- 1Laboratoire de Microbiologie Signaux et Microenvironnement, LMSM EA4312, Université de Rouen Normandie, Normandie Université, Évreux, France
- 2CiTCoM UMR 8038 CNRS, Faculté des Sciences Pharmaceutiques et Biologiques, Équipe Produits Naturels, Analyses et Synthèses (PNAS), Université Paris Descartes, Paris, France
- 3Centre de Recherche Scientifique et Technique en Analyses Physico-Chimiques, CRAPC, Bou Ismaïl, Algeria
Pseudomonas aeruginosa is capable to deploy a collection of virulence factors that are not only essential for host infection and persistence, but also to escape from the host immune system and to become more resistant to drug therapies. Thus, developing anti-virulence agents that may directly counteract with specific virulence factors or disturb higher regulatory pathways controlling the production of virulence armories are urgently needed. In this regard, this study reports that Pistacia lentiscus L. fruit cyclohexane extract (PLFE1) thwarts P. aeruginosa virulence by targeting mainly the pyocyanin pigment production by interfering with 4-hydroxy-2-alkylquinolines molecules production. Importantly, the anti-virulence activity of PLFE1 appears to be associated with membrane homeostasis alteration through the modulation of SigX, an extracytoplasmic function sigma factor involved in cell wall stress response. A thorough chemical analysis of PLFE1 allowed us to identify the ginkgolic acid (C17:1) and hydroginkgolic acid (C15:0) as the main bioactive membrane-interactive compounds responsible for the observed increased membrane stiffness and anti-virulence activity against P. aeruginosa. This study delivers a promising perspective for the potential future use of PLFE1 or ginkgolic acid molecules as an adjuvant therapy to fight against P. aeruginosa infections.
Introduction
Bacterial infections still constitute a serious public health threat even though their prevention and treatment have been improved over the last decades. The effects of common antibiotics are no longer effective against microbial threats including Enterococcus faecium, Staphylococcus aureus, Acinetobacter baumannii, Pseudomonas aeruginosa, and Enterobacter species, also known as the “ESKAPE” pathogens group (Pendleton et al., 2013). In a recent report published by the World Health Organization (WHO), P. aeruginosa was categorized as one of the “critical priority pathogens” for which there is an urgent need for the discovery of alternative and innovative new therapies (WHO, 2017).
Pseudomonas aeruginosa is predominantly responsible for different life-threatening infections in humans, including the respiratory system, burn and wound, urinary tract as well as medical implant devices (Malhotra et al., 2019). This notorious multidrug resistant opportunistic Gram-negative bacterium deploys a wide variety of virulence factors and host-degrading enzymes as well as multiple secondary metabolites (Moradali et al., 2017). Pyocyanin is an important virulence factor produced and secreted abundantly by nearly 95% of P. aeruginosa isolates (Price-Whelan et al., 2006). This phenazine-derived pigment, blue-green in color, confers a greenish hue to the sputum of cystic fibrosis (CF) individuals suffering P. aeruginosa chronic lung infection (Lau et al., 2004). Moreover, pyocyanin is a highly diffusible redox-active secondary metabolite which plays an important role in several physiological processes (Lau et al., 2004; Hall et al., 2016). Several studies demonstrated the harmful effects of pyocyanin on the host immune function leading to the evasion of defense mechanisms. The proliferation of lymphocytes, the expression of IL-2 receptors on T-cells, the secretion of immunoglobulin by B-lymphocytes and of a number of cytokines such as interleukin (IL)-2 have been shown to be negatively altered by the pigment pyocyanin, resulting ultimately in a decreased immune response (Nutman et al., 1987; Allen et al., 2005; Hall et al., 2016). Importantly, the virulence factor pyocyanin plays a key role in modulating multi-drug efflux pump expression in P. aeruginosa biofilms through the BrlR transcriptional regulator which is known to mediate antibiotic resistance (Liao et al., 2013; Wang et al., 2018). Therefore, pyocyanin production hindrance may have consequences regarding the cytotoxic effects and the full virulence of P. aeruginosa during infections related to airways in CF.
The sophisticated quorum sensing (QS) circuitry in P. aeruginosa strongly controls the biosynthesis of pyocyanin. This process starts with the synthesis of the N−acyl−L−homoserine lactone (AHL) type signal molecules followed by the Pseudomonas quinolone signaling (PQS). Next, PQS regulates the expression of phzA-G operons resulting in the production of phenazine-1-carboxylic acid (PCA) which is then modified to produce predominantly pyocyanin via the action of the enzymes encoded by phzM and phzS (Mavrodi et al., 2001). In addition, pyocyanin biosynthesis and regulation have been also linked to SigX, an extracytoplasmic function sigma factor (ECFσ) that plays an essential role in the cell wall stress response network (Gicquel et al., 2013; Blanka et al., 2014; Chevalier et al., 2019; Otero-Asman et al., 2019). In P. aeruginosa, the ECFσ SigX is a global regulator that modulates the expression of more than 300 genes, including several genes involved in pyocyanin biosynthetic pathways (Gicquel et al., 2013; Blanka et al., 2014; Schulz et al., 2015). More importantly, SigX has been reported to be involved in the regulation of fatty acid biosynthesis, which triggers changes in cell membrane phospholipid composition that affect membrane fluidity and envelope integrity (Boechat et al., 2013; Duchesne et al., 2013; Blanka et al., 2014; Fléchard et al., 2018). SigX is also involved in physiological processes as important as iron uptake, virulence, motility, attachment, biofilm formation, and antibiotic resistance and susceptibility via direct and/or indirect governance (Brinkman et al., 1999; Gicquel et al., 2013; Blanka et al., 2014; Chevalier et al., 2019).
In view of this, developing anti-virulence agents that may precisely hinder the production, secretion, or function of virulence determinants or interfere with their regulation has emerged as an alternative promising strategy to fight against bacterial pathogens (Wagner et al., 2016; Soukarieh et al., 2018). Plants have long been used in traditional medicine to prevent or treat infectious diseases in many countries (Mahady, 2005). Hydrophilic and lipophilic extracts from plants were reported to contain abundant and diverse range of bioactive compounds with anti-virulence properties (Silva et al., 2016; Calvert et al., 2018; Soukarieh et al., 2018). Pistacia lentiscus L., a plant commonly known as mastic tree or lentisc, is an evergreen shrub of the family of Anacardiaceae widespread all around the Mediterranean area where it grows wild in a variety of ecosystems (Zohary, 1952; Al-saghir and Porter, 2012). Medicinal uses of the fruit, galls, resin, and leaves of P. lentiscus L. are described since antiquity. However, they may differ either in the therapeutic indication or in the plant part used for medicinal purpose, depending of the geographical area (Bozorgi et al., 2013; Rauf et al., 2017).
The current study aims to explore the anti-virulence potential of P. lentiscus L. fruit, and the molecular mechanism of action, which underpins its major bioactive compounds against P. aeruginosa. Herein, we report that P. lentiscus L. fruit cyclohexane extract (PLFE1) can function as a potent pyocyanin inhibitor while being devoid of any antibacterial activity as judged by cell growth and viability assays. We show that PLFE1 interferes with 4-hydroxy-2-alkylquinolines molecules production, which might explain its anti-pyocyanin activity. We also demonstrate that PLFE1 is able to increase significantly membrane stiffness in P. aeruginosa. Interestingly, the cell wall stress response ECFσ SigX is found to be a key regulatory element in this phenomenon, which might respond to the presence of envelope-interactive compounds in PLFE1. Furthermore, comprehensive chemical analyses of PLFE1 and its derived fractions allowed us to identify the ginkgolic acid (C17:1) and hydroginkgolic acid (C15:0) as the major bioactive compounds and we confirmed that they are responsible of the main anti-virulence activity against the pathogen P. aeruginosa.
Results
Pyocyanin Production Inhibition by P. lentiscus L. fruit Extracts
Planktonic cultures of wild-type P. aeruginosa H103 were exposed to cyclohexane, ethyl acetate, methanol, and water P. lentiscus L. fruit extracts at concentrations of 100, 50, 25, 12.5, 6.25, 3.12, 1.6 and 0.8 μg mL–1 and assayed for interference with pyocyanin production (Figure 1A). Pyocyanin levels from H103 strain cultures untreated with P. lentiscus L. fruit extracts and treated with 1% v/v DMSO were also measured as negative control and defined as 100% pyocyanin production. The PLFE1 (cyclohexane extract) showed statistically significant inhibition of pyocyanin biosynthesis at the all concentration tested. The PLFE1 at 100 μg mL–1 concentration caused the maximum percent reduction in pyocyanin production (82%; P < 0.0001) over the untreated control with an IC50 value of 4.9 μg mL–1. In addition, PLFE1 inhibitory activity was shown to be dose-dependent. The PLFE2 (ethyl acetate extract) was also capable of inhibiting pyocyanin biosynthesis following the same pattern as observed for PLFE1 but not in a drastic manner where approximately 50% of inhibition of this pigment production was observed at 100 μg mL–1 (P < 0.001). There was no significant or slight variation in pyocyanin production by H103 strain when exposed to PLFE3 (methanol extract) or PLFE4 (water extract). Of all the P. lentiscus L. fruit extracts tested for pyocyanin inhibition, PLFE1 cyclohexane extract showed remarkably better inhibitory activity and was therefore selected for all further sets of experiments. Further, the inhibitory activity of PLFE1 on phenazine biosynthetic pathway was investigated at the level of gene expression. In the presence of PLFE1 at 100 μg mL–1, RT-qPCR analyses results showed significant down-regulation of the phzA gene involved in the production of phenazine-1-carboxylic acid (PCA), phzM, and phzS which converts PCA into the derivative pyocyanin (Figure 1B). However, the expression levels of the phzH gene were not significantly different in H103 strain treated with PLFE1 as compared to untreated H103 (Figure 1B). In summary, the reduction of the expression levels of genes involved in phenazine biosynthesis correlate with pyocyanin production inhibition indicating that PLFE1 has the ability to function as a strong inhibitor of the pyocyanin virulence factor in P. aeruginosa H103 strain.
