- 1Centro de Investigação de Montanha (CIMO), Instituto Politécnico de Bragança, Bragança, Portugal
- 2BioSystems & Integrative Sciences Institute (BioISI), Plant Functional Biology Centre, University of Minho, Braga, Portugal
Olive knot (OK) is a widespread bacterial disease, caused by Pseudomonas savastanoi pv. savastanoi (Pss), which currently has not effective control methods. The use of naturally occurring microbial antagonists, such as bacteria, as biocontrol agents could be a strategy to manage this disease. The objective of this work was to select bacteria from olive tree phyllosphere able to antagonize Pss using in vitro and in planta experiments. The elucidation of their modes of action and the potential relationship between antagonism and bacteria origin has been investigated, as well. To this end, 60 bacterial isolates obtained from the surface and inner tissues of different organs (leaves, twigs, and knots), from two olive cultivars of varying susceptibilities to OK, were screened for their in vitro antagonistic effect against Pss. A total of 27 bacterial strains were able to significantly inhibit Pss growth, being this effect linked to bacteria origin. Strains from OK-susceptible cultivar and colonizing the surface of plant tissues showed the strongest antagonistic potential. The antagonistic activity was potentially due to the production of volatile compounds, siderophores and lytic enzymes. Bacillus amyloliquefaciens P41 was the most effective antagonistic strain and their capacity to control OK disease was subsequently assayed using in planta experiments. This strain significantly reduces OK disease severity (43.7%), knots weight (55.4%) and population size of Pss (26.8%), while increasing the shoot dry weight (55.0%) and root water content (39.6%) of Pss-infected olive plantlets. Bacterial isolates characterized in this study, in particular B. amyloliquefaciens P41, may be considered as promising biocontrol candidates for controlling OK disease.
Introduction
Olive knot (OK) disease, caused by Pseudomonas savastanoi pv. savastanoi (Pss), is a serious threat to olive production worldwide, especially in Mediterranean countries (Quesada et al., 2012). This disease is characterized by the formation of overgrowths (tumorous galls or knots), mainly on the olive tree branches and twigs (Quesada et al., 2010, 2012). These galls promote the decline and death of branches, leading to serious losses in terms of yield and olive oil quality (Quesada et al., 2012). Control of OK disease is difficult, being mainly based on the removal of infected branches by pruning and application of foliar sprays with copper-based compounds (Quesada et al., 2012). With such limited available options for OK disease control, the use of biocontrol agents represents a promising environmentally friendly strategy for this disease management. Indeed, several bacteria, including Pseudomonas (Zadeh et al., 2008; Krid et al., 2010), Bacillus (Krid et al., 2010, 2012) and Rhizobium (Kacem et al., 2009), have already displayed antagonistic activity against Pss under in vitro conditions. This antimicrobial activity was attributed to the production of bacteriocins by Rhizobium (Kacem et al., 2009) and Pseudomonas (Lavermicocca et al., 2002). However, other compounds produced by these three bacterial genera might also be involved in the inhibition of Pss, as previously reported for other phytopathogens (e.g., Sasirekha and Srividya, 2016; Zengerer et al., 2018). These include siderophores (Sasirekha and Srividya, 2016), lytic enzymes, antibiotics, hydrogen cyanide (Weller, 2007; Gerami et al., 2013; Zengerer et al., 2018), lipopeptides (Touré et al., 2004), and antimicrobial volatile compounds (Hernández-León et al., 2015). Nevertheless, using in planta assays, Pseudomonas was not able to suppress OK disease development (Maldonado-González et al., 2013) and Bacillus strains revealed a variable efficiency in reducing knot weights (Krid et al., 2012; Ghanney et al., 2016). Thus, for a most successful identification of biocontrol agents, the performance of both in vitro and in planta experiments has been recommended (De Silva et al., 2019). Ideally, such a screening process should include microorganisms, which are already adapted to the crop, as well as resident microbiota in the same environment where the biocontrol approach will be used (Ozaktan et al., 2012). This is of particular importance when considering olive tree phyllosphere-associated bacterial communities, as most of their members [living either in the surface (as epiphytes) or in the interior of plant tissues (as endophytes)] are unique to their host genotype and/or plant organ (Mina et al., 2020a). Indeed, we have previously reported a own phyllospheric bacterial community in two olive genotypes with different degrees of susceptibility to OK disease (cv. Cobrançosa and cv. Verdeal Transmontana, being the former less susceptible to OK; Mina et al., 2020a). Olive tree leaves and twigs from each cultivar also displayed different bacterial communities (Mina et al., 2020a). As far as we know, no studies have examined if the antagonistic effects of a specific bacterial strain is related to the origin of the isolate, in terms of host cultivar and/or plant organ.
In the present study, the antagonistic activity of epiphytic and endophytic bacteria, isolated from leaves, twigs and knots of two olive cultivars displaying variable susceptibilities to OK (cvs. Cobrançosa and Verdeal Transmontana), was evaluated against Pss through in vitro assays. Their antagonistic mode of action was investigated by the production of lytic enzymes, siderophores, and antibacterial volatile compounds. The ability of the most antagonistic isolates to control OK disease was further evaluated by performing in planta assays (olive pot experiments). This study aims to answer the following questions: (i) Is the antagonistic effect displayed by bacteria against Pss linked to their origin in terms of host (i.e., genotype susceptibility to OK), plant organ (i.e., leaf, twig, knot) and/or microbial habitat (epiphyte vs. endophyte)? (ii) Which mechanisms are involved in the antagonistic effect displayed by native bacteria against Pss? (iii) What is the potential of native bacteria in controlling OK disease development and in reducing Pss population on olive phyllosphere? By combining the mechanisms of antagonistic bacterial agents with host plant features (susceptibility, type of tissue, microbial habitat), we expect to increase the likelihood of finding more effective biocontrol agents.
Materials and Methods
Bacterial Isolates and Inocula Production
The epiphytic and endophytic bacterial isolates tested for their antagonistic effect against Pss were obtained from the microbial collection of the Mountain Research Center (CIMO), Instituto Politécnico de Bragança (Portugal). These isolates were originally isolated and identified from symptomless olive tree leaves and twigs, as well as from knots, of cvs. Cobrançosa and Verdeal Transmontana growing in Mirandela (Northeast of Portugal), as referred in Mina et al. (2020a,b). Briefly, for the isolation of epiphytes, bacterial suspensions made from pieces of plant tissues in peptone water were poured over nutrient agar plates. Endophytes were isolated from the same plant pieces, by inoculating surface sterilized plant fragments previously dissected into small segments (ca. 4–5 mm) on nutrient agar plates (Mina et al., 2020a,b). A total of 60 isolates [stored in 30% (v/v) glycerol at −80°C] were selected for this study, including five isolates from each population (2 plant cultivars × 3 plant organs × 2 microbial habitats; Supplementary Table S1). These isolates were selected based on their exclusive occurrence in a specific microbial habitat (epiphyte or endophyte), organ (leaf, twig or knot), cultivar (Cobrançosa or Verdeal Transmontana), and capacity to grow on artificial media. Pss strain EnVN39 was obtained from the same bacterial collection, being isolated from the inner tissues of active knots of naturally infected olive trees cv. Verdeal Transmontana (Mirandela, Portugal). This Pss isolate had been previously identified by sequencing a portion of the ptz gene by using specific primers (Pss1, 5′-TGGGTTGCTACTTGTACCGGA-3′ and Pss2, 5′-CCGTGTACTACGTTCAGCGAG-3; Basim and Ersoy, 2001). Bacterial inocula to be used for in vitro and in planta assays were prepared by transferring bacterial cells onto Luria Bertani agar (LBA) medium (10 g/L peptone, 5 g/L yeast extract, 5 g/L sodium chloride, 10 g/L agar). Bacteria (2-days-old cultures) were suspended on 5 mL liquid LB medium and shaken on a rotary shaker (100 rpm) for 24 or 48 h at room temperature. Bacterial cell densities were adjusted to an optical density at 600 nm (OD600 = 0.5), corresponding to a concentration of 108 CFU/mL (as determined by plotting standard curves using Bacillus and Pseudomonas growth as standards).
In vitro Antagonistic Activity
The antagonistic activity against Pss of bacterial isolates was assessed by dual culture assays. Two sterile filter paper discs (5 mm diameter) were placed 3 cm apart onto the surface of LBA medium (10 mL/9 cm-Petri dishes). Each disc was then soaked with 5 μL of Pss or antagonist and left to dry in laminar flow cabinet, before being incubated at 25 ± 2°C in the dark. Control plates were performed with single inoculated discs (for each Pss and antagonist). For each bacterial isolate-Pss combination, five replicates were done and the whole experiment was repeated twice. For each testing bacteria, daily measurements of the internal radius (i.e., the radial growth toward the interacting bacterial colony) were performed. Measurements were performed until no further growth was observed, at least for one of the interacting species. The growth rate (mm/day) of each bacterial isolate was estimated from the slope of the linear regression of the increase of radial growth of the colony (mm), along the cultivation time (in days). The percentage of growth rate reduction for both pathogen and antagonist was estimate in comparison with control plates, by using the following equation: [(GC-GDC)/GC]∗100, where GC is the growth rate of the Pss/antagonist colony in control plates and GDC is the growth rate of the Pss/antagonist colony in the dual-culture assay.
Mechanisms Associated With the Antagonistic Activity
Bacterial strains promoting more than 50% of Pss growth inhibition, while not being significantly inhibited by Pss, were considered as displaying relevant antagonistic activity (Supplementary Table S2). These isolates were further screened for the production of different compounds related to phytopathogen biocontrol, including antibacterial volatile compounds, siderophores, and lytic enzymes (lipases and proteases).
