- 1Laboratório de Biologia Molecular, Instituto de Ciências Biológicas, Universidade Federal de Goiás, Goiânia, Brazil
- 2Programa de Pós-graduação em Genética e Biologia Molecular, Universidade Federal de Goiás, Goiânia, Brazil
- 3Departamento de Biologia Celular, Instituto de Biologia, Universidade de Brasília, Brasília, Brazil
Copper is an essential micronutrient for the performance of important biochemical processes such as respiration detoxification, and uptake of metals like iron. Studies have shown that copper deprivation is a strategy used by the host against pathogenic fungi such as Cryptoccocus neoformans and Candida albicans during growth and development of infections in the lungs and kidneys. Although there are some studies, little is known about the impact of copper deprivation in members of the Paracoccidioides genus. Therefore, using isobaric tag labeling (iTRAQ)-Based proteomic approach and LC-MS/MS, we analyzed the impact of in vitro copper deprivation in the metabolism of Paracoccidioides brasiliensis. One hundred and sixty-four (164) differentially abundant proteins were identified when yeast cells were deprived of copper, which affected cellular respiration and detoxification processes. Changes in cellular metabolism such as increased beta oxidation and cell wall remodeling were described.
Introduction
Based on the ability to cycle between reduced and oxidized states the metal copper is an essential micronutrient to organisms. Copper acts as a cofactor for superoxide dismutase Cu/Zn (Sod1p), an important detoxification enzyme, and cytochrome c oxidase (Coxp), fundamental to the cellular respiration process. Copper in the cupric (Cu2+) state is reduced to (Cu1+) by iron reductases (Fre1p, Fre2p) in the extracellular medium (Hassett and Kosman, 1995) and transported into cells by high affinity transporters (Ctr1p, Ctr3p, Ctr4p) (Dancis et al., 1994; Waterman et al., 2012). Then, copper is distributed by chaperones as Ccs1p, with an N-terminal domain responsible for transferring the metal to Sod1p. This process is fundamental for the activation of this enzyme (Fukuoka et al., 2017). The Atx1p chaperone delivers copper to a P-type ATPase (Ccc2p) present in the membrane of the Golgi complex (Lin et al., 1997). In Saccharomyces cerevisiae, when there is a compromise in the activity of Sod1p, the Atx1p 47 is described as a protein that protects against reactive oxygen species (Lin et al., 1997; Smith et al., 2017). Furthermore, the Cox17p chaperone is an important copper donor to cytochrome c oxidase (Coxp) (Horng et al., 2004), present in the respiratory chain complex IV. The enzyme is a terminal oxidase of the respiratory chain and presents a highly conserved heme-copper catalytic structure (Khalimonchuk and Rödel, 2005).
Since copper is an essential trace element presenting toxic properties when in excess, organisms developed sophisticated mechanisms to provide the micronutrient to biological processes while preventing Cu1+ toxicity. This includes the increase in the production of toxic hydroxyl radicals, damaging DNA and proteins (Urbanski and Berêsewicz, 2000; García-Santamarina and Thiele, 2015). Mechanisms that allow copper homeostasis at an appropriate concentration operates at several levels. Copper homeostasis in fungi is obtained at both transcriptional and post-transcriptional levels (Gross et al., 2000). In the model fungus S. cerevisiae the cell surface metaloreductases Fre1p and Fre2p reduce Cu2+ to Cu1+ (Georgatsou et al., 1997). Also, the high-affinity transporters Ctr1p and Ctr3p, regulated at transcriptional level by copper availability, are responsible for the metal uptake (Pena et al., 2000).
Stringent control of Cu uptake is also critical for avoiding excessive intracellular accumulation and toxicity (Peña et al., 1999). Under the exposure to a high-Cu environment, S. cerevisiae transcriptional factor MAC1 is rapidly degraded, and that response is accompanied by a decreased expression of the target Ctrp transporters at both the transcriptional and post-translational levels (Zhu et al., 1998). Another transcriptional factor responsive to excessive copper is ACE1; submitted to these conditions this transcriptional factor induces the expression of copper detoxification genes such as Sod1, Cup1, and Crs5 (Smith et al., 2017). In C. albicans the response to copper excess involves the activation of the copper extrusion pump Crp1p, responsible for transporting copper from the cytoplasm to the extracellular environment coupled to the expression of copper metallothionein Cup1p, which binds copper when at high concentration inside the cells (Weissman et al., 2000).
An important aspect of host-pathogen interaction is the homeostasis of copper during infection. In this sense, the host can sequester copper in a process known as nutritional immunity, which prevents the pathogen from obtaining the metal. For example, the opportunistic fungal pathogen C. albicans faces copper nutritional immunity since the calprotectin protein from the host, sequester Cu by binding Cu2+ with subpicomolar affinity, inducing copper starvation (Besold et al., 2018). It has also been shown that copper poisoning is used as microbicidal strategy to control bacterial and fungal infections (Mackie et al., 2016; Ladomersky et al., 2017; Wiemann et al., 2017). Infection based studies suggest that macrophages elevate copper levels inside the phagosome by locating the P-type copper ATPase pump (ATP7a) to the phagosome membrane. In addition, such studies also demonstrated that copper detoxifying fungal machinery is required for virulence (Mackie et al., 2016; Wiemann et al., 2017).
Members of the Paracoccidioides genus are the causative agents of paracoccidioidomycosis (PCM), a fungal disease highly prevalent in Latin America (Restrepo, 1985; Martinez, 2015). The infection begins after inhalation of conidia or mycelial fragments that reach the alveoli where they differentiate into yeast cells, a process highly dependent on temperature and crucial for the disease establishment (McEwen et al., 1987; San-Blas et al., 2002). Several studies have demonstrated the importance of copper for different organisms (Peña et al., 1999; Gross et al., 2000; Ding et al., 2014a). However, little is known about the impact of this metal deprivation in the genus Paracoccidioides. Previous in silico analysis have shown that Paracoccidioides spp. have homologous copper homeostasis-related proteins such as metalloreductases (Fre1p, Fre3p), high and low affinity copper transporters Ctr3p and Ctr2p respectively, copper metalloregulatory transcription factor (Mac1), metallochaperone (Atx1p), transporting P-type ATPase (Ccc2p); and enzymes related to the detoxification process (Silva et al., 2011).
In this work we suggested that P. brasiliensis can face copper deprivation upon macrophage infection. We also observed that in vitro copper depletion affected the activity of cytochrome c oxidase. The latter is a process accompanied by increase in the expression of the enzyme alternative oxidase (Aoxp), associated with an alternative respiratory pathway. A decrease in the expression of copper dependent Sod1p influenced the detoxification capacity, leading to an increased expression of thioredoxin and superoxide dismutase Mn/Fe (Sod5p). Enzymes of the pentose phosphate pathway which is related to the production of NADPH this being associated with detoxification processes, as well as the chaperone Atx1p, were increased. Up-regulation of proteins of beta-oxidation, the glyoxylate cycle, and cell wall remodeling were also identified.
Materials and Methods
Microorganism and Growth Conditions
P. brasiliensis Pb18 (ATCC 32069) was employed in this work. The yeast cells were cultivated for 4 days, at 36°C in BHI solid medium supplemented with 4% (w/v) glucose. For the experiment involving copper depletion P. brasiliensis yeast cells were incubated in McVeigh/Morton medium (MMcM) (Restrepo and Jimenez, 1980). The copper depleted medium was prepared without CuSO4 and supplemented with 50 μM bathocuproinedisulfonic acid (BCS). For control, the medium was supplemented with 10 μM CuSO4.
P. brasiliensis Cell Viability Analysis
After the cultivation of yeast cells, viability was determined by membrane integrity analysis using propidium iodide as dead cells marker, as previously described (Grossklaus et al., 2013). Yeast cells (5 × 106 yeast cells/mL) were centrifuged at 3500 × g at 4°C for 5 min and the supernatant was discarded. Propidium iodide (1 μg/mL) was added to the cell’s suspension for 20 min in the dark and at room temperature; the cells were analyzed by flow cytometer (BD® Accuri C6 Flow Cytometer). A minimal of 10,000 events per sample was acquired with the FL-3-H channel.
Macrophage Infection and Generation of Macrophage ATP7a-Silenced Cells
Murine macrophage cell line J774 A. 1 (BCRJ Cell Bank, Rio de Janeiro, accession number 0121) was employed. The macrophages were maintained in RPMI medium (RPMI 1640, Vitrocell, Brazil), the latter being supplemented with non-essential amino acids (Sigma-Aldrich, St. Louis, MO, United States) and 10% (w/v) fetal bovine serum (FBS), at 37°C in 5% CO2. 1 × 106 macrophages were seeded into each well of a 24 well tissue plate and IFN-γ (1 U/mL) (Sigma–Aldrich, St. Louis, MO, United States) was added for 24 h at 37°C in 5% CO2 for macrophage activation.
ATP7a silenced macrophage cells were generated by transient transfection of a siRNA double-stranded, against the ATP7a gene (Silencer siRNA mouse ATP7a Cat. No. AM16708, Thermo Fisher Scientific, Waltham, MA, United States), using Lipofectamine 2000 as the transfection reagent, following the Lipofectamine 2000 protocol (Invitrogen, Cat. No. 11668-027), in RMPI 1640 medium without any supplementation. A scramble siRNA was used as transfection negative control (Silencer Negative Control Cat. No. AM4611, Thermo Fisher Scientific, Waltham, MA, United States).
Viability of 24, 48, and 96 h macrophages was confirmed by microscopy using Trypan blue dye. Trizol was added in each well, and the total RNA was isolated. RNAs from non-silenced macrophages were obtained as control. After reverse transcription, the ATP7a inhibition was evaluated by qRT-PCR, using the TaqMan gene expression assay (Thermo Fisher Scientific, Waltham, MA, United States; ATP7a TaqMan Cat. No. 437663). The transcript of α-tubulin (TaqMan Cat. No. 492936) was used for normalization of transcript amplification. The best processing time for subsequent transfections was set to 48 h after the quality analysis of 24, 4, and 96 h of ATP7a silencing transcriptional profiles. The Gene ID of these primers are described in Supplementary Table 1.
For fungal burden analysis in the ATP7a silenced macrophages, 5 × 106 cells/mL of silenced and non-silenced macrophages were co-cultivated with 1 × 106 yeast cells/mL in RMPI 1640 medium without supplementation. The cells were co-cultivated for 24 h (Dantas et al., 2009) at 37°C in 5% CO2 to allow fungal internalization. Each well was washed twice with 1 mL of PBS 1X to get rid of non-internalized yeast cells. Infected macrophages were lysed with ice-cold ultrapure sterile water, and dilutions of the lysates containing the phagocytized yeast cells were plated in BHI agar and incubated at 37°C in 5% CO2 atmosphere. After 7 days of incubation the number of CFU was determined.
Protein Extraction
Yeast cells cultivated in presence of copper (10 μM CuSO4) and absence of this metal (50 μM BCS), were harvested at a 24 h time-point and centrifuged at 5000 × g, 4°C for 10 min. The supernatant was discarded, and the cells were washed three times with PBS 1X. Afterward, the cells were resuspended in an extraction buffer containing 20 mM Tris–HCl pH 8.8; 2 mM CaCl2 with a mixture of nuclease and protease inhibitors (GE Healthcare). Following this step, the cells were distributed in tubes, glass beads were added, and the cells were disrupted on ice in a bead beater apparatus for 5 cycles of 30 s. Next, the cells were centrifuged at 10,000 g for 10 min at 4°C, three times and the quantification of protein extracts was performed as described (Lacerda Pigosso et al., 2017).
In-Solution Protein Digestion
150 μg of protein extract of each condition/replicate was prepared for trypsin digestion (Queiroz et al., 2014). Ice-cold acetone was added to the protein suspension, followed by incubation at −20°C. After, the samples were centrifuged at 20,000 × g for 15 min at 4°C and re-suspended in 8 M urea in 0.05 M triethylammonium bicarbonate buffer (TEAB), pH 7.9. Next, proteins were reduced with 0.005 M DTT for 25 min at 55°C and alkylated with 0.014 M iodoacetamide for 40 min at room temperature, in the dark. Samples were diluted fivefold with 0.001 M CaCl2 in 0.025 M TEAB, pH 7.9. Modified trypsin (Promega, Madison, WI, United States) was added in a 1:50 (w/w) substrate ratio. The samples were incubated overnight at 37°C, followed by acidification with 0.1% (v/v) TFA and desalting on homemade C18 microcolumns in P200 low-binding tips. Thereafter, all samples were lyophilized in speed vacuum (Queiroz et al., 2014).