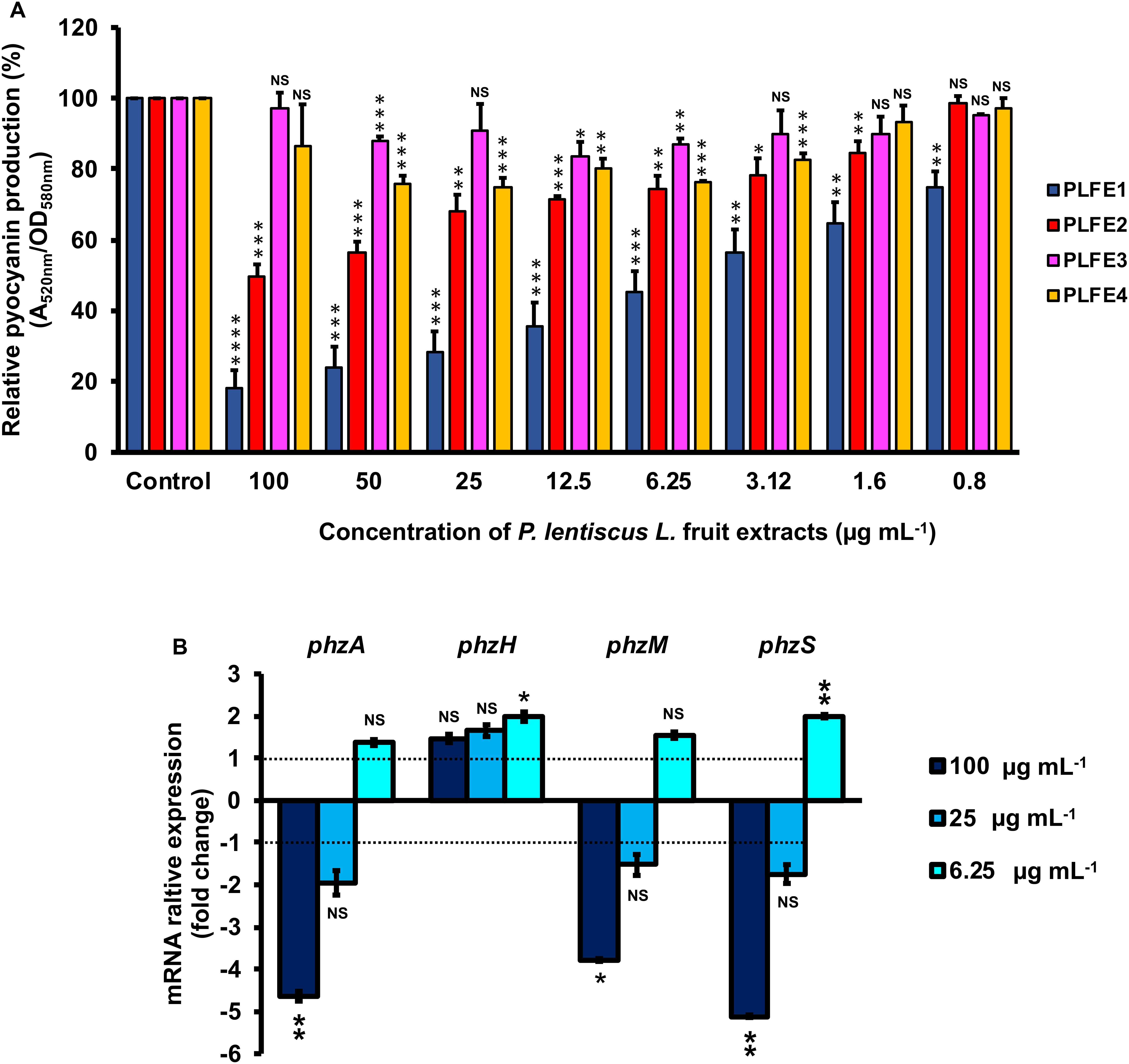
Figure 1. Pistacia lentiscus L. fruit extract (PLFE) is a potent inhibitor of pyocyanin production in Pseudomonas aeruginosa strain H103. (A) Effect of various concentrations of P. lentiscus cyclohexane (PLFE1), ethyl acetate (PLFE2), methanol (PLFE3), and water (PLFE4) extracts on pyocyanin production. (B) Relative expression levels of representative genes from phenazine biosynthesis pathway in H103 treated with PLFE1 at 100, 25, and 6.25 μg mL–1 compared to the relative mRNA levels in the control condition (H103 untreated). Values represent the mean (± SEM) of three independent assays. Statistics were achieved by a two-tailed t test: ****, P < 0.0001; ***, P = 0.0001 to 0.001; **, P = 0.001 to 0.01; *, P = 0.01 to 0.05; NS (Not Significant), P ≥ 0.05.
P. aeruginosa Virulence Attenuation by PLFE1
We next sought to evaluate anti-virulence effect of PLFE1 on P. aeruginosa strain H103 using human lung A549 cells and Caenorhabditis elegans infection models. As depicted in Figure 2A, the results showed lower LDH release (20%; P ≤ 0.05) in A549 cells after their infection with H103 treated with PLFE1 (100 μg mL–1) for 20 h incubation. A549 cells infected with non-treated strain H103 were used as a control. This result revealed that PLFE1 exhibited significant P. aeruginosa anti-virulence effect in human lung A549 cells. In addition, the impact of PLFE1 on in vivo virulence of P. aeruginosa strain H103 was assessed using a C. elegans fast-killing infection assay. When C. elegans worms were placed on a lawn of H103 strain, the percentage of nematodes survival drastically decreased (65%; P < 0.0001) after 24 h incubation as compared to C. elegans fed with E. coli OP50 (Figure 2B). Interestingly, the presence of PLFE1 (100 μg mL–1) significantly protected C. elegans from killing by P. aeruginosa (27%; P < 0.0001) as compared to C. elegans when applied to lawns of untreated H103 (Figure 2B). Altogether, these data indicate that PLFE1 attenuated the virulence of P. aeruginosa strain H103 in human lung A549 cells and C. elegans infection models.
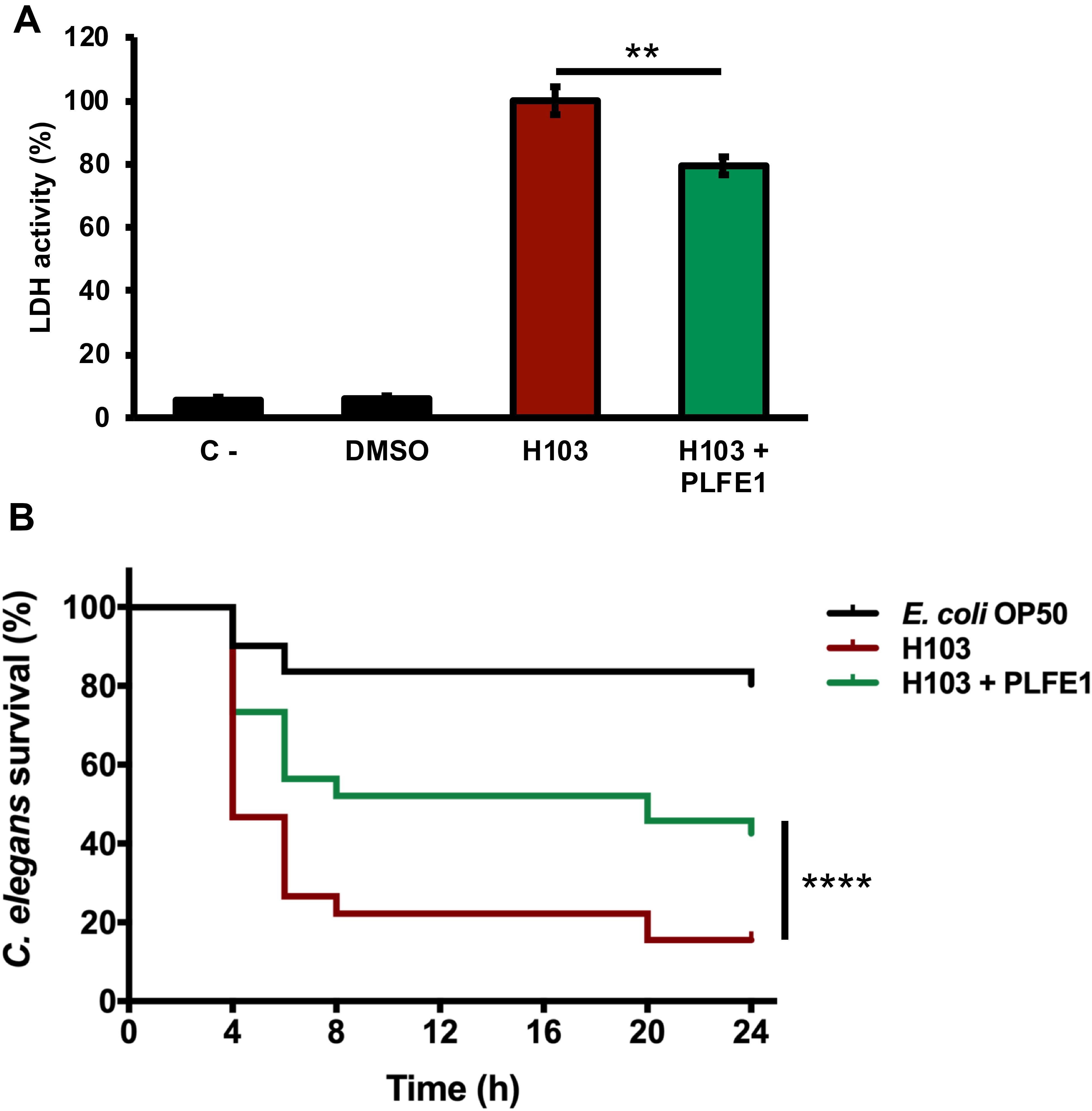
Figure 2. Virulence attenuation on Pseudomonas aeruginosa by PLFE1. (A) Anti-virulence effects of PLFE1 in human A549 lung cells infection model. The presence of PLFE1 (100 μg mL–1) significantly protected A549 lung cells from lysis after 20 h infection. Data are presented as the mean ± SEM values of four independent experiments performed in duplicate. ** P = 0.001 to 0.01 (two-tailed t test) versus untreated cells. (B) Pseudomonas aeruginosa H103 virulence attenuation in a C. elegans infection model by PLFE1. Sixty L4-stage nematodes per experimental group were placed on lawns of E. coli OP50 (black) or H103 strain in the absence (red) or presence of PLFE1 at 100 μg mL–1 (green). Alive nematodes were scored at 4-, 6-, 8-, 20- and 24-h after the start of the assay. ****, P < 0.0001 (log-rank [Mantel-Cox] test) versus untreated cells.
Absence of Effect of PLFE1 on P. aeruginosa Growth and Cell Viability
The impact of PLFE1 (cyclohexane extract) on the cell growth of P. aeruginosa H103 was monitored at 37°C over the course of 24 h. None of the concentrations assayed (100, 50, 25, 12.5, 6.25, 3.12, 1.6 and 0.8 μg mL–1) had an impact on planktonic cell growth of H103 strain as compared to the untreated culture (H103 strain grown in presence of 1 % v/v DMSO) (Supplementary Figure S1A). Moreover, the effect of PFLE1 on H103 cell viability was evaluated by flow cytometry using BacLight live/dead stain. Similarly, the results showed that at all the concentrations tested, PLFE1 did not affect the cell viability according to normalized events counting of live, injured, and dead cells when compared to the control condition (Supplementary Table S1 and Supplementary Figure S1B). Based on these results, it can be concluded that the inhibition of pyocyanin pigment production by P. lentiscus L. fruit extract (PLFE1) was achieved without affecting the growth of the bacteria and cell viability, at least in vitro.
PLFE1 Modulates the Production of 4-Hydroxy-2-Alkylquinolines (HAQs) Molecules
We next sought to assess whether the inhibition of pyocyanin production was directly due to PLFE1 on Pqs QS system that is known to tightly regulate pyocyanin biosynthesis. P. aeruginosa H103 cultures were exposed to different concentrations of PLFE1 (100, 50, 25, 12.5, 6.25, 3.12, 1.6 and 0.8 μg mL–1) and then HAQs molecules were extracted twice by ethyl acetate. The production of HAQs was determined using a PAO1 ΔpqsA CTX-pqsA::lux biosensor strain which does not produce HAQs molecules and shows response to exogenous HAQs. The H103 cultures treated with 100, 50, and 25 μg mL–1 of PLFE1 showed significant reduced HAQs production (Figure 3A). Additionally, we measured the expression levels of pqsA, pqsH, pqsL, and pqsR genes representative of Pqs QS system by using RT-qPCR. The assays were performed after 24 h of growth of H103 untreated or treated with 100, 25, and 6.25 μg mL–1 PLFE1 (Figure 3B). The pqsA, pqsH and pqsL genes involved in the biosynthesis of the major molecules from the HAQs family, namely 3,4-dihydroxy-2-heptylquinoline [termed the Pseudomonas quinolone signal (PQS)], its precursor 4-hydroxy-2-heptylquinoline (HHQ) and 4-hydroxy-2-heptylquinoline N-oxide (HQNO) were all significantly down-regulated upon exposure to PLFE1 at 100 and 25 μg mL–1. However, the expression of pqsR (mvfR) encoding for the cognate receptor/regulator of HHQ and PQS molecules did not change in the presence of 100 and 25 μg mL–1 PLFE1. Taken together, these data indicate that PLFE1 extract reduces gene expression levels of the Pqs QS system that correlates with the reduction in HAQs molecules production, suggesting that PLFE1 exhibits its anti-pyocyanin production effect possibly through an anti-QS activity.