Volatiles Production
A volatile assay was designed and performed for evaluating the potential effect of volatiles produced by antagonists on Pss growth. This assay was performed as previously described for in vitro antagonistic activity (see section “In vitro Antagonistic Activity”), but using significant modifications. Before inoculation, a long strip of agar (1 cm wide) was removed from the mid portion of LBA medium (10 mL/9 cm-Petri dishes). In one side of this plate, one sterile filter paper disc (5 mm diameter) soaked with 5 μL of Pss was placed in the center, whereas in the opposite side a bacterial antagonist suspension (5 μL) was spread over the agar using a sterile cotton swab. The use of LB medium instead of antagonist suspension was used as control. The plates were sealed and following incubation at 25 ± 2°C in the dark, during the same period as used in dual-culture assay, the Pss colony area was measured and compared with control. The percentage of growth rate reduction was determined using the formula presented in the above section. This assay was performed considering five replicates for each antagonistic-Pss combination and repeated twice.
Siderophores and Lytic Enzymes Production
Siderophores production was evaluated using Chrome azurol S (CAS) medium, prepared according to Pérez-Miranda et al. (2007). CAS medium (10 mL) was plated in 9 cm Petri dishes, followed by the application of a LBA medium overlay (10 mL). Dual culture assays were established as described above, but using CAS plates. After an incubation period at 25 ± 2°C in the dark, during the same period as used in dual-culture assay, the orange zone formed around the bacterial colonies (an indication of siderophore production) was measured.
The production of proteases and lipases was assessed through dual-culture assays, but using LBA medium supplemented with corresponding enzyme substrates, according to Maria et al. (2005). Briefly, for protease activity, dual cultures were established on LBA medium supplemented with 0.4% (w/v) gelatin (Prolabo), at pH 6.0. After an incubation at 25 ± 2°C in the dark, during the same period as used in dual-culture assay, the plates were flooded with saturated aqueous ammonium sulfate (Prolabo). As the undigested gelatin precipitates with ammonium sulfate, the appearance of a clear area around the colony is an indication of protease activity. For lipase activity, dual cultures were established on LBA medium supplemented with 1% (v/v) Tween 20 (Aldrich). A clear zone around the colony indicates the production of lipase.
For both siderophores and lytic enzymes assays were performed five replicates for each antagonistic-Pss combination and the whole experiment was repeated twice. Controls were performed as previously described by using single inoculated discs in each plate. The level of siderophore production and enzyme activity was evaluated by using the formula D-d, where D is the area of colony plus orange (for siderophores) or clearing (for enzymes) zone, and d is the area of colony (both in mm2). For each antagonist, the percentage of increase on siderophore/lytic enzyme production in the presence of the pathogen was determined by using the equation [(ADC-AC)/AC]∗100, where AC is the siderophore/lytic enzyme production in control plates and ADC is the same in dual-culture assay.
In planta Biocontrol Assays
The bacterial strain that exhibited the greatest inhibitory effect on Pss growth in dual-culture assays (isolate P41 – Bacillus amyloliquefaciens) was selected for in planta assays (pot experiments). This study aims to assess the biocontrol ability of this isolate in planta against OK disease development, without compromising plant growth. As direct activation of plant defense is commonly associated with a reduced plant growth (Stenberg, 2017), the impact of isolate P41 on plant growth would be studied as well.
Production of Olive Plantlets
Pot experiments were performed with 2-year-old olive plantlets (cv. Cobrançosa), obtained from propagation of olive tree semi-wood cuttings. To improve rooting, the base of cuttings was treated with indole-3-butyric acid (IBA, 3 000 ppm) and transferred to basal heated benches filled with sand and perlite mixture (1:1). Cuttings were automatically sprayed for 10 s, every 40 min, and kept under greenhouse conditions (day/night thermal regime of 23°/18° ± 2°C, 10 h light/14 h dark photoperiod and 70 ± 10% relative humidity) for 3 months. Rooted cuttings were then selected and transplanted to plastic pots of two liters, containing the same growth mixture as before, and maintained for 2 years under the same greenhouse conditions. During this plant growth period, plants were irrigated every 2 days.
Plant Inoculation
Both Pss and antagonistic isolate (P41) inocula were prepared as previously described in section “Bacterial Isolates and Inocula Production,” but using LB media containing 1% (w/v) of agar. Inoculation of olive plantlets with Pss and/or antagonistic P41 isolate was performed according to Penyalver et al. (2006) with minor changes. A V-shaped wound (1 cm long, 5 mm wide, and about 2 mm deep) was made on the main stem with a sterile scalpel. This wound was used for performing the plant inoculations with 10 μL of Pss and/or selected antagonist (P41). Controls were inoculated with 10 μL sterile LB culture medium containing 1% (w/v) of agar. Following inoculation, each wound was wrapped with Parafilm, which was removed 1 week later. For each treatment (Pss, P41, and Pss + P41) and control, a total of 30 olive plantlets were inoculated, in a total of 120 plants. All plants were maintained at 26°C, 8 h light/16 h dark photoperiod and 70% of relative humidity, and watered when needed.
Evaluated Parameters
Disease rating was visually recorded after 14, 28, 42, 56, and 70 days post-inoculation (DPI) by using the severity scale of Matas et al. (2012), where 1 = no knots; 2 = mild wound thickening; 3 = small knot at the wound base; 4 = small knots at both the base and top of the wound; 5 = knot completely covering the wound; 6 = knot larger than the wound (Supplementary Figure S1). OK disease development was monitored in the same 10 arbitrarily selected trees of each treatment throughout the assay. The obtained data were used to calculate the area under the disease progress curve (AUDPC) for each treatment using the following formula (Madden et al., 2007):
where Yi represents disease severity (1 to 6 scale) on the ith date, ti is the time in days at the ith observation, and n is the total number of observations used to record OK disease development. For each time point (14, 28, 42, and 56 DPI), five plants were randomly selected and brought to the laboratory, for estimation of knot weight per plant, assessment of Pss population sizes, and evaluation of plant growth. At 70 DPI, the monitored plants used for disease severity were also used for these evaluations. The collected plants were firstly separated into leaves, stems and roots. The average knot fresh weight per plant was estimated by weighting the stem fragments comprising the 1 cm long wound used for the inoculation. The obtained fragments were further used to estimate Pss population densities. For this, the stem fragment was cut in small pieces, which were immersed in 5 mL of peptone water (10 g/L peptone, 5 g/L sodium chloride) and shaken for 10 min at 100 rpm at room temperature. Suspension aliquots (1 mL) were plated in triplicate in 10 mL of PVF-1 medium (10 mL/L glycerol, 30 g/L sucrose, 2.5 g/L Difco casamino acids, 1.96 g/L K2HPO4.3H2O, 0.4 g/L MgSO4.7H2O, 0.4 g/L SDS, 16 g/L agar, pH adjusted to 7.1 with HCl) and incubated at 25 ± 2°C, in the dark, until bacterial growth. Daily observations were performed in order to count the fluorescent bacteria Pss colonies. Results are presented as CFU per mL. Plant development was evaluated by registering total shoot height and root length. Shoot height was registered on the beginning of the assay and in each recording date (14, 28, 42, 56, and 70 DPI). Harvested plants were used to evaluate roots length, as well as stems and roots fresh (fw) and dry (dw) weights. For this, stems and roots were separately used to determine fresh weight (fw), oven-dried at 60°C for 3 days, and then weighed again to determine dry weight (dw). Water content was determined by dry weight/fresh weigh ratio and expressed as a percentage. Leaves near the inoculation site were used for measuring the photosynthetic pigment contents. Chlorophyll a (chl a), chlorophyll b (chl b), and carotenoids (car) contents were determined spectrophotometrically after methanolic extraction of fresh leaves, according to Ozerol and Titus (1965). Total chlorophyll was calculated by the sum of both chlorophyll a and b content. Results are presented as mg of pigment per g of leaf.
Statistical Analysis
Statistical analyses were carried out using R software (R Core Team, 2018). To evaluate how microbial habitat (epiphyte/endophyte), plant organ (leaves/stems/knots), and plant cultivar (Cobrançosa/Verdeal Transmontana) are related with antagonistic potential against Pss, a multiple factor analysis (MFA) was performed by using the FactoMineR package (Le et al., 2008) from R software. Data from in vitro and in planta assays were analyzed by multifactorial analysis of variance (ANOVA) and means were compared using Tukey post hoc test at p-value < 0.05 by using agricolae package (functions aov and TukeyHSD, respectively) from R software.
Results
In this work, the bacterial isolates previously obtained from the phyllosphere (epiphytes and endophytes) of two olive cultivars (Cobrançosa and Verdeal Transmontana, more tolerant and susceptible to OK disease, respectively) and plant organs (leaves, twigs and knots) (Mina et al., 2020a,b) were further studied (Supplementary Table S1). The 60 isolates belonged to 22 different bacterial genera and to 40 species.
Bacteria Colonizing Different Cultivars and Microbial Habitats Displayed Different Inhibitory Effects Against Pss
Almost 50% (27) of the tested isolates (15 epiphytes and 12 endophytes) were able to significantly inhibit the Pss growth, with inhibitions ranging from 15.8 to 85.2% (Figure 1 and Supplementary Table S2). From these, most belonged to Pseudomonas (8), Bacillus (4), and Alcaligenes (4) genera, representing together 59.3% of the antagonistic isolates. Interestingly, within isolates of the same species a variable antagonistic activity was observed. For example, different B. amyloliquefaciens and Alcaligenes faecalis isolates displayed contrasting antagonistic potential (Figure 1). The number of bacterial strains with capacity to significantly inhibit Pss growth was similar regardless of the plant organ from which they were isolated (9 isolates/organ). By contrast, differences were found among cultivars, displaying cv. Verdeal Transmontana a higher number of antagonistic bacteria than cv. Cobrançosa (15 vs. 12 isolates, respectively).
