Noguchi, T., Yamamoto, M., et al. (2017). Rapid identification of different Escherichia coli sequence type 131 clades. Antimicrob. Agents Chemother. 61, 1–9. doi: 10.1128/AAC.00179-17
McArthur, A. G., Waglechner, N., Nizam, F., Yan, A., Azad, M. A., Baylay, A. J., et al. (2013). The comprehensive antibiotic resistance database. Antimicrob. Agents Chemother. 57, 3348–3357. doi: 10.1128/AAC.00419-13
McGann, P., Snesrud, E., Maybank, R., Corey, B., Ong, A. C., Clifford, R., et al. (2016). Escherichia coli harboring mcr-1 and blaCTX-M on a novel IncF plasmid: first report of mcr-1 in the United States. Antimicrob. Agents Chemother. 60, 4420–4421. doi: 10.1128/AAC.01103-16
Mora, A., García-Peña, F. J., Alonso, M. P., Pedraza-Diaz, S., Ortega-Mora, L. M., Garcia-Parraga, D., et al. (2018). Impact of human-associated Escherichia coli clonal groups in antarctic pinnipeds: presence of ST73, ST95, ST141 and ST131. Sci. Rep. 8:4678. doi: 10.1038/s41598-018-22943-0
Mora, A., Herrera, A., López, C., Dahbi, G., Mamani, R., Pita, J.-M., et al. (2011). Characteristics of the Shiga-toxin-producing enteroaggregative Escherichia coli O104:H4 German outbreak strain and of STEC strains isolated in Spain. Int. Microbiol. 14, 121–141. doi: 10.2436/20.1501.01.142
Nicolas-Chanoine, M.-H., Bertrand, X., and Madec, J.-Y. (2014). Escherichia coli ST131, an intriguing clonal group. Clin. Microbiol. Rev. 27, 543–574. doi: 10.1128/CMR.00125-13
Nicolas-Chanoine, M. H., Blanco, J., Leflon-Guibout, V., Demarty, R., Alonso, M. P., Caniça, M. M., et al. (2008). Intercontinental emergence of Escherichia coli clone O25:H4-ST131 producing CTX-M-15. J. Antimicrob. Chemother. 61, 273–281. doi: 10.1093/jac/dkm464
Nicolas-Chanoine, M.-H., Petitjean, M., Mora, A., Mayer, N., Lavigne, J.-P., Boulet, O., et al. (2017). The ST131 Escherichia coli H22 subclone from human intestinal microbiota: comparison of genomic and phenotypic traits with those of the globally successful H30 subclone. BMC Microbiol. 17:71. doi: 10.1186/s12866-017-0984-8
Olaitan, A. O., Chabou, S., Okdah, L., Morand, S., and Rolain, J.-M. (2016). Dissemination of the mcr-1 colistin resistance gene. Lancet Infect. Dis. 16:147. doi: 10.1016/S1473-3099(15)00540-X
Olaitan, A. O., Morand, S., and Rolain, J.-M. (2014). Mechanisms of polymyxin resistance: acquired and intrinsic resistance in bacteria. Front. Microbiol. 5:643. doi: 10.3389/fmicb.2014.00643
Orlek, A., Stoesser, N., Anjum, M. F., Doumith, M., Ellington, M. J., Peto, T., et al. (2017). Plasmid classification in an era of whole-genome sequencing: application in studies of antibiotic resistance epidemiology. Front. Microbiol. 8:182. doi: 10.3389/fmicb.2017.00182
Ortiz de la Tabla, V., Ortega, A., Buñuel, F., Pérez-Vázquez, M., Marcos, B., and Oteo, J. (2017). Detection of the high-risk clone ST131 of Escherichia coli carrying the colistin resistance gene mcr-1 and causing acute peritonitis. Int. J. Antimicrob. Agents 49, 115–116. doi: 10.1016/j.ijantimicag.2016.10.003
Partridge, S. R., Di Pilato, V., Doi, Y., Feldgarden, M., Haft, D. H., Klimke, W., et al. (2018). Proposal for assignment of allele numbers for mobile colistin resistance (mcr) genes. J. Antimicrob. Chemother. 73, 2625–2630. doi: 10.1093/jac/dky262