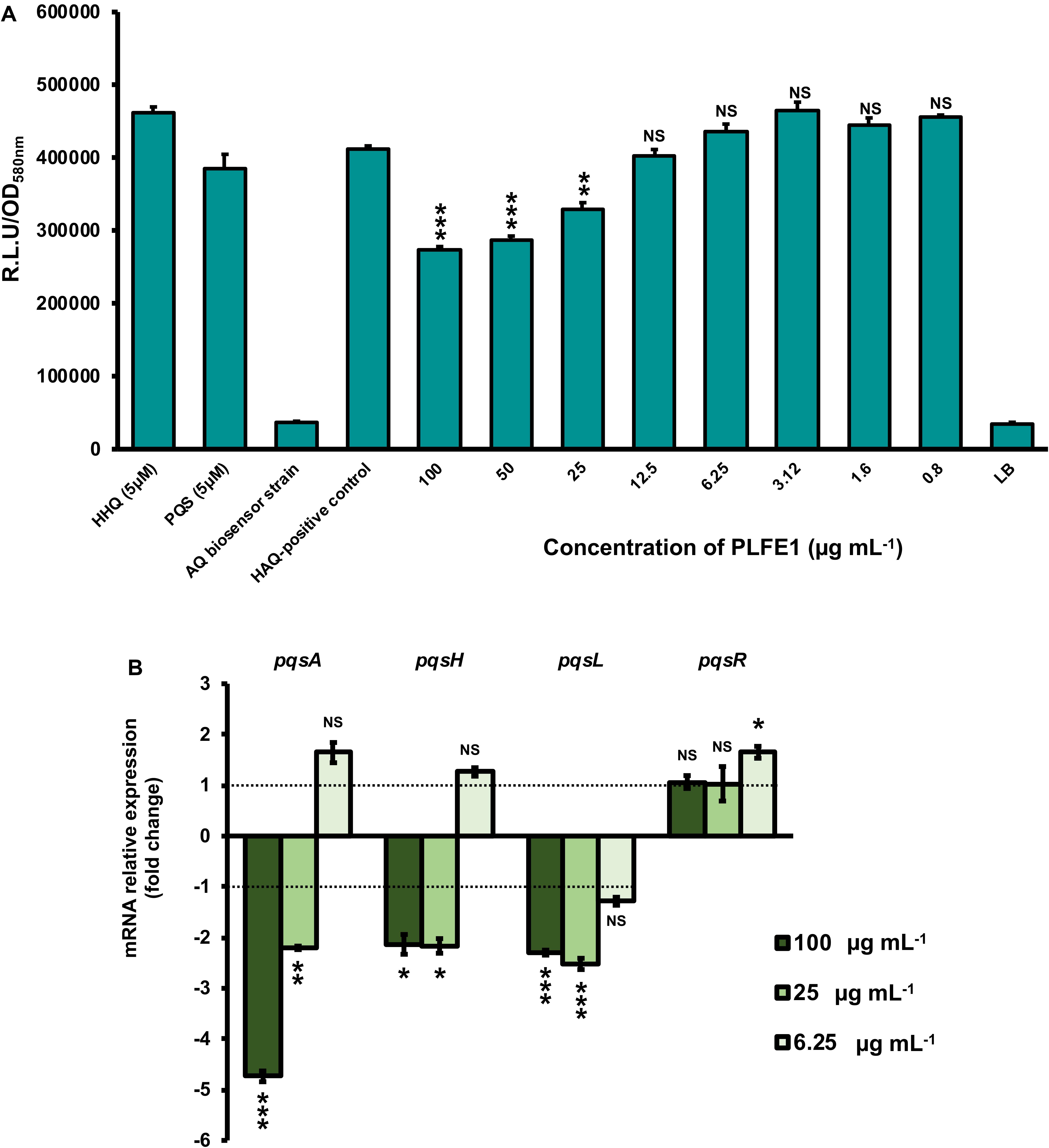
Figure 3. PLFE1 decreases HAQ-molecules production. (A) Normalized mean maximal bioluminescence output from the HAQ-biosensor strain in the presence of crude ethyl acetate HAQs extracts prepared from cultures of H103 treated with different concentrations of PLFE1 compared to positive control conditions (HHQ and PQS at 5 μM; HAQs crude ethyl acetate extract from H103 untreated) and to negative control conditions (HAQs crude ethyl acetate extract from HAQ-biosenor strain and LB medium). (B) Relative expression levels of representative genes from HAQ QS system in H103 treated with PLFE1 at 100, 25, and 6.25 μg mL–1 compared to the relative mRNA levels in the control condition (H103 untreated). Values represent the mean (± SEM) of three independent assays. Statistics were achieved by a two-tailed t test: ***, P = 0.0001 to 0.001; **, P = 0.001 to 0.01; *, P = 0.01 to 0.05; NS (Not Significant), P ≥ 0.05.
PLFE1 Leads to Increased Membrane Stiffness
Further, we evaluated the effect of PLFE1 on P. aeruginosa strain H103 membrane fluidity homeostasis. Planktonic cell growths of H103 exposed to PLFE1 at concentrations of 100, 50, 25, 12.5, 6.25, 3.12, 1.6 and 0.8 μg mL–1 were assayed for fluorescence anisotropy (FA) using 1,6-diphenyl-1,3,5-hexatriene (DPH) fluorescent probe (Figure 4A). Anisotropy of H103 cells increased in a concentration dependent-manner to achieve a maximum FA value of 0.223 ± 0.003 (P < 0.001) at a concentration of 100 μg mL–1 of PLFE1 in comparison to the FA value of untreated control (0.186 ± 0.002). This result reflects a significant decrease of approximately 20% of membrane fluidity (membrane rigidification) revealing physiological changes and adaptations in the cellular envelope of H103 in response to PLFE1 exposure. Interestingly, mRNA expression levels of sigX encoding the extracytoplasmic function sigma factor (ECFσ) SigX that is required to maintain cell envelope integrity, and its known representative targets accA, accB, and fabY that are involved in fatty acid biosynthesis were significantly decreased upon exposure of H103 strain to PLFE1 at 100 and 25 μg mL–1 when compared to mRNA expression levels in the control condition (untreated H103) (Figure 4B). Further, sigX expression was monitored during growth in the presence of PLFE1 at 100, 25 and 6.25 μg mL–1 by using a transcriptional fusion construction where the sigX promoter region was fused to the promoter-less luxCDABE cassette in the replicative pAB133 vector (pAB-PsigX) (Supplementary Figure S2). Remarkably, in the presence of PLFE1, the relative bioluminescence output from H103 harboring pAB-PsigX increased in a dose dependent-manner reaching significant maximal activity at 100 μg mL–1 during the transition from the late exponential phase to early stationary phase (Figure 4C; Supplementary Figure S2A). However, bioluminescence activity of pAB-PsigX decreased significantly in a concentration dependent-manner during the late stationary phase achieving minimal activity at 100 μg mL–1 of PLFE1 (Figure 4C; Supplementary Figure S2A), in line with the RT-qPCR results presented in Figure 4B. These results reveal that P. aeruginosa exposure to PLFE1 induced increased membrane stiffness suggesting alterations in envelope homeostasis most likely through the activation of the cell wall stress ECFσ SigX. To further validate this hypothesis, we used a ΔsigX deletion mutant (Bouffartigues et al., 2012) to determine the FA in the absence or presence of various concentrations of PLFE1. The FA values appeared to be unaffected in ΔsigX in response to PLFE1 exposure compared to the control condition (untreated ΔsigX) (Supplementary Figure S2A). Altogether, these data indicate that PLFE1 contains bioactive membrane-interactive compounds that reduce membrane fluidity, in which the ECFσ SigX seems to play a key role.
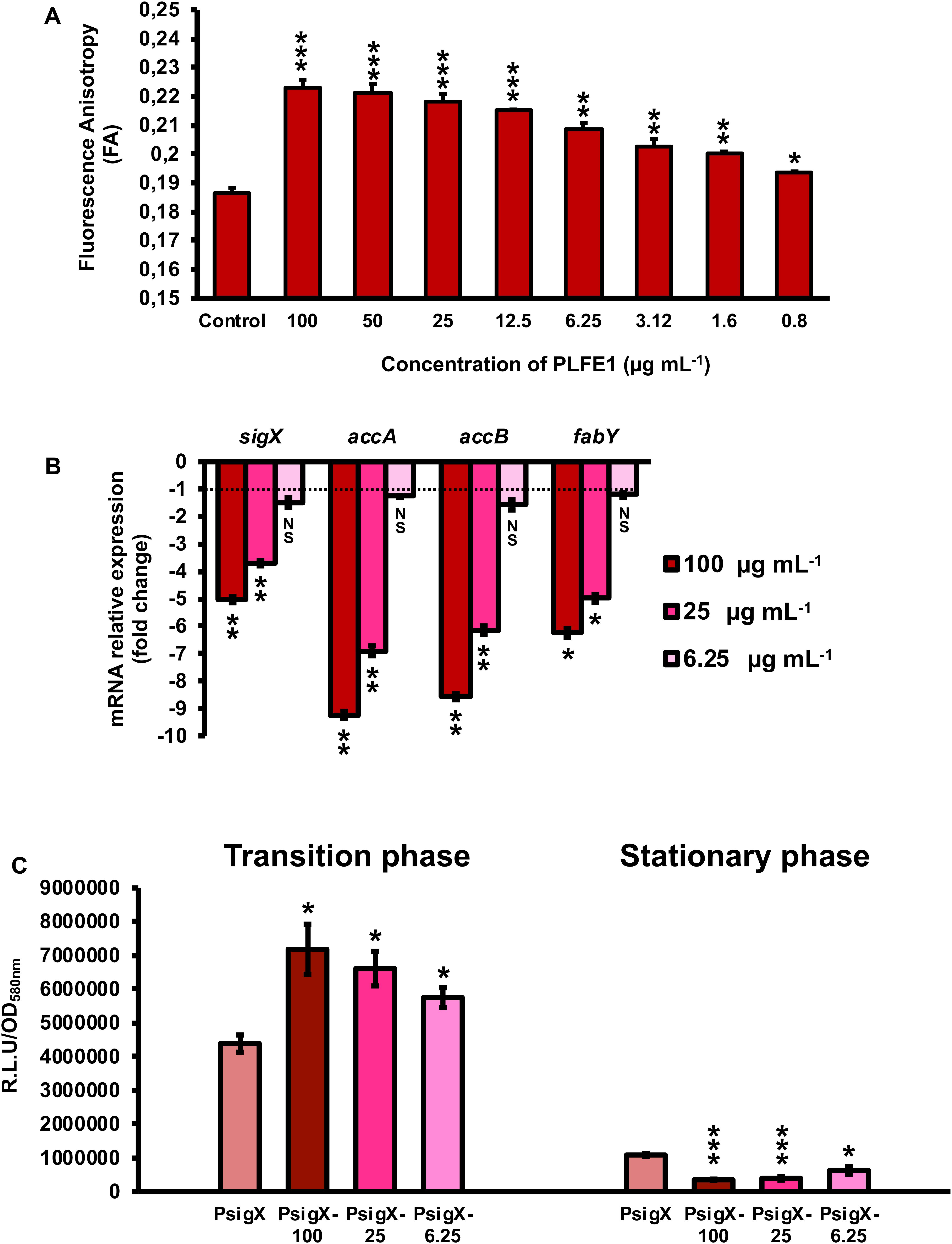
Figure 4. PLFE1 induces membrane stiffness. (A) Fluorescence anisotropy (membrane fluidity) measurements in Pseudomonas aeruginosa strain H103 exposed to various concentrations of PLFE1 compared to the control condition (H103 untreated). (B) Relative expression levels of sigX encoding the SigX extracytoplasmic function sigma factor (ECFσ) and its known target genes (accA, accB, and fabY) involved in fatty acid biosynthesis in H103 treated with PLFE1 at 100, 25, and 6.25 μg mL–1 compared to the relative mRNA levels in the control condition (H103 untreated). (C) Relative bioluminescence levels of H103 harboring the pAB-PsigX plasmid (sigX promoter region) treated with PLFE1 at 100, 25, and 6.25 μg mL–1 compared to the relative bioluminescence levels in the control condition (H103-pAB-PsigX untreated). Data at both transition and stationary phases are displayed. Values represent the mean (± SEM) of three independent assays. Statistics were achieved by a two-tailed t test: ***, P = 0.0001 to 0.001; **, P = 0.001 to 0.01; *, P = 0.01 to 0.05; NS (Not Significant), P ≥ 0.05.