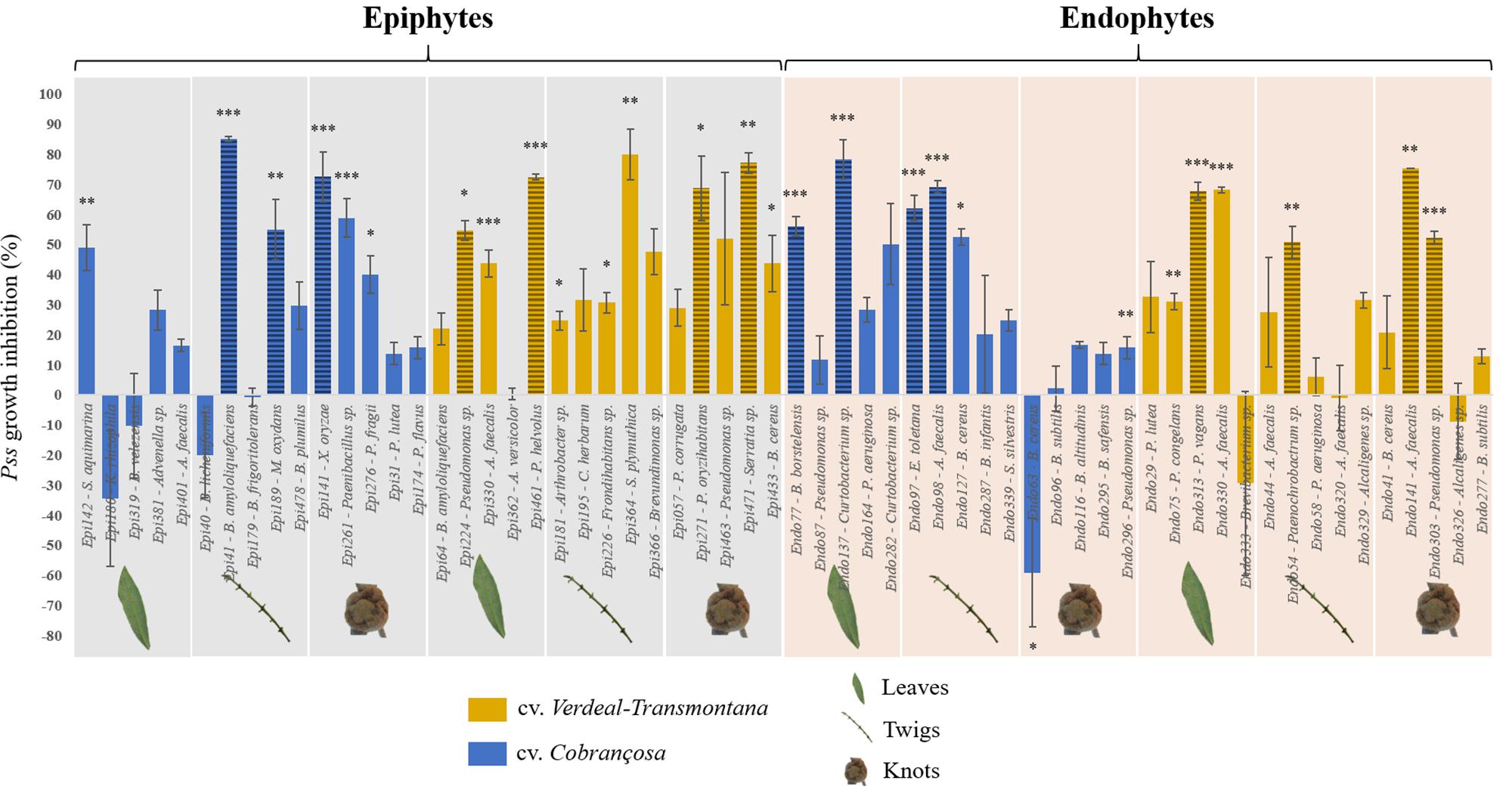
Figure 1. Growth inhibition of Pseudomonas savastanoi pv. savastanoi (Pss) after interacting with different epiphytic and endophytic bacterial isolates, obtained from leaves, twigs and knots of olive tree cvs. Cobrançosa and Verdeal Transmontana. Results are presented as the percentage of inhibition in dual-culture assays considering single inoculated controls. Horizontal lines indicate the bacterial species further used for evaluating the inhibition mechanisms. Significant differences to control (Pss single culture) are indicated by asterisks (*p < 0.05; **p < 0.01; ***p < 0.001).
Using a multifactorial correspondence analysis, the inhibition of Pss growth by the antagonists were significantly high correlated with dimension 1 (R2 = 0.77, p < 0.001), while the qualitative variables (cultivar, microbial habitat and organ) were associated to dimension 2 (Figure 2). The potential to inhibit Pss growth was more associated to those isolates obtained from different plant cultivars (R2 = 0.59, p < 0.001) and microbial habitats (R2 = 0.42, p < 0.001), than from distinct plant organs (R2 = 0.26, p = 0.007). Indeed, the bacterial isolates from cv. Verdeal Transmontana revealed more potential to inhibit Pss growth (estimated coefficient = 0.64, p < 0.001) than those isolated from cv. Cobrançosa. Also, epiphytes presented higher antagonistic potential (estimated coefficient = 0.46, p < 0.001) than endophytes. Considering plant organs, twigs were related to isolates with higher antagonistic ability (estimated coefficient = 0.55, p = 0.002) than leaves or knots.
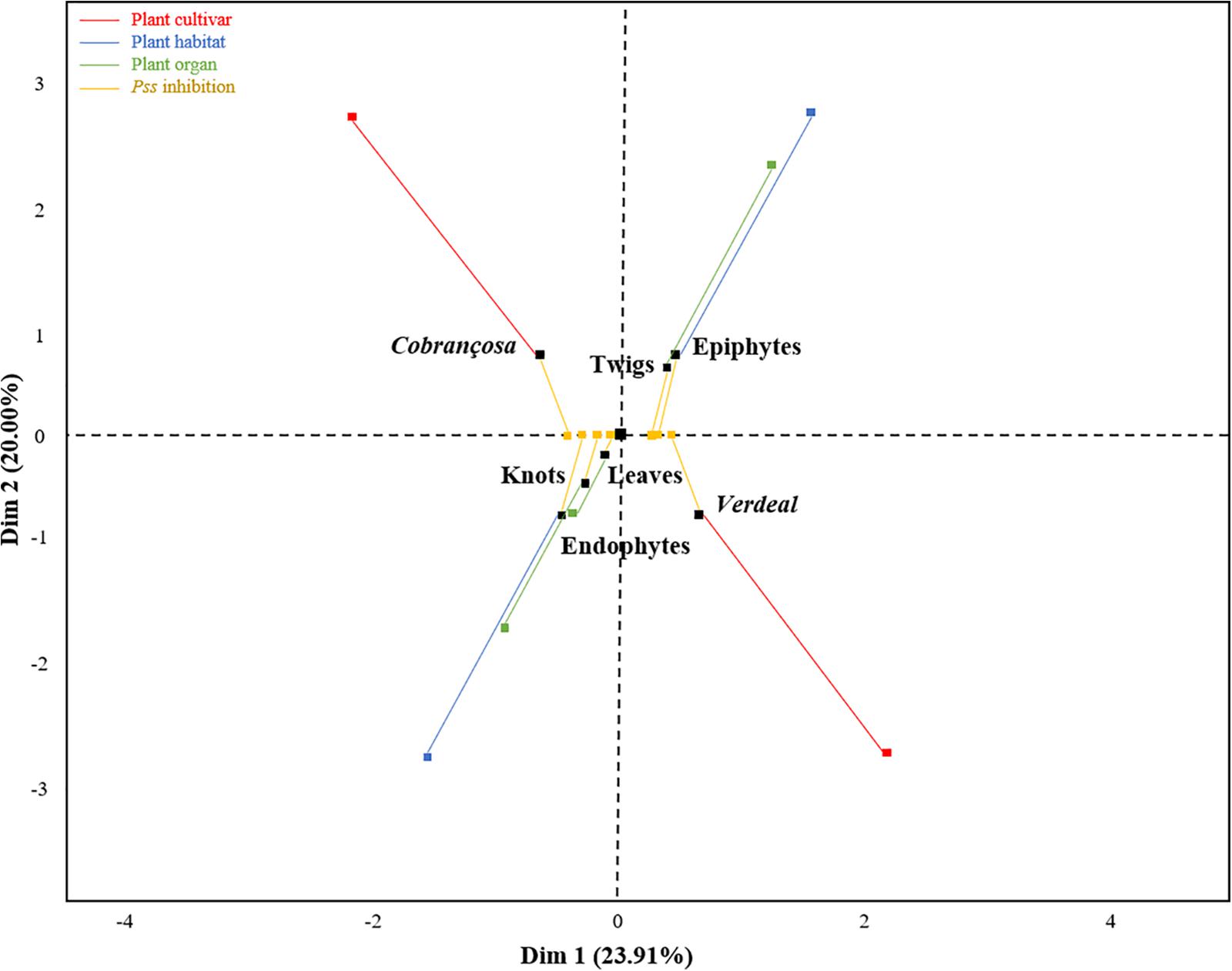
Figure 2. Association of Pss growth inhibition ability of tested bacterial isolates with plant cultivars (Cobrançosa and Verdeal Transmontana), organs (leaves, twigs, and knots) and microbial habitats (epiphytes and endophytes), as revealed by a multiple factor analysis (MFA). The first two dimensions represent 43.91% of the total variance. Dimension 1 is associated to the inhibition ability of antagonists (contribution of 77.3%, p < 0.001), while dimension 2 is associated to microbial habitat (contribution of 42.7%, p < 0.001), cultivar (41.4%, p < 0.001), and organ (15.9%, p < 0.001). An individual (black square) is at the barycenter of corresponding partial points (colored squares), thus representing an individual as seen by a single group. Therefore, each black square represents an individual viewed by two groups of variables: Pss inhibition (yellow squares) and their presence regarding cultivar (red squares)/microbial habitat (blue squares)/plant organ (green squares).
