- 1Research School of Biology, ACT, Australian National University, Canberra, ACT, Australia
- 2Department of Oral Biology and Biomedical Sciences, MAHSA University, Selangor, Malaysia
- 3School of Molecular Sciences, University of Western Australia, Perth, WA, Australia
- 4Graham Centre for Agricultural Innovation, Charles Sturt University, Wagga Wagga, NSW, Australia
- 5Plus 3 Australia Pty Ltd., Hawker, ACT, Australia
Septoria nodorum blotch is a major disease of wheat caused by the fungus Parastagonospora nodorum. Recent studies have demonstrated that secondary metabolites, including polyketides and non-ribosomal peptides, produced by the pathogen play important roles in disease and development. However, there is currently no knowledge on the composition or biological activity of the volatile organic compounds (VOCs) secreted by P. nodorum. To address this, we undertook a series of growth and phytotoxicity assays and demonstrated that P. nodorum VOCs inhibited bacterial growth, were phytotoxic and suppressed self-growth. Mass spectrometry analysis revealed that 3-methyl-1-butanol, 2-methyl-1-butanol, 2-methyl-1-propanol, and 2-phenylethanol were dominant in the VOC mixture and phenotypic assays using these short chain alcohols confirmed that they were phytotoxic. Further analysis of the VOCs also identified the presence of multiple sesquiterpenes of which four were identified via mass spectrometry and nuclear magnetic resonance as β-elemene, α-cyperone, eudesma-4,11-diene and acora-4,9-diene. Subsequent reverse genetics studies were able to link these molecules to corresponding sesquiterpene synthases in the P. nodorum genome. However, despite extensive testing, these molecules were not involved in either of the growth inhibition or phytotoxicity phenotypes previously observed. Plant assays using mutants of the pathogen lacking the synthetic genes revealed that the identified sesquiterpenes were not required for disease formation on wheat leaves. Collectively, these data have significantly extended our knowledge of the VOCs in fungi and provided the basis for further dissecting the roles of sesquiterpenes in plant disease.
Introduction
The Dothideomycete fungus Parastagonospora nodorum is the causal agent of Septoria nodorum blotch, a significant foliar global disease of wheat. Once considered a simplistic pathogen that caused disease through the secretion of lytic enzymes, seminal studies over the last decade have demonstrated that P. nodorum facilitates disease through the use of small proteins called effectors (Oliver et al., 2012). To date, three effectors from P. nodorum have been described, ToxA, Tox1 and Tox3 (Friesen et al., 2006; Liu et al., 2009, Liu et al., 2012). Each of these proteins interact in a gene-for-gene for manner with specific cognate susceptibility genes in the host leading to host cell death and disease. More recent studies have demonstrated that as well as inducing necrosis, each of these effectors appears to also function in repressing host defence responses highlighting the complex nature of this interaction (Breen et al., 2016; Liu et al., 2016, McDonald and Solomon, 2018).
However, it has been recently shown that ToxA, Tox1, and Tox3 are not the only molecules responsible for P. nodorum to successfully infect wheat (Tan et al., 2014, 2015). Tan et al. (2015) used a reverse genetic approach to generate a strain of P. nodorum lacking each of the effector genes and showed that the resulting mutant, albeit being less pathogenic, retained the ability to cause disease (Tan et al., 2015). Indeed, recent studies have examined the role of several polyketide secondary metabolites synthesized by P. nodorum and shown that some have a role in facilitating disease on wheat (Chooi et al., 2015a, b, 2017, Li et al., 2018). However, there are many more secondary metabolites encoded for within the P. nodorum genome that potentially play a role in the interaction of the pathogen with its host (Chooi et al., 2014; Muria-Gonzalez et al., 2015).
Another group of molecules that have yet to be characterized in terms of their role or requirement in septoria nodorum blotch are the volatile organic compounds (VOCs). VOCs are small carbon-based molecules that readily evaporate and are ubiquitously produced by most forms of life (Kesselmeier and Staudt, 1999). It has been proposed that VOCs play important roles as signals in inter and intra-organismic interactions which surpasses the involvement of other diffusible molecules (Kanchiswamy et al., 2015). Microorganisms are known to be a rich source of VOCs displaying antibacterial, antifungal, and phytotoxic properties, but also acting as chemical cues that help structuring microbial communities (Kanchiswamy et al., 2015). VOCs typically produced by microorganisms are complex blends of chemicals. The composition and role of VOCs though in fungi, particularly plant pathogens, are poorly understood.
To address this knowledge gap, we firstly explored the biological activity of the VOCs emitted by P. nodorum and undertook an initial identification and characterization of the major components. As a result of this, several sesquiterpene molecules were identified and the genes required for their synthesis characterized. This study has shed further light on the chemical diversity synthesized by these fungi and has raised further questions as to the roles of small molecules generated by this devastating wheat pathogen.
Materials and Methods
Volatile Compound-Mediated Growth Competition Assays
To test potential activities of P. nodorum VOCs, four fungi were used for growth antagonist assays: P. nodorum, Fusarium oxysporum f. sp. lycopersici, Eutiarosporella tritici-australis and Zymoseptoria tritici. Escherichia coli, Pseudomonas syringae, Sinorhizobium meliloti, and three other bacteria isolated from within surface sterilized wheat seeds, Bacillus cereus, Sphingobacterium multivorum, and Flavobacterium sp. were also used for the growth assays.
In one side of a segmented Petri dish (9 cm diameter), 25 μl of a P. nodorum spore solution (1 × 106 spores/ml) was inoculated on Fries agar (1.5%) (Supplementary Table S1) and incubated at 22°C in 12-12 h dark and light. After two weeks, the other compartment of the segmented Petri dish was inoculated with the test organisms. The fungi were inoculated onto potato dextrose agar (PDA), the bacteria on Lysogeny broth (LB) agar. Water agar (1%) was used for the germination of wheat (Triticum aestivum cv. Grandin) and Medicago truncatula for which 8 and 10 seeds per plate were used respectively (Supplementary Table S1). Plates were sealed with parafilm after the test organisms were inoculated. The effect of the VOCs was then visually monitored daily.
To assess the phytotoxic and fungistatic effect of 2-methyl-1-propanol, 2-methyl-1-butanol, 3-methyl-1-butanol and 2-phenylethanol, each of the compounds was placed on a 1 cm2 filter paper on a section of a segmented Petri dish. On the other half of the dish was placed either wheat seeds or P. nodorum was inoculated, on the appropriate medium as described above. An additional treatment was also performed containing a mix of these compounds in the proportions found in the chromatographic analysis of P. nodorum VOCs. 1 mM of each of the pure compounds (74 to 122 ppm) and 100 ppm for the mix were used in these assays, considering a free internal volume of the petri dish of 48.9 cm3 (the total volume minus 15 ml of test medium). Plates were sealed using parafilm immediately after the compound was added, however it cannot be excluded that the molecules did not undergo some degree of diffusion through the membrane.
Each experiment was repeated twice using at least three Petri dishes per treatment. The number of seeds germinated per plate as well as radicle and coleoptile lengths were recorded and the average and standard deviation were calculated. T-tests were performed comparing each treatment against the control to determine statistical significance. Qualitative data was not statistically evaluated.
Volatile Molecule Analysis
To identify the individual components of the VOCs, slanted Fries agar head-space (HS) vials (20 ml) were inoculated with P. nodorum. Vials with cotton stoppers were incubated for one week at 22°C in 12-12 h dark and light cycles. Vials were sealed with silicon/Teflon septa crimp caps 24 h prior to the analysis. Three mock-inoculated vials were used as controls. To calculate the retention indices, 5 μl of an alkane mix at 20 ppm in CH2Cl2 was added to a HS vial (Kováts, 1958; van Den Dool and Dec Kratz, 1963). To confirm the identity of ethyl acetate, 2-methyl-1-propanol, 2-methyl-1-butanol, 3-methyl-1-butanol and 2-phenylethanol within the fungal VOCs, a mix following the proportions found in solid phase micro-extractions in line with a gas chromatography-mass spectrometry (SPME-GC-MS) analysis of the fungal cultures (10:14:26:53:11 respectively) was prepared using pure compounds and 1 μl of the mix was added to a HS vial.
To evaluate the in planta production of sesquiterpenes, the distal 5 cm of the second leaf of 2-week old wheat seedlings were excised and sprayed with a 1 × 106 P. nodorum spores/ml solution containing 0.02% tween 20. The cut end of each leaf was embedded in a HS vial containing 2 ml of water agar (1%). Vials were closed with silicon/Teflon septa crimp caps and incubated for 3 days at 22°C in 12-12 h dark and light cycles. Three mock-inoculated samples were used as controls.
The SPME-GC-MS analyses were performed in an Agilent 7890A gas chromatograph coupled to a single quadrupole Agilent 5975 mass spectrometer using a Gerstel MPS 2XL autosampler. The column for the analyses was a Varian CP9013-1Factor 4 5 m s 350°C: 40 m x 250 (μm x 0.25 (μm. Elution was performed with He flow at 1.5 ml/min and temperature programed from 40°C (hold 3 min) to 180°C at 4°C/min and then to 220°C (hold 5 min) at 10°C/min. The mass spectrometer was operated in the electron ionization (EI) mode with ionization energy of 70 eV and scanning the mass range of m/z 40-600. Temperatures were set to: GC inlet, 240°C; GC transfer line, 240°C; MS source, 200°C; and quadrupole 250°C. Volatiles were adsorbed onto a SPME fiber coated with divinylbenzene/carboxen/polydimethylsiloxane (DVB/CAR/PDMS) (1 cm, 23 Ga, 50/30 μm film thickness, Supelco) for 120 min at 30°C after a 5 min equilibration. The fiber was desorbed in the injector at 240°C (splitless mode 2 min). The fibers were conditioned by keeping them in the GC injector at 240°C for 10 min.
All GC-MS experiments were done in triplicate. Data was acquired using MSD ChemStation E.02.01.1177 (© Agilent Technologies, Inc.). Analysis of the data was performed using ChemStation and MS Search NIST Mass Spectral Search Program [Version 2.0g] for the NIST/EPA/NIH Mass Spectral Library [NIST Standard Reference Database 1A Version NIST 11] build May 19 2011 (© National Institute of Standards and Technology).