Isobaric Tag Labeling
Biological triplicates of protein samples from yeast cells were labeled with iTRAQ as previously described (Araújo et al., 2019). Desalting of the samples was performed, and 50 μg of the material was resuspended in 17 μL of 300 mM TEAB. The iTRAQ marker (Reagents Multiplex Kit, Sigma Aldrich) resuspended in 70 μL of ethanol, was added afterward. The samples were incubated for 2 h at room temperature. Equimolar amounts of iTRAQ label were mixed in all samples (copper starvation labeled with 114; control; with 115).
LC-MS/MS
iTRAQ labeled peptides were analyzed in three technical repetitions each and fractionated using a nano-UHPLC Dionex Ultimate 3000 (Thermo Fisher Scientific) coupled with an Orbitrap EliteTM Hybrid Ion Trap-Orbitrap Mass Spectrometer (Thermo Fisher Scientific) as previously described (Queiroz et al., 2014). Each fraction was loaded onto a pre-column (110 μm × 200 nm) packed in-house with C18 ResiproSilPur of 5 μm with 120 Åpores (Dr. Maisch GmbH, Ammerbuch, Germany). A second chromatography was carried out in column (75 μm × 35 nm) packed in- house with C18 ResiproSilPur of 3 μm and with 120 Åpores (Dr. Maisch GmbH, Ammerbuch, Germany). The process was eluted using a gradient from 100% solvent A [0.1% (v/v) formic acid] and 26% solvent B [0.1% (v/v) formic acid, 95% (v/v) acetonitrile] for 180 min, followed by 26–100% solvent B for 5 min and 100% solvent B for 8 min (a total of 193 min at 200 nL/min). After each run, the column was washed with 90% solvent B and re-equilibrated with solvent A. Mass spectra were acquired in positive ion mode, by applying data-dependent automatic survey MS scan and tandem mass spectra (MS/MS) acquisition modes. Each MS scan in the Orbitrap analyzer (mass range = m/z 350–1800, resolution = 120,000) was followed by MS/MS of the fifteen most intense ions in the LTQ. Fragmentation in the LTQ was performed by high-energy collision-induced dissociation (HCD), and selected sequenced ions sequences were dynamically excluded every 15 s.
MS/MS Spectra Processing
Raw data processing used Proteome Discoverer v.1.3 beta (Thermo Fisher Scientific). Searches of the Raw files used Proteome Discoverer with Mascot v.2.3 algorithm against the P. brasiliensis database, downloaded using the Database on Demand tool in UniProt/SWISS-PROT1 and NCBI2 database. For false discovery rates, the number of proteins, protein groups and peptides were filtered to values below 1%. Using Proteome Discoverer two peptides per protein was the minimum value accepted for identification. Raw data was deposited in the Peptide Atlas database3 with the following access number PASS01563.
Data Analysis
The data consisted of three replicates containing global proteome. To increase the reliability, the acceptance criteria were applied, as following: proteins identified with at least two peptides; with high or medium FDR and in at least 2 of 3 replicates. For statistical analysis, unpaired Student’s t-test was applied. Statistical difference was set at p ≤ 0.05. In this way, two quantitative estimates provided for each protein were utilized: the fold change ratios of differential expression between labeled protein extracts; the p value, as cited above. Functional categories were determined by search in Blast2GO platform4, Pedant on MIPS-Functional Catalog5 and KEGG database6. Sequence annotation was assessed using a Blastp algorithm7.
cDNA Synthesis and Quantitative Real Time PCR (RT-qPCR) Analysis
Yeast cells after in vitro copper deprivation were harvested at time-points 0, 4, 8, 12, and 24 h. Then, cells were treated with TRIzol (SIGMA-ALDRICH) and the RNA was extracted following the manufacture’s protocol. Then, total RNA was treated with DNase (RQ1 RNase-free DNase, Promega) and subjected to reverse transcription (SuperScript III First-Strand Synthesis SuperMix; Invitrogen, Life Technologies) according to the manufacturer’s recommendation. SYBR green PCR master mix (Applied Biosystems, Foster City, CA, United States) was used in the RT-qPCR assays performed in a Step OnePlus system (Applied Biosystems). Normalization used the gene encoding the L34 protein (PADG_04085). Standard curves were generated by 1:5 dilution of the cDNA, and the relative expression levels of the transcripts were calculated using the standard curve method for relative quantification (Bookout et al., 2006). The used oligonucleotides are described in Supplementary Table 1.
Analysis of Chitin and Glucan Amount in the Cell Wall of P. brasiliensis During in vitro Copper Deprivation
Calcoflour White (CFW, Sigma-Aldrich) and Aniline Blue (AB, Sigma-Aldrich) were used to stain P. brasiliensis yeast cells to evaluate the effect of copper depletion in the composition of the cell wall, since CFW and AB specifically binds chitin and glucan respectively. P. brasiliensis grew in copper depletion or in presence of this metal for 24 h. The cells were stained with the dyes described above and analyzed by fluorescence microscopy as already detailed (De Curcio et al., 2017). In synthesis, for analysis of chitin amount, the cells were fixed in 100% methanol at -80°C for 20 min. The cells were collected, stained with CFW (100 μg/mL in PBS 1 X) for 30 min and washed with PBS 1 X. For analyses of glucan, the cells of P. brasiliensis were incubated with aniline blue solution (Sigma) for 5 min and subsequently washed twice with PBS 1 X. Both cells stained with CFW and aniline blue were visualized in a fluorescence microscope (Zeiss Axiocam MRc-Scope A1). The minimum of 50 cells for each microscope slides, in triplicates, were used to evaluate fluorescence intensity of the cells. The software provided the fluorescence intensity (in pixels) and the standard error of each analysis. Statistical comparisons were performed using the Student’s t-test and p ≤ 0.05 were considered statistically significant.
Analysis of Mitochondrial Activity of P. brasiliensis Exposed to in vitro Copper Deprivation
The mitochondrial activity was monitored using the Rhodamine 123 (Sigma-Aldrich) and Mitotracker Green FM (Sigma-Aldrich) fluorescent dyes. Yeast cells were stained according to the manufacturer’s instructions. Initially, yeast cells were harvested by centrifugation of 8000 × g for 10 min at 4°C, diluted in PBS 1X to 106 cells/mL and stained during 45 min at 37°C with Mitotracker (400nM). Then, the cells were washed with PBS 1 X and labeled with Rhodamine (2.4 μM) for 45 min at 37°C. Afterward, the cells were washed three times with PBS 1X and analyzed by fluorescence microscopy (Zeiss Axiocam MRc – Scope A1) at 450–490 nm for Mitotracker (FS09) and 515–575 nm filter (FS15) for Rhodamine. All experiments were performed in triplicate and the minimum of 50 cells for each microscope slides were assessed to measure of the fluorescence intensity (in pixels). Student’s t-test and p ≤ 0.05 were considered statistically significant as described by Chaves et al. (2019).
Reactive Oxygen Species Evaluation
The generation of reactive oxygen species (ROS) was evaluated using dichlorofluorescein 2′, 7′-diacetate (DCFDA) (Sigma-Aldrich) as described (Ikeda and Sawamura, 2008). P. brasiliensis yeast cells were cultivated in presence or absence of copper and collected at 12 and 24 h of treatment. The cells were harvested by centrifugation of 2000 g for 5 min at 4°C, diluted in PBS 1X to 106 cells/mL. Then, 1 mL was transferred to a clean eppendorf, added of 1 μL of DCFDA and the mixture could stand for 30 min, in a dark environment. The cells were washed twice with PBS 1X and observed under fluorescence microscopy (Zeiss Axiocam MRc – Scope A1) using the 490–516 nm filter (FS09).
Enzymatic Activities and Biochemical Tests
For confirmation of differential regulation of some proteins, enzymatic activities and biochemical tests were performed. Cytochrome C oxidase (CCO) activity was performed employing the Cytochrome C Oxidase Assay Kit (CYTOCOX1-Sigma Aldrich) following the manufacturer’s instructions. The CCO activity was evaluated by a colorimetric assay based on observation of the decrease in absorbance of ferrocytochrome C at 550 nm caused by its oxidation to ferricytochrome C by CCO. Triplicates were obtained for each condition. The results were considered statistically significant at p ≤ 0.01 by Student’s t-test.
Free thiol levels were determined using the Ellman’s reagent, 5, 5′-dithio-bis-(2-nitrobenzoic acid) (DTNB – Sigma Aldrich). A total of 106 yeast cells/mL incubated in the presence or absence of copper, were centrifuged for 5 min at 8000 × g and lysed by the addition of 0.5 mL lysis buffer [50 mM Tris-Cl, 150 mM NaCl, 50 mM ethylenediaminetetraacetic acid (EDTA), pH 7.2] and glass beads in equal volume of the cellular pellet. The cells were disrupted on ice in a bead beater apparatus for 5 cycles of 30 s. After centrifugation, 100 μL of the supernatant was added to 100 μL of 500 mM phosphate buffer, pH 7.5, followed by the addition of 20 mL of 1 mM DTNB. Absorbance was measured at 412 nm using a plate reader. Triplicates were obtained for each condition, as previously described (Lacerda Pigosso et al., 2017). Results were considered statistically significant at p ≤ 0.01 by Student’s t-test.
Obtaining the Recombinant Protein Atxp and Polyclonal Antibodies Production
The vector pET32a:Pb18Atx1p was synthesized by GenOne (Rio de Janeiro, Brazil). Briefly, the Atx1 sequence from P. brasiliensis (PADG_02352) was codon optimized for Escherichia coli, and cloned into BamHI restriction site from vector pET32a (Novagen, Merck-Millipore). For bacterial transformation, 150 ng of DNA from plasmid pET32a:Pb18Atx1p was added to the E. coli BL21 competent cell suspension and kept on ice for 1 min. The cells were transferred to an electroporation cuvette and subject to 1800 -Volt electric field to allow entry of the plasmid into the cell. After electroporation, cells were resuspended in 900 μL Luria-Bertani (LB) medium and incubated at 37°C while shaking for 1 h. Aliquots were plated in LB medium containing 100 μg/mL ampicillin for selection of the transformed bacteria. Recombinant protein induction was performed by addition of Isopropyl-β-D thiogalactopyranoside (IPTG) at 0.1 mM. Protein extracts were submitted to 12% SDS-PAGE followed by coomassie blue staining. The bacteria lysis was performed by adding 500 μg/mL lysozyme for 1 h, under constant stirring, at room temperature and sonication in 5 pulses of 10 min. The supernatant from the solubilization process was used for purification of the recombinant Atx1p by nickel column affinity chromatography.
Purified P. brasiliensis recombinant Atx1p was inoculated into 6–8-week-old male BALB/c mice to produce polyclonal antibodies. The purified protein was cut from the polyacrylamide gel, macerated in grinder with 2 mL of PBS 1 X, and 200 μL of the solution were applied to the mice. Three immunizations were performed, 15 days apart, intraperitoneally. Fifteen days after the last immunization, the animals were anesthetized with sodium thiopental (40 mg/kg) and lidocaine hydrochloride (2 mg/kg), and blood puncture was performed. The animals were then euthanized by cervical dislocation. Sera collected from unimmunized mice were used as negative control (preimmune). All procedures were approved by the Animal Use Ethics Committee of the Federal University of Goiás (CEUA-PRPI-UFG), under protocol number 092/17.
Western Blotting and Immunofluorescence Assays
Proteins in SDS-PAGE were transferred to a nitrocellulose membrane that was then incubated with polyclonal anti-Atx1p antibodies at 1:2000 dilution, for 2 h at room temperature. After washing, the membranes were incubated with peroxidase-coupled mouse anti-IgG secondary antibody (1:1000 dilution). The reaction was revealed by chemiluminescence with the ECL Western Blotting substrate kit (GE Healthcare). Negative control was obtained with preimmune mice serum (1: 500 dilution).
For immunofluorescence, 106 yeast cells/mL were fixed in ice cold pure methanol for 3 h at 20°C. After washing with PBS 1 X, part of the fixed cells was permeabilized with 0.25% triton X-100 (Sigma-Aldrich) for 20 min. Subsequently, cells were incubated for 30 min at room temperature, in the dark in blocking buffer containing 3% (w/v) bovine serum albumin (BSA-Sigma), 0.2% (v/v), tween 20 in PBS, followed by incubation with the primary anti-Atx1p polyclonal antibodies at 1:500 dilution, for 1 h. Subsequently there is an addition of fluorescein isothiocyanate-labeled mouse secondary antibody- FITC (Sigma) at 1:750 dilution, for 1 h. Cells were washed three times with PBS 1 X. Images were taken in bright field and at 450–490 nm for visualization of FITC fluorophore, using the Axio Scope A1 fluorescence microscope. Digital images were acquired using AxionVision software (Carl Zeiss AG, Germany).
