- 1Department of Plant and Microbial Biology, University of Zurich, Zurich, Switzerland
- 2Polyphor AG, Allschwil, Switzerland
- 3Department of Chemistry, University of Zurich, Zurich, Switzerland
Pseudomonas aeruginosa is an opportunistic human pathogen and a leading cause of nosocomial infections. Due to its high intrinsic and adaptive resistance to antibiotics, infections caused by this organism are difficult to treat and new therapeutic options are urgently needed. Novel peptidomimetic antibiotics that target outer membrane (OM) proteins have shown great promise for the treatment of P. aeruginosa infections. Here, we have performed genome-wide mutant fitness profiling using transposon sequencing (Tn-Seq) to identify resistance determinants against the recently described peptidomimetics L27-11, compounds 3 and 4, as well as polymyxin B2 (PMB) and colistin (COL). We identified a set of 13 core genes that affected resistance to all tested antibiotics, many of which encode enzymes involved in the modification of the lipopolysaccharide (LPS) or control their expression. We also identified fitness determinants that are specific for antibiotics with similar structures that may indicate differences in their modes of action. These results provide new insights into resistance mechanisms against these peptide antibiotics, which will be important for future clinical development and efforts to further improve their potency.
Introduction
Antimicrobial resistance is a global threat for the effective treatment of infections and is a major cause of mortality (Prestinaci et al., 2015). The rapid spread of antimicrobial resistance in bacteria is largely driven by antimicrobial exposure, often in connection with the misuse of antimicrobial compounds in health care and animal husbandry (Baquero et al., 1998; Chantziaras et al., 2014; Holmes et al., 2016). To restrict the spread of antimicrobial resistance and increased mortality rate, the World Health Organization developed a framework of regulations early in the 21st century (WHO, 2001). Yet the emergence of multidrug resistant pathogenic bacteria, including the so-called ESKAPE pathogens (Enterococcus faecium, Staphylococcus aureus, Klebsiella pneumoniae, Acinetobacter baumannii, Pseudomonas aeruginosa, and Enterobacter species) is particularly alarming (Rice, 2008). Among the ESKAPE pathogens, the Gram-negative bacterium P. aeruginosa is one of the leading sources of nosocomial infections associated with morbidity and mortality (Kang et al., 2003). P. aeruginosa is a facultative anaerobic Gram-negative bacterium, which is able to perform denitrification in the presence of nitrate or nitrite (Filiatrault et al., 2005; Williams et al., 2006; Palmer et al., 2007) and pyruvate/arginine fermentation when nitrate is absent (Eschbach et al., 2004; Schreiber et al., 2006). Due to its enormous metabolic versatility, this bacterium can adapt to a wide range of environments (Frimmersdorf et al., 2010).
The extensive use and misuse of antibiotics belonging to the polymyxin (POL) family [polymyxin B2 (PMB) and colistin (COL)], which constitutes the last therapeutic option for the treatment of some Gram-negative multidrug resistant infections, has led to the development of resistance to these antibiotics in different microorganisms (Rice, 2008; Carattoli et al., 2017; Xiong et al., 2017; Zhang et al., 2017). POLs are cationic peptides with a high affinity for negatively charged molecules such as the lipopolysaccharides (LPS) of the outer membrane (OM) of Gram-negative bacteria (Rabanal and Cajal, 2017). After binding to the LPS, the POLs displace the bridging divalent cations and initiate a process leading to permeabilization of the OM (Rabanal and Cajal, 2017). The lethal action of POLs is thought to be the result of the depolarizing effect on the cytoplasmic membrane (CM), but alternative mechanisms such as oxidative stress or interaction with OM proteins have also been suggested (Storm et al., 1977; van der Meijden and Robinson, 2015; Yu et al., 2015).
Polymyxin resistance is often associated with modifications to the LPS. In P. aeruginosa the two-component systems PmrAB, PhoPQ, ColRS, ParRS, and CprRS control expression of the LPS modification operon arnBCADTEF, responsible for the addition of 4-amino-4-deoxy-L-arabinose (L-Ara4N) to lipid A (McPhee et al., 2003; Olaitan et al., 2014). In addition to LPS modification, the multidrug efflux system MexXY–OprM was demonstrated to increase the fitness of the organism in the presence of POLs (Baron et al., 2016; Puja et al., 2020). Previous work has shown that a low concentration of magnesium (Mg2+), which is sensed via the PhoPQ and PmrAB systems (McPhee et al., 2006), induces expression of the arn operon and consequently leads to POL resistance (Brown and Melling, 1969; Brown and Watkins, 1970; Groisman et al., 1997). Mg2+ limitation not only triggers LPS modification but also induces quorum sensing, biofilm formation and the production of the virulence factors phenazine and pyochelin (Guina et al., 2003; Mulcahy and Lewenza, 2011).
Novel cationic peptidomimetic antibiotics have recently been reported (Robinson, 2013) that show potent and selective action against Pseudomonas spp., including the opportunistic pathogen P. aeruginosa (Srinivas et al., 2010; Werneburg et al., 2012; Schmidt et al., 2013; Urfer et al., 2016). These antimicrobial compounds include L27-11 and the closely related clinical candidate murepavadin (also known as POL7080; Wach et al., 2018), which has a very low minimum inhibitory concentration (MIC90 2 μg/mL) against P. aeruginosa (Ekkelenkamp et al., 2020). These compounds target the OM protein LptD, which is required for the transport of LPS to the OM (Srinivas et al., 2010; Werneburg et al., 2012; Schmidt et al., 2013). A more recently described family of chimeric peptides (referred to as chimeras), including compounds 3 and 4, show potent bactericidal activity against all the Gram-negative ESKAPE pathogens E. coli, P. aeruginosa, A. baumanii, and K. pneumoniae. These compounds bind to both LPS and the main component (BamA) of the β-barrel folding complex (BAM; Figures 1A,B) required for the folding and insertion of β-barrel proteins into the OM of Gram-negative bacteria (Luther et al., 2019). At present, these antimicrobial peptides are in preclinical development, however, knowledge about the mechanisms of resistance against these new classes of peptidomimetic antibiotics is scarce.
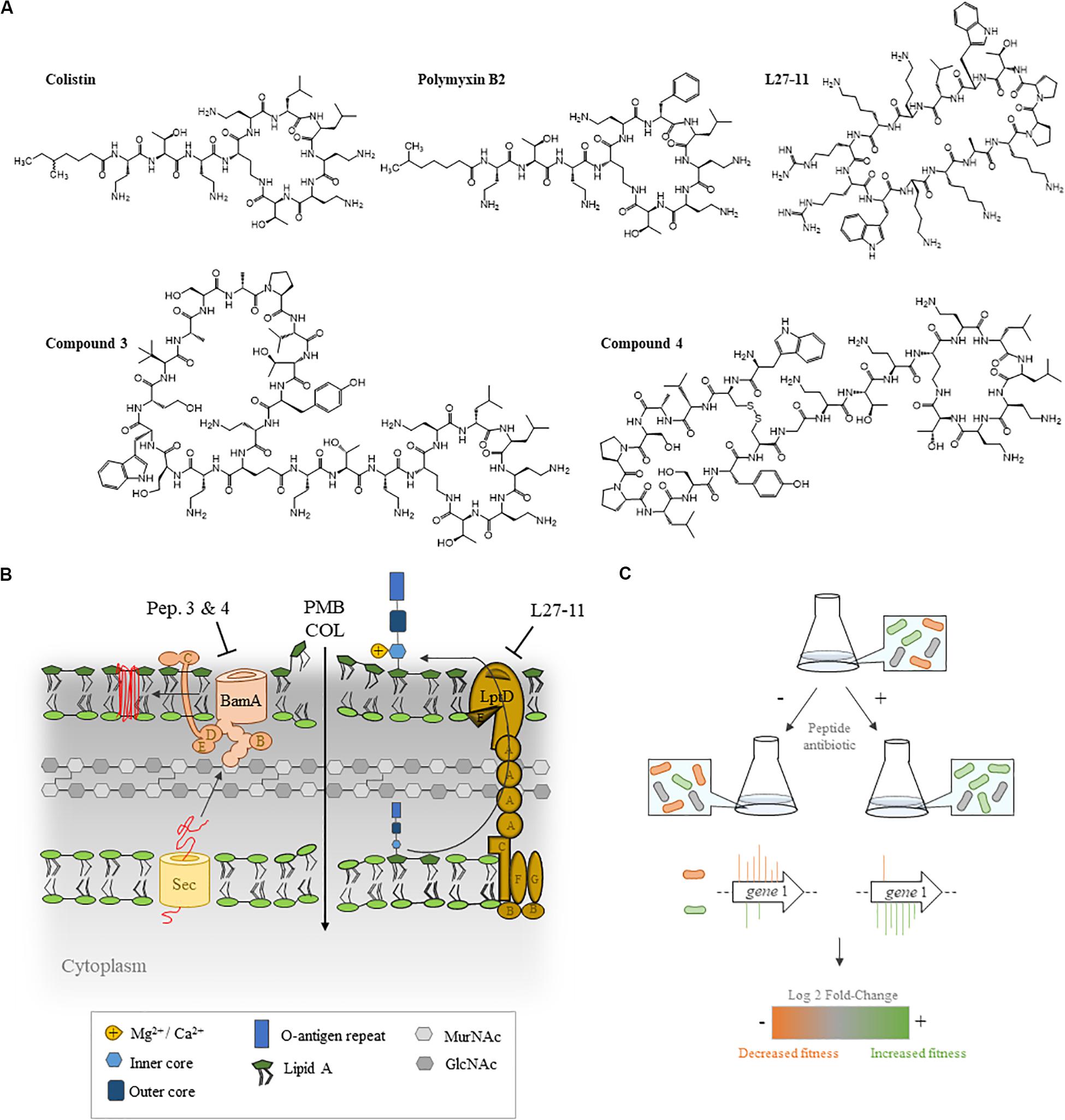
Figure 1. Experimental set-up of the transposon sequencing (Tn-Seq) analysis. (A) Structures of the five antibiotics employed in this study (B) and their cellular targets. Polymyxins (POLs) permeabilize the OM, leading to cytoplasmic membrane (CM) depolarization and cell lysis. Chimeras 3 and 4 bind to both lipopolysaccharide (LPS) and BamA, the main component of the β-barrel assembly machinery complex. L27-11 targets the OM protein LptD, which is involved in the transport of LPS to the cell surface. (C) A pool of P. aeruginosa UCBPP-PA14 transposon (Tn) insertion mutants was grown in the absence or presence of antibiotics and the number of Tn insertion sites were determined by Tn-Seq.
Several methods have been employed to identify intrinsic and adaptive resistance factors, including the isolation and characterization of resistant mutants (Fernández et al., 2013; Gutu et al., 2013) and the genome-wide analysis of gene expression of bacteria grown in the presence of sub-minimal inhibitory concentrations (sub-MICs) of the antibiotics (Cheah et al., 2016; Khaledi et al., 2016). The recently developed high-throughput transposon sequencing (Tn-Seq) technique has been demonstrated to be an alternative method to gain mechanistic insights into multidrug resistance mechanisms (Gallagher et al., 2011; Murray et al., 2015). Tn-Seq involves creating a saturated transposon (Tn) library of mutants, next-generation sequencing of the Tn insertion, and mapping the insertion sites to a reference genome. This technique has been widely used to identify genes that provide a fitness benefit for growth under a particular condition, as mutants with a growth defect would be depleted compared to a respective control culture (Murray et al., 2015; Solaimanpour et al., 2015; Rajagopal et al., 2016; Santiago et al., 2018).