Inhibition Mechanisms Used to Restrain Pss Growth
To clarify the inhibition mechanism behind each antagonistic activity, the production of antibacterial volatile compounds, siderophores and lytic enzymes (lipases and proteases) were studied when bacterial isolates were growing in co-culture with Pss. This study was performed only for those bacterial strains that inhibited more than 50% of Pss growth and were not significantly inhibited by Pss (Supplementary Table S2). In total, 15 antagonistic isolates (7 epiphytes and 8 endophytes), belonging to 12 genera (Pseudomonas, Pseudoclavibacter, Serratia, Bacillus, Microbacterium, Xanthomonas, Pantoea, Paenochrobactrum, Alcaligenes, Brevibacillus, Curtobacterium, and Erwinia) were selected based on these criteria.
From all tested isolates, eight were able to significantly affect the growth of Pss through volatiles emission, being P41, P461, and D144 those displaying the highest inhibition rates (36.5 ± 5.8, 34.2 ± 7.0, and 32.3 ± 10.41%, respectively) (Figure 3A). The production of siderophores by bacterial antagonists was variable according to the co-culture, being significantly increased [D144 (54.4 ± 8.0%), D97 (35.1 ± 8.3%) and P41 (26.4 ± 5.4%)] or decreased [P461 (−55.8 ± 0.81%)] when compared to single inoculated controls (Figure 3B and Supplementary Table S3). When compared to control, only three epiphytic isolates significantly increased the production of lipase when challenged by Pss pathogen, P271 (47.5 ± 2.1%), P141 (47.1 ± 6.1%), and P41 (26.6 ± 4.8%) (Figure 3C and Supplementary Table S3). A significant increase in protease production when in co-culture with Pss was only observed for two endophytic isolates, D54 (87.9 ± 27.2%) and D144 (33.3 ± 4.0%).
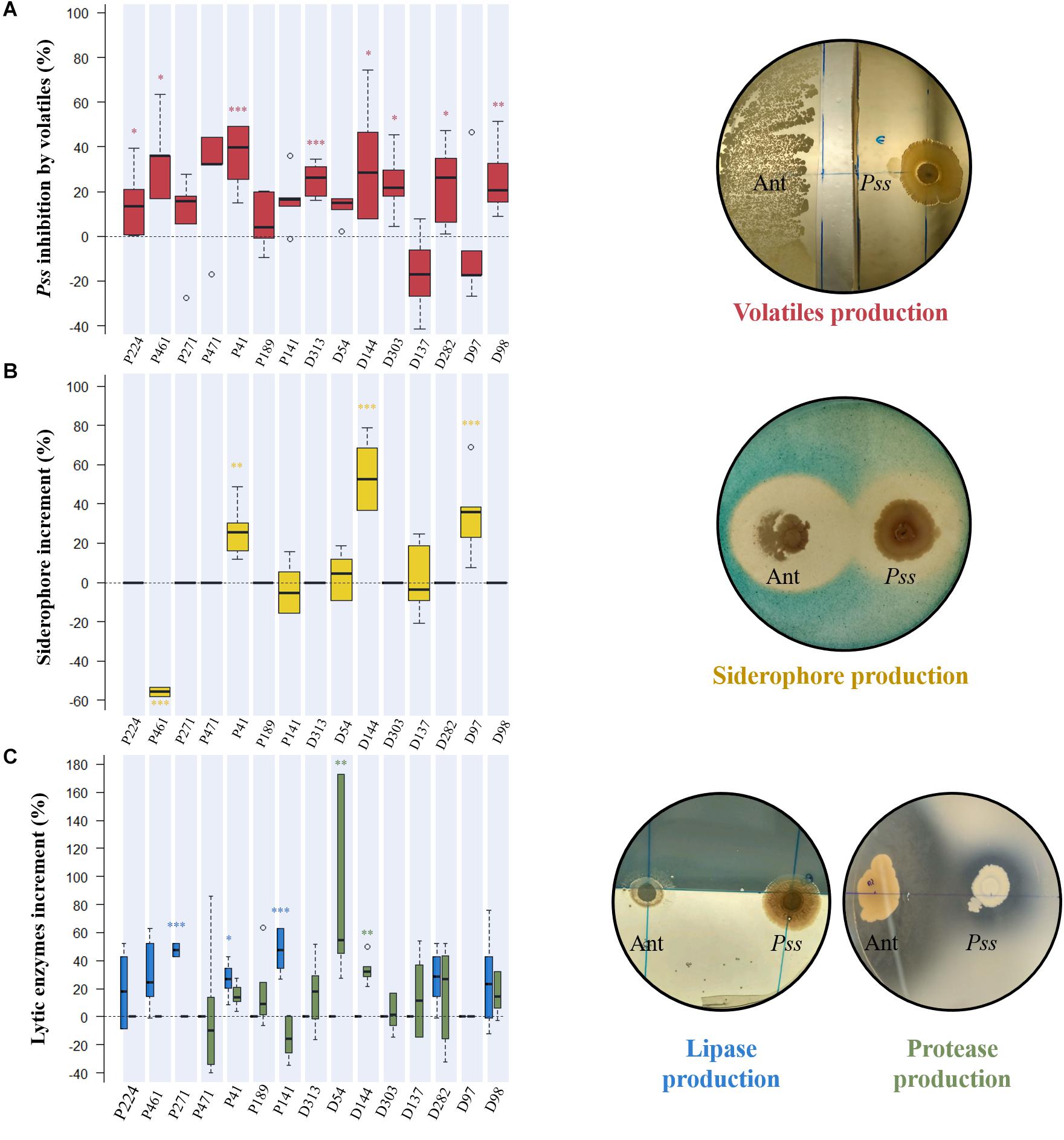
Figure 3. Evaluation of the inhibition mechanism displayed by each antagonistic isolate against Pseudomonas savastanoi pv. savastanoi (Pss). The production of antibacterial volatile compounds by antagonists was assessed by estimating their inhibitory effect on Pss growth (%) in dual-culture assays (A), while the production of siderophores (B) and lytic enzymes (lipase-blue, and protease-green) (C) were evaluated by their increase in dual-culture assays when compared to single inoculated controls. Significant differences from controls are indicated by asterisks (*p < 0.05; **p < 0.01; ***p < 0.001). Representative plates of different dual-cultures assays with antagonist (Ant) and pathogen (Pss) are presented.
P41 Significantly Affected OK Disease Development, While Increasing Plant Growth
An initial screening revealed that B. amyloliquefaciens (P41 isolate) exhibited the greatest inhibitory effect against Pss and was the greatest producer of inhibitory compounds. These features make this strain a good candidate to be explored as a biological control agent against Pss. Therefore, this isolate was selected for proceeding with in planta assays and determine its capacity to suppress OK disease development. This effect was evaluated by the progression of OK disease severity curve (AUDPC), knots weight and pathogen abundance on the inoculated area of olive plants treated with Pss (Pss alone or Pss + P41, Figure 4). Non-inoculated plants and inoculated with P41 were used as controls. AUDPC disease severity curve was significantly (p < 0.01) higher in olive plantlets solely inoculated with Pss, when compared to plantlets inoculated with Pss + P41 and controls (Figure 4A). This result was observed after only 14 DPI, being further enhanced up to the end of the assay (an overall increase of 1.8-fold, p < 0.01). After 56 DPI, those plantlets inoculated exclusively with Pss also revealed a higher knot weight (up to 2.2-fold, p < 0.05) when compared to plants inoculated with Pss + P41 (Figure 4B). In addition, the Pss abundance on the inoculated area become higher in Pss inoculated plantlets in comparison with Pss + P41 plantlets at 14 DPI (up to 1.3-fold, p < 0.05) (Figure 4C). These results were observed until the end of the assay. Despite the capacity of P41 in reducing OK disease development, some control plants (exclusively treated with P41) also developed symptoms similar to OK (Figure 4A). However, the disease severity curve did not significantly differ from non-inoculated plantlets (negative control).
