- 1Department of Environmental Sciences, Aquatic and Stable Isotope Biogeochemistry, University of Basel, Basel, Switzerland
- 2Department of Marine Science, University of Gothenburg, Gothenburg, Sweden
- 3Department of Biology and Nordic Center for Earth Evolution, University of Southern Denmark, Odense, Denmark
Understanding the biogeochemical controls on the partitioning between nitrogen (N) removal through denitrification and anaerobic ammonium oxidation (anammox), and N recycling via dissimilatory nitrate (NO3–) reduction to ammonium (DNRA) is crucial for constraining lacustrine N budgets. Besides organic carbon, inorganic compounds may serve as electron donors for NO3– reduction, yet the significance of lithotrophic NO3– reduction in the environment is still poorly understood. Conducting incubation experiments with additions of 15N-labeled compounds and reduced inorganic substrates (H2S, Fe2+, Mn2+), we assessed the role of alternative electron donors in regulating the partitioning between the different NO3–-reducing processes in ferruginous surface sediments of Lake Lugano, Switzerland. In sediment slurry incubations without added inorganic substrates, denitrification and DNRA were the dominant NO3–-reducing pathways, with DNRA contributing between 31 and 46% to the total NO3– reduction. The contribution of anammox was less than 1%. Denitrification rates were stimulated by low to moderate additions of ferrous iron (Fe2+ ≤ 258 μM) but almost completely suppressed at higher levels (≥1300 μM). Conversely, DNRA was stimulated only at higher Fe2+ concentrations. Dissolved sulfide (H2S, i.e., sum of H2S, HS– and S2−) concentrations up to ∼80 μM, strongly stimulated denitrification, but did not affect DNRA significantly. At higher H2S levels (≥125 μM), both processes were inhibited. We were unable to find clear evidence for Mn2+-supported lithotrophic NO3– reduction. However, at high concentrations (∼500 μM), Mn2+ additions inhibited NO3– reduction, while it did not affect the balance between the two NO3– reduction pathways. Our results provide experimental evidence for chemolithotrophic denitrification or DNRA with Fe2+ and H2S in the Lake Lugano sediments, and demonstrate that all tested potential electron donors, despite the beneficial effect at low concentrations of some of them, can inhibit NO3– reduction at high concentration levels. Our findings thus imply that the concentration of inorganic electron donors in lake sediments can act as an important regulator of both benthic denitrification and DNRA rates, and suggest that they can exert an important control on the relative partitioning between microbial N removal and N retention in lakes.
Introduction
The water quality of lakes in Switzerland has greatly improved over the last few decades due to the ban of phosphates in laundry detergents, improved wastewater management and modern treatment technologies (Jakob et al., 2002; Zobrist and Reichert, 2006; Zobrist et al., 2018). Phosphate concentrations have largely returned to pre-eutrophication levels, yet reactive nitrogen levels in Swiss lakes are still relatively high, likely due to continued inputs from agriculture (Zobrist and Reichert, 2006). Lake sediments are hot spots of N transformations and play an important role in the remediation of excess reactive N inputs through nitrate (NO3–)-reducing processes (e.g., Wenk et al., 2014). Efficient NO3– elimination in lakes is mainly due to denitrification, the microbially mediated dissimilatory reduction of nitrate to gaseous N2 using organic or inorganic substrates (organotrophic vs. chemolithotrophic denitrification). The anaerobic oxidation of ammonium (anammox) with NOx– to N2 can also play a role in N removal in lake sediments (Schubert et al., 2006; Wenk et al., 2013; Crowe et al., 2017). Conversely, dissimilatory reduction of nitrate to ammonium (DNRA), results in the retention of reactive N in the environment. The coupling and the partitioning of the different NO3–-transforming metabolisms will thus determine the ultimate fate of reactive N.
Denitrification and DNRA compete for the same substrates and electron acceptors (NO2–, NO3–). In field and laboratory incubations, the ratio of organic carbon (OC) to NO3– availability has been shown to control the relative importance of the different N-transforming processes (Kraft et al., 2014; Hardison et al., 2015; Palacin-Lizarbe et al., 2019). For instance, when the OC/NO3– ratio is low, NO3– is commonly reduced via denitrification. On the other hand, when the OC/NO3– ratio is high, DNRA is often the dominant N-reduction pathway (Nizzoli et al., 2010; Yoon et al., 2015; van den Berg et al., 2015, 2016; Chutivisut et al., 2018).
Traditionally, NO3– reduction via denitrification and DNRA has been considered as purely organotrophic processes (Tiedje, 1988). Geochemical evidence (Froelich et al., 1979) and the discovery of nitrate-reducing microorganisms using Fe2+ as substrate (e.g., Hafenbradl et al., 1996; Straub et al., 1996) stimulated research on the microbiology and biogeochemistry of this novel mode of N-transformation. Culturing studies, as well as sediment incubation experiments provided putative evidence that this process is performed by diverse microbes in a variety of aquatic environments (Table 1). However, bacterial culture experiments with high substrate concentrations (e.g., 2.5–4 mM NO3–, 10 mM Fe2+; Weber et al., 2006b) are not representative for natural conditions. On the other hand, experimental studies with environmentally relevant substrate concentrations did not always investigate the end products of NO3– reduction (i.e., N2 vs. NH4+ production; Chakraborty and Picardal, 2013; Laufer et al., 2016). Recent work has shown an increasing contribution of DNRA relative to denitrification with increasing environmental Fe2+ concentration (Roberts et al., 2014; Robertson et al., 2016; Robertson and Thamdrup, 2017). Yet, the environmental relevance of Fe2+-dependent nitrate transformations is still poorly understood, as is the effect of Fe2+ on the partitioning between N-removal by denitrification and N-retention in the case of DNRA. Similarly, knowledge on the potential role of Mn2+, which has also been suggested as potential electron donor for chemolitotrophic denitrification (Aller, 1990; Luther et al., 1997), is completely lacking in lacustrine sediments.
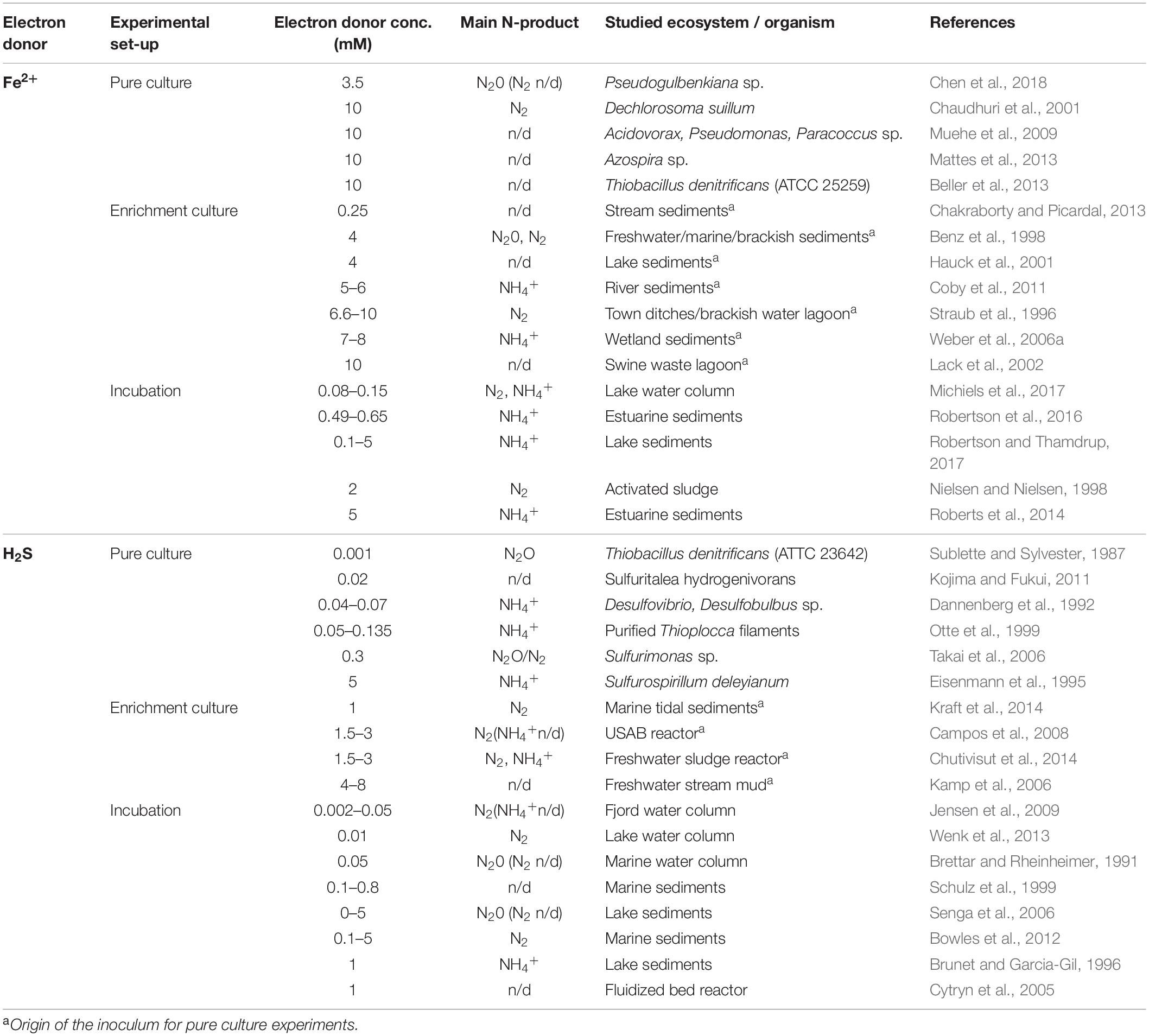
Table 1. Overview of prior experimental studies that have investigated Fe(II) and H2S oxidation coupled to nitrate reduction.
In contrast, the importance of H2S as a direct substrate for both denitrification and DNRA is well established (Table 1). In organic-rich lake sediments, sulfate is efficiently reduced to sulfide (Holmer and Storkholm, 2001), which reacts with iron species to sulfur intermediates or FeS (Zopfi et al., 2004). Some nitrate reducers can use both dissolved and particulate forms of reduced sulfur for their metabolism (e.g., Dannenberg et al., 1992; Kamp et al., 2006; Yan et al., 2018). In natural environments, NO3– reduction coupled to sulfide oxidation has been studied in water columns (e.g., Jensen et al., 2009; Wenk et al., 2013; Table 1) and sediments (Brunet and Garcia-Gil, 1996; Senga et al., 2006). However, studies on the end products of sulfide-dependent NO3– reduction pathways (i.e., N2 vs. NH4+) under environmentally relevant substrate concentrations are still scarce.
In the present study we aimed to assess the role of different potential inorganic electron donors (Fe2+, H2S, Mn2+) in regulating the overall rates and the partitioning between N-removing (denitrification, anammox) and N-recycling (DNRA) processes in the ferruginous sediments of the eutrophic southern basin of Lake Lugano (Switzerland). Previous studies in this seasonally anoxic basin demonstrated that an important portion of the external NO3– load is removed through sedimentary denitrification (Wenk et al., 2014). By conducting incubation experiments with 15N-labeled substrates and additions of different inorganic electron donors, we determined whether, and to what extent, lithotrophic nitrate reduction is significant under environmentally relevant substrate concentrations.
Materials and Methods
Sampling Site
The south alpine Lake Lugano is located on the Swiss/Italian border and is divided by a shallow sill into a permanently stratified northern basin and a eutrophic, monomictic southern basin (Barbieri and Polli, 1992). Using a gravity corer, we collected sediments at two locations in the southern basin: Figino (8°53′37′′E, 45°57′31′′N, 94 m depth) and Melide (8°57′29′′E, 45°56′22′′N, 85 m depth; Supplementary Figure 1). Sampling campaigns took place in 2015 (December), 2016 (March and September) and 2017 (March and June). Depth profiles of water column temperature, conductivity, and oxygen concentrations were obtained by a winch-operated CTD (Idronaut Ocean Seven 316Plus). Bottom water concentration data for NO3–, NH4+, total dissolved Fe, Mn, and S were determined within the frame of a long-term monitoring campaign promoted by the International Commission for the Protection of Italian-Swiss Waters (CIPAIS; Commissione Internazionale per la Protezione delle Acque Italiano-Svizzere) on behalf of the Administration of the Canton of Ticino, and were provided by F. Lepori (SUPSI; University of Applied Sciences and Arts of Southern Switzerland).
Sediment Depth Profiles
Upon return to the laboratory, duplicate sediment cores from each site were sectioned into 1-cm slices in the uppermost 6 cm of the core, and 2-cm slices from 6 to 20 cm depth. Samples were taken for porosity, TOC, particulate FeII/FeIII, total Mn, and dissolved pore water constituents (Fe2+, Mn2+, H2S, SO42–, NH4+, NO3–, and NO2–). For porewater collection, sediment sections were rapidly transferred to 50 mL Falcon tubes and centrifuged at 4700 rpm for 10 min. The supernatant was immediately filtered (0.2 μm), and samples (1 mL) for Fe2+ and Mn2+ were fixed with hydrochloric acid (40 μL 1 M HCl). Samples for H2S determination were stabilized with 20 μL aqueous zinc acetate solution (20% w/v) and stored at 6°C until analysis. The remaining filtered porewater was frozen until analysis of all other compounds. Oxygen microprofiles were measured in the laboratory (21°C) using an amperometric microsensor with a tip diameter of 100 μm (Unisense).