Petty, N. K., Zakour, N. L. B., Stanton-Cook, M., Skippington, E., Totsika, M., Forde, B. M., et al. (2014). Global dissemination of a multidrug resistant Escherichia coli clone. Proc. Natl. Acad. Sci. U.S.A. 111, 5694–5699. doi: 10.1073/pnas.1322678111
Pham Thanh, D., Thanh Tuyen, H., Nguyen Thi Nguyen, T., Chung The, H., Wick, R. R., Thwaites, G. E., et al. (2016). Inducible colistin resistance via a disrupted plasmid-borne mcr-1 gene in a 2008 Vietnamese Shigella sonnei isolate. J. Antimicrob. Chemother. 71, 2314–2317. doi: 10.1093/jac/dkw173
Poirel, L., Kieffer, N., Brink, A., Coetze, J., Jayol, A., and Nordmann, P. (2016). Genetic features of MCR-1-producing colistin-resistant Escherichia coli isolates in South Africa. Antimicrob. Agents Chemother. 60, 4394–4397. doi: 10.1128/AAC.00444-16
Poirel, L., Madec, J., Lupo, A., Schink, A., Kieffer, N., Nordmann, P., et al. (2018). Antimicrobial resistance in Escherichia coli. Microbiol. Spectr. 6, 1–27. doi: 10.1128/microbiolspec.ARBA-0026-2017
Randall, L. P., Horton, R. A., Lemma, F., Martelli, F., Duggett, N. A. D., Smith, R. P., et al. (2018). Longitudinal study on the occurrence in pigs of colistin-resistant Escherichia coli carrying mcr-1 following the cessation of use of colistin. J. Appl. Microbiol. 125, 596–608. doi: 10.1111/jam.13907
Reid, C. J., McKinnon, J., and Djordjevic, S. P. (2019). Clonal ST131-H22 Escherichia coli strains from a healthy pig and a human urinary tract infection carry highly similar resistance and virulence plasmids. Microb. Genomics 5:e000295. doi: 10.1099/mgen.0.000295
Ren, J., Ahlgren, N. A., Lu, Y. Y., Fuhrman, J. A., and Sun, F. (2017). VirFinder: a novel k-mer based tool for identifying viral sequences from assembled metagenomic data. Microbiome 5:69. doi: 10.1186/s40168-017-0283-5
Roberts, M. C. (2008). Update on macrolide-lincosamide-streptogramin, ketolide, and oxazolidinone resistance genes. FEMS Microbiol. Lett. 282, 147–159. doi: 10.1111/j.1574-6968.2008.01145.x
Schink, A.-K., Kadlec, K., Kaspar, H., Mankertz, J., and Schwarz, S. (2013). Analysis of extended-spectrum- -lactamase-producing Escherichia coli isolates collected in the GERM-Vet monitoring programme. J. Antimicrob. Chemother. 68, 1741–1749. doi: 10.1093/jac/dkt123
Seemann, T. (2014). Prokka: rapid prokaryotic genome annotation. Bioinformatics 30, 2068–2069. doi: 10.1093/bioinformatics/btu153
Sekse, C., Holst-Jensen, A., Dobrindt, U., Johannessen, G. S., Li, W., Spilsberg, B., et al. (2017). High throughput sequencing for detection of foodborne pathogens. Front. Microbiol. 8:2029. doi: 10.3389/fmicb.2017.02029
Skov, R. L., and Monnet, D. L. (2016). Plasmid-mediated colistin resistance (mcr-1 gene): three months later, the story unfolds. Eurosurveillance 21:30155. doi: 10.2807/1560-7917.ES.2016.21.9.30155
Snesrud, E., He, S., Chandler, M., Dekker, J. P., Hickman, A. B., McGann, P., et al. (2016). A model for transposition of the colistin resistance gene mcr-1 by IS Apl1. Antimicrob. Agents Chemother. 60, 6973–6976. doi: 10.1128/AAC.01457-16