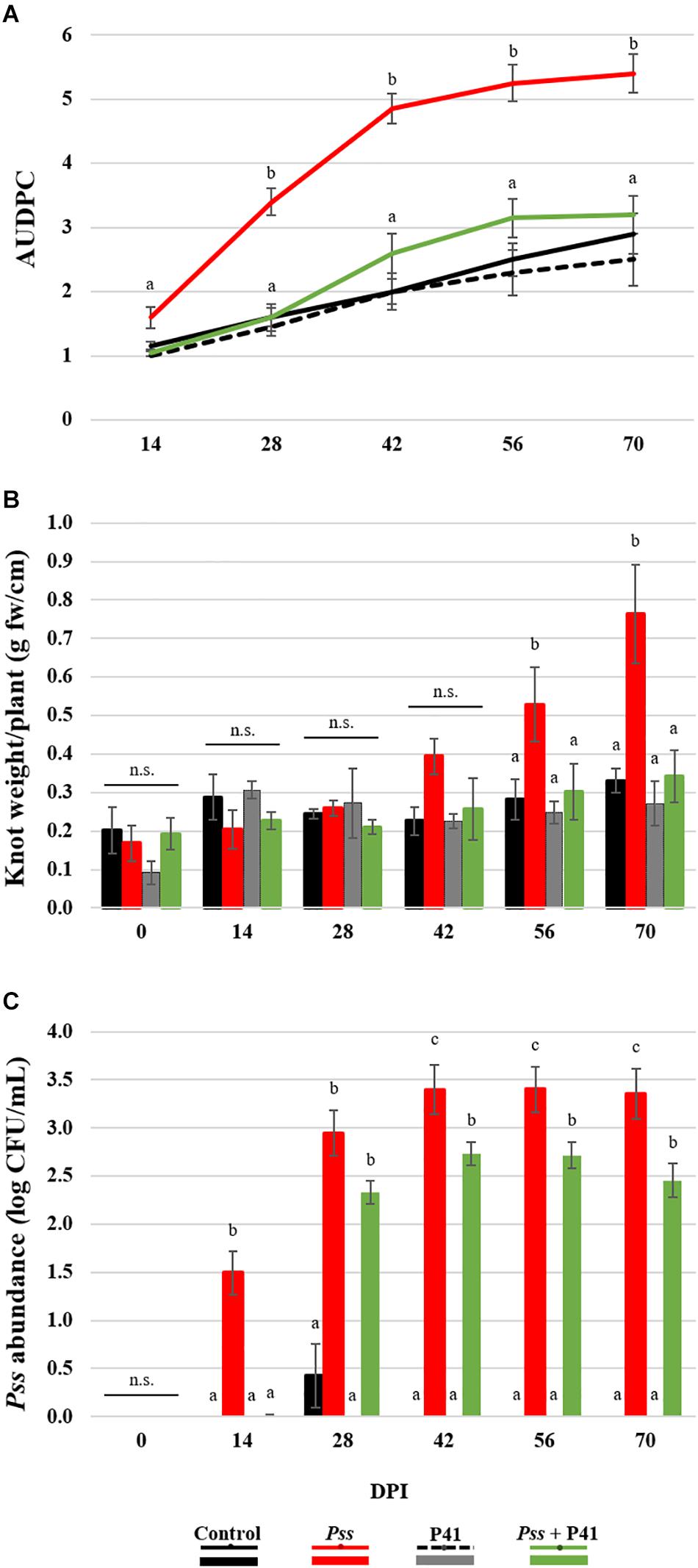
Figure 4. Effect of Bacillus amyloliquefaciens (P41 isolate) on olive knot disease progression determined by in planta assays. The ability to suppress disease development was evaluated by OK disease severity curves (AUDPC) (A), knot fresh weight per plant (B) and Pseudomonas savastanoi pv. savastanoi (Pss) population density on the inoculated area of olive plants (C), determined on olive plantlets inoculated with the pathogen (Pss), with both antagonist and pathogen (Pss + P41), with the antagonist (P41) and with LB medium (negative control). Data is presented as means ± SE (n = 10 for severity; n = 5 for the knots weight and Pss density). Statistically significant (p < 0.05) differences between the four treatments, in each day post-inoculation (DPI), are indicated by different letters (n.s.-non-significant).
The biocontrol effect of microbial agents against phytopathogens has been suggested to potentially compromise plant growth (Huot et al., 2014). Therefore, several plant growth parameters, including shoot and root length, fresh and dry weight, as well as water content and leaf pigments content, were evaluated during pot assays (Figure 5 and Supplementary Table S4). There were no significant differences on the growth parameters evaluated between plantlets inoculated with P41 and with Pss + P41. By the end of the assay, both displayed higher shoot dry weight (up to 2.8-fold and 2.2-fold, respectively, p < 0.01) and root water content (up to 1.3-fold and 1.7-fold, respectively, p < 0.01), when compared to plants inoculated solely with Pss. Inoculation of plants with any of the tested bacterial strains caused a reduction in shoot height when compared to non-inoculated plants, reaching statistically significant differences after 70 days post-inoculation. No significant differences were observed on pigment contents between treatments (Supplementary Table S4).
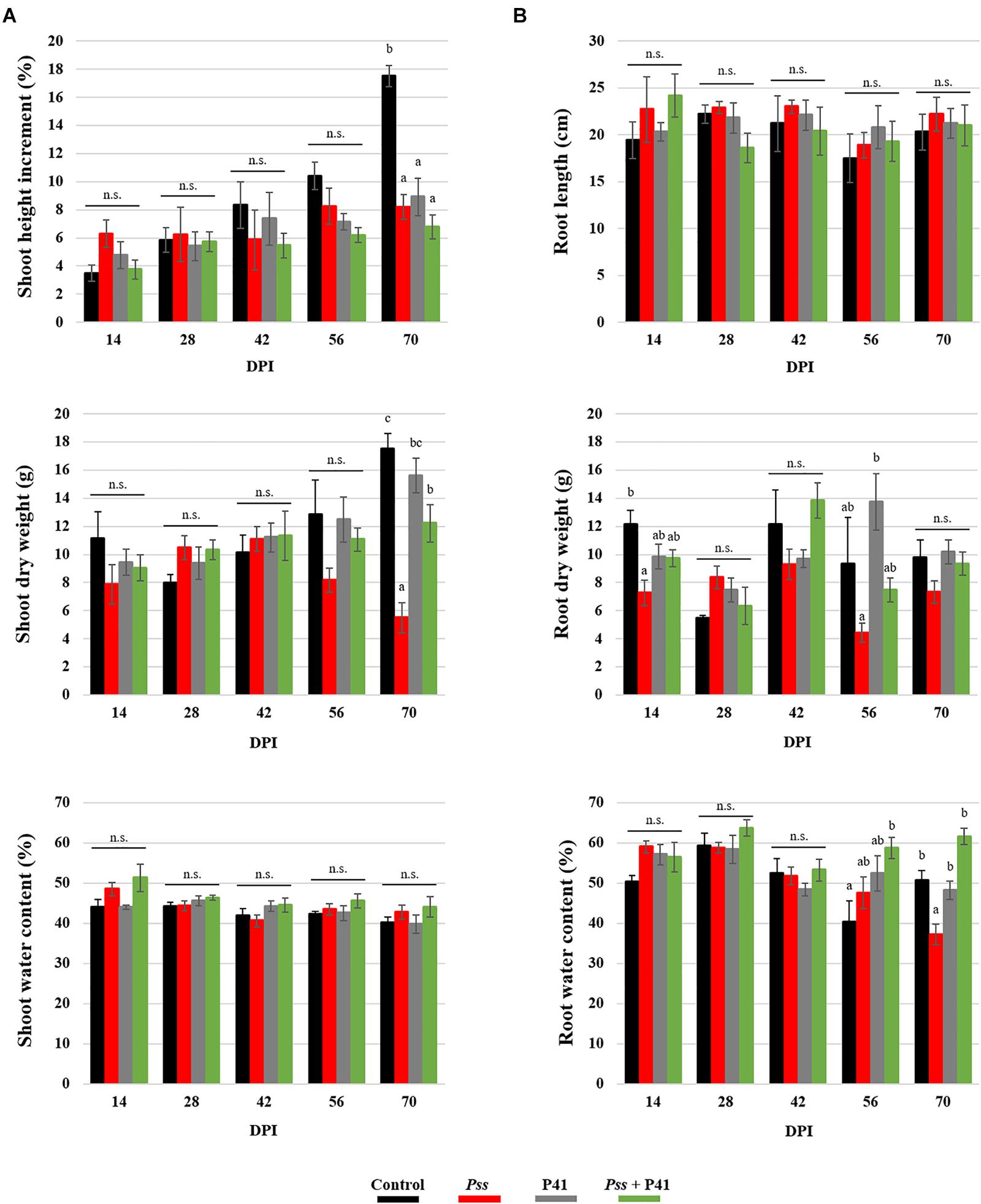
Figure 5. Effect of Bacillus amyloliquefaciens (P41 isolate) on olive plant growth determined by in planta assays. Plant growth parameters were evaluated at the level of shoots (A) and roots (B) of olive plantlets inoculated with the pathogen (Pss), with both antagonist and pathogen (Pss + P41), with the antagonist (P41) and with LB medium (negative control). Data is presented as means ± SE (n = 5). Statistically significant (p < 0.05) differences between the four treatments, in each day post-inoculation (DPI), are indicated by different letters (n.s.-non-significant).