In this study, we constructed a highly saturated Tn insertion library in the model organism P. aeruginosa University of California Berkeley Plant Pathology (UCBPP)-PA14 and used Tn-Seq to identify genes that provide a fitness benefit for growth in the presence of sub-MICs of PMB, COL and the novel peptidomimetic antibiotics L27-11, 3, and 4 (Figure 1C). Our data show that LPS modification influences susceptibility to all the antibiotics tested. However, we also identified distinct resistance determinants for each of the antibiotics, indicating differences in the mechanisms of resistance. Finally, we demonstrate that the production of spermidine promotes resistance against POLs and L27-11.
Results
Construction and Growth of a P. aeruginosa UCBPP-PA14 Transposon Library in the Presence of Sub-Lethal Concentrations of Different Peptide Antibiotics
A modified version of the suicide plasmid pLG99 (pLG99-Gm), which carries Tn23 (Gallagher et al., 2013), was used to generate more than 500,000 insertion mutants in P. aeruginosa UCBPP-PA14. The Tn5 derivative Tn23 inserts randomly in the genome (Gallagher et al., 2013). In order to challenge the Tn mutants with the antibiotics, we first determined the MICs of PMB, COL and the peptidomimetic antibiotics L27-11, 4 and 3 in P. aeruginosa UCBPP-PA14 grown in basal medium 2 (BM2) supplemented with either high (2 mM) or low (20 μM) Mg2+ (Table 1). Previous work has shown that under Mg2+-limiting conditions P. aeruginosa induces expression of the arn gene cluster, which encodes enzymes that add an L-Ara4N moiety to the phosphate group of lipid A, thereby conferring resistance to POLs and other cationic antimicrobial peptides (McPhee et al., 2003, 2006). Accordingly, we determined that the MICs of PMB and COL for cells grown with 20 μM Mg2+ are at least 100-fold higher compared to cells grown in the presence of high Mg2+ (2 mM). In contrast to these POLs, we observed only a minor twofold change in susceptibility of P. aeruginosa UCBPP-PA14 to the peptidomimetic antibiotics 3 and 4 when grown with 20 μM Mg2+. The MIC for L27-11 was found to be approximately five times lower under limiting Mg2+ (20 μM) than under replete Mg2+ (2 mM) conditions. For the Tn-Seq experiments, we grew the Tn library of mutants in BM2 containing 20 μM Mg2+ for approximately 12 generations in the presence of sub-MICs of the different antibiotics that slowed down growth by 30% compared to the untreated control. The low Mg2+ concentration of the medium was used to induce resistance to the antimicrobial peptides (Table 1).
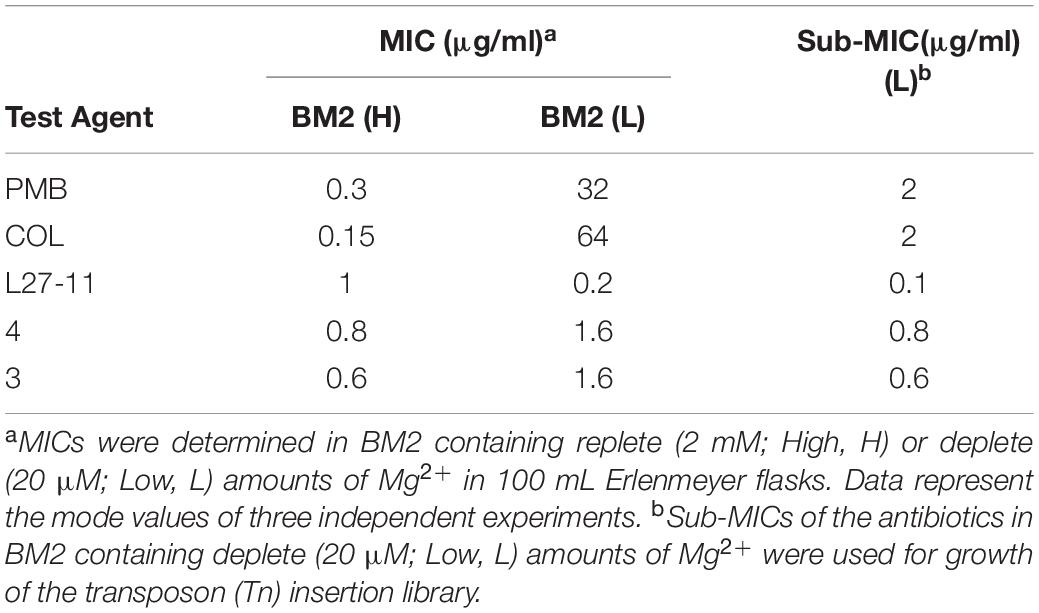
Table 1. Minimum inhibitory concentrations (MICs) of polymyxin B2 (PMB), colistin (COL), L27-11, 3 and 4 in basal medium 2 (BM2) in Pseudomonas aeruginosa UCBPP-PA14 wild-type.
Identification of Fitness Determinants Involved in Susceptibility to Polymyxins, L27-11, and Peptides 3 and 4
After extraction of genomic DNA from the harvested Tn pools of each treatment, the Tn-Seq circle method (Gallagher et al., 2013) was employed to estimate the number of Tn insertion sites. Sequencing of the pools from the PMB and COL treatments resulted in 10.1 and 9.3 million reads, respectively, while the pools of the treatments with peptidomimetic antibiotics and the untreated controls resulted in 5.0–10.6 million reads (Supplementary Table S1). For all the samples, we were able to map more than 97% of the reads to the P. aeruginosa UCBPP-PA14 reference genome (Winsor et al., 2016). The number of unique insertion sites per gene was determined (Solaimanpour et al., 2015) and the insertion frequency of the Tn was calculated by dividing the size of the P. aeruginosa UCBPP-PA14 genome (6.5 Mbp) by the total number of unique insertion sites. We estimated that the Tn insertion sites are on average spaced by 14 bp in non-essential genes.
We next calculated the unique insertion density (UID) of each gene (Solaimanpour et al., 2015), which is the number of unique Tn insertions divided by the length of the gene. The UIDs were further normalized by the total number of unique insertions in each sample (normalized UIDs). The genes providing a fitness benefit for each of the treatments were determined by selecting a log2-fold change (based on normalized UIDs) of at least -1.0 between untreated and treated samples, as detailed in the section “Materials and Methods.” Figure 2 shows the log2-fold change of normalized UIDs of PMB-treated versus untreated samples plotted against the average normalized UIDs under both conditions. With the criteria employed, our analysis identified 58 fitness determinants following treatment with PMB, 42 for COL, 47 for L27-11, 75 for compound 4, and 43 for compound 3 (Supplementary Tables S2–S7). These genes were assigned to functional categories according to clusters of orthologous groups (Huerta-Cepas et al., 2017; Supplementary Figure S1).
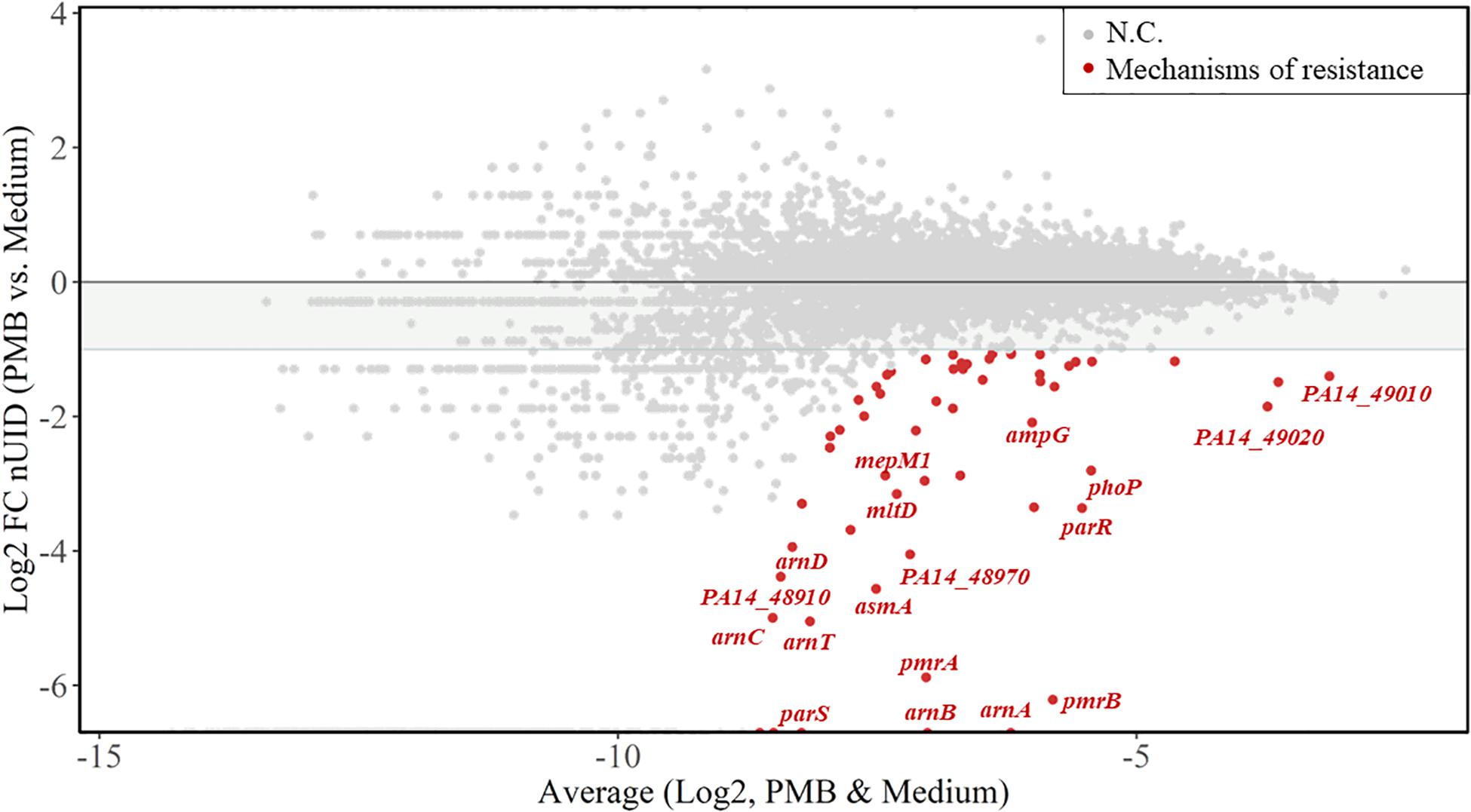
Figure 2. Resistance fitness determinants for polymyxin B2 (PMB). The MA-plot (M for log ratio and A for mean average) shows the average of the normalized unique insertion density (nUID) between the PMB-treated sample and the untreated sample (log2) on the x-axis and the fold change of the nUID between the two samples (log2) on the y-axis. The 58 genetic determinants likely involved in bacterial fitness upon PMB exposure are highlighted in red and genes of interest are named. NC, no change.