Sediment Slurry Incubations
Seasonal variation of the potential benthic N-transformation processes was investigated through sediment slurry incubation experiments performed at different times of the year (December 2015, March and September 2016). For the preparation of the slurries, fresh surface sediment (upper 2 cm) was homogenized, and aliquots of 1 g sediment were transferred into 120 mL serum bottles and complemented with 80 mL of anoxic (He purged for 45 min) artificial lake water (Smith et al., 2002; Supplementary Table 1). The artificial lake water was free of NO3–, NO2–, and NH4+, reducing the production of N2 from NOx present in natural bottom waters. Serum bottles were sealed with blue chlorobutyl-rubber stoppers and crimped before purging with He for 10 min to remove O2 and to lower the N2 background for further isotopic measurements. Slurries were pre-incubated overnight on a shaker table (80 rpm) at 8°C in the dark to remove any traces of O2, which may still have been present after the initial purging. Labeled 15N (e.g., Na15NO3–, 15NH4Cl; 15N 99%; Cambridge Isotopes Laboratories; final conc. ∼120 and 71 μM, respectively; Supplementary Table 2) and 14N-substrates (e.g., 14NO2–; final conc. ∼34 μM) were added to identify and quantify potential rates of denitrification, DNRA, and anammox (Nielsen, 1992; Thamdrup and Dalsgaard, 2002). During substrate addition and sampling, the slurries were transferred to an anaerobic chamber with N2 atmosphere, and were then returned to the shaker table held at 8°C. Gas samples for 15N-N2 isotope analysis were taken from the headspace at each time point (4 in total) using a 5 mL gas-tight glass syringe (Hamilton). Two milliliters of the gas were transferred to 3 mL Exetainers (Labco) pre-filled with anoxic Milli-Q water. In exchange for the extracted gas sample 2 mL of anoxic Milli-Q water were added to the incubation vials to maintain constant pressure inside. Exetainers with the gas samples were stored upside down at room temperature until isotopic measurement of N2. At Tinitial and Tend, 6 mL liquid samples were collected, and immediately filtered for nutrient determination and quantification of DNRA rates. The incubation time for each treatment was determined based on preliminary tests and lasted 3–4 days. Liquid samples were kept frozen or acidified with sulfamic acid (40 mM final concentration; Klueglein and Kappler, 2013) until further analysis of dissolved nutrients and metals, respectively. Preliminary tests, during which samples were taken at four time points, consistently showed linear 15NH4+ production over time. We also tested for the adsorption of NH4+ to sediment material following an adapted procedure of Behrendt et al. (2013). Briefly, sediment slurries were prepared as described above and known quantities of NH4Cl were added, corresponding to final concentrations 5, 10, 25, and 50 μM. The percentage of adsorption was calculated from the difference between the target concentration and the actually determined concentration in the supernatant minus the NH4+ concentration in control slurries without NH4+ addition. All preparations were done in triplicates.
Incubation Experiments With Different Electron Donors
To assess the influence of various inorganic electron donors on the mode and regulation of benthic N-transformations, we performed incubation experiments with microbial biomass from fresh sediment. Sediments used were collected in March and June 2017 for Fe2+, H2S, and Mn2+ addition experiments. Through stirring and centrifugation, microbial biomass was separated from the sediment matrix in order to minimize the effect of the sedimentary organic matter, solid-phase iron sulfides and metal-oxyhydroxides. Briefly, the first two centimeters of duplicate sediment cores were sectioned and transferred into a 2-L Erlenmeyer flask with anoxic (He purged) artificial lake water (Supplementary Table 1), which was immediately closed using a thick gray rubber stopper. After intense shaking and stirring, the slurry was transferred into 50 mL Falcon tubes and centrifuged at 300 rpm for 3 min to separate solid-phase particles from detached microbial biomass. The supernatants were pooled, and aliquots of 70 mL were transferred into 120 mL serum bottles (in triplicate) that were then sealed and crimped. The liquid phases were purged with He (10 min) and pre-incubated on a shaker table (80 rpm) in the dark at 8°C. Different volumes of anoxically prepared solutions of FeCl2⋅4H2O (100 mM), Na2S⋅9H2O (50 mM), and MnCl2⋅4H2O (50 mM) were added (Supplementary Table 2). Then the pH was adjusted with anoxic HCl or NaOH (1 M) to the pH of control incubations without added substrate, and kept constant (±0.2 units) for the duration of the experiment. Substrate additions and subsampling of gas or liquid phase was done in an anaerobic chamber, as described above. Samples (1 mL) for Fe2+ and Mn2+ determination were fixed with 40 mM sulfamic acid (Klueglein and Kappler, 2013). One mL aliquots for H2S determination were preserved with 20 μL zinc acetate (20% w/v). The experiment was started with the addition of Na15NO3– (∼116 ± 11 μM) and lasted 5–7 days (Supplementary Table 2). Incubations took place in the dark, under gentle agitation at 8°C. Given the stark deviation from in situ conditions (i.e., the separation of microbial biomass from sediment and modification of the natural solute concentrations), these incubations were not intended to assess absolute benthic N transformation rates that are representative for the natural conditions. Instead, these incubations allowed us to investigate any differential stimulation/inhibition by the tested electron donors within a well-controlled experimental set-up. As a consequence, results below are presented as percent (%) “stimulation” or “inhibition” of a given NO3–-reducing processes relative to the corresponding controls without additions. Student’s t-tests (P < 0.05, Excel) were applied to determine significant differences between measured rates.
15N-Based Rate Measurements
Denitrification and anammox rates were calculated using 15N isotope-pairing technique (Nielsen, 1992), through monitoring the production of 14N15N or 15N15N (Nielsen, 1992; Thamdrup and Dalsgaard, 2002) using a Delta V Advantage isotope-ratio mass spectrometer (IRMS; Thermo Fisher Scientific) coupled to a gas chromatograph for gas purification. Rates of denitrification and anammox were calculated from the total accumulation of single (14N15N) and double-labeled 15N-N2 (15N15N) over time as determined by linear regression analysis of excess 14N15N/14N14N and 15N15N/14N14N trends (Supplementary Figure 2). In incubations with 15NH4+ and 14NO2–,
where FNH4+ is the fraction of 15N in NH4+ (Thamdrup and Dalsgaard, 2002). As anammox was insignificant, the following equation was used to quantify denitrification in incubations with 15NO3–,
DNRA rates were quantified by oxidation of NH4+ to N2 using alkaline hypobromite (Risgaard-Petersen et al., 1995), as in Robertson et al. (2016). The produced N2 was then analyzed by GC-IRMS as described above. In incubations with 15NO3–, DNRA rates were determined by linear regression of the concentration of 15NH4+ versus time,
15NH4+ standards were prepared in parallel with the samples in order to test the efficiency of the hypobromite oxidation step (recovery typically > 95%).
Chemical Analyses
Nitrite concentrations were determined colorimetrically according to Hansen and Koroleff (1999). Concentrations of NOx– (i.e., NO3– + NO2–) were measured by chemiluminescence detection using a NOx-analyzer (Antek Model 745; Braman and Hendrix, 1989). Nitrate concentrations were then calculated from the difference between NOx- and NO2–. Ammonium in porewater and incubation experiments was measured generally by suppression-ion chromatography with conductivity detection (940 Professional IC Vario, Metrohm, Switzerland). In treatments with Mn2+ additions, NH4+ was measured spectrophotometrically, due to interferences during the ion-chromatographic separation. Photometric quantification of NH4+ was done using the indophenol method (Krom, 1980).
Dissolved Fe2+ in porewater and incubation samples was measured with ferrozine (Stookey, 1970). Solid-phase reactive FeII and FeIII was quantified by extraction in 0.5 M HCl for 1 h according to Jensen and Thamdrup (1993) and subsequent analysis with ferrozine. Manganese concentrations in the acidic sediment extracts (including MnII and MnIV) and in Mn2+-amended incubations were determined using inductively coupled plasma optical emission spectrometry (ICP-OES; Agilent Technologies 5100).
Dissolved sulfide (H2S, i.e., sum of H2S, HS–, and S2−) in the porewater was quantified by the colorimetric methylene blue method (Cline, 1969). Concentrations in slurries were affected by the presence of Fe2+ and, hence, the partial removal of the added H2S from solution through precipitation as FeS. Therefore, both the added quantity and the measured concentration in the incubations are reported. Porewater chloride and sulfate (SO42–) concentrations were quantified by suppressed anion chromatography with conductivity detection (940 Professional IC Vario, Metrohm).
Results
Geochemical Setting of Sampling Sites
Fine organic-rich (∼8% OC dry mass) sediments were found at both sampling stations in the southern basin of Lake Lugano. High microbial activity within the sediments was reflected by the shallow O2 penetration depths of 3.7 ± 0.6 and 2 ± 0.5 mm at Figino and Melide, respectively (n = 4, data not shown), as determined by microsensor measurements in the laboratory with oxygenated (∼220–235 μM O2) lake water covering the sediment surface. Active bioturbation by higher organisms was not evident, consistent with seasonal bottom-water anoxia for more than 7 months (e.g., Blees et al., 2014). Typically, deep-hypolimnion oxygenation occurs once a year during winter mixing. In 2016, however, the bottom water at Figino remained anoxic throughout the year (Figure 1), whereas at Melide at least low oxygen concentrations were measured throughout most of the annual cycle. The bottom-water concentrations of the different nitrogen compounds varied seasonally as a function of water column stratification conditions. Ammonium concentrations 2 m above the sediments increased during the thermal stratification period at both stations, reaching 68 and 128 μM at Figino and Melide, respectively. With the onset of water-column mixing in February, the downwelling of oxygenated water led to the almost complete oxidation of ammonium and the concomitant production of NO2– and NO3–. The sum of produced NO2– and NO3– did not match the loss of NH4+, suggesting that most NOx– was further metabolized by respiratory processes in the water column and/or the surface sediments. The bottom water concentrations of NO3– varied between 25–65 μM and 1–67 μM at Figino and Melide, respectively, depending on the season (Figure 1). Nitrite concentrations ranged between 0.05 and 14 μM at both stations.
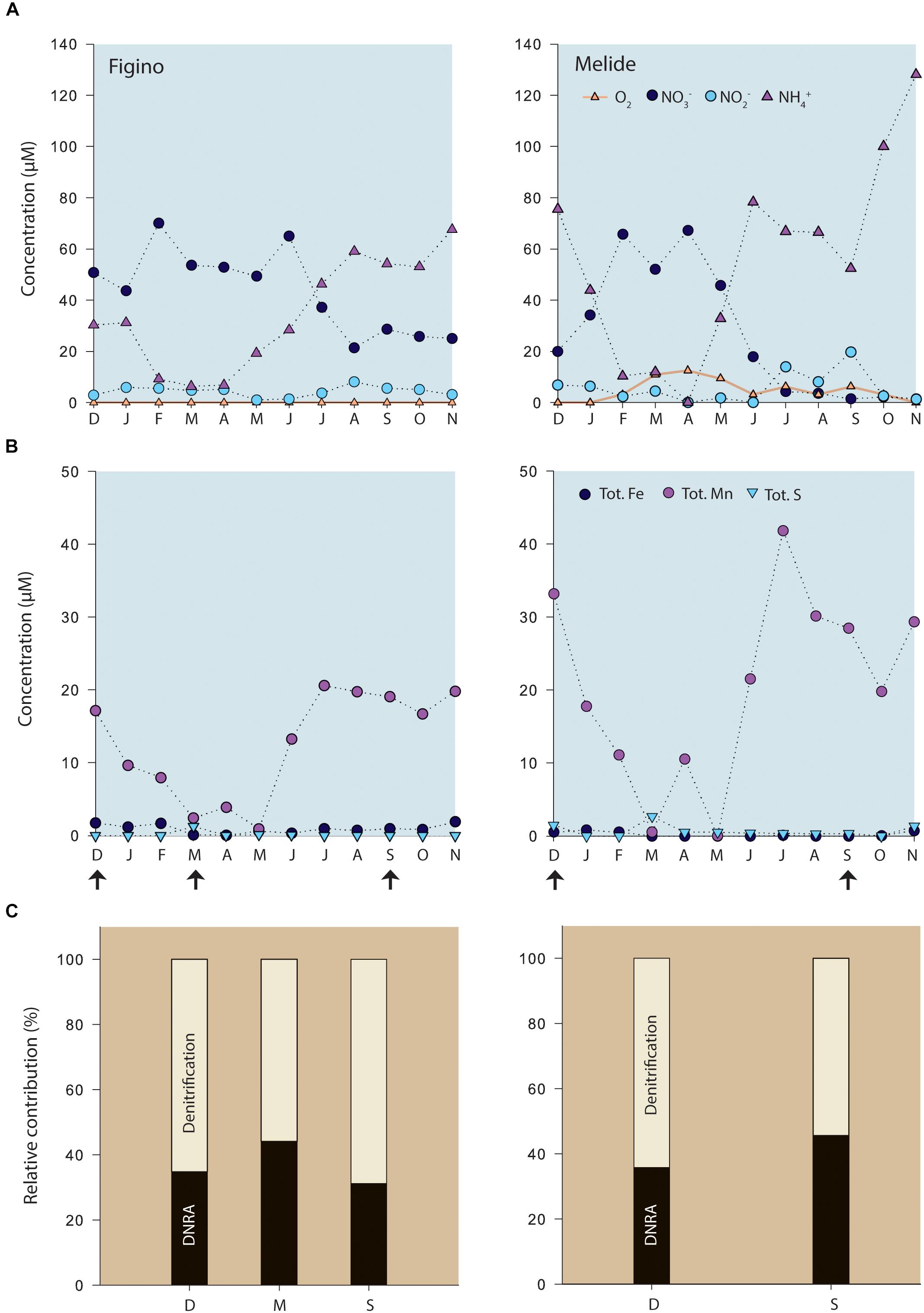
Figure 1. (A) Seasonal variation of bottom-water (2 m above sediment surface) concentrations of dissolved oxygen and nitrogen compounds, and (B) total dissolved Fe, Mn, and S at the two study locations in the south basin of Lake Lugano (Supplementary Figure 1). No oxygen was detected at Figino during that year. (C) Relative contribution of DNRA and denitrification (%) to the total benthic NO3– reduction, as determined by sediment slurry incubation experiments at selected time points (arrows). Capital letters at x-axes correspond to the first letter of each month from December 2015 to November 2016.
Porewater concentration data of dissolved N compounds (NH4+, NO2–, and NO3–), as well as dissolved and particulate phases of Mn and Fe are shown in Figure 2. At both stations, NH4+ concentrations near the sediment-water interface were ∼600 μM and increased with depth indicating the active mineralization of organic matter. In the surface sediments, NO3– and NO2– concentrations were below detection limit (Figure 2), despite the presence of NOx in the bottom waters at the time of sampling (Figure 1), indicating that the sediments represent an efficient sink for these compounds. The porewaters at both stations were further characterized by high concentrations of Mn2+ and Fe2+. Dissolved Mn2+ concentrations were almost identical at both stations (∼350–400 μM, Figure 2) and did not show any strong variation with depth. Concentrations of Fe2+ were much higher at Figino, where they increased from ∼150 μM at the sediment-water interface to ∼900 μM at 20 cm depth (Figure 2). Dissolved Fe2+ and Mn2+ concentrations in the surface sediment layers (0–2 cm) varied throughout the year at both stations, though no clear temporal trends were observed (Cojean, 2019). Particulate FeII, FeIII, and total Mn concentrations were relatively constant with depth, and similar between sites (Figure 2). Free H2S was not detected in the porewater at either of the two stations (detection limit 1 μM).