Discussion
In this study, we tested the biocontrol potential of several bacteria isolated from the olive tree phyllosphere against Pss, by combining in vitro and in planta assays. Amongst the 60 isolates tested, it was observed, for the first time, differences in their effectiveness in inhibiting in vitro growth of Pss depending on their origin. Bacterial strains isolated from OK-susceptible cultivar cv. Verdeal Transmontana displayed higher antagonistic effect against Pss than the ones isolated from OK-tolerant cv. Cobrançosa. This apparent contradiction led us to hypothesize that isolates from both olive cultivars may use different modes of action to protect host plant from Pss infection. Microbial antagonists may use a battery of mechanisms against phytopathogens, broadly classified into direct or indirect, depending respectively on the requirement of interspecies physical contact or not (Hajek and Eilenberg, 2018). Isolates from cv. Cobrançosa could stimulate/prime the immunity of host plant to indirectly combat Pss invasions, while isolates from cv. Verdeal Transmontana seem to act directly against the pathogen. However, this assumption still needs to be confirmed with further work. We also found that epiphytes exhibit a higher inhibition potential against Pss than endophytes, which makes epiphytes as the first layer of plant defense, comprising the most promising agents for OK disease biocontrol. This aspect is of particular importance for this pathosystem, since the infection of olive tree is believed to be caused by the epiphytic Pss (Quesada et al., 2010). From the 27 isolates that significantly inhibited Pss, the antagonistic effect was highest within Pseudomonas, Bacillus, and Alcaligenes. Both Pseudomonas and Bacillus have been reported to be the most promising biocontrol agents of several plant diseases, in particular the ones affecting roots (Chowdhury et al., 2015; De Vrieze et al., 2018; Fan et al., 2018). Accordingly, the use of these two genera as biocontrol agents in the phyllosphere has been less studied than in the rhizosphere. However, a number of studies have already reported the effectiveness of Pseudomonas and Bacillus strains, isolated from the phyllosphere of different woody crops, namely lemon (Michavila et al., 2017) and apple (Mikiciński et al., 2016) trees, to control bacterial pathogens that infect their aboveground organs. Interestingly, isolates belonging to Pseudomonas (Zadeh et al., 2008; Krid et al., 2010) and Bacillus (Krid et al., 2010, 2012) were previously reported to be effective in antagonizing Pss in in vitro assays, as observed in our work. For the first time, A. faecalis isolates revealed the capacity to strongly inhibit Pss in vitro growth. This species has previously displayed ability to inhibit the growth of other phytopathogens under in vitro conditions (Lahlali et al., 2020), as well as capacity to induce plant defenses against fusarium wilt via lipopolysaccharide production (Patel et al., 2020). These features make this species as a potential candidate for OK biocontrol as well.
Most of the in vitro inhibition of Pss exhibited by tested antagonistic bacteria should have been caused by the induction of volatile organic compounds (VOCs) upon bacterial interaction. Indeed, eight bacterial strains out of the tested 15 strains able to antagonize Pss, increased their production of VOCs in co-culture. The antibacterial activity of produced VOCs by microbial antagonists has been previously reported and proposed as an effective biological control strategy against several phytopathogens (Ossowicki et al., 2017; Bui et al., 2019). These compounds may act as promoters of plant defense responses (Erb, 2018) and/or as inhibitors of phytopathogens growth (Enespa and Chandra, 2017). Furthermore, due to their highly diffusible capacity, microbial VOCs are considered ideal for biocontrol approaches because they do not require the contact between antagonist and pathogen to perform their activity (Contarino et al., 2019). Previous studies have shown VOCs potential from Pseudomonas (Agisha et al., 2019), Bacillus (Gao et al., 2018), and Alcaligenes (Gong et al., 2019) to inhibit the growth of different pathogens, such as fungal, oomycete, and nematode, as well as disease suppression caused by them. Besides VOCs, both siderophores and lytic enzymes could be also involved in the inhibition mechanism of Pss growth. An induction in the production of iron binding ligands (siderophores) was detected in three bacterial antagonists in the presence of Pss. Siderophores are known to sequester iron from the culture medium, making the iron unavailable to interacting bacteria (in this case Pss) and restricting their growth. Iron is essential for growth and pathogenesis of almost all species of phytopathogenic bacteria (Shanmugaiah et al., 2015; Pi and Helmann, 2017). Suppression of phytopathogens by bacterial antagonists through siderophore-mediated competition for iron has been already reported either in in vitro (Akter et al., 2016) or in field (Sasirekha and Srividya, 2016) conditions. There are some previous studies reporting the capacity to suppress several pathogens through siderophores production by Pseudomonas, Bacillus (review by Carmona-Hernandez et al., 2019) and Alcaligenes (Sayyed and Chincholkar, 2009) genera. From the tested bacterial antagonists, five revealed an induction on the lytic enzymatic activity (lipases or proteases) in the presence of Pss. Previous studies have similarly reported strong enhancement of these enzymes in bacterial antagonists during in vitro interaction with several plant pathogens (Amaresan et al., 2012; Geetha et al., 2014). We hypothesize that bacterial antagonists may inhibit Pss growth by secreting lipase and protease enzymes that will degrade pathogen cellular components. Indeed, it was within the bacterial isolates that displayed the greatest antagonistic activity toward Pss that the increment on the production of these enzymes was observed. This was particularly noticed for A. faecalis (D144; protease), Pseudomonas oryzihabitans (P271, lipase), and B. amyloliquefaciens (P41; lipase). Strains of these genera were previously reported to produced proteases and lipases that inhibit pathogen growth (Durairaja et al., 2018; Lahlali et al., 2020). The effective role of such enzymes as well as of siderophores in inhibiting Pss growth and germination should be confirmed. Accordingly, further research should test the effect of purified lipase, protease and siderophores from the antagonistic bacterial isolates on Pss growth and germination.
Among the different bacterial strains tested, the P41 isolate (previously identified as B. amyloliquefaciens) revealed the most promising antagonistic traits against Pss under in vitro conditions. This isolate obtained from twigs of cv. Cobrançosa, displayed the highest of all antimicrobial activities against Pss. Previous studies have similarly identified this species as one of the most efficient in inhibiting either bacterial (Abdallah et al., 2018) or fungal (Freitas et al., 2019) plant pathogens using in vitro conditions. In the present study, the mechanisms involved in Pss inhibition by B. amyloliquefaciens P41 probably comprise the secretion of inhibitory compounds/enzymes (VOCs and lipases) and competition for nutrients (through the production of siderophores). These mechanisms were also observed by other authors for this species (Kejela et al., 2016; Jamali et al., 2018). In addition, the genome analysis of B. amyloliquefaciens revealed the capacity of this species to produce other secondary metabolites aimed to suppress plant pathogens or enhance/mediate the defense responses of host plants against plant pathogens (Chowdhury et al., 2013). In agreement with in vitro assays, the inoculation of olive plantlets with B. amyloliquefaciens P41 together with Pss reduced significantly the OK disease severity, knots weight and Pss population size, when compared to pathogen (Pss) inoculated plants. Likewise, other studies have demonstrated the beneficial effects of B. amyloliquefaciens on disease suppression on other crops, such as tomato (Gautam et al., 2019), apple (Zhang et al., 2015), pistachio (Siahmoshteh et al., 2017), and lettuce (Chowdhury et al., 2013). Besides displaying biocontrol traits, several strains of B. amyloliquefaciens have been previously described as plant growth promoters (Asari et al., 2016; Abdallah et al., 2018). Contrasting with these reports, in the present study, B. amyloliquefaciens P41 did not promote plant growth when compared to non-inoculated control for most evaluated plant growth parameters. However, when B. amyloliquefaciens P41 was inoculated, either alone or in combination with Pss, a significant increase of both shoot dry weight and root water content were observed when compared to plants inoculated solely with Pss. This result suggests once more the increased capacity given to the host plant to deal with OK disease in the presence of B. amyloliquefaciens P41.
As far as we know, this study illustrates for the first time the potential role of B. amyloliquefaciens as a biological agent for controlling OK disease in olive trees, which has been an underexploited matter. Only Bacillus subtilis F1 (isolated from olive leaves) and Bacillus mojavensis A-BC-7 (isolated from the olive phylloplane) have demonstrated some efficiency, using in planta assays, in reducing the Pss population and knots size (Krid et al., 2012; Ghanney et al., 2016). Furthermore, the use of B. amyloliquefaciens as a biocontrol agent is of great significance because some strains of this species are already commercially available (e.g., Serenade, Bayer Crop Science; RhizoVital42, Abitep GmbH) for use as biocontrol agents against other plant pathogens (Chowdhury et al., 2015). However, we should emphasize that different strains could produce slightly distinct secondary products/enzymes with antibacterial activity, which would result in variable biocontrol efficiency. The comparison of B. amyloliquefaciens P41 and commercial available strains, regarding the biocontrol efficacy of produced antimicrobial compounds against OK disease would be an interesting approach for selecting the most appropriate BCA for controlling this olive disease.
Conclusion
This work discloses the olive phyllosphere as harboring a number of bacterial strains with great potential to be used as biocontrol agents against Pss. In particular, B. amyloliquefaciens P41, revealed a great potential for the management of OK disease, by simultaneously promoting plant growth and reducing the disease severity of Pss-infected olive plantlets. Although promising results were obtained by using this strain in greenhouse assays, further experiments are needed to determine biocontrol effectiveness under field conditions and using different cultivars. The biocontrol mechanisms displayed by this strain also need to be further investigated.
Data Availability Statement
The raw data supporting the conclusions of this article will be made available by the authors, without undue reservation.
Author Contributions
DM performed the experiments, analyzed the data, and contributed to the manuscript writing. JP and TL-N contributed to the study design and manuscript editing. PB contributed to the study design, data analysis, and manuscript writing. All authors contributed to the article and approved the submitted version.
Funding
This work was funded by FEDER funds through COMPETE (Programa Operacional Factores de Competitividade), national funds through FCT (Fundação para a Ciência e a Tecnologia) and by Horizon 2020, the European Union’s Framework Programme for Research and Innovation, within the project PRIMA/0002/2018 (INTOMED – Innovative tools to combat crop pests in the Mediterranean), and the Mountain Research Center – CIMO (UIDB/00690/2020 and UIDB/04046/2020).
Conflict of Interest
The authors declare that the research was conducted in the absence of any commercial or financial relationships that could be construed as a potential conflict of interest.
Acknowledgments
DM thanks FCT for SFRH-BD-105341/2014 grant.