Polymyxins and Peptidomimetics Share Mechanisms of Resistance
Our Tn-Seq analysis identified 13 genes that had an impact on susceptibility to all peptide antibiotics tested (Figure 3A and Table 2). Many of these genes have previously been shown to affect resistance to PMB or COL, including five genes of the arn gene cluster (PA14_18330 to PA14_18370), which directs the addition of L-Ara4N to lipid A. We also identified several genes of the regulatory systems that are known to be involved in the regulation of the arn gene cluster (Jeannot et al., 2017). Among these genes were the two-component systems pmrAB (PA14_63150 and PA14_63160), parRS (PA14_41260 and PA14_41270; except for treatment with peptide 3) and the response regulatory gene phoP of the PhoPQ system (PA14_49170 and PA14_49180). Another response regulatory gene that affects expression of the arn cluster, colR of the ColSR system (PA14_56940 and PA14_56950), showed a reduced number of Tn insertions only for the PMB treatment, while higher UIDs were observed for treatments with peptides 3 and 4 (Log2-fold change -1.0 versus + 1.29/1.15). In addition to the genes involved in LPS modification, PA14_58090 encoding a hypothetical protein and two genes coding for bacteriophage Pf1 proteins (PA14_48970 and PA14_49000; Kneale, 1983; Davis et al., 1995) provided a fitness benefit for all tested peptide antibiotics (Table 2). An involvement of the Pf1 phage in enhanced resistance to peptide antibiotics is further supported by the finding that various other Pf1 genes (PA14_48900, PA14_48910, PA14_48940, PA14_48980, PA14_48990, PA14_49010, and PA14_49020) were identified upon treatment with the different antibiotics (Supplementary Table S7). It has been demonstrated that the filamentous phages produced by P. aeruginosa cause the biofilm matrix to self-assemble into a liquid crystal that provides tolerance to aminoglycoside antibiotics (Secor et al., 2015). In the list of the 13 core genes, we also identified PA14_42920 coding for the protein HsiJ2, which is predicted to be the basal plate component (TssK-2) of the second type VI secretion system (H2-T6SS; Lesic et al., 2009; Sana et al., 2012).
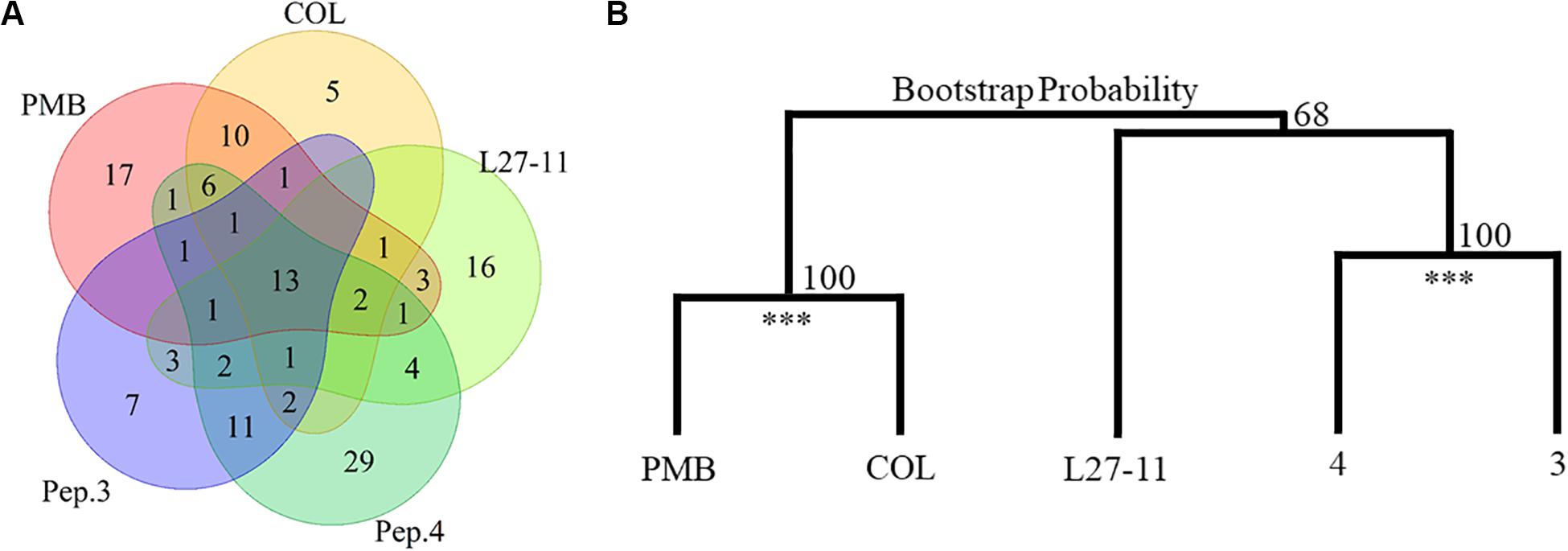
Figure 3. Number and clustering of fitness determinants. (A) The identified resistance determinants for the investigated antibiotics substantially overlap. Thirteen genes impact susceptibility to all tested antibiotics and besides these genes, PMB and colistin (COL) share 21 fitness determinants and peptides (Pep.) 3 and 4 have 19 common genetic determinants. (B) Cluster dendrogram of the Tn-Seq generated in response to PMB, COL, L27-11, peptides 3 and 4 treatments using the Ward criterion and the Euclidean distance. Peptides PMB/COL and 4/3 were clustered in 100% of the 100,000 iterations (Bootstrap Probability; ***p-value < 0.01).
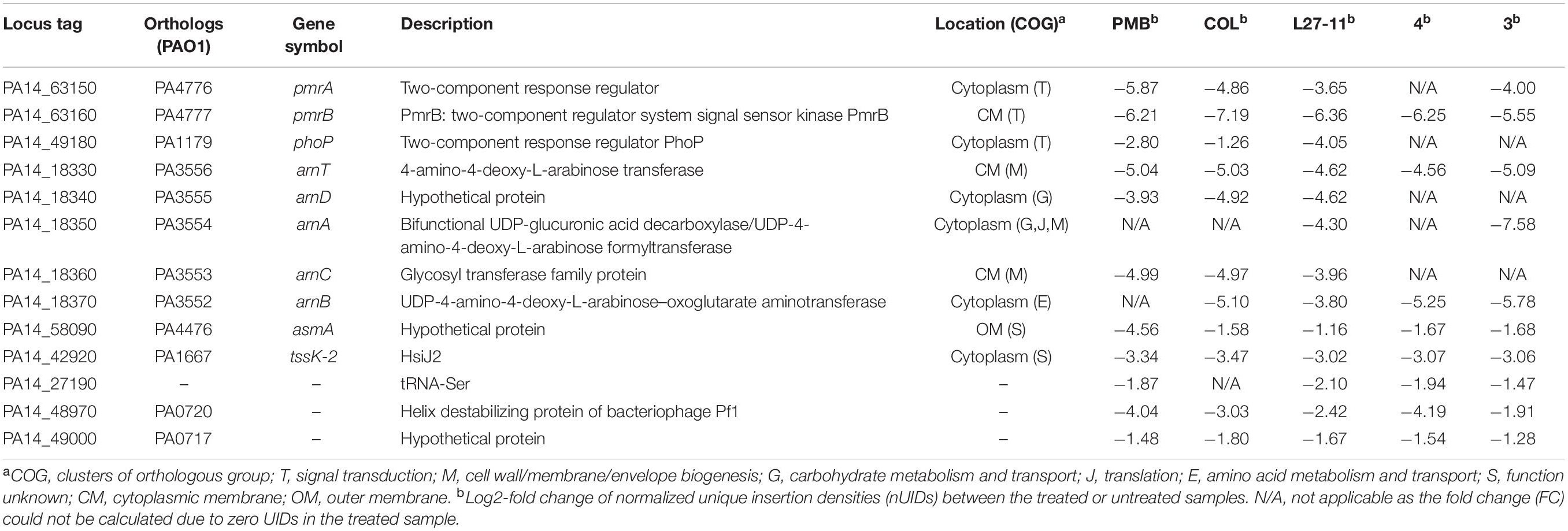
Table 2. Genetic determinants affecting susceptibility to all antimicrobial peptides tested (PMB, COL, L27-11, peptides 3 and 4).
To assess possible cross-resistances, we compared the fitness determinants required for growth in the presence of each of the peptides (Figure 3A). Besides the 13 core genes, the genetic determinants involved in PMB and COL susceptibility, two antibiotics with similar mechanisms of action (Kwa et al., 2007), overlap by 21 genes. Likewise, we observed an overlap of 19 genes between compounds 3 and 4, which target both LPS and BamA (Luther et al., 2019). In contrast, L27-11, which targets the OM protein LptD, showed fewer overlaps with the other antibiotics (9 with the POLs and 14 with peptides 3 and 4). These data suggest that the commonalities and differences between the sets of genes affecting susceptibility to the different antibiotics may be indicative of their diverse modes of action. This observation is further supported by a hierarchical clustering analysis of the Tn-Seq data, which revealed that PMB and COL and peptides 3 and 4 are clustered on two branches, while L27-11 forms a separate branch (Figure 3B).
Resistance Factors Involved in Cell Envelope Biogenesis
A detailed analysis of our Tn-Seq data revealed that among the 21 fitness factors specifically affecting resistance to the two POLs were several genes affecting the structure and composition of the cell envelope (Supplementary Table S7). For example, we identified a gene involved in LPS biosynthesis, PA14_23370, which encodes an uridine diphosphate (UDP)-N-acetylglucosamine 2-epimerase (Burrows et al., 1996; Higgins et al., 2017). In agreement with our data, it has been shown that mutation of the orthologous gene PA3148 in P. aeruginosa PAO1 increased OM permeability for the bovine neutrophil antimicrobial peptide indolicidin (Gooderham et al., 2008). Interestingly, more Tn insertions were observed in PA14_23370 when the mutant library was treated with L27-11, relative to the untreated control, indicating an enrichment of this mutant in the presence of L27-11 (Supplementary Table S7). Among the core fitness genes is PA14_58090 encoding an OM protein with a C-terminus homologous to AsmA, which was suggested to play a role in LPS biosynthesis in E. coli (Deng and Misra, 1996). While the exact function of AsmA is unclear, it has been speculated that AsmA plays a role in OM biogenesis by coordinating the assembly of OM proteins with the biosynthesis of LPS (Martorana et al., 2014). We also identified three genes potentially important for resistance to POLs and chimeras that encode proteins involved in the recycling of peptidoglycan (Sonnabend et al., 2020). The lytic transglycosylase SltB1 (PA14_12080) and the murein endopeptidase MepM1 (PA14_08540), which cleave peptidoglycan cross-connections, and the AmpG permease (PA14_57100), which imports peptidoglycan catabolites into the cytoplasm where they are degraded. To verify a role of AsmA and peptidoglycan turnover in antibiotic susceptibility, we tested respective Tn mutants in BM2 supplemented with 20 μM Mg2+. Mutants with defects in asmA, mepM1, sltB1, and ampG exhibited increased susceptibilities to POLs (2–16 fold). In contrast, with the exceptions of the twofold increased sensitivities of the mepM1 and asmA mutants to the two chimeras and peptide 3, respectively, the mutations did not affect the sensitivity to the peptidomimetics (Table 3).