Results
P. brasiliensis Survival in ATP7a Silenced Macrophages
To investigate the influence of copper on the survival of P. brasiliensis inside J774A.1 macrophage, we knocked down the P-type copper ATPase pump (ATP7a) of this defense cell. This protein is responsible for translocation of copper from the Golgi to phagolysosome during infection (White et al., 2009). ATP7a silenced J774A.1 macrophage cell line was obtained. The transfection of host cells with siRNAs against ATP7a transcripts promoted a 15 times reduction in copper ATPase mRNA levels (Figure 1A) and such treatment did not affect macrophage viability (Figure 1B). The data analyzes demonstrates that in ATP7a-silenced macrophages, the number of recovered CFU from P. brasiliensis was significantly reduced when compared to control macrophages (Figure 1C).
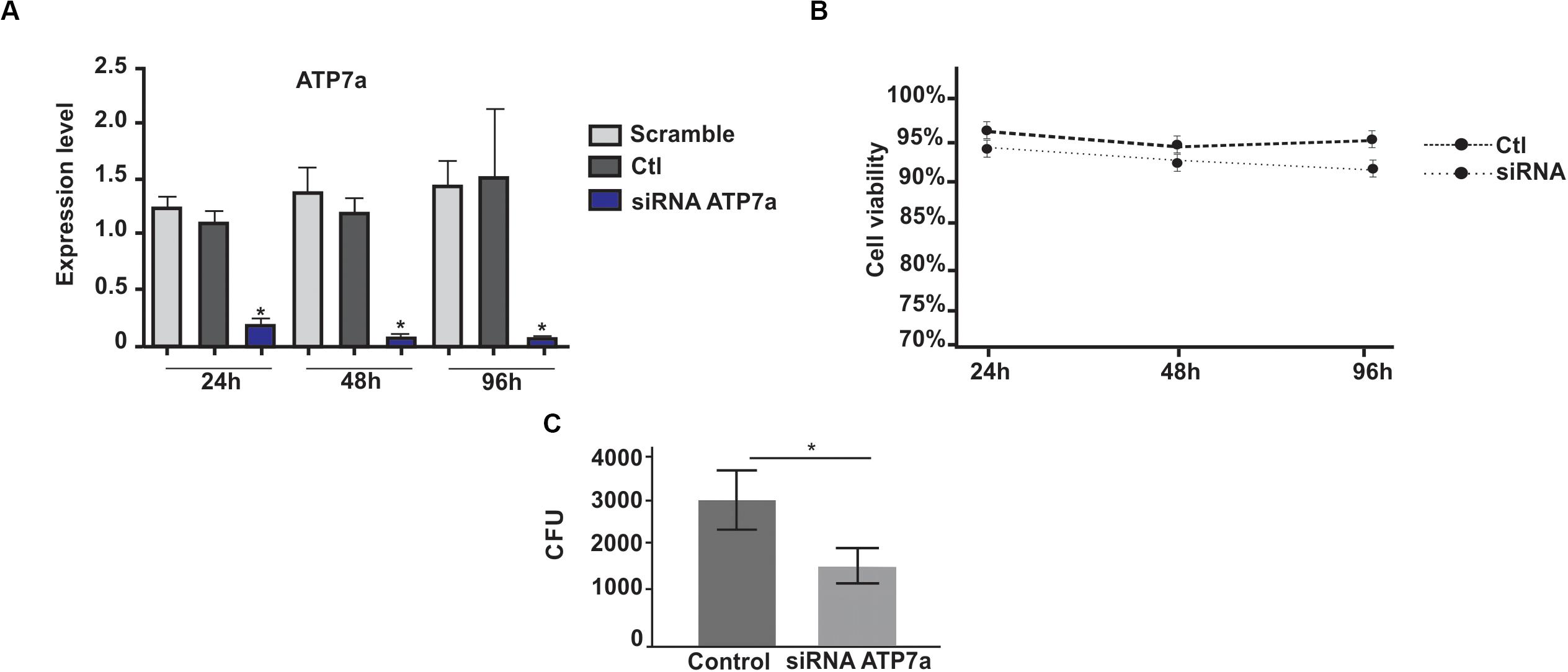
Figure 1. Recovering of P. brasiliensis yeast cells after 24 h infection in silenced ATP7a macrophages. (A) Transcription levels of ATP7a after 24, 48, and 96 h of transfection with ATP7a siRNAs. Scramble – macrophages transfected with a scramble RNA; Ctl – non- transfected macrophages; siRNA – ATP7a- silenced macrophages. (B) Viability of macrophage cells silenced for ATP7a. (C) The cells of P. brasiliensis (Pb18) were used for infection during 24 h in wild type macrophages or silenced for ATP7a. After the infection period, the yeast cells were recovered from both macrophages and the number of fungi was determined by counting Colony Forming Units (CFU). Data are expressed as mean ± standard error (represented using error bars). A statistically significant difference was determined by Student’s t-test, (*) represents p ≤ 0.05. Control (non-silenced macrophages), SiRNA for ATP7a protein (ATP7a silenced macrophages).
Fungal Viability and Analysis of Expression of the Copper Transporter Ctr3 Transcript in Response to in vitro Copper Deprivation
To determine the impact of copper deprivation on fungal viability, flow cytometry was employed. The results demonstrated that, although the fungus was deprived of copper, cellular viability was not affected within the first 24 h (Figure 2A). After 48 h of growth in MMcM medium there was a decrease in the fungus viability, probably due to the consumption of nutrients in the liquid medium after several h of cultivation. The expression profile of the gene encoding the high affinity copper transport Ctr3p in P. brasiliensis was evaluated at different times of copper deprivation. After 24 h of deprivation high induction of Ctr3 was observed (Figure 2B), indicating that the fungus was in copper deprivation.
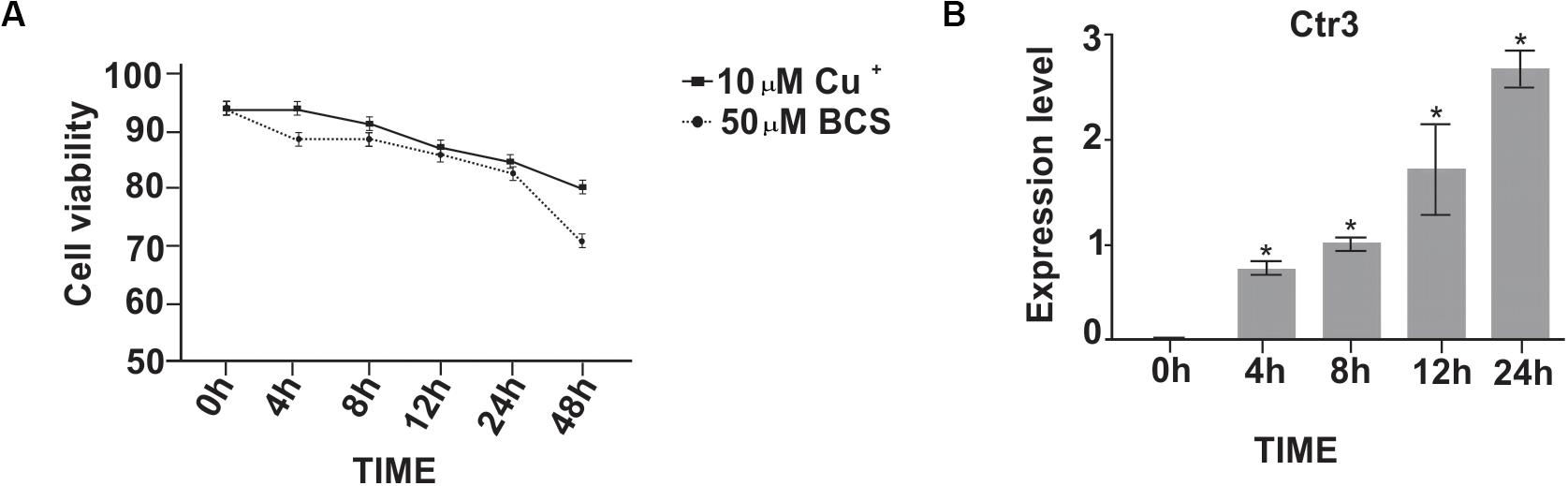
Figure 2. Viability of P. brasiliensis at different time points of copper deprivation and expression analysis of Ctr3 gene. (A) The viability of yeast cells of P. brasiliensis at time-points of copper deprivation was analyzed. The yeast cells were copper deprived by the addition of 50 μM bathocuproinedisulfonic (BCS); 10 μM of CuSO4 was added to control. The viability was determined using flow cytometer (BD® Accuri C6 Flow Cytometer) and at least 10,000 events were analyzed. (B) Expression analysis of the Ctr3 gene in yeast cells during copper deprivation: Relative levels of expression of the Ctr3 gene (Genbank PADG_05084) after treatment with 50 μM BCS at different time-points. Gene expression values were normalized using the expression values of the transcript coding for L34 (GenBank accession number (PADG_04085) Data are expressed as mean ± standard deviation from triplicates. *Statistically significant (p < 0.05).
Proteomic Analysis of Yeast Cells Submitted to in vitro Copper Deprivation
Proteomic response of yeasts cells subjected to copper deprivation was performed. In this work the iTRAQ method was used to perform proteomic analysis. This method allows to analyze simultaneously multiple samples and has been extensively used in innumerous quantitative proteomics studies (Ruppen et al., 2010). Thus, isobaric tag labeling- iTRAQ permitted us to perform the quantitative comparison of P. brasiliensis proteome in two different conditions. Using this method, a total of 164 proteins were differentially expressed in copper deprivation, considering p ≤ 0.05 and were categorized according to their cellular functions using the Functional Catalog (FunCat2). The main biological processes regulated by copper deprivation include metabolism (22%), energy (17%), and protein fate (15%) (Supplementary Figure 1). Among the differentially expressed proteins, 155 increased in abundance (Supplementary Table 2) and 09 decreased in abundance (Supplementary Table 3).
Beta Oxidation and Glyoxylate Cycle Increase in Yeast Cells During in vitro Copper Deprivation
Proteins related to the pathways, as follows: beta-oxidation: acetyl-CoA acyltransferase (PADG_00382 and PADG_01687), acyl-CoA dehydrogenase (PADG_02852), carnitine O-acetyltransferase (PADG_07023); to the glyoxylate cycle: isocitrate lyase (PADG_04709), aconitate hydratase (PADG_11845) malate dehydrogenase (PADG_08059), and to the TCA cycle: isocitrate dehydrogenase (PADG_04249) were increased, strongly connecting beta oxidation and the glyoxylate cycle in copper-deprived yeast cells (Figure 3).
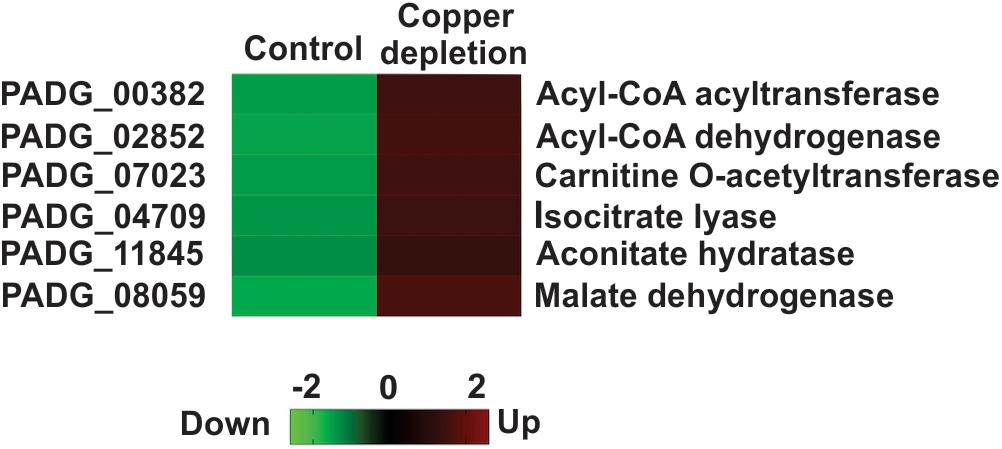
Figure 3. Metabolic pathways regulated in P. brasiliensis in response to in vitro copper deprivation. Heat map showing increased quantity of proteins from beta oxidation, TCA and glyoxylate cycle in copper deprived P. brasiliensis yeast cells. Colors on the heat map indicate differential accumulation; red increased and green decreased. The heat map was constructed by GraphPad Prism software. Copper deprivation (50 μM BCS) and control (10 μM of CuSO4).