Isolation and Identification of Major Bioactive Compounds of PLFE1
To isolate and identify the major constituents of PLFE1, multiple chemical analyses were performed. The fractionation of PLFE1 by medium pressure liquid chromatography (MPLC) allowed to recover a total of 13 fractions that we labelled as PLFE1-(1-13) (Supplementary Table S2). Thin layer chromatography (TLC) examination showed that the major compounds of PLFE1 were present in the fractions PLFE1-(2-4). These last were then selected for further analyses in order to identify the main compounds present in each fraction. For PLFE1-2, 1H-NMR spectra, proton signals between 7.36 and 6.78 ppm showed the presence of a tri-substituted aromatic ring. The signal at 5.36 ppm [t J = 4.6 Hz, 2H] accounted for an olefinic double bond. The major derivative ginkgolic acid (C17:1) with a molecular formula of C24H38O3 (m/z 373.1 [M-H]–) displayed one unsaturation in its aliphatic chain. Ozonolysis reaction of PLFE1-2 allowed to localize the double bond of ginkgolic acid (C17:1) between positions C-8′ and C-9′ (Figure 5). LC-ESI-MS analyses corroborated the presence of ginkgolic acid (C17:1) in PLFE1-2 and showed the occurrence of hydroginkgolic acid (C15:0) derivative and a mixture of both ginkgolic acids (C17:1/C15:0) in PLFE1-3 and PLFE1-4, respectively (Figure 5). Altogether, these results indicate that ginkgolic acid (C17:1) and hydroginkgolic acid (C15:0) are the main metabolites in PLFE1 and might be responsible for P. aeruginosa virulence attenuation.
Ginkgolic Acid-Enriched Fractions From PLFE1 Are Involved in Virulence Attenuation of P. aeruginosa
To further assay whether GA (C17:1), GA (15:1) or a mixture of both GA (C17:1/C15:0) are the main bioactive compounds involved in the anti-virulence activity against P. aeruginosa, their effect on pyocyanin production and virulence attenuation in human lung A549 infection model were evaluated. Consistent with the results of pyocyanin inhibition by the crude extract PLFE1, H103 strain treated with GA-enriched fractions showed a dose-dependent reduction of pyocyanin production when compared to untreated H103 (Figure 6A). Interestingly, the fractions enriched in GA (C17:1) or GA mix of (C17:1)/(C15:0) showed approximately 90% pyocyanin inhibition activity at 100 μg mL–1. The GA (C17:1) and GA (C15:0) displayed an IC50 of 6.3 μg mL–1 (16.75 μM) and 12.5 μg mL–1 (35.75 μM), respectively (Supplementary Figure S3). Looking at virulence attenuation, H103 strain exposed to GA-enriched fractions showed significant reduced LDH release (20%) as compared to the control condition (H103 untreated) revealing that GA-enriched fractions were able to protect the human lung A549 line cells (Figure 6B). Taken together, these results provide important insights into the involvement of GA-enriched fractions on the anti-virulence activity against P. aeruginosa.
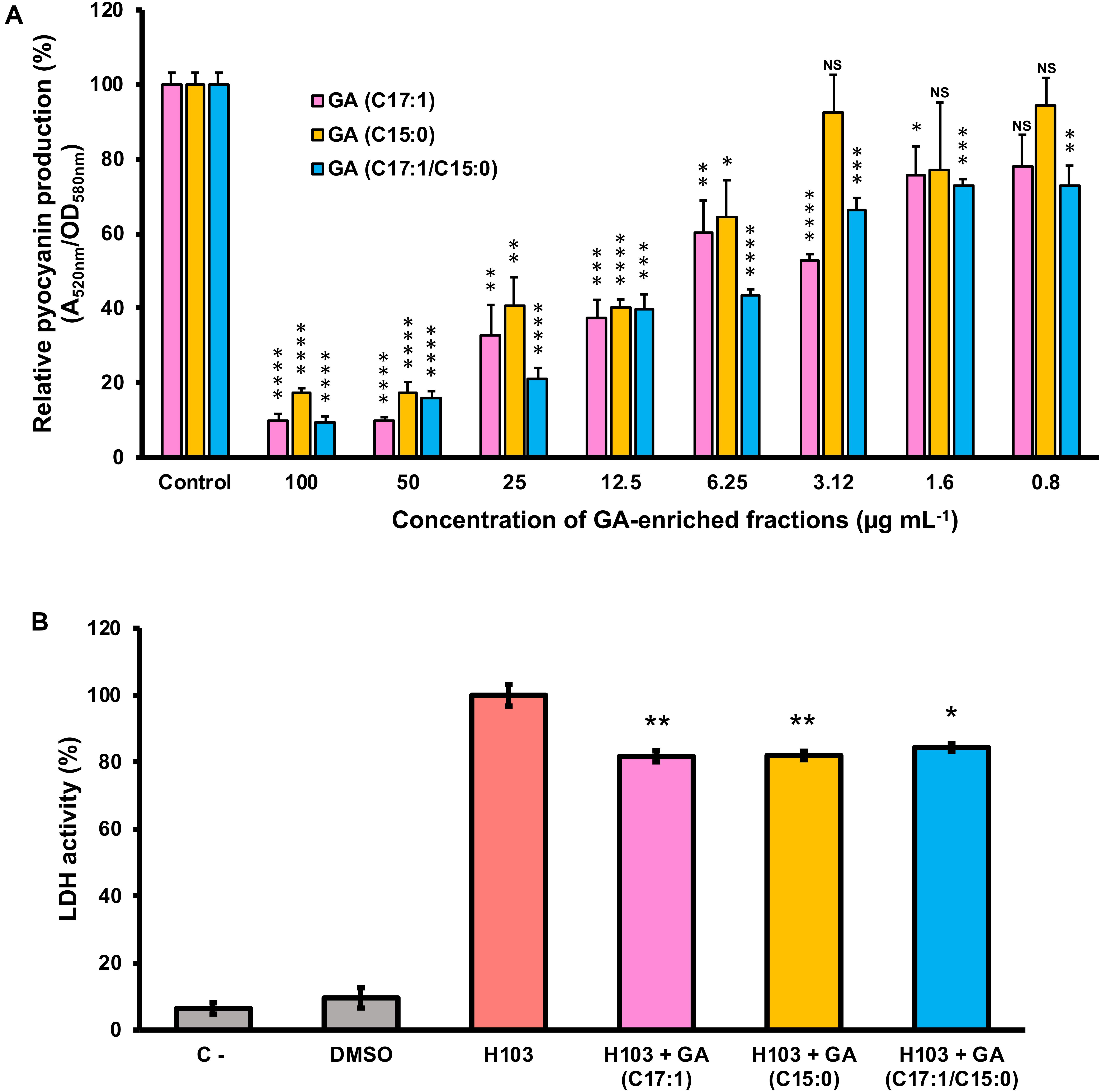
Figure 6. Effect of GA-enriched fractions from PLFE1 on Pseudomonas aeruginosa virulence. (A) Pyocyanin production upon exposure to different concentrations of GA-enriched fractions. (B) Anti-virulence of GA-enriched in human A549 lung cells infection model. The presence of GA-enriched fractions (100 μg mL–1) significantly protected A549 lung cells from lysis after 20 h infection. Data are presented as the mean ± SEM values of four independent experiments performed in duplicate. Statistics were achieved by a two-tailed t test: ****, P < 0.0001; ***, P = 0.0001 to 0.001; **, P = 0.001 to 0.01; *, P = 0.01 to 0.05; NS (NotSignificant), P ≥ 0.05.
Cytotoxicity of PLFE1 and GA-Enriched Fractions
Our last goal for this study was to investigate the potential of PLFE1 and its GA-enriched fractions to induce cytotoxicity in the human lung A549 cells. As depicted in Figure 7A, no significant differences in cytotoxicity levels were found between A549 cells treated at various concentrations of crude extract PLFE1 after 1 h incubation as compared to the control condition (untreated A549 cells). However, a moderate cytotoxic effect was observed when A549 cells were incubated for more than 3 h in the presence of PLFE1 at concentrations above 12.5 μg mL–1. Remarkably, when A549 cells were treated with PLFE1 at IC50 value of pyocyanin activity inhibition (4.9 μg mL–1) there was no differences in cytotoxicity at different time points incubation assayed (1, 3, 6, and 24 h) (Figure 7B). Moreover, similar interesting trend results were obtained for the GA-enriched fraction mix of both GA (C17:1/C15:0) when tested at 5.4 μg mL–1 IC50 value of pyocyanin inhibition (Figure 7B). On the other hand, after 3 h incubation, a slight cytotoxicity increase was observed when A549 cells were exposed to enriched fractions of GA (C17:1) and GA (C15:0) separately at their IC50 values (6.3 and 12.5 μg mL–1, respectively) (Figure 7B). However, this cytotoxicity increased by about 3-fold after 24 h exposure as compared to the control condition (A549 cells treated with DMSO). In summary, these results indicate that PLFE1 and its complex GA-enriched fraction (C17:1/C15:0) displayed no significant cytotoxicity in the human lung A549 line cells when tested at their IC50 pyocyanin inhibition values.