The Role of LPS Modification in Peptide Antibiotic Susceptibility
To further explore the role of LPS modification in susceptibility, we determined the expression of the arnB and pmrB genes by qPCR in Mg2+ replete (2 mM) BM2 in the presence and absence of sub-MICs of POLs (0.1 μg/ml), L27-11 (0.4 μg/ml), peptides 3 (0.2 μg/ml) and 4 (0.3 μg/ml). The high Mg2+ concentration was chosen to inhibit LPS modification through the PhoPQ and PmrAB systems and to determine whether the antibiotics are capable of inducing LPS modification. Both genes were found to be up-regulated when the medium was supplemented with the antimicrobial peptides (Supplementary Table S8). A six to eightfold induction was observed with peptide 4, while the other antibiotics caused a two to threefold induction of arnB and pmrB expression. These data are in full agreement with previous work showing that various cationic peptides including PMB induce the pmrAB operon (McPhee et al., 2003) and suggest that peptide 4 is particularly efficient in inducing resistance.
We next tested Tn insertion mutants with defects in the biosynthesis or regulation of LPS modification for increased resistance against the peptide antibiotics in BM2 supplemented with 20 μM Mg2+. Strains with an inactivated LPS modification system (arnB) or defects in the regulators controlling expression of the arn gene cluster (pmrB and parR) exhibited markedly increased sensitivities to POLs (4–64 fold) and to a lesser degree (one to eightfold) to L27-11 and peptides 4 and 3 (Table 3). Previous work has shown that the PhoP-PhoQ system is highly expressed under limiting Mg2+ conditions and is involved in increased resistance to PMB via activation of the PmrA-PmrB system (Jeannot et al., 2017). Under Mg2+-limiting growth conditions, a phoQ mutant of P. aeruginosa PAO1 exhibited constitutive PMB resistance whereas a phoP mutant was unaffected, as in P. aeruginosa PhoP is not required for activation of LPS modification in low Mg2+ media (Macfarlane et al., 2000). Both the Tn-Seq data (Table 2) and the susceptibility testing of mutants (Table 3) are fully in line with these reports. Interestingly, the phoP mutant was found to be as susceptible to L27-11 and peptide 3 as the wild-type strain, despite the fact that the Tn-Seq analysis suggested a role of PhoP in increased resistance to these antibiotics. The ParR-ParS system can trigger upregulation of LPS modification in the presence of sub-MIC concentrations of POLs (Fernández et al., 2010). Mutants in parR and parS were shown to be highly sensitive to PMB and COL but not to other antimicrobial peptides (Fernández et al., 2010). Accordingly, we observed that a parR Tn insertion mutant exhibited increased sensitivity for POLs but none of the peptidomimetics (Table 3). Previous work has shown that deletion or disruption of the colRS genes, individually or jointly, abolishes the PMB resistance of a ΔphoQ mutant, but has no significant effect on susceptibility in a wild-type strain (Gutu et al., 2013). In line with these results, we only identified the colR gene in our Tn-Seq analysis, and a colS mutant displayed only a modest twofold increase in sensitivity for POLs and L27-11 (Table 3).
Spermidine Protects Cells Against L27-11
We noticed that mutants in three genes (PA14_63110 to PA14_63130), which are located immediately upstream of pmrAB were underrepresented in our Tn-Seq analysis of the L27-11 treatment (Supplementary Table S4). Gene PA14_63130 was also identified as important for increased resistance under treatment with peptide 4. This gene cluster is involved in the biosynthesis of spermidine, which has been shown to protect bacterial membranes from oxidative stress and antibiotics, including PMB, possibly by competing for cation binding sites (Kwon and Lu, 2007; Johnson et al., 2012). To test the protective effect of spermidine against the peptide antibiotics, we determined the MICs of each antimicrobial compound in the wild type in the presence of spermidine. In agreement with our Tn-Seq analysis, we observed an eightfold increase in the MIC for L27-11 and a protective effect was also seen for PMB (Table 4). To further validate the role of spermidine for resistance to L27-11 and PMB, we tested a PA14_63120 (speE) Tn mutant, which is unable to produce spermidine, for antibiotic susceptibility. The speE mutant exhibited increased susceptibilities to L27-11 and PMB (two to fourfold), confirming that spermidine can protect P. aeruginosa against these antibiotics (Table 3). Our data are in accordance with previous work that has shown that polyamines induce the expression of phoPQ and that the increased resistance to polymyxin B was abolished in a phoP but not in a phoQ mutant, suggesting that the PhoPQ system crosstalks with an unknown polyamine-specific signal transduction pathway in P. aeruginosa (Kwon and Lu, 2006, 2007). By contrast, exogenous spermidine increased the susceptibility of P. aeruginosa to the peptides 3 and 4. This is reminiscent of a previous report that showed that exogenous spermine and spermidine increased the MIC of imipenem against P. aeruginosa (Kwon and Lu, 2006, 2007), likely by blocking the OM porin OprD, which facilitates the penetration of imipenem through the OM (Hancock and Brinkman, 2002).
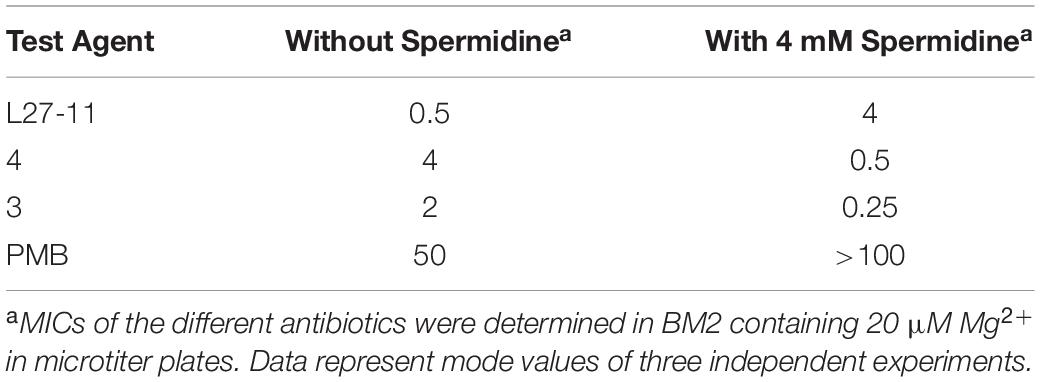
Table 4. Minimum inhibitory concentrations of the peptides (PMB, L27-11, chimeras 3 and 4) in the presence (4 mM) or absence of spermidine in P. aeruginosa UCBPP-PA14 wild-type.
Collectively, these data suggest that the peptidomimetics have specific and complex interactions with the negatively charged cell envelope, perhaps through interactions with OM proteins such as BamA, a known target of these antibiotics (Luther et al., 2019).
PMB Induces Interbacterial Competition
Confirming the results of the Tn-Seq analysis, all tested mutants showed increased sensitivity to the POLs (Table 3), except the tssK-2 mutant, which exhibited wild-type levels of sensitivity to all tested antibiotics (data not shown). Previous studies revealed that the T6SS of P. aeruginosa is activated upon PMB treatment (Ho et al., 2013). In addition, expression of the orthologous gene in P. aeruginosa strain PAO1, PA1667, was shown to be induced by treatment with a sub-MIC of COL (Cummins et al., 2009). When a 1:1 mixture of the wild-type and a tssK-2 Tn mutant was grown in BM2 containing 20 μM Mg2+ in the presence of a sub-lethal concentration of PMB, we observed that the tssK-2 mutant was out-competed (Figure 4). Importantly, this effect was only seen in the presence of PMB. These data suggest that tssK-2 has no direct function in antibiotic susceptibility but that the peptide antibiotics trigger bacterial T6SS-dependent competition. Additional work will be required to unravel the molecular mechanisms of TssK-2-mediated growth antagonism and to investigate whether other components of the second T6SS of P. aeruginosa UCBPP-PA14 are required for the function of TssK-2.
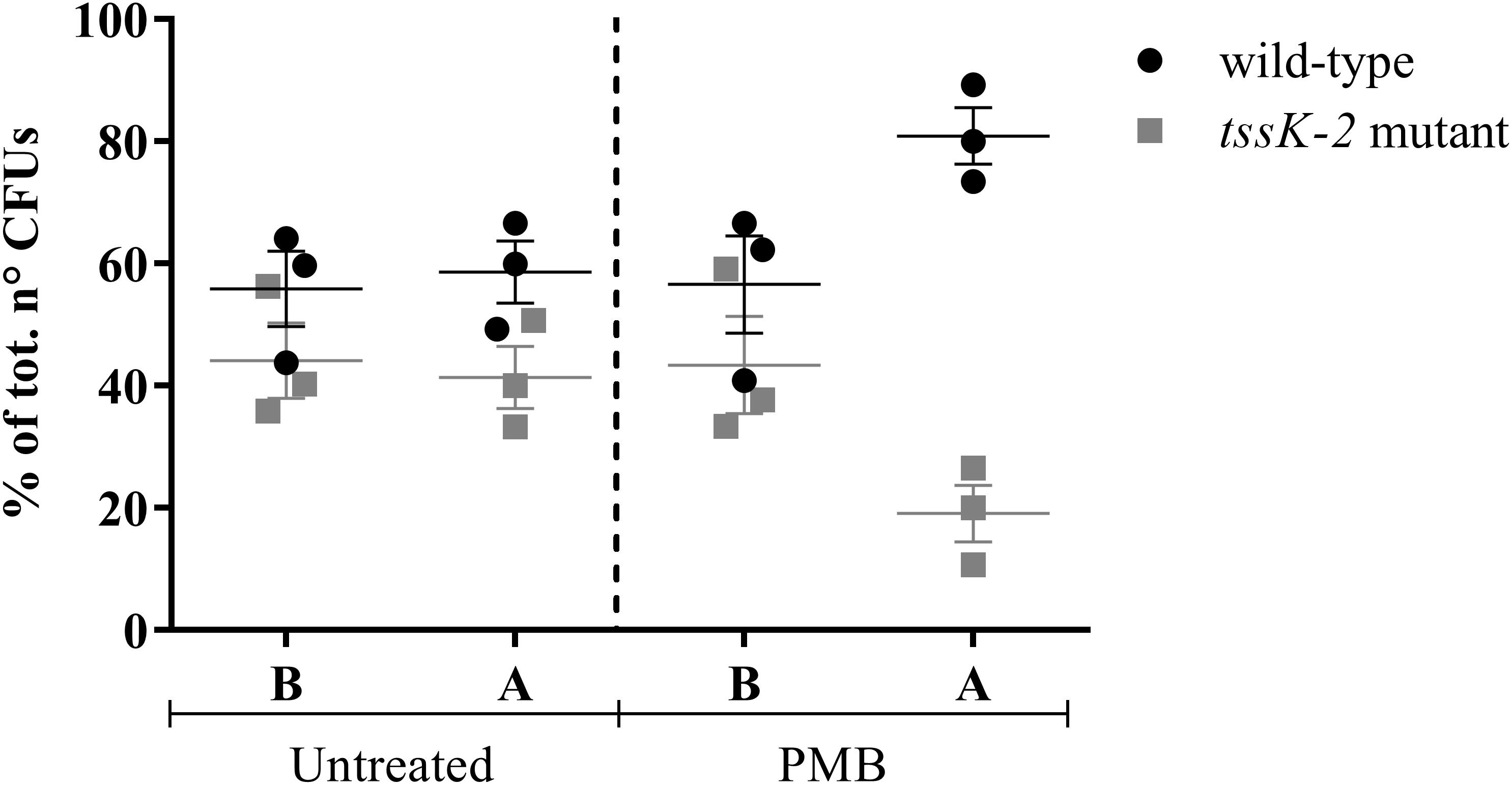
Figure 4. Competition between Pseudomonas aeruginosa UCBPP-PA14 wild-type and the mutant tssK-2. The strains were mixed (1:1; B) and grown until the end of the exponential phase (A) in 20 ml of basal medium 2 (BM2; 20 μM Mg2+) in the presence (2 μg/ml) or absence (untreated) of PMB. Data represent mean ± SEM of three independent experiments.