Oxidative Stress Response Increases in Yeast Cells During in vitro Copper Deprivation, Due to Accumulation of ROS
An important enzyme related to the detoxification of P. brasiliensis, Zn/Cu superoxide dismutase (Sod1p) (PADG_07418), was decreased. On the other hand, the following were accumulated: thioredoxin (PADG_05504), Mn/Fe superoxide dismutase (Sod5p) (PADG_01954) and glutathione-S-transferase (GST) (PADG_02526). This suggests that copper deprivation promoted an increase in the formation of reactive radicals. Furthermore, we identified increased levels of 6-phosphogluconate dehydrogenase (PADG_03651), enzyme of the phosphate pentose pathway (Figure 4A). This may be related to the production of NADPH, an important reducing agent that increases the efficiency of detoxification enzymes. A decrease abundance of Sod1p, as well as an increase of detoxification enzymes such as thioredoxin, GSTp and Sod5p suggest that copper deprivation has affected the control of free radicals in the cell. The evaluation of thioredoxin provided increased enzyme activity during copper deprivation (Figure 4B). In addition, ROS detection by fluorescence microscopy using 2,7 dichlorofluorescein marker s, when compared to control, demonstrated increase of ROS after 12 and 24 h in copper-deprived yeast cells compared to control (Figure 5). In this way, data suggest that copper deprivation induced oxidative stress in yeast cells of P. brasiliensis, which account for the increase in the cell’s response.
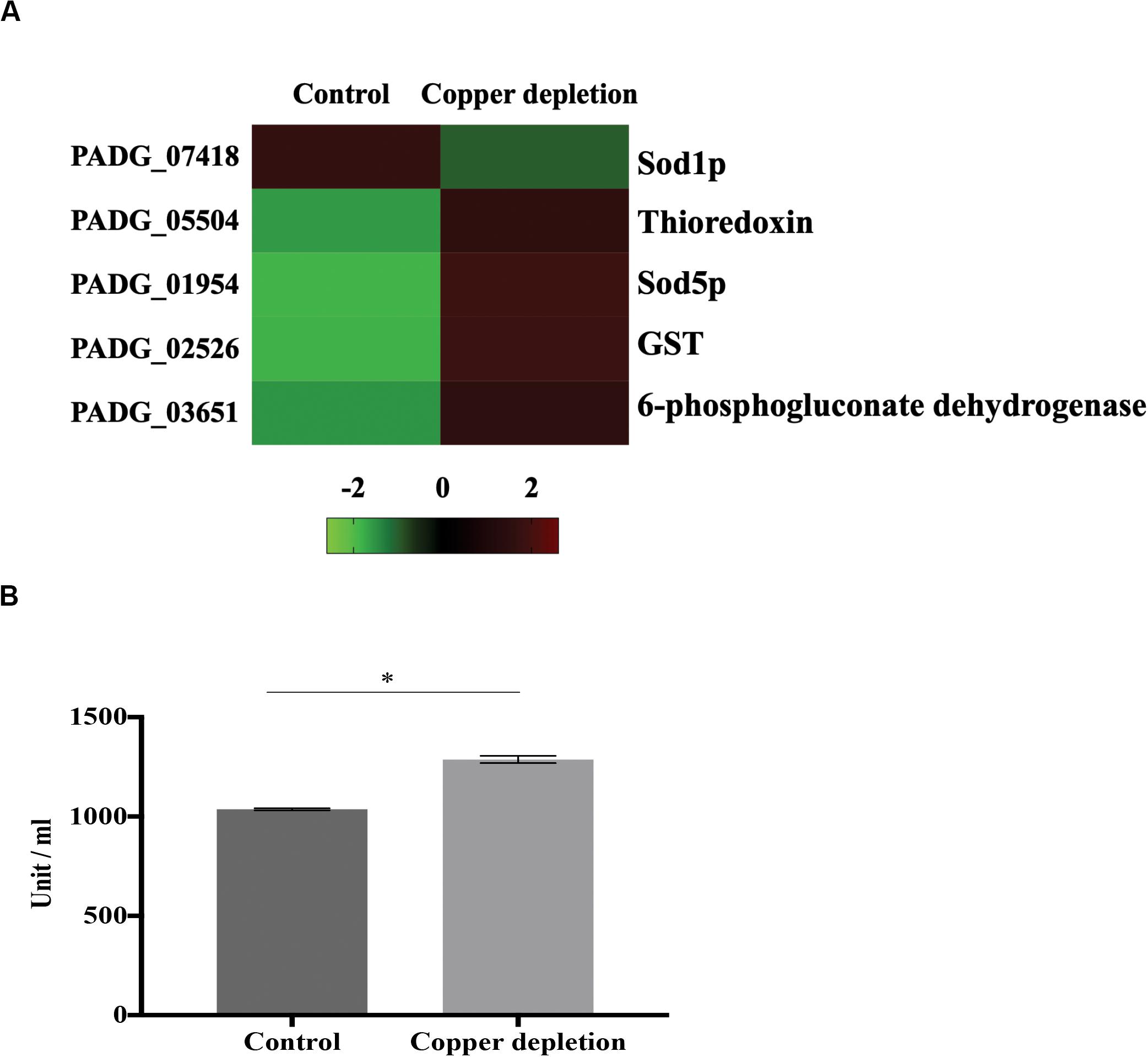
Figure 4. P. brasiliensis activates the response to oxidative stress during in vitro copper deprivation (A) Proteins involved with response to oxidative stress were increased during this metal deprivation. The proteins involved with this response are demonstrated on the heat map; with increased proteins indicated by red color and decreased by green color. Only Sod1p decreased by copper deprivation. (B) To determine the enzymatic activity of thioredoxin, yeast cells were treated with 50 μM BCS or 10 μM of CuSO4 (control) for 24 h The data were obtained by determining the thiol reduction level in yeast cells, cultivated in the presence or absence of copper. The absorbance was measured at 412 nm. Data are expressed as mean ± standard error (represented using error bars), (∗) represents p ≤ 0.05.
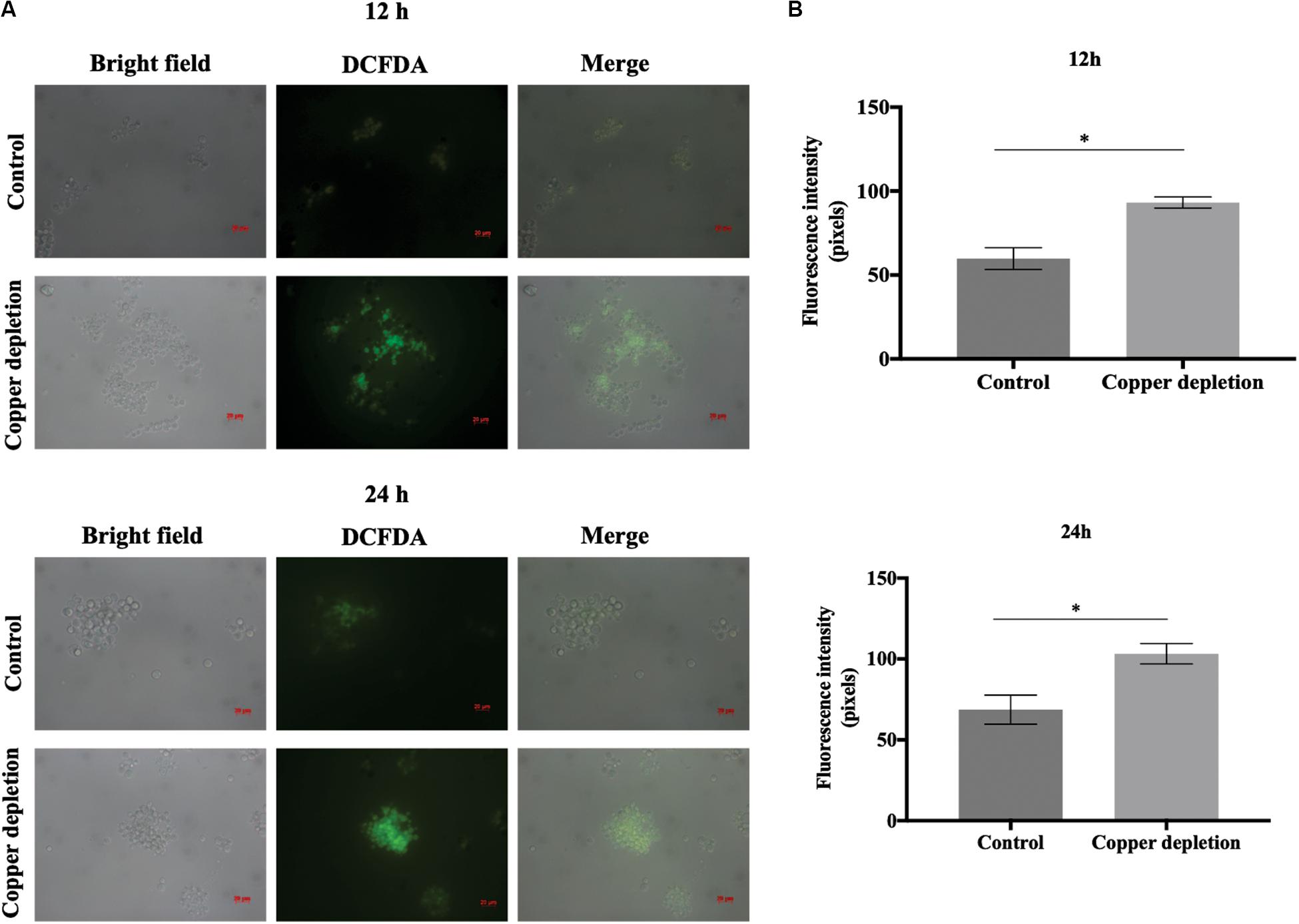
Figure 5. Copper deprivation results in an increase of reactive oxygen species (ROS) (A) Detection of ROS during 12 and 24 h of copper deprivation Yeast cells were grown with and without copper, labeled with dichlorofluorescein 2′, 7′-diacetate (DCFDA) and analyzed by fluorescence microscopy. 50 μM BCS indicated copper depletion; control: 10 μM CuSO4. Bars indicate the standard deviation (B) Fluorescence intensity graphs. The minimum quantity of 50 cells per triplicate were used to construct the graphs. All representative images were magnified 400x. The data for the fluorescence intensity evaluation were obtained through the AxioVision Software (Carl Zeiss). *This demonstrates a significant difference between the samples with the p-value of ≤ 0.05. The error bars represent the standard deviation of the samples in triplicates. Control (10 μM of CuSO4) and copper deprivation (50 μM BCS).
The Metabolism of the Cell Wall Increases During in vitro Copper Deprivation
Enzymes involved in cell wall synthesis, UDP-N-acetylglucosamine pyrophosphorylase (PADG_04312), UDP-galactopyranose mutase (PADG_00912) and 1,4-alpha-glucan branching enzyme (PADG_12426), increased in the copper-deprived yeast cells (Figure 6A). The images shown in Figure 6B represent an increased fluorescence of yeast cells in copper deprivation when stained with the glucan-binding dye, aniline blue. The fluorescence of chitin was similar in yeast cells that were either deprived, or not, of copper. In this sense, fluorescence intensity quantification confirms that only the amount of glucan increased (Figure 6C). Possibly glucose produced by gluconeogenesis could be used to produce polymers involved in the remodeling the fungal cell wall.