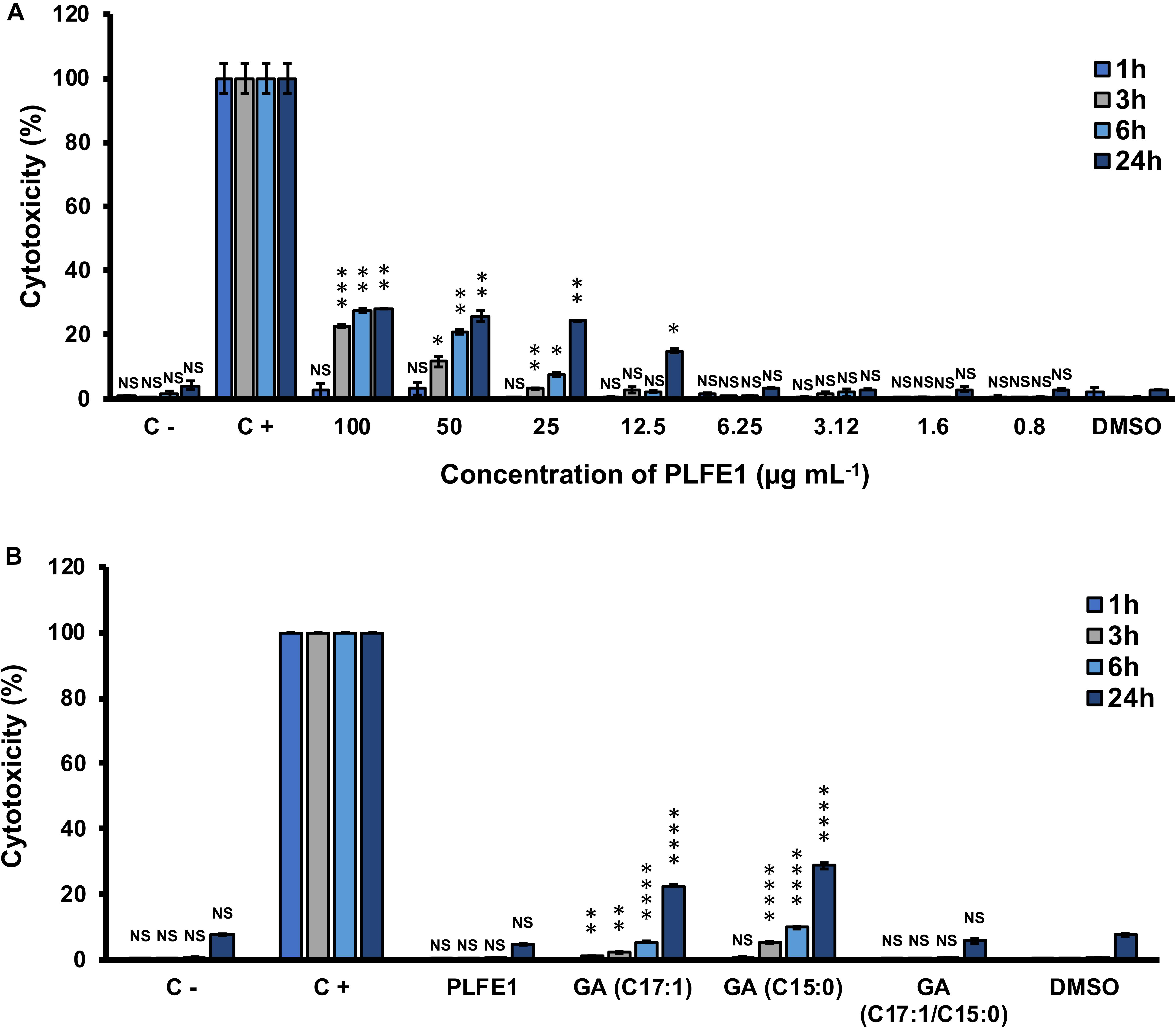
Figure 7. Cytotoxic effect of PLFE1 and its GA-enriched fractions on human lung A549 line cells. (A) PLFE1 was assayed at different concentrations. (B) Cytotoxicity of GA-enriched fractions exhibiting pyocyanin production inhibition. LDH release was determined at 1, 3, 6, and 24 h. DMSO was used as a vehicle control. Data are displayed as the mean ± SEM values of four independent experiments performed in duplicate. Statistics were achieved by a two-tailed t test: ****, P < 0.0001 ***, P = 0.0001 to 0.001; **, P = 0.001 to 0.01; *, P = 0.01 to 0.05; NS (Not Significant), P ≥ 0.05.
Discussion
Antibiotic-resistant pathogens are threatening individuals and public health over the world to a such extent that new therapeutic strategies need to be developed urgently (Wagner et al., 2016; WHO, 2017). The anti-virulence approaches represent an attracting alternative to the unmet clinical need in the treatment of bacterial infections (Rasko and Sperandio, 2010; Dickey et al., 2017). These new therapies target essentially the prevention of virulence factors production by pathogens, rather than their survival, and they also aim to thwart the regulatory mechanisms controlling their expression (Soukarieh et al., 2018; Martínez et al., 2019). In addition, by targeting essential virulence determinants, it is possible that the emergence and spread of bacterial resistance will be reduced due to decreased selective pressure and the natural microbiota will be preserved. In line with this scenario, the current study was designed to explore new anti-virulence agents to fight against the multidrug-resistant P. aeruginosa that is responsible for many different infections. Thus, we based our research on the traditional medicine reported to treat several infectious diseases. In particular, the fruit of P. lentiscus L. is used for the treatment of respiratory tracts infections, and in ointments for articular pain, burn wounds, and ulcer among other traditional uses (Bozorgi et al., 2013; Rauf et al., 2017).
Our findings show that P. lentiscus L. fruit cyclohexane extract (PLFE1) contains potent inhibitory compounds that restrict P. aeruginosa virulence by abolishing pyocyanin pigment production, which is known to facilitate colonization of the host and subsequent infection (Lau et al., 2004; Hall et al., 2016). This anti-virulence activity does not perturb growth and cell viability, which can minimize selective pressures towards the development of resistance compared to conventional antibiotics (Rasko and Sperandio, 2010). Nonetheless, additional investigations need to be undertaken to assess whether P. aeruginosa can develop resistance towards PLFE1 upon repeated exposure. Further, by exploring the mechanistic behind the anti-pyocyanin activity, we have shown that PLFE1 interferes with the HAQs QS-molecules production. Since for P. aeruginosa infections QS is the master regulator involved in the expression of many virulence factors such as phenazines, exoproteases (elastase, alkaline protease), siderophores, and toxins among others, its inhibition represents a valuable adjuvant therapy that might be used to potentiate the activity of the available antibiotics applied to handle early P. aeruginosa infections (Soukarieh et al., 2018; Martínez et al., 2019). In the last five years there was a large amount of published investigations reporting natural products highlighting their potential in targeting bacterial virulence factors (Silva et al., 2016). Several plant-derived compounds that belong to alkaloids, organosulfurs, coumarins, flavonoids, phenolic acids, phenylpropanoids, terpenoids among other chemical classes have been found to be active against pyocyanin pigment production (Silva et al., 2016). However, there is still a significant lack on the specific underlying molecular mechanisms of action.
One important finding of the present study was that membrane stiffness in P. aeruginosa has significantly increased upon exposure to PLFE1. From the data of SigX transcriptional fusion analysis, we assumed that PLFE bioactive compounds interact with P. aeruginosa cell membranes leading to enhanced promoter activity of sigX during the late exponential and early stationary phases in a dose-dependent manner, thereby, triggering a cell wall stress response. Then, at late stationary phase, the activity of sigX decreased, thereby, resulting in increased membrane stiffness as an adaptation response. Furthermore, we demonstrate that PLFE1 decreases the expression of the ECFσ SigX, which is involved in the regulation of membrane lipid composition (Boechat et al., 2013; Blanka et al., 2014), and thus, triggers modifications on membrane fluidity and homeostasis. Accordingly, our data show a decreased expression levels of accA and accB encoding subunits of the biotin-dependent enzyme acetyl-CoA carboxylase complex (ACC) that catalyzes the first step in fatty acid biosynthesis in P. aeruginosa (Kondakova et al., 2015). In addition, fabY (PA5174) encoding the β-keto acyl synthase (FabY) involved in fatty acid biosynthesis, is another gene that showed decreased expression in presence of the PLFE1. Interestingly, these results mirror those observed in P. aeruginosa sigX mutant and over-expressing strains when compared to the wild-type strain (Boechat et al., 2013; Blanka et al., 2014; Fléchard et al., 2018). Moreover, ΔsigX cells display reduced membrane fluidity (membrane rigidification), HAQs QS-molecules production, pyocyanin pigment production, and virulence towards a C. elegans model (Gicquel et al., 2013; Fléchard et al., 2018). Overall, these data provide evidences that PLFE1 most likely contain membrane-interactive compounds, as it is the case for many plant extracts (Kopec̈ et al., 2013; Tsuchiya, 2015). PLFE1 interaction with membrane lipid bilayers seems to be involved in the increased membrane stiffness, which might trigger the modulation of the ECFσ SigX, which in turn regulates, directly or indirectly, the expression of genes involved in production of secreted factors such as the virulence factor pyocyanin, HAQs QS-molecules, fatty acid biosynthesis among others as being part of its large regulon (Schulz et al., 2015). Thus, our discoveries propose that PLFE1 exerts its anti-virulence activity through a new potential mechanism that at least involves the ECFσ SigX since no impact of PLFE1 was observed in membrane fluidity of the ΔsigX mutant strain.
Herein, the fractionation of PLFE1 led to the isolation and identification of fractions mainly enriched in C17:1 and C15:0 ginkgolic acids (GA) or a mix of both GA (C17:1/C15:0). To the best of our knowledge, the presence of these chemical constituents in P. lentiscus L. fruit has never been previously reported. However, according to the literature, GA has already been isolated from Ginkgo biloba that has long been used in traditional Chinese medicine (Mahadevan and Park, 2008). The GA are a mixture of several 2-hydroxy-6-alkybenzoic acid congeners that differ in carbon alkyl group length and unsaturation and are structurally similar to salicylic acid, which is reported to impact negatively the pathogenicity of P. aeruginosa PA14 strain by decreasing pyocyanin, elastase, and protease production (Prithiviraj et al., 2005). A number of pharmacological effects have been attributed to GA, such as anti-bacterial, anti-fungal, insecticidal, anti-tumor, and neuroprotective effects among others (Satyan et al., 1998; Ahlemeyer and Krieglstein, 2003; Yang et al., 2004; van Beek and Montoro, 2009). Moreover, GA are also proven to be effective as anti-biofilm molecules against bacterial pathogens such as Escherichia coli O157:H7 strain, Staphylococcus aureus, Streptococcus mutans, Salmonella spp., and Listeria spp. (He et al., 2013; Lee et al., 2014; Wu et al., 2016). In line with our results, antibacterial activity studies show that GA did not affect Gram-negative bacteria survival, however, they exhibit strong anti-microbial activity against Gram-positive bacteria (Yang et al., 2004; Choi et al., 2009; He et al., 2013; Hua et al., 2017). Interestingly, our results indicate that PLFE1 anti-virulence properties can be mostly attributed to the identified GA-enriched fractions (C17:1, C15:0, C17:1/C15:0) since they are able to function as pyocyanin production inhibitors without negative effect on bacterial growth. Moreover, these GA-enriched fractions are capable of inducing P. aeruginosa membrane rigidification (data not shown). This result is supported by the fact that the lipid soluble components of the cell wall of Gram-negative bacteria is shown to intercept GA compounds (Hua et al., 2017).
Following demonstration of PLFE1 and GA-enriched fractions for their in vitro anti-virulence properties against P. aeruginosa, we assayed them for in vivo efficacy using the human lung A549 cells and the nematode C. elegans as models host. In both infection models, the virulence of P. aeruginosa upon exposure to PLFE1 at a dose of 100 μg mL−1 is shown to be significantly attenuated at least partly as a result of decreased production of the pyocyanin virulence factor. Pyocyanin has been reported to have many damaging and toxic effects on mammalian cells most likely through a mechanism involving oxidative stress (Hall et al., 2016; Ray et al., 2015). However, because A549 cells lungs damage and fast killing of C. elegans are multifactorial, we cannot exclude that essential mediators other than pyocyanin such as hydrogen cyanide (HCN) and effectors of the type III secretion system might be involved (Mahajan-Miklos et al., 1999; Gallagher and Manoil, 2001; Engel and Balachandran, 2009; Cezairliyan et al., 2013). Further, the evaluation of the cytotoxicity of PLFE1 and GA molecules using A549 lung human cells indicated no or slight cytotoxicity at IC50 values. Taken together, these studies deliver a promising perspective for the potential future development of PLFE1 or GA molecules as an adjuvant agent to fight against P. aeruginosa infections. Nonetheless, it remains to be verified whether PLFE1 or GA molecules have an antibiotic-potentiating activity, both in vitro and in vivo, against the pathogen P. aeruginosa.
Conclusion
Overall, the data of this study suggest that the anti-virulence efficacy of PLFE1 and GA-enriched fractions might possibly be attributed to their interaction with the lipid bilayer membranes of P. aeruginosa resulting in the modification of membrane fluidity. Therefore, the increased membrane stiffness in presence of PLFE1 and GA-enriched fractions appears to be mediated through the modulation of the ECFσ SigX, which is known to be involved in the regulation of P. aeruginosa virulence. These findings need further experimental evidences, not least in terms of identifying the specific molecular mechanisms of action leading to impact the ECFσ SigX as being a potential molecular target to alter the expression of virulence in P. aeruginosa.