Discussion
Recent research has identified novel antibiotics that target essential OM proteins (Ghequire et al., 2018; Storek et al., 2018a; Hart et al., 2019; Imai et al., 2019; Luther et al., 2019; Sousa, 2019). While these compounds show great promise for clinical applications, it is unclear how fast resistance against this new class of antibiotic may evolve and what the underlying mechanisms of resistance are. These will be important data for the clinical development of these antibiotics. In the case of the peptidomimetics L27-11 and murepavadin (POL7080), earlier studies linked a greatly increased resistance to both compounds to rare mutations in the target OM protein LptD (Srinivas et al., 2010; Werneburg et al., 2012). It is important to note, however, that our Tn-Seq analysis is unlikely to identify Tn insertions into essential genes, since these are mostly lethal. In addition, the mechanism of action of peptides 3 and 4 involves an interaction with BamA, an essential component of the BAM complex in the OM (Luther et al., 2019). Nevertheless, it is important to enquire whether mutations in other genes can affect the susceptibility of Gram-negative bacteria to these antibiotics. This is of particular importance in clinical development, where significant increases in MIC might influence the outcome of antibiotic treatment.
The aim of this study was, therefore, to identify genetic determinants in P. aeruginosa UCBPP-PA14 that confer a fitness benefit when exposed to the peptidomimetic L27-11, which targets the OM protein LptD (Srinivas et al., 2010; Werneburg et al., 2012) and the chimeras 3 and 4, which bind to BamA (Luther et al., 2019). To allow a detailed comparison, we have also included POLs in this study, which permeabilize the OM and are thought to depolarize the CM and cause cell lysis. Many studies have investigated the mechanisms of resistance to POLs in various Gram-negative bacteria using classical genetic approaches (Macfarlane et al., 2000; Fernández et al., 2010, 2013). More recently, Murray et al. (2015) employed a Tn-Seq approach to identify resistance mechanisms of P. aeruginosa PAO1 against PMB. However, this study only identified a single gene that encodes the hypothetical protein PA14_66150, but none of the well-characterized resistance determinants.
Here we identified a core set of 13 genes that impact upon various levels of susceptibility to all peptide antibiotics tested. Most of these genes encode enzymes for the addition of L-Ara4N to lipid A or are involved in the complex regulation of their expression. However, a more detailed analysis revealed important differences in the role of LPS modification in resistance to the tested peptide antibiotics. In accordance with previous studies (Groisman et al., 1997; McPhee et al., 2006), we observed that under low Mg2+ conditions (20 μM), which induces LPS modification, the MICs of the wild-type strain for PMB and COL is increased more than 100-fold relative to those under replete Mg2+ condition (2 mM; Table 1). In contrast, the MICs for peptides 3 and 4 only increased by a modest 2- and 2.5-fold, respectively, and in the case of compound L27-11 sensitivity was found to be even increased. To further evaluate the role of LPS modification, we determined the MICs of defined arnB (defective in LPS modification) and pmrB (deficient in the regulation of the arn biosynthetic cluster) mutants for the different antibiotics. Both mutants were found to be highly sensitive to PMB and COL (the MIC of the arnB mutant against PMB was decreased 64-fold) relative to the wild-type, whereas the MICs for the peptidomimetics 3, 4 and L27-11 were only decreased two to eightfold (Table 3). However, even the relatively small differences in susceptibility were sufficient to allow selection of mutants in the LPS modification pathway in our Tn-Seq analysis, indicating the high sensitivity of the experimental approach. Our data also support the findings of a recent study, in which strains resistant to POL7080 were isolated and shown to carry a mutation in the pmrB gene (Romano et al., 2019).
Our data demonstrate the important and intriguing trend that L-Ara4N modification of lipid A has only a moderate effect on susceptibility of P. aeruginosa to the peptidomimetics, although this lipid A modification is of major importance for resistance to the POLs in Gram-negative bacteria. This result might be explained by a different mechanism of action for the peptidomimetics, involving a specific binding to OM proteins. Interestingly, a mechanism of action involving an interaction with BamA has been proposed for lectin-like bacteriocins (LlpAs), which have been demonstrated to bind both BamA and LPS via their amino- and carboxy-terminal domains, respectively (Ghequire et al., 2018). In agreement with our results on compounds 3 and 4, the authors have shown that the absence of LPS binding is insufficient to cause full resistance to the LlpAs (Ghequire et al., 2018). In addition, the bactericidal activity of a recently described monoclonal antibody MAB1, which binds an epitope in the external loop L4 on BamA, was found to be linked to OM fluidity, which in turn is affected by LPS structure (Storek et al., 2018b). Hence, LPS modification may not only reduce the attraction of the antibiotics to the OM but could also impact membrane fluidity and thereby affect the antimicrobial activities of the compounds.
It is interesting to note that the three peptidomimetics are active against polymyxin-resistant strains (Sader et al., 2018; Luther et al., 2019), similar to what has been reported for some new generation polymyxins (Velkov et al., 2014). It is thought that these more hydrophobic polymyxin derivatives have increased potency, because they are insensitive to changes of the hydrophobic interior of the OM due to modification of lipid A acylation (Han et al., 2018). However, in the light of recent findings that some antibiotics bind specifically to essential OM proteins (Srinivas et al., 2010; Werneburg et al., 2012; Vetterli et al., 2018), it is possible that the novel polymyxin derivatives, in addition to their membrane-permeabilizing activities, also target membrane proteins. Additional work will be required to investigate this possibility. In addition to general resistance determinants, our Tn-Seq analysis also identified genes that provide a fitness benefit for only certain peptidomimetic antibiotics. This not only increases our understanding of the mode(s) of action but may also open new options for increasing the efficacy of these antibiotics.
Materials and Methods
Bacterial Strains and Growth Conditions
Bacterial strains, plasmids and primers used in this study are listed in Supplementary Table S9. Bacterial strains were routinely grown in lysogeny broth (LB; Miller, 1972) or BM2 (Fernández et al., 2012) supplemented with glucose as the sole carbon source. Media were supplemented with appropriate antibiotics using the following concentrations (in micrograms per milliliter): (i) for Escherichia coli, gentamicin (Gm) 10, kanamycin (Kan) 25, and trimethoprim (Tp) 50; (ii) for P. aeruginosa, Gm 20, nalidixic acid (Nal) 10.
Tn-Seq Methodology
The pLG99 plasmid carries the Tn5 derivative Tn23, which is composed of an outward-facing rhamnose-inducible promoter, a pMB1 replication origin and a lacZ gene, into which the gentamicin cassette has been inserted. To do so, the plasmid used for Tn delivery, pLG99 (Gallagher et al., 2013), was modified as follows: The gentamicin cassette of plasmid pBBR1MCS-5 (Kovach et al., 1995) was PCR amplified using the primers Gm_BclI_Fw and Gm_BclI_Rv. The amplicon was cleaned (QIAquick PCR Purification kit, Cat No./ID:28106), digested with BclI (New England Biolabs, Cat No. R0160S) and ligated into pLG99 cut with the same enzyme.
To construct a Tn library in P. aeruginosa UCBPP-PA14 (Rahme et al., 1995), 50 ml LB cultures of the donor strain E. coli CC118λ-pir/PLG99:Gm, the helper strain E. coli pRK2013 (Phadnis and Berg, 1987) and the recipient P. aeruginosa UCBPP-PA14 were harvested in the stationary phase, washed and resuspended in 6 ml 0.9% NaCl. Two hundred fifty microliters of the donor and the helper strains were mixed and incubated at room temperature for 10 min before 250 μl of the recipient strain was added. Next, 150 μl samples of the mix were inoculated onto 0.45 μM nitrocellulose membrane filters (Merck Millipore, Ref. HAQP02500), which were placed on LB agar plates. Following incubation at 37°C for 24 h, cells of nine filters were resuspended in 6 ml of 0.9% NaCl and 0.5 ml of this suspension was spread on 12 cm–2 square petri dishes containing LB agar supplemented with Gm 20 μg ml–1 and Nal 10 μg ml–1. After 20 – 24 h of incubation, the colonies were collected from the plates (417 square petri dishes in total) using 800 μl of LB supplemented with antibiotics. Afterward, 1.5 ml aliquots were amended with 50% glycerol and stored at -80°C.
To assess the concentrations of the peptides for challenging the library, the MICs of the peptides against P. aeruginosa UCBPP-PA14 were determined. An overnight culture of the strain was washed with minimal medium BM2 containing 20 μM Mg2SO4 (Fernández et al., 2012), the optical density (OD600) was adjusted to 0.2 and 1 ml of this suspension was added to 19 ml of BM2 supplemented with 20 μM Mg2SO4, 0.002% tween 80 (Sigma-Aldrich), 0.2% of Rhamnose (Sigma-Aldrich) and different concentrations of the peptide antibiotics (POLs: 8, 12, 16, 32, and 64 μg/ml; L27-11: 0.1, 0.2, and 0.4 μg/ml; chimeras: 0.4, 0.8, and 1.6 μg/ml; 100 mL Erlenmeyer). MICs were defined as no growth after 10 h, a time point when the non-treated culture reached the stationary phase. The library was grown overnight (starting OD600 = 0.01) in BM2 containing 20 μM Mg2SO4 to an OD600 of 0.6, harvested and grown (starting OD600 = 0.01) under the same conditions exponentially for six generations with and without the peptides. Cells were harvested (OD600 = 0.6), grown one more time under the same conditions for six generations before the cells were harvested and stored at -80°C.