Snesrud, E., McGann, P., and Chandler, M. (2018). The birth and demise of the ISApl1-mcr-1-ISApl1 composite transposon: the vehicle for transferable colistin resistance. MBio 9:e02381-17. doi: 10.1128/mBio.02381-17
Sonnevend, Á, Ghazawi, A., Alqahtani, M., Shibl, A., Jamal, W., Hashmey, R., et al. (2016). Plasmid-mediated colistin resistance in Escherichia coli from the Arabian Peninsula. Int. J. Infect. Dis. 50, 85–90. doi: 10.1016/j.ijid.2016.07.007
Spurbeck, R. R., Dinh, P. C., Walk, S. T., Stapleton, A. E., Hooton, T. M., Nolan, L. K., et al. (2012). Escherichia coli isolates that carry vat, fyua, chua, and yfcv efficiently colonize the urinary tract. Infect. Immun. 80, 4115–4122. doi: 10.1128/IAI.00752-12
Stoesser, N., Mathers, A. J., Moore, C. E., Day, N. P., and Crook, D. W. (2016a). Colistin resistance gene mcr-1 and pHNSHP45 plasmid in human isolates of Escherichia coli and Klebsiella pneumoniae. Lancet Infect. Dis. 16, 285–286. doi: 10.1016/S1473-3099(16)00010-4
Stoesser, N., Sheppard, A. E., Pankhurst, L., De Maio, N., Moore, C. E., Sebra, R., et al. (2016b). Evolutionary history of the global emergence of the Escherichia coli epidemic clone ST131. MBio 7:e02162-15. doi: 10.1128/mBio.02162-15
Stoesser, N., Sheppard, A. E., Peirano, G., Sebra, R., Lynch, T., Anson, L., et al. (2016c). Complete sequencing of plasmids containing bla OXA-163 and bla OXA-48 in Escherichia coli sequence type 131. Antimicrob. Agents Chemother. 60, 6948–6951. doi: 10.1128/AAC.01130-16
Sullivan, M. J., Petty, N. K., and Beatson, S. A. (2011). Easyfig: a genome comparison visualizer. Bioinformatics 27, 1009–1010. doi: 10.1093/bioinformatics/btr039
Sun, J., Fang, L.-X., Wu, Z., Deng, H., Yang, R.-S., Li, X.-P., et al. (2017). Genetic analysis of the IncX4 plasmids: implications for a unique pattern in the mcr-1 acquisition. Sci. Rep. 7:424. doi: 10.1038/s41598-017-00095-x
Sun, J., Zhang, H., Liu, Y.-H., and Feng, Y. (2018). Towards understanding MCR-like colistin resistance. Trends Microbiol. 26, 794–808. doi: 10.1016/j.tim.2018.02.006
Tegetmeyer, H. E., Jones, S. C. P., Langford, P. R., and Baltes, N. (2008). ISApl1, a novel insertion element of Actinobacillus pleuropneumoniae, prevents ApxIV-based serological detection of serotype 7 strain AP76. Vet. Microbiol. 128, 342–353. doi: 10.1016/j.vetmic.2007.10.025
Trobos, M., Christensen, H., Sunde, M., Nordentoft, S., Agerso, Y., Simonsen, G. S., et al. (2009). Characterization of sulphonamide-resistant Escherichia coli using comparison of sul2 gene sequences and multilocus sequence typing. Microbiology 155, 831–836. doi: 10.1099/mic.0.024190-0
Vielva, L., de Toro, M., Lanza, V. F., and de la Cruz, F. (2017). PLACNETw: a web-based tool for plasmid reconstruction from bacterial genomes. Bioinformatics 33, 3796–3798. doi: 10.1093/bioinformatics/btx462
Wang, X., Wang, Y., Zhou, Y., Li, J., Yin, W., Wang, S., et al. (2018). Emergence of a novel mobile colistin resistance gene, mcr-8, in NDM-producing Klebsiella pneumoniae. Emerg. Microbes Infect. 7, 1–9. doi: 10.1038/s41426-018-0124-z
Wayne, P. (2017). Performance Standards for Antimicrobial Susceptibility Testing, 27th End (CLSI Supplement M100S). Pittsburgh, PA: Clinical and Laboratory Standards Institute.