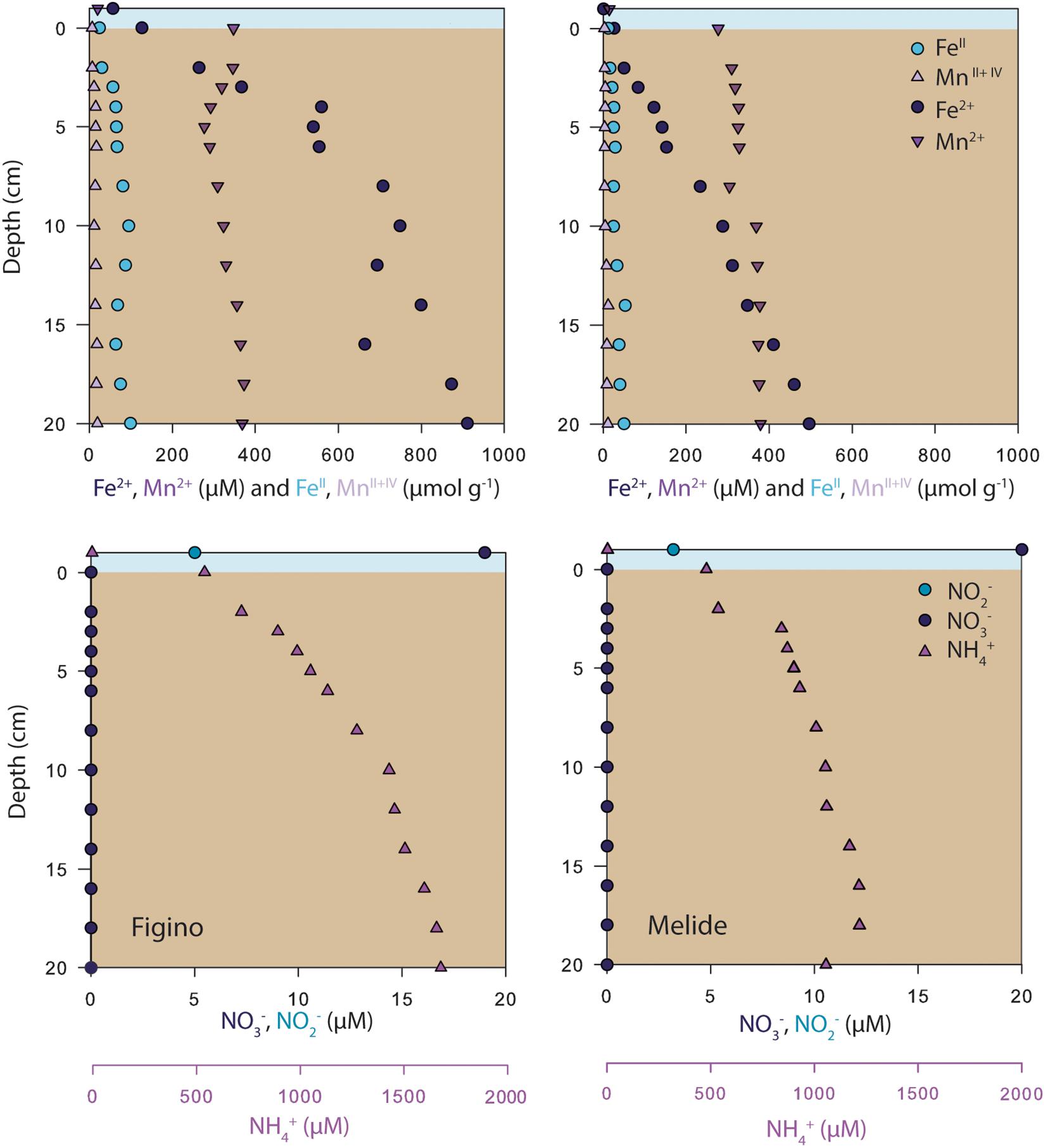
Figure 2. Vertical profiles of porewater solutes (Fe2+, Mn2+, NO3–, NO2–, NH4+) and particulate phases (FeII and total Mn) in sediments sampled in June 2017 at Figino (left) and Melide (right). The bottom water samples were collected 2 m above the surface sediments.
Benthic N-Transformations
We determined potential rates of benthic denitrification, DNRA, and anammox in anoxic slurry incubation experiments with fresh surface-sediment material, collected at different times of the year (Figure 1). At each site, similar results were obtained for the different sampling months. In 15NO3– amended slurries, production rates of 15N-N2 via denitrification were consistently higher than 15NH4+ production through dissimilatory nitrate reduction to ammonium (DNRA). Adsorption of generated 15NH4+ to sediment particles could lead to underestimated DNRA rates. Initial adsorption tests showed, however, that NH4+ did not bind significantly to the mineral phases in our dilute slurries. Hence, the measured DNRA rates can be considered as not being affected significantly by adsorption artifacts. The contribution of DNRA relative to total nitrate reduction varied seasonally between 31 and 46% (Figure 1). The ratio of denitrification to DNRA (DEN:DNRA) varied accordingly between 1.2 and 2.2. Despite this relatively consistent partitioning between denitrification and DNRA at each station, the absolute potential rates differed markedly across sites. For all sampling dates, except for December 2015, denitrification was higher at Figino than Melide, with measured maximum rates of 85 and 53 nmol N g–1 wet sediment d–1, respectively. In contrast, DNRA rates were slightly higher at Melide than at Figino, with maximum rates of 44 and 38 nmol N g–1 wet sediment d–1, respectively. In slurries amended with 15NH4+ and natural abundance NO2–, N2 production rates were close to 0.01 nmol N g–1 wet sediment day–1 at both sites, indicating that the contribution of anammox to the total N-removal was <1% (data not shown). Anammox was therefore not further investigated in this study.
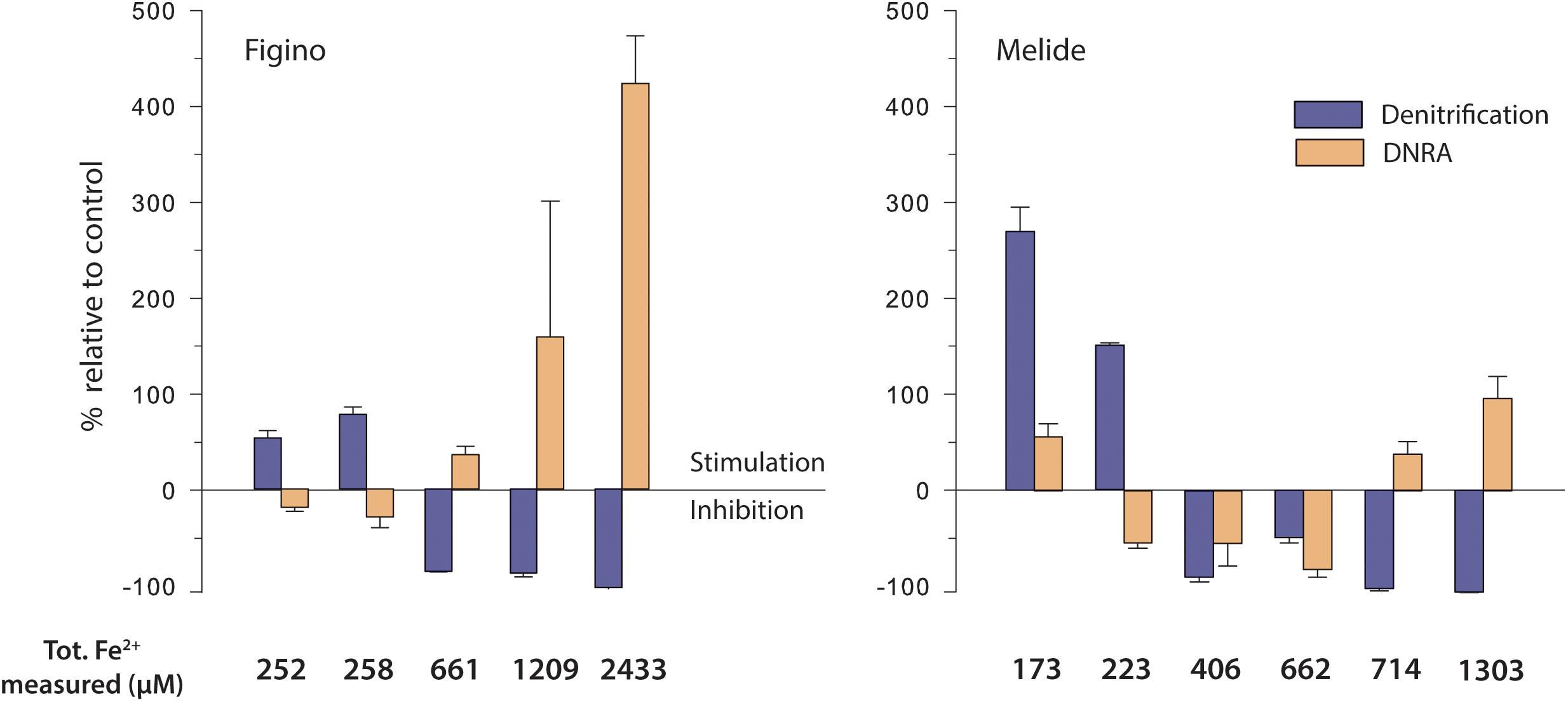
Figure 3. Effect of dissolved ferrous iron on denitrification and DNRA rates in anoxic sediment-biomass incubation experiments amended with 15NO3– and different Fe2+ concentrations. Stimulation (positive values)/inhibition (negative values) is expressed relative to the respective controls without Fe2+ additions for each set of experiments (see Supplementary Table 2). Error bars show standard errors (n = 3).
Incubations With Microbial Biomass Only
Incubations with microbial biomass that had been separated from most of the organic and inorganic sedimentary solids were established as a baseline for the treatments amended with inorganic electron donors (Fe2+, H2S, and Mn2+). These experiments were designed to investigate any differential stimulation/inhibition by the tested electron donors under controlled conditions. The removal from sedimentary solids had a major influence on the potential rates and the relative contribution of DNRA to NO3– reduction, which decreased from about 31–46% in the regular slurry incubations to <12%. Given the significant deviation from in situ conditions, this is not too surprising. In fact, our observation provides putative evidence for nitrate-reduction/partitioning controls other than those that were specifically tested here. More specifically, during the separation step, total particulate organic carbon (POC) was reduced from ∼ 300 to 55 mg L–1 (Figino) and ∼ 180 to 25 mg L–1 (Melide), while dissolved organic carbon (DOC) concentrations increased from about 510–900 mg L–1 and 640–1400 mg L–1 for Figino and Melide, respectively. Thus, our findings hint at the importance of organic matter as regulatory factor determining the ratio between denitrification and DNRA (as shown earlier, Nizzoli et al., 2010; Chutivisut et al., 2018), or alternatively suggest that DNRA bacteria are to a larger extent particle-associated. The goal of the present study, however, was to explore the role of inorganic electron donors as potential substrates for anaerobic NO3–-reduction processes, and their impact on the repartition between denitrification and nitrate ammonification.
Incubations With Fe2+ Additions
In order to investigate the effect of Fe2+ on NO3– reduction at environmentally relevant concentrations, we conducted incubation experiments with additions of 15NO3– and various concentrations of dissolved Fe2+ (Table 2). While we aimed to lower the ambient Fe2+ concentration by separating the microbial biomass from most of the Fe-containing sediment solids and dilution of sediment porewater with Fe-free artificial lake water, total Fe2+ background concentrations in the controls were still about 201 and 113 μM at Figino and Melide, respectively, suggesting desorption of Fe2+ or dissolution of Fe(II) phases during the separation of microbial biomass. These background Fe2+ levels represent 20% of the average ambient Fe2+ porewater concentration.
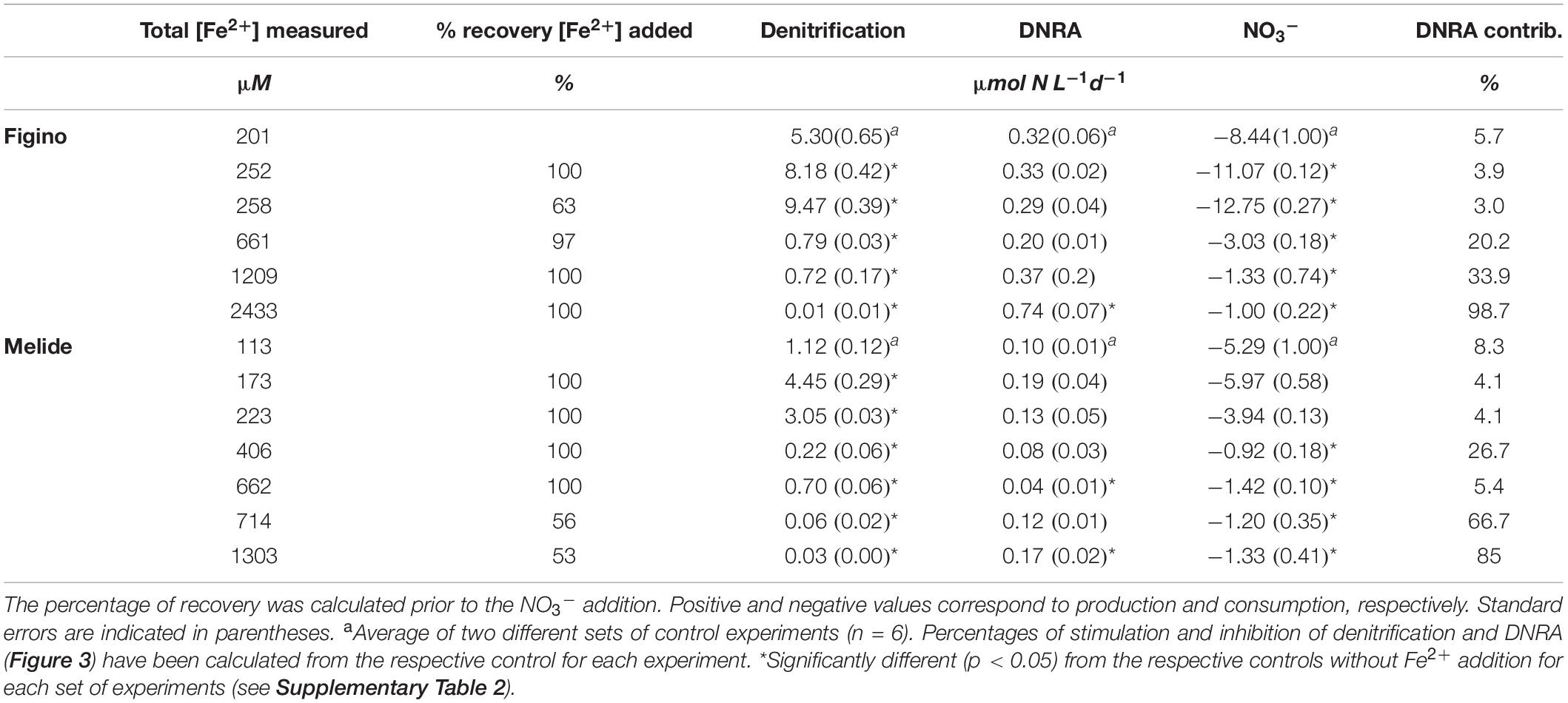
Table 2. Summary of denitrification, DNRA, NO3– consumption rates (all μmol N L–1 d–1), and the contribution of DNRA to total nitrate reduction (%) in 15NO3– addition incubation experiments with different Fe2+ amendments.