Sequencing of the Libraries Challenged With Peptide Antibiotics
Genomic DNA was extracted using the GenElute Bacterial Genomic DNA Kits (Cat. No. NA2100-1KT). The libraries were prepared using the Tn-Seq circle method (Gallagher et al., 2011) with the following modifications: the NEBNext Ultra II DNA Library Prep Kit for Illumina (Cat No. E7645S) was used for end repair and adaptor ligation. DNA fragments were separated on 1.5% agarose gels and the DNA was recovered using the QIAquick Gel Extraction Kit (Qiagen, Cat No./ID:28704). Amplification of the circularized DNA was done with the Phusion High-Fidelity DNA Polymerase (Thermo Fisher Scientific, Cat. No. F530L). Finally, the library was sequenced with the modified forward primer “T23_SEQ_G” and the reverse primer “PE_READ2_SEQ” using the MiSeq reagent kit (V2 300, cycles; Illumina, Ref. MS-102-2002) on the Illumina MiSeq platform. The adapters and the quality of the demultiplexed FASTQ reads were trimmed using the command line “cutadapt –a adapter –q quality –o output.fastq.gz input.fastq.gz” (Martin, 2011). The open source software Tn-Seq Explorer (Solaimanpour et al., 2015) was used to map the pair reads into the genome of P. aeruginosa UCBPP-PA14 (Winsor et al., 2016) and to count the number of Tn insertions using the default “–very-sensitive” command. The UID was determined with the same software by dividing the number of unique insertions in each gene per its length. The UID of each sample was normalized by the total number of unique insertion sites. The fold change (log2) was calculated by comparing the normalized UID (nUID) between the treated cells and the medium control, and this ratio was used to create a MA-plot, which shows the log2-fold change of the normalized UIDs on the y-axis and the average of the normalized UIDs between the PMB-treated sample and the untreated control on the x-axis. Each dot on the MA-plot refers to a gene and red dots depict fitness determinants. From each Tn-Seq data, our custom R-program selected the genes with a log2-fold change of at least -1.0 and a difference of at least 0.005 in the nUID between the untreated and the treated sample, which removed nUID smaller than 0.005 as low insertion counts may lead to false positive results (Wickham, 2017). The clusters of orthologous groups were assigned by using the online EggNOG 4.5.1 mapper (Huerta-Cepas et al., 2017) and Fisher tests were carried out online1 to assess potential over-representation of an EggNOG category. The R package “pvclust” was used in order to cluster the peptides according to the whole Tn-Seq data (nUID) with the Ward criterion and the Euclidean distance (Suzuki and Shimodaira, 2006) and a Venn diagram was constructed to compare the mechanisms of resistance (Dusa, 2018).
Validation of the Tn-Seq Data
To validate the potential implication of some genes in the resistance to the peptidomimetic antimicrobial compounds, we looked for the respective mutant strains in the collection of single Tn insertion P. aeruginosa UCBPP-PA14 mutants (Liberati et al., 2006). The localization of Tn insertion was verified by PCR. After confirming the insertion mutants, the arnB mutant was grown in the absence and presence of the antibiotic in the same condition as the library was grown (vide supra). To test all the 11 strains in a high throughput way, the mutants were challenged with the peptide antibiotics in 200 μl BM2 containing 20 μM Mg2+ in 96-well-plates (Ref. 655161, Greiner bio-one) and the growth was recorded at the end of the exponential phase with the microplate reader “Infinite M200 PRO” (TECAN). To test the effect of spermidine (4 mM; Sigma-Aldrich) on the activity of the antimicrobial compounds, the standard twofold dilution MIC in 96-well-plates was performed (Srinivas et al., 2010).
Quantitative Reverse Transcription PCR (qRT-PCR)
Ribonucleic acid (RNA) was extracted from early stationary cultures of the UCBPP-PA14 wild-type strain grown in BM2 containing 2 mM Mg2SO4 in the absence (control sample) or presence of antimicrobial peptides (approximately MIC30 corresponding to 0.1, 0.4, 0.3, and 0.2 μg/ml, for respectively, PMB, L27-11, 4 and 3). The RNA was further purified using the RNeasy Qiagen kit (Qiagen, Germany). First strand cDNA was synthesized using random primers (Invitrogen, United States) and Murine Leukemia Virus (MLV) reverse transcriptase (Promega, United States). qPCR was performed on the generated cDNA using Brilliant III Ultra-Fast SYBR® Green QPCR Master Mix (Agilent, United States) and a Mx3000P instrument (Agilent, United States). Relative expression levels were calculated using the ΔΔ CT method (Pfaffl, 2001) and the rpoD gene (PA14_07520) was used as the reference gene for normalization.
Data Availability Statement
The datasets generated in this study can be found in the NCBI short reads archive (SRA) platform in the Bioproject “Pseudomonas aeruginosa PA14 Tn-Seq (Peptide Antibiotics),” accession no. PRJNA562484 (https://www.ncbi. nlm.nih.gov/sra/?term=SRR11023345). Each sample can be found with the following accession numbers: BM2 dataset n°1, SRR11023345; BM2 PMB dataset, SRR11023344; BM2 COL dataset, SRR11023343; BM2 dataset n°2, SRR11023342; BM2 L27-11 dataset, SRR11023341; BM2 dataset n°3, SRR11023340; BM2 chimera 4 dataset, SRR11023339; and BM2 chimera 3 dataset, SRR11023338.
Author Contributions
LE designed the study. AV constructed the Tn-Seq library and together with KZ, HL, and MU performed molecular genetics and microbiological studies. AV, MU, KZ, and GP collected and analyzed the data. JR and DO provided peptidomimetic antibiotics. AV, GP, JR, and LE wrote the manuscript, which was seen and agreed by all authors. All authors contributed to the interpretation of results.
Funding
This project was funded by the Swiss National Science Foundation (SystemsX.ch – IPhD project 51PHP0_163556).
Conflict of Interest
HL, MU, and DO were employed by Polyphor AG.
The remaining authors declare that the research was conducted in the absence of any commercial or financial relationships that could be construed as a potential conflict of interest.
Acknowledgments
We thank Stefano Gualdi, Dr. Lucy Poveda, and Dr. Steven Higgins for help in optimizing the Tn-Seq circle method, Lennart Opitz for demultiplexing the data, Dr. Marta Pinto for help in data analysis, and Myriam Gwerder for help in the MIC establishment.
Supplementary Material
The Supplementary Material for this article can be found online at: https://www.frontiersin.org/articles/10.3389/fmicb.2020.01681/full#supplementary-material
Footnotes
References
Baquero, F., Negri, M.-C., Morosini, M.-I., and Blázquez, J. (1998). Antibiotic-selective environments. Clin. Infect. Dis. 27, S5–S11. doi: 10.1086/514916
Baron, S., Hadjadj, L., Rolain, J. M., and Olaitan, A. O. (2016). Molecular mechanisms of polymyxin resistance: knowns and unknowns. Int. J. Antimicrob. Agents 48, 583–591. doi: 10.1016/j.ijantimicag.2016.06.023
Brown, M. R., and Melling, J. (1969). Role of divalent cations in the action of polymyxin B and EDTA on Pseudomonas aeruginosa. Microbiology 59, 263–274. doi: 10.1099/00221287-59-2-263
Brown, M. R., and Watkins, W. M. (1970). Low magnesium and phospholipid content of cell walls of Pseudomonas aeruginosa resistant to polymyxin. Nature 227, 1360–1361. doi: 10.1038/2271360a0
Burrows, L. L., Charter, D. F., and Lam, J. S. (1996). Molecular characterization of the Pseudomonas aeruginosa O5 (PAO1) B-band lipopolysaccharide gene cluster. Mol. Microbiol. 22, 481–495. doi: 10.1046/j.1365-2958.1996.1351503.x
Carattoli, A., Villa, L., Feudi, C., Curcio, L., Orsini, S., Luppi, A., et al. (2017). Novel plasmid-mediated colistin resistance mcr-4 gene in Salmonella and Escherichia coli, Italy 2013, Spain and Belgium, 2015 to 2016. Eurosurveillance 22:30589. doi: 10.2807/1560-7917.es.2017.22.31.30589
Chantziaras, I., Boyen, F., Callens, B., and Dewulf, J. (2014). Correlation between veterinary antimicrobial use and antimicrobial resistance in food-producing animals: a report on seven countries. J. Antimicrob. Chemother. 69, 827–834. doi: 10.1093/jac/dkt443
Cheah, S. E., Johnson, M. D., Zhu, Y., Tsuji, B. T., Forrest, A., Bulitta, J. B., et al. (2016). Polymyxin resistance in Acinetobacter baumannii: genetic mutations and transcriptomic changes in response to clinically relevant dosage regimens. Sci. Rep. 6:26233. doi: 10.1038/srep26233
Cummins, J., Reen, F. J., Baysse, C., Mooij, M. J., and O’Gara, F. (2009). Subinhibitory concentrations of the cationic antimicrobial peptide colistin induce the Pseudomonas quinolone signal in Pseudomonas aeruginosa. Microbiology 155, 2826–2837. doi: 10.1099/mic.0.025643-0
Davis, K. G., Plyte, S. E., Robertson, S. R., Cooper, A., and Kneale, G. G. (1995). Comparison of Pf1 and Fd gene 5 proteins and their single-stranded DNA complexes by NMR spectroscopy and differential scanning calorimetry. Biochemistry 34, 148–154. doi: 10.1021/bi00001a018
Deng, M., and Misra, R. (1996). Examination of AsmA and its effect on the assembly of Escherichia coli outer membrane proteins. Mol. Microbiol. 21, 605–612. doi: 10.1111/j.1365-2958.1996.tb02568.x
Dusa, A. (2018). Venn: Draw Venn Diagrams. Available online at: https://cran.r-project.org/package=venn (accessed January 6, 2020).
Ekkelenkamp, M. B., Canton, R., Diez-Aguilar, M., Tunney, M. M., Gilpin, D. F., Bernardini, F., et al. (2020). Susceptibility of Pseudomonas aeruginosa recovered from cystic fibrosis patients to murepavadin and 13 comparator antibiotics. Antimicrob. Agents Chemother. 64:e01541-19.