Supplementary Material
The Supplementary Material for this article can be found online at: https://www.frontiersin.org/articles/10.3389/fmicb.2020.02051/full#supplementary-material
References
Abdallah, D. B., Frikha-Gargouri, O., and Tounsi, S. (2018). Rizhospheric competence, plant growth promotion and biocontrol efficacy of Bacillus amyloliquefaciens subsp. plantarum strain 32a. Biol. Control. 124, 61–67. doi: 10.1016/j.biocontrol.2018.01.013
Agisha, V. N., Kumar, A., Eapen, S. J., Sheoran, N., and Suseelabhai, R. (2019). Broad-spectrum antimicrobial activity of volatile organic compounds from endophytic Pseudomonas putida BP25 against diverse plant pathogens. Biocontrol Sci. Technol. 29, 1069–1089. doi: 10.1080/09583157.2019.1657067
Akter, S., Kadir, J., Juraimi, A. S., and Saud, H. M. (2016). In vitro evaluation of Pseudomonas bacterial isolates from rice phylloplane for biocontrol of Rhizoctonia solani and plant growth promoting traits. J. Environ. Biol. 37, 597–602.
Amaresan, N., Jayakumar, V., Kumar, K., and Thajuddin, N. (2012). Endophytic bacteria from tomato and chilli, their diversity and antagonistic potential against Ralstonia solanacearum. Arch. Phytopathol. Plant Protect. 45, 344–355. doi: 10.1080/03235408.2011.587273
Asari, S., Matzén, S., Petersen, M. A., Bejai, S., and Meijer, J. (2016). Multiple effects of Bacillus amyloliquefaciens volatile compounds: plant growth promotion and growth inhibition of phytopathogens. FEMS Microbiol. Ecol. 92:fiw070. doi: 10.1093/femsec/fiw070
Basim, H., and Ersoy, A. (2001). Identification of Pseudomonas savastanoi pv. savastanoi, olive knot pathogen, by polymerase chain reaction. Phytopathol. 91:6
Bui, H. X., Hadi, B. A. R., Oliva, R., and Schroeder, N. E. (2019). Beneficial bacterial volatile compounds for the control of root-knot nematode and bacterial leaf blight on rice. Crop Prot. 135:104792. doi: 10.1016/j.cropro.2019.04.016
Carmona-Hernandez, S., Reyes-Pérez, J. J., Chiquito-Contreras, R. G., Rincon-Enriquez, G., Cerdan-Cabrera, C. R., and Hernandez-Montiel, L. G. (2019). Biocontrol of postharvest fruit fungal diseases by bacterial antagonists: a review. Agronomy 9:121. doi: 10.3390/agronomy9030121
Chowdhury, S. P., Dietel, K., Rändler, M., Schmid, M., Junge, H., Borriss, R., et al. (2013). Effects of Bacillus amyloliquefaciens FZB42 on lettuce growth and health under pathogen pressure and its impact on the rhizosphere bacterial community. PLoS One 8:e68818. doi: 10.1371/journal.pone.0068818
Chowdhury, S. P., Hartmann, A., Gao, X., and Borriss, R. (2015). Biocontrol mechanism by root-associated Bacillus amyloliquefaciens FZB42 – a review. Front. Microbiol. 6:780.
Contarino, R., Brighina, S., Fallico, B., Cirvilleri, G., Parafati, L., and Restuccia, C. (2019). Volatile organic compounds (VOCs) produced by biocontrol yeasts. Food Microbiol. 82, 70–74.
De Silva, N. I., Brooks, S., Lumyong, S., and Hyde, K. D. (2019). Use of endophytes as biocontrol agents. Fungal Biol. Rev. 33, 133–148. doi: 10.1016/j.fbr.2018.10.001
De Vrieze, M., Germanier, F., Vuille, N., and Weisskopf, L. (2018). Combining different potato-associated Pseudomonas strains for improved biocontrol of Phytophthora infestans. Front. Microbiol. 9:2573.
Durairaja, K., Velmurugan, P., Park, J-H., Chang, W-S., Park, Y-J., Senthilkumar, P., et al. (2018). Characterization and assessment of two biocontrol bacteria against Pseudomonas syringae wilt in Solanum lycopersicum and its genetic responses Microbiol. Res. 206, 43–49. doi: 10.1016/j.micres.2017.09.003
Enespa, S., and Chandra, P. (2017). “Microbial volatiles as chemical weapons against pathogenic fungi”, in Volatiles and Food Security: Role of Volatiles in Agro-ecosystems, eds D.K. Choudhary, A.K. Sharma, P. Agarwal, A. Varma, and N. Tuteja (Singapore: Springer), 227–254.
Erb, M. (2018). Volatiles as inducers and suppressors of plant defense and immunity - origins, specificity, perception and signaling. Curr. Opin. Plant Biol. 44, 117–121. doi: 10.1016/j.pbi.2018.03.008
Fan, B., Wang, C., Song, X., Ding, X., Wu, L., Wu, H., et al. (2018). Bacillus velezensis FZB42 in 2018: the gram-positive model strain for plant growth promotion and biocontrol. Front. Microbiol. 9, 2491.
Freitas, M. A., Medeiros, F. H. V., Melo, I. S., Pereira, P. F., Peñaflor, M. F. G. V., Bento, J. M. S., et al. (2019). Stem inoculation with bacterial strains Bacillus amyloliquefaciens (GB03) and Microbacterium imperiale (MAIIF2a) mitigates Fusarium root rot in cassava. Phytoparasitica 47, 135–142. doi: 10.1007/s12600-018-0706-2
Gao, H., Li, P., Xu, X., Zeng, Q., and Guan, W. (2018). Research on volatile organic compounds from Bacillus subtilis CF-3: biocontrol effects on fruit fungal pathogens and dynamic changes during fermentation. Front. Microbiol. 9:456.
Gautam, S., Chauhan, A., Sharma, R., Sehgal, R., and Shirkot, C. K. (2019). Potential of Bacillus amyloliquefaciens for biocontrol of bacterial canker of tomato incited by Clavibacter michiganensis ssp. michiganensis. Microb. Pathog. 130, 196–203. doi: 10.1016/j.micpath.2019.03.006
Geetha, K., Venkatesham, Hindumathi A., and Bhadraiah, B. (2014). Isolation, screening and characterization of plant growth promoting bacteria and their effect on Vigna radita (L.) R. Wilczek. Int. J. Curr. Microbiol. App. Sci. 3, 799–809.
Gerami, E., Hassanzadeh, N., Abdollahi, H., Ghasemi, A., and Heydari, A. (2013). Evaluation of some bacterial antagonists for biological control of fire blight disease. J. Plant Pathol. 95, 127–134.
Ghanney, N., Locantore, P., Nahdi, S., Ferchichi, A., and Iacobellis, N. S. (2016). Potential biocontrol effect of the phylloplane bacterium Bacillus mojavensis ABC-7 on the olive knot disease. J. Plant Pathol. Microbiol. 7:3.
Gong, A-D., Wu, N-N., Kong, X-W., Zhang, Y-M., Hu, M-J., Gong, S. -J., et al. (2019). Inhibitory effect of volatiles emitted from Alcaligenes faecalis N1-4 on Aspergillus flavus and aflatoxins in storage. Front. Microbiol. 10:1419.
Hajek, A. E., and Eilenberg, J. (2018). “Biology and ecology of microorganisms for control of plant diseases”, in Natural Enemies: An Introduction to Biological Control, ed. A. E. Hajek (Cambridge: Cambridge University Press), 291–307.
Hernández-León, R., Rojas-Solís, D., Contreras-Pérez, M., Orozco-Mosqueda, M. C., Macías-Rodríguez, L. I., de la Cruz, H. R., et al. (2015). Characterization of the antifungal and plant growth-promoting effects of diffusible and volatile organic compounds produced by Pseudomonas fluorescens strains. Biol. Control. 81, 83–92. doi: 10.1016/j.biocontrol.2014.11.011
Huot, B., Yao, J., Montgomery, B. L., and He, S. Y. (2014). Growth–defense tradeoffs in plants: a balancing act to optimize fitness. Mol. Plant. 7, 1267–1287. doi: 10.1093/mp/ssu049
Jamali, H., Sharma, A., Kushwaha, P., Roohi, P. L. K., and Srivastava, A. K. (2018). Exploitation of multifarious abiotic stresses, antagonistic activity and plant growth promoting attributes of Bacillus amyloliquefaciens AH53 for sustainable agriculture production. Int. J. Curr. Microbiol. App. Sci. 7, 751–763. doi: 10.20546/ijcmas.2018.710.083
Kacem, M., Kazouz, F., Merabet, C., Rezki, M., De Lajudie, P., and Bekki, A. (2009). Antimicrobial activity of Rhizobium sp. strains against Pseudomonas savastanoi, the agent responsible for the olive knot disease in Algeria. Grasas y Aceites 60, 139–146. doi: 10.3989/gya.074808
Kejela, T., Thakkar, V. R., and Thakor, P. (2016). Bacillus species (BT42) isolated from Coffea arabica L. rhizosphere antagonizes Colletotrichum gloeosporioides and Fusarium oxysporum and also exhibits multiple plant growth promoting activity. BMC Microbiol. 16:277.
Krid, S., Rhouma, A., Mogou, I., Quesada, J. M., Nesme, X., and Gargouri, A. (2010). Pseudomonas savastanoi endophytic bacteria in olive tree knots and antagonistic potential of strains of Pseudomonas fluorescens and Bacillus subtilis. J. Plant Pathol. 92, 335–341.