In all incubation experiments, we observed systematic trends across treatments and between the two sites. At both stations, the lower Fe2+ additions (≤258 μM) significantly enhanced N2 production, with maximum stimulation relative to the controls of 75 and 250%, at Figino and Melide, respectively (Figure 3). With increasing Fe2+ concentrations (≥406 μM), however, N2 production decreased to ∼0.01 μmol N L–1 d–1, indicating almost complete inhibition of denitrification at the highest Fe2+ concentration (Figure 3 and Table 2). In contrast, DNRA was partly inhibited by Fe2+ at the low and intermediate concentrations, while the highest Fe2+ levels (≥2433 and 1303 μM at Figino and Melide, respectively) enhanced DNRA by 408% (Figino) and 88% (Melide) relative to the corresponding controls (Figure 3). Consequently, the relative contribution of DNRA to the total NO3– reduction compared to denitrification (i.e., the DEN:DNRA ratio) varied according to the final Fe2+ concentration in the incubations. In the controls, DNRA contributed not more than ∼8% to the total nitrate reduction rate. At low Fe2+ concentrations, when denitrification was Fe-stimulated the most, the importance of DNRA relative to denitrification decreased (Table 2). In contrast, at Fe2+ concentrations higher than 661 μM (Figino) and 406 μM (Melide), the DNRA contribution was ≥20% at both sites (with one exception at 662 μM Fe2+ at Melide; Table 2), clearly indicating the differential stimulation/inhibition of DNRA versus denitrification by Fe2+.
Consistent with the 15N-tracer results, nitrate consumption increased relative to controls when low concentrations of Fe2+ were added, particularly at Figino (Table 2). With increasing Fe2+ concentrations (i.e., 661 μM and higher), NO3– reduction was significantly reduced (Table 2). The nitrate concentration measurements revealed that the net amount of 15NO3– removed did not match up with the determined products (NO2–, 15N-N2 and/or 15NH4+). In most experiments, nitrate consumption was ∼1.3 times higher than the sum of measured 15N-labeled products, which may be due to the assimilation or storage uptake by microorganisms and algal cells. The total recovery of dissolved Fe2+ added at the beginning of the incubation (after 2–5 days of pre-incubation for pH stabilization) was complete in most treatments (70–100%). But in all experiments, added Fe2+ was mostly found in the particulate phase, with only 2–6% remaining in solution. This suggests that Fe2+ may have sorbed to surfaces, like remaining sedimentary solids.
Incubations With H2S Additions
Similar to the treatments with Fe2+, H2S was added at different concentrations to the incubations amended with 15NO3–. In all treatments, denitrification was a more important NO3–-reducing pathway than DNRA. In control experiments without H2S addition, 15N-N2 production was 8 and 12 times higher than of 15NH4+ at Figino and Melide, respectively (Table 3). Upon addition of H2S, the largest fraction of sulfide was removed from solution by reacting with Fe or Mn. Both, the targeted H2S (“added”) concentration as well as the actual concentrations of dissolved H2S (“measured”) are therefore presented in Table 3. Values reported below represent the targeted concentrations of H2S, unless stated otherwise.
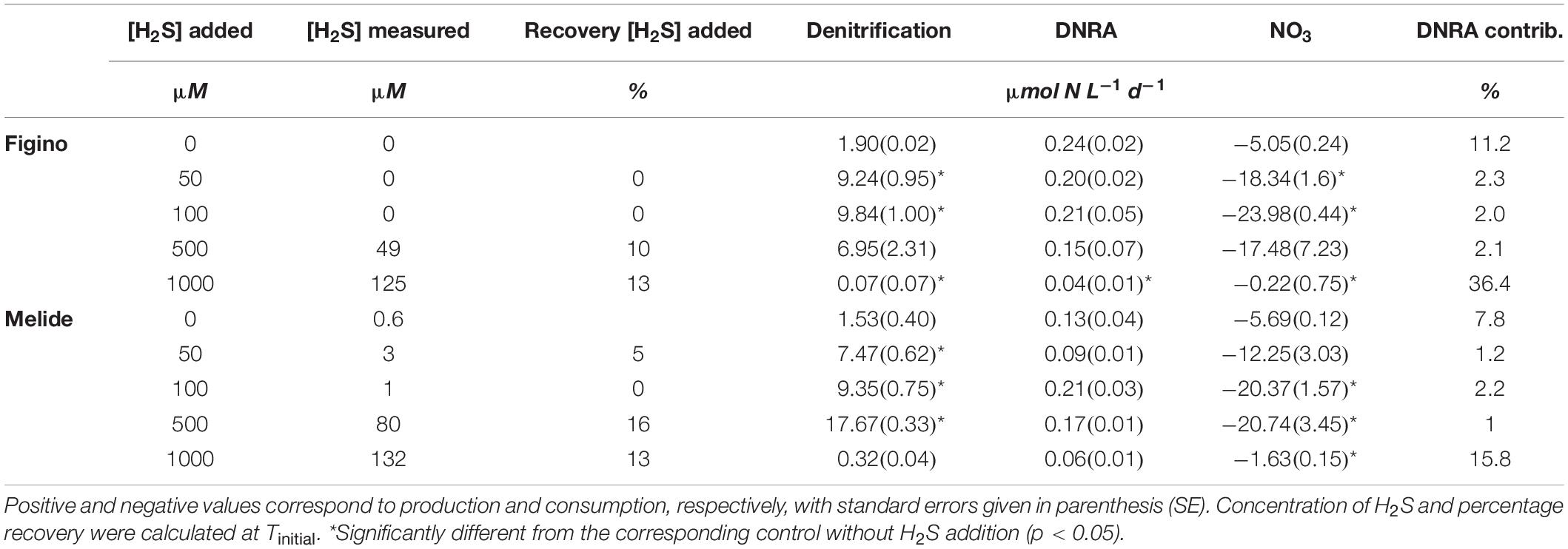
Table 3. Transformation rates of denitrification, DNRA, NO3– consumption rates (all μmol N L–1 d–1), and DNRA contribution to total nitrate reduction (%) from experiments supplemented with 15NO3– and H2S.
Denitrification was significantly enhanced when sulfide was added in the range of 50–500 μM H2S (corresponding to ≤80 μM of measured dissolved H2S; Table 3). Within this concentration range, denitrification was stimulated in proportion to increasing H2S at Melide, while it remained relatively constant at Figino. At 100 and 500 μM H2S, the stimulation was highest and reached 414% (Figino) and 959% (Melide), respectively, relative to unamended control incubations (Figure 4). The addition of H2S with a targeted concentration of 1 mM, resulted in measured dissolved H2S concentrations ≥ 125 μM, and caused a 96 and 80% decline in the denitrification rates at Figino and Melide, respectively (Figure 4). The response of the nitrate ammonifiers to the different H2S concentrations was less pronounced and, in most cases, statistically not significant (Table 3). At Figino, 15NH4+ production decreased slightly with increasing H2S concentration, while at Melide, DNRA was stimulated by 60 and 30% with 100 and 500 μM H2S added, respectively (Figure 4). At both sites, DNRA seemed also to be inhibited at the highest H2S levels, although to a lesser extent than denitrification (83 and 47% DNRA decrease at Figino and Melide, respectively).
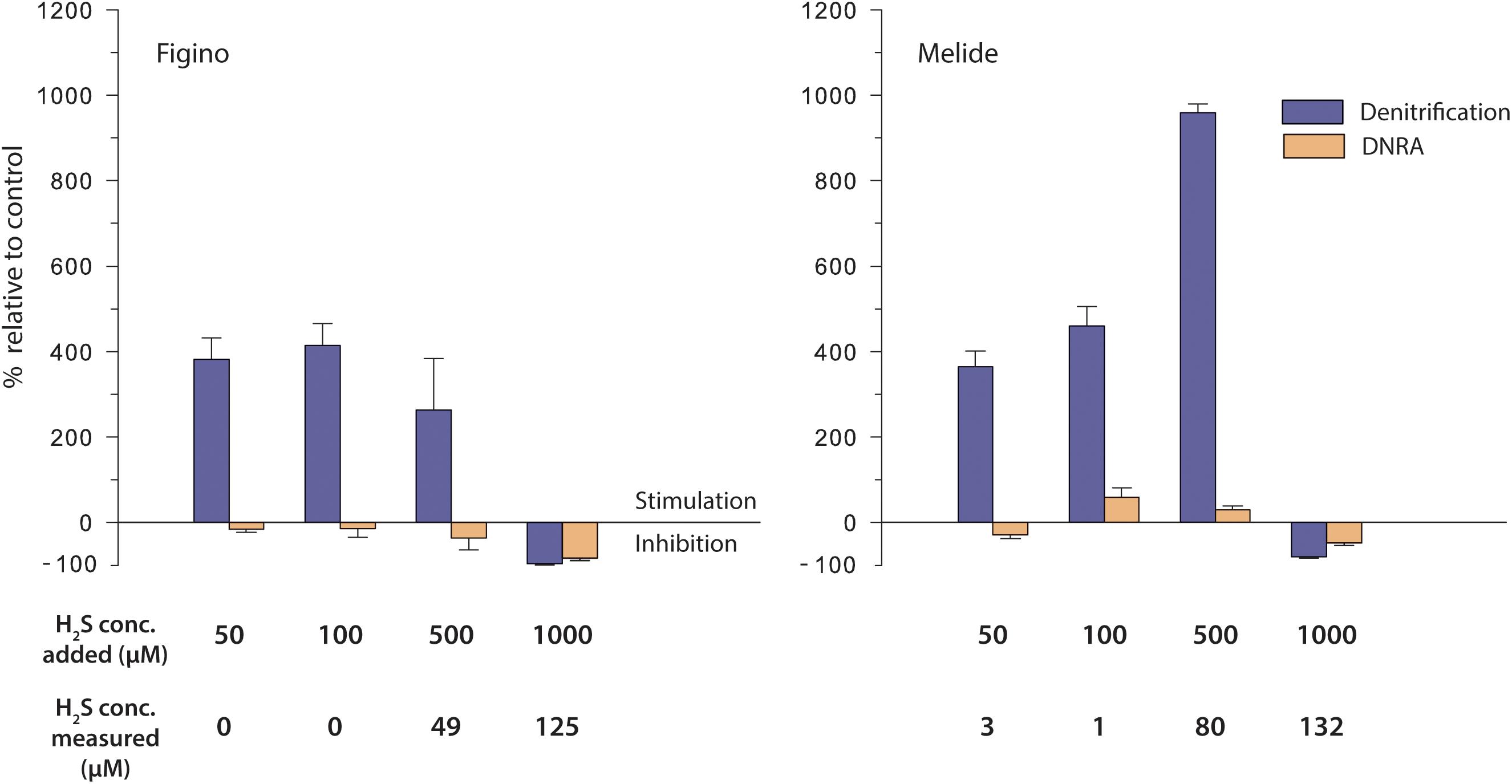
Figure 4. Effect of sulfide on denitrification and DNRA in anoxic sediment biomass incubation experiments amended with 15NO3– and different H2S additions. Stimulation (positive values) / inhibition (negative values) is expressed relative to controls without additions. Error bars represent standard errors (n = 3).
In incubations exposed to ≤500 μM H2S added, the relative contribution of DNRA to the total nitrate reduction decreased to a minimum of ∼2% (Figino) and ∼1% (Melide), respectively (compared to ∼11 and 8% in the controls, respectively). At the highest measured H2S concentration, the DNRA contribution increased to about 36% (Figino) and 16% (Melide), suggesting that the balance between denitrification and DNRA is regulated by the environmental H2S concentration, as was also the case with Fe2+. These experiments were repeated with sediment material collected in March 2017 and displayed very similar patterns (data not shown).
In line with the 15N tracer results, NO3– consumption increased with increasing H2S availability (up to 500 μM H2S added; Table 3). As for the other amendment experiments with Fe2+, NO3– consumption was not balanced by the formation of NO2– and 15N-N2/15NH4+ production. In control incubations, SO42– accumulated at a rate of 1.9 ± 1.8 μmol L–1 d–1. The production increased with the amount of added H2S and reached a maximum rate of 5.9 ± 0.5 μmol L–1 d–1 in the experiment where 500 μM of H2S was added. The rate dropped to 0.3 ± 1.5 μmol L–1 d–1 at the highest H2S concentration tested (Table 3).