Eschbach, M., Schreiber, K., Trunk, K., Buer, J., Jahn, D., and Schobert, M. (2004). Long-term anaerobic survival of the opportunistic pathogen Pseudomonas aeruginosa via pyruvate fermentation. J. Bacteriol. 186, 4596–4604. doi: 10.1128/jb.186.14.4596-4604.2004
Fernández, L., Álvarez-Ortega, C., Wiegand, I., Olivares, J., Kocíncová, D., Lam, J. S., et al. (2013). Characterization of the polymyxin B resistome of Pseudomonas aeruginosa. Antimicrob. Agents Chemother. 57, 110–119. doi: 10.1128/aac.01583-12
Fernández, L., Gooderham, W. J., Bains, M., McPhee, J. B., Wiegand, I., and Hancock, R. E. W. (2010). Adaptive resistance to the “last hope” antibiotics polymyxin B and colistin in Pseudomonas aeruginosa is mediated by the novel two-component regulatory system ParR-ParS. Antimicrob. Agents Chemother. 54, 3372–3382. doi: 10.1128/aac.00242-10
Fernández, L., Jenssen, H., Bains, M., Wiegand, I., Gooderham, W. J., and Hancock, R. E. (2012). The two-component system CprRS senses cationic peptides and triggers adaptive resistance in Pseudomonas aeruginosa independently of ParRS. Antimicrob. Agents Chemother. 56, 6212–6222. doi: 10.1128/aac.01530-12
Filiatrault, M. J., Wagner, V. E., Bushnell, D., Haidaris, C. G., Iglewski, B. H., and Passador, L. (2005). Effect of anaerobiosis and nitrate on gene expression in Pseudomonas aeruginosa. Infect. Immun. 73, 3764–3772. doi: 10.1128/iai.73.6.3764-3772.2005
Frimmersdorf, E., Horatzek, S., Pelnikevich, A., Wiehlmann, L., and Schomburg, D. (2010). How Pseudomonas aeruginosa adapts to various environments: a metabolomic approach. Environ. Microbiol. 12, 1734–1747. doi: 10.1111/j.1462-2920.2010.02253.x
Gallagher, L. A., Ramage, E., Patrapuvich, R., Weiss, E., Brittnacher, M., and Manoil, C. (2013). Sequence-defined transposon mutant library of Burkholderia thailandensis. mBio 4:e00604-13.
Gallagher, L. A., Shendure, J., and Manoil, C. (2011). Genome-scale identification of resistance functions in Pseudomonas aeruginosa using Tn-seq. mBio 2:e00315-10.
Ghequire, M. G. K., Swings, T., Michiels, J., Buchanan, S. K., and De Mot, R. (2018). Hitting with a BAM: selective killing by lectin-like bacteriocins. mBio 9:e02138-17.
Gooderham, W. J., Bains, M., McPhee, J. B., Wiegand, I., and Hancock, R. E. (2008). Induction by cationic antimicrobial peptides and involvement in intrinsic polymyxin and antimicrobial peptide resistance, biofilm formation, and swarming motility of PsrA in Pseudomonas aeruginosa. J. Bacteriol. 190, 5624–5634. doi: 10.1128/jb.00594-08
Groisman, E. A., Kayser, J., and Soncini, F. C. (1997). Regulation of polymyxin resistance and adaptation to low-Mg2+ environments. J. Bacteriol. 179, 7040–7045. doi: 10.1128/jb.179.22.7040-7045.1997
Guina, T., Wu, M., Miller, S. I., Purvine, S. O., Yi, E. C., Eng, J., et al. (2003). Proteomic analysis of Pseudomonas aeruginosa grown under magnesium limitation. J. Am. Soc. Mass Spectrom. 14, 742–751. doi: 10.1016/s1044-0305(03)00133-8
Gutu, A. D., Sgambati, N., Strasbourger, P., Brannon, M. K., Jacobs, M. A., Haugen, E., et al. (2013). Polymyxin resistance of Pseudomonas aeruginosa phoQ mutants is dependent on additional two-component regulatory systems. Antimicrob. Agents Chemother. 57, 2204–2215. doi: 10.1128/aac.02353-12
Han, M.-L., Velkov, T., Zhu, Y., Roberts, K. D., Brun, A. P., and Le, et al. (2018). Polymyxin-induced lipid A deacylation in Pseudomonas aeruginosa perturbs polymyxin penetration and confers high-level resistance. ACS Chem. Biol. 13, 121–130. doi: 10.1021/acschembio.7b00836
Hancock, R. E. W., and Brinkman, F. S. L. (2002). Function of Pseudomonas porins in uptake and efflux. Annu. Rev. Microbiol. 56, 17–38. doi: 10.1146/annurev.micro.56.012302.160310
Hart, E. M., Mitchell, A. M., Konovalova, A., Grabowicz, M., Sheng, J., Han, X., et al. (2019). A small-molecule inhibitor of BamA impervious to efflux and the outer membrane permeability barrier. Proc. Natl. Acad. Sci. U.S.A. 116, 21748–21757. doi: 10.1073/pnas.1912345116
Higgins, S., Sanchez-Contreras, M., Gualdi, S., Pinto-Carbó, M., Carlier, A., and Eberl, L. (2017). The essential genome of Burkholderia cenocepacia H111. J. Bacteriol. 199:e00260-17.
Ho, B. T., Basler, M., and Mekalanos, J. J. (2013). Type 6 secretion system-mediated immunity to type 4 secretion system-mediated horizontal gene transfer. Science 342, 250–253. doi: 10.1126/science.1243745
Holmes, A. H., Moore, L. S., Sundsfjord, A., Steinbakk, M., Regmi, S., Karkey, A., et al. (2016). Understanding the mechanisms and drivers of antimicrobial resistance. Lancet 387, 176–187.
Huerta-Cepas, J., Forslund, K., Coelho, L. P., Szklarczyk, D., Jensen, L. J., von Mering, C., et al. (2017). Fast genome-wide functional annotation through orthology assignment by eggNOG-mapper. Mol. Biol. Evol. 34, 2115–2122. doi: 10.1093/molbev/msx148
Imai, Y., Meyer, K. J., Iinishi, A., Favre-Godal, Q., Green, R., Manuse, S., et al. (2019). A new antibiotic selectively kills Gram-negative pathogens. Nature 576, 459–464.
Jeannot, K., Bolard, A., and Plésiat, P. (2017). Resistance to polymyxins in Gram-negative organisms. Int. J. Antimicrob. Agents 49, 526–535. doi: 10.1016/j.ijantimicag.2016.11.029
Johnson, L., Mulcahy, H., Kanevets, U., Shi, Y., and Lewenza, S. (2012). Surface-localized spermidine protects the Pseudomonas aeruginosa outer membrane from antibiotic treatment and oxidative stress. J. Bacteriol. 194, 813–826. doi: 10.1128/jb.05230-11
Kang, C. I., Kim, S. H., Kim, H. B., Park, S. W., Choe, Y. J., Oh, M. D., et al. (2003). Pseudomonas aeruginosa bacteremia: risk factors for mortality and influence of delayed receipt of effective antimicrobial therapy on clinical outcome. Clin. Infect. Dis. 37, 745–751. doi: 10.1086/377200
Khaledi, A., Schniederjans, M., Pohl, S., Rainer, R., Bodenhofer, U., Xia, B., et al. (2016). Transcriptome profiling of antimicrobial resistance in Pseudomonas aeruginosa. Antimicrob. Agents Chemother. 60, 4722–4733. doi: 10.1128/aac.00075-16
Kneale, G. G. (1983). Dissociation of the Pf1 nucleoprotein assembly complex and characterization of the DNA binding protein. Biochim. Biophys. Acta 739, 216–224. doi: 10.1016/0167-4781(83)90032-5
Kovach, M. E., Elzer, P. H., Hill, D. S., Robertson, G. T., Farris, M. A., Roop, R. M., et al. (1995). Four new derivatives of the broad host range cloning vector PBBR1MCS, carrying different antibiotic resistance cassettes. Gene 166, 175–176. doi: 10.1016/0378-1119(95)00584-1
Kwa, A., Kasiakou, S. K., Tam, V. H., and Falagas, M. E. (2007). Polymyxin B: similarities to and differences from colistin (polymyxin E). Expert Rev. Anti Infect. Ther. 5, 811–821. doi: 10.1586/14787210.5.5.811
Kwon, D. H., and Lu, C. D. (2006). Polyamines increase antibiotic susceptibility in Pseudomonas aeruginosa. Antimicrob. Agents Chemother. 50, 1623–1627. doi: 10.1128/AAC.50.5.1623-1627.2006
Kwon, D. H., and Lu, C. D. (2007). Polyamine effects on antibiotic susceptibility in bacteria. Antimicrob. Agents Chemother. 51, 2070–2077. doi: 10.1128/aac.01472-06
Lesic, B., Starkey, M., He, J., Hazan, R., and Rahme, L. G. (2009). Quorum sensing differentially regulates Pseudomonas aeruginosa type VI secretion locus I and homologous loci II and III, which are required for pathogenesis. Microbiology 155, 2845–2855. doi: 10.1099/mic.0.029082-0
Liberati, N. T., Urbach, J. M., Miyata, S., Lee, D. G., Drenkard, E., Wu, G., et al. (2006). An ordered, nonredundant library of Pseudomonas aeruginosa strain PA14 transposon insertion mutants. Proc. Natl. Acad. Sci. U.S.A. 103, 2833–2838. doi: 10.1073/pnas.0511100103
Luther, A., Urfer, M., Zahn, M., Müller, M., Wang, S. Y., Mondal, M., et al. (2019). Chimeric peptidomimetic antibiotics against Gram-negative bacteria. Nature 576, 452–458.
Macfarlane, E. L. A., Kwasnicka, A., and Hancock, R. E. W. (2000). Role of Pseudomonas aeruginosa Phop-PhoQ in resistance to antimicrobial cationic peptides and aminoglycosides. Microbiology 146, 2543–2554. doi: 10.1099/00221287-146-10-2543
Martin, M. (2011). Cutadapt removes adapter sequences from high-throughput sequencing reads. EMBnet J. 17, 10–12. doi: 10.14806/ej.17.1.200
Martorana, A. M., Motta, S., Silvestre, D., Di, Falchi, F., Dehò, G., et al. (2014). Dissecting Escherichia coli outer membrane biogenesis using differential proteomics. PLoS One 9:e100941. doi: 10.1371/journal.pone.0100941
McPhee, J. B., Bains, M., Winsor, G., Lewenza, S., Kwasnicka, A., Brazas, M. D., et al. (2006). Contribution of the PhoP-PhoQ and PmrA-PmrB two-component regulatory systems to Mg2+-induced gene regulation in Pseudomonas aeruginosa. J. Bacteriol. 188, 3995–4006. doi: 10.1128/jb.00053-06
McPhee, J. B., Lewenza, S., and Hancock, R. E. (2003). Cationic antimicrobial peptides activate a two-component regulatory system, PmrA-PmrB, that regulates resistance to polymyxin B and cationic antimicrobial peptides in Pseudomonas aeruginosa. Mol. Microbiol. 50, 205–217. doi: 10.1046/j.1365-2958.2003.03673.x
Miller, J. H. (1972). Experiments in Molecular Genetics. Cold Spring Harbor, NY: Cold Spring Harbor Laboratory Press.
Mulcahy, H., and Lewenza, S. (2011). Magnesium limitation is an environmental trigger of the Pseudomonas aeruginosa biofilm lifestyle. PLoS One 6:e23307. doi: 10.1371/journal.pone.0023307
Murray, J. L., Kwon, T., Marcotte, E. M., and Whiteley, M. (2015). Intrinsic antimicrobial resistance determinants in the superbug Pseudomonas aeruginosa. mBio 6:e01603-15.