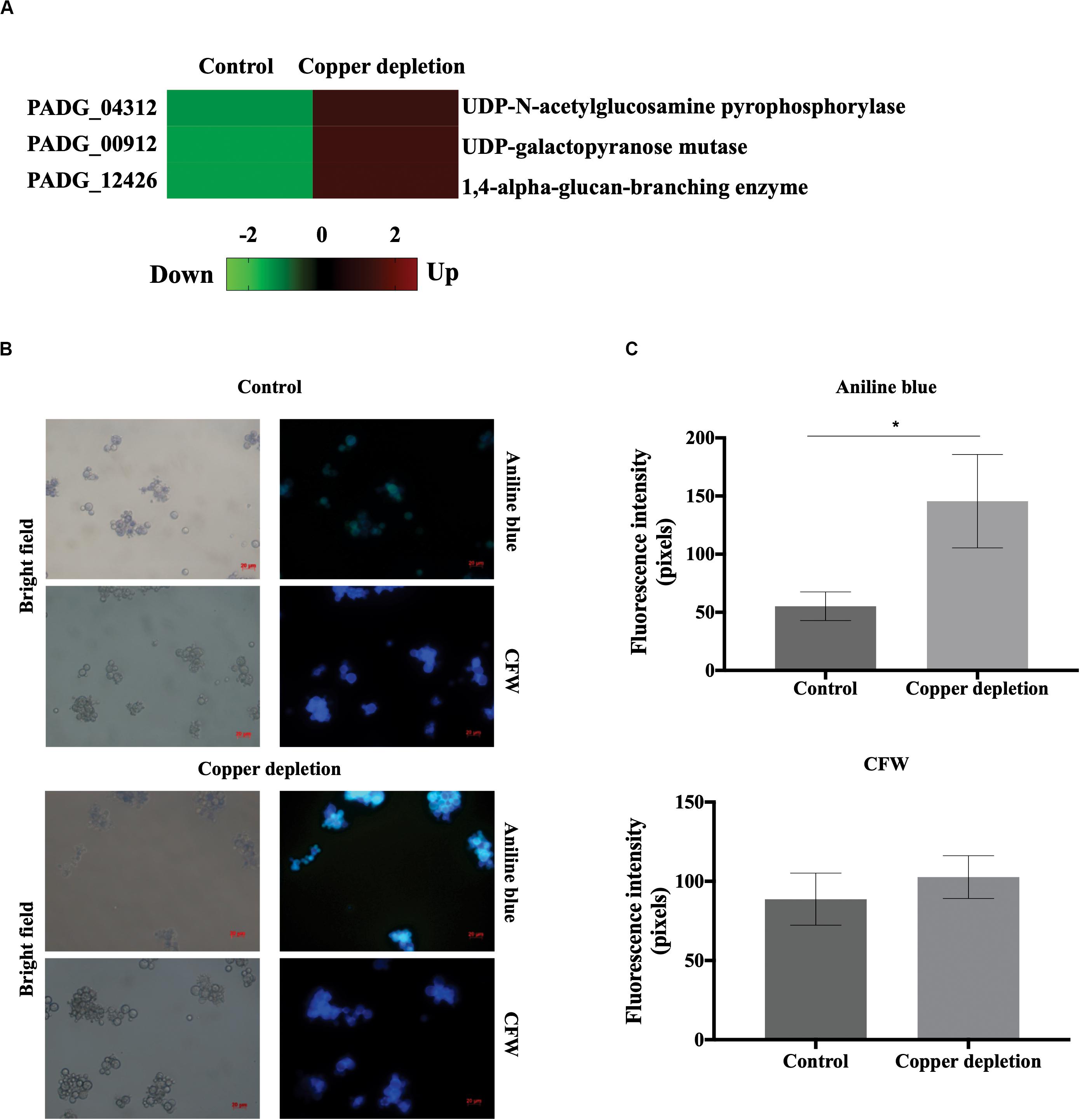
Figure 6. Evaluation of the cell wall metabolism in the yeast cells of P. brasiliensis in copper deprivation. (A) Heat map showing increased accumulation of proteins related to the cell wall metabolism during copper deprivation, with increased (red color) and decreased (green color) proteins. (B) Yeast cells were incubated in medium containing 10 μM of CuSO4 (control) or 50 μM BCS (copper depletion) during 24 h. For analysis of the amount of glucan and chitin the yeast cells were stained with aniline blue and Calcofluor White (CFW) respectively and analyzed by fluorescence microscopy. Bars represent the standard deviation. (C) Fluorescence intensity in control and copper-deprived yeast cells, both labeled with aniline blue and CFW. To determine significant differences in each condition, pixels intensity values were obtained from triplicates from at least 50 cells, each *indicated with p-value of ≤ 0.05. All representative images were magnified 400x, control (10 μM of CuSO4) and copper deprivation (50 μM BCS).
In Vitro Copper Deprivation Induces Alternative Oxidase and Decreased Mitochondrial Activity
The enzyme alternative oxidase (Aoxp) (PADG_03747) increased in yeast cells when in copper deprivation (Supplementary Table 2). Aoxp is accumulated when there is a compromise in activity of cytochrome c oxidase (Coxp) activity (Horne et al., 2001). Copper is a necessary cofactor for Coxp activity. In this context, the absence of copper could activate the alternative system, mediated by Aoxp, to compensate for a possible loss of Coxp activity due to the reduction of copper levels. In fact, alternative oxidase (PADG_03747) accumulated. Considering the important role of copper in Coxp activity, we hypothesized that the increase in Aoxp accumulation should be related to decreased Coxp activity caused by copper deprivation. In this way, two strategies were performed. The first analysis was to evaluate the enzymatic activity of Coxp. When in comparison to control, activity of Coxp reduced in copper deprivation (Figure 7A). Then, we evaluated the activity of the electron transport chain, by fluorescence microscopy. Analysis of rhodamine fluorescence demonstrated mitochondrial electron transport chain repression in copper depletion (Figures 7B,C). On the other hand, mitochondrial integrity was not compromised, since mitotracker labeling did not show significant differences between treated and control (Figures 7B,C). This data corroborates that copper deprivation could repress electron transport chain, without affecting the integrity of mitochondria.
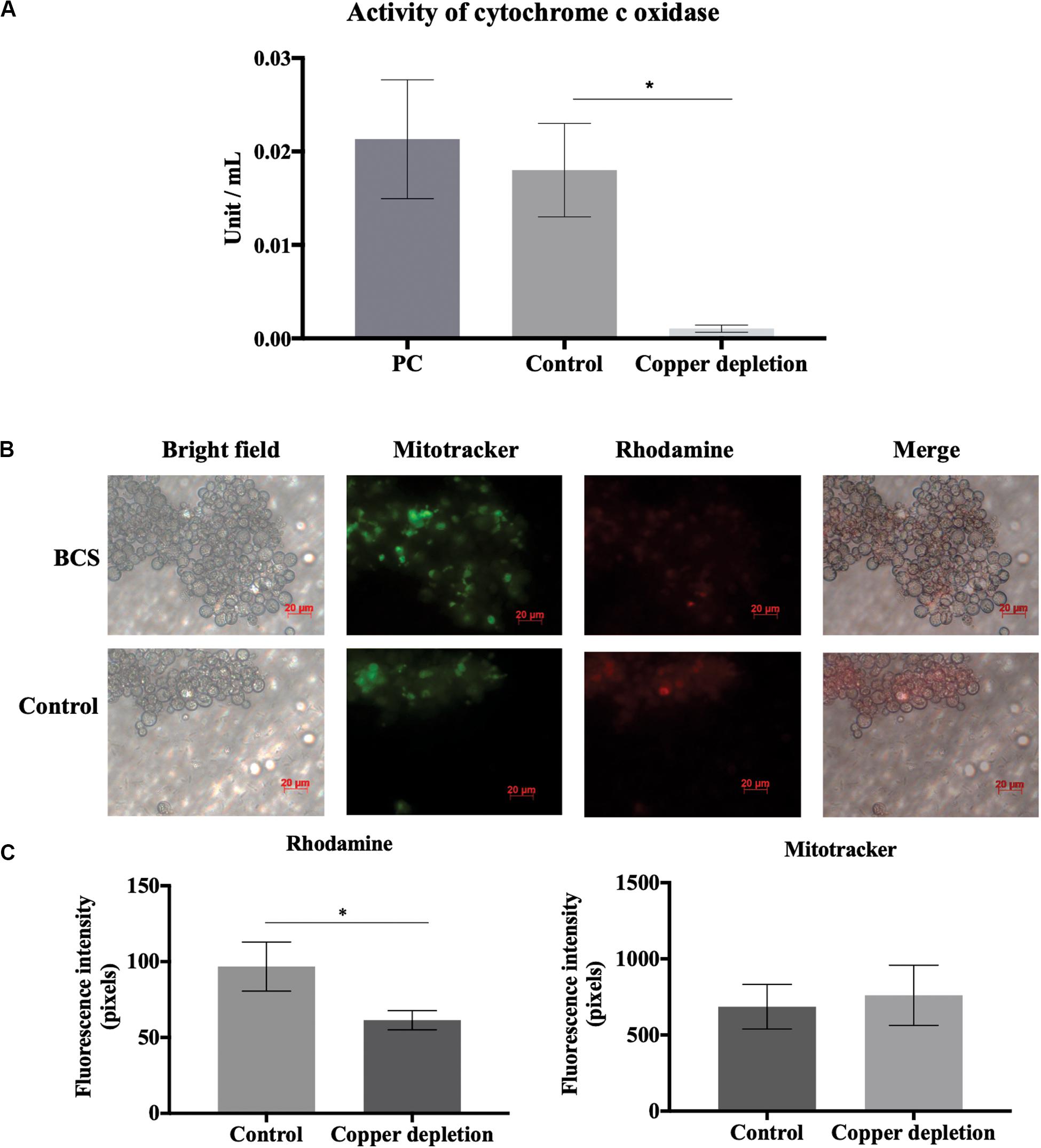
Figure 7. Activity of cytochrome c oxidase (Coxp) and oxidative phosphorylation are regulated by copper deprivation. (A) Yeast cells of P. brasiliensis were incubated in the presence and absence of copper, and the enzymatic activity of Coxp was evaluated. PC represents the positive control of the Assay Kit (Sigma-Aldrich – CYTOCOX1). Bars represent the standard deviation. (B) Yeast cells of P. brasiliensis were labeled with mitotracker and rhodamine for verification of integrity and mitochondrial activity, respectively, by fluorescence microscopy. (C) Fluorescence intensity graph of yeast cells labeled with mitotracker and rhodamine. The data for fluorescence intensity evaluation were obtained through the AxioVision Software (Carl Zeiss). The values of fluorescence intensity (in pixels) and the standard error of each analysis were used to plot the graph. Data are expressed as mean ± standard error (represented using error bars), (∗) representing p ≤ 0.05. All representative images were magnified 400x, copper deprivation (50 μM BCS) and control (10 μM of CuSO4).
The Cytoplasmic Atx1p Copper Chaperone Is Induced in Copper Deprivation: Additional Confirmatory Assays
Proteomic analysis demonstrated that the expression of a copper chaperone Atx1p (PADG_02352), increased in copper deprivation (Supplementary Table 2). Studies described that Atx1p plays a critical role in detoxification of reactive oxygen species, which increased in P. brasiliensis during in vitro copper depletion. In view of this affirmative we performed gene expression analysis by qRT-PCR, which confirmed proteomic data (Figure 8A). Using polyclonal anti-Atx1p antibodies (Supplementary Figure 2) immunofluorescence of Atx1p in P. brasiliensis yeast cells was performed. Fluorescence was observed only when permeabilizing the cell, indicating that Atx1p presents cytoplasmic localization in P. brasiliensis (Figure 8B).