Disruption of P. nodorum Sesquiterpene Synthases
Parastagonospora nodorum sesquiterpene synthases (Sts), SNOG_03562 (Sts1), and SNOG_04807 (Sts2), were individually disrupted in P. nodorum wild type (SN15) by split marker homologous recombination of a phleomycin resistance cassette. 1.5 Kb 5’ and 3’ flanking regions for each gene were amplified from P. nodorum genomic DNA (primers in Supplementary Table S2). The phleomycin resistance gene was amplified as two overlapping amplicons, Phl and Leo, from the pAN8-1 plasmid (primers in Supplementary Table S2) (Mattern et al., 1988). 5’ flanks were PCR fused to Leo while 3’ flanks were PCR fused to Phl (primers in Supplementary Table S2). P. nodorum was then transformed by a PEG-protoplast method as previously reported (Solomon et al., 2004).
To assess the copy number of the phleomycin cassette in the transformants, qRT-PCR primers were designed for the phleomycin resistance gene; elongation factor 1α, actin and SnToxA primers were used to normalize the data (primers in Supplementary Table S2). As a phleomycin single copy reference, a tox3 knock out strain was used (Tan et al., 2014).
Characterization of P. nodorum Sesquiterpenes
To isolate and characterize the product of Sts1 and Sts2, the coding sequences were cloned into the linearized plasmid backbone (XW55) from YEplac-ADH2p (primers in Supplementary Table S2) (Lee and DaSilva, 2005, Chooi et al., 2015a). In vivo yeast recombination cloning using each gene and XW55 was performed with the Frozen-EZ Yeast Transformation IITM Kit (Zymo Research, Irvine, CA) and competent Saccharomyces cerevisiae BJ5464-NpgA according to manufacturer’s protocol. Positive transformants were selected by PCR from colonies grown on synthetic dropout agar lacking uracil.
Medium scale P. nodorum fermentations for the isolation of α-cyperone were performed using 8 l of liquid Fries medium inoculated with 4 × 106 spores. Cultures were incubated in the dark during 10 days at 22 °C and shaking at 120 rpm.
For the isolation of acora-4,9-diene, and eudesma-4,1-diene and β-elemene, transformed S. cerevisiae harboring Sts1 or Sts2 were inoculated in in 6 ml synthetic dropout agar lacking uracil (Supplementary Table S1) for 72 h at 28°C 200 rpm. Each of these seed cultures was used to inoculate 5 l YPD broth (Supplementary Table S1) and incubated 90 h at 22°C at 200 rpm.
Fungal cultures were lyophilized and low to medium polarity compounds were extracted with dichloromethane. Yeast cultures were centrifuged and the cells subjected to acetone extraction. Both, dichloromethane and acetone were evaporated in a rotary evaporator. The sesquiterpenes from the extracts were isolated by acetonitrile/hexane partition. Sesquiterpenes were purified by SiO2 hexane flash chromatography followed by C18 water/acetonitrile flash chromatography. Purity of terpenes was assessed by GC-MS. Isopentane was used to recover the terpenes from the water-acetonitrile mixture.
GC-MS2 was performed to identify β-elemene and α-cyperone by comparison with standards in a Finnigan TraceGC ultra (Thermo Scientific) coupled to an iontrap Finnigan Polaris Q (Thermo Scientific) mass spectrometer. β-elemene was injected onto a BPX70 30 m × 0.25 mm id (SGE Analytical Science) while α-cyperone in a Varian CP9013-1Factor4 5 ms column, which were eluted with He (inlet pressure 15 psi; injection port 200°C; interface 250°C; source 200°C). For β-elemene the column was temperature programed from 60°C (hold 1 min) to 100°C at 25oC/min, then to 150 at 10 oC/min, and finally to 240°C at 10°C/min (hold 3.5 min); for α-cyperone the program started at 60°C (hold 1 min) to 200°C at 30°C/min, then to 220 at 3°C/min, and finally to 325°C at 30°C/min (hold 1 min). The mass spectrometer was operated in the electronic ionization (EI) mode with ionization energy of 70 eV, scanning the mass range of m/z 50–450. For MS/MS experiments, the precursor ions were selected with a peak width of 1.0 amu over 12ms. The ions were excited at 1 V for 15 ms with q = 0.3 and the products scanned over a mass range of m/z 100–250. Data analysis was performed employing XcaliburTM 1.4 (©Thermo Scientific) software.
The eudesma-4,11-diene (1) and the acora-4,9-diene (4) were dissolved in CDCl3 and analyzed by NMR. 1H NMR, 13C NMR, HSQC, and HMBC were performed in an AvanceTMIII HD 300 MHz NanoBay NMR device (Bruker).
P. nodorum Infection Assays
To test the requirement of Sts genes for infection, the mutants of P. nodorum lacking the Sts1 and Sts2 genes were inoculated onto the second leaf of two-week-old wheat seedlings (cv. Axe) which was attached to a styrofoam platform using double sided sticky tape and spayed with a spore solution (1 × 106 spores/ml containing 0.02% tween 20). 0.02% tween 20 was used as control. Inoculated seedlings were incubated for 48 h at 22°C in a dark moisture chamber. After the initial 48 h incubation, the inoculated plants were grown at 85% humidity, 20°C during the day and 12°C at night with 16-8 h light/dark cycles. The leaves were collected at five days post inoculation to evaluate the disease. These assays were performed twice with a minimum of four leaves per treatment used.
Results
Parastagonospora nodorum VOCs Have Phytotoxic, Antibiotic, and Self-Inhibitory Properties
To assess if volatile emissions of P. nodorum harbor bioactive VOCs, split plate assays were performed to evaluate a series of biological activities including phytotoxicity, fungitoxicity and bactericidal (Figure 1). Segmented Petri dishes were used to prevent molecules diffusing through the media and ensure that any observable activities could be solely attributed to the volatile compounds. To assay for phytotoxicity, seeds from the host of P. nodorum, wheat cv. Grandin, and a dicotyledonous plant, Medicago truncatula, were used. P. nodorum VOCs had a strong effect on the radicle elongation and hypocotyl or coleoptile growth which were significantly reduced when the seeds were germinated in the presence of P. nodorum but appeared unaffected in the control plates (no fungal inoculation). The effect on bacteria was mixed as there was no observable impact on the growth of a variety of different strains including Escherichia coli, Pseudomonas syringae, Bacillus cereus, or Flavobacterium sp. in the presence of the fungal VOCs (Supplementary Figure S1). In contrast, there was a strong reduction in the growth of the nitrogen-fixing bacterium Sinorhizobium meliloti and also Sphingobacterium multivorum when P. nodorum was cultured in the same Petri dish (Figure 1). There was no apparent impact on the growth of any of the fungi tested when grown with P. nodorum with the exception of the apparent self-inhibition of P. nodorum growth (by its own VOCs) (Figure 1 and Supplementary Figure S1).
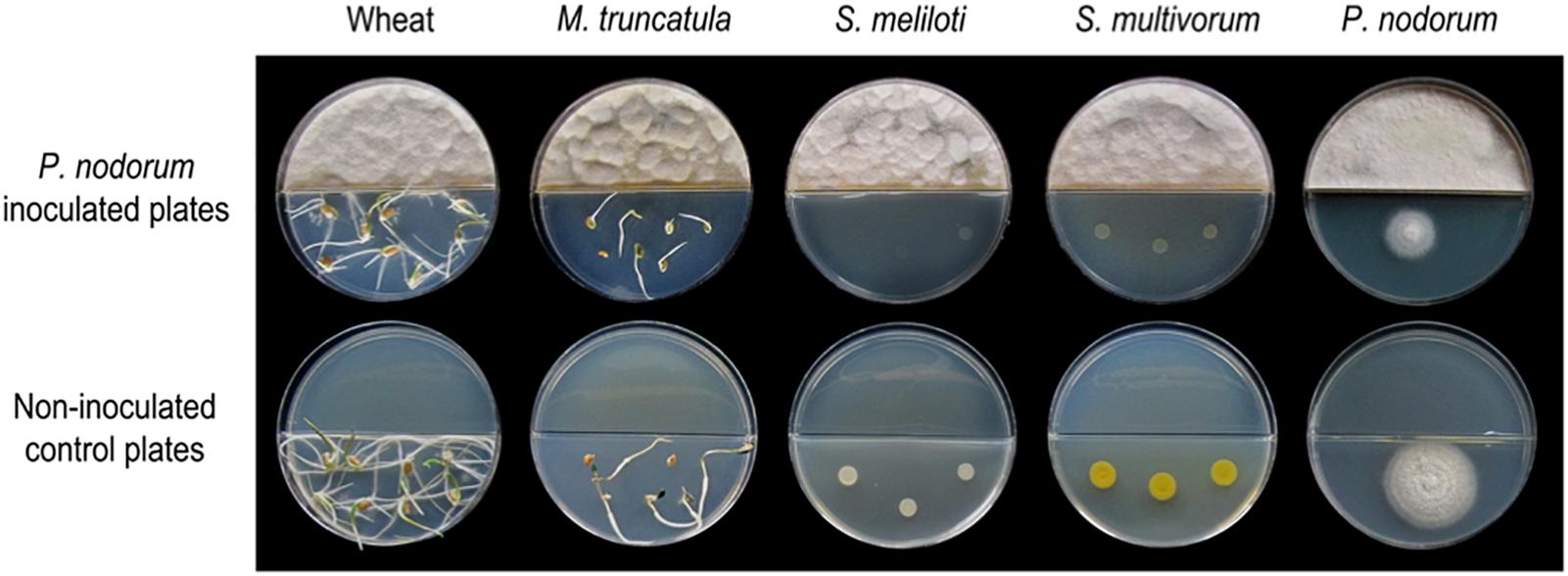
Figure 1. Split plate assays of the effect of VOCs produced by wild type P. nodorum (SN15). The top row of plates show the inhibition caused by P. nodorum VOCs on wheat and Medicago seeds, S. meliloti, S. multivorum, and P. nodorum. The bottom row of plates shows the growth of each of the test organisms in the absence of P. nodorum.