Olaitan, A. O., Morand, S., and Rolain, J. M. (2014). Mechanisms of polymyxin resistance: acquired and intrinsic resistance in bacteria. Front. Microbiol. 5:643. doi: 10.3389/fmicb.2014.00643
Palmer, K. L., Brown, S. A., and Whiteley, M. (2007). Membrane-bound nitrate reductase is required for anaerobic growth in cystic fibrosis sputum. J. Bacteriol. 189, 4449–4455. doi: 10.1128/jb.00162-07
Pfaffl, M. W. (2001). A new mathematical model for relative quantification in real-time RT-PCR. Nucleic Acids Res. 29:e45. doi: 10.1093/nar/29.9.e45
Phadnis, S. H., and Berg, D. E. (1987). Identification of base pairs in the outside end of insertion sequence IS50 that are needed for IS50 and Tn5 transposition. Genetics 84, 9118–9122. doi: 10.1073/pnas.84.24.9118
Prestinaci, F., Pezzotti, P., and Pantosti, A. (2015). Antimicrobial resistance: a global multifaceted phenomenon. Pathog. Glob. Health 109, 309–318. doi: 10.1179/2047773215Y.0000000030
Puja, H., Bolard, A., Noguès, A., Plésiat, P., and Jeannot, K. (2020). The efflux pump MexXY/OprM contributes to the tolerance and acquired resistance of Pseudomonas aeruginosa to colistin. Antimicrob. Agents Chemother. 64:e02033-19.
Rabanal, F., and Cajal, Y. (2017). Recent advances and perspectives in the design and development of polymyxins. Nat. Prod. Rep. 34, 886–908. doi: 10.1039/c7np00023e
Rahme, L. G., Stevens, E. J., Wolfort, S. F., Shao, J., Tompkins, R. G., and Ausubel, F. M. (1995). Common virulence factors for bacterial pathogenicity in plants and animals. Science 268, 1899–1902. doi: 10.1126/science.7604262
Rajagopal, M., Martin, M. J., Santiago, M., Lee, W., Kos, V. N., Meredith, T., et al. (2016). Multidrug intrinsic resistance factors in Staphylococcus aureus identified by profiling fitness within high-diversity transposon libraries. mBio 7:e00950-16.
Rice, L. B. (2008). Federal funding for the study of antimicrobial resistance in nosocomial pathogens: no ESKAPE. J. Infect. Dis. 197, 1079–1081. doi: 10.1086/533452
Robinson, J. A. (2013). Protein epitope mimetics in the age of structural vaccinology. J. Pept. Sci. 19, 127–140. doi: 10.1002/psc.2482
Romano, K. P., Warrier, T., Poulsen, B. E., Nguyen, P. H., Loftis, A. R., Saebi, A., et al. (2019). Mutations in pmrB confer cross-resistance between the LptD inhibitor POL7080 and colistin in Pseudomonas aeruginosa. Antimicrob. Agents Chemother. 63:e00511-19.
Sader, H. S., Flamm, R. K., Dale, G. E., Rhomberg, P. R., and Castanheira, M. (2018). Murepavadin activity tested against contemporary (2016-17) clinical isolates of XDR Pseudomonas aeruginosa. J. Antimicrob. Chemother. 73, 2400–2404. doi: 10.1093/jac/dky227
Sana, T. G., Hachani, A., Bucior, I., Soscia, C., Garvis, S., Termine, E., et al. (2012). The second type VI secretion system of Pseudomonas aeruginosa strain PAO1 is regulated by quorum sensing and Fur and modulates internalization in epithelial cells. J. Biol. Chem. 287, 27095–27105. doi: 10.1074/jbc.m112.376368
Santiago, M., Lee, W., Fayad, A. A., Coe, K. A., Rajagopal, M., Do, T., et al. (2018). Genome-wide mutant profiling predicts the mechanism of a Lipid II binding antibiotic. Nat. Chem. Biol. 14, 601–608. doi: 10.1038/s41589-018-0041-4
Schmidt, J., Patora-Komisarska, K., Moehle, K., Obrecht, D., and Robinson, J. A. (2013). Structural studies of β-hairpin peptidomimetic antibiotics that target LptD in Pseudomonas sp. Bioorg. Med. Chem. 21, 5806–5810. doi: 10.1016/j.bmc.2013.07.013
Schreiber, K., Boes, N., Eschbach, M., Jaensch, L., Wehland, J., Bjarnsholt, T., et al. (2006). Anaerobic survival of Pseudomonas aeruginosa by pyruvate fermentation requires an Usp-type stress protein. J. Bacteriol. 188, 659–668. doi: 10.1128/jb.188.2.659-668.2006
Secor, P. R., Sweere, J. M., Michaels, L. A., Malkovskiy, A. V., Lazzareschi, D., Katznelson, E., et al. (2015). Filamentous bacteriophage promote biofilm assembly and function. Cell Host Microbe 18, 549–559. doi: 10.1016/j.chom.2015.10.013
Solaimanpour, S., Sarmiento, F., and Mrázek, J. (2015). Tn-seq explorer: a tool for analysis of high-throughput sequencing data of transposon mutant libraries. PLoS One 10:e0126070. doi: 10.1371/journal.pone.0126070
Sonnabend, M. S., Klein, K., Beier, S., Angelov, A., Kluj, R., Mayer, C., et al. (2020). Identification of drug resistance determinants in a clinical isolate of Pseudomonas aeruginosa by high-density transposon mutagenesis. Antimicrob. Agents Chemother. 64:e01771-19.
Sousa, M. C. (2019). New antibiotics target the outer membrane of bacteria. Nature 576, 389–390. doi: 10.1038/d41586-019-03730-x
Srinivas, N., Jetter, P., Ueberbacher, B. J., Werneburg, M., Zerbe, K., Steinmann, J., et al. (2010). Peptidomimetic antibiotics target outer-membrane biogenesis in Pseudomonas aeruginosa. Science 327, 1010–1013. doi: 10.1126/science.1182749
Storek, K. M., Auerbach, M. R., Shi, H., Garcia, N. K., Sun, D., Nickerson, N. N., et al. (2018a). Monoclonal antibody targeting the β-barrel assembly machine of Escherichia coli is bactericidal. Proc. Natl. Acad. Sci. U.S.A. 115, 3692–3697. doi: 10.1073/pnas.1800043115
Storek, K. M., Vij, R., Sun, D., Smith, P. A., Koerber, J. T., and Rutherford, S. T. (2018b). The Escherichia coli β-Barrel assembly machinery is sensitized to perturbations under high membrane fluidity. J. Bacteriol. 201, e517–e518.
Storm, D. R., Rosenthal, K. S., and Swanson, P. E. (1977). Polymyxin and related peptide antibiotics. Annu. Rev. Biochem. 46, 723–763. doi: 10.1146/annurev.bi.46.070177.003451
Suzuki, R., and Shimodaira, H. (2006). Pvclust?: an R package for assessing the uncertainty in hierarchical clustering. Bioinformatics 22, 1540–1542. doi: 10.1093/bioinformatics/btl117
Urfer, M., Bogdanovic, J., Monte, F., Lo, Moehle, K., Zerbe, K., et al. (2016). A peptidomimetic antibiotic targets outer membrane proteins and disrupts selectively the outer membrane in Escherichia coli. J. Biol. Chem. 291, 1921–1932. doi: 10.1074/jbc.m115.691725
van der Meijden, B., and Robinson, J. A. (2015). Synthesis of a polymyxin derivative for photolabeling studies in the gram-negative bacterium Escherichia coli. J. Pept. Sci. 21, 231–235. doi: 10.1002/psc.2736
Velkov, T., Roberts, K. D., Nation, R. L., Wang, J., Thompson, P. E., and Li, J. (2014). Teaching “old” polymyxins new tricks: new-generation lipopeptides targeting Gram-negative “superbugs.” ACS Chem. Biol. 9, 1172–1177. doi: 10.1021/cb500080r
Vetterli, S. U., Zerbe, K., Müller, M., Urfer, M., Mondal, M., Wang, S.-Y., et al. (2018). Thanatin targets the intermembrane protein complex required for lipopolysaccharide transport in Escherichia coli. Sci. Adv. 4:eaau2634. doi: 10.1126/sciadv.aau2634
Wach, A., Dembowsky, K., and Dale, G. E. (2018). Pharmacokinetics and safety of intravenous murepavadin infusion in healthy adult subjects administered single and multiple ascending doses. Antimicrob. Agents Chemother. 62:e02355-17.
Werneburg, M., Zerbe, K., Juhas, M., Bigler, L., Stalder, U., Kaech, A., et al. (2012). Inhibition of lipopolysaccharide transport to the outer membrane in Pseudomonas aeruginosa by peptidomimetic antibiotics. ChemBioChem 13, 1767–1775. doi: 10.1002/cbic.201200276
WHO (2001). WHO Global Strategy for Containment of Antimicrobial Resistance. Geneva: World Health Organization, 1–99.
Wickham, H. (2017). Ggplot2: elegant graphics for data analysis. J. Statist. Softw. 77, 1–3. doi: 10.18637/jss.v077.b02
Williams, H. D., Zlosnik, J. E., and Ryall, B. (2006). Oxygen, cyanide and energy generation in the cystic fibrosis pathogen Pseudomonas aeruginosa. Adv. Microb. Physiol. 52, 1–71. doi: 10.1016/s0065-2911(06)52001-6
Winsor, G. L., Griffiths, E. J., Lo, R., Dhillon, B. K., Shay, J. A., and Brinkman, F. S. (2016). Enhanced annotations and features for comparing thousands of Pseudomonas genomes in the Pseudomonas genome database. Nucleic Acids Res. 44, D646–D653. doi: 10.1093/nar/gkv1227
Xiong, J., Déraspe, M., Iqbal, N., Krajden, S., Chapman, W., Dewar, K., et al. (2017). Complete genome of a panresistant Pseudomonas aeruginosa strain, isolated from a patient with respiratory failure in a Canadian community hospital. Genome Announc. 5:e00458-17.
Yu, Z., Qin, W., Lin, J., Fang, S., and Qiu, J. (2015). Antibacterial mechanisms of polymyxin and bacterial resistance. Biomed Res. Int. 2015:679109. doi: 10.1155/2015/679109
Keywords: transposon sequencing, antibiotic resistance, polymyxins, peptidomimetics, Pseudomonas aeruginosa
Citation: Vitale A, Pessi G, Urfer M, Locher HH, Zerbe K, Obrecht D, Robinson JA and Eberl L (2020) Identification of Genes Required for Resistance to Peptidomimetic Antibiotics by Transposon Sequencing. Front. Microbiol. 11:1681. doi: 10.3389/fmicb.2020.01681
Received: 22 April 2020; Accepted: 26 June 2020;
Published: 23 July 2020.
Edited by:
Paolo Visca, Roma Tre University, ItalyReviewed by:
Francesco Imperi, Roma Tre University, ItalyAlessandra Polissi, University of Milan, Italy
Robert E. W. Hancock, The University of British Columbia, Canada
Copyright © 2020 Vitale, Pessi, Urfer, Locher, Zerbe, Obrecht, Robinson and Eberl. This is an open-access article distributed under the terms of the Creative Commons Attribution License (CC BY). The use, distribution or reproduction in other forums is permitted, provided the original author(s) and the copyright owner(s) are credited and that the original publication in this journal is cited, in accordance with accepted academic practice. No use, distribution or reproduction is permitted which does not comply with these terms.
*Correspondence: Leo Eberl, bGViZXJsQGJvdGluc3QudXpoLmNo; bGVvLmViZXJsQGJvdGluc3QudXpoLmNo