Materials and Methods
Bacterial Strains, Media and Growth Conditions
The P. aeruginosa H103 (Hancock and Carey, 1979), H103-pAB-PsigX (this work), and H103-ΔsigX (Bouffartigues et al., 2012) used in this study are all derivatives of P. aeruginosa wild-type PAO1. Planktonic cultures were grown aerobically for 24 h at 37°C in LB broth on a rotary shaker (180 rpm) from an initial inoculum adjusted to an OD at 580 nm of 0.08. The antibiotics stock solutions used in this study were sterilized by filtration through 0.22-μm filters, aliquoted into daily-use volumes and kept at −20°C. Each set of experiments was performed at least three times.
Collection and Preparation of P. lentiscus L. Fruit Extracts
Fruits of P. lentiscus L. were collected within the wilaya of Jijel, Algeria, in September 2016, with the collect agreement (48/MC/DGCE/DSPEC/2016) delivered by the Research Centre on Analytical Chemistry (CRAPC). A voucher herbarium specimen is deposited in the herbarium of PNAS laboratory, Université Paris Descartes (France). A sample of 8.98 g of fruits was subjected to a pressurized solvent extraction (PSE) using a Speed Extractor E-914 (Büchi) equipped with four cells (120 mL) and a collector with four flat bottom vials (220 mL) successively with cyclohexane, ethyl acetate, methanol, and water. Maximum pressure and temperature were adjusted to 100 bar and to 50°C, respectively. Two extraction cycles with a hold-on time of 15 min were performed in each case. Solvents were removed under reduced pressure. A total of 4 extracts were obtained (Supplementary Table S3). Solutions at 10 mg mL–1 were prepared in DMSO of each extract and stored at 4°C until use.
Pyocyanin Quantification Assay
To perform pyocyanin pigment quantification assay, P. aeruginosa H103 cells untreated (grown in presence of 1% DMSO) and treated with 100, 50, 25, 12.5, 6.25, 3.12, 1.6 and 0.8 μg mL–1 of P. lentiscus L. fruit extracts (PLFE1-4) or ginkgolic acid-enriched fractions (PLFE1-(2-4)) were grown on 96-well microtiter plate for 24 h at 37°C on a rotary shaker (180 rpm). Then, supernatants samples were collected by centrifugation and extracted with chloroform. The chloroform layer (blue layer) was acidified by adding 0.5 M HCl. The absorbance of the HCl layer (pink layer) was recorded at 520 nm using the Spark 20 M multimode Microplate Reader controlled by SparkControlTM software Version 2.1 (Tecan Group Ltd.) and the data were normalized for bacterial cell density (OD580nm).
Virulence Attenuation of P. aeruginosa Using Human Lung A549 Line Cells
The human lung A549 cells were cultured in Dulbecco’s Modified Eagle’s Medium (DMEM, Lonza, BioWhittaker®) supplemented with 4.5 g L–1 glucose, L-Glutamine, 10% heat-inactivated (30 min, 56°C) fetal bovine serum (FBS), and 100 Units mL–1 each of penicillin and streptomycin antibiotics. Cells were grown at 37°C under the atmosphere of 5% CO2 and 95% air with regularly medium change until a confluent monolayer was obtained. The anti-virulence effect of PLFE1 or ginkgolic acid-enriched fractions (PLFE1-(2-4)) on P. aeruginosa H103 was determined using an enzymatic assay (PierceTM LDH Cytotoxicity Assay Kit, Thermo ScientificTM), which measures lactate dehydrogenase (LDH) released from the cytosol of damaged A549 cells into the supernatant. After overnight incubation with P. aeruginosa H103 (108 CFU mL–1) previously treated or untreated (control condition), the supernatants from confluent A549 monolayers grown on 24-well tissue culture plates were collected and the concentration of the LDH release was quantified. A549 cells exposed to 1X Lysis Buffer were used as a positive control of maximal LDH release (100% lysis) as specified by the manufacturer’s recommendations. The background level (0% LDH release) was determined with serum free culture medium. The LDH release assays were also used to determine the cytotoxicity of PLFE1 or ginkgolic acid-enriched fractions (PLFE1-(2-4)) in A549 cells upon direct exposure after 1-, 3-, 6-, and 24-h incubation.
Caenorhabditis elegans Fast-Killing Infection Assay
The C. elegans wild-type Bristol strain N2 worms were grown at 22°C on nematode growth medium (NGM) agar plates using Escherichia coli OP50 as a food source. The P. aeruginosa-C. elegans fast-kill infection assay was performed as described previously (Blier et al., 2011) with minor modifications. Briefly, cultures of P. aeruginosa H103 strain untreated or treated with PLFE1 at 100 μg mL–1 were seeded on a 24-well plate containing in each well 1 mL of Peptone-Glucose-Sorbitol (PGS) agar. Control wells were seeded with 25 μL of E. coli OP50 from an overnight culture. The plate was then incubated at 37°C for 24 h to make bacterial lawns and then shifted to 22°C for 4 h. For each assay, 15 to 20 L4-synchronized worms were added to the killing and control lawns and incubated at 22°C. Worm survival was scored at 4-, 6-, 8-, 20-, and 24-h after the start of the assay, using an Axiovert S100 optical microscope (Zeiss).
Bacterial Cell Viability Assays by Flow Cytometry
Cell viability assays of P. aeruginosa H103 cells untreated and treated by different concentrations of P. lentiscus L. fruit extract (PLFE1) were assessed by using the LIVE/DEADTM BacLightTM Bacterial Viability and Counting Kit, for flow cytometry (Invitrogen, Molecular Probes). Briefly, P. aeruginosa H103 untreated and treated suspensions collected after 24 h of incubation with shaking (180 rpm) at 37°C were washed twice and diluted in filter-sterilized PBS to reach a final density of 1 × 106 CFU mL–1, then stained following the manufacturer’s instructions. The data were acquired using CytoFlex S flow cytometer (Beckman coulter Life science). An aliquot of cells was killed with 100% ethanol and used as a control of death cells. The SYTO9 stained cells were detected by an excitation with 22 mW blue laser at 488 and with emission wavelength at 525 nm (green, with band pass filter of 40 nm), while the PI (Propidium iodide) stained cells were detected by 690 nm (red, with band pass filter of 50 nm).
Extraction and Quantification of HAQs
Planktonic cultures of P. aeruginosa H103 untreated (control DMSO 1%) and treated by 100, 50, 25, 12.5, 6.25, 3.12, 1.6 and 0.8 μg mL–1 of PLFE1 collected after 24 h of incubation with shaking (180 rpm) at 37°C were subjected to HAQ molecules extraction following the technique described in a previous study (Tahrioui et al., 2019). HAQs were quantified by a combined spectrophotometer/luminometer microplate assay using the biosensor strain PAO1 pqsA CTX-lux::pqsA (Fletcher et al., 2007). The HAQ biosensor strain was grown overnight and OD was measured and adjusted with fresh LB medium to achieve OD580nm of 1. For each test well, 5 μL of HAQs crude extracts were diluted in 100 μL of LB and added to 100 μL of 1 in 50 dilution of the HAQ biosensor strain. Further, bioluminescence and OD580nm were monitored in specialized white sided and clear bottom 96-well microtiter plate every 15 min for 24 h at 37°C using the Spark 20M multimode Microplate Reader controlled by SparkControlTM software Version 2.1 (Tecan Group Ltd.). Both HHQ and PQS synthetic standards (Sigma-Aldrich) at a final concentration of 5 μM, used as positive controls, were added to 1 in 100 dilution of the HAQ biosensor strain, as both activate bioluminescence production. The recorded bioluminescence as relative light units (R.L.U) were normalized to OD580nm of cultures suspensions.
Membrane Fluidity Measurement by Fluorescence Anisotropy
Fluorescence anisotropy analysis of P. aeruginosa cells were performed as previously described (Fléchard et al., 2018). Briefly, cell pellets of P. aeruginosa H103 untreated (control DMSO 1%) and treated by 100, 50, 25, 12.5, 6.25, 3.12, 1.6 and 0.8 μg mL–1 of PLFE1 collected after 24 h of incubation with shaking (180 rpm) at 37°C were washed two times (7500 × g, 5 min, 25°C) in 0.01 M MgSO4 and resuspended in the same wash solution to reach an OD580nm of 0.1. One μL of a 4 mM of 1,6-diphenyl-1,3,5-hexatriene (DPH) stock solution (Sigma-Aldrich) in tetrahydrofuran was added to 1 mL aliquot of the resuspended cultures and incubated in the dark for 30 min at 37°C to allow the probe to incorporate into the cytoplasmic membrane. Measurement of the fluorescence polarization was performed using the Spark 20M multimode Microplate Reader, equipped with an active temperature regulation system (Te-CoolTM, Tecan Group Ltd.). Excitation and emission wavelengths were set to 365 and 425 nm, respectively, and the Fluorescence Anisotropy (FA) was calculated according to Lakowicz (2006). Three measurements were performed for each sample and data were recorded using SparkControlTM software (Version 2.1, Tecan Group Ltd.). The relationship between fluorescence polarization and membrane fluidity is an inverse one, where increasing anisotropy values correspond to a more rigid membrane and vice versa. All values are reported as means of triplicate analyses for each experimental variable.
Transcriptional Fusion PsigX::luxCDABE Construction and sigX Promoter Activity Analysis in Response to PLFE1
The promoter region of the sigX gene (PsigX) was amplified by PCR with primers PsigX-SacI-F and PsigX-SpeI-R (Supplementary Table S4) incorporating SacI and SpeI linkers, respectively. The promoter region of sigX gene was fused to the luxCDABE cassette in the promoterless pAB133 vector (GmR) (Bazire et al., 2010). Upon amplification, DNA was digested with the appropriate restriction enzymes, and cloned into pAB133 generating pAB-PsigX vector. The pAB-PsigX plasmid was then transformed separately into one shotTM E. coli TOP10 competent cells (Invitrogen). The constructions were confirmed by DNA sequencing (Sanger sequencing services, Genewiz). Finally, the plasmids were transferred by electroporation into P. aeruginosa H103 strain. For bioluminescence assays, P. aeruginosa H103 strains harboring pAB-PsigX reporter vector and pAB133 promoter-less vector were grown in LB medium supplemented with gentamycin at 50 μg mL–1. Then, untreated and treated cultures with PLFE1 at 100, 25, and 6.25 μg mL–1 were prepared from an initial inoculum adjusted to an OD580nm value of 0.08 in LB (without gentamycin) in specialized white sided 96-well microtiter plates with a flat transparent bottom (NuncTM MicroWellTM, Thermo ScientificTM). Bioluminescence and growth (OD580nm) were monitored every 15 min for 24 h at 37°C with shaking (180 rpm) using the Spark 20M multimode Microplate Reader controlled by SparkControlTM software Version 2.1 (Tecan Group Ltd.). The relative light units (R.L.U) of the negative control strain (P. aeruginosa harboring the promoter-less vector pAB133) were subtracted from those containing the studied promoter. The bioluminescence values in R.L.U and Log(OD580nm) values were plotted.
Reverse Transcription-Quantitative PCR Analyses (RT-qPCR)
Total RNAs from three independent H103 untreated and treated cultures with PLFE1 at 100, 25, and 6.25 μg mL–1 grown for 24 h with shaking (180 rpm) at 37°C were isolated by the hot acid-phenol method (Bouffartigues et al., 2012) followed by treatment with Turbo DNA-freeTM kit (Invitrogen) according to the manufacturer’s protocol. Synthesis of cDNAs and RT-qPCR were achieved as previously described (Bouffartigues et al., 2015) using the oligonucleotides listed in Supplementary Table S4. The mRNAs levels were calculated by comparing the threshold cycles (Ct) of target genes with those of control sample groups and the relative quantification was measured using the 2–ΔΔCt method (Pfaffl, 2001) using DataAssistTM software (Applied Biosystems).