Incubations With Mn2+ Additions
As for the Fe2+ addition experiments, we aimed to lower the ambient Mn2+ concentration in the microbial-biomass separation step (see above), but dissolved Mn2+ background concentrations remained substantial, at ∼30 and ∼36 μM for Figino and Melide incubations, respectively. Both in the controls and in all incubations amended with Mn2+, production of 15N-N2 was consistently greater than of 15NH4+. At the Figino station, denitrification and DNRA rates in unamended controls equaled 0.97 ± 0.14 and 0.13 ± 0.03 μmol L–1 d–1, respectively, and at Melide 1.24 ± 0.09 and 0.06 ± 0.02 μmol L–1 d–1, respectively. At Figino, at moderate Mn2+ addition (95 μM), denitrification was stimulated by about 40% compared to the corresponding controls (Figure 5). At higher Mn2+ concentrations, denitrification activity decreased with increasing Mn2+ concentration. At Melide, no stimulation of denitrification was observed. Our results revealed a proportional reduction of 15N-N2 formation with increasing Mn2+, corresponding to ∼80% inhibition at the highest Mn2+ concentration tested. At both stations, DNRA rates also decreased with rising Mn2+ levels.
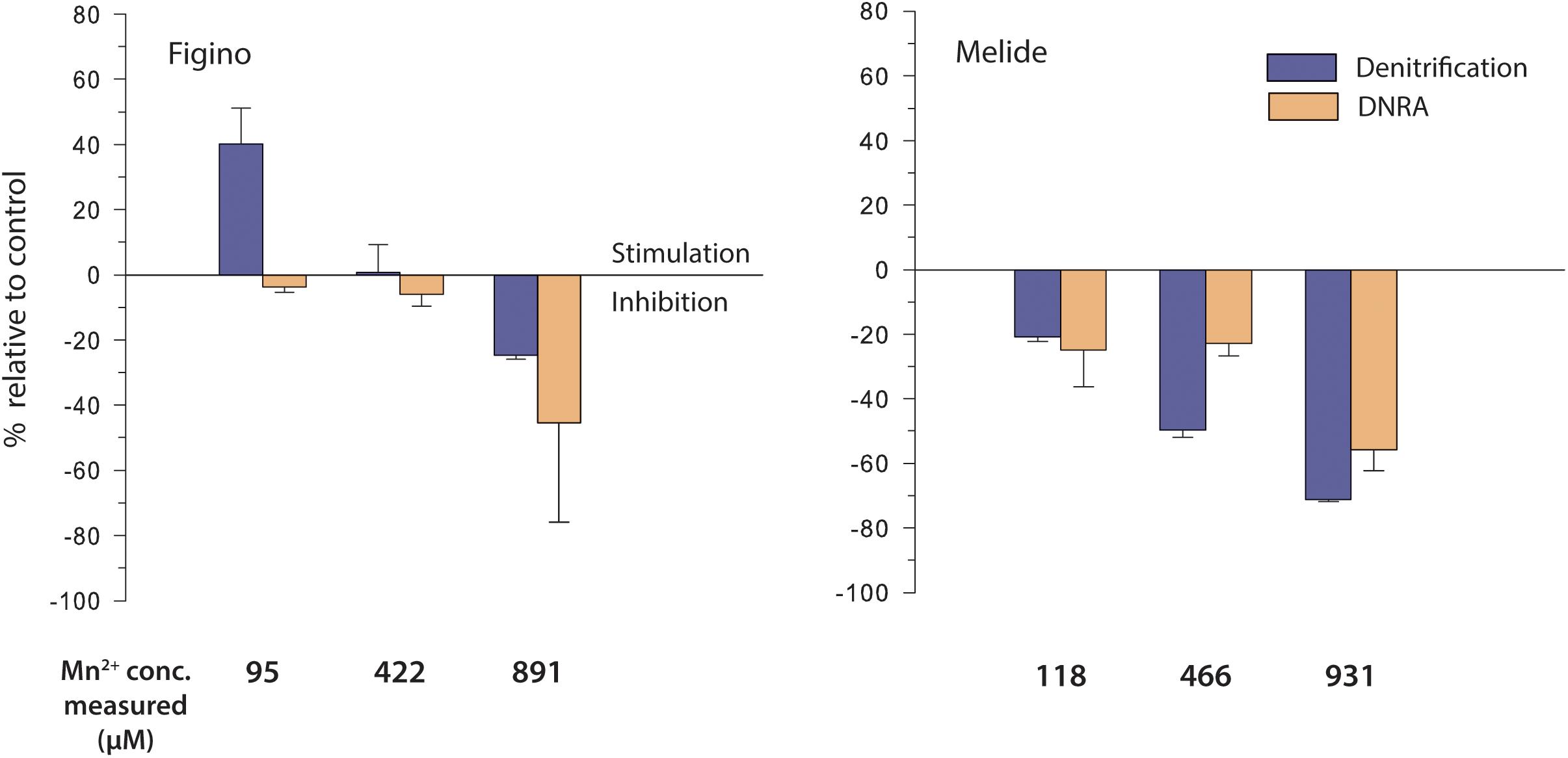
Figure 5. Effect of dissolved manganese on denitrification and DNRA in anoxic sediment biomass incubation experiments amended with 15NO3– and different Mn2+concentrations. Stimulation (positive values) / inhibition (negative values) is expressed relative to controls without additions. Error bars show standard errors (n = 3).
Transformation rates based on NO3– and Mn2+ concentration changes were very similar between sites and among treatments. In control incubations, nitrate consumption rates equaled 4.9 ± 0.5 μmol L–1 d–1 at both stations, and decreased to 2.4 ± 0.4 μmol L–1 d–1 in incubations supplemented with the highest Mn2+ concentration (891 and 931 μM at Figino and Melide, respectively). On the other hand, Mn2+ removal rates increased with increasing Mn2+ from 0 ± 0.2 μmol L–1 d–1 in the controls to 3.0 ± 1.6 μmol L–1 d–1 at highest Mn2+ levels. The lack of any significant stimulation of either DNRA or denitrification in most treatments supplemented with Mn2+ suggests that Mn2+, while being removed from the liquid phase (e.g., through adsorption onto particles), did not play a major role as electron donor for NO3– reduction in Lake Lugano sediments.
Discussion
Denitrification Versus DNRA in Lake Lugano Sediments
During the sampling campaigns in this study, denitrification was the main N-reduction process in slurries of Lake Lugano (South Basin) sediments. At both stations, the DNRA contribution to the total NO3– reduction showed moderate fluctuations between the different seasons, ranging between 31 to 46% but no clear seasonal trend was observed despite of the seasonal variations in bottom water oxygenation and NO3– contents. The contribution of DNRA to NO3– reduction was significantly higher than observed previously in flow-through whole-core incubations performed with sediments from the same basin (Wenk et al., 2014). Wenk et al. (2014) reported a maximum DNRA contribution to NO3– reduction of ∼12%, but also suggested that these measurements should be considered conservative, because they neither accounted for the production of 14NH4+ from ambient background NO3– nor for product NH4+ potentially retained in the intact sediment cores. The use of intact sediment cores provides a more accurate representation of in situ N-cycling conditions by maintaining the biogeochemical zonation of the sediment relative to slurry methods (Hansen et al., 2000; Robertson et al., 2019). However, relative potential process rates and their specific regulating factors can better be resolved through controlled manipulation experiments (Robertson et al., 2019), such as those performed here (discussed below).
Similar partitioning of the two dissimilative NO3–-reducing processes, with a relatively large contribution of DNRA has been observed in a wide range of environments, particularly in reduced sediments with high organic matter content and comparatively low nitrate levels (Burgin and Hamilton, 2007; Dong et al., 2011). The contribution of DNRA to total NO3– reduction can be particularly important in both estuarine sediments (∼5–91%; An and Gardner, 2002; Megonigal et al., 2004; Gardner et al., 2006; Burgin and Hamilton, 2007; Dong et al., 2009; Giblin et al., 2013; Roberts et al., 2014; Plummer et al., 2015; Kessler et al., 2019) and freshwater lake sediments (∼3–50%; Nizzoli et al., 2010; Wenk et al., 2014; Robertson and Thamdrup, 2017). Yet, the natural variability in these studied environments is quite high. The OC/NO3– ratio is often considered an important regulating factor of the relative contribution of the different benthic NO3–-reducing processes. DNRA can be stimulated at high OC and low (limiting) NO3– levels, while denitrification is generally dominant when NO3– is not limiting (Kraft et al., 2014; van den Berg et al., 2016). Differences with respect to the occurrence of DNRA versus denitrification across different ecosystems, however, may also be attributed to variable H2S and Fe2+ availability, with DNRA presumably being favored over denitrification at high levels of these inorganic substrates.
Biogeochemical Control of Fe2+on Denitrification and DNRA
In order to investigate the effect of inorganic substrates on NO3– reduction rates and on the partitioning between denitrification and DNRA we performed incubation experiments with sediment biomass, from which most of the sediment solids have been removed. For the investigation of Fe2+, H2S and Mn2+, which may adsorb to solid phases or react with particle-associated compounds, the exclusion of particulates is helpful in following the consumption of electron donors over time. While the transformation rates in such a system cannot be directly compared to the volume-based biogeochemical rate measurements with natural sediment, these incubations provide insights as to the metabolic potential for stimulation of denitrification and DNRA by alternative electron donors within a carefully controlled system.
The sediments in the southern basin of Lake Lugano are rich in dissolved Fe2+ and Mn2+ and show seasonal variation in the concentrations of these solutes in the sediment surface layer (Figure 2; Lazzaretti et al., 1992). The apparent relation to fluctuations in bottom water oxygenation and the presence of NO3– suggests a potential coupling between NO3– reduction and Fe2+ oxidation. Our results revealed a complex control from Fe2+ on the balance between denitrification and DNRA with relative stimulation of the former and latter at low and high concentrations, respectively. The relative stimulation of DNRA at high Fe2+ concentration (≥2433 and 1303 μM Fe2+ at Figino and Melide, respectively), agrees qualitatively with observations by Robertson et al. (2016) and Robertson and Thamdrup (2017), who reported a stimulation of DNRA and Fe2+ oxidation rates in estuarine and lake sediments with Fe2+ additions ranging between 165 and 5000 μM. However, they did not observe a relative stimulation of denitrification at the lower range of Fe2+ concentrations tested, which appears to contrast with the present study, where denitrification was favored over DNRA at the lower Fe2+ concentrations (≤258 μM) at both stations. Our observations are in line, however, with a pure-culture study by Chakraborty et al. (2011) who also observed enhanced rates of denitrification and increased growth yields in presence of environmentally relevant substrate concentrations (≤250 μM Fe2+, 20 μM acetate, 100 μM NO3–) for mixotrophic Acidovorax sp., while at higher Fe2+ levels growth ceased as cells became encrusted with FeIII-oxides. Indeed, Acidovorax sp. and Siderooxydans sp., another abundant and putative Fe2+-oxidizing NO3–-reducing bacterium, have been detected in the top sediment layers at Figino and Melide using 16S rRNA gene amplicon sequencing (Cojean, 2019). To date, microbial Fe2+-dependent NO3– reduction is mainly attributed to microbes that sustain their growth energy from the mixotrophic use of Fe2+ together with an organic co-substrate (Straub et al., 1996; Melton et al., 2014).
The coupling between Fe2+ oxidation and denitrification has also been highlighted in a variety of natural environments including lake waters (Michiels et al., 2017), marine and estuarine sediments (Laufer et al., 2016; Robertson et al., 2016), soils (Ratering and Schnell, 2001), and activated sludge from waste-water treatment plants (Nielsen and Nielsen, 1998). However, the majority of these studies used millimolar substrate concentrations in order to investigate the influence of Fe2+ on NO3– reduction (Table 1).
In contrast to results from previous studies, it appears that in our experiments, nitrate ammonifiers did not preferentially use Fe2+ as electron donor. At low Fe2+ levels, there was no indication for any Fe2+-induced stimulation of DNRA. In this regard, we argue that the increase in the relative contribution of DNRA to total nitrate reduction at higher Fe2+ levels, may not have been a direct result of an increase in the rate of DNRA coupled to Fe2+ oxidation. Rather, we speculate that nitrate ammonifiers were less sensitive than denitrifiers to high Fe2+ levels, and that very high concentrations of Fe2+ may have indirectly favored DNRA bacteria by suppression of organotrophic denitrification and a resulting increased availability of organic substrates.
In previous studies investigating the coupling between Fe2+ oxidation and NO3– reduction, inhibition of denitrification under Fe-rich conditions has been attributed to cell encrustation from FeIII-oxide formation around the cell membrane and inside the periplasm (Kappler et al., 2005; Muehe et al., 2009; Klueglein et al., 2014; Nordhoff et al., 2017), which affects bacterial metabolism by limiting substrate uptake, and may even lead to cell damage. However, cell encrustation was, so far, only observed using millimolar Fe2+ concentrations whereas our data displayed a significant inhibition of denitrification at around 400 μM Fe2+ already, pointing to a potential direct Fe2+ toxicity effect on the metabolism. Iron toxicity under anoxic conditions has previously been attributed to inhibition of the F-ATPase (Dunning et al., 1998) and replacement of active-site metal cofactors (Crichton, 2009). But so far, this toxicity effect has only been examined in cultures of anoxygenic phototrophs and streptococci (Dunning et al., 1998; Poulain and Newman, 2009). Our results show that Fe2+ inhibition on denitrification in natural habitats may occur at lower Fe2+ concentration than previously thought based on studies of pure or enrichment culture experiments.
Stimulation of Denitrification by H2S
In lake sediments, H2S is continuously produced by mineralization of sulfur-containing biomass and respiratory SO42– reduction. In ferruginous surface sediments like in the south basin of Lake Lugano, rapid reaction of H2S with dissolved Fe2+ or Fe-oxides leaves the porewater free of dissolved H2S (Lazzaretti et al., 1992). Despite this, independent molecular analyses using 16S rRNA gene sequencing (Cojean, 2019) revealed a variety of sulfur-oxidizing bacteria, among them several abundant taxa with a metabolic potential for anaerobic respiration with nitrate or nitrite (e.g., Sulfuritalea sp., Sulfurimonas sp., Sulfurovum sp. Thiobacillus sp.). Moreover, among the three inorganic substrates tested in this study, sulfide additions showed the strongest stimulation on NO3– consumption and denitrification.