The Major P. nodorum VOCs Are Short Chain Alcohols
To dissect the chemical basis of the bioactivities described above, the identities of the volatile molecules were determined using a combination of solid phase micro-extractions (SPME) from the headspace (HS) of ten days old fungal cultures in slanted Fries agar vials and subsequent analysis by gas chromatography-mass spectrometry (GC-MS) and spectral comparison against pure standard and the NIST library. Within the P. nodorum VOCs mixture, several alcohols and esters were identified as being the most prominent signals (percentage of area of the whole chromatogram) (Table 1): 3-methyl-1-butanol (representing 5.36% of the VOCs mixture), 2-methyl-1-butanol (2.6%), 2-methyl-1-propanol (1.43%) and 2-phenylethanol (1.13%). Many other volatile molecules were also identified in peaks with smaller areas. The polyketide mellein (0.9%) was also detected along with some sesquiterpenes of which two were putatively identified as β-elemene and eudesma-4,11-diene.
The Four Most Abundant P. nodorum VOCs Are Phytotoxic
The activity of the four most prominent volatile molecules identified in the chromatograms from the head space of P. nodorum (3-methyl-1-butanol, 2-methyl-1-butanol, 2-methyl-1-propanol, 2-phenylethanol) were assayed to assess their impact on the growth of P. nodorum and wheat seedling development. These compounds were tested independently at an atmospheric concentration of 1 mM. Additionally, a mixture of these compounds following the in vitro proportions, was prepared and tested at 100 ppm. Neither the independent pure VOCs nor the mixture had any effect on P. nodorum growth suggesting that these molecules are not responsible for the inhibitory effect described above (data not shown). In contrast, a 27% decrease in germination was observed when wheat seeds were exposed to 3-methyl-1-butanol, although no other treatment had a significant effect on germination (Figure 2). However, both radicle and coleoptile elongation were repressed in all treatments; 3-methyl-1-butanol showed the greatest inhibition (100% and 83% respectively) while 2-methyl-1-propanol showed the least inhibition (36% and 31% respectively).
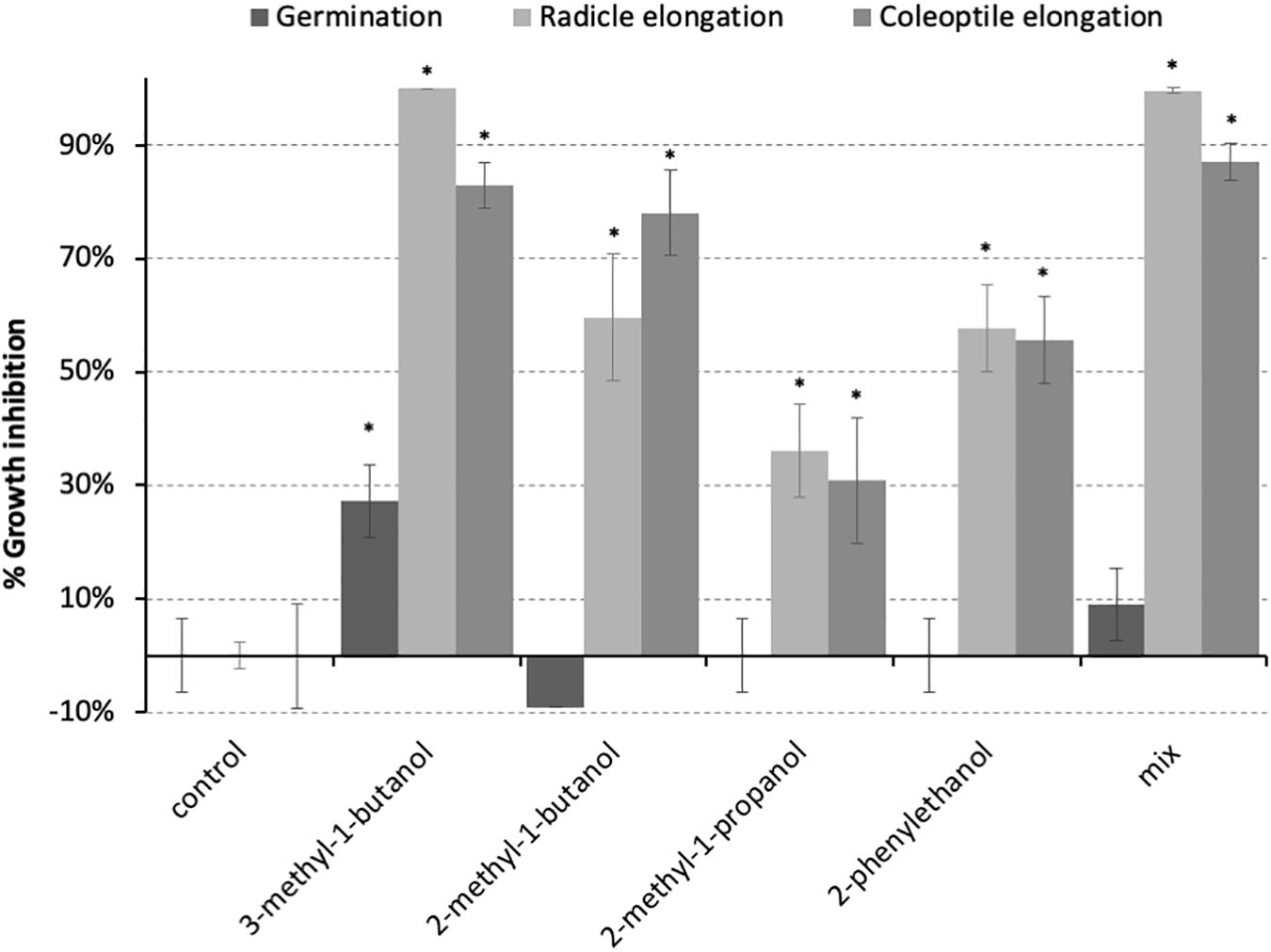
Figure 2. Inhibition of wheat seedling development by the predominant VOCs produced by P. nodorum. The bars represent the inhibitory activity on the developmental stage. 3-methyl-1-butanol, 2-methyl-1-butanol, 2-methyl-1-propanol, and 2-phenylethanol were tested at 1 mM. Error bars show the standard deviation and asterisks indicate statistically significant differences compared to the control (p < 0.05).
In planta Production of Sesquiterpenes
In addition to the bioactive short chain alcohols, we were also interested in the presence of the sesquiterpenes found in the axenic culture VOCs. To determine if these molecules played a potential role in disease development, the production of sesquiterpenes was assayed for during infection and compared to those produced in axenic culture. VOCs were extracted from vials containing either infected leaves or the fungus grown axenically and analyzed by HS-SPME-GC-MS. Interestingly the same sesquiterpenes produced in vitro by P. nodorum were also found in planta as well as others not previously observed (Figure 3). Eudesma-4,11-diene (sesquiterpene 1), β-elemene (sesquiterpene 2) and α-cyperone (sesquiterpene 3) were putatively identified by comparing the acquired data against the NIST database. Another interesting compound was sesquiterpene 4, the most abundant sesquiterpene detected from P. nodorum, in Fries cultures and in wheat leaves. However, despite a fragmentation pattern and molecular weight typical of a sesquiterpene, its match against entries present in the NIST library was not high enough to confidently assign a possible identity.
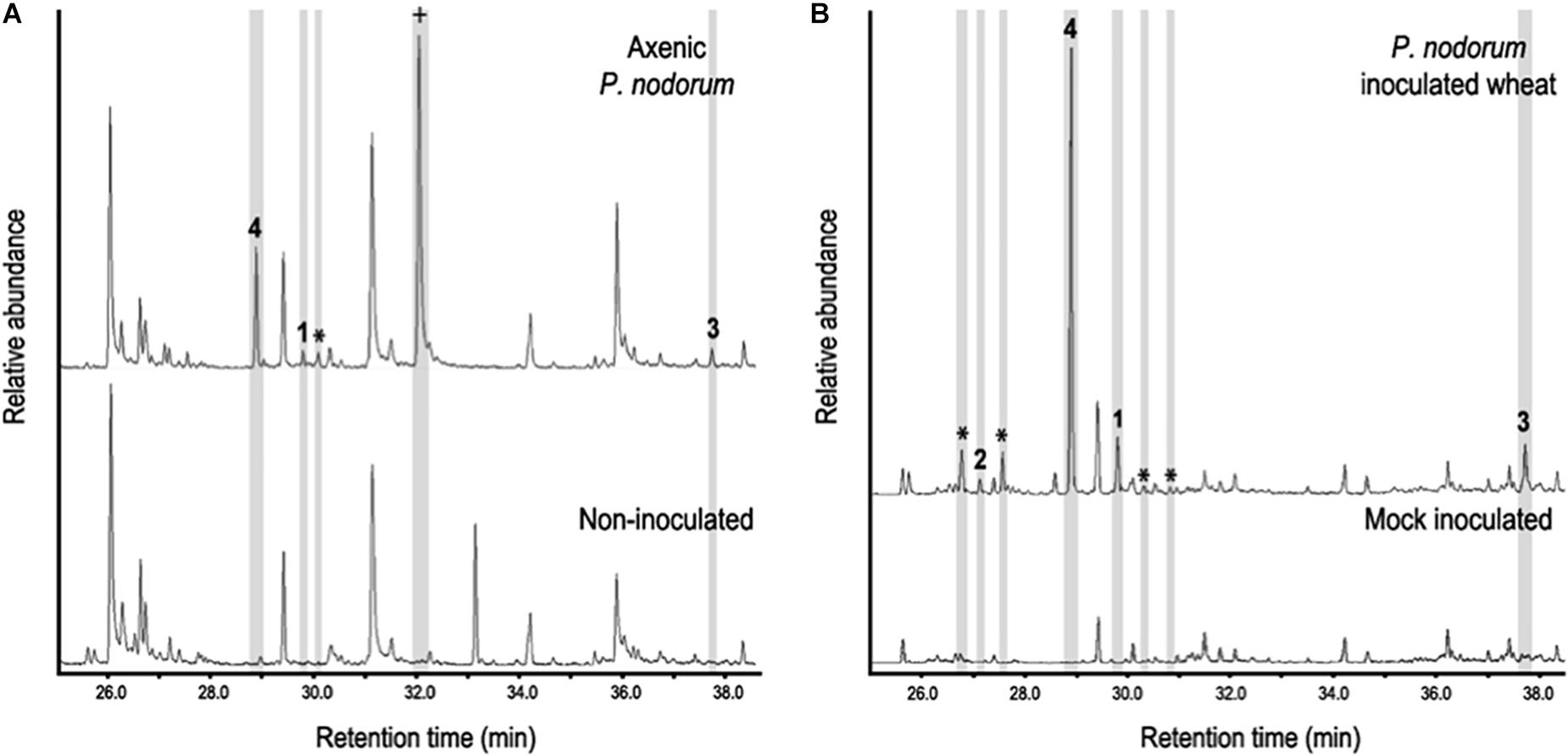
Figure 3. (A) Sesquiterpene profile of P. nodorum growing on Fries media (upper panel) and uninoculated Fries media (lower panel). (B) The sesquiterpene profile of wheat leaves infected with P. nodorum (upper panel) and mock inoculated wheat leaves (low panel). Compounds 1-4 are shown and other sesquiterpenes peaks are stared (*). Mellein is indicated as a cross (+).