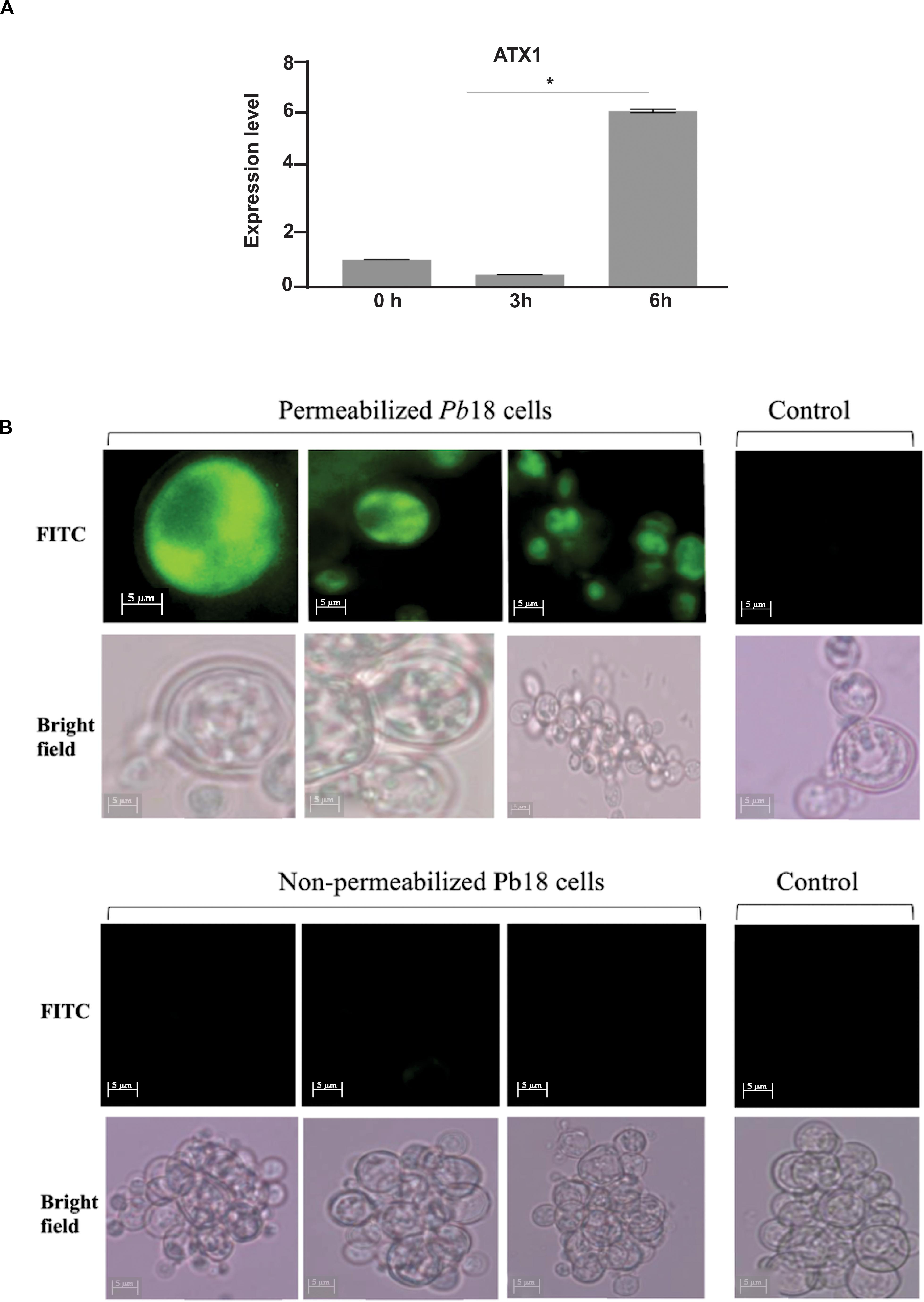
Figure 8. Expression of the transcript and cell localization of Atx1p in P. brasiliensis. (A) Expression analysis of Atx1 in yeast cells during copper deprivation. Relative levels of expression of the Atx1 gene in treatment with 50 mM BCS at different time points. Gene expression values were normalized using the expression values of the transcript coding for L34 (GenBank accession number (PADG_04085). *Statistically significant (p < 0.05). (B) Atx1p immunofluorescence. The immunofluorescence assay was performed with triton X-100 permeabilized and non-permeabilized cells, incubated with anti-Atx1p polyclonal antibodies for 1 h, followed by incubation with the isothiocyanate-labeled mouse anti-IgG secondary antibody fluorescein – FITC for 1 h. Fluorescence was only observed in permeabilized cells. Images were taken in bright field and at 450–490 nm wavelength for viewing FITC fluorophore at 1000x magnification using the Axio Scope A1 fluorescence microscope. Digital images were acquired using AxionVision.
Discussion
During host infection, pathogenic fungi may find different copper concentrations on dependence of the infected tissue. C. neoformans finds high levels of copper in the lungs at the onset of infection, whereas reduced levels are found during brain infection (Sun et al., 2014). In our study, we infected macrophages silenced for expression of type P copper ATPase (ATP7a) which pumps copper into the phagolysosome. After CFU counting, we noticed, compared to non-silenced macrophages, a lower fungal recovery. Copper accumulation inside macrophages is a strategy used to limit the growth of fungi such as Aspergillus fumigatus (Song et al., 2019). In this sense, A. fumigatus had an increased survival during infection in ATP7a-deficient zebrafish (Wiemann et al., 2017). In contrast, the data obtained in this work suggest that P. brasiliensis is deprived of copper when inside macrophages and that silencing of ATP7a enhanced this deprivation, further compromising the fungus survival. This finding is very surprising since is not in agreement with previous published data for microbial invaders (White et al., 2009; Ladomersky et al., 2017; Wiemann et al., 2017). The reduction on fungal survival observed upon ATP7a silencing could be a result of an intensified copper limiting environment. In agreement we have previously demonstrated that expression of Crt3 gene is increased in yeast cells infecting macrophages (Dantas et al., 2009). We cannot exclude a hypothetical decreased phagocytosis ability of silenced macrophages. The latter hypothesis is less likely since decreased levels of ATP7a do not cause unrelated effects in macrophages (White et al., 2009). However, additional experiments should be performed to clarify this fact.
Copper is a micronutrient necessary for the activity of enzymes that participate in important processes. These important processes are related to detoxification (Peña et al., 1998), respiration (Horng et al., 2004) and capture of metals (Georgatsou et al., 1997). Therefore, the absence of copper may compromise the fungus’ ability to adapt, survive and infect the host (Ding et al., 2014b; De Oliveira et al., 2014; Broxton and Culotta, 2016). Through a proteomic approach, we identified differentially accumulated proteins related to beta oxidation, glyoxylate cycle, pentose phosphate pathway and cell wall polymer synthesis, as well as others compromised with cellular respiration and detoxification.
Metabolic reprogramming by activation of alternative pathways of carbon consumption is a mechanism characterized in P. brasiliensis; this way it can survive in hostile conditions imposed by the host (Parente-Rocha et al., 2015; Chaves et al., 2019). Our data suggest that in copper deprivation occurs a shift of metabolism toward beta oxidation and glyoxylate cycle. This is due to a significant increase in enzymes. In this sense, during the infection of macrophages by P. brasiliensis, glucose availability is low and, for the survival of this fungus, oxidation of fatty acids is its energy source (Parente-Rocha et al., 2015). In interferon-gamma primate or non- macrophages, activation of the glyoxylate cycle, is a common strategy used by this fungus (Chaves et al., 2019). The gene induction o of the glyoxylate cycle and beta-oxidation is the strategy employed by P. brasiliensis during infection in mouse lung; this is also described during the infection of A. fumigatus conidia in neutrophils (Sugui et al., 2008; Lacerda Pigosso et al., 2017). Therefore, the activation of those alternative pathways for energy production could be a mechanism used for P. brasiliensis when in response to copper deprivation in vitro and in vivo.
In view of the protein identification related to cell wall remodeling- the UDP-N-acetylglucosamine pyrophosphorylase (PADG_4312), the UDP-galactopyranose mutase (PADG_00912) and the 1,4-alpha-glucan-branching enzyme (PADG_12426)- we suggest that cell wall remodeling could occur in P. brasiliensis when in copper deprivation. In fact, by fluorescence microscopy, we identified an increase of glucans in the cell wall of copper-deprived P. brasiliensis. The remodeling of the cell wall is also described during the infection of macrophages by P. brasiliensis, in which there is an increase of the glucan and chitin synthesis. This can allow for the multiplication and fungal growth within these defense cells (Chaves et al., 2019). Interestingly, in copper depletion, the synthesis of cell wall polymers was increased, and the glucose produced through the intermediates of glyoxylate cycle could be used to remodel the cell wall.
The ability of P. brasiliensis in copper depletion to defend against reactive oxygen species seems affected in a critical way. Indeed, our results show that there was an increase in reactive oxygen species in 12 and 24 h of copper deprivation. Members of the Paracoccidioides genus present six isoforms of superoxide dismutases (Sod1-6) in their genomes (Tamayo et al., 2016). Sods 1, 3, and 4 present Cu/Zn domains. Sod3p is extracellularly located and Sod4p is cytoplasmic and both present low level of transcripts. The other Sods 2, 5, and 6 present Fe/Mn domains. The isoforms 2 and 5 are mitochondrial, while Sod6p is cytosolic. All the isoforms transcripts are poorly expressed in mycelia and yeast cells in the genus Paracoccidioides (Tamayo et al., 2016). In our analysis Sod1 decreased and Sod 5 increased in yeast cells during copper depletion, accounting for Cu/Zn domains in Sod1p. Additionally, thioredoxin and GST increased in abundance in copper depletion.
The 6-phosphogluconate dehydrogenase (PADG_03651) related to the production of NADPH via the pentose phosphate pathway also accumulated. NADPH plays an important role in maintaining yeast cell antioxidant activity (Kim et al., 2011). Fungi such as P. lutzii and S. cerevisiae, under oxidative stress requires NADPH, produced by the pentose phosphate pathway, due to its reducing power (Kim et al., 2011; Grossklaus et al., 2013). Pseudomonas fluorescens, requires NADPH when the organism undergoes oxidative stress (Singh et al., 2007).
We also identified increase in the chaperone Atx1p in proteomic analyzes. In S. cerevisiae Atx1p is a cytosolic protein and facilitates the delivery of copper of the cell surface copper transporter (Ctr1p) to Ccc2p and Fet3p in the secretory pathway (Lin and Culotta, 1995). Therefore, the location of this chaperone in P. brasiliensis was analyzed since the compartment in which Atx1p is described directly influences the delivery of intracellular copper, and consequently the availability of this micronutrient inside the cell, influence on the expression of genes and proteins. In addition, Atx1p also may act in the response to oxidative stress, and presents important role in S. cerevisiae, protecting the fungal cell against superoxide anion and hydrogen peroxide toxicity (Lin and Culotta, 1995; Lin et al., 1997).
Although there was a significant increase in some proteins of the respiratory chain, we detected by biochemical assays, that Cox1p activity was strongly reduced during copper deprivation. The role of copper as a cofactor of the enzyme Coxp indicates that changes in the levels of this micronutrient might affect the respiratory process. The presence of this metal ensures the transport of electrons and the reduction of molecular oxygen to water from the respiratory chain complex IV (Horne et al., 2001). In S. cerevisiae, copper depletion caused by deletion of the Mac1 results in reduction of the fungus respiratory capacity (Jungmann et al., 1993). Additionally, an alternative oxidase Aoxp was increased in this work. These results suggest that copper deprivation led to impaired respiratory activity in P. brasiliensis and that positive Aoxp regulation would compensate for this loss. This enzyme is described in plants and fungi as an important component acting as an alternative respiratory route during impairment in Coxp activity (Horne et al., 2001; Vishwakarma et al., 2015). The ascomycete Podospora anserina shows induction of an alternative pathway, mediated by the alternative oxidase when in copper deprivation (Borghouts et al., 2002).
Aoxp also functions as a detoxifying agent in mitochondria in fungi, as well as in the Paracoccidioides genus (Hernández et al., 2015; Smith et al., 2017). A relationship between efficiency of the respiratory process, production of ROS and role of superoxide dismutase and Aoxp was described (Broxton and Culotta, 2016). Aoxp contributes to the adaptation of C. albicans to copper deprivation, compensating for the deficiencies of Coxp activity but also for the loss of Sod1p activity by minimizing mitochondrial ROS (Broxton and Culotta, 2016).
We provide evidence that during copper deprivation in P. brasiliensis an alternative oxidase form of respiration is induced that is not coupled to ATP synthesis but maintains mitochondrial superoxide at low levels putatively compensating for decrease in Sod1p. Considering the low activity of Sod1p, both Sod5p and Aoxp may act to compensate for the loss of efficiency in the mitochondrial detoxification process and at the same time compensate the respiratory activity of the fungus. Further, thioredoxin and GST could compensate the decrease in Sod1 activity in the cytosol, a schematic drawing is depicted in Figure 9. Therefore, in the present study we proposes an adaptive response of P. brasiliensis to in vitro copper deprivation, a condition putatively faced by the fungus during infection, at least in macrophages. Metabolic shift toward beta-oxidation and glyoxylate cycle, ROS detoxification and respiration seem to be the most affected processes by the deprivation of this metal. In synthesis, copper deprivation compromised the activity of Sod1p, putatively affecting detoxification. This probably lead to increase in thioredoxin, GST, Sod5p, and Atx1p in addition, to up-regulation of the pentose phosphate pathway enzyme, 6-phosphogluconate dehydrogenase, related to the production of NADPH probably enhancing the response to the increase in ROS generated by copper deprivation.