Stimulation of denitrification by H2S has been observed in pure and enrichment cultures (Senga et al., 2006; Campos et al., 2008; Table 1), stratified water columns (Brettar and Rheinheimer, 1991; Burgin et al., 2012; Wenk et al., 2013), sediments (Brunet and Garcia-Gil, 1996; Hayakawa et al., 2013; Deng et al., 2015), but also in engineered systems such as anaerobic digesters (Sher et al., 2008). Often, the degree of H2S-induced stimulation was directly related to the H2S concentration. In natural systems, denitrification was enhanced primarily at lower H2S concentration (<100 μM; e.g., Senga et al., 2006; Burgin et al., 2012; Bowles et al., 2012), while it was strongly inhibited at higher sulfide levels (discussed below). At first sight, denitrification in our study may appear less sensitive toward high H2S additions. Considering, however, that most of the added H2S (>84%) was removed from solution by reaction with Fe or Mn, we find that our results are in good agreement with these reports. It is likely that increased free H2S concentrations ≥ 125 μM (Table 3) in our treatments with highest H2S addition inhibited microbial NO3– reduction. Uncharged sulfide (H2S) can easily diffuse across cell membranes and it is therefore recognized as the most toxic form of the compound (Barton et al., 2014), exerting a stronger inhibitory effect on bacteria than FeS, S0 or S2O32–. At elevated concentrations (>100 μM), free H2S can act as growth-inhibitor by e.g., denaturing proteins through disruption of disulfide cross-links between polypeptide chains, or inactivating the redox centers of metalloenzymes, and it can, ultimately lead to cell death (Khan et al., 1990; Wu et al., 2015). In contrast, FeS has been considered as a non-toxic repository of suitable electrons for sulfur bacteria, and inhibition of denitrification by FeS was only observed at >10 mmol S L–1 (Garcia-Gil and Golterman, 1993). To date, knowledge on specific effects of H2S on NO3–-reducing microbial groups is scarce. A study on marine microbial communities reports a lag of bacterial growth of potential denitrifiers (Vibrio sp., Marinobacter sp., Pseudomonas stutzeri) with increasing H2S concentration (Mirzoyan and Schreier, 2014). Based on pure culture studies reporting on increased concentrations of N-intermediates (NO2–, NO, N2O) in the presence of H2S, it has been proposed that H2S can have an inhibitory effect on the respective N-compound reducing enzymes (Sørensen et al., 1980; Aelion and Warttinger, 2009). Aside from such conclusions based on the accumulation of N-intermediates, however, knowledge is lacking regarding the mechanisms of inhibition by H2S at the enzyme level.
As opposed to denitrification, DNRA is often enhanced under highly sulfidic conditions (Brunet and Garcia-Gil, 1996; Gardner et al., 2006; Lu et al., 2013; Plummer et al., 2015) and may even dominate reductive NO3– transformation, leading to the retention of reactive N in the ecosystem (An and Gardner, 2002; Dong et al., 2011; Murphy et al., 2020). Some pure cultures of H2S-oxidizing DNRA bacteria can grow at millimolar concentrations of free H2S (e.g., Eisenmann et al., 1995), showing that physiological mechanisms exist that allow them to cope with such high levels of this toxic compound. This contrasts with our observations where DNRA activity was somewhat suppressed already in the presence of low H2S concentrations, and, just as denitrification, inhibited at free H2S concentrations > 125 μM. Unlike previous studies in other environments (e.g., Brunet and Garcia-Gil, 1996; Otte et al., 1999; Sayama et al., 2005) we do not find that H2S is the preferred substrate for DNRA bacteria in the ferruginous sediments in the south basin of Lake Lugano where the autochthonous microbial communities are not exposed to significant concentrations of free dissolved H2S. We speculate therefore that the selective pressure was not sufficient to enable communities of sulfide-tolerant DNRA bacteria to become enriched. As a consequence, the microbial communities are not well adapted, and thus sensitive, to high H2S concentrations. It seems that low free H2S concentrations, along with FeS and S0 as the most important reduced sulfur species fueling NO3– reduction, exert a beneficial remediation effect by increasing the contribution of N-removal (denitrification) relative to N-retention (DNRA).
Inhibitory Effects of High Mn2+ Concentration on Nitrate Reduction
Based on thermodynamic considerations (Luther et al., 1997) and porewater concentration profiles (Aller, 1990; Schulz et al., 1994), Mn2+-driven nitrate reduction has been suggested to occur in natural systems, particularly in manganese-rich sediments, but solid experimental proof is still lacking (Madison et al., 2013). The main goal of this part of our work was to assess, whether nitrate-dependent Mn2+ oxidation exists in Mn2+-rich freshwater sediments. Excluding the Figino Station, where denitrification seemed to be stimulated in the presence of 100 μM Mn2+, our results show that rates of both denitrification and DNRA decreased with increasing Mn2+ concentrations. No comparable stimulation was observed in sediments from Melide. Based on these results, we conclude that Mn2+ does not play a biogeochemically relevant role as reductant for NO3– in the sediments under investigation. In line with our data, Schippers et al. (2005) did not find enhanced manganese oxidation with sensitive 54Mn radiotracer experiments, in the anoxic water column of the Black Sea when NO3–, NO2–, or N2O was present. On the other hand, a stimulation of denitrification and DNRA with Mn2+ addition was observed in the anoxic water column of the Baltic Sea. It remained unclear, however, whether this stimulation was due to an indirect effect of Mn2+, e.g., by the removal of an inhibitory substance (Bonaglia et al., 2016).
In most of our experiments, Mn2+ clearly exerted an inhibitory effect on both denitrification and DNRA. The reason for this observation is not clear, but could reflect a general toxicity of high Mn2+ concentrations on the activity of microbes. Mn toxicity has been reported for mammals, plants, and prokaryotes (Hohle and O’Brian, 2014), where Mn over-accumulation is often correlated with deficiencies in other transition-metals of physiological relevance. This can then lead to mismetallation of regulatory transcription factors and key enzymes, affecting growth, sensitivity toward reactive oxygen species, and virulence (Zeinert et al., 2018). For instance, Mn can bind to the ferric uptake regulator (Fur) in the Gamma-Proteobacterium E. coli. As a consequence, iron import systems are repressed, intracellular Fe levels drop, and, for example, heme synthesis is impeded (Martin et al., 2015). Mn also interferes competitively with secondary Fe import (Martin et al., 2015), and high extracellular Mn2+ levels can also competitively inhibit magnesium (Mg2+) uptake (Silver and Clark, 1971). Manganese toxicity has been investigated in detail for the soil Alpha-Proteobacterium Bradyrhizobium japonicum (Hohle and O’Brian, 2014). It was demonstrated that Mn2+ can enter the cells through open Mg2+ transporters under low Mg conditions, and exert a toxic effect by displacing Mg in proteins or other macromolecules (Hohle and O’Brian, 2014). Replacing Mg2+ with Mn2+ as a cofactor in enzymes affects their activity and possibly lead to the disregulation of metabolic pathways (Hohle and O’Brian, 2014).
Whether the mechanisms of Mn2+ toxicity established in model organisms also apply to complex environmental communities is not known yet. In our experiments, where we added up to 900 μM MnCl2 (in presence of only 0.34 mM Mg2+; Supplementary Table 1) and observed a decreasing N-transformation activity with increasing Mn2+ levels, Mn2+ toxicity by interference with Mg2+ homoeostasis is plausible. Yet much more research with environmentally relevant microorganisms is required to better understand the effects of increased transition metal concentrations such as Mn and Fe on environmental microbes and the biogeochemical processes they catalyze.
Conclusion
Benthic NO3– reduction in the South Basin of Lake Lugano is mainly driven by denitrification and DNRA, whereas anammox is negligible. Our results show that Fe2+ and H2S are important controlling factors for the partitioning between denitrification and DNRA, and that the effect is concentration-dependent. Nitrate was mostly reduced through denitrification at lower levels of dissolved H2S (<80 μM) and Fe2+ (<258 μM). The relative contribution of DNRA to the overall benthic N reduction increased under highly ferruginous conditions (∼1000 μM Fe2+), possibly as a consequence of reduced substrate competition with denitrifiers, which were almost completely inhibited. The role of Mn2+ as electron donor for NO3– reduction was negligible, as was its influence on the partitioning between denitrification and nitrate ammonification. All three inorganic substrates, however, had strong inhibitory effects at concentrations significantly higher than the prevailing environmental concentrations. Our study implies that the fate of N may be linked to changes in the availability of inorganic substrates within surface sediments.
During periods of bottom water anoxia in eutrophic lakes, reduced inorganic species diffuse out of the sediment into the bottom waters. In the south basin of Lake Lugano, and potentially other iron-rich eutrophic lakes, the zone of Fe2+ driven NO3– reduction will extend far into the water column. The prevailing conditions of low Fe2+ concentrations and nearly absent free H2S should be favorable for denitrification rather than DNRA. Periods of extended anoxia under enhanced stratified conditions, however, may lead to the accumulation of significant amounts of Fe2+, Mn2+, or even H2S (Lazzaretti et al., 1992; Lehmann et al., 2015), and the conditions for denitrification and DNRA in near-bottom lake waters or surface sediments may thereby develop from a stimulating into an inhibitory mode, with important implications for the ultimate fate of reactive N in a lake.
We clearly demonstrated the differential role Fe2+, H2S, and Mn2+ can play in regulating the partitioning between denitrification and DNRA. Predictions, however, on how these potential inorganic electron donors act together to possibly shift the balance between the two N-cycling processes and to regulate the overall fixed N-elimination rate in lake sediments remains a challenge.
Data Availability Statement
The raw data supporting the conclusions of this article will be made available by the authors, without undue reservation.
Author Contributions
JZ and ML initiated the project. AC performed sample collection and conducted the experimental work. AC, ML, and JZ performed the data analysis and interpretation. AC and JZ prepared the manuscript with input from ML, ER, and BT.
Funding
This study was funded by the Swiss National Science Foundation (SNF) project 153055 granted to JZ and ML.
Conflict of Interest
The authors declare that the research was conducted in the absence of any commercial or financial relationships that could be construed as a potential conflict of interest.
Acknowledgments
Thomas Kuhn is acknowledged for great technical support in the laboratory. Stefano Beatrizotti, Fabio Lepori, Marco Simona (SUPSI), and Maciej Bartosiewicz, Guangyi Su, and Jana Tischer (Uni Basel) are thanked for their help during sampling campaigns on the lake. Fabio Lepori is also thanked for sharing water-column hydrochemical data, and Anna-Neva Visser for fruitful discussions.
Supplementary Material
The Supplementary Material for this article can be found online at: https://www.frontiersin.org/articles/10.3389/fmicb.2020.01158/full#supplementary-material
References
Aelion, C. M., and Warttinger, U. (2009). Low sulfide concentrations affect nitrate transformations in freshwater and saline coastal retention pond sediments. Soil Biol. Biochem. 41, 735–741.
Aller, R. C. (1990). Bioturbation and manganese cycling in hemipelagic sediments. Philos. Trans. R. Soc. Lond. A 331, 51–68.
An, S., and Gardner, W. S. (2002). Dissimilatory nitrate reduction to ammonium (DNRA) as a nitrogen link, versus denitrification as a sink in a shallow estuary Laguna Madre (Baffin Bay, Texas). Mar. Ecol. Prog. Ser. 41, 41–50.
Barbieri, A., and Polli, B. (1992). Description of Lake Lugano. Aquat. Sci. 54, 181–183. doi: 10.1136/bmjgh-2019-002025
Barton, L. L., Fardeau, M. L., and Fauque, G. D. (2014). Hydrogen sulfide: a toxic gas produced by dissimilatory sulfate and sulfur reduction and consumed by microbial oxidation. Met. Ions Life Sci. 14, 237–277. doi: 10.1007/978-94-017-9269-1_10
Behrendt, A., De Beer, D., and Stief, P. (2013). Vertical activity distribution of dissimilatory nitrate reduction in coastal marine sediments. Biogeosciences 10, 7509–7523.
Beller, H. R., Zhou, P., Legler, T. C., Chakicherla, A., Kane, S., Letain, T. E., et al. (2013). Genome-enabled studies of anaerobic, nitrate-dependent iron oxidation in the chemolithoautotrophic bacterium Thiobacillus denitrificans. Front. Microbiol. 4:249. doi: 10.3389/fmicb.2013.00249
Benz, M., Brune, A., and Schink, B. (1998). Anaerobic and aerobic oxidation of ferrous iron at neutral pH by chemoheterotrophic nitrate-reducing bacteria. Arch. Microbiol. 169, 159–165.
Blees, J., Niemann, H., Wenk, C. B., Zopfi, J., Schubert, C. J., Jenzer, J. S., et al. (2014). Bacterial methanotrophs drive the formation of a seasonal anoxic benthic nepheloid layer in an alpine lake. Limnol. Oceanogr. 59, 1410–1420.
Bonaglia, S., Klawonn, I., De Brabandere, L., Deutsch, B., Thamdrup, B., and Brüchert, V. (2016). Denitrification and DNRA at the Baltic Sea oxic-anoxic interface: substrate spectrum and kinetics. Limnol. Oceanogr. 61, 1900–1915.
Bowles, M. W., Nigro, L. M., Teske, A. P., and Joye, S. B. (2012). Denitrification and environmental factors influencing nitrate removal in Guaymas Basin hydrothermally altered sediments. Front. Microbiol. 3:377. doi: 10.3389/fmicb.2012.00377
Braman, R. S., and Hendrix, S. A. (1989). Nanogram nitrite and nitrate determination in environmental and biological materials by vanadium(III) reduction with chemiluminescence detection. Anal. Chem. 61, 2715–2718.
Brettar, I., and Rheinheimer, G. (1991). Denitrification in the Central Baltic: evidence for H2S-oxidation as motor of denitrification at the oxic-anoxic interface. Mar. Ecol. Prog. Ser. 77, 157–169.
Brunet, R. C., and Garcia-Gil, L. J. (1996). Sulfide-induced dissimilatory nitrate reduction to ammonia in anaerobic freshwater sediments. FEMS Microbiol. Ecol. 21, 131–138.
Burgin, A. J., and Hamilton, S. K. (2007). Have we overemphasized the role of denitrification in aquatic ecosystems? A review of nitrate removal pathways. Front. Ecol. Environ. 5, 89–96. doi: 10.1890/1540-929520075[89:HWOTRO]2.0.CO;2
Burgin, A. J., Hamilton, S. K., Jones, S. E., and Lennon, J. T. (2012). Denitrification by sulfur-oxidizing bacteria in a eutrophic lake. Aquat. Microb. Ecol. 66, 283–293.