The Biosynthetic Genes of P. nodorum Sesquiterpenes
The P. nodorum genome encodes three sesquiterpene synthases (Chooi et al., 2014), Sts1, Sts2, and Sts3. Previous studies have demonstrated that Sts1 and 2 are expressed during infection, but not Sts3. Furthermore, analysis of the Sts3 gene sequence revealed that it appears truncated implying that it isn’t functional and thus it was not considered for further study (Ipcho et al., 2012). To directly link the molecules identified above to the genes, Sts1 and Sts2 were disrupted individually in the P. nodorum genome through homologous recombination. Disruption cassettes were constructed to independently replace Sts1 and Sts2 with a phleomycin resistance marker. P. nodorum was transformed and positive colonies were selected from phleomycin-containing plates. Correct disruption of the genes was verified by PCR and strains containing a single copy of the disruption cassette were selected by qPCR.
Single copy transformants of P. nodorum strains lacking Sts1 (sts1) and Sts2 (sts2) were selected for further analysis by HS-SPME-GC-MS (Figure 4). The signals of sesquiterpenes 1, 2, and 3 along with three other unidentified sesquiterpenes present in wild-type P. nodorum were absent in the sts2 mutants indicating this gene codes for the core biosynthetic enzyme of the three putative sesquiterpenes plus some other sesquiterpene structures (same mass and similar fragmentation pattern). The mutants lacking sts1 were missing three unidentified compounds putatively identified as sesquiterpenes, including sesquiterpene 4, suggesting that Sts1 is responsible for its synthesis.
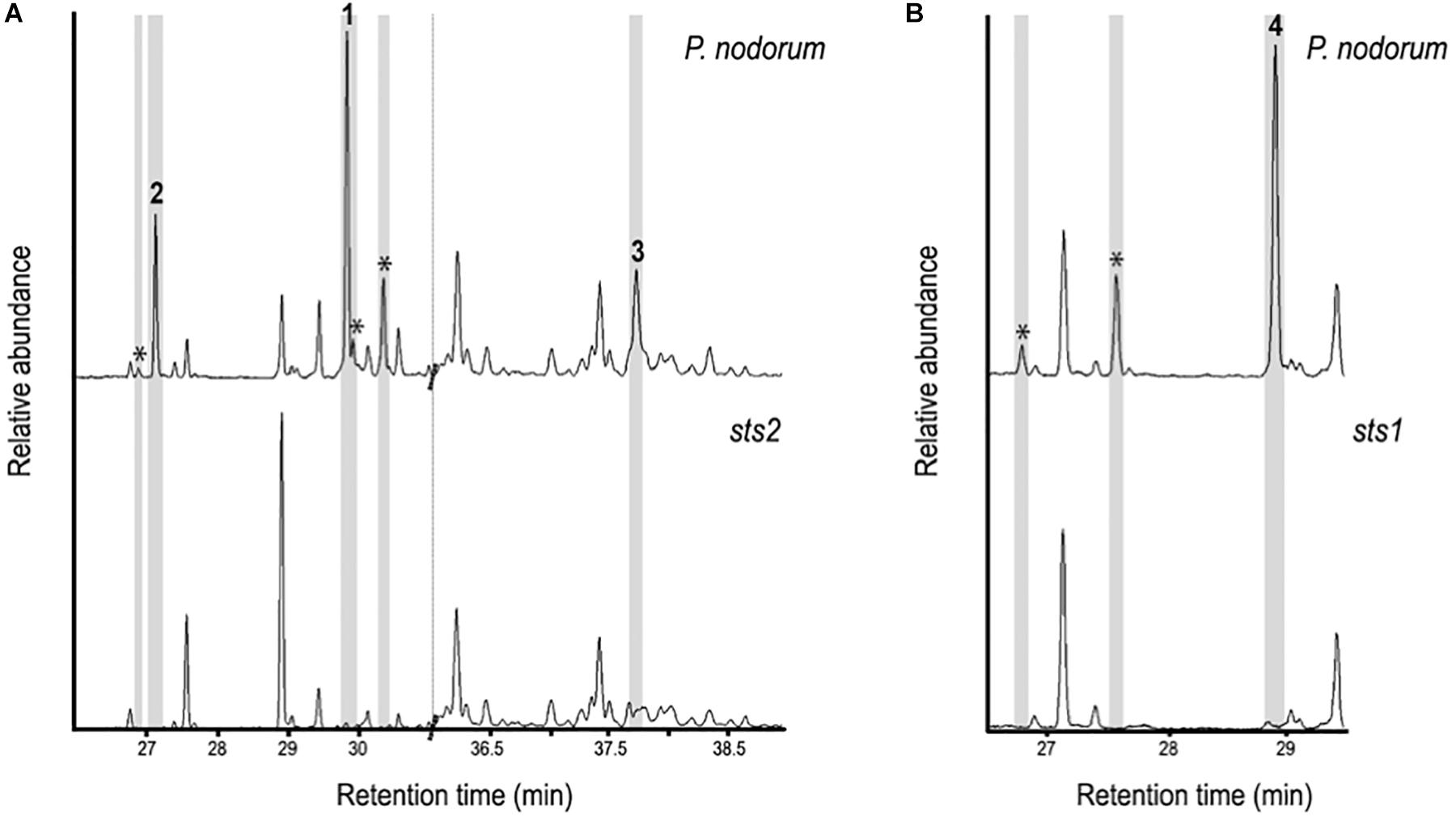
Figure 4. GC-MS chromatograms comparing sesquiterpenes in the extracts from wild-type P. nodorum to mutant strains lacking Sts2 (A) and Sts1 (B). The presence of 1 – 4 in the wild-type strain is highlighted as well as other sesquiterpenes peaks which are highlighted (*).
Heterologous Expression of Sts1 and Sts2 Reveals Its Prolificity and Allows the Sesquiterpenes Isolation
To confirm the identity of these sequiterpenes, an isolation from medium scale fermentation of P. nodorum in Fries media was undertaken. Sesquiterpene 3 was isolated by silica flash chromatography followed by C18 flash chromatography. However, the isolation of sesquiterpenes 1, 2, and 4 was not achieved. Consequently, the Sts1 and Sts2 genes were heterologously expressed in yeast to confirm 1 and 2 as eudesma-4,11-diene and β–elemene respectively. Acetone extracts from small scale cultures of the yeast strains harboring the sesquiterpene synthases were analyzed by GC-MS and the production of 1, 2, and 4 by the heterologous expression of Sts2 and Sts1 was confirmed (Figure 5). Interestingly, sesquiterpene 3 (putatively α-cyperone) was not detected, suggesting it is modified post synthesis.
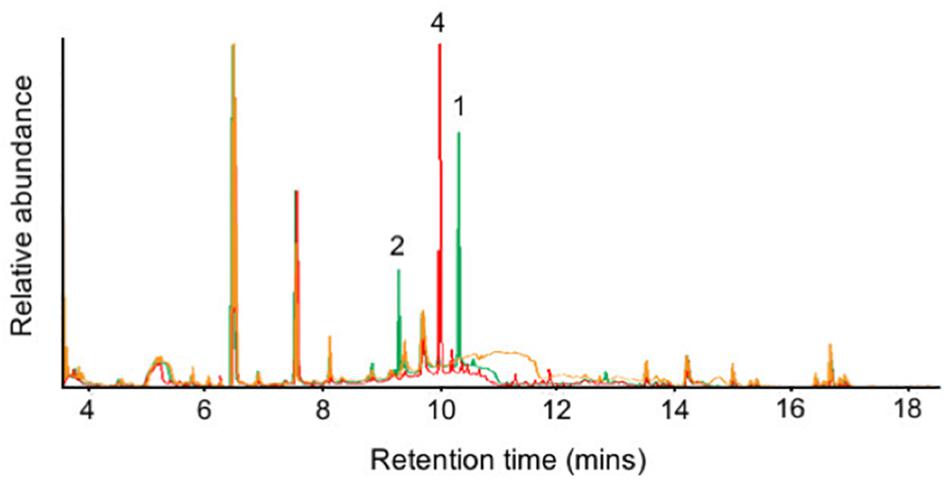
Figure 5. GC-MS chromatograms of the extracts from the Sts1 and Sts2 heterologous expression in yeast. Overlapped chromatograms of extracts from yeast harbouring Sts1 (red), Sts2 (green) and an empty vector (orange). The presence of 1, 2, and 4 is indicated.
Sesquiterpenes 1 and 2, and 4 were isolated by silica followed by C18 flash chromatography of acetone extracts from medium scale YPDA fermentations of yeast carrying Sts2 and Sts1 respectively. Subsequent GC-MS analysis of the sesquiterpene fractions confirmed that 4 is the major product of Sts1 but also that 14 other unidentified terpenes are synthesized by the same enzyme (Supplementary Figure S2). Similarly, the predominant sesquiterpene produced by Sts2 is 1 along with 10 other molecules including compound 2 (Supplementary Figure S3).
The Identity of the P. nodorum Sesquiterpenes Are Confirmed by MS2 and NMR
Commercial standards of β-elemene and α-cyperone were purchased and analyzed by GC-MS2 to confirm the identities of sesquiterpenes 2 and 3. Identical retention times in addition to a comparison of the MS2 fragmentation profiles that demonstrated a complete overlap of the major ions present in the standards compared to the extracted samples confirmed the identities of 2 and 3 (Figure 6 and Supplementary Figures S4, S5).