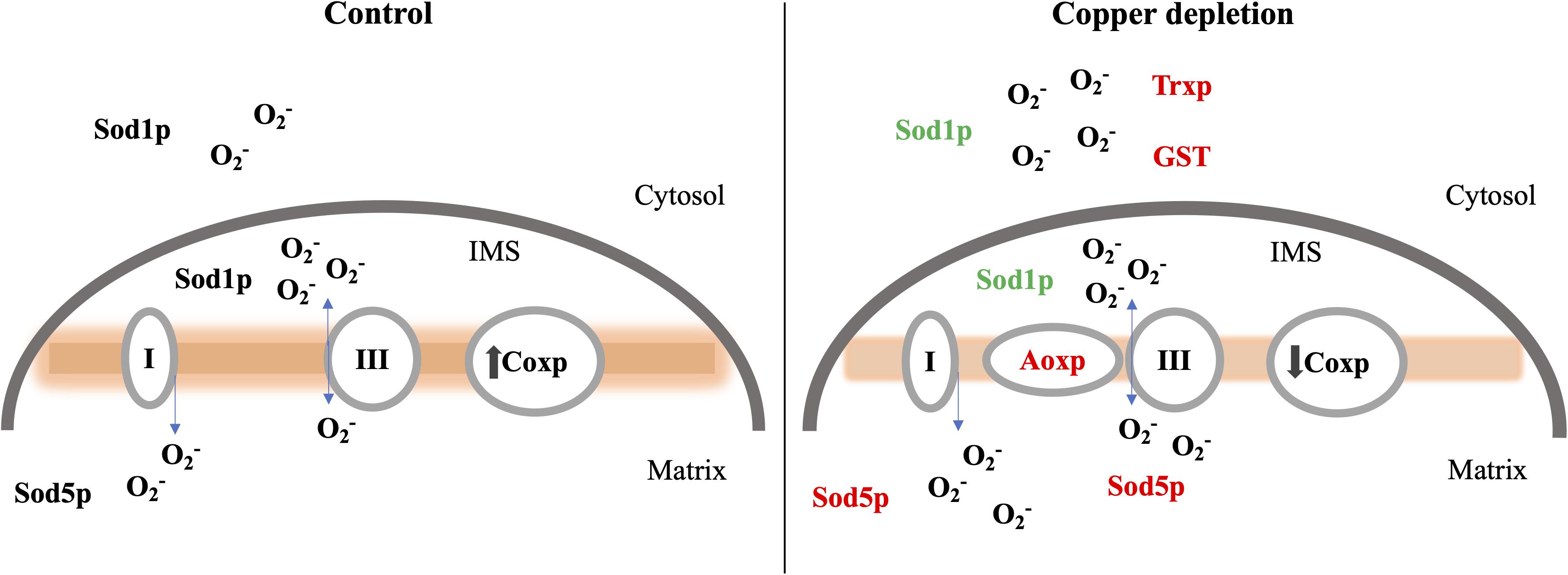
Figure 9. Changes in cytosolic and mitochondrial protein expression in response to copper deprivation. During copper deprivation the decrease of Sod1p in the cytosol and mitochondrial intermembrane space (IMS) as well as the decrease in cytochrome c oxidase (Cox1p) activity, promotes increase in the activity of the alternative oxidase enzyme (Aoxp) as well as an increase in superoxide dismutase Mn/Fe (Sod5p), thioredoxin (Trxp) and glutathione S transferase (GST). Proteins in red were increased; in green represents the protein with reduced abundance during copper deprivation.
Data Availability Statement
The datasets presented in this study can be found in online repositories. The names of the repository/repositories and accession number(s) can be found at: http://www.peptideatlas.org/, PASS01563.
Ethics Statement
The animal study was reviewed and approved by the Animal Use Ethics Committee of the Federal University of Goiás (CEUA-PRPI-UFG), under protocol number 092/17.
Author Contributions
CA and GP conceived and designed the experiments. GP, JP, JC, CM, GT, and AC performed the experiments. GP, AB, WF, CR, MP, MS, and CA analyzed and interpreted the data. CA contributed to reagents and materials. GP, JC, and CA analyzed the data and wrote the manuscript. All authors contributed to the article and approved the submitted version.
Funding
This work was carried out at Laboratório de Biologia Molecular (LBM) of Universidade Federal de Goiás (UFG) and was funded by Conselho Nacional de Desenvolvimento Científico e Tecnológico (CNPq) and Fundação de Amparo à Pesquisa do Estado de Goiás (FAPEG). This work was part of the National Institute of Science and Technology (NICT) of host-pathogen interaction (HPI). GP was a fellow from FAPEG. JP was a fellow from FAPEG and Coordenação de Aperfeiçoamento de Pessoal de Nível Superior (CAPES). CA, MP, and CR were fellows from CNPq. Financing Agency for Studies and Projects (FINEP/CT-INFRA, grants 0439/2011 and 0694/2013) also funded this work.
Conflict of Interest
The authors declare that the research was conducted in the absence of any commercial or financial relationships that could be construed as a potential conflict of interest.
Supplementary Material
The Supplementary Material for this article can be found online at: https://www.frontiersin.org/articles/10.3389/fmicb.2020.01834/full#supplementary-material
FIGURE S1 | Functional classification of differentially expressed proteins in yeast cells after 24 h of copper deprivation. All identified proteins were classified according to Functional Catalog (FunCat2). For the experiments the yeast cells were cultured in MMcM in the presence of copper (10 μM of CuSO4) or in the deprivation of this metal (50 μm BCS), afterward the proteins were extracted and analyzed by ITRAQ.
FIGURE S2 | Obtaining the recombinant Atx1p. (A) PET32a:Atx1 vector. In red the atx1 gene fused to the histidine tail (trxA-His6-atx1); black Ampicillin resistance gene (AmpR). (B) Standardization of induction of recombinant Atx1p with IPTG. M, molecular weight marker; T0, Control; T30, Induction time of 30 min; T1h, Induction time of 1 h; T2h, Induction time of 2 h; T3, Induction time of 3 h. (C) Purification of recombinant Atx1p. The recombinant protein was purified by nickel column affinity chromatography and the material was analyzed on 12% polyacrylamide gel. 1, Molecular weight marker; 2, Nickel resin after binding to recombinant Atx1p; 3, First wash with buffer containing 20 mM imidazole; 4, Second wash with buffer containing 20 mM imidazole; 5, Eluted fraction of recombinant Atx1p in buffer containing 250 mM imidazole. The arrow indicates the recombinant Atx1p. (D) Analysis of the reactivity of polyclonal anti-Atx1 antibodies by Western blotting. 1, Detection of Atx1p from P. brasiliensis yeast cells protein extract using anti-Atx1 antibodies; 2, Negative control – Reactivity of Atx1p with sera of pre-immune animals. The arrow indicates native Atx1p protein.
TABLE S1 | Oligonucleotides employed in the experiments.
TABLE S2 | Paracoccidioides brasiliensis proteins with increased abundance in yeast cells after 24 h of copper depletion.
TABLE S3 | Paracoccidioides brasiliensis proteins with decreased abundance in yeast cells after 24 h of copper depletion.
Footnotes
- ^ http://www.uniprot.org/
- ^ www.ncbi.nlm.nih.gov/genome/?term=Paracoccidioides
- ^ http://www.peptideatlas.org/
- ^ https://www.blast2go.com/
- ^ https://fungidb.org/fungidb/
- ^ http://www.genome.jp/kegg/
- ^ https://blast.ncbi.nlm.nih.gov/Blast.cgi
References
Araújo, D. S., Pereira, M., Portis, I. G., De Castro Moreira Dos Santos, A., Fontes, W., De Sousa, M. V., et al. (2019). Metabolic peculiarities of Paracoccidioides brasiliensis dimorphism as demonstrated by iTRAQ labeling proteomics. Front. Microbiol. 10:555. doi: 10.3389/fmicb.2019.00555
Besold, A. N., Gilston, B. A., Radin, J. N., Ramsoomair, C., Culbertson, E. M., Li, C. X., et al. (2018). Role of calprotectin in withholding zinc and copper from Candida albicans. Infect. Immun. 86, 1–16. doi: 10.1128/IAI.00779-17
Bookout, A. L., Cummins, C. L., Kramer, M. F., Pesola, J. M., Mangelsdorf, J. D., Mangelsdorf, D. J., et al. (2006). High-Throughput Real-Time Quantitative Reverse Transcription PCR. Curr. Protoc. Mol. Biol. 73, 15.8.1–15.8.28. doi: 10.1002/0471142727.mb1508s73
Borghouts, C., Scheckhuber, C. Q., Stephan, O., and Osiewacz, H. D. (2002). Copper homeostasis and aging in the fungal model system Podospora anserina: differential expression of PaCtr3 encoding a copper transporter. Int. J. Biochem. Cell Biol. 34, 1355–1371. doi: 10.1016/S1357-2725(02)00078-X
Broxton, C. N., and Culotta, V. C. (2016). An adaptation to low copper in Candida albicans involving SOD Enzymes and the alternative oxidase. PLoS One 11:e0168400. doi: 10.1371/journal.pone.0168400
Chaves, E. G. A., Parente-Rocha, J. A., Baeza, L. C., Araújo, D. S., Borges, C. L., de Oliveira, M. A. P., et al. (2019). Proteomic analysis of Paracoccidioides brasiliensis during infection of alveolar macrophages primed or not by interferon-gamma. Front. Microbiol. 10:96. doi: 10.3389/fmicb.2019.00096
Dancis, A., Haile, D., Yuan, D. S., and Klausnert, R. D. (1994). The Saccharomyces cerevisiae copper transport protein (Ctrlp). J. Biol. Chem. 269, 25660–25667.
Dantas, S. F. I. M., Vieira de Rezende, T. C., Bailão, A. M., Taborda, C. P., da Silva Santos, R., Pacheco de Castro, K., et al. (2009). Identification and characterization of antigenic proteins potentially expressed during the infectious process of Paracoccidioides brasiliensis. Microbes Infect. 11, 895–903. doi: 10.1016/j.micinf.2009.05.009
De Curcio, J. S., Silva, M. G., Silva Bailão, M. G., Báo, S. N., Casaletti, L., Bailão, A. M., et al. (2017). Identification of membrane proteome of Paracoccidioides lutzii and its regulation by zinc. Futur. Sci. OA 3:FSO232. doi: 10.4155/fsoa-2017-0044
De Oliveira, H. C., De Fátima Da Silva, J., Matsumoto, M. T., Marcos, C. M., Peres Da Silva, R., Moraes Da Silva, R. A., et al. (2014). Alterations of protein expression in conditions of copper-deprivation for Paracoccidioides lutzii in the presence of extracellular matrix components. BMC Microbiol. 14:302. doi: 10.1186/s12866-014-0302-7
Ding, C., Festa, R. A., Chen, Y., Espart, A., Palacios, Ò, Capdevila, M., et al. (2014a). Cryptococcus neoformans copper detoxification machinery is critical for fungal virulence. Cell Host Microbe 3, 265–276. doi: 10.1016/j.chom.2013.02.002
Ding, C., Festa, R. A., Sun, T. S., and Wang, Z. Y. (2014b). Iron and copper as virulence modulators in human fungal pathogens. Mol. Microbiol. 93, 10–23. doi: 10.1111/mmi.12653
Fukuoka, M., Tokuda, E., Nakagome, K., Wu, Z., Nagano, I., and Furukawa, Y. (2017). An Essential Role of N-terminal Domain of Copper Chaperone in the Enzymatic Activation of Cu/Zn-Superoxide Dismutase. Amsterdam: Elsevier Inc.