PLFE1 Fractionation
Two hundred mg of PFLE1 were subjected to fractionation by medium MPLC on 9.6 g of silice 60 M with cyclohexane/ethyl acetate as mobile phase solvent mixture with increasing polarity. A total of 13 fractions were recovered and designated PLFE1-(1-13) (Supplementary Table S2).
Structure Determination of Compounds Present in PLFE1-(2-4) Fractions by NMR and Ozonolysis
To determine the structure of the main compounds of PLFE1-(2-4), 1D and 2D NMR experiments were conducted in a Bruker 400 MHz spectrometer apparatus and recorded in deuterated chloroform (CDCl3). To identify the double bond position of the main compound identified in PLFE1-2, an ozonolysis reaction was carried out. Briefly, a solution of 10 mg (0.027 mmol, 1.0 equiv) of PLFE1-2 was put in a round bottom flask containing 20 mL of distilled dichloromethane and a small amount of Sudan III. A stream of ozone (O3) was bubbled into the solution at −78°C until the pink solution became colorless (10 min). Then, 200 μL of dimethyl sulfide [(CH3)2S] (2.7 mmol, 100 equiv) were added to the reaction mixture and stirred for 1 h at 20°C. From the reaction mixture, 1 μl was directly analyzed by GC-MS.
Gas Chromatography-Mass Spectrometry (GC-MS) Analyses
The GC-MS analyses were carried out in a Hewlett Packard GC 6890/MSD 5972 apparatus equipped with HP-5 (30 m × 0.25 mm × 0.25 μm). Carried gas was Ar with a flow of 0.9 mL min–1, split 1:20, oven was programmed increasing from 180°C to 270°C at 8°C min–1 with an initial and final hold of 1 and 65 min, respectively. The inlet and GC-MS interface temperature were kept at 240°C. The temperature of EI (electron impact) 70 eV was 220°C with full scan (80-500 m/z). The injection volume was 1 μL. Identification of constituents was achieved by comparing their mass spectra with those of the Mass Spectra Library (NIST 98) compounds.
Statistical Analyses
Statistical significance was evaluated using R1 (R Development Core Team, 2017). The data were statistically analyzed using two-sample unpaired two-tailed t test to calculate P-values. The mean with standard error of the mean (SEM) of at least three independent biological experiments were calculated and plotted. C. elegans survival curves were prepared using R to perform a statistical log-rank (Mantel-Cox) test.
Data Availability Statement
The authors declare that all relevant data supporting the findings of the study are available in this article and its Supplementary Material, or from the corresponding author upon request.
Author Contributions
AT, SyC, EB, SB, RG, and OL conceived and designed the experiments. AT, SO, OA, NB, OM, and DT conducted experiments and analyzed the data. AT, SyC, PC, EB, SO, SmC, MK, SM, MF, and NO contributed to the writing of the manuscript. All authors proofread the final draft and approved the final manuscript.
Funding
The LMSM is supported by the Région Normandie (France), Evreux Portes de Normandie (France) and European FEDER funds. PNAS and CRAPC are partners of the European Union’s H2020-MSCA-RISE-2015 EXANDAS (Exploitation of aromatic plants’ by-products for the development of novel cosmeceuticals and food supplements, Grant Agreement 691247). AT is supported by a post-doctoral fellowship from European Union (FEDER) and Région Normandie (France). DT is supported by a doctoral fellowship from the Région Normandie (France). OCA was the recipient of a postgraduate fellowship from Campus France and FUNAI (Nigeria). SO is supported by a CONICYT’s scholarship (Comisión Nacional de Investigación Científica y Tecnológica from Chile). The funders had no role in study design, data collection and interpretation, or the decision to submit this work for publication. The Caenorhabditis elegans wild-type Bristol strain N2 was provided by the Caenorhabditis Genetics Center (CGC), which is funded by NIH Office of Research Infrastructure Programs (P40 OD010440).
Conflict of Interest
The authors declare that the research was conducted in the absence of any commercial or financial relationships that could be construed as a potential conflict of interest.
Acknowledgments
We gratefully acknowledge Prof. Paul Williams (Centre for Biomolecular Sciences, University of Nottingham) for providing PAO1 ΔpqsA CTX-pqsA::lux biosensor strain and Mr. Abdelhamid Boudjerda for plant material collection.
Supplementary Material
The Supplementary Material for this article can be found online at: https://www.frontiersin.org/articles/10.3389/fmicb.2020.01068/full#supplementary-material
Footnotes
References
Ahlemeyer, B., and Krieglstein, J. (2003). Neuroprotective effects of Ginkgo biloba extract. Cell. Mol. Life Sci. 60, 1779–1792. doi: 10.1007/s00018-003-3080-1
Allen, L., Dockrell, D. H., Pattery, T., Lee, D. G., Cornelis, P., Hellewell, P. G., et al. (2005). Pyocyanin production by Pseudomonas aeruginosa induces neutrophil apoptosis and impairs neutrophil-mediated host defenses in vivo. J. Immunol. 174, 3643–3649. doi: 10.4049/jimmunol.174.6.3643
Al-saghir, G. M., and Porter, M. D. (2012). Taxonomic revision of the Genus pistacia L. (Anacardiaceae). Am. J. Plant Sci. 3, 12–32. doi: 10.4236/ajps.2012.31002
Bazire, A., Shioya, K., Soum-Soutéra, E., Bouffartigues, E., Ryder, C., Guentas-Dombrowsky, L., et al. (2010). The sigma factor AlgU plays a key role in formation of robust biofilms by nonmucoid Pseudomonas aeruginosa. J. Bacteriol. 192, 3001–3010. doi: 10.1128/JB.01633-09
Blanka, A., Schulz, S., Eckweiler, D., Franke, R., Bielecka, A., Nicolai, T., et al. (2014). Identification of the alternative sigma factor SigX regulon and its implications for Pseudomonas aeruginosa pathogenicity. J. Bacteriol. 196, 345–356. doi: 10.1128/JB.01034-13
Blier, A. S., Veron, W., Bazire, A., Gerault, E., Taupin, L., Vieillard, J., et al. (2011). C-type natriuretic peptide modulates quorum sensing molecule and toxin production in Pseudomonas aeruginosa. Microbiology 157, 1929–1944. doi: 10.1099/mic.0.046755-0
Boechat, A. L., Kaihami, G. H., Politi, M. J., Lépine, F., and Baldini, R. L. (2013). A novel role for an ECF sigma factor in fatty acid biosynthesis and membrane fluidity in Pseudomonas aeruginosa. PLoS ONE 8:e84775. doi: 10.1371/journal.pone.0084775
Bouffartigues, E., Gicquel, G., Bazire, A., Bains, M., Maillot, O., Vieillard, J., et al. (2012). Transcription of the oprF gene of Pseudomonas aeruginosa is dependent mainly on the sigX sigma factor and is sucrose induced. J. Bacteriol. 194, 4301–4311. doi: 10.1128/JB.00509-12
Bouffartigues, E., Moscoso, J. A., Duchesne, R., Rosay, T., Fito-Boncompte, L., Gicquel, G., et al. (2015). The absence of the Pseudomonas aeruginosa OprF protein leads to increased biofilm formation through variation in c-di-GMP level. Front. Microbiol. 6:630. doi: 10.3389/fmicb.2015.00630
Bozorgi, M., Memariani, Z., Mobli, M., Salehi Surmaghi, M. H., Shams-Ardekani, M. R., and Rahimi, R. (2013). Five Pistacia species (P. vera, P. atlantica, P. terebinthus, P. khinjuk, and P. lentiscus): a review of their traditional uses, phytochemistry, and pharmacology. ScientificWorldJournal 2013, 219815. doi: 10.1155/2013/219815
Brinkman, F. S. L., Schoofs, G., Hancock, R. E. W., and De Mot, R. (1999). Influence of a putative ECF sigma factor on expression of the major outer membrane protein, OprF, in Pseudomonas aeruginosa and Pseudomonas fluorescens. J. Bacteriol. 2013, 4746–4756.
Calvert, M. B., Jumde, V. R., and Titz, A. (2018). Pathoblockers or antivirulence drugs as a new option for the treatment of bacterial infections. Beilstein J. Org. Chem. 14, 2607–2617. doi: 10.3762/bjoc.14.239
Cezairliyan, B., Vinayavekhin, N., Grenfell-Lee, D., Yuen, G. J., Saghatelian, A., and Ausubel, F. M. (2013). Identification of Pseudomonas aeruginosa phenazines that kill Caenorhabditis elegans. PLoS Pathog. 9:e1003101. doi: 10.1371/journal.ppat.1003101
Chevalier, S., Bouffartigues, E., Bazire, A., Tahrioui, A., Duchesne, R., Tortuel, D., et al. (2019). Extracytoplasmic function sigma factors in Pseudomonas aeruginosa. Biochim. Biophys. Acta – Gene Regul. Mech. 1862, 709–721. doi: 10.1016/j.bbagrm.2018.04.008
Choi, J. G., Jeong, S. I., Ku, C. S., Sathishkumar, M., Lee, J. J., Mun, S. P., et al. (2009). Antibacterial activity of hydroxyalkenyl salicylic acids from sarcotesta of Ginkgo biloba against vancomycin-resistant Enterococcus. Fitoterapia 80, 18–20. doi: 10.1016/j.fitote.2008.09.001
Dickey, S. W., Cheung, G. Y. C., and Otto, M. (2017). Different drugs for bad bugs: antivirulence strategies in the age of antibiotic resistance. Nat. Rev. Drug Discov. 16, 457–471. doi: 10.1038/nrd.2017.23
Duchesne, R., Bouffartigues, E., Oxaran, V., Maillot, O., Bénard, M., Feuilloley, M. G. J., et al. (2013). A proteomic approach of SigX function in Pseudomonas aeruginosa outer membrane composition. J. Proteom. 94, 451–459. doi: 10.1016/j.jprot.2013.10.022
Engel, J., and Balachandran, P. (2009). Role of Pseudomonas aeruginosa type III effectors in disease. Curr. Opin. Microbiol. 12, 61–66. doi: 10.1016/j.mib.2008.12.007
Fléchard, M., Duchesne, R., Tahrioui, A., Bouffartigues, E., Depayras, S., Hardouin, J., et al. (2018). The absence of SigX results in impaired carbon metabolism and membrane fluidity in Pseudomonas aeruginosa. Sci. Rep. 8:17212. doi: 10.1038/s41598-018-35503-3
Fletcher, M. P., Diggle, S. P., Cámara, M., and Williams, P. (2007). Biosensor-based assays for PQS, HHQ and related 2-alkyl-4-quinolone quorum sensing signal molecules. Nat. Protoc. 2, 1254–1262. doi: 10.1038/nprot.2007.158
Gallagher, L. A., and Manoil, C. (2001). Pseudomonas aeruginosa PAO1 kills Caenorhabditis elegans by cyanide poisoning. J. Bacteriol. 183, 6207–6214. doi: 10.1128/JB.183.21.6207-6214.2001
Gicquel, G., Bouffartigues, E., Bains, M., Oxaran, V., Rosay, T., Lesouhaitier, O., et al. (2013). The extra-cytoplasmic function sigma factor sigX modulates biofilm and virulence-related properties in Pseudomonas aeruginosa. PLoS ONE 8:e80407. doi: 10.1371/journal.pone.0080407
Hall, S., McDermott, C., Anoopkumar-Dukie, S., McFarland, A. J., Forbes, A., Perkins, A. V., et al. (2016). Cellular effects of pyocyanin, a secreted virulence factor of Pseudomonas aeruginosa. Toxins (Basel) 8, 236. doi: 10.3390/toxins8080236
Hancock, R. E. W., and Carey, A. M. (1979). Outer membrane of Pseudomonas aeruginosa: heat- and 2-mercaptoethanol-modifiable proteins. J. Bacteriol. 140, 902–910.