The isolated sesquiterpene 1, the putative eudesma-4,1-diene, and unknown sesquiterpene 4 were subjected to 1H NMR, 13C NMR, HMBC and HSQC experiments (Supplementary Figures S6–S13). Supplementary Tables S3, S4 present the assignments and chemical shifts (δ) for all carbons and the corresponding hydrogens as well as the correlations obtained from the HMBC experiment for sesquiterpene 1 and sesquiterpene 4 respectively. The identity of sesquiterpene 1 was confirmed to be eudesma-4,11-diene, and sesquiterpene 4 is proposed to be acora-4,9-diene (Figure 6) based on comparison to previously reported NMR data (Iwabuchi et al., 1989; Chen and Lin, 1993).
Sts1 and Sts2 Are Not Required for Bacterial Growth Inhibition, Phytotoxicity or for the Infection of Wheat by P. nodorum
A segmented plate growth assay was used to determine if the molecules derived from either Sts1 or Sts2 are involved in the biological activities described above. As previously observed, volatiles from wild type P. nodorum reduced wheat seed germination and inhibited the growth of S. multivorum as well as self-growth. The growth of both the sts1 or sts2 mutants caused the same phenotypic effects as the wild type inferring that the products of either gene are not responsible for these inhibitory activities (data not shown).
The role of the Sts1 and Sts2 terpenes in pathogenicity was evaluated by inoculating the second leaf of two-week-old wheat seedlings with wild type P. nodorum and each of the mutants. Symptom development for the sts1 and sts2 mutants was unaffected compared to the wild type suggesting that the genes do not play a role in disease as assayed in this attached leaf system (Supplementary Figure S14).
Discussion
The roles and functions of volatile organic compounds produced by fungi are poorly understood. As such, we embarked upon a study to determine if the VOCs produced by the wheat pathogen P. nodorum were biologically active, and if so, resolve their identity. In this study, we have demonstrated that the wheat pathogen P. nodorum produces a range of VOCs that harbor intrinsic biological activities including inhibiting effects on plant seedlings, bacterial growth and also self-growth.
Initial assays clearly displayed that P. nodorum secretes bioactive VOCs as observed through the growth inhibition and phytotoxicity. It was also interesting to see evidence that the secreted VOCs may have a role in self growth regulation of the pathogen. Previous studies have demonstrated that volatiles can function in fungal self-inhibition of growth. For example, 1-octen-3-ol, a short chain alcohol produced by Penicillium paneum, and the sesquiterpene thujopsene from Penicillium decumbes, are known to inhibit the growth of the source fungi (Chitarra et al., 2004; Polizzi et al., 2011). Similarly, the selective inhibition of the P. nodorum VOC complement against bacteria observed is not without precedent. It has been previously demonstrated that compounds produced by sponge-associated Arctic microbial communities show a strong inhibitory activity against the opportunistic pathogenic bacterial Burkholderia cepacia complex but not to other pathogenic bacteria (Papaleo et al., 2012; Romoli et al., 2013). Why VOCs from P. nodorum would harbor this specificity is unclear but it has been suggested that such selectivity may be a reflection of how different organisms respond differently to the same chemical cue or alternatively it may be a consequence of possible fitness differences among individuals. Such an effect of VOCs on shaping microbial communities has been previously proposed (Tirranen and Gitelson, 2006).
An analysis of the P. nodorum VOC chromatograms revealed that the four most prominent compounds are well-described short chain alcohols (Table 1). 3-Methyl-1-butanol and 2-methyl-1-butanol have been previously demonstrated to inhibit the growth of the fungal pathogen Sclerotinia sclerotiorum (Fialho et al., 2011). Similarly, 2-phenylethanol affects gene expression and interferes in epigenetic regulation leading to the growth inhibition of multiple fungi including Aspergillus flavus, Neurospora crassa and Penicillium spp. (Lester, 1965; Hua et al., 2014, Liu et al., 2014). In contrast, low concentrations of 2-phenylethanol stimulates, rather than inhibits, the growth of A. flavus, revealing a hormetic behavior of this compound (Chang et al., 2015). These data may suggest the involvement of these short chain alcohols in metabolic regulation and could be generalized communication signals in fungi. Furthermore, considering that these molecules are produced by a broad range of organisms, one could hypothesize that communication occurs at various levels ranging from interspecific to inter-kingdom crosstalk. Interestingly, when tested in pure form or artificially blended, these VOCs had no observable effect on the development of P. nodorum. While there is a possibility that the tested concentration (1 mM) is not sufficient for triggering an observable effect, the most plausible explanation for the lack of response from P. nodorum is that other compounds we have not tested maybe involved in regulating the pathogen’s growth, either singularly, or in combination with one of the major VOCs.
The effect of the four main P. nodorum volatile alcohols over plant and bacterial development has also been previously described. Akin to our observations, VOCs from truffles (Tuber spp.) inhibited the development of Arabidopsis thaliana (Splivallo et al., 2007). Within the volatile emissions of tuber fruiting bodies, 3-methyl-1-butanol inhibited A. thaliana germination at 130 ppm while 2-phenylethanol was inhibitory at 13 ppm and caused discoloration of the cotyledons of germinated seedlings at 130 ppm (Splivallo et al., 2007).
In contrast, VOCs mixtures produced by rhizobacteria containing 3-methyl-1-butanol, 2-methyl-1-butanol and 2-methyl-1-propanol promote growth of A. thaliana (Ryu et al., 2003; Farag et al., 2006). It is possible that this differential effect is caused by variations in the proportion of the components of the volatilomes. It is known that differences in VOC levels in the soil correlate to changes in microbial soil populations (McNeal and Herbert, 2009). If we consider that interaction between organisms is a biological network that interweaves at different levels (Pritchard and Birch, 2011), it could be speculated that common volatile metabolites may help to coordinate the network by allowing organisms to eavesdrop on the communication signals from their neighbors (Baldwin et al., 2006; Cha et al., 2011, Caruso and Parachnowitsch, 2016). Assuming that VOCs have an active role on interspecific communication, it is important to highlight an epistemological weak point of this study; the VOCs bouquet is expected to be dynamic and depending on the challenging (test) organism, P. nodorum emissions may differ from the compound profile of the axenic headspace vials analyzed by GC-MS.
Together with the short chain alcohols, a suite of sesquiterpenes were also identified in the VOC mixture. The presences of eudesma-4,11-diene (1), β-elemene (2), α-cyperone (3), and acora-4,9-diene (4) were all confirmed through a combination of mass spectrometry and NMR analysis. Subsequent reverse genetics and overexpression experiments then confirmed that the sesquiterpene synthase genes in P. nodorum, Sts1 and Sts2, were responsible for their biosynthesis. Given the presence and abundance of these molecules during infection of wheat by the pathogen, it was surprising that mutant strains of the fungus lacking these molecules appeared unaffected in terms of development or pathogenicity. Indeed, information is scarce on what precisely the functions of these sesquiterpenes identified above are.
Many plants emit eudesma-4,11-diene (1) as a minor component of biologically active VOCs mixtures or essential oils (Yoshida et al., 1967; Chu et al., 2011, Lee and Vairappan, 2011; Takahashi et al., 2011, Wang et al., 2011). While 1 is produced by some basidiomycete and ascomycete fungi and by some actinomycetes, no biological activity has been described for either fungi or bacteria (Rösecke et al., 2000; Ayoub et al., 2009, Brock and Dickschat, 2013; Rabe et al., 2013, Yuan et al., 2013). Furthermore, 1 is produced (along with other sesquiterpenes) by soldier termites from different species and Ceroplastes ceriferu, a scale insect (Naya et al., 1978; Braekman et al., 1993, Krasulova et al., 2012). Similarly, many plants and insects such as termites, aphids, butterflies and lady beetles, produce β-elemene (2) (Bowers et al., 1977; Baker et al., 1981, Everaerts et al., 1993; Quintana et al., 2003, Omura et al., 2006; Adio, 2009, Fassotte et al., 2014). The ascomycetes Penicillium clavigerum, Penicillium roqueforti and an endophytic Nodulisporium sp., and the basidiomycetes Inonotus obliquus and Piptoporus betulinus, are known to produce β-elemene (Fischer et al., 1999; Rösecke et al., 2000, Jeleń, 2002; Ayoub et al., 2009, Sanchez-Fernandez et al., 2016). However, no role for this molecule in fungi has been identified.
The distribution of α-cyperone (3) seems to be more restricted. The molecule was first identified from the rhizomes of Cyperus rotundus, a medicinal plant which is also classified as an invasive grass (Bradfield et al., 1936). It has been postulated that 3 is causal to the described antimicrobial, phytotoxic, insecticidal, anti-inflammatory and antimalarial activities in essential oils from C. rotundus (Komai et al., 1977, Dadang et al., 1996, Mojab et al., 2009; Pirzada et al., 2015). In fungi there is just one report corresponding to a stereoisomer of 3 isolated from an endophytic Ascochyta sp. from Meliotus dentatus but it has the opposite configuration to the plant isolated α-cyperone (Krohn et al., 2007). Importantly, there are no reports on what this change in stereochemistry has on the function of the molecule. Ascochyta and P. nodorum are closely related fungi so it would not be unexpected if the α-cyperone identified in this study was also the opposite stereochemistry to the plant-derived molecule. As such, it is difficult to infer what the function of α-cyperone in P. nodorum may be.
In contrast to the widespread occurrence of molecules 1–3 discussed above, acora-4,9-diene (4), synthesized by Sts1 in P. nodorum has only been found in the oils of vetiver (Chrysopogon zizanioides), in the seeds of carrot (Daucus carota), and in the glandular trichome exudates from leaves of Japanese rose (Rosa rugosa), with no biological activity described up to date (Kaiser and Naegeli, 1972; Hashidoko et al., 1992, Mazzoni et al., 1999).
Proposing an ecological role for Sts1 and Sts2 sesquiterpenes in the P. nodorum-wheat pathosystem is difficult due to the diversity of producers and reported activities of eudesma-4,11-diene (1) and β-elemene (2), the uncertainty of the P. nodorum α-cyperone (3) stereochemistry, the lack of information about acora-4,9-diene (4), and the absence of evident effects on pathogenicity, phytotoxicity, antimicrobial or self-regulating properties. Nonetheless, the in planta production of these molecules suggests its possible involvement in the fungal-plant interaction. Subtle changes in the interaction conferring some “competitive” advantage to the pathogen may not be easily detected in the laboratory pathogenicity tests which are not indicative of the full disease cycle of the pathogen.