García-Santamarina, S., and Thiele, D. J. (2015). Copper at the fungal pathogen-host axis. J. Biol. Chem. 290, 18945–18953. doi: 10.1074/jbc.R115.649129
Georgatsou, E., Mavrogiannis, L. A., Fragiadakis, G. S., and Alexandraki, D. (1997). The yeast Fre1p/Fre2p cupric reductases facilitate copper uptake and are regulated by the copper-modulated Mac1p activator. J. Biol. Chem. 272, 13786–13792. doi: 10.1074/jbc.272.21.13786
Gross, C., Kelleher, M., Iyer, V. R., Brown, P. O., and Winge, D. R. (2000). Identification of the copper regulon in Saccharomyces cerevisiae by DNA microarrays. J. Biol. Chem. 275, 32310–32316. doi: 10.1074/jbc.M005946200
Grossklaus, D. A., Bailão, A. M., Rezende, T. C. V., Borges, C. L., de Oliveira, M. A. P., Parente, J. A., et al. (2013). Response to oxidative stress in Paracoccidioides yeast cells as determined by proteomic analysis. Microbes Infect. 15, 347–364. doi: 10.1016/j.micinf.2012.12.002
Hassett, R., and Kosman, D. J. (1995). Evidence for Cu(II) reduction as a component of copper uptake by Saccharomyces cerevisiae. J. Biol. Chem. 270, 128–134. doi: 10.1074/jbc.270.1.128
Hernández, O., Araque, P., Tamayo, D., Restrepo, A., Herrera, S., Mcewen, J. G., et al. (2015). Alternative oxidase plays an important role Paracoccidioides brasiliensis cellular homeostasis and morphological transition. Med. Mycol. 15, 205–214. doi: 10.1093/mmy/myu091
Horne, J. T., Derek, W., and Hollomon, P. M. W. (2001). Fungal respiration: a fusion of standard and alternative components. Biochim. Biophys. Acta Bioenerg. 1504, 179–195. doi: 10.1016/S0005-2728(00)00251-6
Horng, Y. C., Cobine, P. A., Maxfield, A. B., Carr, H. S., and Winge, D. R. (2004). Specific copper transfer from the Cox17 metallochaperone to both Sco1 and Cox11 in the assembly of yeast cytochrome c oxidase. J. Biol. Chem. 279, 35334–35340. doi: 10.1074/jbc.M404747200
Ikeda, R., and Sawamura, K. (2008). Bacterial and H2O2 stress-induced apoptosis-like events in Cryptococcus neoformans. Res. Microbiol. 159, 628–634. doi: 10.1016/j.resmic.2008.07.006
Jungmann, J., Reins, H. A., Lee, J., Romeo, A., Hassett, R., Kosman, D., et al. (1993). MAC1, a nuclear regulatory protein related to Cu-dependent transcription factors is involved in Cu/Fe utilization and stress resistance in yeast. EMBO J. 12, 5051–5056. doi: 10.1002/j.1460-2075.1993
Khalimonchuk, O., and Rödel, G. (2005). Biogenesis of cytochrome c oxidase. Mitochondrion 5, 363–388. doi: 10.1016/j.mito.2005.08.002
Kim, I. S., Sohn, H. Y., and Jin, I. (2011). Adaptive stress response to menadione-induced oxidative stress in Saccharomyces cerevisiae KNU5377. J. Microbiol. 49, 816–823. doi: 10.1007/s12275-011-1154-6
Lacerda Pigosso, L., Baeza, L. C., Vieira Tomazett, M., Batista Rodrigues Faleiro, M., Brianezi Dignani de Moura, V. M., Melo Bailão, A., et al. (2017). Paracoccidioides brasiliensis presents metabolic reprogramming and secretes a serine proteinase during murine infection. Virulence 8, 1417–1434. doi: 10.1080/21505594.2017.1355660
Ladomersky, E., Khan, A., Shanbhag, V., Cavet, J. S., Chan, J., Weisman, G. A., et al. (2017). Host and pathogen copper-transporting P-Type ATPases function antagonistically during Salmonella Infection. Infect. Immun. 85, 1–9. doi: 10.1128/IAI.00351-17
Lin, S. J., and Culotta, V. C. (1995). The ATX1 gene of Saccharomyces cerevisiae encodes a small metal homeostasis factor that protects cells against reactive oxygen toxicity. Proc. Natl. Acad. Sci. U.S.A. 92, 3784–3788. doi: 10.1073/pnas.92.9.3784
Lin, S. J., Pufahl, R. A., Dancis, A., O’Halloran, T. V., and Culotta, V. C. (1997). A role for the Saccharomyces cerevisiae ATX1 gene in copper trafficking and iron transport. J. Biol. Chem. 272, 9215–9220. doi: 10.1074/jbc.272.14.9215
Mackie, J., Szabo, E. K., Urgast, D. S., Ballou, E. R., Childers, D. S., MacCallum, D. M., et al. (2016). Host-imposed copper poisoning impacts fungal micronutrient acquisition during systemic Candida albicans infections. PLoS One 11:e0158683. doi: 10.1371/journal.pone.0158683
Martinez, R. (2015). Epidemiology of Paracoccidioidomycosis. Rev. do Inst. Med. Trop. São Paulo 57(Suppl. 1), 11–20. doi: 10.1590/S0036-46652015000700004
McEwen, J. G., Bedoya, V., Patiño, M. M., Salazar, M. E., and Restrepo, A. (1987). Experimental murine paracoccidiodomycosis induced by the inhalation of conidia. J. Med. Vet. Mycol. 25, 165–175. doi: 10.1080/02681218780000231
Parente-Rocha, J. A., Parente, A. F. A., Baeza, L. C., Bonfim, S. M. R. C., Hernandez, O., McEwen, J. G., et al. (2015). Macrophage interaction with Paracoccidioides brasiliensis yeast cells modulates fungal metabolism and generates a response to oxidative stress. PLoS One 10:e0137619. doi: 10.1371/journal.pone.0137619
Peña, M. M. O., Koch, K. A., and Thiele, D. J. (1998). Dynamic regulation of copper uptake and detoxification genes in Saccharomyces cerevisiae. Mol. Cell. Biol. 18, 2514–2523. doi: 10.1128/mcb.18.5.2514
Peña, M. M. O., Lee, J., and Thiele, D. J. (1999). A delicate balance: homeostatic control of copper uptake and distribution. J. Nutr. 129, 1251–1260. doi: 10.1093/jn/129.7.1251
Pena, M. M. O., Puig, S., and Thiele, D. J. (2000). Characterization of the Saccharomyces cerevisiae high affinity copper transporter Ctr3. J. Biol. Chem. 275, 33244–33251. doi: 10.1074/jbc.M005392200
Queiroz, R. M. L., Charneau, S., Mandacaru, S. C., Schwämmle, V., Lima, B. D., Roepstorff, P., et al. (2014). Quantitative proteomic and phosphoproteomic analysis of Trypanosoma cruzi amastigogenesis. Mol. Cell. Proteomics 13, 3457–3472. doi: 10.1074/mcp.M114.040329
Restrepo, A. (1985). The ecology of Paracoccidioides brasiliensis: a puzzle still unsolved. Sabouraudia 23, 323–334. doi: 10.1080/00362178585380481
Restrepo, A., and Jimenez, B. (1980). Paracoccidioides brasiliensis, growth of culture, yeast phase in a chemically defined. J. Clin. Microbiol. 12, 279–281. doi: 10.1128/jcm.12.2.279-281.1980
Ruppen, I., Grau, L., Orenes-Piñero, E., Ashman, K., Gil, M., Algaba, F., et al. (2010). Differential protein expression profiling by iTRAQ-two-dimensional LC-MS/MS of human bladder cancer EJ138 cells transfected with the metastasis suppressor KiSS-1 gene. Mol. Cell. Proteomics 9, 2276–2291. doi: 10.1074/mcp.M900255-MCP200
San-Blas, G., NiÑo-Vega, G., and Iturriaga, T. (2002). Paracoccidioides brasiliensis and paracoccidioidomycosis: molecular approaches to morphogenesis, diagnosis, epidemiology, taxonomy and genetics. Med. Mycol. 40, 225–242. doi: 10.1080/mmy.40.3.225.242
Silva, M. G., Schrank, A., Bailão, E. F. L. C., Bailão, A. M., Borges, C. L., Staats, C. C., et al. (2011). The homeostasis of iron, copper, and zinc in Paracoccidioides brasiliensis, Cryptococcus neoformans var. Grubii, and Cryptococcus gattii: a comparative analysis. Front. Microbiol. 2:49. doi: 10.3389/fmicb.2011.00049
Singh, R., Mailloux, R. J., Puiseux-Dao, S., and Appanna, V. D. (2007). Oxidative stress evokes a metabolic adaptation that favors increased NADPH synthesis and decreased NADH production in Pseudomonas fluorescens. J. Bacteriol. 189, 6665–6675. doi: 10.1128/JB.00555-07
Smith, A. D., Logeman, B. L., and Thiele, D. J. (2017). Copper acquisition and utilization in fungi. Physiol. Behav. 176, 139–148. doi: 10.1016/j.physbeh.2017.03.040
Song, J., Li, R., and Jiang, J. (2019). Copper homeostasis in Aspergillus fumigatus: opportunities for therapeutic development. Front. Microbiol. 10:774. doi: 10.3389/fmicb.2019.00774
Sugui, J. A., Kim, H. S., Zarember, K. A., Chang, Y. C., Gallin, J. I., Nierman, W. C., et al. (2008). Genes differentially expressed in conidia and hyphae of Aspergillus fumigatus upon exposure to human neutrophils. PLoS One 3:e0002655. doi: 10.1371/journal.pone.0002655
Sun, T. S., Ju, X., Gao, H. L., Wang, T., Thiele, D. J., Li, J. Y., et al. (2014). Reciprocal functions of Cryptococcus neoformans copper homeostasis machinery during pulmonary infection and meningoencephalitis. Nat. Commun. 5:5550. doi: 10.1038/ncomms6550
Tamayo, D., Muñoz, J. F., Lopez, Á, Urán, M., Herrera, J., Borges, C. L., et al. (2016). Identification and analysis of the role of superoxide dismutases isoforms in the pathogenesis of Paracoccidioides spp. PLoS Negl. Trop. Dis. 10:e0004481. doi: 10.1371/journal.pntd.0004481
Urbanski, N. K., and Berêsewicz, A. (2000). Generation of. OH initiated by interaction of Fe2+ and Cu+ with dioxygen; comparison with the Fenton chemistry. Acta Biochim. Pol. 47, 951–962. doi: 10.18388/abp.2000_3950
Vishwakarma, A., Tetali, S. D., Selinski, J., Scheibe, R., and Padmasree, K. (2015). Importance of the alternative oxidase (AOX) pathway in regulating cellular redox and ROS homeostasis to optimize photosynthesis during restriction of the cytochrome oxidase pathway in Arabidopsis thaliana. Ann. Bot. 116, 555–569. doi: 10.1093/aob/mcv122
Waterman, S. R., Park, Y. D., Raja, M., Qiu, J., Hammoud, D. A., O’Halloran, T. V., et al. (2012). Role of CTR4 in the virulence of Cryptococcus neoformans. mBio 3:e00285-12. doi: 10.1128/mBio.00285-12
Weissman, Z., Berdicevsky, I., Cavari, B. Z., and Kornitzer, D. (2000). The high copper tolerance of Candida albicans is mediated by a P-type ATPase. Proc. Natl. Acad. Sci. U.S.A. 97, 3520–3525. doi: 10.1073/pnas.97.7.3520
White, C., Lee, J., Kambe, T., Fritsche, K., and Petris, M. J. (2009). A role for the ATP7A copper-transporting ATPase in macrophage bactericidal activity. J. Biol. Chem. 284, 33949–33956. doi: 10.1074/jbc.M109.070201
Wiemann, P., Perevitsky, A., Lim, F. Y., Shadkchan, Y., Knox, B. P., Landero Figueora, J. A., et al. (2017). Aspergillus fumigatus copper export machinery and reactive oxygen intermediate defense counter host copper-mediated oxidative antimicrobial offense. Cell Rep. 19, 1008–1021. doi: 10.1016/j.celrep.2017.04.019
Keywords: copper depletion, pathogenic fungus, proteomic analysis, cell wall, ITRAQ
Citation: Petito G, de Curcio JS, Pereira M, Bailão AM, Paccez JD, Tristão GB, de Morais COB, de Souza MV, de Castro Moreira Santos Junior A, Fontes W, Ricart CAO and de Almeida Soares CM (2020) Metabolic Adaptation of Paracoccidioides brasiliensis in Response to in vitro Copper Deprivation. Front. Microbiol. 11:1834. doi: 10.3389/fmicb.2020.01834
Received: 13 April 2020; Accepted: 13 July 2020;
Published: 10 August 2020.
Edited by:
Carlos Pelleschi Taborda, University of São Paulo, BrazilReviewed by:
Rosana Puccia, Federal University of São Paulo, BrazilSandro Rogerio Almeida, University of São Paulo, Brazil
Elizabeth R. Ballou, University of Birmingham, United Kingdom
Copyright © 2020 Petito, de Curcio, Pereira, Bailão, Paccez, Tristão, de Morais, de Souza, de Castro Moreira Santos, Fontes, Ricart and de Almeida Soares. This is an open-access article distributed under the terms of the Creative Commons Attribution License (CC BY). The use, distribution or reproduction in other forums is permitted, provided the original author(s) and the copyright owner(s) are credited and that the original publication in this journal is cited, in accordance with accepted academic practice. No use, distribution or reproduction is permitted which does not comply with these terms.
*Correspondence: Célia Maria de Almeida Soares, Y21hc29hcmVzQGdtYWlsLmNvbQ==
†These authors have contributed equally to this work