Wu, R., Yi, L., Yu, L., Wang, J., Liu, Y., Chen, X., et al. (2018). Fitness advantage of mcr-1–bearing IncI2 and IncX4 plasmids in vitro. Front. Microbiol. 9:331. doi: 10.3389/fmicb.2018.00331
Yang, Y.-Q., Li, Y.-X., Lei, C.-W., Zhang, A.-Y., and Wang, H.-N. (2018). Novel plasmid-mediated colistin resistance gene mcr-7.1 in Klebsiella pneumoniae. J. Antimicrob. Chemother. 73, 1791–1795. doi: 10.1093/jac/dky111
Yin, W., Li, H., Shen, Y., Liu, Z., Wang, S., Shen, Z., et al. (2017). Novel plasmid-mediated colistin resistance Gene mcr-3 in Escherichia coli. mBio 8:e00543-17. doi: 10.1128/mBio.00543-17
Zankari, E., Allesøe, R., Joensen, K. G., Cavaco, L. M., Lund, O., and Aarestrup, F. M. (2017). PointFinder: a novel web tool for WGS-based detection of antimicrobial resistance associated with chromosomal point mutations in bacterial pathogens. J. Antimicrob. Chemother. 72, 2764–2768. doi: 10.1093/jac/dkx217
Zankari, E., Hasman, H., Cosentino, S., Vestergaard, M., Rasmussen, S., Lund, O., et al. (2012). Identification of acquired antimicrobial resistance genes. J. Antimicrob. Chemother. 67, 2640–2644. doi: 10.1093/jac/dks261
Zeng, K., Doi, Y., Patil, S., Huang, X., and Tian, G.-B. (2016). Emergence of the plasmid-mediated mcr-1 gene in colistin-resistant Enterobacter aerogenes and Enterobacter cloacae: TABLE 1. Antimicrob. Agents Chemother. 60, 3862–3863. doi: 10.1128/AAC.00345-16
Zhao, F., and Zong, Z. (2016). Kluyvera ascorbata carrying the mcr-1 colistin resistance gene from hospital sewage. Antimicrob. Agents Chemother. 60:AAC.1165-16. doi: 10.1128/AAC.01165-16
Zhong, L.-L., Phan, H. T. T. T., Shen, C., Vihta, K.-D., Sheppard, A. E., Huang, X., et al. (2018). High rates of human fecal carriage of mcr-1–positive multidrug-resistant Enterobacteriaceae emerge in China in association with successful plasmid families. Clin. Infect. Dis. 66, 676–685. doi: 10.1093/cid/cix885
Zurfluh, K., Klumpp, J., Nüesch-Inderbinen, M., and Stephan, R. (2016a). Full-length nucleotide sequences of mcr-1 -harboring plasmids isolated from extended-spectrum-β-lactamase-producing Escherichia coli isolates of different origins. Antimicrob. Agents Chemother. 60, 5589–5591. doi: 10.1128/AAC.00935-16
Keywords: Escherichia coli, ST131, swine, colistin, mcr-1.1, core genome, plasmidome
Citation: Flament-Simon S-C, de Toro M, Mora A, García V, García-Meniño I, Díaz-Jiménez D, Herrera A and Blanco J (2020) Whole Genome Sequencing and Characteristics of mcr-1–Harboring Plasmids of Porcine Escherichia coli Isolates Belonging to the High-Risk Clone O25b:H4-ST131 Clade B. Front. Microbiol. 11:387. doi: 10.3389/fmicb.2020.00387
Received: 17 December 2019; Accepted: 24 February 2020;
Published: 24 March 2020.
Edited by:
Benjamin Andrew Evans, University of East Anglia, United KingdomReviewed by:
Catherine M. Logue, University of Georgia, United StatesChitrita Debroy, Pennsylvania State University (PSU), United States
Copyright © 2020 Flament-Simon, de Toro, Mora, García, García-Meniño, Díaz-Jiménez, Herrera and Blanco. This is an open-access article distributed under the terms of the Creative Commons Attribution License (CC BY). The use, distribution or reproduction in other forums is permitted, provided the original author(s) and the copyright owner(s) are credited and that the original publication in this journal is cited, in accordance with accepted academic practice. No use, distribution or reproduction is permitted which does not comply with these terms.
*Correspondence: Jorge Blanco, am9yZ2UuYmxhbmNvQHVzYy5lcw==
†These authors have contributed equally to this work