Aside from the ecological role of the sesquiterpenes, the linking of genes to products in this study also provides an opportunity to better understand the biosynthesis of the identified sesquiterpenes. A non-redundant BlastP analysis of Sts1 and Sts2 suggested that the two proteins are related to trichodiene and aristolochene synthases respectively (Supplementary Figure S15). These two enzymes have many similarities; in both cases the linear precursor of their products is farnesyl pyrophosphate (FPP) which loses its phosphate group and cyclizes into a cationic cyclic intermediate. The difference is the type of carbocycle produced, which depends on the tertiary structure of the enzyme. While trichodiene synthase produces a bisabolyl cation, the aristolochene synthase produces a germacranyl cation (Cane, 1990). The sequence similarity of Sts1 and trichodiene synthases is congruent with the fact that the structure of acora-4,9-diene, and the other 14 putative sesquiterpenes produced by Sts1, seems to require a bisabolene intermediate (Figure 7). Conversely, a germacranyl intermediate is the likely intermediary of eudesma-4,11-diene, β-elemene, and the other 10 products of Sts2, which corresponds to the similarity between this enzyme and aristolochene synthases (Figure 7). The generation of the multiple products by a single sesquiterpene synthase is due to the intermediaries, bisabolyl and germacranyl cations in this case, suffering spontaneous rearrangements with minimal involvement of the biosynthetic enzyme. Terpene cyclases displaying a higher control over these subsequent reactions may produce fewer structures or even a single product (Christianson, 2008). The generation of a wide spectrum of sesquiterpenes or other chemical structures increase the chances of some of these molecules having the right conformation to interact with diverse biological targets and affecting other organisms.
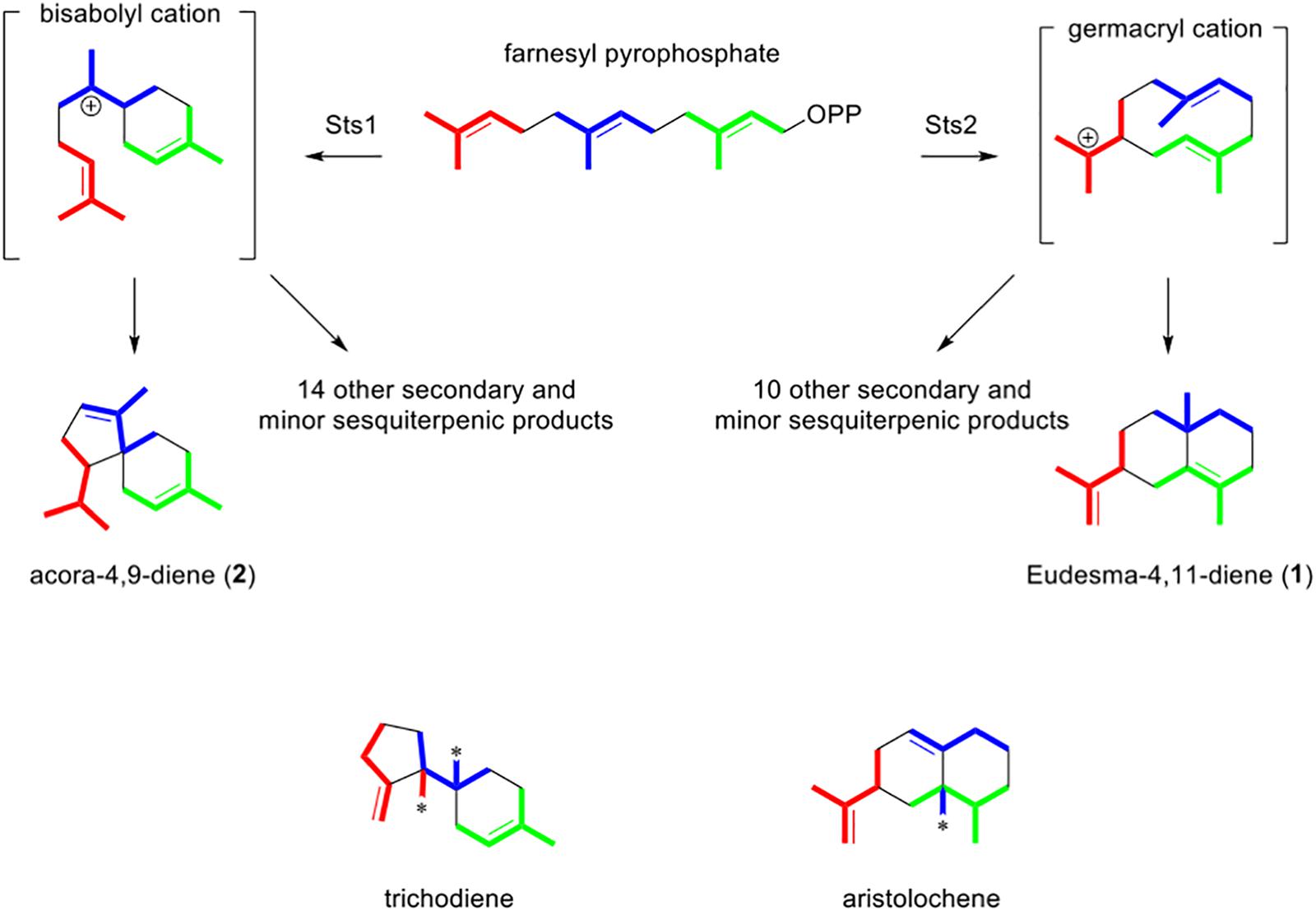
Figure 7. Folding pattern of farnesyl pyrophosphate (FPP) in P. nodorum Sts1 and Sts2. Color indicate the different isoprene units. Asterisks in methyl groups of trichothecene and aristolochene indicate a rearrangement and the color indicates to which isoprene unit it corresponds.
Even though many biological interactions are established through VOCs, the roles and synthesis of these compounds in fungi are poorly understood. In this study we have found that, in addition to the well-characterized proteinaceous effectors produced by P. nodorum as disease determinants, VOCs produced by this pathogen in vitro also trigger a response in the host plant as well as having effects on other microorganisms. Additionally, the discovery of P. nodorum sesquiterpenes represents the first report of terpenes in this pathogen and complementary techniques were used to link these sesquiterpene structures to their respective biosynthetic genes. Despite these advances, this study exemplifies the many unknowns that remain pertaining to VOCs in fungi and highlights their potential for future research. Unfortunately, the complexity of the volatile bouquets and the chemical signals conveyed by them represent a major challenge when teasing apart biological activities and ecological roles.
Author’s Note
This manuscript has been released as a Pre-Print on BioRxiv (Muria-Gonzalez et al., 2019).
Data Availability Statement
All datasets generated for this study are included in the article/Supplementary Material.
Author Contributions
MM-G, Y-HC, and PS contributed to the design and concept of the study. MM-G, SB, OM, CW, and YY contributed to the experimentation and collection of data. Y-HC and RB contributed to data analysis. MM-G, Y-HC and PS wrote the manuscript. All authors intellectually contributed to the study and revision of the manuscript.
Conflict of Interest
RB was employed by Plus 3 Australia Pty Ltd.
The remaining authors declare that the research was conducted in the absence of any commercial or financial relationships that could be construed as a potential conflict of interest.
Acknowledgments
MM-G is a recipient of an Australian Government Endeavour Award and a Mexican CONACYT scholarship. Y-HC is Australian Research Council Future Fellow (FT160100233).