Krid, S., Triki, M. A., Gargouri, A., and Rhouma, A. (2012). Biocontrol of olive knot disease by Bacillus subtilis isolated from olive leaves. Ann. Microbiol. 62, 149–154. doi: 10.1007/s13213-011-0239-0
Lahlali, R., Aksissou, W., Lyousfi, N., Ezrari, S., Blenzar, A., Tahiri, A., et al. (2020). Biocontrol activity and putative mechanism of Bacillus amyloliquefaciens (SF14 and SP10), Alcaligenes faecalis ACBC1, and Pantoea agglomerans ACBP1 against brown rot disease of fruit. Microb. Pathog. 139:103914. doi: 10.1016/j.micpath.2019.103914
Lavermicocca, P., Lonigro, S. L., Valerio, F., Evidente, A., and Visconti, A. (2002). Reduction of olive knot disease by a bacteriocin from Pseudomonas syringae pv. ciccaronei. Appl. Environ. Microbiol. 68, 1403–1407. doi: 10.1128/aem.68.3.1403-1407.2002
Le, S., Josse, J., and Husson, F. (2008). FactoMineR: an R package for multivariate analysis. J. Stat. Softw. 25, 1–18.
Madden, L. V., Hughes, G., and van den Bosch, F. (2007). The study of plant disease epidemics. St. Paul: The American Phytopathological Society (APS).
Maldonado-González, M. M., Prieto, P., Ramos, C., and Mercado-Blanco, J. (2013). From the root to the stem: interaction between the biocontrol root endophyte Pseudomonas fluorescens PICF7 and the pathogen Pseudomonas savastanoi NCPPB 3335 in olive knots. Microb. Biotechnol. 6, 275–287. doi: 10.1111/1751-7915.12036
Maria, G. L., Sridhar, K. R., and Raviraja, N. S. (2005). Antimicrobial and enzyme activity of mangrove endophytic fungi of southwest coast of India. J. Agric. Tech. 1, 67–80.
Matas, I. M., Lambertsen, L., Rodriguez-Moreno, L., and Ramos, C. (2012). Identification of novel virulence genes and metabolic pathways required for full fitness of Pseudomonas savastanoi pv. savastanoi in olive (Olea europaea) knots. New Phytol. 196, 1182–1196. doi: 10.1111/j.1469-8137.2012.04357.x
Michavila, G., Adler, C., De Gregorio, P. R., Lami, M. J., Caram Di Santo, M. C., Zenoff, A. M., et al. (2017). Pseudomonas protegens CS1 from the lemon phyllosphere as a candidate for citrus canker biocontrol agent. Plant Biol. J. 19, 608–617. doi: 10.1111/plb.12556
Mikiciński, A., Sobiczewski, P., Puławska, J., and Maciorowski, R. (2016). Control of fire blight (Erwinia amylovora) by a novel strain 49M of Pseudomonas graminis from the phyllosphere of apple (Malus spp.). Eur. J. Plant. Pathol. 145, 265–276. doi: 10.1007/s10658-015-0837-y
Mina, D., Pereira, J. A., Lino-Neto, T., and Baptista, P. (2020a). Epiphytic and endophytic bacteria on olive tree phyllosphere: exploring tissue and cultivar effect. Microb. Ecol. 80, 145–157.
Mina, D., Pereira, J. A., Lino-Neto, T., and Baptista, P. (2020b). Impact of plant genotype and plant habitat in shaping bacterial pathobiome: a comparative study in olive tree. Sci. Rep. 10:3475.
Ossowicki, A., Jafra, S., and Garbeva, P. (2017). The antimicrobial volatile power of the rhizospheric isolate Pseudomonas donghuensis P482. PLoS One 12:e0174362. doi: 10.1371/journal.pone.0174362
Ozaktan, H., Erdal, M., Akkopru, A., and Aslan, E. (2012). Biological control of bacterial blight of walnut by antagonistic bacteria. J. Plant Pathol. 94, 53–56.
Ozerol, N. H., and Titus, J. F. (1965). The determination of total chlorophyll in methanol extracts. Trans. III.. State Acad. Sci. 58, 15–19.
Patel, S., Thaker, V., Kuvad, R., and Saraf, M. (2020). Role of lipopolysaccaride extracted from Alcaligenes faecalis as elicitor for the induction of plant defense against fusarium wilt. J. Plant Pathol. 102, 351–357. doi: 10.1007/s42161-019-00425-0
Penyalver, R., García, A., Ferrer, A., Bertolini, E., Quesada, J. M., Salcedo, C. I., et al. (2006). Factors affecting Pseudomonas savastanoi pv. savastanoi plant inoculations and their use for evaluation of olive cultivar susceptibility. Phytopathology 96, 313–319. doi: 10.1094/phyto-96-0313
Pérez-Miranda, S., Cabirol, N., George-Téllez, R., Zamudio-Riviera, L. S., and Fernández, F. J. (2007). O-CAS, a fast and universal method for siderophore detection. J. Microbiol. Meth. 70, 127–131. doi: 10.1016/j.mimet.2007.03.023
Pi, H., and Helmann, J. D. (2017). Ferrous iron efflux systems in bacteria. Metallomics 9, 840–851. doi: 10.1039/c7mt00112f
Quesada, J. M., Penyalver, R., and López, M. M. (2012). “Epidemiology and control of plant diseases caused by phytopathogenic bacteria: the case of olive knot disease caused,” by Pseudomonas savastanoi pv. savastanoi, in Plant Pathology, ed. C. J. Cumagun (InTechOpen), 299–326.
Quesada, J. M., Penyalver, R., Pérez-Panades, J., Salcedo, C. I., Carbonell, E. A., and Lopez, M. M. (2010). Dissemination of Pseudomonas savastanoi pv. savastanoi populations and subsequent appearance of olive knot disease. Plant Pathol. 59, 262–269.
R Core Team (2018). R: A language and environment for statistical computing. Vienna: R Foundation for Statistical Computing.
Sasirekha, B., and Srividya, S. (2016). Siderophore production by Pseudomonas aeruginosa FP6, a biocontrol strain for Rhizoctonia solani and Colletotrichum gloeosporioides causing diseases in chilli. Agric. Nat. Resour. 50, 250–256. doi: 10.1016/j.anres.2016.02.003
Sayyed, R. Z., and Chincholkar, S. B. (2009). Siderophore-producing Alcaligenes feacalis exhibited more biocontrol potential vis-à-vis chemical fungicide. Curr. Microbiol. 58, 47–51. doi: 10.1007/s00284-008-9264-z
Shanmugaiah, V., Nithya, K., Harikrishnan, H., Jayaprakashvel, M., and Balasubramanian, N. (2015). “Biocontrol mechanisms of siderophores against bacterial plant pathogens,” in Sustainable Approaches to Controlling Plant Pathogenic Bacteria, eds V. R. Kannan and K. K. Bastas (Boca Raton, FL: CRC Press Book), 167–190 doi: 10.1201/b18892-9
Siahmoshteh, F., Siciliano, I., Banani, H., Hamidi-Esfahani, Z., Razzaghi-Abyaneh, M., Gullino, M. L., et al. (2017). Efficacy of Bacillus subtilis and Bacillus amyloliquefaciens in the control of Aspergillus parasiticus growth and aflatoxins production on pistachio. Int. J. Food Microbiol. 254, 47–53. doi: 10.1016/j.ijfoodmicro.2017.05.011
Stenberg, J. A. (2017). A conceptual framework for integrated pest management. Trends Plant Sci. 22, 759–769. doi: 10.1016/j.tplants.2017.06.010
Touré, Y., Ongena, M., Jacques, P., Guiro, A., and Thonart, P. (2004). Role of lipopeptides produced by Bacillus subtilis GA1 in the reduction of grey mould disease caused by Botrytis cinerea on apple. J. Appl. Microbiol. 96, 1151–1160. doi: 10.1111/j.1365-2672.2004.02252.x
Weller, D. M. (2007). Pseudomonas biocontrol agents of soilborne pathogens: looking back over 30 years. Phytopathol. 97, 250–256. doi: 10.1094/phyto-97-2-0250
Zadeh, R., Khavazi, K., Asgharzadeh, A. M., and De Mot, H. R. (2008). Biocontrol of Pseudomonas savastanoi pv. savastanoi, causative agent of olive knot disease: antagonistic potential of nonpathogenic rhizosphere isolates of fluorescent Pseudomonas. Commun. Agric. Appl. Biol. Sci. 73, 199–203.
Zengerer, V., Schmid, M., Bieri, M., Müller, D. C., Remus-Emsermann, M. N. P., Ahrens, C. H., et al. (2018). Pseudomonas orientalis F9: a potent antagonist against phytopathogens with phytotoxic effect in the apple flower. Front. Microbiol. 9:145.
Keywords: Olea europaea, olive knot, biocontrol, Bacillus amyloliquefaciens, antagonistic mechanisms
Citation: Mina D, Pereira JA, Lino-Neto T and Baptista P (2020) Screening the Olive Tree Phyllosphere: Search and Find Potential Antagonists Against Pseudomonas savastanoi pv. savastanoi. Front. Microbiol. 11:2051. doi: 10.3389/fmicb.2020.02051
Received: 09 June 2020; Accepted: 05 August 2020;
Published: 25 August 2020.
Edited by:
Kevin Garcia, North Carolina State University, United StatesReviewed by:
Oliver Baars, North Carolina State University, United StatesDaniel Passos Da Silva, BioVectra, Canada
Copyright © 2020 Mina, Pereira, Lino-Neto and Baptista. This is an open-access article distributed under the terms of the Creative Commons Attribution License (CC BY). The use, distribution or reproduction in other forums is permitted, provided the original author(s) and the copyright owner(s) are credited and that the original publication in this journal is cited, in accordance with accepted academic practice. No use, distribution or reproduction is permitted which does not comply with these terms.
*Correspondence: Paula Baptista, cGJhcHRpc3RhQGlwYi5wdA==