He, J., Wang, S., Wu, T., Cao, Y., Xu, X., and Zhou, X. (2013). Effects of ginkgoneolic acid on the growth, acidogenicity, adherence, and biofilm of Streptococcus mutans in vitro. Folia Microbiol. (Praha) 58, 147–153. doi: 10.1007/s12223-012-0191-9
Hua, Z., Wu, C., Fan, G., Tang, Z., and Cao, F. (2017). The antibacterial activity and mechanism of ginkgolic acid C15:1. BMC Biotechnol. 17:5. doi: 10.1186/s12896-016-0324-3
Kondakova, T., D’Heygère, F., Feuilloley, M. J., Orange, N., Heipieper, H. J., and Duclairoir Poc, C. (2015). Glycerophospholipid synthesis and functions in Pseudomonas. Chem. Phys. Lipids 190, 27–42. doi: 10.1016/j.chemphyslip.2015.06.006
Kopec̈, W., Telenius, J., and Khandelia, H. (2013). Molecular dynamics simulations of the interactions of medicinal plant extracts and drugs with lipid bilayer membranes. FEBS J. 280, 2785–2805. doi: 10.1111/febs.12286
Lakowicz, J. R. (2006). Principles of Fluorescence Spectroscopy, ed. J. R. Lakowicz. Berlin: Springer, 353–382.
Lau, G. W., Hassett, D. J., Ran, H., and Kong, F. (2004). The role of pyocyanin in Pseudomonas aeruginosa infection. Trends Mol. Med. 10, 599–606. doi: 10.1016/j.molmed.2004.10.002
Lee, J. H., Kim, Y. G., Ryu, S. Y., Cho, M. H., and Lee, J. (2014). Ginkgolic acids and Ginkgo biloba extract inhibit Escherichia coli O157: H7 and Staphylococcus aureus biofilm formation. Int. J. Food Microbiol. 174, 47–55. doi: 10.1016/j.ijfoodmicro.2013.12.030
Liao, J., Schurr, M. J., and Sauer, K. (2013). The merR-like regulator brlR confers biofilm tolerance by activating multidrug efflux pumps in Pseudomonas aeruginosa biofilms. J. Bacteriol. 195, 3352–3363. doi: 10.1128/JB.00318-13
Mahadevan, S., and Park, Y. (2008). Multifaceted therapeutic benefits of Ginkgo biloba L.: chemistry, efficacy, safety, and uses. J. Food Sci. 73, R14–R19. doi: 10.1111/j.1750-3841.2007.00597.x
Mahady, G. (2005). Medicinal plants for the prevention and treatment of bacterial infections. Curr. Pharm. Des. 11, 2405–2427. doi: 10.2174/1381612054367481
Mahajan-Miklos, S., Tan, M. W., Rahme, L. G., and Ausubel, F. M. (1999). Molecular mechanisms of bacterial virulence elucidated using a Pseudomonas aeruginosa-Caenorhabditis elegans pathogenesis model. Cell 96, 47–56. doi: 10.1016/S0092-8674(00)80958-7
Malhotra, S., Hayes, D., and Wozniak, D. J. (2019). Cystic fibrosis and Pseudomonas aeruginosa: the host-microbe interface. Clin. Microbiol. Rev. 32, e138–e118. doi: 10.1128/CMR.00138-18
Martínez, O. F., Cardoso, M. H., Ribeiro, S. M., and Franco, O. L. (2019). Recent advances in anti-virulence therapeutic strategies with a focus on dismantling bacterial membrane microdomains, toxin neutralization, quorum-sensing interference and biofilm inhibition. Front. Cell. Infect. Microbiol. 9:74. doi: 10.3389/fcimb.2019.00074
Mavrodi, D. V., Bonsall, R. F., Delaney, S. M., Soule, M. J., Phillips, G., and Thomashow, L. S. (2001). Functional analysis of genes for biosynthesis of pyocyanin and phenazine-1-carboxamide from Pseudomonas aeruginosa PAO1. J. Bacteriol. 183, 6454–6465. doi: 10.1128/JB.183.21.6454-6465.2001
Moradali, M. F., Ghods, S., and Rehm, B. H. A. (2017). Pseudomonas aeruginosa lifestyle: a paradigm for adaptation, survival, and persistence. Front. Cell. Infect. Microbiol. 7:39. doi: 10.3389/fcimb.2017.00039
Nutman, J., Berger, M., Chase, P. A., Dearborn, D. G., Miller, K. M., Waller, R. L., et al. (1987). Studies on the mechanism of T cell inhibition by the Pseudomonas aeruginosa phenazine pigment pyocyanine. J. Immunol. 138, 3481–3487.
Otero-Asman, J. R., Wettstadt, S., Bernal, P., and Llamas, M. A. (2019). Diversity of extracytoplasmic function sigma (σECF) factor-dependent signaling in Pseudomonas. Mol. Microbiol. 112, 356–373. doi: 10.1111/mmi.14331
Pendleton, J. N., Gorman, S. P., and Gilmore, B. F. (2013). Clinical relevance of the ESKAPE pathogens. Expert Rev. Anti Infect. Ther. 11, 297–308. doi: 10.1586/eri.13.12
Pfaffl, M. W. (2001). A new mathematical model for relative quantification in real-time RT-PCR. Nucleic Acids Res. 29, 45. doi: 10.1093/nar/29.9.e45
Price-Whelan, A., Dietrich, L. E. P., and Newman, D. K. (2006). Rethinking “secondary” metabolism: physiological roles for phenazine antibiotics. Nat. Chem. Biol. 2, 71–78. doi: 10.1038/nchembio764
Prithiviraj, B., Bais, H. P., Weir, T., Suresh, B., Najarro, E. H., Dayakar, B. V., et al. (2005). Down regulation of virulence factors of Pseudomonas aeruginosa by salicylic acid attenuates its virulence on Arabidopsis thaliana and Caenorhabditis elegans. Infect. Immun. 73, 5319–5328. doi: 10.1128/IAI.73.9.5319-5328.2005
R Development Core Team (2017). R: A Language and Environment for Statistical Computing. Vienna: R Foundation for statistical computing.
Rasko, D. A., and Sperandio, V. (2010). Anti-virulence strategies to combat bacteria-mediated disease. Nat. Rev. Drug Discov. 9, 117–128. doi: 10.1038/nrd3013
Rauf, A., Patel, S., Uddin, G., Siddiqui, B. S., Ahmad, B., Muhammad, N., et al. (2017). Phytochemical, ethnomedicinal uses and pharmacological profile of genus Pistacia. Biomed. Pharmacother. 86, 393–404. doi: 10.1016/j.biopha.2016.12.017
Ray, A., Rentas, C., Caldwell, G. A., and Caldwell, K. A. (2015). Phenazine derivatives cause proteotoxicity and stress in C. elegans. Neurosci. Lett. 584, 23–27. doi: 10.1016/j.neulet.2014.09.055
Satyan, K. S., Jaiswal, A. K., Ghosal, S., and Bhattacharya, S. K. (1998). Anxiolytic activity of ginkgolic acid conjugates from Indian Ginkgo biloba. Psychopharmacology (Berl) 136, 148–152. doi: 10.1007/s002130050550
Schulz, S., Eckweiler, D., Bielecka, A., Nicolai, T., Franke, R., Dötsch, A., et al. (2015). Elucidation of sigma factor-associated networks in Pseudomonas aeruginosa reveals a modular architecture with limited and function-specific crosstalk. PLoS Pathog. 11:e1004744. doi: 10.1371/journal.ppat.1004744
Silva, L. N., Zimmer, K. R., Macedo, A. J., and Trentin, D. S. (2016). Plant natural products targeting bacterial virulence factors. Chem. Rev. 116, 9162–9236. doi: 10.1021/acs.chemrev.6b00184
Soukarieh, F., Williams, P., Stocks, M. J., and Cámara, M. (2018). Pseudomonas aeruginosa quorum sensing systems as drug discovery targets: current position and future perspectives. J. Med. Chem. 61, 10385–10402. doi: 10.1021/acs.jmedchem.8b00540
Tahrioui, A., Duchesne, R., Bouffartigues, E., Rodrigues, S., Maillot, O., Tortuel, D., et al. (2019). Extracellular DNA release, quorum sensing, and PrrF1/F2 small RNAs are key players in Pseudomonas aeruginosa tobramycin-enhanced biofilm formation. NPJ Biofilms Microbiomes 5:15. doi: 10.1038/s41522-019-0088-3
Tsuchiya, H. (2015). Membrane interactions of phytochemicals as their molecular mechanism applicable to the discovery of drug leads from plants. Molecules 20, 18923–18966. doi: 10.3390/molecules201018923
van Beek, T. A., and Montoro, P. (2009). Chemical analysis and quality control of Ginkgo biloba leaves, extracts, and phytopharmaceuticals. J. Chromatogr. A 1216, 2002–2032. doi: 10.1016/j.chroma.2009.01.013
Wagner, S., Sommer, R., Hinsberger, S., Lu, C., Hartmann, R. W., Empting, M., et al. (2016). Novel strategies for the treatment of Pseudomonas aeruginosa infections. J. Med. Chem. 59, 5929–5969. doi: 10.1021/acs.jmedchem.5b01698
Wang, F., He, Q., Yin, J., Xu, S., Hu, W., and Gu, L. (2018). BrlR from Pseudomonas aeruginosa is a receptor for both cyclic di-GMP and pyocyanin. Nat. Commun. 9:2563. doi: 10.1038/s41467-018-05004-y
WHO (2017). Global Priority List of Antibiotic-Resistant Bacteria to Guide Research, Discovery, and Development of New Antibiotics. Genova: World Health Organization.
Wu, Y., Park, K. C., Choi, B. G., Park, J. H., and Yoon, K. S. (2016). The antibiofilm effect of Ginkgo biloba extract against Salmonella and listeria isolates from poultry. Foodborne Pathog. Dis. 13, 229–238. doi: 10.1089/fpd.2015.2072
Yang, X., Zhu, W., Chen, J., Qian, Z., and Xie, J. (2004). Study on anti-bacterium activity of ginkgolic acids and their momomers. Zhong Yao Cai. 27, 661–663.
Keywords: Pistacia lentiscus, fruit-derived extract, ginkgolic acids, anti-virulence, Pseudomonas aeruginosa, membrane stiffness, ECFσ SigX
Citation: Tahrioui A, Ortiz S, Azuama OC, Bouffartigues E, Benalia N, Tortuel D, Maillot O, Chemat S, Kritsanida M, Feuilloley M, Orange N, Michel S, Lesouhaitier O, Cornelis P, Grougnet R, Boutefnouchet S and Chevalier S (2020) Membrane-Interactive Compounds From Pistacia lentiscus L. Thwart Pseudomonas aeruginosa Virulence. Front. Microbiol. 11:1068. doi: 10.3389/fmicb.2020.01068
Received: 18 March 2020; Accepted: 29 April 2020;
Published: 26 May 2020.
Edited by:
Catherine Ann Wakeman, Texas Tech University, United StatesReviewed by:
Rodolfo García-Contreras, National Autonomous University of Mexico, MexicoAngela Wilks, University of Maryland, Baltimore, United States
Copyright © 2020 Tahrioui, Ortiz, Azuama, Bouffartigues, Benalia, Tortuel, Maillot, Chemat, Kritsanida, Feuilloley, Orange, Michel, Lesouhaitier, Cornelis, Grougnet, Boutefnouchet and Chevalier. This is an open-access article distributed under the terms of the Creative Commons Attribution License (CC BY). The use, distribution or reproduction in other forums is permitted, provided the original author(s) and the copyright owner(s) are credited and that the original publication in this journal is cited, in accordance with accepted academic practice. No use, distribution or reproduction is permitted which does not comply with these terms.
*Correspondence: Ali Tahrioui, YWxpLnRhaHJpb3VpQHVuaXYtcm91ZW4uZnI=