Campos, J. L., Carvalho, S., Portela, R., Mosquera-Corral, A., and Méndez, R. (2008). Kinetics of denitrification using sulphur compounds: effects of S/N ratio, endogenous and exogenous compounds. Bioresour. Technol. 99, 1293–1299.
Chakraborty, A., and Picardal, F. (2013). Induction of nitrate-dependent Fe(II) oxidation by Fe(II) in Dechloromonas sp. Strain UWNR4 and Acidovorax sp. Strain 2AN. Appl. Environ. Microbiol. 79, 748–752. doi: 10.1128/AEM.02709-12
Chakraborty, A., Roden, E. E., Schieber, J., and Picardal, F. (2011). Enhanced growth of Acidovorax sp. Strain 2AN during nitrate-dependent Fe(II) oxidation in batch and continuous-flow systems. Appl. Environ. Microbiol. 77, 8548–8556. doi: 10.1128/AEM.06214-11
Chaudhuri, S. K., Lack, J. G., and Coates, J. D. (2001). Biogenic magnetite formation through anaerobic biooxidation of Fe(II). Appl. Environ. Microbiol. 67, 2844–2848.
Chen, D., Liu, T., Li, X., Li, F., Luo, X., Wu, Y., et al. (2018). Biological and chemical processes of microbially mediated nitrate-reducing Fe(II) oxidation by Pseudogulbenkiana sp. Strain 2002. Chem. Geol. 476, 59–69.
Chutivisut, P., Isobe, K., Powtongsook, S., Pungrasmi, W., and Kurisu, F. (2018). Distinct microbial community performing dissimilatory nitrate reduction to ammonium (DNRA) in a high C/NO3- reactor. Microbes Environ. 33, 264–271. doi: 10.1264/jsme2.ME17193
Chutivisut, P., Pungrasmi, W., and Powtongsook, S. (2014). Denitrification and dissimilatory nitrate reduction to ammonium (DNRA) activities in freshwater sludge and biofloc from Nile Tilapia aquaculture systems. J. Water Environ. Tech. 12, 347–356.
Cline, J. D. (1969). Spectrophotometric determination of hydrogen sulfide in natural waters. Limnol. Oceanogr. 14, 454–458.
Coby, A. J., Picardal, F., Shelobolina, E., Xu, H., and Roden, E. E. (2011). Repeated anaerobic microbial redox cycling of iron. Appl. Environ. Microbiol. 77, 6036–6042.
Cojean, A. N. Y. (2019). Environmental Controls of Benthic Nitrogen Cycling in Lake Lugano South Basin, Switzerland – Pathways, Rates, Isotopic Signatures and Microbial Communities. Ph.D. thesis, University of Basel, Basel.
Crichton, R. R. (2009). Inorganic Biogeochemistry of iron Metabolism: From Molecular Mechanisms to Clinical Consequences, 3rd Edn. New York, NY: John Wiley and Sons.
Crowe, S. A., Treusch, A. H., Forth, M., Li, J., Magen, C., Canfield, D. E., et al. (2017). Novel anammox bacteria and nitrogen loss from Lake Superior. Sci. Rep. 7:13757. doi: 10.1038/s41598-017-12270-1
Cytryn, E., Minz, D., Gelfand, I., Neori, A., Gieseke, A., De Beer, D., et al. (2005). Sulfide-oxidizing activity and bacterial community structure in a fluidized bed reactor from a zero-discharge mariculture system. Environ. Sci. Technol. 39, 1802–1810.
Dannenberg, S., Kroder, M., Dilling, W., and Cypionka, H. (1992). Oxidation of H2, organic compounds and inorganic sulfur compounds coupled to reduction of O2 or nitrate by sulfate-reducing bacteria. Arch. Microbiol. 159, 93–99.
Deng, F., Hou, L., Liu, M., Zheng, Y., Yin, G., Li, X., et al. (2015). Dissimilatory nitrate reduction processes and associated contribution to nitrogen removal in sediments of the Yangtze Estuary. J. Geophys. Res. Biogeosci. 120, 1521–1531.
Dong, L. F., Naqasima-Sobey, M., Smith, C. J., Rusmana, I., Phillips, W., Stott, A., et al. (2011). Dissimilatory reduction of nitrate to ammonium, not denitrification or anammox, dominates benthic nitrate reduction in tropical estuaries. Limnol. Oceanogr. 56, 279–291.
Dong, L. F., Smith, C. J., Papaspyrou, S., Stott, A., Osborn, A. M., and Nedwell, D. B. (2009). Changes in benthic denitrification, nitrate ammonification, and anammox process rates and nitrate and nitrite reductase gene abundances along an estuarine nutrient gradient (the Colne Estuary, United Kingdom). Appl. Environ. Microbiol. 75, 3171–3179. doi: 10.1128/AEM.02511-08
Dunning, J. C., Ma, Y., and Marquis, R. E. (1998). Anaerobic killing of oral streptococci by reduced, transition metal cations. Appl. Environ. Microbiol. 64, 27–33.
Eisenmann, E., Beuerle, J., Sulger, K., Kroneck, P. M. H., and Schumacher, W. (1995). Lithotrophic growth of Sulfurospirillum deleyianum with sulfide as electron donor coupled to respiratory reduction of nitrate to ammonia. Arch. Microbiol. 164, 180–185.
Froelich, P. N., Klinkhammer, G. P., Bender, M. L., Luedtke, N. A., Heath, G. R., Cullen, D., et al. (1979). Early oxidation of organic matter in pelagic sediments of the eastern equatorial Atlantic: suboxic diagenesis. Geochim. Cosmochim. Acta 43, 1075–1090.
Garcia-Gil, L. J., and Golterman, H. L. (1993). Kinetics of FeS-mediated denitrification in sediments from the Camargue (Rhone delta, southern France). FEMS Microbiol. Ecol. 13, 85–92.
Gardner, W. S., McCarthy, M. J., An, S., and Sobolev, D. (2006). Nitrogen fixation and dissimilatory nitrate reduction to ammonium (DNRA) support nitrogen dynamics in Texas estuaries. Limnol. Oceanogr. 51, 558–568.
Giblin, A. E., Tobias, C. R., Song, B., Weston, N., Banta, G. T., and Rivera-Monroy, V. H. (2013). The importance of dissimilatory nitrate reduction to ammonium (DNRA) in the nitrogen cycle of coastal ecosystems. Oceanography 26, 124–131.
Hafenbradl, D., Keller, M., Dirmeier, R., Rachel, R., Roßnagel, P., Burggraf, S., et al. (1996). Ferroglobus placidus gen. nov., sp. nov., a novel hyperthermophilic archaeum that oxidizes Fe2+ at neutral pH under anoxic conditions. Arch. Microbiol. 166, 308–314.
Hansen, H. P., and Koroleff, F. (1999). “Determination of nutrients,” in Methods of Seawater Analysis, 3rd Edn, (Weinheim: Wiley-VCH), 159–228.
Hansen, J. W., Thamdrup, B., and Jørgensen, B. B. (2000). Anoxic incubation of sediment in gas-tight plastic bags: a method for biogeochemical process studies. Mar. Ecol. Prog. Ser. 208, 273–282.
Hardison, A. K., Algar, C. K., Giblin, A. E., and Rich, J. J. (2015). Influence of organic carbon and nitrate loading on partitioning between dissimilatory nitrate reduction to ammonium (DNRA) and N2 production. Geochim. Cosmochim. Acta 164, 146–160.
Hauck, S., Benz, M., Brune, A., and Schink, B. (2001). Ferrous iron oxidation by denitrifying bacteria in profundal sediments of a deep lake (Lake Constance). FEMS Microbiol. Ecol. 37, 127–134.
Hayakawa, A., Hatakeyama, M., Asano, R., Ishikawa, Y., and Hidaka, S. (2013). Nitrate reduction coupled with pyrite oxidation in the surface sediments of a sulfide-rich ecosystem. J. Geophys. Res. Biogeosci. 118, 639–649.
Hohle, T. H., and O’Brian, M. R. (2014). Magnesium-dependent processes are targets of bacterial manganese toxicity. Mol. Microbiol. 93, 736–747. doi: 10.1111/mmi.12687
Holmer, M., and Storkholm, P. (2001). Sulphate reduction and sulphur cycling in lake sediments: a review. Freshw. Biol. 46, 431–451.
Jakob, A., Binderheim-Bankay, E., and Davis, J. S. (2002). National long-term surveillance of Swiss rivers. Verh. Internat. Verein. Limnol. 28, 1101–1106. doi: 10.1016/j.scitotenv.2015.01.091
Jensen, H. S., and Thamdrup, B. (1993). Iron-bound phosphorus in marine sediments as measured by bicarbonate-dithionite extraction. Hydrobiology 253, 47–59.
Jensen, M. M., Petersen, J., Dalsgaard, T., and Thamdrup, B. (2009). Pathways, rates, and regulation of N2 production in the chemocline of an anoxic basin, Mariager Fjorg, Denmark. Mar. Chem. 113, 102–113.
Kamp, A., Stief, P., and Schulz-Vogt, H. (2006). Anaerobic sulfide oxidation with nitrate by a freshwater Beggiatoa enrichment culture. Appl. Environ. Microbiol. 72, 4755–4760.
Kappler, A., Schink, B., and Newman, D. K. (2005). Fe(III) mineral formation and cell encrustation by the nitrate-dependent Fe(II)-oxidizer strain BoFeN1. Geobiology 4, 235–245.
Kessler, A. J., Wawryk, M., Marzocchi, U., Roberts, K. L., Wong, W. W., Risgaard-Petersen, N., et al. (2019). Cable bacteria promote DNRA through iron sulfide dissolution. Limnol. Oceanogr. 64, 1228–1238.
Khan, A. A., Schuler, M. M., Prior, M. G., Yong, S., Coppock, R. W., Florence, L. Z., et al. (1990). Effects of hydrogen sulfide exposure on lung mitochondrial respiratory chain enzymes in rats. Toxicol. Appl. Pharmacol. 103, 482–490.
Klueglein, N., and Kappler, A. (2013). Abiotic oxidation of Fe(II) by reactive nitrogen species in culture of nitrate-reducing Fe(II) oxidizer Acidovorax sp. BoFeN1 – questioning the existence of enzymatic Fe(II) oxidation. Geobiology 11, 180–190.
Klueglein, N., Zeitvogel, F., Stierhof, Y.-D., Floetenmeyer, M., Konhauser, K. O., and Kappler, A. (2014). Potential role of nitrite for abiotic Fe(II) oxidation and cell encrustation during nitrate reduction by denitrifying bacteria. Appl. Environ. Microbiol. 80, 1051–1061. doi: 10.1128/AEM.03277-13
Kojima, H., and Fukui, M. (2011). Sulfuritalea hydrogenivorans gen. nov., sp. nov., a facultative autotroph isolated from a freshwater lake. Int. J. Syst. Evol. Microbiol. 61, 1651–1655. doi: 10.1099/ijs.0.024968-0
Kraft, B., Tegetmeyer, H. E., Sharma, R., Klotz, M. G., Ferdelman, T. G., Hettich, R. L., et al. (2014). The environmental controls that govern the end product of bacterial nitrate respiration. Science 345, 676–679. doi: 10.1126/science.1254070
Krom, M. D. (1980). Spectrophotometric determination of ammonia: a study of a modified Berthelot reaction using salicylate and dichloroisocyanurate. Analyst 105, 305–316.
Lack, J. G., Chaudhuri, S. K., Chakraborty, R., Achenbach, L. A., and Coates, J. D. (2002). Anaerobic biooxidation of Fe(II) by Dechlorosoma suillum. Microbiol. Ecol. 43, 424–431.
Laufer, K., Røy, H., Jørgensen, B. B., and Kappler, A. (2016). Evidence for the existence of autotrophic nitrate-reducing Fe(II)-oxidizing bacteria in marine coastal sediment. Appl. Environ. Microbiol. 82, 6120–6131.
Lazzaretti, M. A., Hanselmann, K. W., Brandl, H., Span, D., and Bachofen, R. (1992). The role of sediments in the phosphorous cycle in Lake Lugano. Seasonal and spatial variability of microbiological processes at the sediment-water surface. Aquat. Sci. 54, 285–299.
Lehmann, M. F., Simona, M., Wyss, S., Blees, J. H., Frame, C. H., Niemann, H., et al. (2015). Powering up the “biogeochemical engine”: the impact of exceptional ventilation of a deep meromictic lake on the lacustrine redox, nutrient and methane balances. Front. Earth Sci. 3:45. doi: 10.3389/feart.2015.00045
Lu, W.-W., Zhang, H.-L., and Shi, W.-M. (2013). Dissimilatory nitrate reduction to ammonium in an anaerobic agricultural soil as affected by glucose and free sulfide. J. Soil Biol. 58, 98–104.
Luther, G. W., Sundby, B., Lewis, B. J., Brendel, P. J., and Silverberg, N. (1997). Interactions of manganese with the nitrogen cycle: Alternative pathways to dinitrogen. Geochim. Cosmochim. Acta 61, 4043–4052.
Madison, A. S., Tebo, B. M., Mucci, A., Sundby, B., and Luther, G. W. (2013). Abundant porewater Mn(III) is a major component of the sedimentary redox system. Science 341, 875–878. doi: 10.1126/science.1241396
Martin, J. E., Waters, L. S., Storz, G., and Imlay, J. A. (2015). The Escherichia coli small Protein MntS and exporter MntP optimize the intracellular concentration of manganese. PLoS Genet. 11:e1004977. doi: 10.1371/journal.pgen.1004977
Mattes, A., Gould, D., Taupp, M., and Glasauer, S. (2013). A novel autotrophic bacterium isolated from an engineered wetland system links nitrate-coupled iron oxidation to the removal of As, Zn and S. Water Air Soil Pollut. 224:1490.
Megonigal, J. P., Hines, M. E., and Visscher, P. T. (2004). ““Anaerobic metabolism: linkages to trace gases and aerobic processes”,” in Biogeochemistry, ed. W. H. Schlesinger (Oxford: Elsevier-Pergamon), 317–424.