Supplementary Material
The Supplementary Material for this article can be found online at: https://www.frontiersin.org/articles/10.3389/fmicb.2020.00466/full#supplementary-material
References
Adio, A. M. (2009). (-)-trans-β-elemene and related compounds: occurrence, synthesis, and anticancer activity. Tetrahedron 65, 5145–5159. doi: 10.1016/j.tet.2009.04.062
Ayoub, N., Schultze, W., and Lass, D. (2009). Volatile constituents of the medicinal fungus chaga Inonotus obliquus (Pers.: Fr.) Pilát (Aphyllophoromycetideae). Int. J. Med. Mushrooms 11, 55–60. doi: 10.1615/intjmedmushr.v11.i1.60
Baker, R., Coles, H. R., Edwards, M., Evans, D. A., Howse, P. E., and Walmsley, S. (1981). Chemical composition of the frontal gland secretion of Syntermes soldiers (Isoptera, Termitidae). J. Chem. Ecol. 7, 135–145. doi: 10.1007/BF00988641
Baldwin, I. T., Halitschke, R., Paschold, A., von Dahl, C. C., and Preston, C. A. (2006). Volatile signaling in plant-plant interactions: “Talking Trees” in the genomics era. Science 311, 812–815. doi: 10.1126/science.1118446
Bowers, W. S., Nishino, C., Montgomery, M. E., Nault, L. R., and Nielson, M. W. (1977). Sesquiterpene progenitor, germacrene A: an alarm pheromone in aphids. Science 196, 680–681. doi: 10.1126/science.558651
Bradfield, A. E., Hegde, B. H., Rao, B. S., Simonsen, J. L., and Gillam, A. E. (1936). 152. α-Cyperone, a sesquiterpene ketone from the oil of Cyperus rotundus. J. Chem. Soc. (Resumed) 1936, 667–677. doi: 10.1039/jr9360000667
Braekman, J. C., Remacle, A., and Roisin, Y. (1993). Soldier defensive secretion of three Amitermes species. Biochem. Syst. Ecol. 21, 661–666. doi: 10.1016/0305-1978(93)90070-8
Breen, S., Williams, S. J., Winterberg, B., Kobe, B., and Solomon, P. S. (2016). Wheat PR-1 proteins are targeted by necrotrophic pathogen effector proteins. Plant J. 88, 13–25. doi: 10.1111/tpj.13228
Brock, N. L., and Dickschat, J. S. (2013). PR toxin biosynthesis in Penicillium roqueforti. ChemBioChem 14, 1189–1193. doi: 10.1002/cbic.201300254
Cane, D. E. (1990). Enzymic formation of sesquiterpenes. Chem. Rev. 90, 1089–1103. doi: 10.1021/cr00105a002
Caruso, C. M., and Parachnowitsch, A. L. (2016). Do plants eavesdrop on floral scent signals? Trends Plant Sci. 21, 9–15. doi: 10.1016/j.tplants.2015.09.001
Cha, D. H., Linn, C. E., Teal, P. E. A., Zhang, A., Roelofs, W. L., and Loeb, G. M. (2011). Eavesdropping on plant volatiles by a specialist moth: significance of ratio and concentration. PLoS One 6:e17033. doi: 10.1371/journal.pone.0017033
Chang, P. K., Hua, S. S. T., Sarreal, S. B. L., and Li, R. W. (2015). Suppression of aflatoxin biosynthesis in Aspergillus flavus by 2-phenylethanol is associated with stimulated growth and decreased degradation of branched-chain amino acids. Toxins 7, 3887–3902. doi: 10.3390/toxins7103887
Chen, Y.-J., and Lin, W.-Y. (1993). Total synthesis of (±)-acoradiene via radical cyclization. Tetrahedron 49, 10263–10270. doi: 10.1016/s0040-4020(01)80555-x
Chitarra, G. S., Abee, T., Rombouts, F. M., Posthumus, M. A., and Dijksterhuis, J. (2004). Germination of Penicillium paneum conidia is regulated by 1-octen-3-ol, a volatile self-inhibitor. Appl. Environ. Microbiol. 70, 2823–2829. doi: 10.1128/aem.70.5.2823-2829.2004
Chooi, Y.-H., Krill, C., Barrow, R. A., Chen, S., Trengove, R., Oliver, R. P., et al. (2015a). An In planta-expressed -polyketide synthase produces (R)-mellein in the wheat pathogen Parastagonospora nodorum. Appl. Environ. Microbiol. 81, 177–186. doi: 10.1128/AEM.02745-14
Chooi, Y.-H., Muria-Gonzalez, M. J., Mead, O. L., and Solomon, P. S. (2015b). SnPKS19 encodes the polyketide synthase for alternariol mycotoxin biosynthesis in the wheat pathogen Parastagonospora nodorum. Appl. Environ. Microbiol. 81, 5309–5317. doi: 10.1128/AEM.00278-15
Chooi, Y.-H., Muria-Gonzalez, M. J., and Solomon, P. S. (2014). A genome-wide survey of the secondary metabolite biosynthesis genes in the wheat pathogen Parastagonospora nodorum. Mycology 5, 192–206. doi: 10.1080/21501203.2014.928386
Chooi, Y.-H., Zhang, G., Hu, J., Muria-Gonzalez, M. J., Tran, P. N., Pettitt, A., et al. (2017). Functional genomics-guided discovery of a light-activated phytotoxin in the wheat pathogen Parastagonospora nodorum via pathway activation. Environ. Microbiol. 19, 1975–1986. doi: 10.1111/1462-2920.13711
Christianson, D. W. (2008). Unearthing the roots of the terpenome. Curr. Opin. Chem. Biol. 12, 141–150. doi: 10.1016/j.cbpa.2007.12.008
Chu, S. S., Jiang, G. H., and Liu, Z. L. (2011). Insecticidal compounds from the essential oil of Chinese medicinal herb Atractylodes chinensis. Pest Manag. Sci. 67, 1253–1257. doi: 10.1002/ps.2180
Dadang, Ohsawa, K., Kato, S., and Yamamoto, I. (1996). Insecticidal compound in tuber of Cyperus rotundus L. against the diamondback moth larvae. J. Pestic. Sci. 21, 444–446. doi: 10.1584/jpestics.21.444
Everaerts, C., Roisin, Y., Le Quere, J. L., Bonnard, O., and Pasteels, J. M. (1993). Sesquiterpenes in the frontal gland secretions of nasute soldier termites from New Guinea. J. Chem. Ecol. 19, 2865–2879. doi: 10.1007/BF00980588
Farag, M. A., Ryu, C.-M., Sumner, L. W., and Paré, P. W. (2006). GC–MS SPME profiling of rhizobacterial volatiles reveals prospective inducers of growth promotion and induced systemic resistance in plants. Phytochemistry 67, 2262–2268. doi: 10.1016/j.phytochem.2006.07.021
Fassotte, B., Fischer, C., Durieux, D., Lognay, G., Haubruge, E., Francis, F., et al. (2014). First evidence of a volatile sex pheromone in lady beetles. PLoS One 9:e115011. doi: 10.1371/journal.pone.0115011
Fialho, M. B., de Moraes, M. H. D., Tremocoldi, A. R., and Pascholati, S. F. (2011). Potential of antimicrobial volatile organic compounds to control Sclerotinia sclerotiorum in bean seeds. Pesqu. Agropecu. Bras 46, 137–142. doi: 10.1590/s0100-204x2011000200004
Fischer, G., Schwalbe, R., Möller, M., Ostrowski, R., and Dott, W. (1999). Species-specific production of microbial volatile organic compounds (MVOC) by airborne fungi from a compost facility. Chemosphere 39, 795–810. doi: 10.1016/s0045-6535(99)00015-6
Friesen, T. L., Stukenbrock, E. H., Liu, Z., Meinhardt, S., Ling, H., Faris, J. D., et al. (2006). Emergence of a new disease as a result of interspecific virulence gene transfer. Nat. Genet. 38, 953–956. doi: 10.1038/ng1839
Hashidoko, Y., Tahara, S., and Mizutani, J. (1992). Sesquiterpene hydrocarbons in glandular trichome exudate of Rosa rugosa leaves. Z. Naturforsch. C J. Biosci. 47, 353–359. doi: 10.1515/znc-1992-0606
Hua, S. S., Beck, J. J., Sarreal, S. B., and Gee, W. (2014). The major volatile compound 2-phenylethanol from the biocontrol yeast, Pichia anomala, inhibits growth and expression of aflatoxin biosynthetic genes of Aspergillus flavus. Mycotoxin Res. 30, 71–78. doi: 10.1007/s12550-014-0189-z
Ipcho, S. V. S., Hane, J. K., Antoni, E. A., Ahren, D. A. G., Henrissat, B., Friesen, T. L., et al. (2012). Transcriptome analysis of Stagonospora nodorum: gene models, effectors, metabolism and pantothenate dispensability. Mol. Plant Pathol. 13, 531–545. doi: 10.1111/j.1364-3703.2011.00770.x
Iwabuchi, H., Yoshikura, M., and Kamisako, W. (1989). Studies on the sequiterpenoids of panax ginseng C. A. MEYER. III. Chem. Pharm. Bull. 37, 509–510. doi: 10.1248/cpb.37.509
Jeleń, H. H. (2002). Volatile sesquiterpene hydrocarbons characteristic for Penicillium roqueforti strains producing PR toxin. J. Agric. Food Chem. 50, 6569–6574. doi: 10.1021/jf020311o
Kaiser, R., and Naegeli, P. (1972). Biogenetically significant components in vetiver oil. Tetrahedron Lett. 13, 2009–2012. doi: 10.1016/s0040-4039(01)85026-7
Kanchiswamy, C. N., Malnoy, M., and Maffei, M. E. (2015). Chemical diversity of microbial volatiles and their potential for plant growth and productivity. Front. Plant Sci. 6:151. doi: 10.3389/fpls.2015.00151
Kesselmeier, J., and Staudt, M. (1999). Biogenic volatile organic compounds (VOC): an overview on emission, physiology and ecology. J. Atmos. Chem. 33, 23–88.
Komai, K., Iwamura, J., and Ueki, K. (1977). Isolation, identification and physiological activities of sesquiterpenes in purple nutsedge tubers. J. Weed Sci. Technol. 22, 14–18. doi: 10.3719/weed.22.14
Kováts, E. (1958). Gas-chromatographische charakterisierung organischer verbindungen. Teil 1: retentionsindices aliphatischer halogenide, alkohole, aldehyde und ketone. Helv. Chim. Acta 41, 1915–1932. doi: 10.1002/hlca.19580410703
Krasulova, J., Hanus, R., Kutalova, K., Sobotnik, J., Sillam-Dusses, D., Tichy, M., et al. (2012). Chemistry and anatomy of the frontal gland in soldiers of the sand termite Psammotermes hybostoma. J. Chem. Ecol. 38, 557–565. doi: 10.1007/s10886-012-0123-9
Krohn, K., Kock, I., Elsässer, B., Flörke, U., Schulz, B., Draeger, S., et al. (2007). Bioactive natural products from the endophytic fungus Ascochyta sp. from Meliotus dentatus – configurational assignment by solid-state CD and TDDFT calculations. Eur. J. Org. Chem. 2007, 1123–1129. doi: 10.1002/ejoc.200600907
Lee, K. M., and DaSilva, N. A. (2005). Evaluation of the Saccharomyces cerevisiae ADH2 promoter for protein synthesis. Yeast 22, 431–440. doi: 10.1002/yea.1221
Lee, T. K., and Vairappan, C. S. (2011). Antioxidant, antibacterial and cytotoxic activities of essential oils and ethanol extracts of selected South East Asian herbs. J. Med. Plants Res. 5, 5284–5290.
Lester, G. (1965). Inhibition of growth, synthesis, and permeability in Neurospora crassa by phenethyl alcohol. J. Bacteriol. 90, 29–37. doi: 10.1128/jb.90.1.29-37.1965
Li, H., Hu, J., Wei, H., Solomon, P. S., Vuong, D., Lacey, E., et al. (2018). Chemical ecogenomics-guided discovery of phytotoxic α-pyrones from the fungal wheat pathogen Parastagonospora nodorum. Org. Lett. 20, 6148–6152. doi: 10.1021/acs.orglett.8b02617
Liu, P., Cheng, Y., Yang, M., Liu, Y., Chen, K., Long, C.-A., et al. (2014). Mechanisms of action for 2-phenylethanol isolated from Kloeckera apiculata in control of Penicillium molds of citrus fruits. BMC Microbiol. 14:242. doi: 10.1186/s12866-014-0242-2
Liu, Z., Faris, J. D., Oliver, R. P., Tan, K. C., Solomon, P. S., McDonald, M. C., et al. (2009). SnTox3 acts in effector triggered susceptibility to induce disease on wheat carrying the Snn3 gene. PLoS Pathog. 5:e1000581. doi: 10.1371/journal.ppat.1000581
Liu, Z., Gao, Y., Kim, Y. M., Faris, J. D., Shelver, W. L., de Wit, P. J. G. M., et al. (2016). SnTox1, a Parastagonospora nodorum necrotrophic effector, is a dual-function protein that facilitates infection while protecting from wheat-produced chitinases. New Phytol. 211, 1052–1064. doi: 10.1111/nph.13959
Liu, Z., Zhang, Z., Faris, J. D., Oliver, R. P., Syme, R., McDonald, M. C., et al. (2012). The cysteine rich necrotrophic effector SnTox1 produced by Stagonospora nodorum triggers susceptibility of wheat lines harboring Snn1. PLoS Pathog. 8:e1002467. doi: 10.1371/journal.ppat.1002467
Mattern, I. E., Punt, P. J., and Van den Hondel, C. A. M. J. J. (1988). A vector for Aspergillus transformation conferring phleomycin resistance. Fungal Genet. Rep. 35:13.