Melton, E. D., Swanner, E. D., Behrens, S., Schmidt, C., and Kappler, A. (2014). The interplay of microbially mediated and abiotic reaction in the biogeochemical Fe cycle. Nat. Rev. Microbiol. 12, 797–808. doi: 10.1038/nrmicro3347
Michiels, C. C., Darchambeau, F., Roland, F. A. E., Morana, C., Llirós, M., Garcia-Armisen, T., et al. (2017). Iron-dependent nitrogen cycling in a ferruginous lake and the nutrient status of Proterozoic oceans. Nat. Geosci. 10, 217–222.
Mirzoyan, N., and Schreier, H. (2014). Effect of sulfide on growth of marine bacteria. Arch. Microbiol. 196, 279–287. doi: 10.1007/s00203-014-0968-0
Muehe, E. M., Gerhardt, S., Schink, B., and Kappler, A. (2009). Ecophysiology and the energetic benefit of mixotrophic Fe(II) oxidation by various strains of nitrate-reducing bacteria. FEMS Microbiol. Ecol. 70, 335–343. doi: 10.1111/j.1574-6941.2009.00755.x
Murphy, A. E., Bulseco, A., Ackerman, R., Vineis, J., and Bowen, J. L. (2020). Sulfide addition favors respiratory ammonification (DNRA) over complete denitrification and alters the active microbial community in salt marsh sediments. Environ. Microbiol. doi: 10.1111/1462-2920.14969
Nielsen, J. L., and Nielsen, P. H. (1998). Microbial nitrate-dependent oxidation of ferrous iron in activated sludge. Environ. Sci. Technol. 32, 3556–3561.
Nielsen, L. P. (1992). Denitrification in sediment determined from nitrogen isotope pairing. FEMS Microbiol. Ecol. 86, 357–362.
Nizzoli, D., Carraro, E., Nigro, V., and Viaroli, P. (2010). Effect of organic enrichment and thermal regime on denitrification and dissimilatory nitrate reduction to ammonium (DNRA) in hypolimnetic sediments of two lowland lakes. Water Res. 44, 2715–2724. doi: 10.1016/j.watres.2010.02.002
Nordhoff, M., Tominski, C., Halama, M., Byrne, J. M., Obst, M., Kleindienst, S., et al. (2017). Insights into nitrate-reducing Fe(II) oxidation mechanisms through analysis of cell-mineral associations, cell encrustation, and mineralogy in the chemolithoautotrophic enrichment culture KS. Appl. Environ. Microbiol. 83:e00752-17. doi: 10.1128/AEM.00752-17
Otte, S., Kuenen, G., Nielsen, L. P., Paerl, H. W., Zopfi, J., Schulz, H. N., et al. (1999). Nitrogen, carbon, and sulfur metabolism in natural Thioploca samples. Appl. Environ. Microbiol. 65, 3148–3157.
Palacin-Lizarbe, C., Camarero, L., Hallin, S., Jones, C. M., Cáliz, J., Casamayor, E. O., et al. (2019). The DNRA-denitrification dichotomy differentiates nitrogen transformation pathways in mountain lake benthic habitats. Front. Microbiol. 10:1229. doi: 10.3389/fmicb.2019.01229
Plummer, P., Tobias, C., and Cady, D. (2015). Nitrogen reduction pathways in estuarine sediments: influences of organic carbon and sulfide. J. Geophys. Res. Biogeosci. 120, 1958–1972.
Poulain, A. J., and Newman, D. K. (2009). Rhodobacter capsulatus catalyzes light-dependent Fe(II) oxidation under aerobic conditions as a potential detoxification mechanism. Appl. Environ. Microbiol. 75, 6639–6646.
Ratering, S., and Schnell, S. (2001). Nitrate-dependent iron(II) oxidation in paddy soil. Environ. Microbiol. 3, 100–109.
Risgaard-Petersen, N., Rysgaard, S., and Revsbech, N. P. (1995). Combined microdiffusion-hypobromite oxidation method for determining nitrogen-15 isotope in ammonium. Soil Sci. Soc. Am. J. 59, 1077–1080.
Roberts, K. L., Kessler, A. J., Grace, M. R., and Cook, P. L. M. (2014). Increased rates of dissimilatory nitrate reduction to ammonium (DNRA) under oxic conditions in a periodically hypoxic estuary. Geochim. Cosmochim. Acta 133, 313–324.
Robertson, E. K., Bartoli, M., Brüchert, V., Dalsgaard, T., Hall, P. O. J., Hellemann, D., et al. (2019). Application of the isotope pairing technique in sediments: use, challenges, and new directions. Limnol. Oceanogr. Methods 17, 112–136.
Robertson, E. K., Roberts, K. L., Burdorf, L. D. W., Cook, P., and Thamdrup, B. (2016). Dissimilatory nitrate reduction to ammonium coupled to Fe(II) oxidation in sediments of a periodically hypoxic estuary. Limnol. Oceanogr. 61, 365–381.
Robertson, E. K., and Thamdrup, B. (2017). The fate of nitrogen is linked to iron(II) availability in a freshwater lake sediment. Geochim. Cosmochim. Acta 205, 84–99.
Sayama, M., Risgaard-Petersen, N., Nielsen, L., Fossing, H., and Christensen, P. B. (2005). Impact of bacterial NO3- transport on sediment biogeochemistry. Appl. Environ. Microbiol. 71, 7575–7577.
Schippers, A., Neretin, L. N., Lavik, G., Leipe, T., and Pollehne, F. (2005). Manganese(II) oxidation driven by lateral oxygen intrusions in the western Black Sea. Geochim. Cosmochim. Acta 69, 2241–2252.
Schubert, C. J., Durisch-Kaiser, E., Wehrli, B., Thamdrup, B., Lam, P., and Kuypers, M. M. (2006). Anaerobic ammonium oxidation in a tropical freshwater system (Lake Tanganyika). Environ. Microbiol. 8, 1857–1863.
Schulz, H. D., Dahmke, A., Schinzel, U., Wallmann, K., and Zabel, M. (1994). Early diagenetic processes, fluxes, and reaction rates in sediments of the South Atlantic. Geochim. Cosmochim. Acta 58, 2041–2060.
Schulz, H. N., Brinkhoff, T., Ferdelman, T. G., Hernández-Mariné, M., Teske, A., and Jørgensen, B. B. (1999). Dense populations of a giant sulfur bacterium in Namibian shelf sediments. Science 284, 493–495.
Senga, Y., Mochida, K., Fukumori, R., Okamoto, N., and Seike, Y. (2006). N2O accumulation in estuarine and coastal sediments: the influence of H2S on dissimilatory nitrate reduction. Estuar. Coast. Shelf Sci. 67, 231–238.
Sher, Y., Schneider, K., Schwermer, C. U., and Van Rijn, J. (2008). Sulfide-induced nitrate reduction in the sludge of an anaerobic digester of a zero-discharge recirculating mariculture system. Water Res. 42, 4386–4392. doi: 10.1016/j.watres.2008.07.031
Silver, S., and Clark, D. (1971). Magnesium transport in Escherichia coli. J. Biol. Chem. 246, 569–576.
Smith, E. J., Davison, W., and Hamilton-Taylor, J. (2002). Methods for preparing synthetic freshwaters. Water Res. 36, 1286–1296.
Sørensen, J., Tiedje, J. M., and Firestone, R. B. (1980). Inhibition by sulfide of nitric and nitrous oxide reduction by denitrifying Pseudomonas fluorescens. Appl. Environ. Microbiol. 39, 105–108.
Stookey, L. L. (1970). Ferrozine – A new spectrophotometric reagent for iron. Anal. Chem. 42, 779–781.
Straub, K. L., Benz, M., Schink, B., and Widdel, F. (1996). Anaerobic, nitrate-dependent microbial oxidation of ferrous iron. Appl. Environ. Microbiol. 62, 1458–1460.
Sublette, K. L., and Sylvester, N. D. (1987). Oxidation of hydrogen sulfide by continuous cultures of Thiobacillus denitrificans. Biotechnol. Bioeng. 29, 753–758.
Takai, K., Suzuki, M., Nakagawa, S., Miyazaki, M., Suzuki, Y., Inagaki, F., et al. (2006). Sulfurimonas paralvinellae sp. nov., a novel mesophilic, hydrogen- and sulfur-oxidizing chemolithoautotroph within the Epsilonproteobacteria isolated from a deep-sea hydrothermal vent polychaete nest, reclassification of Thiomicrospira denitrificans as Sulfurimonas denitrificans comb. nov. and emended description of the genus Sulfurimonas. Int. J. Syst. Evol. Microbiol. 56, 1725–1733. doi: 10.1099/ijs.0.64255-0
Thamdrup, B., and Dalsgaard, T. (2002). Production of N2 through anaerobic ammonium oxidation coupled to nitrate reduction in marine sediments. Appl. Environ. Microbiol. 68, 1312–1318.
Tiedje, J. M. (1988). “Ecology of denitrification and dissimilatory nitrate reduction to ammonium,” in Environmental Microbiology of Anaerobes, ed. A. J. B. Zehnder (New York, NY: John Wiley and Sons), 179–244.
van den Berg, E. M., Boleij, M., Kuenen, J. G., Kleerebezem, R., and Van Loosdrecht, M. C. M. (2016). DNRA and denitrification coexist over a broad rand of acetate/N-NO3- ratios, in a chemostat enrichment culture. Front. Microbiol. 7:1842. doi: 10.3389/fmicb.2016.01842
van den Berg, E. M., van Dongen, U., Abbas, B., and van Loosdrecht, M. C. M. (2015). Enrichment of DNRA bacteria in a continuous culture. ISME J. 9, 2153–2161.
Weber, K. A., Achenbach, L. A., and Coates, J. D. (2006a). Microbes pumping iron: anaerobic microbial iron oxidation and reduction. Nat. Rev. Microbiol. 4, 752–764.
Weber, K. A., Pollock, J., Cole, K. A., O’Connor, S. M., Achenbach, L. A., and Coates, J. A. (2006b). Anaerobic nitrate-dependent iron(II) bio-oxidation by a novel lithoautotrophic betaproteobacterium, Strain 2002. Appl. Environ. Microbiol. 72, 686–694.
Wenk, C. B., Blees, J., Zopfi, J., Veronesi, M., Bourbonnais, A., Schubert, C. J., et al. (2013). Anaerobic ammonium oxidation (anammox) bacteria and sulfide-dependent denitrifiers coexist in the water column of a meromictic south-alpine lake. Limnol. Oceanogr. 58, 1–12.
Wenk, C. B., Zopfi, J., Gardner, W. S., McCarthy, M. J., Niemann, H., Veronesi, M., et al. (2014). Partitioning between benthic and pelagic nitrate reduction in the Lake Lugano south basin. Limnol. Oceanogr. 59, 1421–1433.
Wu, G., Wan, F., Fu, H., Li, N., and Gao, H. (2015). A matter of timing: contrasting effects of hydrogen sulfide on oxidative stress response in Shewanella oneidensis. J. Bacteriol. 197, 3563–3572. doi: 10.1128/JB.00603-15
Yan, R., Kappler, A., Muehe, E. M., Knorr, K.-H., Horn, M. A., Poser, A., et al. (2018). Effect of reduced sulfur species on chemolithoautotrophic pyrite oxidation with nitrate. Geomicrobiol. J. 36, 19–29. doi: 10.1080/01490451.2018.1489915
Yoon, S., Cruz-Garcia, C., Sanford, R., Ritalahti, K. M., and Löffler, F. E. (2015). Denitification versus respiratory ammonification: environmental controls of two competing dissimilatory NO3-/NO2- reduction pathways in Shewanella loihica strain PV-4. ISME J. 9, 1093–1104. doi: 10.1038/ismej.2014.201
Zeinert, R., Martinez, E., Schmitz, J., Senn, K., Usman, B., Anantharaman, V., et al. (2018). Structure-function analysis of manganese exporter proteins across bacteria. J. Biol. Chem. 293, 5715–5730. doi: 10.1074/jbc.M117.790717
Zobrist, J., and Reichert, P. (2006). Bayesian estimation of export coefficients from diffuse and point sources in Swiss watersheds. J. Hydrol. 329, 207–223.
Zobrist, J., Schoenenberger, U., Figura, S., and Hug, S. (2018). Long-term trends in Swiss rivers sampled continuously over 39 years reflect changes in geochemical processes and pollution. Environ. Sci. Pollut. Res. 25, 16788–16809. doi: 10.1007/s11356-018-1679-x
Zopfi, J., Ferdelman, T. G., and Fossing, H. (2004). “Distribution and fate of sulfur intermediates – sulfite, tetrathionate, thiosulfate, and elemental sulfur - in marine sediments,” in Sulfur Biogeochemistry – Past and Present, eds J. P. Amend, K. J. Edwards, and T. W. Lyons (Boulder, CO: Geological Society of America), 97–116.
Keywords: NO3– reduction, N-processes partitioning, denitrification, DNRA, inorganic electron donors, lake sediments, eutrophic lake
Citation: Cojean ANY, Lehmann MF, Robertson EK, Thamdrup B and Zopfi J (2020) Controls of H2S, Fe2 +, and Mn2 + on Microbial NO3–-Reducing Processes in Sediments of an Eutrophic Lake. Front. Microbiol. 11:1158. doi: 10.3389/fmicb.2020.01158
Received: 20 March 2020; Accepted: 06 May 2020;
Published: 16 June 2020.
Edited by:
Dennis A. Bazylinski, University of Nevada, Las Vegas, United StatesReviewed by:
Ronald Oremland, United States Geological Survey (USGS), United StatesMeng Zhang, Nanyang Technological University, Singapore
Copyright © 2020 Cojean, Lehmann, Robertson, Thamdrup and Zopfi. This is an open-access article distributed under the terms of the Creative Commons Attribution License (CC BY). The use, distribution or reproduction in other forums is permitted, provided the original author(s) and the copyright owner(s) are credited and that the original publication in this journal is cited, in accordance with accepted academic practice. No use, distribution or reproduction is permitted which does not comply with these terms.
*Correspondence: Adeline N. Y. Cojean, YWRlbGluZS5jb2plYW5AdW5pbmUuY2g=
†Present address: Adeline N. Y. Cojean, Centre for Hydrogeology and Geothermics, University of Neuchâtel, Neuchâtel, Switzerland