Mazzoni, V., Tomi, F., and Casanova, J. (1999). A daucane-type sesquiterpene from Daucus carota seed oil. Flavour Fragr. J. 14, 268–272. doi: 10.1002/(sici)1099-1026(199909/10)14:5<268::aid-ffj823>3.0.co;2-z
McDonald, M. C., and Solomon, P. S. (2018). Just the surface: advances in the discovery and characterization of necrotrophic wheat effectors. Curr. Opin. Microbiol. 46, 14–18. doi: 10.1016/j.mib.2018.01.019
McNeal, K. S., and Herbert, B. E. (2009). Volatile organic metabolites as indicators of soil microbial activity and community composition shifts. Soil Sci. Soc. Am. J. 73, 579–588. doi: 10.2136/sssaj2007.0245
Mojab, F., Vahidi, H., Nickavar, B., and Kamali-nejad, M. (2009). Chemical components of essential oil and antimicrobial effects of rhizomes from Cyperus rotundus L. J. Med. Plants 8, 91–97. doi: 10.1038/srep45231
Muria-Gonzalez, M. J., Chooi, Y.-H., Breen, S., and Solomon, P. S. (2015). The past, present and future of secondary metabolite research in the Dothideomycetes. Mol. Plant Pathol. 16, 92–107. doi: 10.1111/mpp.12162
Muria-Gonzalez, M. J., Yap, H. Y. Y., Breen, S., Mead, O., Wang, C., Chooi, Y.-H., et al. (2019). Volatile molecules secreted by the wheat pathogen Parastagonospora nodorum are involved in development and phytotoxicity. bioRxiv [Preprint]. doi: 10.1101/729509
Naya, Y., Miyamoto, F., and Takemoto, T. (1978). Formation of enantiomeric sesquiterpenes in the secretions of scale insects. Experientia 34, 984–986. doi: 10.1007/bf01915301
Oliver, R. P., Friesen, T. L., Faris, J. D., and Solomon, P. S. (2012). Stagonospora nodorum: from pathology to genomics and host resistance. Annu. Rev. Phytopathol. 50, 23–43. doi: 10.1146/annurev-phyto-081211-173019
Omura, H., Honda, K., and Feeny, P. (2006). From terpenoids to aliphatic acids: further evidence for late-instar switch in osmeterial defense as a characteristic trait of swallowtail butterflies in the tribe papilionini. J. Chem. Ecol. 32, 1999–2012. doi: 10.1007/s10886-006-9124-x
Papaleo, M. C., Fondi, M., Maida, I., Perrin, E., Lo Giudice, A., Michaud, L., et al. (2012). Sponge-associated microbial Antarctic communities exhibiting antimicrobial activity against Burkholderia cepacia complex bacteria. Biotechnol. Adv. 30, 272–293. doi: 10.1016/j.biotechadv.2011.06.011
Pirzada, A. M., Ali, H. H., Naeem, M., Latif, M., Bukhari, A. H., and Tanveer, A. (2015). Cyperus rotundus L.: traditional uses, phytochemistry, and pharmacological activities. J. Ethnopharmacol. 174, 540–560. doi: 10.1016/j.jep.2015.08.012
Polizzi, V., Fazzini, L., Adams, A., Picco, A. M., Saeger, S., Peteghem, C., et al. (2011). Autoregulatory properties of (+)-thujopsene and influence of environmental conditions on its production by Penicillium decumbens. Microb. Ecol. 62, 838–852. doi: 10.1007/s00248-011-9905-9
Pritchard, L., and Birch, P. (2011). A systems biology perspective on plant–microbe interactions: biochemical and structural targets of pathogen effectors. Plant Sci. 180, 584–603. doi: 10.1016/j.plantsci.2010.12.008
Quintana, A., Reinhard, J., Faure, R., Uva, P., Bagneres, A. G., Massiot, G., et al. (2003). Interspecific variation in terpenoid composition of defensive secretions of European Reticulitermes termites. J. Chem. Ecol. 29, 639–652.
Rabe, P., Citron, C. A., and Dickschat, J. S. (2013). Volatile terpenes from actinomycetes: a biosynthetic study correlating chemical analyses to genome data. ChemBioChem 14, 2345–2354. doi: 10.1002/cbic.201300329
Romoli, R., Papaleo, M. C., De Pascale, D., Tutino, M. L., Michaud, L., LoGiudice, A., et al. (2013). GC–MS volatolomic approach to study the antimicrobial activity of the antarctic bacterium Pseudoalteromonas sp. TB41. Metabolomics 10, 42–51. doi: 10.1007/s11306-013-0549-2
Rösecke, J., Pietsch, M., and König, W. A. (2000). Volatile constituents of wood-rotting basidiomycetes. Phytochemistry 54, 747–750. doi: 10.1016/s0031-9422(00)00138-2
Ryu, C.-M., Farag, M. A., Hu, C.-H., Reddy, M. S., Wei, H.-X., Paré, P. W., et al. (2003). Bacterial volatiles promote growth in Arabidopsis. Proc. Natl. Acad. Sci. U.S.A. 100, 4927–4932. doi: 10.1073/pnas.0730845100
Sanchez-Fernandez, R. E., Diaz, D., Duarte, G., Lappe-Oliveras, P., Sanchez, S., and Macias-Rubalcava, M. L. (2016). Antifungal volatile organic compounds from the endophyte Nodulisporium sp. strain GS4d2II1a: a qualitative change in the intraspecific and interspecific interactions with Pythium aphanidermatum. Microb. Ecol. 71, 347–364. doi: 10.1007/s00248-015-0679-3
Solomon, P. S., Tan, K.-C., Sanchez, P., Cooper, R. M., and Oliver, R. P. (2004). The disruption of a Gα subunit sheds new light on the pathogenicity of Stagonospora nodorum on wheat. Mol. Plant Microbe Interact. 17, 456–466. doi: 10.1094/mpmi.2004.17.5.456
Splivallo, R., Novero, M., Bertea, C. M., Bossi, S., and Bonfante, P. (2007). Truffle volatiles inhibit growth and induce an oxidative burst in Arabidopsis thaliana. New Phytol. 175, 417–424. doi: 10.1111/j.1469-8137.2007.02141.x
Takahashi, M., Inouye, S., and Abe, S. (2011). Anti-Candida and radical scavenging activities of essential oils and oleoresins of Zingiber officinale Roscoe and essential oils of other plants belonging to the family Zingiberaceae. Drug Discov. Ther. 5, 238–245. doi: 10.5582/ddt.2011.v5.5.238
Tan, K.-C., Phan, H. T. T., Rybak, K., John, E., Chooi, Y. H., Solomon, P. S., et al. (2015). Functional redundancy of necrotrophic effectors – consequences for exploitation for breeding. Front. Plant Sci. 6:501. doi: 10.3389/fpls.2015.00501
Tan, K.-C., Waters, O. D. C., Rybak, K., Antoni, E., Furuki, E., and Oliver, R. P. (2014). Sensitivity to three Parastagonospora nodorum necrotrophic effectors in current Australian wheat cultivars and the presence of further fungal effectors. Crop Pasture Sci. 65, 150–158.
Tirranen, L. S., and Gitelson, I. I. (2006). The role of volatile metabolites in microbial communities of the LSS higher plant link. Adv. Space Res. 38, 1227–1232. doi: 10.1016/j.asr.2006.02.038
van Den Dool, H., and Dec Kratz, P. (1963). A generalization of the retention index system including linear temperature programmed gas—liquid partition chromatography. J. Chromatogr. A 11, 463–471. doi: 10.1016/s0021-9673(01)80947-x
Wang, J., Li, J., Li, S., Freitag, C., and Morrell, J. J. (2011). Antifungal activities of Cunninghamia lanceolata heartwood extractives. BioResources 6, 606–614.
Yoshida, T., Endo, K., Ito, S., and Nozoe, T. (1967). Chemical constituents in the essential oil of the leaves of Chamaecyparis taiwanensis. Yakugaku Zasshi 87, 434–439. doi: 10.1248/yakushi1947.87.4_434
Keywords: wheat pathogens, volatiles, disease, sesquiterpenes, Parastagonosopora nodorum
Citation: Muria-Gonzalez MJ, Yeng Y, Breen S, Mead O, Wang C, Chooi Y-H, Barrow RA and Solomon PS (2020) Volatile Molecules Secreted by the Wheat Pathogen Parastagonospora nodorum Are Involved in Development and Phytotoxicity. Front. Microbiol. 11:466. doi: 10.3389/fmicb.2020.00466
Received: 24 October 2019; Accepted: 04 March 2020;
Published: 25 March 2020.
Edited by:
Susanne Zeilinger, University of Innsbruck, AustriaReviewed by:
Francesco Vinale, University of Naples Federico II, ItalyVeronika Ruzsanyi, University of Innsbruck, Austria
Magnus Karlsson, Swedish University of Agricultural Sciences, Sweden
Copyright © 2020 Muria-Gonzalez, Yeng, Breen, Mead, Wang, Chooi, Barrow and Solomon. This is an open-access article distributed under the terms of the Creative Commons Attribution License (CC BY). The use, distribution or reproduction in other forums is permitted, provided the original author(s) and the copyright owner(s) are credited and that the original publication in this journal is cited, in accordance with accepted academic practice. No use, distribution or reproduction is permitted which does not comply with these terms.
*Correspondence: Peter S. Solomon, cGV0ZXIuc29sb21vbkBhbnUuZWR1LmF1
†Present address: Mariano Jordi Muria-Gonzalez, Centre for Crop and Disease Management, Curtin University, Perth, WA, Australia Susan Breen, School of Life Sciences, University of Warwick, Coventry, United Kingdom